- 1School of Agriculture and Biology, Shanghai Jiao Tong University, Shanghai, China
- 2Shanghai Veterinary Research Institute, Chinese Academy of Agricultural Sciences, Shanghai, China
- 3College of Animal Science and Technology, Anhui Agricultural University, Hefei, China
- 4Department of Stomatology, Ditmanson Medical Foundation Chia-Yi Christian Hospital, Chiayi, Taiwan
- 5Department of Cell Biology, School of Life Sciences, Central South University, Changsha, China
- 6West China Hospital, School of Nursing, Sichuan University, Chengdu, China
- 7Department of Pathology, Ditmanson Medical Foundation Chia-Yi Christian Hospital, Chiayi, Taiwan
- 8Department of Cosmetic Science, Chia Nan University of Pharmacy and Science, Tainan, Taiwan
- 9Department of Biotechnology and Bioindustry Sciences, College of Bioscience and Biotechnology, National Cheng Kung University, Tainan, Taiwan
- 10Doctoral Program in Translational Medicine, Rong Hsing Research Center for Translational Medicine, National Chung Hsing University, Taichung, Taiwan
Protozoa exert a serious global threat of growing concern to human, and animal, and there is a need for the advancement of novel therapeutic strategies to effectively treat or mitigate the impact of associated diseases. Omega polyunsaturated fatty acids (ω-PUFAs), including Omega-3 (ω-3) and omega-6 (ω-6), are constituents derived from various natural sources, have gained significant attention for their therapeutic role in parasitic infections and a variety of essential structural and regulatory functions in animals and humans. Both ω-3 and ω-6 decrease the growth and survival rate of parasites through metabolized anti-inflammatory mediators, such as lipoxins, resolvins, and protectins, and have both in vivo and in vitro protective effects against various protozoan infections. The ω-PUFAs have been shown to modulate the host immune response by a commonly known mechanism such as (inhibition of arachidonic acid (AA) metabolic process, production of anti-inflammatory mediators, modification of intracellular lipids, and activation of the nuclear receptor), and promotion of a shift towards a more effective immune defense against parasitic invaders by regulation the inflammation like prostaglandins, leukotrienes, thromboxane, are involved in controlling the inflammatory reaction. The immune modulation may involve reducing inflammation, enhancing phagocytosis, and suppressing parasitic virulence factors. The unique properties of ω-PUFAs could prevent protozoan infections, representing an important area of study. This review explores the clinical impact of ω-PUFAs against some protozoan infections, elucidating possible mechanisms of action and supportive therapy for preventing various parasitic infections in humans and animals, such as toxoplasmosis, malaria, coccidiosis, and chagas disease. ω-PUFAs show promise as a therapeutic approach for parasitic infections due to their direct anti-parasitic effects and their ability to modulate the host immune response. Additionally, we discuss current treatment options and suggest perspectives for future studies. This could potentially provide an alternative or supplementary treatment option for these complex global health problems.
Introduction
Omega-3 and omega-6 polyunsaturated fatty acids are synthesized from the essential fatty acids alpha-linolenic acid and linoleic acid, respectively. They are fundamental components of living cells and have been found to significantly contribute to the prevention and treatment of a variety of health problems (1).
PUFAs can be divided into two primary groups: ω-3 PUFAs and ω-6 PUFAs, with their main distinction lying in the positioning of double bonds along the carbon chain. ω-6 PUFAs have their initial double bonds starting at the sixth carbon atom, whereas ω-3 PUFAs begin at the third carbon atom, measured from the methyl end of the carbon chain, which is referred to as the ω-carbon (2). Linoleic acid (LA) (18:2 ω-6) and arachidonic acid (AA) (20:4 ω-6) are the two most common ω-6 PUFAs in diets. Western diets are high in ω-6 PUFAs and low in ω-3 PUFAs, resulting in a high ω-6/ω-3 ratio of up to 20-30 (3). The three primary ω-3 PUFAs are α-linolenic acid (18:3 ω-3), eicosapentaenoic acid (20:5 ω-3), and docosahexaenoic acid (DHA, 22:6 ω-3). It is important to distinguish between α-LA (an ω-3 precursor) and γ-linolenic acid (GLA), which is 18:3 but belongs to the ω-6 fatty acid series. LA (precursor to ω-6 fatty acids) and α-LA (precursor to ω-3 fatty acids) are essential fatty acids, as mammals cannot synthesize them (2). Considering the adverse effects of synthetic medications, there is a growing trend in the utilization of natural therapies and supplements in the contemporary medical landscape (1).
ω-3 fatty acids such as Eicosapentaenoic acid (EPA), DHA, and Alpha-linolenic acid (ALA), as well as ω-6 fatty acids like LA and AA as shown in Figure 1, are important structural components of the cell membrane. They serve as precursors to bioactive lipid mediators and provide a source of energy. These PUFAs are components of the human and animal diet and are highly regarded as dietary supplements. Numerous studies have previously demonstrated the potential benefits of ω-PUFAs in increasing nutritional consumption, including cardiovascular (4), neurodegenerative (5), inflammatory diseases (6), as well as for some cancer types, mostly prostatic, colorectal, and mammary cancer (7, 8). Concurrently, a considerable number of in vitro and in vivo studies have consistently shown the importance of ω-PUFAs as protective and therapeutic agents against cardiac arrhythmias, hypertriglyceridemia, and inflammation (9).
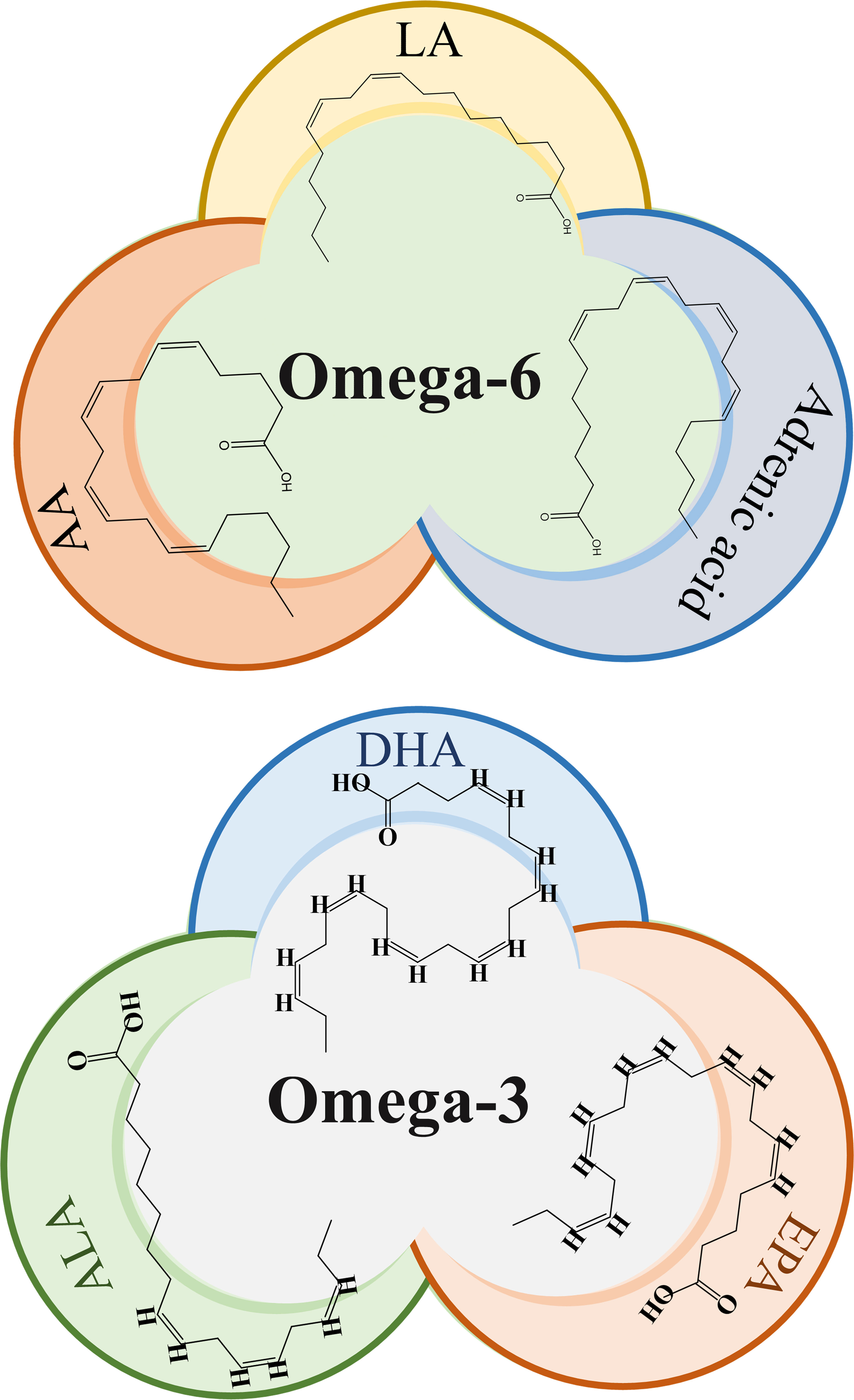
Figure 1 Chemical structure of omega-3 and omega-6 PUFAs. LA, Linoleic acid; AA, Arachidonic acid, ALA, α-linolenic acid; EPA, eicosapentaenoic acid; DHA, docosahexaenoic acid.
