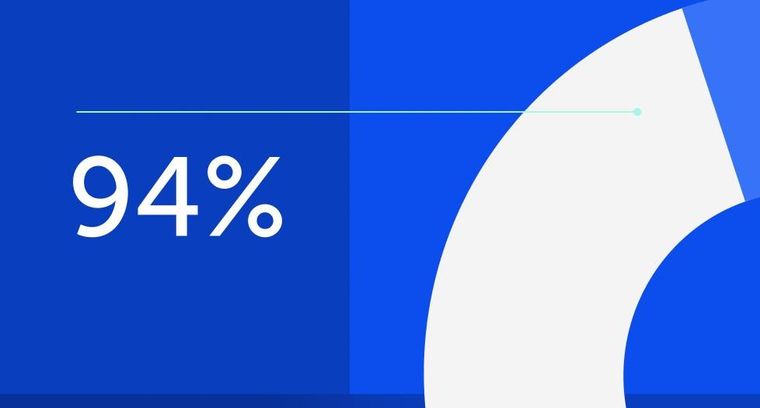
94% of researchers rate our articles as excellent or good
Learn more about the work of our research integrity team to safeguard the quality of each article we publish.
Find out more
REVIEW article
Front. Immunol., 28 February 2024
Sec. Cancer Immunity and Immunotherapy
Volume 15 - 2024 | https://doi.org/10.3389/fimmu.2024.1326753
This article is part of the Research TopicUpdates on the management of GlioblastomaView all 17 articles
The effectiveness of tumor therapy, especially immunotherapy and oncolytic virotherapy, critically depends on the activity of the host immune cells. However, various local and systemic mechanisms of immunosuppression operate in cancer patients. Tumor-associated immunosuppression involves deregulation of many components of immunity, including a decrease in the number of T lymphocytes (lymphopenia), an increase in the levels or ratios of circulating and tumor-infiltrating immunosuppressive subsets [e.g., macrophages, microglia, myeloid-derived suppressor cells (MDSCs), and regulatory T cells (Tregs)], as well as defective functions of subsets of antigen-presenting, helper and effector immune cell due to altered expression of various soluble and membrane proteins (receptors, costimulatory molecules, and cytokines). In this review, we specifically focus on data from patients with glioblastoma/glioma before standard chemoradiotherapy. We discuss glioblastoma-related immunosuppression at baseline and the prognostic significance of different subsets of circulating and tumor-infiltrating immune cells (lymphocytes, CD4+ and CD8+ T cells, Tregs, natural killer (NK) cells, neutrophils, macrophages, MDSCs, and dendritic cells), including neutrophil-to-lymphocyte ratio (NLR), focus on the immune landscape and prognostic significance of isocitrate dehydrogenase (IDH)-mutant gliomas, proneural, classical and mesenchymal molecular subtypes, and highlight the features of immune surveillance in the brain. All attempts to identify a reliable prognostic immune marker in glioblastoma tissue have led to contradictory results, which can be explained, among other things, by the unprecedented level of spatial heterogeneity of the immune infiltrate and the significant phenotypic diversity and (dys)functional states of immune subpopulations. High NLR is one of the most repeatedly confirmed independent prognostic factors for shorter overall survival in patients with glioblastoma and carcinoma, and its combination with other markers of the immune response or systemic inflammation significantly improves the accuracy of prediction; however, more prospective studies are needed to confirm the prognostic/predictive power of NLR. We call for the inclusion of dynamic assessment of NLR and other blood inflammatory markers (e.g., absolute/total lymphocyte count, platelet-to-lymphocyte ratio, lymphocyte-to-monocyte ratio, systemic immune-inflammation index, and systemic immune response index) in all neuro-oncology studies for rigorous evaluation and comparison of their individual and combinatorial prognostic/predictive significance and relative superiority.
In the United States, glioblastoma (diagnosed during 2013–2017) accounts for 48.6% (all ages combined) of all malignant brain and other central nervous system tumors, and five-year survival for patients diagnosed with glioblastoma in 2009–2015 is 7% (3–27%, varied by age) (1). Although the incidence of glioblastoma among primary brain tumors in different countries varies greatly (from ≈8.5% to 69%) (2, 3), five-year relative survival estimates are comparable and are <7% overall (4). The standard of care for the treatment of glioblastoma (maximal safe resection followed by radiotherapy with temozolomide chemotherapy) has not changed much since 2005 (Stupp protocol) (5, 6), and glioblastoma remains incurable (1) despite significant advances in our knowledge of its genetics and molecular biology over the past two decades.
The blood brain barrier is thought to be a key factor limiting the effectiveness of chemotherapy, including targeted agents, in the treatment of glioblastoma/glioma (7–9). Even temozolomide, with features such as 100% oral bioavailability, rapid absorption, excellent biodistribution, and ability to cross the blood-brain barrier because of its small size and lipophilic properties (10), reaches levels in tumor tissue that are only 20% of systemic drug levels (11). In 2016, of the ongoing 98 phase I/II and II glioma clinical trials, 63 studies (64.29%) were reported to include at least one drug able to pass the blood brain barrier (12). Unfortunately, according to systematic reviews and meta-analyses, almost all clinical trials involving targeted drugs and personalized chemotherapy in adult patients with glioblastoma have been unsuccessful (13–17). However, recent phase II trials (NCT02684058 and NCT04775485) found that dabrafenib plus trametinib could be an effective therapy as first-line treatment for pediatric patients with low-grade glioma with BRAF V600 mutations (18), and type II RAF inhibitor tovorafenib could be an effective therapy for BRAF-altered, relapsed/refractory pediatric low-grade glioma (19). Moreover, patients with IDH1 wild-type high-grade gliomas harboring BRAF or NF1 mutations and receiving trametinib monotherapy or in combination with dabrafenib had longer progression-free and overall survival than patients who did not receive genotype-matched targeted therapy (20).
Great hopes are currently placed on combination immunotherapy, including oncolytic virotherapy (16, 21–25). The immune system plays a primary role in the control of tumor development and the effectiveness of anticancer therapy (26–28). Historically, the brain has been considered an immune-privileged organ based on the lack of traditional lymphatic vessels in the brain and experiments with transplantation of foreign tissue into brain tissue and lack of rejection, as well as experiments with peripherally injected dyes that stain peripheral organs, but not the brain, due to the blood-brain barrier, restricting the access of macromolecules and cells into the brain parenchyma (29). Increasing evidence demonstrates that meningeal lymphatic vessels draining to the cervical lymph nodes play an important role in immune surveillance of the brain (30–32) and are essential for mounting an efficient immune response to brain tumors (33, 34). Various types of immune cells, including T cells and dendritic cells, were observed within meningeal lymphatics in both normal and pathological conditions (30–32). In mice with intracranial glioma or metastatic melanoma, dorsal meningeal lymphatic vessels were found to undergo extensive remodeling, and their specific pharmacochemical ablation impaired intratumoral fluid drainage, dendritic cell trafficking, and the efficacy of immunotherapy (33). Despite the partial disruption of the blood-brain barrier in glioblastoma (7), which promotes the infiltration of immune cells (25), nevertheless, human glioblastoma exhibits a predominantly “cold” (“immune-desert”/”immune-excluded”) phenotype, characterized by the absence or exclusion of T cells in the tumor microenvironment (33, 35) and T cell dysfunction, including tolerance and exhaustion (36, 37).
A tumor subdues the immune system, exerting both a local complex inhibitory effect on the tumor tissue microenvironment and systemic immunosuppression through the secretion of many soluble factors (38, 39). Profound immunosuppression and lymphopenia pose a challenge to current treatment strategies, including chemotherapy (38, 40, 41) and especially immunotherapy, the effectiveness of which may critically depend on the state of the patient’s immune system (41, 42). This review focuses on understanding the state of the immune system and the prognostic significance of different immune cell subtypes in patients with glioblastoma before standard therapy. In our accompanying review in Frontiers in Immunology (43), we comprehensively discuss the prognostic significance of standard therapy-related (iatrogenic) systemic immunosuppression and its implications for immunotherapy and oncolytic virotherapy. We provide compelling clinical data indicating that standard therapy affects various immune cell subsets, promoting tumor-related immune deficiency in patients with glioblastoma. Low post-treatment total lymphocyte count (TLC) is a prognostic factor for shorter survival in glioblastoma, and radiation-induced lymphopenia is a prognostic factor for mortality in virtually all solid cancers. Chemotherapy and corticosteroids may exacerbate radiation-induced lymphopenia. Dexamethasone use is a prognostic factor for shorter survival in glioblastoma. In addition, there is growing evidence that immunosuppression associated with standard therapy may be a barrier to immunotherapy, and lymphopenia is significantly associated with response and survival outcomes in patients with advanced cancer receiving immune checkpoint inhibitor therapy. Finally, we discuss how detailed blood and/or tumor immunophenotyping may be valuable for immunotherapy/oncolytic virotherapy research in terms of identifying new or validating the proposed immunological-based prognostic/predictive variables, and suggest what changes/interventions to the standard therapy paradigm should be considered to maintain lymphocytes counts. These reviews should help inform more rational clinical trial design and treatment decisions to potentially improve the effectiveness of immunotherapy/oncolytic virotherapy.
Analysis of peripheral blood obtained from patients with glioma showed shifts in the normal CD4+/CD8+ T cell ratio (from 2:1 closer to 1:1) (44–46). Among 300 chemoradiotherapy- and surgery/biopsy-naïve patients with glioblastoma (median age: 66; range: 21–91), lymphopenia (<1000 cells/µl) was present in 24.7% of patients (18.2% of steroid-naïve and 37.1% of steroid-experienced) (47). Deng et al. reported that 11.9% (out of n=469) of patients with glioblastoma (median age: 60.3; range: 19–94) had grade 3/4 lymphopenia (<500 cells/µl) preoperatively and 15.4% (out of n=628) postoperatively and before standard radiochemotherapy (48). In the elderly group (median age: 71 years), only 57% (out of n=72) of patients had normal baseline total lymphocyte counts (49). In another study, lymphopenia at baseline was detected in 24.3% (out of n=562) of elderly patients with glioblastoma (≥65 years) and was associated with worse overall survival (HR 1.30; 95% CI 1.05–1.62; p=0.02), regardless of O-6-methylguanine-DNA methyltransferase (MGMT) promoter methylation status (50). Similarly, studies reported that lymphopenia or absolute lymphocyte count at baseline in patients with glioblastoma were associated with worse overall survival in univariate and multivariate analysis, independent of the extent of resection, IDH mutation status, and adjuvant therapy (51, 52). However, absolute lymphocyte count at baseline was not correlated with overall survival in univariate or multivariate analysis of other studies (53–59).