Besides their excellent functions in treating innate immune disorders such as allergies or atopic dermatitis, type 1 diabetes (T1D), rheumatoid arthritis (RA), systemic Lupus erythematosus (SLE), and multiple sclerosis (10). ω-PUFAs also showed good potential to prevent various exogenous pathogens, including several kinds of parasites. A recent study has shown that DHA, a representative of ω-3, strongly persuaded autophagy in murine bone marrow-derived macrophages, which may prevent T. gondii infection (11). These PUFAs enhanced recovery and decreased the risk of infection (12). Bioactive derivatives of PUFAs regulate inflammation by influencing the production of molecules such as prostaglandins, leukotrienes, and thromboxane. In addition, specialized pro-resolving mediators (SPMs) like resolvins, protectins, and maresins play an important role in controlling inflammatory responses (13). These SPMs promote anti-inflammatory and pro-resolution properties while maintaining immune function, thereby restoring homeostasis. They accomplish this by downregulating pro-inflammatory cytokines and upregulating anti-inflammatory cytokines. SPMs also help phagocytic cells clear cellular debris and lower oxylipin levels, which promote inflammation. The synthesis of these bioactive mediators from PUFAs such as DHA and EPA involve enzymes such as phospholipases A2 (PLA2s), cyclooxygenases (COX), lipoxygenases (LOX), and cytochrome P450 (CYP450). These mechanisms include inhibiting the metabolism of AA, producing anti-inflammatory mediators, modifying intracellular lipids, and activating nuclear receptors (13, 14). Here, we summarize the sources of ω-3 and ω-6 PUFAs and the current understanding of the therapeutic role of ω-PUFAs in the prevention of various protozoan infections. Moreover, we discuss the current treatment options and suggest future research directions.
Challenges in parasitic diseases and the need for therapeutic progress
Infectious diseases exert significant health impacts on both humans and livestock. The parasite residing in the gut can change the composition of the microbiome, and these changes can have significant effects on gut homeostasis and host immunity (15). Many of these diseases are caused by different parasites. Amongst others, these comprise the causative agents of malaria, coccidiosis, trypanosomiasis, toxoplasmosis, schistosomiasis, etc. Every year, these parasites cause a high rate of mortality and morbidity in endemic countries (16, 17). Recent studies have reported alarming figures, with parasitic infections contributing to a significant percentage of health losses. For instance, in regions affected by malaria, the World Health Organization (WHO) estimated 619,000 malaria deaths globally in 2021 compared to 625,000 in the first year of the pandemic. In 2019, before the pandemic struck, the number of deaths stood at 568,000. The global tally of malaria cases reached 247 million in 2021 compared to 245 million in 2020 and 232 million in 2019 (18).
Similarly, 54.3, 31.7, 70.9, and 52.9% mortality rates associated with the Eimeria parasite have been reported in Turkey, India, Ethiopia, and Nigeria, respectively (19). Meanwhile, Chagas disease affects about 7 million people worldwide (20). These figures underscore the severity of parasitic infections and highlight the urgent need for novel therapeutic agents against these parasites. Meanwhile, vaccines and successful and safe treatment are still deficient, and most of the drugs used were shown drug-resistant. Therefore, novel therapeutic agents against these parasites are urgently needed. Currently, the situation is very serious because in low-income countries the pharmaceutical industries are unable to develop new drugs against these infectious agents. Thus, natural products signify some good opportunities to discover novel therapeutic molecules (16, 17). On the other hand, the occurrence of partial immunity after exposure suggests the potential for a successful and effective vaccine, yet the identification of exact markers for protection remains a complex task (21). The control of parasitic infections needs great attention in the fields of public health, parasitology research, medical science, and political will. However, during the entire spectrum of parasitic diseases of animals and humans, there is an urgent need for better treatment and the search for the best and novel drugs. Recently, natural products have become a beneficial source of treatments for clinical and preventive use, and ω-PUFAs are such kind of attractive components that have gained great attention in clinical research (22).
Sources of omega PUFAs
The consumption of ω-3 PUFAs is generally lacking because of insufficient sources; however, western food is typically the high source of ω-6 PUFAs (23). Most vegetable oils and seeds such as sunflower, corn, wheat, grape seed, rapeseed, poppy seed, palm, hemp, cottonseed, and soybean are rich in ω-6 PUFAs in the form of LA, however, a low proportion of ω-3 ALA. The ALA is frequently found in green leafy vegetables, walnuts, flaxseed, canola oils, and soybeans. However, DHA and EPA are found in fish oils, such as mackerel, salmon, anchovies, trout, sardines, and algae (24–26). AA is a nutritional ω-6 supplement mostly found in poultry, eggs, and meaty organs, while gamma-linolenic acid (GLA) is frequently available in borage oil, evening primrose oil, and rich amounts in human milk. In animal and plant-based diets, these PUFAs originate in different forms such as phospholipids, triacylglycerols, cholesterol esters, and diacylglycerols (27). The animal and plant-based ω-PUFAs sources and the obtained proportion are listed in Table 1.
Parasitic diseases and their available treatments
Toxoplasmosis
Toxoplasmosis is caused by T. gondi, an intracellular pathogen that affects roughly one-third of the global human population. It occurs in nature in a variety of forms, including oocytes, bradyzoites within latent tissue cysts, and actively replicating tachyzoites, which indicate active infection (32). T. gondii can invade nearly all nucleated cells of warm-blooded animals, existing within cells via the development of parasitophorous vacuoles (PV). Alongside PV formation, T. gondii employs various strategies to evade host immune defenses (33). Human transmission occurs through ingestion of contaminated food or water containing sporulated oocytes, consumption of undercooked meat containing latent cysts, vertical transmission from mother to child, or infected allografts during organ transplantation. On the other hand, acquisition via blood products or accidental exposure in laboratory settings is uncommon (34). Toxoplasmosis is a severe infection in immunocompromised patients, resulting in frequent reactivation of latent cysts in patients with chronic infection (35) (Figure 2). The disease is highly prevalence in AIDS patients, but its range has been changed and increased in other immunocompromised persons (36). Toxoplasmosis is particularly life-threatening in hematopoietic stem cell transplant or bone marrow patients (37).
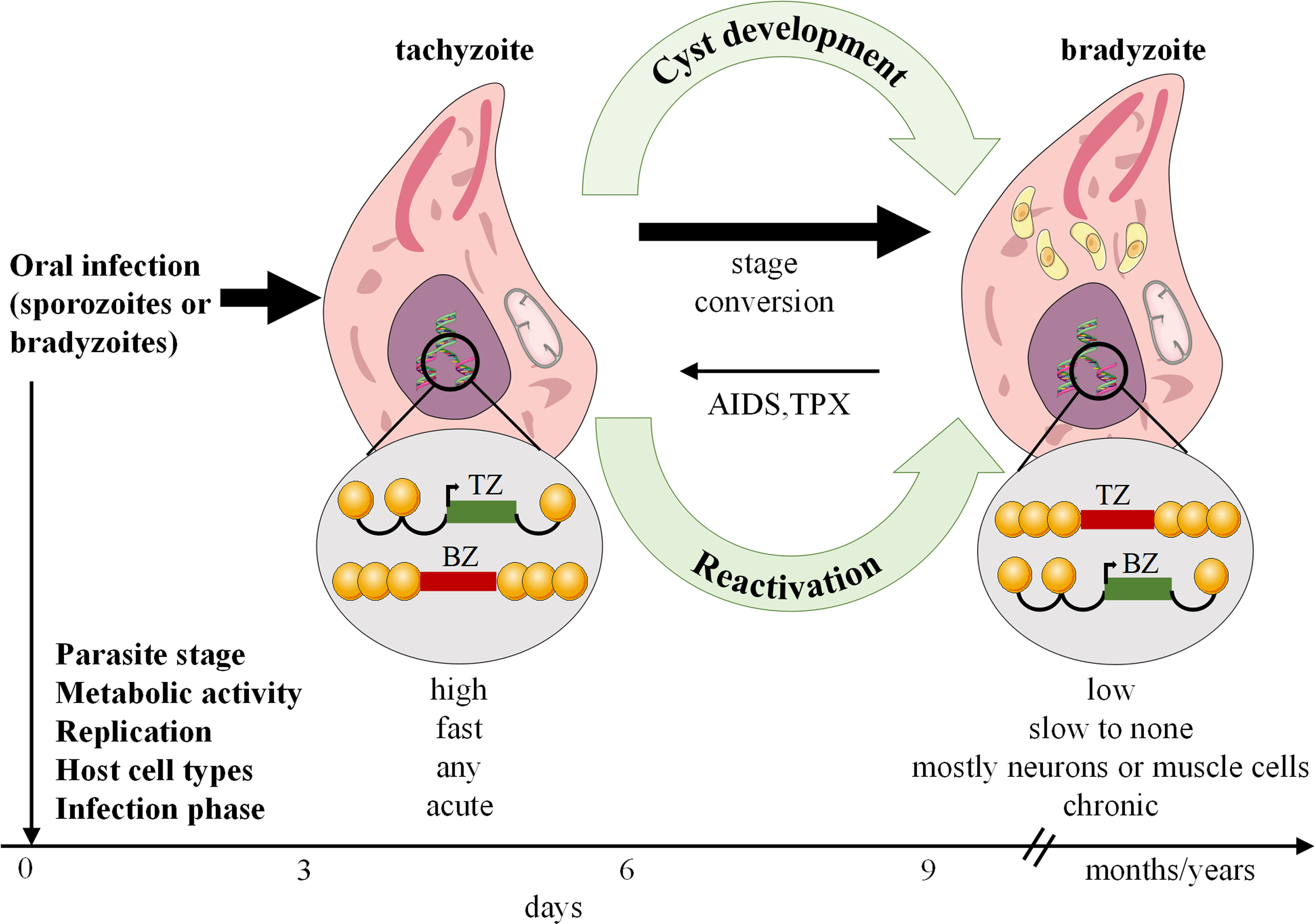
Figure 2 Toxoplasma gondii stage differentiation and its host (cell) microenvironment regulation. After infection, active tachyzoites convert to comparatively latent bradyzoites. The development of bradyzoite comprising tissue cysts for a long time is critical for transmitting the parasite to a new host. In immunocompromised such as AIDS or TPX patients, reconversion of bradyzoites to tachyzoites can occur and lead to serious disease. AIDS, Acquired immunodeficiency syndrome; TPX, Transplant recipients.