In addition to baseline (preoperative/pretreatment) lymphopenia and shifts in the CD4+/CD8+ T cell ratio, it has been repeatedly documented that patients with glioma have decreased serum levels of Th1-type cytokines (IL-2, IL-6, IL-12, TNF-α, and IFN-γ) and increased serum levels of Th2-type cytokines (IL-4 and IL-10) (46, 60–64). Serum, cerebral spinal fluid, or tumor cyst fluid from patients with glioma may suppress the proliferation and/or function of lymphocytes and other immune cells from healthy donors (61, 63, 65, 66).
One of the contributors to systemic immunosuppression is CD4+CD25+FOXP3+ regulatory T cells (Tregs), which are involved in immune tolerance of tumors and compromise cytotoxic T cell function (67). In the majority of studies, elevated Treg fractions were documented among peripheral blood CD4+ T cells of patients with glioblastoma (60, 68–70), even in cases with severe CD4+ T cell lymphopenia (<200 cells/μL) and regardless of steroid use (70). Patients with elevated Treg fractions but not normal Treg fractions showed significant proliferative dysfunction of CD4+ T cells, reduced quantities of Th1-type cytokines, and increased quantities of Th2-type cytokines (70).
Another contributor to systemic immunosuppression is myeloid-derived suppressor cells (MDSCs), a heterogeneous population of early myeloid progenitors and precursor cells that can suppress immune responses mediated by CD4+ and CD8+ T cells (71). The number of circulating MDSCs is higher in patients with glioblastoma than in healthy donors or patients with low-grade gliomas (72–75).
In contrast to changes in the counts of T cell subsets, MDSCs, and neutrophils (discussed below), natural killer (NK) cells (45, 46, 76–78) and natural killer T (NKT) cells (79, 80) were within the normal range in patients with glioblastoma/glioma before standard therapy in the majority of studies, although their cytotoxic activity may have been impaired (44, 81, 82).
Neutrophils account for 50–70% of circulating leukocytes in humans (83). The neutrophil-to-lymphocyte ratio (NLR), derived from the absolute neutrophil and lymphocyte counts of a full blood count, is an easily accessible and measurable marker (84). Changes in the balance between neutrophils and lymphocytes reflect an increase in systemic inflammation and a decrease in anti-tumor adaptive immunity (84). Baseline NLR increases with glioma progression (grade I-IV glioma), with the highest NLR values observed in patients with grade IV gliomas, followed by grade III and grade I-II gliomas (85–92). In addition, in a retrospective study of adult patients with not otherwise specified subtype of glioblastoma (n=89), a weak positive association was found between tumor size and preoperative NLR values (Spearman r=0.3212, p=0.0493) (93). In another retrospective study of patients with glioma (n=64), higher pretreatment NLR was significantly associated with larger tumor diameter (p=0.02) (94). Similarly, a positive correlation between NLR and tumor size in patients with papillary thyroid carcinoma has been repeatedly documented (95). In carcinomas in general, NLR is higher in patients with more advanced or aggressive disease, as evidenced by increased tumor stage, nodal stage, and number of metastatic lesions (84). This correlation might be due to the fact that tumor cells secrete granulocyte colony-stimulating factor (G-CSF) and/or granulocyte-monocyte colony-stimulating factor (GM-CSF), which are not only direct growth factors for tumor cells but may also contribute to increased NLR in patients, shifting bone marrow hematopoiesis from the lymphocyte lineage toward the granulocyte lineage (96–98).
Any type of damage to brain tissue, including surgery- and therapy-related damage, tends to enhance G-CSF/GM-CSF synthesis (96). In addition, patients with glioblastoma treated with steroids have higher neutrophil counts and NLR (48, 54, 55, 78, 99, 100); however, no significant influence of steroid use on neutrophil counts (101) or only a weak correlation between dexamethasone dose and NLR (53, 57) were also reported. It is advisable to measure NLR prior to surgery or other treatments that may increase the neutrophil count. It should also be noted that NLR may change not only under the direct influence of tumor progression, surgery, or steroid treatment, but also of local or systemic infection; inflammatory diseases; thyroid, renal, or hepatic dysfunction; diabetes mellitus; heart diseases; hypertension; obesity; psychologic stress; and other complications in cancer patients (102, 103).
Regional anesthesia for patients with glioma has been proposed as a strategy to reduce postoperative systemic and local inflammatory responses (104). In a retrospective study of patients with glioblastoma (n=119) (104), local anesthesia of the nerves of the scalp during craniotomy, referred to as a “scalp block” (105), was shown to reduce postoperative NLR (104). This reduction was associated with longer median progression-free survival (16.7 versus 6.5 months for patients without a scalp block) (104). However, in another retrospective large cohort study (n=808), the use of a scalp block in glioma resection was not associated with improved progression-free and overall survival (106). Moreover, the use of different anesthetics, including isoflurane, desflurane, and propofol, during glioblastoma surgery is not associated with overall survival (107, 108).
In retrospective studies, lower neutrophil counts before radiochemotherapy were associated with better overall survival (n=164, n=369, and n=2002) (55, 57, 99) independent of steroid use (55). Higher neutrophil counts at relapse were also prognostic for worse overall survival, but only in patients who did not receive bevacizumab (109). However, there are studies that report no prognostic role for neutrophil counts in patients with glioblastoma (52, 56, 58, 59).
Higher NLR was a significant prognostic factor for shorter progression-free survival in some glioblastoma/glioma retrospective studies (110–113) but not in others (51, 54, 114–116) on univariate and/or multivariate analyses. In large meta-analyses, higher NLR in patients with carcinomas was a significant prognostic factor of cancer-specific, progression-free and/or disease-free survival (117, 118).
High baseline (preoperative/pretreatment) NLR was established as an independent predictor of shorter overall survival in patients with glioblastoma/gliomas (Table 1) (51, 52, 54, 56–59, 85, 90–92, 112, 114, 119–128), and this was confirmed by meta-analyses (131–133). However, the independent prognostic significance of NLR remains debatable (55). High NLR during standard therapy was also associated with worse overall survival regardless of steroid use in multivariate analysis (48), and a decline of NLR during or post-therapy was associated with longer overall survival of patients with glioblastoma in multivariate analyses in prospective (53) and retrospective studies (57, 119, 134). NLR was prognostic in patients with recurrent glioblastoma (119, 135). However, in some glioblastoma studies, baseline NLR was not correlated with overall survival (114, 136), or correlated with overall survival in univariate analysis but not in multivariate analysis (55, 56, 85, 127), or vice versa (121). Similarly, postoperative NLR was not correlated with overall survival in either univariate (56, 58) or multivariate analyses (57, 115, 127). It should also be noted that assessment of dynamic changes of NLR (e.g., preoperative, pre-treatment, during and/or post-treatment) may provide more accurate prognostication or prediction of response to therapy in glioma (53, 57) and carcinoma (137–144).
In the majority of glioblastoma/glioma studies (Table 1), high NLR was established as an independent factor for worse outcomes without including MGMT promoter methylation status, steroid use, IDH mutation status, or other prognostic variables in multivariate analyses. Nevertheless, baseline NLR was still prognostically independent of MGMT promoter methylation status (58, 129), IDH mutation status (51, 92, 121), and steroid use (122, 124) in patients with glioblastoma, and patients with increased NLR and requiring steroids had the poorest outcomes (73). Moreover, in a recent prospective glioma study (n=73, 37% with grade III astrocytoma and 63% with grade IV glioma), patients were divided into four groups based on the median baseline NLR and NLR decrease during chemoradiotherapy (53). Patients with baseline NLR <3.5 with NLR decrease during treatment (n=14), baseline NLR <3.5 without NLR decrease during treatment (n=23), baseline NLR ≥3.5 with NLR decrease during treatment (n=24), and baseline NLR ≥3.5 without NLR decrease during treatment (n=12) had median overall survival of 36.5, 19.2, 14.7 and 7.1 months, respectively (53). On univariate analysis, patients with baseline NLR <3.5 and NLR decrease during treatment had lower mortality risk than those with baseline NLR ≥3.5 (HR 0.512; 95% CI 0.291–0.904) or no decrease during treatment (HR 0.519; 95% CI 0.293–0.918) (53). Moreover, NLR decrease during treatment was a significant predictor of overall survival on multivariate analysis [HR 0.380; 95% CI 0.18–0.80)] after adjustment for age, ECOG performance status, extent of resection, IDH mutation status, grade IV tumor, and baseline and time-weighted mean dexamethasone dose (53).
Table 1 High NLR is an independent prognostic factor for overall survival in patients with glioblastoma/gliomas.
Systematic reviews and meta-analyses have provided evidence that high baseline neutrophil count or high NLR were independently associated with adverse overall survival in various types of solid tumors (84, 117, 118, 145–151), while normalization of post-treatment NLR was associated with improved survival (84). Moreover, systematic reviews and/or meta-analyses examining the correlation between NLR and outcomes in patients treated with immune checkpoint inhibitors have reported that higher NLR is a prognostic factor of worse disease control rate, objective response rate, progression-free survival, and/or overall survival in patients with head and neck squamous cell carcinoma (152, 153), gastric carcinoma (154, 155), melanoma (156), metastatic renal cell carcinoma (157, 158), non-small cell lung carcinoma (159), hepatocellular carcinoma (160) and cancer patients in general (161–163). High post-treatment NLR has also been associated with poor survival outcomes in cancer patients treated with immune checkpoint inhibitors (162). Combining NLR assessment with other biomarkers of response to immune checkpoint inhibitors, such as PD-L1 expression, tumor mutation burden, or lymphocyte infiltration, has been shown to provide additional predictive power in identifying patients who respond to treatment (164–169). Finally, in meta-analyses, lower baseline NLR was significantly associated with immune-related adverse events (irAEs) resulting from the use of immune checkpoint inhibitors in cancer patients (170, 171).
There is no standardized cutoff for the prognostic/predictive NLR value. An NLR value ≥4 was associated with poorer overall survival in the majority of the studies of patients with glioblastoma/gliomas with statistically meaningful sample sizes (Table 1). Of note, in a meta-analysis of 75 eligible studies covering more than 20 cancer types, the median cutoff for high NLR with the strongest prognostic effect was 4.0 (range 1.9–7.2) (117), whereas in a prospective study in an unselected general population (individuals aged 45 years, n=8711), the reference NLR value (mean and 95% intervals) was 1.76 (0.83-3.92) (172).