Current treatment options for toxoplasmosis
The preferred treatment for toxoplasmosis is a combination of sulphadiazine and Pyrimethamine (SDZ-PYR), which target the active stage of the infection (38). Additionally, alternative therapies are accessible, such as Pyrimethamine combined with certain antibiotics or the use of sulfamethoxazole-trimethoprim (ST) or atovaquone as monotherapy (34). If the patients cannot accept those molecules, an atovaquone 1500 mg combined with PYR can also be used, and SDZ combined with azithromycin 900 to 1200 mg/j can be prescribed (38). Some studies did not show an anti-parasitic relation between SDZ-PYR and PYR-clindamycin, therefore reinforcing the probability of selecting trimethoprim-sulfamethoxazole (39, 40). Trimethoprim-sulfamethoxazole is also the only successful combination for prophylaxis in patients who are at risk for Toxoplasma recurrence. For systemic treatment in immunocompromised patients, a combination of corticosteroids with anti-parasitic drugs was suggested for ocular toxoplasmosis (8, 41). However, comparative studies on this subject are rare. Due to a deficiency in clinical trials, SDZ-PYR is still the treatment choice when combined with corticosteroids (42). Recently, the pharmaceutical industries have been successfully manufacturing medicines using natural products to treat parasitic infections and discover their possible anti-parasitic properties (43).
Omega-PUFAs efficacy in toxoplasmosis
Lipoxin A4, an eicosanoid mediator resultant from 5-lipoxygenase, has been shown to play a significant role in toxoplasmosis. It has been demonstrated that lipoxin A4, along with other arachidonic acid (AA) derivatives, promotes and enhances cyst burdens in tissues while reducing lethality from encephalitis. Previous studies have indicated a close association between AA, particularly lipoxin A4, and the suppression of cytokine generation such as interleukin 12 (IL-12) and IFN-γ (44). Notably, in a mouse model lacking lipoxin, a reduced number of T. gondii brain cysts were observed, accompanied by higher serum levels of IL-12 and IFN-γ (45). These findings suggest that the increased mortality in lipoxin-deficient mice resulted from cytokine-mediated tissue injury, despite effective parasite control. Antigen-presenting dendritic cells are crucial in controlling intracellular pathogens like T. gondii and certain viruses by producing IL-12. A study in mice revealed that lipoxin A4 analogs reduced IL-12 production by dendritic cells stimulated with T. gondii extract (45). This suggests that lipoxin production by pathogens may serve as a mechanism to modulate host immunity, potentially facilitating chronic infection by minimizing tissue damage. The differing roles of lipoxins in these infection models may be attributed to the dynamics of specific pathogen-host interactions. T. gondii replicates rapidly like Mycobacterium tuberculosis, which can trigger inflammatory reactions and subsequent immunopathology (46). Lipoxins demonstrate beneficial effects on the host, increasing survival by mitigating these inflammatory responses.
T. gondii has a considerable amount of unsaturated fatty acids, which are the structural membrane components that play a significant part in energy metabolism (47, 48). During replication, T. gondii requires a substantial quantity of lipids for membrane biogenesis, thus the propagation rates of tachyzoites depend on the production levels of fatty acids and the synthesis of new membranes. Additionally, the metabolism of fatty acids at the interface between the host and the parasite also influences T. gondii tachyzoites (49–51). Moreover, it has been demonstrated that the interface of fatty acid metabolism induces inflammatory cytokine production in the host and triggers calcium release from neutrophils, thereby facilitating the egress of parasites from infected host cells (52–54). However, Zhou et al. indicated that α-linolenic acid metabolism was significantly disrupted in mice infected with T. gondii (55). The administration of sulfadiazine sodium ameliorates the metabolomic perturbation in mice infected with T. gondii. These alterations in fatty acid metabolism may play a crucial role in facilitating T. gondii tachyzoites within cells. Hence, some authors have recommended the use of suitable nutritional supplementation, such as specific fatty acids (e.g., acyl-coenzyme A: diacylglycerol acyltransferase), in immunocompromised individuals to prevent chronic toxoplasmosis (56, 57).
Possible mechanism of ω-PUFAs in T. gondii infection
Numerous studies have elucidated the essential role of autophagy in removing intracellular pathogens through xenophagy. However, during T. gondii infection, two types of autophagy, canonical and non-canonical, are involved (58, 59). It has been suggested that ω-3 PUFAs play a significant role in regulating T. gondii infection both in vivo and in vitro (11). In primary macrophages, DHA significantly induces autophagy and mediates its activity, which is necessary to regulate and control T. gondii growth intracellularly (11). Moreover, the AMP-activated protein kinase (AMPK) activates the host-defense mechanism by regulating inflammatory responses and innate immunity to control infectious disease agents such as bacteria, viruses, and parasites (60). A recent study demonstrated that the calcium/calmodulin-dependent protein kinase kinase (CaMKK)/AMPK signaling pathways are required to eliminate T. gondii via CD40-induced autophagy (61). The two signaling pathways, CaMKKβ/AMPK-mediated autophagy, are essential for the host to control T. gondii infection. The proposed mechanism for this protection is depicted in Figure 3.
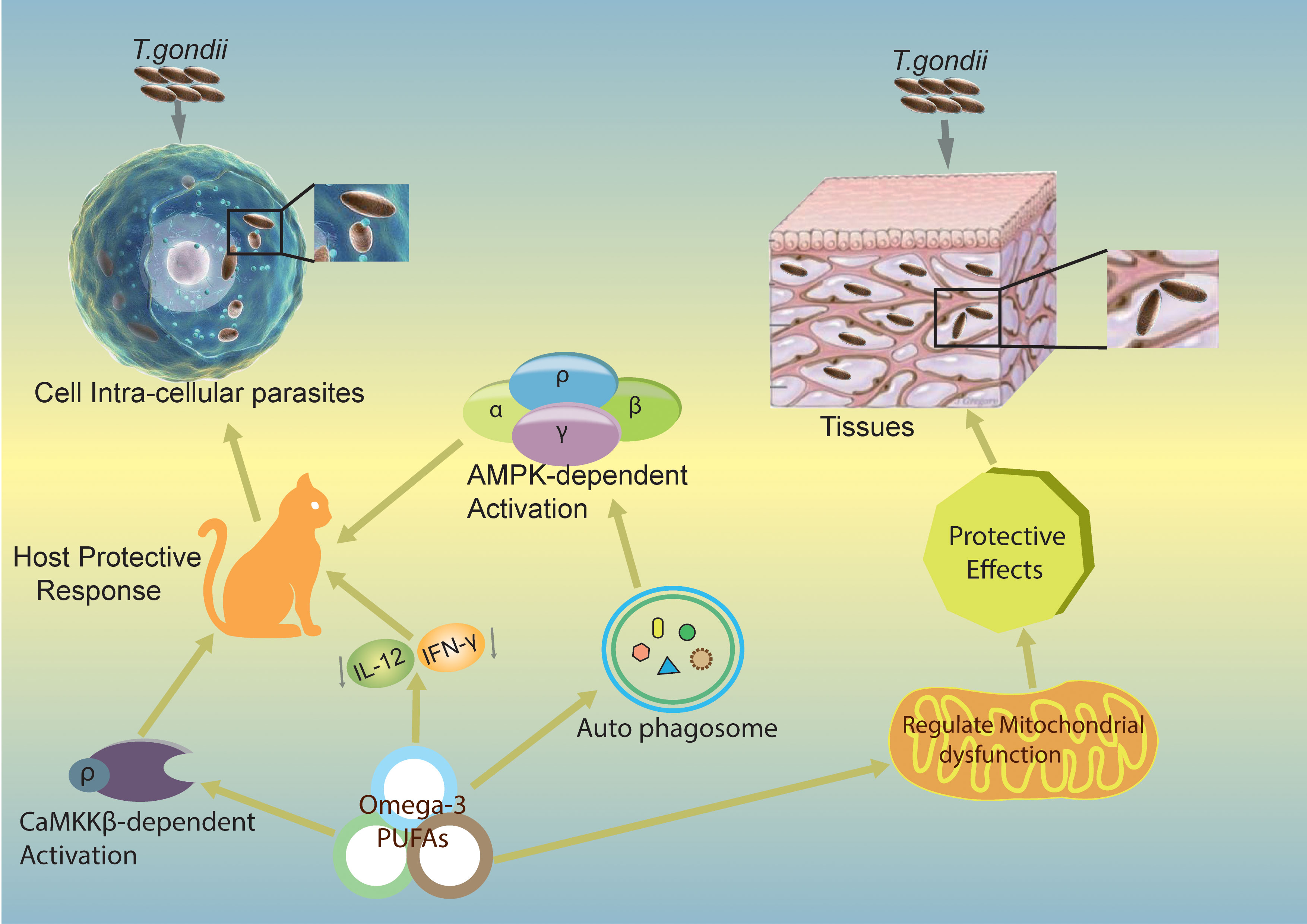
Figure 3 Mechanisms by which ω-3 PUFAs inhibit T. gondii infection. The figure shows potential mechanisms of action of ω-3 PUFAs in different pathways and proposes that ω-3 PUFAs are capable of producing a host-defensive response against T. gondii infection. Omega-3 induces CaMKKβ/AMPK-dependent autophagy signaling pathways activation (11) and decreases IL-12/IL-γ cytokines, which can lead to a produced host-defensive response against T. gondii infection. CaMKKβ, calmodulin-dependent protein kinase kinase β; AMPK, Adenosine monophosphate-activated protein kinase; IL-12, Interleukin 12; IL-γ, Interleukin gamma.
Studies have shown that nutrients play a crucial role in activating and maintaining immune homeostasis. Different nutrients, such as fatty acids, mediate both the acquired and innate immune systems through different pathways such as Toll-like receptor 4 (62). Similarly, various lipid components act as immune-activating factors and energy sources. During T. gondii infection, lipid biosynthesis occurs through salvage pathways, which play an essential role in intracellular pathogenesis and present promising chemotherapeutic targets in lipid synthesis and salvage pathways (49, 63). Moreover, a study recommended that the immunomodulatory functions of ω-3 PUFAs may be useful in inflammatory and infectious diseases (64).
Malaria
Malaria is a mosquito-borne infectious disease caused by a protozoan parasite of the genus Plasmodium (65). The symptoms include fever, vomiting, fatigue, and headaches. In critical cases, malaria can cause seizures, yellow skin, coma, or even death (66). According to the World Health Organization, 228 million malarial cases occurred in 2018 (67).
Current antimalarial drugs
The treatment of malaria and the choice of drug depends on the Plasmodium spp., drug resistance, geographical location, and disease severity (68). The complicated cases of malaria are treated with chloroquine 1000 mg (600 mg base), and it is the best choice of drug in most Plasmodium spp., except P. falciparum, which has become highly resistant in most cases (69). A combination of amodiaquine and artesunate, as well as atovaquone combined with proguanil (250 mg/100 mg), or an artemether 20 mg-lumefantrine 120 mg (twice daily for 3 days), can be used to treat chloroquine-resistant P. falciparum (69). During pregnancy, specified oral quinine and parenteral quinine are beneficial for severe malaria treatment. Doxycycline, mefloquine, primaquine, and atovaquone-proguanil are the drugs chosen for chemoprophylaxis (69). The list of antimalarial drugs and their mechanisms of action are shown in Table 2.
Omega-PUFAs efficacy in malaria
The effects of ω-PUFAs on malarial causative agents such as P. berghei or P. falciparum are the most reliable and easily understood. Studies have shown that indomethacin did not affect cerebral malaria or parasitemia development; however, ω-3 and ω-6 PUFAs acted directly on the pathogen and constrained parasitemia both in vivo and in vitro (82). This inhibition mechanism depends on the concentration of fatty acids, their unsaturation, and chain length. A mouse model infected with P. berghei and treated for 4 days with fish oils rich in PUFAs showed suppressive effects against the parasite (82, 83). In another study, the authors confirmed that human phospholipids-derived PUFAs exert a strong in vitro anti-plasmodium action mainly by hydrolyzing lipoproteins from plasma, thus releasing PUFAs that are toxic to the parasite (84).
The addition of antioxidants or reactive oxygen species decreased ω-3 PUFA’s capability to kill P. falciparum, but the oxidized fatty acid’s addition improved their capability to destroy the parasite (85). A study conducted by Blok et al. showed that there was no requirement of vitamin E deficiency for ω-PUFAs to distress antimalarial resistance (82, 86). Fish oil consumption significantly reduced hepatic and plasma vitamin E. However, it did not alter the immune cell vitamin E status (82). These results strongly proposed that ω-PUFAs increased host survival rate without showing any changes in the host immune system and exerted direct cytotoxic action on Plasmodium spp.
Coccidiosis
Coccidia is a group of obligate intracellular protozoan parasites that fall under the class Conoidasida within the phylum Apicomplexa. Two notable genera within this group are Eimeria, characterized by sporulated oocysts containing four sporocysts, and Isospora, which has sporulated oocysts with two sporocysts. These genera include a wide variety of species capable of infecting birds, mammals, and reptiles, but it is essential to emphasize that most of these species demonstrate a strong preference for specific host species. Infections caused by these parasites are widespread across the globe (87).
Existing strategies to control coccidiosis
Although good animal husbandry can reduce coccidian parasite transmission, some additional measures are important to lower the risk of infection (88). Currently, some coccidiocidal drugs are used as regular prevention and therapies for the disease and inhibit the growth and replication of coccidial populations. When anticoccidial drugs are withdrawn, the infective oocysts contaminate the environment again and continue their life cycle (89).
The combination of several drugs shows more efficiency in controlling coccidiosis. In this regard, since the 1960s, sulfonamide has been used as a potent drug for the control of Eimeria spp. infection, many chemical synthetic drugs, and ionophores compounds were developed or found using coccidiostatic or coccidiocidal agents. However, new potent molecules urgently need to be found and used to control the disease because of the increasing drug resistance (89). To stop drug resistance, the rotation and shuttle systems are urgently needed, and drug type is used to switch after one or several (90). The attenuated and un-attenuated vaccines are also used aiming to control coccidiosis; however, the effectiveness of vaccines is based on oocysts and immunity (91). The practice of live un-attenuated vaccines, such as Inovocox, Coccivac, etc., is inadequate because they induce the risk of live parasites (92). However, live attenuated vaccines such as Paracox and HatchPak CocciIII reduce disease risk, and the intestinal segments of birds are less damaged (93). To fully combat the infection, there is still a required fully effective anticoccidial drug or vaccine according to the species-specific nature of immunity. Moreover, the anticoccidial drug resistance detected in birds worldwide has shown that natural products with efficient anti-coccidial activity will be more efficient (94).
Omega-PUFAs efficacy in coccidiosis
The combination of ω-PUFAs in the diet of chicken challenged with Eimeria tenella decreases the contrary effects on development and gut lesion scores. The inclusion of ω-3 PUFAs can help to control E. tenella infection and improve the resistance of poultry to the pathogen. These effects in broiler chickens were investigated compared to the earlier findings, which showed ω-3 PUFAs afford some protective effects against malaria (95). The carotenoid level in total plasma reduced the infection of coccidia (96), while free radicals such as peroxynitrite levels increased (97). Researchers assumed that DHA, EPA, and LA generate oxidative stress that stops coccidia development by being incorporated into the membranes of the parasite. There, they are extremely prone to oxidation by leucocyte-free radical manufacturing, which affects the coccidian (97). In another study, corn oil was compared with fish oil in E. tenella-infected chickens, in which fish oil successfully reduced lesion score, TNF-α, and inflammatory process (98).
Chagas disease
Chagas disease (CD), commonly known as American trypanosomiasis, is a potentially fatal infection caused by the protozoan parasite Trypanosoma cruzi. T. cruzi is a parasitic protozoan spread to mammalian hosts by blood-sucking bugs called triatomine, and in humans and animals, it leads to anemia, parasitemia, and hepatosplenomegaly (99). The mortality rate during acute T. cruzi infection is rare, but its occurrence results severe decline in leukocytes and circulating platelets (100, 101). Everything that disturbs host immune responses, such as AIDS, aging, chemotherapy, or antirejection treatment resulting in the transplantation of an organ, can endorse a recurrence of this dormant infection with successive morbidity (102, 103).
Trypanocidal agents
Different compounds, such as mercury chloride, arsenic, emetic tartrate, fuchsine, and even the antiseptic gentian violet, were tried to treat the disease. However, the results were unsatisfactory (102, 103). Later in the 1960s, numerous novel compounds, such as the nitrofurans that act as an antimicrobial agent, were tested in which nitrofurfurylidene, known as nifurtimox (RS)-3-methyl-N-[(1E)-(5- nitro-2-furyl) methylene] thiomorpholin-4-amine 1, 1-dioxide) (NF), showed satisfactory results (104).
Nevertheless, the first promising drug for CD treatment was Nifurtimox (NFX), which was used by Packchanian (105), and its clinical trials were started in South America in 1965. In adult, chronically infected patients, the effectiveness of NFX was low, with a rate of 7-8%, but in young children aged < 14 years, this drug showed a higher cure rate of 85.7% (106). The most common adverse effects of NFX are weight loss, anorexia, gastrointestinal symptoms, vomiting, etc. Another drug, benznidazole (BZ), was shown to be useful for the treatment of CD (104). BZ was shown to be less toxic than NFX. However, their efficacy for treating CD is almost similar. Age is a significant factor for BZ effectiveness as well. In children around 6-12 years of age, BZ showed a higher cured efficacy of 56-62% (104). The mechanisms of action of both BZ and NFX are still unclear; however, it has been shown that BZ acts as a reductive stress molecule that affects the trypanothione metabolism of T. cruzi (107). This drug can also induce IFN-γ, raise trypanosomal death, and improve phagocytosis via inhibiting T. cruzi NADH-fumarate reductase (104). NFX produces extremely toxic oxygen metabolites that render T. cruzi to limited reduction products of oxygen, typically hydrogen peroxide (104). The complete list of drugs and their status for the treatment of CD are shown in Table 3.
Omega-PUFAs and T. cruzi
Nutrient supplements and some dietary fats are known to have influenced the function of the immune system (108). They can affect both cellular (T cells, B cells, and natural killer (NK) cells that may modulate the production and activity of cytokines) and innate immunity, influencing survival during infections. These supplements may contribute to the protection against parasitic infections including T. cruzi. Previous studies demonstrated that ω3 and ω6 PUFAs impacted cellular and innate immunity (109, 110). These PUFAs also affected the survival of mice following an experimental T. cruzi infection and showed fewer indications during the acute phase of this parasitic infection (111). The mechanisms for this protection are shown in Figure 4. Recently, many studies stated the trypanocidal actions of some natural medicine or their extracts (107, 113). In some Asian countries such as Vietnam and Uzbekistan, there was much use of plant extracts and their constituents that showed trypanocidal activities against T. cruzi (114).