Neutrophils are plastic populations of immune cells with different functions (173). In rodent models, the immunosuppressive neutrophil populations may promote tumor progression by potentiating tumor invasion, angiogenesis, and metastasis (173). In patients with cancer, the proportion of immunosuppressive neutrophils is dramatically increased (173–175). Neutrophils can suppress the activation and proliferation of cytotoxic T cells (173–176). The predominance of immunosuppressive neutrophils over lymphocytes, which demonstrate the disproportion of the CD4+/CD8+/Treg ratio, provides a clue as to why NLR is a prognostic marker of worse survival across many solid tumor types.
It is worth noting that adult glioblastoma/gliomas in general differ significantly from pediatric gliomas. An overview of the composition of the tumor immune infiltrate across different types of pediatric glioma is given elsewhere (177).
Glioblastoma tissue is abundantly infiltrated by neutrophils (178). Fossati et al. found a strong correlation between glioma tumor grade, the extent of neutrophil infiltration, and the preoperative circulating neutrophil counts (101). Over 70% of all glioma samples analyzed (n=105) showed significant neutrophil infiltration (40–50% of low grade gliomas and 87% of glioblastomas) (101). In another study, neutrophil infiltration was observed in 86% of II-IV glioma samples (n=232), and the level of neutrophil infiltration was significantly correlated with glioma grade (179). Increased neutrophil infiltration was associated with shorter overall survival in patients with glioblastoma (n=152) (58). Tumor-infiltrating neutrophils are an independent prognostic factor for overall survival across different tumor types (146, 180).
Tumor-associated macrophages and microglia are the dominant population of immune cells in the glioblastoma microenvironment, and their heterogeneity and plasticity are discussed extensively elsewhere (181). Microglia/macrophages comprise of ≈10–50% of the glioblastoma mass (182–186). Both M1- and M2-like microglia/macrophages (differentiated by pro-inflammatory and anti-inflammatory polarization/phenotype states, respectively) have been detected in human gliomas (72, 187–194). However, it should be noted that the M1/M2 dichotomy is oversimplified. MDSCs also infiltrate glioblastoma (73, 74, 195, 196). Detailed flow cytometry analysis revealed that MDSCs, microglia, and macrophages constituted approximately 40%, 40%, and 20% of the glioblastoma mass, respectively, and that glioblastoma-associated myeloid cells presented a continuum between the M1- and M2-like phenotypes, with closer alignment to the non-polarized M0 macrophage phenotype (72).
Ionized calcium-binding adaptor molecule-1 (IBA-1) is a pan-marker for all microglia and macrophages. High IBA-1 intensity was correlated with longer survival (193). However, in another study, the number of IBA+ cells was positively correlated with the overall tumor size and edema but not with overall survival (72). CD204+ (scavenger receptor) (193) or CD163+ (scavenger receptor) (194) M2-like microglia/macrophage density was correlated with worse survival, whereas lower expression of CD163 and higher expression of CCL3 (C-C Motif Chemokine Ligand 3), an M1 marker, was correlated with better survival (192). In contrast, Zeiner et al. found that high levels of CD68+ (a pan-macrophage marker), CD206+ (mannose receptor C type 1), and CD163+ tumor-infiltrating macrophage subpopulations in the vital tumor core of patients with IDH1R132H-non-mutant glioblastoma (n=241) were associated with improved survival (187). Finally, Karimi et al. revealed that increased levels of MPO+CD163−P2Y12−CD68+ macrophages were associated with prolonged survival of patients with glioblastoma (184).
NK cells and invariant NKT cells are scarcely present in glioblastoma/glioma tissue (60, 183, 184), and the role of these immune cell subtypes has not been clearly established in patients with glioblastoma/glioma. Nevertheless, there is increasing evidence of NK or NKT cell-based immunotherapy efficacy in rodent glioma models (197, 198). In addition, a local administration of activated haploidentical NK cells (199) or irradiated CAR-NK cells (NK-92/5.28. z) targeting HER2 (200) in patients with recurrent glioblastoma was feasible and safe.
There are plasmacytoid dendritic cells, type 1 and type 2 classical dendritic cells, monocyte-derived dendritic cells, and a new dendritic cell subset, DC3 (201). The heterogeneity and functionality of the dendritic cell compartment in patients with glioblastoma (subsets, counts, and functionality) are poorly characterized and reviewed elsewhere (202).
The density of tumor-infiltrating CD4+, CD8+, and Tregs increases with glioma grade (203–206), with higher levels of infiltrated CD4+ cells than CD8+ cells (203, 207). However, Innocenti et al. reported no difference between CD4+ and CD8+ cell counts in glioblastoma tissue samples (n=59) (208). In general, tumor-infiltrating lymphocytes are differentially distributed in glioblastoma samples, from absent to abundant (183, 209–211). T cell infiltrates are mainly located in the perivascular areas and zones of tumor invasion into the surrounding brain parenchyma and are only infrequently found within the tumor tissue and in the perinecrotic areas (211–215). The percentages of immunological synapses established by tumor-infiltrating lymphocytes with tumor cells are very low (213), and the tumor-infiltrating lymphocytes have a suppressed and functionally impaired state/phenotype (described as tolerance and exhaustion) (36, 37, 60, 69, 216, 217). These observations indicate that although the density of infiltrated lymphocytes varies considerably between patients, tumor-infiltrating lymphocytes cannot readily migrate into the immunosuppressive tumor microenvironment, are mainly arrested in the perivascular or peritumoral space, and are functionally compromised.
There is no clear association between tumor-infiltrating lymphocytes (CD3+, CD4+, or CD8+ T cell infiltrates) and overall survival in patients with glioblastoma/glioma (Table 2) (58, 60, 203, 205, 208–210, 218–230). In addition, based on the FOXP3+ phenotype alone, there was no correlation between overall survival and Treg infiltration in univariate and multivariate analyses of most studies (203–205, 225, 226, 231–233). In meta-analyses, inconsistent correlations between different tumor-infiltrating lymphocyte subsets and overall survival have also been reported for other types of solid cancer (234–243). Cytotoxic CD8+ T cells and memory CD45RO+ T cells are strongly correlated with good outcomes in most cancer types, whereas the prognostic value of Th2, Th17, Tregs, MDSCs, macrophages, and NK populations is inconsistent and varies depending on the cancer type, stage, or study (234–243).
Table 2 Correlation between the density of tumor-infiltrating lymphocytes and overall survival of patients with glioblastoma/gliomas.
The inconsistent correlations between tumor-infiltrating lymphocytes and survival (Table 2) may stem from substantial functional and phenotypic complexity and plasticity of CD4+ and CD8+ T cell subsets discovered by high-dimensional single-cell mass cytometry (cytometry by time-of-flight, CyTOF) (244). Detailed qualitative and quantitative assessments of tumor immune infiltrates using mass cytometry to measure the frequencies and ratios of immune cell subsets and their functional and activation status may be required for accurate prognostication (184, 245–247). Distinct subsets of T cells are anti-inflammatory, pro-inflammatory, or both, and a dual role in cancer immunity has been ascribed to CD3+CD4+ cell subsets (246). For example, in follicular B-cell lymphomas, 12 subsets of intratumoral CD4+ T cells were identified, and specific subpopulations were correlated with poor or improved patient survival (246). In patients with clear cell renal cell carcinoma, 22 subsets were identified among infiltrating T cell lymphocytes, and a distinct immune composition correlated with survival (247). In addition, different prognoses may be obtained depending on the degree of infiltration of immunosuppressive FOXP3(high) Tregs or non-suppressive FOXP3(low) cells (248). For example, colorectal carcinomas with abundantly infiltrated FOXP3(low) T cells demonstrated a much better prognosis than tumors with predominant FOXP3(high) Treg infiltration (248).
It is also evident that not only the density of tumor-infiltrating lymphocytes but also the ratio between immunosuppressive immune cell subsets and cytotoxic lymphocytes have an effect on prognostic significance. In some studies, increased CD8+/CD4+, CD4+/CD8+, CD3+/Treg, or CD8+/Treg ratios, rather than the absolute counts of individual populations, were correlated with survival outcomes in patients with glioblastoma/glioma (203, 229, 232). Furthermore, the immunosuppressive cellular immune landscape in the glioblastoma/glioma microenvironment goes beyond well-defined immune cell subtypes such as Tregs, neutrophils, MDSCs, and tumor-associated macrophages (215, 249). Li et al. revealed that immunosuppressive CD3+CD4+FOXP3− type 1 regulatory T cells occurred at high frequencies within glioblastoma tissue (249). In addition, Waziri et al. found that a great portion of CD3+ T cells within glioblastoma tissue was represented by CD3+CD4+CD56+ T cells (215). However, these T cells did not represent classical invariant NKT cells as they were neither stained with antibodies against an invariant TCR Vβ24 nor with CD1d tetramer loaded with α-Gal-Cer (215). These CD3+CD4+CD56+ T cells might be immunosuppressive, since immunosuppressive CD3+CD4+CD56+CD25+FOXP3+ T cells were identified at a high frequency in hepatocellular carcinoma, and higher infiltration of these cells was inversely correlated with survival (250).
A lack of standardization and recommendations for assessing tumor-infiltrating lymphocytes (technical and methodological differences associated with staining and analysis, including high inter‐observer variability) in glioma tissue, as well as non-uniform patient cohorts, are potential factors contributing to inconsistent correlations between tumor infiltrating lymphocytes and overall survival (Table 2). It is known that pre-analytical variables such as tissue collection, fixation (time, buffer composition, and temperature), processing (dehydration reagents, temperature, and paraffin embedding), storage, staining (manual versus automated, quality, and quantity of antibodies), and other conditions may affect the accurate assessment of CD3+, CD4+, and CD8+ lymphocytes within a tissue (251, 252). Moreover, since the immune infiltrate and individual subpopulations of immune cells are heterogeneously distributed in glioblastoma tissue (e.g., central areas versus marginal areas; perivascular areas versus perinecrotic areas), histochemical sections of different tumor regions will give different insights into immune cell density and diversity. New high‐throughput multiplex immunohistochemistry and immunofluorescence technologies are expanding the ability to obtain additional information on cellular composition and spatial arrangement with greater reproducibility using standardized quantitative protocols (253). Therefore, in-depth spatial immunophenotyping of glioblastoma tissue, together with consensus guidelines for the assessment of glioma-infiltrating lymphocytes, is required to more accurately establish the prognostic power of infiltrating lymphocytes. In general, this also applies to subsets of myeloid cells (254, 255).