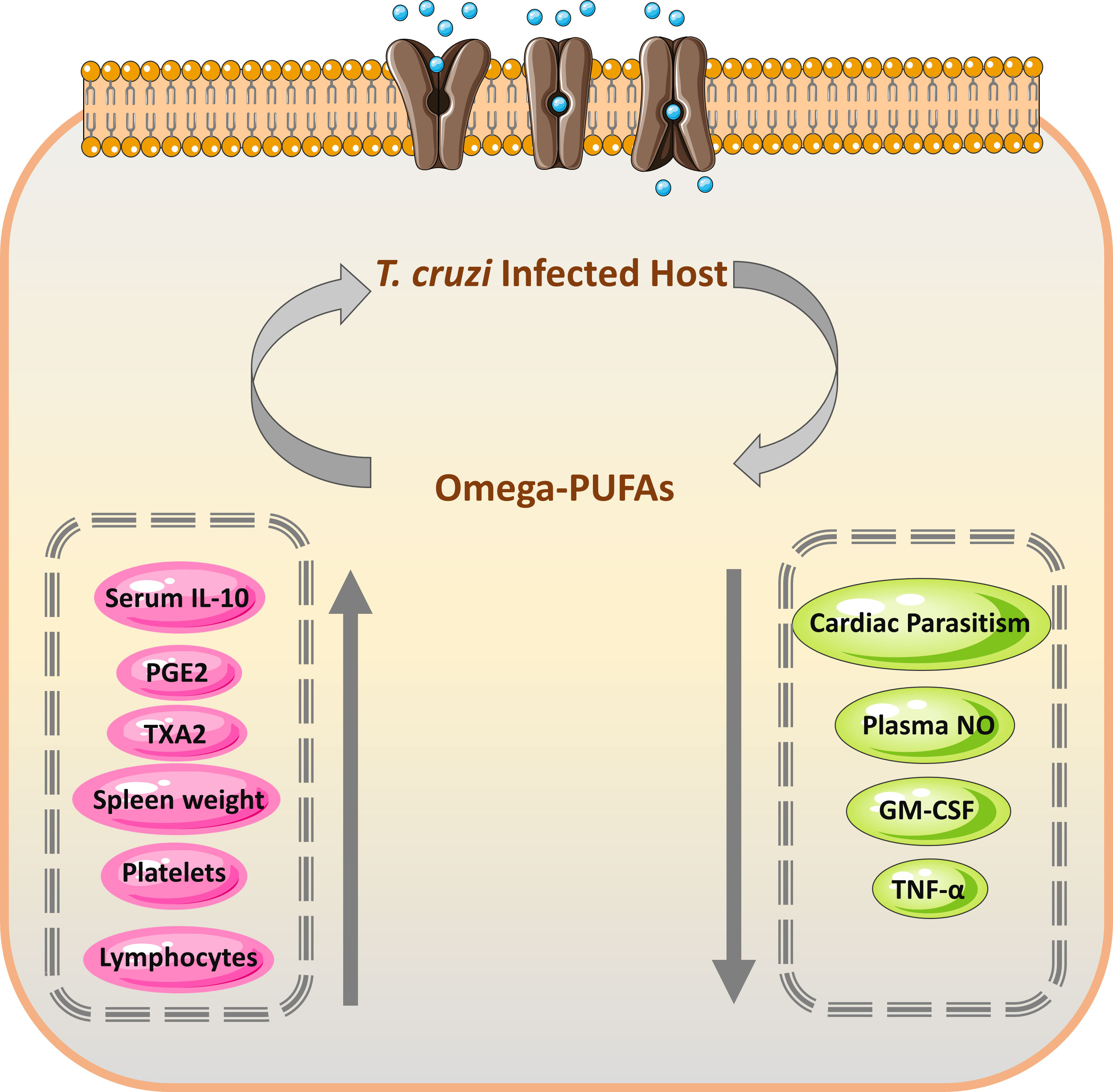
Figure 4 Mechanism and efficacy of omega-PUFAs supplementation on T. cruzi infection. During the acute form of T. cruzi infection, the increased level of prostaglandin E2 (PGE2) results in temporary immunosuppression, which leads to decreased production of TNF-α by the host (112). The immune cells from the omega-PUFAs diet lowered PGE2 amounts and consequently increased the level of TNF-α leading to decreased parasitemia (111). PGE2, Prostaglandin E2; TXA2, thromboxane A2; GM-CSF, Granulocyte-macrophage colony-stimulating factor; TNF-α, Tumor necrosis factor-alpha.
Possible drawbacks related to the use of ω-PUFAs
While omega polyunsaturated fatty acid ω-PUFAs offer numerous health benefits, there are also potential drawbacks associated with their uses. Due to the use of ω-PUFAs, there is the possibility of digestive issues, allergic reactions, and bleeding risk. The possibility that enhances these drawbacks is discussed below.
Inadequate associated studies and clinical trials
In China, ω-3 and ω-6 PUFAs have been widely studied in treating several kinds of cardiovascular diseases, cancer, inflammations, physical damage, etc., while research on the anti-parasitic functions is seldom reported. Moreover, researchers have obtained information on ω-3 and ω-6 PUFAs as described above, but the clinical studies trials are still deficient. These studies are critical to validate the anti-parasitic activities of ω-3 and ω-6 PUFAs.
Dosage
Until now, the recommended dosage of PUFAs has also been an issue in clinical trials. Previously, in cancer studies the researchers used a higher dose level of EPA + DHA ranging from 2.0 to 8.0 g/kg body weight/day in mice (115). Subsequently, the interspecies alteration lower dosage for animals also seemed higher for humans (about 10–20 g/day in 70 kg per person) (116). In human interventional experimental trials, the protective effects of ω-3 PUFAs were observed with a 2.0 g/day dosage (consistent with 0.03 g/kg body weight in a 70 kg person) (117, 118). The best preventive effects of these PUFAs were accompanied by increasing the dosage of tissue lipids or plasma in both humans and animals (115). Excessive PUFAs could lead to unintentional health problems in certain conditions and nutritional standards based on the best available evidence still need to be established.
Administration route
Different routes, such as oral, inhalational, intravenous, and topical, are used to administer substances into the body. The administration route for an active component to the targeted place is significantly important. Experimental trials have shown the effects of ALA on numerous conditions in humans, such as lipid metabolism ailments and cardiovascular diseases, but many of them work on the oral direction as the entry route. For ω-PUFAs, strong solubility and good delivery routes are important because poor solubility and delivery may have influenced the bioavailability of PUFAs (119, 120). Thus, additional human and animal studies are particularly needed to precisely display the administration route of ω-PUFAs with the best delivery ability.
Conclusion and future perspectives
The treatment options for parasitic infections are not sufficiently effective due to significant side effects, necessitating the urgent development of novel therapeutic choices. We propose that ω-3 and ω-6 PUFAs may be potentially effective in ameliorating some parasitic infections and associated abnormal conditions. These PUFAs have been proven to play a key role in modulating immune responses and activating certain signaling pathways in various parasitic infections. The evidence elucidated in the current review suggests that further experiments would be essential to combine these PUFAs with other drug candidates to assess the clinical impact of ω-3 and ω-6 in parasitic infections. Finally, we recommend that future studies focus on:
1.Understanding of the main pathway/s responsible for the activities of ω-3 and ω-6 PUFAs in parasitic infections.
2.Determining the most effective doses for the beneficial role of ω-PUFAs in various parasitic diseases.
3.Exploring the possible effects of ω-3 and ω-6 on emerging treatments, such as microRNAs by targeting signaling pathways and or expression profiles.
4.Examining the possible interactions of ω-3 or ω-6 with well-known anti-parasitic drugs, as well as nutritional supplements.
Author contributions
SUR: Writing – review & editing, Writing – original draft, Software. TW: Writing – review & editing. AQ: Writing – review & editing, Investigation. SN: Writing – review & editing. HU: Writing – review & editing. CC: Writing – review & editing, Validation, Funding acquisition.
Funding
The author(s) declare that no financial support was received for the research, authorship, and/or publication of this article.
Acknowledgments
We thank all the authors of the primary studies included in this article. We also wish to thank reviewers for their kind advice.
Conflict of interest
The authors declare that the research was conducted in the absence of any commercial or financial relationships that could be construed as a potential conflict of interest.
Publisher’s note
All claims expressed in this article are solely those of the authors and do not necessarily represent those of their affiliated organizations, or those of the publisher, the editors and the reviewers. Any product that may be evaluated in this article, or claim that may be made by its manufacturer, is not guaranteed or endorsed by the publisher.
References
1. Alhusseiny SM, El-Beshbishi SN. Omega polyunsaturated fatty acids and parasitic infections: An overview. Acta Trop. (2020) 207:105466. doi: 10.1016/j.actatropica.2020.105466
2. Li X, Bi X, Wang S, Zhang Z, Li F, Zhao AZ. Therapeutic potential of ω-3 polyunsaturated fatty acids in human autoimmune diseases. Front Immunol. (2019) 10:2241. doi: 10.3389/fimmu.2019.02241
3. Kang JX. The importance of omega-6/omega-3 fatty acid ratio in cell function. The gene transfer of omega-3 fatty acid desaturase. World Rev Nutr Diet. (2003) 92:23–36. doi: 10.1159/000073790
4. Kromhout D, de Goede J. Update on cardiometabolic health effects of ω-3 fatty acids. Curr Opin Lipidol. (2014) 25:85–90. doi: 10.1097/MOL.0000000000000041
5. Janssen CIF, Kiliaan AJ. Long-chain polyunsaturated fatty acids (LCPUFA) from genesis to senescence: the influence of LCPUFA on neural development, aging, and neurodegeneration. Prog Lipid Res. (2014) 53:1–17. doi: 10.1016/j.plipres.2013.10.002
6. Calder PC. Marine omega-3 fatty acids and inflammatory processes: Effects, mechanisms and clinical relevance. Biochim Biophys Acta Mol Cell Biol Lipids. (2015) 1851:469–84. doi: 10.1016/j.bbalip.2014.08.010
7. Gu Z, Suburu J, Chen H, Chen YQ. Mechanisms of omega-3 polyunsaturated fatty acids in prostate cancer prevention. BioMed Res Int. (2013) 2013:824563. doi: 10.1155/2013/824563
8. Bougnoux P, Hajjaji N, Maheo K, Couet C, Chevalier S. Fatty acids and breast cancer: Sensitization to treatments and prevention of metastatic re-growth. Prog Lipid Res. (2010) 49:76–86. doi: 10.1038/sj.bjc.6605441
9. Darwesh AM, Bassiouni W, Sosnowski DK, Seubert JM. Can N-3 polyunsaturated fatty acids be considered a potential adjuvant therapy for COVID-19-associated cardiovascular complications? Pharmacol Ther. (2021) 219:107703. doi: 10.1016/j.pharmthera.2020.107703
10. Poggioli R, Hirani K, Jogani VG, Ricordi C. Modulation of inflammation and immunity by omega-3 fatty acids: a possible role for prevention and to halt disease progression in autoimmune, viral, and age-related disorders. Eur Rev Med Pharmacol Sci. (2023) 27:7380–400. doi: 10.26355/eurrev_202308_33310
11. Choi J-W, Lee J, Lee J-H, Park B-J, Lee EJ, Shin S, et al. Omega-3 Polyunsaturated Fatty Acids Prevent Toxoplasma gondii Infection by Inducing Autophagy via AMPK Activation. Nutrients. (2019) 11:2137. doi: 10.3390/nu11092137
12. Gammone MA, Riccioni G, Parrinello G, D'Orazio N. Omega-3 polyunsaturated fatty acids: benefits and endpoints in sport. Nutrients. (2018) 11:46. doi: 10.3390/nu11010046
13. Al-Khalaifah H. Modulatory effect of dietary polyunsaturated fatty acids on immunity, represented by phagocytic activity. Front Vet Sci. (2020) 7:569939. doi: 10.3389/fvets.2020.569939
15. Rahman SU, Gong H, Mi R, Huang Y, Han X, Chen Z. Chitosan protects immunosuppressed mice against Cryptosporidium parvum infection through TLR4/STAT1 signaling pathways and gut microbiota modulation. Front Immunol. (2021) 12:784683. doi: 10.3389/fimmu.2021.784683
16. Schmidt TJ, Khalid SA, Romanha AJ, Alves TM, Biavatti MW, Brun R, et al. The potential of secondary metabolites from plants as drugs or leads against protozoan neglected diseases - part II. Curr Med Chem. (2012) 19:2176–228. doi: 10.2174/092986712800229087
17. Christen P, Cuendet M. Plants as a source of therapeutic and health products. Chimia (Aarau). (2012) 66:320–3. doi: 10.2533/chimia.2012.320
18. MMV. World Malaria Report 2022. Geneva: Geneva: World Health Organization; 2022. Licence: CC BY-NC-SA 3.0 IGO.
19. Fatoba AJ, Adeleke MA. Diagnosis and control of chicken coccidiosis: a recent update. J Parasit Dis. (2018) 42:483–93. doi: 10.1007/s12639-018-1048-1
20. Santos SS, de Araújo RV, Giarolla J, Seoud OE, Ferreira EI. Searching for drugs for Chagas disease, leishmaniasis and schistosomiasis: a review. Int J Antimicrob Agents. (2020) 55:105906. doi: 10.1016/j.ijantimicag.2020.105906
21. Rahman SU, Mi R, Zhou S, Gong H, Ullah M, Huang Y, et al. Advances in therapeutic and vaccine targets for Cryptosporidium: Challenges and possible mitigation strategies. Acta Trop. (2022) 226:106273. doi: 10.1016/j.actatropica.2021.106273
22. Sepúlveda-Arias JC, Gómez-Marin JE, Bobi? B, Naranjo-Galvis CA, Djurkovi?-Djakovi? O. Toxoplasmosis as a travel risk. Travel Med Infect Dis. (2014) 12:592–601. doi: 10.1016/j.tmaid.2014.05.007
23. Moghadasian MH. Advances in dietary enrichment with n-3 fatty acids. Crit Rev Food Sci Nutr. (2008) 48:402–10. doi: 10.1080/10408390701424303
24. Saini RK, Shang XM, Ko EY, Choi JH, Kim D, Keum Y-S. Characterization of nutritionally important phytoconstituents in minimally processed ready-to-eat baby-leaf vegetables using HPLC–DAD and GC–MS. J Food Meas Charact. (2016) 10:341–49. doi: 10.1007/s11694-016-9312-5
25. Saini RK, Shetty NP, Giridhar P. GC-FID/MS analysis of fatty acids in Indian cultivars of moringa oleifera: potential sources of PUFA. J Am Oil Chem Soc. (2014) 91:1029–34. doi: 10.1007/s11746-014-2439-9
26. Harwood JL. Algae: critical sources of very long-chain polyunsaturated fatty acids. Biomolecules. (2019) 9. doi: 10.3390/biom9110708
27. Parmentier M, Al Sayed Mahmoud C, Linder M, Fanni J. Polar lipids: n-3 PUFA carriers for membranes and brain: nutritional interest and emerging processes. OCL. (2007) 14:224–29. doi: 10.1051/ocl.2007.0127
28. U.S. Department of agriculture, agricultural research service. Food data central (2019). Available online at: https://fdc.nal.usda.gov.
29. Banca dati di composizione degli alimenti per studi epidemiologici in Italia (BDA) . Available online at: http://www.bda-ieo.it/wordpress/en/?page_id=23.
30. Calviello G, Serini S, Piccioni E. n-3 polyunsaturated fatty acids and the prevention of colorectal cancer: molecular mechanisms involved. Curr Med Chem. (2007) 14:3059–69. doi: 10.2174/092986707782793934
31. Calviello G, Serini S, Piccioni E. Alzheimers disease and n-3 polyunsaturated fatty acids: beneficial effects and possible molecular pathways involved. Curr Signal Transduct Ther. (2008) 3:152–57. doi: 10.2174/157436208785699659
32. Montoya JG, Liesenfeld O. Toxoplasmosis. Lancet. (2004) 363:1965–76. doi: 10.1016/S0140-6736(04)16412-X
33. Denkers EY. Toll-like receptor initiated host defense against. Toxoplasma gondii. J BioMed Biotechnol. (2010) 2010:737125–25. doi: 10.1155/2010/737125
34. Dunay IR, Gajurel K, Dhakal R, Liesenfeld O, Montoya JG. Treatment of toxoplasmosis: historical perspective, animal models, and current clinical practice. Clin Microbiol Rev. (2018) 31. doi: 10.1128/CMR.00057-17
35. Sullivan WJ, Jeffers V. Mechanisms of Toxoplasma gondii persistence and latency. FEMS Microbiol Rev. (2012) 36:717–33. doi: 10.1111/j.1574-6976.2011.00305.x
36. Robert-Gangneux F, Sterkers Y, Yera H, Accoceberry I, Menotti J, Cassaing S, et al. Molecular diagnosis of toxoplasmosis in immunocompromised patients: a 3-year multicenter retrospective study. J Clin Microbiol. (2015) 53:1677–84. doi: 10.1128/jcm.03282-14
37. Gajurel K, Dhakal R, Montoya JG. Toxoplasma prophylaxis in haematopoietic cell transplant recipients: a review of the literature and recommendations. Curr Opin Infect Dis. (2015) 28:283–92. doi: 10.1097/qco.0000000000000169
38. Konstantinovic N, Guegan H, Stäjner T, Belaz S, Robert-Gangneux F. Treatment of toxoplasmosis: Current options and future perspectives. Food Waterborne Parasitol. (2019) 15:e00036. doi: 10.1093/ps/76.10.1355
39. Hernandez AV, Thota P, Pellegrino D, Pasupuleti V, Benites-Zapata VA, Deshpande A, et al. A systematic review and meta-analysis of the relative efficacy and safety of treatment regimens for HIV-associated cerebral toxoplasmosis: is trimethoprim-sulfamethoxazole a real option? HIV Med. (2017) 18:115–24. doi: 10.1111/hiv.12402
40. Wei HX, Wei SS, Lindsay DS, Peng HJ. A systematic review and meta-analysis of the efficacy of anti-Toxoplasma gondii medicines in humans. PLoS One. (2015) 10:e0138204. doi: 10.1371/journal.pone.0138204
41. Ozgonul C, Besirli CG. Recent developments in the diagnosis and treatment of ocular toxoplasmosis. Ophthalmic Res. (2017) 57:1–12. doi: 10.1159/000449169
42. Butler NJ, Furtado JM, Winthrop KL, Smith JR. Ocular toxoplasmosis II: clinical features, pathology and management. Clin Exp Ophthalmol. (2013) 41:95–108. doi: 10.1111/j.1442-9071.2012.02838.x
43. Kavitha N, Noordin R, Chan K-L, Sasidharan S. In vitro anti-Toxoplasma gondii activity of root extract/fractions of Eurycoma longifolia Jack. BMC complement altern Med. (2012) 12:91–1. doi: 10.1186/1472-6882-12-91
44. Aliberti J, Serhan C, Sher A. Parasite-induced lipoxin A4 is an endogenous regulator of IL-12 production and immunopathology in Toxoplasma gondii infection. J Exp Med. (2002) 196:1253–62. doi: 10.1084/jem.20021183
45. Russell CD, Schwarze J. The role of pro-resolution lipid mediators in infectious disease. Immunology. (2014) 141:166–73. doi: 10.1111/imm.12206