Gliomas with IDH mutation exhibit a unique immune landscape due to the role of the oncometabolite R-2-hydroxyglutarate (2-HG) in glioma immune evasion (256–258). The infiltration of CD3+ (259), CD4+ (260), CD8+ (260, 261) T lymphocytes, Tregs (262), monocyte-derived macrophages (263), neutrophils (260), overall CD45+ immune cells, including macrophages, dendritic cells, and T cells (264) was reduced in IDH-mutant gliomas compared with IDH-wild type gliomas. However, another study documented a higher relative abundance of dendritic cells and CD8+ T lymphocytes in IDH-mutant gliomas compared with IDH-wild type gliomas (265). The activity of tumor-infiltrating immune subsets may be significantly compromised by R-2-hydroxyglutarate, which was shown to impair monocyte differentiation into dendritic cells and dendritic cell functionality (265), proliferative potential and effector functions of T cells (266–269), and NK cell-mediated killing (270), promote an immunosuppressive phenotype of macrophages (271) and reduce intratumoral vasculature density (272). Due to a distinct immune microenvironment, including distorted immune cell infiltration and impaired immune cell activity, IDH-mutant gliomas may be potentially more resistant to immunotherapy. Small-molecule IDH inhibitors may reverse R-2-hydroxyglutarate mediated immune suppression and sensitize IDH-mutant gliomas to immunotherapy (256, 273, 274). Moreover, in a double-blind phase III trial in patients with grade 2 IDH-mutant glioma (NCT04164901), vorasidenib, an inhibitor of mutant IDH1/2 enzymes, was shown to significantly improve progression-free survival compared with the placebo group (median progression-free survival, 27.7 versus 11.1 months) (275).
In 2006, using unsupervised expression profiling on a cohort of 76 grade III and IV astrocytoma samples to classify tumors into prognostic groups, Philips et al. defined subtypes based on a 35-gene signature termed proneural, mesenchymal, and proliferative (276). The proneural subtype, containing the majority of grade III astrocytoma samples, was distinguished by markedly better prognosis compared with other subtypes (276). In 2010, Verhaak et al. described a gene expression-based molecular classification (840-gene set classifier) of glioblastoma samples into the proneural, neural, classical, and mesenchymal subtypes (77% subtype concordance) (277). Somatic mutations and/or DNA copy number aberrations/overexpression of EGFR, NF1, and PDGFRA/IDH1 predominantly defined the classical, mesenchymal, and proneural subtypes, respectively. MGMT promoter methylation status was not associated with subtypes (277). In meta-analyses, IDH mutations are associated with better progression-free and overall survival in patients with glioma (278–280). Surprisingly, overall survival of patients with the proneural subtype was not significantly different from other gene expression subtypes defined according to the classification of Verhaak et al. (281, 282). However, Noushmehr et al. found the glioma cytosine-phosphate-guanine (CpG) island methylator phenotype (G-CIMP) (281). Tumors with this phenotype were predominantly of the proneural subtype and were strongly associated with IDH1 mutations, and proneural G-CIMP-positive patients had significantly better survival than proneural G-CIMP-negative patients or all other non-proneural patients in univariate and multivariate analysis (281). Later, Turcan et al. (283) and Brennan et al. (284) confirmed that the survival advantage of the proneural subtype was conferred by G-CIMP status, strongly associated with IDH-mutant gliomas, with proneural non-G-CIMP and other subtypes demonstrating similar and less favorable outcomes. Further studies showed that IDH-mutant G-CIMP-positive tumors with 1p/19q codel status were associated with better overall survival than IDH-mutant G-CIMP-positive tumors with 1p/19q non-codel status (285, 286), and G-CIMP-low non-codel subgroup, based on the extent of DNA methylation, had poorer outcome compared with G-CIMP-high non-codel subgroup in IDH-mutant gliomas (287–290). Finally, by combining epigenetic signature and gene copy number variations, Li et al. separated IDH-mutant glioblastoma into G-CIMP-high group and G-CIMP-low group without CDKN2A and MET alteration with favorable and comparable overall survival, while G-CIMP-low group with CDKN2A and/or MET alteration showed significantly shorter overall survival in univariate and multivariate analysis (290). Currently, the combination of these parameters allows for improved prediction of outcome (291).
In 2017, applying unsupervised transcriptome analysis after filtering glioblastoma overexpressed genes to 369 IDH-wild type glioblastoma samples, Wang et al. confirmed three subtypes previously designated mesenchymal, proneural, and classical (≥93% subtype concordance; defined 50-gene signatures for each subtype with overlap with Verhaak et al.’s 840-gene set ranging from 42% to 54%), while the neural phenotype was suggested to be non-tumor specific (292). In addition, strong associations between subtypes and genomic abnormalities in previously reported subtype-defining genes were also confirmed (292). Again, patient survival did not differ significantly between the three subtypes (292). In 2019, using a 500-gene set classifier (only 108 genes matched Verhaak et al.’s 840-gene set) on six different datasets (three TCGA-cohorts and three Asian-cohorts), Teo et al. also confirmed the classical, mesenchymal, and proneural subtypes, with similar survival outcomes between subtypes (293). Finally, in 2023, White et al. also found no significant difference in overall survival between subtypes in the GLIOTRAIN (n=123), TCGA (n=164), and CGGA (n=693) cohorts after stratifying the mesenchymal, proneural, and classical subtypes according to Wang et al.’ report (294). Interestingly, the authors introduced novel glioblastoma tumor microenvironment subtypes for IDH wild type glioblastoma (TMEHigh, TMEMed, and TMELow), characterized by significantly different expression of genes specific to all immune and endothelial cell markers. However, stratification into these new subtypes showed no association with overall survival in the GLIOTRAIN, TCGA, CGGA, and DUKE cohorts (294).
Using The Cancer Genome Atlas (TCGA) database, Doucette et al. analyzed the mRNA expression levels of immune system genes (cytokines, cell markers, and signaling pathways) between four glioblastoma subtypes and found that the mesenchymal subtype was the most proinflammatory due to the preferential enrichment of both pro-inflammatory and immunosuppressive genes compared with other subtypes (295). Rutledge et al., using histochemical analysis, found that tumor-infiltrating lymphocytes were enriched in the mesenchymal subtype and strongly associated with mutations in NF1 and RB1, while lymphocytes were depleted in the classical subtype, EGFR-amplified, and homozygous PTEN-deleted tumors. However, no association with survival was found (209). Wang et al. documented the increased presence of macrophages/microglia and neuroglial cells in mesenchymal subtype, and NF1 deactivation was associated with macrophage/microglia recruitment (292). The activated natural killer cell gene signature was significantly reduced in the mesenchymal subtype, the resting memory CD4+ T cell gene signature was significantly reduced in the proneural subtype, and the activated dendritic cell gene signature was significantly greater in the classical subtype (292). In an immunohistochemical study of the immune infiltrate of the four glioblastoma subtypes, Martinez-Lage et al. found that the mesenchymal and proneural subtypes were the most and least immunogenic, respectively (194). The percentage of CD3+, CD4+, and CD5+ lymphocytes differed significantly between the mesenchymal and proneural or classical subtypes but not other subtypes. The percentage of CD8+ did not differ between the four subtypes. The percentage of CD163+ and CD68+ macrophages/microglia in the mesenchymal subtype was also significantly different compared with the classical or proneural subtypes, with no difference between them (194). In another immunohistological study of three glioblastoma subtypes defined according to the classification of Wang et al., the numbers of IBA1+ microglia/macrophage cells and CD3+ and FOXP3+ lymphocytes were significantly higher in the mesenchymal subtype compared with other subtypes, with no significant difference between the proneural and classical subtypes (296). The number of CD8+ differed significantly only between the mesenchymal and proneural subtypes (296). On the contrary, Han et al. reported that the number of CD4+, CD8+, and FoxP3+ lymphocytes did not vary greatly between the subtypes defined according to the classification of Verhaak et al. (203). Finally, using flow cytometry, Gabrusiewicz et al. found that the frequency of MDSCs, microglia, and macrophages in the proneural and neural subtypes was not significantly different; the classical subtype had a markedly higher percentage of MDSCs than macrophages, whereas the mesenchymal subtype was predominantly infiltrated with microglia (72).
Taken together, glioma/glioblastoma transcriptome subtypes in different studies were defined using different numbers of only partially overlapping gene sets, and in each case the subtypes (except for the classification of Philips et al.) did not differ prognostically unless at least proneural G-CIMP status was not taken into account, despite the fact that the proneural subtype is characterized by the presence of prognostic IDH mutations much more often than other subtypes, on the one hand, and the existence of the greatest immunological difference between the proneural/IDH mutant and mesenchymal subtypes, on the other hand. These observations might explain why stratification of glioblastoma patients based on the transcriptomic subtypes has not translated into clinical practice. Considering the enormous inter- and intratumoral cellular heterogeneity and plasticity, as well as the evolution of the cancer genome and phenotype, clinically meaningful subtyping of glioblastoma based on the transcriptome alone is challenging. However, there is some retrospective clinical evidence that mesenchymal or TMEHigh glioblastoma might respond better to immunotherapy (vaccine, checkpoint inhibitor or oncolytic virus) (294, 297).
There is growing interest in evaluating neoadjuvant immunotherapy in neuro-oncology (298–300). Neoadjuvant immunotherapy is advantageous over adjuvant immunotherapy, since it is applied before lymphotoxic standard radio-/chemotherapy (43). However, in patients with glioblastoma/glioma before standard therapy, tumor-related immunosuppression involves deregulation of many components of immunity, including changes in expression of different soluble and membrane proteins, reduced T lymphocyte counts (lymphopenia), increased NLR, increased levels or ratios of circulating and tumor-infiltrating immunosuppressive immune subsets (e.g., macrophages, MDSCs, and Tregs), and defective functions of antigen-presenting, helper, and effector immune cell subsets due to altered expression of receptors, costimulatory molecules, and cytokines (Figure 1). In this condition, the effectiveness of neoadjuvant immunotherapy for glioblastoma/glioma might be suboptimal.