46. Bafica A, Scanga CA, Serhan C, MaChado F, White S, Sher A, et al. Host control of Mycobacterium tuberculosis is regulated by 5-lipoxygenase-dependent lipoxin production. J Clin Investig. (2005) 115:1601–06. doi: 10.1172/JCI23949
47. Ramakrishnan S, Serricchio M, Striepen B, Bütikofer P. Lipid synthesis in protozoan parasites: a comparison between kinetoplastids and apicomplexans. Prog Lipid Res. (2013) 52:488–512. doi: 10.1016/j.plipres.2013.06.003
48. Ramakrishnan S, Docampo MD, Macrae JI, Pujol FM, Brooks CF, van Dooren GG, et al. Apicoplast and endoplasmic reticulum cooperate in fatty acid biosynthesis in apicomplexan parasite Toxoplasma gondii. J Biol Chem. (2012) 287:4957–71. doi: 10.1074/jbc.M111.310144
49. Ramakrishnan S, Docampo MD, MacRae JI, Ralton JE, Rupasinghe T, McConville MJ, et al. The intracellular parasite Toxoplasma gondii depends on the synthesis of long-chain and very long-chain unsaturated fatty acids not supplied by the host cell. Mol Microbiol. (2015) 97:64–76. doi: 10.1111/mmi.13010
50. Formaglio P, Tavares J, Ménard R, Amino R. Loss of host cell plasma membrane integrity following cell traversal by Plasmodium sporozoites in the skin. Parasitol Int. (2014) 63:237–44. doi: 10.1016/j.parint.2013.07.009
51. Qian J, Zhao T, Guo L, Li S, He Z, He M, et al. Mitochondrial ADP/ATP carrier 1 is important for the growth of Toxoplasma tachyzoites. Microbiol Spectr. (2023) 11:e0004023. doi: 10.1128/spectrum.00040-23
52. Arlia-Ciommo A, Svistkova V, Mohtashami S, Titorenko VI. A novel approach to the discovery of anti-tumor pharmaceuticals: searching for activators of liponecrosis. Oncotarget. (2016) 7:5204–25. doi: 10.18632/oncotarget.6440
53. Mulvihill MM, Nomura DK. Therapeutic potential of monoacylglycerol lipase inhibitors. Life Sci. (2013) 92:492–97. doi: 10.1016/j.lfs.2012.10.025
54. Yang J, Liu S, Zhao Q, Li X, Jiang K. Gut microbiota-related metabolite alpha-linolenic acid mitigates intestinal inflammation induced by oral infection with. Toxoplasma gondii. Microbiome. (2023) 11:273. doi: 10.1186/s40168-023-01681-0
55. Zhou C-X, Gan Y, Elsheikha HM, Chen X-Q, Cong H, Liu Q, et al. Sulfadiazine sodium ameliorates the metabolomic perturbation in mice infected with Toxoplasma gondii. (2019) 63:e00312–19. doi: 10.1128/aac.00312-19
56. Zhang J, Qin XIN, Zhu YU, Zhang S, Zhang X-W, Lu HE. Mechanism of dexamethasone in the context of Toxoplasma gondii infection. Parasitol. (2017) 144:1551–59. doi: 10.1017/S0031182017001111
57. Nolan SJ, Romano JD, Kline JT, Coppens I. Novel approaches to kill Toxoplasma gondii by exploiting the uncontrolled uptake of unsaturated fatty acids and vulnerability to lipid storage inhibition of the parasite. Antimicrob Agents Chemother. (2018) 62:e00347–18. doi: 10.1128/AAC.00347-18
58. Selleck EM, Orchard RC, Lassen KG, Beatty WL, Xavier RJ, Levine B, et al. A noncanonical autophagy pathway restricts Toxoplasma gondii growth in a strain-specific manner in IFN-γ-activated human cells. mBio. (2015) 6:e01157. doi: 10.1128/mBio.01157-15
59. Lévêque MF, Berry L, Cipriano MJ, Nguyen H-M, Striepen B, Besteiro S. Autophagy-related protein ATG8 has a noncanonical function for apicoplast inheritance in Toxoplasma gondii. mBio. (2015) 6:e01446. doi: 10.1128/mBio.01446-15
60. Silwal P, Kim JK, Yuk J-M, Jo E-K. AMP-activated protein kinase and host defense against infection. Int J Mol Sci. (2018) 19:3495. doi: 10.3390/ijms19113495
61. Liu E, Lopez Corcino Y, Portillo J-AC, Miao Y, Subauste CS. Identification of Signaling Pathways by Which CD40 Stimulates Autophagy and Antimicrobial Activity against Toxoplasma gondii in Macrophages. Infect Immun. (2016) 84:2616–26. doi: 10.1128/IAI.00101-16
62. Wolowczuk I, Verwaerde C, Viltart O, Delanoye A, Delacre M, Pot B, et al. Feeding our immune system: impact on metabolism. Clin Dev Immunol. (2008) 2008:639803–03. doi: 10.1155/2008/639803
63. Coppens I. Targeting lipid biosynthesis and salvage in apicomplexan parasites for improved chemotherapies. Nat Rev Microbiol. (2013) 11:823–35. doi: 10.1038/nrmicro3139
64. Husson M-O, Ley D, Portal C, Gottrand M, Hueso T, Desseyn J-L, et al. Modulation of host defence against bacterial and viral infections by omega-3 polyunsaturated fatty acids. J Infect. (2016) 73:523–35. doi: 10.1016/j.jinf.2016.10.001
65. Sato S. Plasmodium—a brief introduction to the parasites causing human malaria and their basic biology. J Physiol Anthropol. (2021) 40:1. doi: 10.1186/s40101-020-00251-9
66. Mariki M, Mduma N, Mkoba E. Characterisation of malaria diagnosis data in high and low endemic areas of Tanzania. East Afr Health Res J. (2022) 6:171–79. doi: 10.24248/eahrj.v6i2.696
67. Organization WH. World malaria report 2020: 20 years of global progress and challenges. Geneva: World Health Organization; 2020. Licence: CC BY-NC-SA 3.0 IGO
68. H SR, T RK, S GK. StatPearls. Treasure Island (FL: StatPearls Publishing (2020). Apr 21. Antimalarial medications.
69. Organization WH. Guidelines for the treatment of malaria. 3rd ed. Geneva: World Health Organization (2015). Available at: https://www.afro.who.int/publications/guidelines-treatment-malaria-third-edition
70. Wang J, Zhang C-J, Chia WN, Loh CCY, Li Z, Lee YM, et al. Haem-activated promiscuous targeting of artemisinin in. Plasmodium falciparum Nat Commun. (2015) 6:10111. doi: 10.1038/ncomms10111
71. Tilley L, Straimer J, Gnädig NF, Ralph SA, Fidock DA. Artemisinin action and resistance in Plasmodium falciparum. Trends Parasitol. (2016) 32:682–96. doi: 10.1016/j.pt.2016.05.010
72. Combrinck JM, Mabotha TE, Ncokazi KK, Ambele MA, Taylor D, Smith PJ, et al. Insights into the role of heme in the mechanism of action of antimalarials. ACS Chem Biol. (2013) 8:133–37. doi: 10.1021/cb300454t
73. Eastman RT, Fidock DA. Artemisinin-based combination therapies: a vital tool in efforts to eliminate malaria. Nat Rev Microbiol. (2009) 7:864–74. doi: 10.1038/nrmicro2239
74. Cui L, Su XZ. Discovery, mechanisms of action and combination therapy of artemisinin. Expert Rev Anti Infect Ther. (2009) 7:999–1013. doi: 10.1586/eri.09.68
75. Srivastava IK, Vaidya AB. A mechanism for the synergistic antimalarial action of atovaquone and proguanil. Antimicrob Agents Chemother. (1999) 43:1334–9. doi: 10.1128/aac.43.6.1334
76. Hudson AT, Randall AW. Naphthoquinone derivatives. US5053432A. (1991). Available at: https://patents.google.com/patent/US5053432A/en
77. Russell PB, Hitchings GH. 2,4-diaminopyrimidines as antimalarials. III. 5-aryl derivatives. J Am Chem Soc. (1951) 73:3763–70. doi: 10.1021/ja01152a060
78. Lumb V, Das MK, Singh N, Dev V, Khan W, Sharma YD. Multiple origins of Plasmodium falciparum dihydropteroate synthetase mutant alleles associated with sulfadoxine resistance in India. Antimicrob Agents Chemother. (2011) 55:2813–17. doi: 10.1128/AAC.01151-10
79. Chen C, Tang L-H, Chun J. Studies on a new antimalarial compound: pyronaridine. Tran R Soc Trop Med Hyg. (1992) 86:7–10. doi: 10.1016/0035-9203(92)90414-8
80. Croft SL, Duparc S, Arbe-Barnes SJ, Craft JC, Shin C-S, Fleckenstein L, et al. Review of pyronaridine anti-malarial properties and product characteristics. Malar J. (2012) 11:270. doi: 10.1186/1475-2875-11-270
81. Ebstie YA, Abay SM, Tadesse WT, Ejigu DA. Tafenoquine and its potential in the treatment and relapse prevention of Plasmodium vivax malaria: the evidence to date. Drug Des Devel Ther. (2016) 10:2387–99. doi: 10.2147/dddt.s61443
82. Anderson M, Fritsche KL. (n-3) fatty acids and infectious disease resistance. J Nutr. (2002) 132:3566–76. doi: 10.1093/jn/132.12.3566
83. Kumaratilake LM, Robinson BS, Ferrante A, Poulos A. Antimalarial properties of n-3 and n-6 polyunsaturated fatty acids: in vitro effects on Plasmodium falciparum and in vivo effects on P. berghei. J Clin Invest. (1992) 89:961–7. doi: 10.1172/JCI115678
84. Guillaume C, Payré C, Jemel I, Jeammet L, Bezzine S, Naika GS, et al. In vitro anti-Plasmodium falciparum properties of the full set of human secreted phospholipases A2. (2015) 83:2453–65. doi: 10.1128/IAI.02474-14
85. Silva AR, Moraes BPT, Gonçalves-de-Albuquerque CF. Mediterranean diet: lipids, inflammation, and malaria infection. Int J Mol Sci. (2020) 21:4489. doi: 10.3390/ijms21124489
86. Blok WL, Vogels MTE, Curfs JHAJ, Eling WMC, Buurman WA, van der Meer JWM. Dietary fish-oil supplementation in experimental gram-negative infection and in cerebral malaria in mice. J Infect Dis. (1992) 165:898–903. doi: 10.1093/infdis/165.5.898
87. Berman JJ. Chapter 4 - eukaryotes. In: Berman JJ, editor. Taxonomic Guide to Infectious Diseases, 2nd ed. Academic Press (2019). p. 121–68. Available at: https://www.sciencedirect.com/book/9780128175767/taxonomic-guide-to-infectious-diseases
88. McDonald V, Shirley MW. Past and future: vaccination against. Eimeria. Parasitol. (2009) 136:1477–89. doi: 10.1017/s0031182009006349
89. M LR, F-C SH. Protozoal infections, in diseases of poultry. Saif YM, editor. Ames, Iowa, USA: Blackwell Publishing (2009). p. 1352.
90. Gussem MD. (2007). Coccidiosis in poultry: review on diagnosis, control, prevention and interaction with overall gut health, in: proceedings of the 16th European symposium on poultry nutrition, Strasbourg, France. pp. 253–61.
91. Allen PC, Fetterer RH. Recent advances in biology and immunobiology of Eimeria species and in diagnosis and control of infection with these coccidian parasites of poultry. Clin Microbiol Rev. (2002) 15:58–65. doi: 10.1093/ps/76.6.814
92. Williams RB. Fifty years of anticoccidial vaccines for poultry (1952-2002). Avian Dis. (2002) 46:775–802. doi: 10.1637/0005-2086(2002)046[0775:FYOAVF]2.0.CO;2
93. Sharman PA, Smith NC, Wallach MG, Katrib M. Chasing the golden egg: vaccination against poultry coccidiosis. Parasite Immunol. (2010) 32:590–8. doi: 10.1111/j.1365-3024.2010.01209.x
94. Abbas RZ, Iqbal Z, Blake D, Khan MN, Saleemi MK. Anticoccidial drug resistance in fowl coccidia: the state of play revisited. World's Poul Sci J. (2011) 67:337–50. doi: 10.1017/S004393391100033X
95. Levander OA, Fontela R, Morris VC, Ager AL. Protection against murine cerebral malaria by dietary-induced oxidative stress. J Parasitol. (1995) 81:99–103. doi: 10.2307/3284013
96. Allen PC, Danforth HD. Effects of dietary supplementation with n-3 fatty acid ethyl esters on coccidiosis in chickens. Poul Sci. (1998) 77:1631–35. doi: 10.1093/ps/77.11.1631
97. Allen PC. Production of free radical species during Eimeria maxima infections in chickens. Poul Sci. (1997) 76:814–21. doi: 10.1093/ps/76.6.814
98. Korver DR, Wakenell P, Klasing KC. Dietary fish oil or lofrin, a 5-lipoxygenase inhibitor, decrease the growth-suppressing effects of coccidiosis in broiler chicks. Poul Sci. (1997) 76:1355–63. doi: 10.1093/ps/76.10.1355
99. Repka D, Rangel HA, Atta AM, Gavino VA, Piedrabuena AE. Experimental Chagas' disease in mice infected with one LD50 of parasite. Rev Bras Biol. (1985) 45:309–16.
100. Marcondes MCG, Borelli P, Yoshida N, Russo M. Acute Trypanosoma cruzi infection is associated with anemia, thrombocytopenia, leukopenia, and bone marrow hypoplasia: reversal by nifurtimox treatment. Microbes Infect. (2000) 2:347–52. doi: 10.1016/s1286-4579(00)00333-6
101. DM A, C R, dS F, ET C, VR L, PF P. Involvement of nitric oxide (NO) and TNF-α in the oxidative stress associated with anemia in experimental Trypanosoma cruzi infection. FEMS Immunol Med Microbiol. (2004) 41:69–77. doi: 10.1016/j.femsim.2004.01.005
102. Dias LC, Dessoy MA, Silva JJN, Thiemann OH, Oliva G, Andricopulo AD. Quimioterapia da doença de Chagas: estado da arte e perspectivas no desenvolvimento de novos fármacos. Química Nova. (2009) 32:2444–57. doi: 10.1590/S0100-40422009000900038
103. Rodriques Coura J, de Castro SL. A critical review on Chagas disease chemotherapy. Mem Inst Oswaldo Cruz. (2002) 97:3–24. doi: 10.1590/s0074-02762002000100001
104. Sales Junior PA, Molina I, Fonseca Murta SM, Sánchez-Montalvá A, Salvador F, Corrêa-Oliveira R, et al. Experimental and clinical treatment of chagas disease: A review. Am J Trop Med Hyg. (2017) 97:1289–303. doi: 10.4269/ajtmh.16-0761
105. Packchanian A. Chemotherapy of experimental Chagas' disease with nitrofuran compounds. Antibiot Chemother (Northfield). (1957) 7:13–23.
106. Bermudez J, Davies C, Simonazzi A, Real JP, Palma S. Current drug therapy and pharmaceutical challenges for Chagas disease. Acta Trop. (2016) 156:1–16. doi: 10.1016/j.actatropica.2015.12.017
107. Maya JD, Cassels BK, Iturriaga-Vásquez P, Ferreira J, Faúndez M, Galanti N, et al. Mode of action of natural and synthetic drugs against Trypanosoma cruzi and their interaction with the mammalian host. Biochem Physiol. (2007) 146:601–20. doi: 10.1016/j.cbpa.2006.03.004
108. Field CJ, Johnson IR, Schley PD. Nutrients and their role in host resistance to infection. J Leukoc Biol. (2002) 71:16–32. doi: 10.1189/jlb.71.1.16
109. Fritsche K. Fatty acids as modulators of the immune response. Annu Rev Nutr. (2006) 26:45–73. doi: 10.1146/annurev.nutr.25.050304.092610
110. Calder PC. Omega-3 polyunsaturated fatty acids and inflammatory processes: nutrition or pharmacology? Br J Clin Pharmacol. (2013) 75:645–62. doi: 10.1111/j.1365-2125.2012.04374.x
111. Lovo-Martins MI, Malvezi AD, da Silva RV, Zanluqui NG, Tatakihara VLH, Câmara NOS, et al. Fish oil supplementation benefits the murine host during the acute phase of a parasitic infection from. Trypanosoma cruzi Nutr Res. (2017) 41:73–85. doi: 10.1016/j.nutres.2017.04.007
112. Pinge-Filho P, Tadokoro CE, Abrahamsohn IA. Prostaglandins mediate suppression of lymphocyte proliferation and cytokine synthesis in acute Trypanosoma cruzi infection. Cell Immunol. (1999) 193:90–8. doi: 10.1006/cimm.1999.1463
113. Jeffrey W, Lockman ADH. Recent developments in the identification of chemotherapeutics for chagas disease. Curr Med Chem. (2005) 12:945–59. doi: 10.2174/0929867053507289
114. Kiuchi F, Matsuo K, Itano Y, Ito M, Honda G, Qui TK, et al. Screening of natural medicine used in Vietnaam for trypanocidal activity against epi-amastigotes of Trypanosoma cruzi. Nat. Med. (2002) 56:64–8. F K, k M, al ATe.
115. Fasano E, Serini S, Cittadini A, Calviello G. Long-chain n-3 PUFA against breast and prostate cancer: Which are the appropriate doses for intervention studies in animals and humans? Crit Rev Food Sci Nutr. (2017) 57:2245–62. doi: 10.1080/10408398.2013.850060
116. Kumar N, Afjei R, Massoud TF, Paulmurugan R. Comparison of cell-based assays to quantify treatment effects of anticancer drugs identifies a new application for Bodipy-L-cystine to measure apoptosis. Sci Rep. (2018) 8:16363. doi: 10.1038/s41598-018-34696-x
117. Bougnoux P, Hajjaji N, Ferrasson MN, Giraudeau B, Couet C, Le Floch O. Improving outcome of chemotherapy of metastatic breast cancer by docosahexaenoic acid: a phase II trial. Br J Cancer. (2009) 101:1978–85. doi: 10.1038/sj.bjc.6605441
118. Yee LD, Lester JL, Cole RM, Richardson JR, Hsu JC, Li Y, et al. Omega-3 fatty acid supplements in women at high risk of breast cancer have dose-dependent effects on breast adipose tissue fatty acid composition. Am J Clin Nutr. (2010) 91:1185–94. doi: 10.3945/ajcn.2009.29036
119. Jung SW, Lee SW. The antibacterial effect of fatty acids on Helicobacter pylori infection. Korean J Intern Med. (2016) 31:30–5. doi: 10.3904/kjim.2016.31.1.30
Keywords: omega polyunsaturated fatty acids, prevention, parasitic infections, mechanism of action, supportive therapy
Citation: Rahman SU, Weng T-N, Qadeer A, Nawaz S, Ullah H and Chen C-C (2024) Omega-3 and omega-6 polyunsaturated fatty acids and their potential therapeutic role in protozoan infections. Front. Immunol. 15:1339470. doi: 10.3389/fimmu.2024.1339470
Received: 16 November 2023; Accepted: 21 March 2024;
Published: 03 April 2024.
Edited by:
Diana Bahia, Federal University of Minas Gerais, BrazilReviewed by:
Hussam Askar, Al Azhar University, EgyptSahibzada Waheed Abdullah, Chinese Academy of Agricultural Sciences, China
Copyright © 2024 Rahman, Weng, Qadeer, Nawaz, Ullah and Chen. This is an open-access article distributed under the terms of the Creative Commons Attribution License (CC BY). The use, distribution or reproduction in other forums is permitted, provided the original author(s) and the copyright owner(s) are credited and that the original publication in this journal is cited, in accordance with accepted academic practice. No use, distribution or reproduction is permitted which does not comply with these terms.
*Correspondence: Sajid Ur Rahman, ZHJfc2FqaWQyMjZAeWFob28uY29t; Chien-Chin Chen, aGxtYXJrY0BnbWFpbC5jb20=
†These authors have contributed equally to this work