Figure 1 Glioblastoma/glioma-related systemic and local immunosuppression. Before standard therapy, patients may have reduced counts of circulating T lymphocytes (lymphopenia); however, in the majority of studies, the increased fractions of regulatory T cells (Tregs) are documented. The numbers of circulating myeloid-derived suppressor cells (MDSCs) and neutrophils are higher in patients with glioblastoma than in healthy donors or patients with low-grade gliomas. The counts of circulating natural killer (NK) and NKT cells are within the normal range in the majority of studies. The density of tumor-infiltrating CD4+, CD8+, and Tregs increases with glioma grade; however, lymphocytes are differentially distributed in tissue samples, from absent to abundant. Glioblastoma tissue is abundantly infiltrated by neutrophils and macrophages, while NK cells and NKT cells are scarcely present. Serum levels of Th1-type cytokines (IL-2, IL-6, IL-12, TNF-α, and IFN-γ) are reduced, while serum levels of Th2-type cytokines (IL-4 and IL-10) are increased. Serum, cerebral spinal fluid, or tumor cyst fluid from patients can suppress the proliferation and/or function of lymphocytes and other immune cells from healthy donors. Consequently, patients have defective functions of antigen-presenting, helper, and effector immune cell subsets due to altered expression of different soluble and membrane proteins (receptors, costimulatory molecules, and cytokines). The tumor-infiltrating lymphocytes have suppressed and functionally impaired state/phenotype. The Figure was generated using Servier Medical Art, provided by Servier, licensed under a Creative Commons Attribution 3.0 unported license.
The spatial complexity and phenotypic diversity of the immune infiltrate (multicellular spatial organization) in patients with glioblastoma/gliomas at presentation, as well as the prognostic and/or predictive significance of immune cell subsets/signatures remain poorly characterized and largely unknown (255, 301, 302). As discussed above, the accurate prognostic assessment of immune infiltrate by traditional immunochistochemistry is influenced by too many analytical variables and factors. Although multidimensional single-cell approaches such as multiplexed ion beam imaging, imaging mass cytometry, and mass cytometry has become powerful tools for characterizing different immune cell subsets in immunology and identifying specific immune signatures associated with survival and response to immunotherapy in cancer patients (184, 245–247, 303–306) and may also help better decipher immune deregulation in patients with glioblastoma/gliomas and identify putative predictive or prognostic markers of therapeutic response and improved survival, but at present they should still be considered discovery tools in preclinical research and clinical trials rather than for routine clinical practice (303–305, 307–312). It is also worth considering the factor of the availability and quality of tissue for assessing spatially distributed immune infiltrate in glioblastoma. Moreover, in various reports, approximately 15-20% of cases present as unresectable glioblastoma (diagnostic “biopsy-only”) (313). However, the actual number of such patients may be higher. In the National Cancer Database, the percentage of patients with unresectable glioblastoma among patients diagnosed from 2004 to 2013 with known survival and extent of resection is 28.5% (314). Kole et al. documented that among 1325 patients with biopsy-only glioblastoma who received radiochemotherapy, the median overall survival was 9.2 months (313), and Harlay et al. reported that among 139 patients who underwent biopsy only, 54 (39%) and 68 (49%) were amenable to standard radiochemotherapy or chemotherapy only, with the median overall survival of 14 months (95% CI, 9.65-18.71) and 8 months (95% CI, 4.62-7.67), respectively (315). This relatively large group of patients is particularly in need of new treatment strategies and should not be ignored.
What is needed is a cost-effective, easily accessible and repeatedly validated prognostic and predictive factor, available to all patients, that can be dynamically assessed (at baseline, during and/or after treatment) and rapidly integrated into all ongoing and planned clinical studies of various forms of therapy in all clinical centers around the world. In general, immune-related biomarkers derived from blood rather than tumor tissue may be more suitable in terms of these requirements. NLR has prognostic value in patients with solid tumors across cancer types, stages of disease, and treatment strategies, including immunotherapy. However, although NLR has often been correlated with the effectiveness of immune checkpoint inhibitors in carcinomas, NLR has only rarely been taken into account when evaluating the effectiveness of vaccines, oncolytic viruses, and other immunotherapies in cancer patients. For patients with glioblastoma, this may also be due to the fact that the vast majority of clinical trials of all forms of therapy in general are non-randomized and/or uncontrolled (316, 317). It is also important to note that evaluation of the prognostic/predictive significance of NLR has been largely limited to retrospective studies, with very few of the studies based on prospectively collected samples [e.g (318–320)], including glioblastoma/glioma (53, 112). Since the number of prospective studies evaluating NLR in oncology is limited, NLR has not yet entered routine clinical practice as a stratification/predictive factor.
The prognostic/predictive power of NLR may be further improved by combining NLR assessment with other biomarkers. According to recent meta-analyses and systematic reviews, absolute/total lymphocyte count (ALC/TLC, especially post-treatment) (321), platelet-to-lymphocyte ratio (PLR) (133, 322), lymphocyte-to-monocyte ratio (LMR) (323), systemic immune-inflammation index (SII), calculated by platelet count×neutrophil count/lymphocyte count (129, 324), and systemic immune response index (SIRI), calculated by neutrophil count×monocyte/lymphocyte count (325–327) are the emerging prognostic factors in glioblastoma/glioma. In a retrospective study, Yang et al. developed and compared the SII-NLR, SII-PLR, and NLR-PLR, and SII-NLR-PLR scoring systems and found that the combination of these inflammatory markers demonstrated greater predictive accuracy for overall survival at one and two years than any single indicator in patients with glioblastoma (n=208), with the best scoring system being SII-NLR (129). The authors constructed a nomogram including age, Karnofsky Performance Status (KPS), extent of resection, MGMT promoter methylation status, chemoradiotherapy, and SII-NLR score to predict 2-year survival in patients with glioblastoma (the c-index of the nomogram was 0.848 (95% CI 0.836–0.861) and 0.843 (95% CI 0.830–0.855) excluding MGMT promoter methylation status) (129).
Finding methods to reduce NLR is another important area of research in clinical oncology. In patients with recurrent glioblastoma (n=18), treatment with recombinant interleukin-7 restored and maintained total lymphocyte counts without serious toxicity and irrespective of steroid and temozolomide use (328). Interleukin-7 is currently considered the most potent therapeutic candidate for the treatment of lymphopenia in cancer and non-cancer patients (41). As we have discussed, NLR may be affected by many physiological and pathological confounding factors, including psychological/emotional stress in cancer patients. It is known that cortisol and epinephrine may increase neutrophil and decrease lymphocyte counts (329–331). In a randomized trial of lung cancer patients (n=80), psychological intervention was shown to significantly reduce NLR compared with usual care (332).
Glioma progression and molecular characteristics (e.g., IDH mutations or mesenchymal gene signature) have distinct effects on major immune cell subsets, and conversely, different proportions of immune cell subsets and their polarization or activation states may have different effects on tumor progression, response to therapy, and survival. All attempts to identify a reliable prognostic immune marker in glioblastoma tissue have led to markedly contradictory results, which can be explained, among other things, by the unprecedented level of spatial heterogeneity of the immune infiltrate and the significant phenotypic and functional diversity of immune subpopulations. High NLR has been repeatedly established as an independent prognostic factor for shorter overall survival in patients with glioblastoma and carcinomas, and its combination with other markers of the immune response significantly improves the accuracy of prediction; however, more prospective studies are needed to confirm the prognostic/predictive power of NLR. We call for incorporating the dynamic assessment of NLR and other emerging blood inflammatory markers (e.g., platelet-to-lymphocyte ratio, lymphocyte-to-monocyte ratio, systemic immune-inflammation index, and systemic immune response index) into all neuro-oncology trials to carefully evaluate and compare their individual and combinatorial prognostic/predictive significance and relative superiority.
AAS: Conceptualization, Funding acquisition, Investigation, Writing – original draft, Writing – review & editing. AOS: Writing – review & editing. MPV: Writing – review & editing. AAC: Writing – review & editing. OVA: Writing – review & editing. KAP: Writing – review & editing. VPC: Writing – review & editing, Supervision.
The author(s) declare financial support was received for the research, authorship, and/or publication of this article. The work was financially supported by the Russian Science Foundation (RSF), grant no. 22-75-10087. The funder had no role in data collection and analysis, decision to publish, or preparation of the manuscript.
The authors declare that the research was conducted in the absence of any commercial or financial relationships that could be construed as a potential conflict of interest.
All claims expressed in this article are solely those of the authors and do not necessarily represent those of their affiliated organizations, or those of the publisher, the editors and the reviewers. Any product that may be evaluated in this article, or claim that may be made by its manufacturer, is not guaranteed or endorsed by the publisher.
1. Miller KD, Ostrom QT, Kruchko C, Patil N, Tihan T, Cioffi G, et al. Brain and other central nervous system tumor statistics, 2021. CA Cancer J Clin. (2021) 71:381–406. doi: 10.3322/caac.21693
2. Xiao D, Yan C, Li D, Xi T, Liu X, Zhu D, et al. National Brain Tumour Registry of China (NBTRC) statistical report of primary brain tumours diagnosed in China in years 2019-2020. Lancet Reg Heal West Pacific. (2023) 34:100715. doi: 10.1016/j.lanwpc.2023.100715
3. Girardi F, Rous B, Stiller CA, Gatta G, Fersht N, Storm HH, et al. The histology of brain tumors for 67 331 children and 671 085 adults diagnosed in 60 countries during 2000-2014: A global, population-based study (CONCORD-3). Neuro Oncol. (2021) 23:1765–76. doi: 10.1093/neuonc/noab067
4. Walker EV, Zhou Y, Wu Y, Liu J, Climans SA, Davis FG, et al. The incidence and prevalence of primary central nervous system (CNS) tumours in Canada (2010–2017), and the survival of patients diagnosed with CNS tumours (2008–2017). Curr Oncol. (2023) 30:4311–28. doi: 10.3390/curroncol30040329
5. Stupp R, Mason WP, van den Bent MJ, Weller M, Fisher B, Taphoorn MJB, et al. Radiotherapy plus concomitant and adjuvant temozolomide for glioblastoma. N Engl J Med. (2005) 352:987–96. doi: 10.1056/NEJMoa043330
6. Stupp R, Hegi ME, Mason WP, van den Bent MJ, Taphoorn MJB, Janzer RC, et al. Effects of radiotherapy with concomitant and adjuvant temozolomide versus radiotherapy alone on survival in glioblastoma in a randomised phase III study: 5-year analysis of the EORTC-NCIC trial. Lancet Oncol. (2009) 10:459–66. doi: 10.1016/S1470-2045(09)70025-7
7. Sarkaria JN, Hu LS, Parney IF, Pafundi DH, Brinkmann DH, Laack NN, et al. Is the blood–brain barrier really disrupted in all glioblastomas? A critical assessment of existing clinical data. Neuro Oncol. (2018) 20:184–91. doi: 10.1093/neuonc/nox175
8. Wu D, Chen Q, Chen X, Han F, Chen Z, Wang Y. The blood–brain barrier: Structure, regulation, and drug delivery. Signal Transduct Target Ther. (2023) 8:217. doi: 10.1038/s41392-023-01481-w
9. Heffron TP. Small molecule kinase inhibitors for the treatment of brain cancer. J Med Chem. (2016) 59:10030–66. doi: 10.1021/acs.jmedchem.6b00618
10. Moody CL, Wheelhouse RT. The medicinal chemistry of imidazotetrazine prodrugs. Pharm (Basel). (2014) 7:797–838. doi: 10.3390/ph7070797
11. Stepanenko AA, Chekhonin VP. On the critical issues in temozolomide research in glioblastoma: Clinically relevant concentrations and MGMT-independent resistance. Biomedicines. (2019) 7. doi: 10.3390/biomedicines7040092
12. Guishard AF, Yakisich JS, Azad N, Iyer AKV. Translational gap in ongoing clinical trials for glioma. J Clin Neurosci. (2018) 47:28–42. doi: 10.1016/j.jocn.2017.10.001
13. Mowforth OD, Brannigan J, El Khoury M, Sarathi CIP, Bestwick H, Bhatti F, et al. Personalised therapeutic approaches to glioblastoma: A systematic review. Front Med. (2023) 10:1166104. doi: 10.3389/fmed.2023.1166104
14. Fazzari FGT, Rose F, Pauls M, Guay E, Ibrahim MFK, Basulaiman B, et al. The current landscape of systemic therapy for recurrent glioblastoma: A systematic review of randomized-controlled trials. Crit Rev Oncol Hematol. (2022) 169:103540. doi: 10.1016/j.critrevonc.2021.103540
15. He X, Zhao W, Huang J, Xu J, Niu S, Zhang Q, et al. Characteristics and trends of globally registered glioma clinical trials in the past 16 years. Ther Adv Neurol Disord. (2022) 15:17562864221114356. doi: 10.1177/17562864221114355
16. Stepanenko AA, Chekhonin VP. Recent advances in oncolytic virotherapy and immunotherapy for glioblastoma: A glimmer of hope in the search for an effective therapy? Cancers (Basel). (2018) 10:492. doi: 10.3390/cancers10120492
17. Scherm A, Ippen FM, Hau P, Baurecht H, Wick W, Gempt J, et al. Targeted therapies in patients with newly diagnosed glioblastoma—A systematic meta-analysis of randomized clinical trials. Int J Cancer. (2023) 152:2373–82. doi: 10.1002/ijc.34433
18. Bouffet E, Hansford JR, Garrè ML, Hara J, Plant-Fox A, Aerts I, et al. Dabrafenib plus trametinib in pediatric glioma with BRAF V600 mutations. N Engl J Med. (2023) 389:1108–20. doi: 10.1056/NEJMoa2303815
19. Kilburn LB, Khuong-Quang D-A, Hansford JR, Landi D, van der Lugt J, Leary SES, et al. The type II RAF inhibitor tovorafenib in relapsed/refractory pediatric low-grade glioma: the phase 2 FIREFLY-1 trial. Nat Med. (2024) 30(1):207–17. doi: 10.1038/s41591-023-02668-y
20. Ghanem P, Fatteh M, Kamson DO, Balan A, Chang M, Tao J, et al. Druggable genomic landscapes of high-grade gliomas. Front Med. (2023) 10:1254955. doi: 10.3389/fmed.2023.1254955
21. Hervás-Corpión I, Alonso MM. Oncolytic viruses as treatment for adult and pediatric high-grade gliomas: On the way to clinical success. Int Rev Cell Mol Biol. (2023) 379:169–88. doi: 10.1016/bs.ircmb.2023.04.001
22. Singh K, Batich KA, Wen PY, Tan AC, Bagley SJ, Lim M, et al. Designing clinical trials for combination immunotherapy: A framework for glioblastoma. Clin Cancer Res. (2022) 28:585–93. doi: 10.1158/1078-0432.CCR-21-2681
23. Andersen BM, Reardon DA. Immunotherapy approaches for adult glioma: knowledge gained from recent clinical trials. Curr Opin Neurol. (2022) 35:803–13. doi: 10.1097/WCO.0000000000001118
24. Agosti E, Zeppieri M, De Maria L, Tedeschi C, Fontanella MM, Panciani PP, et al. Glioblastoma immunotherapy: A systematic review of the present strategies and prospects for advancements. Int J Mol Sci. (2023) 24:15037. doi: 10.3390/ijms242015037
25. Jain P, Vashist S, Panjiyar BK. Navigating the immune challenge in glioblastoma: Exploring immunotherapeutic avenues for overcoming immune suppression. Cureus. (2023) 15(9):e46089. doi: 10.7759/cureus.46089
26. Thol K, Pawlik P, McGranahan N. Therapy sculpts the complex interplay between cancer and the immune system during tumour evolution. Genome Med. (2022) 14:137. doi: 10.1186/s13073-022-01138-3
27. Candeias SM, Gaipl US. The immune system in cancer prevention, development and therapy. Anticancer Agents Med Chem. (2015) 16:101–7. doi: 10.2174/1871520615666150824153523
28. Gonzalez H, Hagerling C, Werb Z. Roles of the immune system in cancer: from tumor initiation to metastatic progression. Genes Dev. (2018) 32:1267–84. doi: 10.1101/gad.314617.118
29. Habashy KJ, Mansour R, Moussalem C, Sawaya R, Massaad MJ. Challenges in glioblastoma immunotherapy: mechanisms of resistance and therapeutic approaches to overcome them. Br J Cancer. (2022) 127:976–87. doi: 10.1038/s41416-022-01864-w
30. Melloni A, Liu L, Kashinath V, Abdi R, Shah K. Meningeal lymphatics and their role in CNS disorder treatment: moving past misconceptions. Front Neurosci. (2023) 17:1184049. doi: 10.3389/fnins.2023.1184049
31. Laaker C, Baenen C, Kovács KG, Sandor M, Fabry Z. Immune cells as messengers from the CNS to the periphery: The role of the meningeal lymphatic system in immune cell migration from the CNS. Front Immunol. (2023) 14:1233908. doi: 10.3389/fimmu.2023.1233908
32. Tavares GA, Louveau A. Meningeal lymphatics: An immune gateway for the central nervous system. Cells. (2021) 10:3385. doi: 10.3390/cells10123385
33. Hu X, Deng Q, Ma L, Li Q, Chen Y, Liao Y, et al. Meningeal lymphatic vessels regulate brain tumor drainage and immunity. Cell Res. (2020) 30:229–43. doi: 10.1038/s41422-020-0287-8
34. Song E, Mao T, Dong H, Boisserand LSB, Antila S, Bosenberg M, et al. VEGF-C-driven lymphatic drainage enables immunosurveillance of brain tumours. Nature. (2020) 577:689–94. doi: 10.1038/s41586-019-1912-x
35. Thorsson V, Gibbs DL, Brown SD, Wolf D, Bortone DS, Ou Yang T-H, et al. The immune landscape of cancer. Immunity. (2018) 48:812–830.e14. doi: 10.1016/j.immuni.2018.03.023
36. Woroniecka KI, Rhodin KE, Chongsathidkiet P, Keith KA, Fecci PE. T-cell dysfunction in glioblastoma: Applying a new framework. Clin Cancer Res. (2018) 24:3792–802. doi: 10.1158/1078-0432.CCR-18-0047
37. Watowich MB, Gilbert MR, Larion M. T cell exhaustion in Malignant gliomas. Trends Cancer. (2023) 9:270–92. doi: 10.1016/j.trecan.2022.12.008
38. Himes BT, Geiger PA, Ayasoufi K, Bhargav AG, Brown DA, Parney IF. Immunosuppression in glioblastoma: Current understanding and therapeutic implications. Front Oncol. (2021) 11:770561. doi: 10.3389/fonc.2021.770561
39. Grabowski MM, Sankey EW, Ryan KJ, Chongsathidkiet P, Lorrey SJ, Wilkinson DS, et al. Immune suppression in gliomas. J Neurooncol. (2021) 151:3–12. doi: 10.1007/s11060-020-03483-y
40. Galluzzi L, Buqué A, Kepp O, Zitvogel L, Kroemer G. Immunological effects of conventional chemotherapy and targeted anticancer agents. Cancer Cell. (2015) 28:690–714. doi: 10.1016/j.ccell.2015.10.012
41. Ménétrier-Caux C, Ray-Coquard I, Blay J-Y, Caux C. Lymphopenia in Cancer Patients and its Effects on Response to Immunotherapy: An opportunity for combination with Cytokines? J Immunother Cancer. (2019) 7:85. doi: 10.1186/s40425-019-0549-5
42. Wang R, Xiong K, Wang Z, Wu D, Hu B, Ruan J, et al. Immunodiagnosis — the promise of personalized immunotherapy. Front Immunol. (2023) 14:1216901. doi: 10.3389/fimmu.2023.1216901
43. Stepanenko AA, Sosnovtseva AO, Valikhov MP, Chernysheva AA, Abramova OV, Naumenko VA, et al. The need for paradigm shift: prognostic significance and implications of standard therapy-related systemic immunosuppression in glioblastoma for immunotherapy and oncolytic virotherapy. Front Immunol. (2024) 15:1326757. doi: 10.3389/fimmu.2024.1326757
44. Peraçoli MT, Montelli TC, Soares AM, Parise-Fortes MR, Alquati SA, Ueda A, et al. Immunological alterations in patients with primary tumors in central nervous system. Arq Neuropsiquiatr. (1999) 57:539–46. doi: 10.1590/s0004-282x1999000400001
45. Bhondeley MK, Mehra RD, Mehra NK, Mohapatra AK, Tandon PN, Roy S, et al. Imbalances in T cell subpopulations in human gliomas. J Neurosurg. (1988) 68:589–93. doi: 10.3171/jns.1988.68.4.0589
46. Gousias K, Markou M, Arzoglou V, Voulgaris S, Vartholomatos G, Kostoula A, et al. Frequent abnormalities of the immune system in gliomas and correlation with the WHO grading system of Malignancy. J Neuroimmunol. (2010) 226:136–42. doi: 10.1016/j.jneuroim.2010.05.027
47. Chongsathidkiet P, Jackson C, Koyama S, Loebel F, Cui X, Farber SH, et al. Sequestration of T cells in bone marrow in the setting of glioblastoma and other intracranial tumors. Nat Med. (2018) 24:1459–68. doi: 10.1038/s41591-018-0135-2
48. Deng D, Hammoudeh L, Youssef G, Chen Y-H, Shin K-Y, Lim-Fat MJ, et al. Evaluating hematologic parameters in newly diagnosed and recurrent glioblastoma: Prognostic utility and clinical trial implications of myelosuppression. Neuro-oncology Adv. (2023) 5:vdad083. doi: 10.1093/noajnl/vdad083
49. Mendez JS, Govindan A, Leong J, Gao F, Huang J, Campian JL. Association between treatment-related lymphopenia and overall survival in elderly patients with newly diagnosed glioblastoma. J Neurooncol. (2016) 127:329–35. doi: 10.1007/s11060-015-2037-1
50. Song AJ, Ding K, Alnahhas I, Laperriere NJ, Perry J, Mason WP, et al. Impact of lymphopenia on survival for elderly patients with glioblastoma: A secondary analysis of the CCTG CE.6 (EORTC 26062-22061, TROG03.01) randomized clinical trial. Neuro-oncology Adv. (2021) 3:vdab153. doi: 10.1093/noajnl/vdab153
51. Marini A, Dobran M, Aiudi D, Pesaresi A, di Somma LGM, Iacoangeli M. Pre-operative hematological markers as predictive factors for overall survival and progression free survival in glioblastomas. Clin Neurol Neurosurg. (2020) 197:106162. doi: 10.1016/j.clineuro.2020.106162
52. Gan Y, Zhou X, Niu X, Li J, Wang T, Zhang H, et al. Neutrophil-lymphocyte ratio (NLR) is an independent prognostic factor in the elderly patients with high-grade gliomas. World Neurosurg. (2019) 127:e261–7. doi: 10.1016/j.wneu.2019.03.085
53. Sharma P, Medhi P, Bhattacharyya M, Nath J, Kalita A. Prognostic significance of neutrophil-lymphocyte ratio in patients of high-grade glioma undergoing adjuvant chemoradiation: A prospective study. Asian Pacific J Cancer Prev. (2023) 24:3487–94. doi: 10.31557/APJCP.2023.24.10.3487
54. Hsu EJ, Thomas J, Maher EA, Youssef M, Timmerman RD, Wardak Z, et al. Neutrophilia and post-radiation thrombocytopenia predict for poor prognosis in radiation-treated glioma patients. Front Oncol. (2022) 12:1000280. doi: 10.3389/fonc.2022.1000280
55. Le Rhun E, Oppong FB, Vanlancker M, Stupp R, Nabors B, Chinot O, et al. Prognostic significance of therapy-induced myelosuppression in newly diagnosed glioblastoma. Neuro Oncol. (2022) 24:1533–45. doi: 10.1093/neuonc/noac070
56. Lopes M, Carvalho B, Vaz R, Linhares P. Influence of neutrophil-lymphocyte ratio in prognosis of glioblastoma multiforme. J Neurooncol. (2018) 136:173–80. doi: 10.1007/s11060-017-2641-3
57. Mason M, Maurice C, McNamara MG, Tieu MT, Lwin Z, Millar B-A, et al. Neutrophil–lymphocyte ratio dynamics during concurrent chemo-radiotherapy for glioblastoma is an independent predictor for overall survival. J Neurooncol. (2017) 132:463–71. doi: 10.1007/s11060-017-2395-y
58. Han S, Liu Y, Li Q, Li Z, Hou H, Wu A. Pre-treatment neutrophil-to-lymphocyte ratio is associated with neutrophil and T-cell infiltration and predicts clinical outcome in patients with glioblastoma. BMC Cancer. (2015) 15:617. doi: 10.1186/s12885-015-1629-7
59. Bambury RM, Teo MY, Power DG, Yusuf A, Murray S, Battley JE, et al. The association of pre-treatment neutrophil to lymphocyte ratio with overall survival in patients with glioblastoma multiforme. J Neurooncol. (2013) 114:149–54. doi: 10.1007/s11060-013-1164-9
60. Kmiecik J, Poli A, Brons NHC, Waha A, Eide GE, Enger PØ, et al. Elevated CD3+ and CD8+ tumor-infiltrating immune cells correlate with prolonged survival in glioblastoma patients despite integrated immunosuppressive mechanisms in the tumor microenvironment and at the systemic level. J Neuroimmunol. (2013) 264:71–83. doi: 10.1016/j.jneuroim.2013.08.013
61. Dix AR, Brooks WH, Roszman TL, Morford LA. Immune defects observed in patients with primary Malignant brain tumors. J Neuroimmunol. (1999) 100:216–32. doi: 10.1016/s0165-5728(99)00203-9
62. Kumar R, Kamdar D, Madden L, Hills C, Crooks D, O’Brien D, et al. Th1/Th2 cytokine imbalance in meningioma, anaplastic astrocytoma and glioblastoma multiforme patients. Oncol Rep. (2006) 15:1513–6. doi: 10.3892/or.15.6.1513
63. Harshyne LA, Nasca BJ, Kenyon LC, Andrews DW, Hooper DC. Serum exosomes and cytokines promote a T-helper cell type 2 environment in the peripheral blood of glioblastoma patients. Neuro Oncol. (2016) 18:206–15. doi: 10.1093/neuonc/nov107
64. Albulescu R, Codrici E, Popescu ID, Mihai S, Necula LG, Petrescu D, et al. Cytokine patterns in brain tumour progression. Mediators Inflammation. (2013) 2013:979748. doi: 10.1155/2013/979748
65. Avril T, Vauleon E, Tanguy-Royer S, Mosser J, Quillien V. Mechanisms of immunomodulation in human glioblastoma. Immunotherapy. (2011) 3:42–4. doi: 10.2217/imt.11.39
66. Domenis R, Cesselli D, Toffoletto B, Bourkoula E, Caponnetto F, Manini I, et al. Systemic T cells immunosuppression of glioma stem cell-derived exosomes is mediated by monocytic myeloid-derived suppressor cells. PloS One. (2017) 12:e0169932. doi: 10.1371/journal.pone.0169932
67. Shan F, Somasundaram A, Bruno TC, Workman CJ, Vignali DAA. Therapeutic targeting of regulatory T cells in cancer. Trends Cancer. (2022) 8:944–61. doi: 10.1016/j.trecan.2022.06.008
68. Crane CA, Ahn BJ, Han SJ, Parsa AT. Soluble factors secreted by glioblastoma cell lines facilitate recruitment, survival, and expansion of regulatory T cells: implications for immunotherapy. Neuro Oncol. (2012) 14:584–95. doi: 10.1093/neuonc/nos014
69. Mohme M, Schliffke S, Maire CL, Rünger A, Glau L, Mende KC, et al. Immunophenotyping of newly diagnosed and recurrent glioblastoma defines distinct immune exhaustion profiles in peripheral and tumor-infiltrating lymphocytes. Clin Cancer Res. (2018) 24(17):4187–200. doi: 10.1158/1078-0432.CCR-17-2617
70. Fecci PE, Mitchell DA, Whitesides JF, Xie W, Friedman AH, Archer GE, et al. Increased regulatory T-cell fraction amidst a diminished CD4 compartment explains cellular immune defects in patients with Malignant glioma. Cancer Res. (2006) 66:3294–302. doi: 10.1158/0008-5472.CAN-05-3773
71. Gao J, Liu W-R, Tang Z, Fan J, Shi Y-H. Myeloid-derived suppressor cells in cancer. iLIVER. (2022) 1:81–9. doi: 10.1016/j.iliver.2022.06.002
72. Gabrusiewicz K, Rodriguez B, Wei J, Hashimoto Y, Healy LM, Maiti SN, et al. Glioblastoma-infiltrated innate immune cells resemble M0 macrophage phenotype. JCI Insight. (2016) 1. doi: 10.1172/jci.insight.85841
73. Gielen PR, Schulte BM, Kers-Rebel ED, Verrijp K, Petersen-Baltussen HMJM, ter Laan M, et al. Increase in both CD14-positive and CD15-positive myeloid-derived suppressor cell subpopulations in the blood of patients with glioma but predominance of CD15-positive myeloid-derived suppressor cells in glioma tissue. J Neuropathol Exp Neurol. (2015) 74:390–400. doi: 10.1097/NEN.0000000000000183
74. Raychaudhuri B, Rayman P, Ireland J, Ko J, Rini B, Borden EC, et al. Myeloid-derived suppressor cell accumulation and function in patients with newly diagnosed glioblastoma. Neuro Oncol. (2011) 13:591–9. doi: 10.1093/neuonc/nor042
75. Rodrigues JC, Gonzalez GC, Zhang L, Ibrahim G, Kelly JJ, Gustafson MP, et al. Normal human monocytes exposed to glioma cells acquire myeloid-derived suppressor cell-like properties. Neuro Oncol. (2010) 12:351–65. doi: 10.1093/neuonc/nop023
76. Rapp M, Özcan Z, Steiger H-J, Wernet P, Sabel MC, Sorg RV. Cellular immunity of patients with Malignant glioma: prerequisites for dendritic cell vaccination immunotherapy. J Neurosurg. (2006) 105:41–50. doi: 10.3171/jns.2006.105.1.41
77. Gustafson MP, Lin Y, New KC, Bulur PA, O’Neill BP, Gastineau DA, et al. Systemic immune suppression in glioblastoma: the interplay between CD14+HLA-DRlo/neg monocytes, tumor factors, and dexamethasone. Neuro Oncol. (2010) 12:631–44. doi: 10.1093/neuonc/noq001
78. Chitadze G, Flüh C, Quabius ES, Freitag-Wolf S, Peters C, Lettau M, et al. In-depth immunophenotyping of patients with glioblastoma multiforme: Impact of steroid treatment. Oncoimmunology. (2017) 6:e1358839. doi: 10.1080/2162402X.2017.1358839
79. Hunn MK, Farrand KJ, Broadley KWR, Weinkove R, Ferguson P, Miller RJ, et al. Vaccination with irradiated tumor cells pulsed with an adjuvant that stimulates NKT cells is an effective treatment for glioma. Clin Cancer Res. (2012) 18:6446–59. doi: 10.1158/1078-0432.CCR-12-0704
80. Dhodapkar KM, Cirignano B, Chamian F, Zagzag D, Miller DC, Finlay JL, et al. Invariant natural killer T cells are preserved in patients with glioma and exhibit antitumor lytic activity following dendritic cell-mediated expansion. Int J Cancer. (2004) 109:893–9. doi: 10.1002/ijc.20050
81. Kong D-S, Nam D-H, Kang S-H, Lee JW, Chang J-H, Kim J-H, et al. Phase III randomized trial of autologous cytokine-induced killer cell immunotherapy for newly diagnosed glioblastoma in korea. Oncotarget. (2017) 8:7003–13. doi: 10.18632/oncotarget.12273
82. Lee C-C, You J-F, Wang Y-C, Lan S-W, Wei K-C, Chen K-T, et al. Gross total resection promotes subsequent recovery and further enhancement of impaired natural killer cell activity in glioblastoma patients. Brain Sci. (2022) 12:1144. doi: 10.3390/brainsci12091144
83. Massara M, Persico P, Bonavita O, Mollica Poeta V, Locati M, Simonelli M, et al. Neutrophils in gliomas. Front Immunol. (2017) 8:1349. doi: 10.3389/fimmu.2017.01349
84. Guthrie GJK, Charles KA, Roxburgh CSD, Horgan PG, McMillan DC, Clarke SJ. The systemic inflammation-based neutrophil-lymphocyte ratio: Experience in patients with cancer. Crit Rev Oncol Hematol. (2013) 88:218–30. doi: 10.1016/j.critrevonc.2013.03.010
85. Auezova R, Ryskeldiev N, Doskaliyev A, Kuanyshev Y, Zhetpisbaev B, Aldiyarova N, et al. Association of preoperative levels of selected blood inflammatory markers with prognosis in gliomas. Onco Targets Ther. (2016) 9:6111–7. doi: 10.2147/OTT.S113606
86. Zadora P, Dabrowski W, Czarko K, Smolen A, Kotlinska-Hasiec E, Wiorkowski K, et al. Preoperative neutrophil–lymphocyte count ratio helps predict the grade of glial tumor – A pilot study. Neurol Neurochir Pol. (2015) 49:41–4. doi: 10.1016/j.pjnns.2014.12.006
87. Zheng S-H, Huang J, Chen M, Wang B-L, Ou Q-S, Huang S-Y. Diagnostic value of preoperative inflammatory markers in patients with glioma: A multicenter cohort study. J Neurosurg. (2018) 129(3):583–92. doi: 10.3171/2017.3.JNS161648
88. Wang P-F, Meng Z, Song H-W, Yao K, Duan Z-J, Yu C-J, et al. Preoperative changes in hematological markers and predictors of glioma grade and survival. Front Pharmacol. (2018) 9:886. doi: 10.3389/fphar.2018.00886
89. Wilson JRF, Saeed F, Tyagi AK, Goodden JR, Sivakumar G, Crimmins D, et al. Pre-operative neutrophil count and neutrophil-lymphocyte count ratio (NLCR) in predicting the histological grade of paediatric brain tumours: A preliminary study. Acta Neurochir (Wien). (2018) 160(4):793–800. doi: 10.1007/s00701-017-3388-5
90. Wang J, Xiao W, Chen W, Hu Y. Prognostic significance of preoperative neutrophil-to-lymphocyte ratio and platelet-to-lymphocyte ratio in patients with glioma. EXCLI J. (2018) 17:505–12. doi: 10.17179/excli2017-978
91. Yang T, Mao P, Chen X, Niu X, Xu G, Bai X, et al. Inflammatory biomarkers in prognostic analysis for patients with glioma and the establishment of a nomogram. Oncol Lett. (2019) 17:2516–22. doi: 10.3892/ol.2018.9870
92. Weng W, Chen X, Gong S, Guo L, Zhang X. Preoperative neutrophil–lymphocyte ratio correlated with glioma grading and glioblastoma survival. Neurol Res. (2018) 40:917–22. doi: 10.1080/01616412.2018.1497271
93. Serban GM, Tamas CI, Tamas F, Balasa AF. Preoperative immune-inflammatory status of the patients with newly-diagnosed glioblastoma – could it genuinely predict their survival? Cureus. (2023) 15(8):e43802. doi: 10.7759/cureus.43802
94. Chim ST, Sanfilippo P, O’Brien TJ, Drummond KJ, Monif M. Pretreatment neutrophil-to-lymphocyte/monocyte-to-lymphocyte ratio as prognostic biomarkers in glioma patients. J Neuroimmunol. (2021) 361:577754. doi: 10.1016/j.jneuroim.2021.577754
95. Goksu M, Alakus H. Relationship between tumor size and neutrophil–lymphocyte ratio in patients with papillary thyroid carcinoma. Cerrahpasa Med J. (2023) 47:19–22. doi: 10.5152/cjm.2022.21062
96. Kast RE, Hill QA, Wion D, Mellstedt H, Focosi D, Karpel-Massler G, et al. Glioblastoma-synthesized G-CSF and GM-CSF contribute to growth and immunosuppression: Potential therapeutic benefit from dapsone, fenofibrate, and ribavirin. Tumor Biol. (2017) 39:101042831769979. doi: 10.1177/1010428317699797
97. He K, Liu X, Hoffman RD, Shi R, Lv G, Gao J. G-CSF / GM-CSF -induced hematopoietic dysregulation in the progression of solid tumors. FEBS Open Bio. (2022) 12:1268–85. doi: 10.1002/2211-5463.13445
98. Kumar A, Taghi Khani A, Sanchez Ortiz A, Swaminathan S. GM-CSF: A double-edged sword in cancer immunotherapy. Front Immunol. (2022) 13:901277. doi: 10.3389/fimmu.2022.901277
99. Schernberg A, Nivet A, Dhermain F, Ammari S, Escande A, Pallud J, et al. Neutrophilia as a biomarker for overall survival in newly diagnosed high-grade glioma patients undergoing chemoradiation. Clin Transl Radiat Oncol. (2018) 10:47–52. doi: 10.1016/j.ctro.2018.04.002
100. Vaios EJ, Nahed BV, Muzikansky A, Fathi AT, Dietrich J. Bone marrow response as a potential biomarker of outcomes in glioblastoma patients. J Neurosurg. (2017) 127:132–8. doi: 10.3171/2016.7.JNS16609
101. Fossati G, Ricevuti G, Edwards SW, Walker C, Dalton A, Rossi ML. Neutrophil infiltration into human gliomas. Acta Neuropathol. (1999) 98:349–54. doi: 10.1007/s004010051093
102. Balta S, Demirkol S, Ozturk C. The neutrophil lymphocyte ratio in patients with glioblastoma multiforme. J Neurooncol. (2014) 117:195–6. doi: 10.1007/s11060-013-1355-4
103. Buonacera A, Stancanelli B, Colaci M, Malatino L. Neutrophil to lymphocyte ratio: an emerging marker of the relationships between the immune system and diseases. Int J Mol Sci. (2022) 23:3636. doi: 10.3390/ijms23073636
104. Zheng L, Hagan KB, Villarreal J, Keerty V, Chen J, Cata JP. Scalp block for glioblastoma surgery is associated with lower inflammatory scores and improved survival. Minerva Anestesiol. (2017) 83(11):1137–45. doi: 10.23736/S0375-9393.17.11881-X
105. Osborn I, Sebeo J. “Scalp block” During craniotomy: A classic technique revisited. J Neurosurg Anesthesiol. (2010) 22:187–94. doi: 10.1097/ANA.0b013e3181d48846
106. Cata JP, Bhavsar S, Hagan KB, Arunkumar R, Shi T, Grasu R, et al. Scalp blocks for brain tumor craniotomies: A retrospective survival analysis of a propensity match cohort of patients. J Clin Neurosci. (2018) 51:46–51. doi: 10.1016/j.jocn.2018.02.022
107. Privorotskiy A, Bhavsar SP, Lang FF, Hu J, Cata JP. Impact of anesthesia and analgesia techniques on glioblastoma progression. A narrative review. Neuro-oncology Adv. (2020) 2:vdaa123. doi: 10.1093/noajnl/vdaa123
108. Cata JP, Hagan KB, Bhavsar SDO, Arunkumar R, Grasu R, Dang A, et al. The use of isoflurane and desflurane as inhalational agents for glioblastoma surgery. A survival analysis. J Clin Neurosci. (2017) 35:82–7. doi: 10.1016/j.jocn.2016.10.006
109. Bertaut A, Truntzer C, Madkouri R, Kaderbhai CG, Derangère V, Vincent J, et al. Blood baseline neutrophil count predicts bevacizumab efficacy in glioblastoma. Oncotarget. (2016) 7:70948–58. doi: 10.18632/oncotarget.10898
110. Clavreul A, Lemée J-M, Soulard G, Rousseau A, Menei P. A simple preoperative blood count to stratify prognosis in isocitrate dehydrogenase-wildtype glioblastoma patients treated with radiotherapy plus concomitant and adjuvant temozolomide. Cancers (Basel). (2021) 13:5778. doi: 10.3390/cancers13225778
111. Lopes M, Carvalho B, Vaz R, Linhares P. Influence of neutrophil–lymphocyte ratio in prognosis of glioblastoma multiforme. J Neurooncol. (2018) 136:173–80. doi: 10.1007/s11060-017-2641-3
112. Alexiou G, Vartholomatos E, Voulgaris S, Zagorianakou P. Prognostic significance of neutrophil-to-lymphocyte ratio in glioblastoma. Neuroimmunol Neuroinflamm. (2014) 1:131. doi: 10.4103/2347-8659.143666
113. Ma L, Li G, Wei M. Neutrophil-to-lymphocyte ratio and its changes are related to grade II–IV glioma recurrence. Cancer Manag Res. (2020) 12:9429–34. doi: 10.2147/CMAR.S267523
114. Yersal Ö, Odabaşi E, Özdemir Ö, Kemal Y. Prognostic significance of pre-treatment neutrophil-to-lymphocyte ratio and platelet-to-lymphocyte ratio in patients with glioblastoma. Mol Clin Oncol. (2018) 9:453–8. doi: 10.3892/mco.2018.1695
115. Garrett C, Becker TM, Lynch D, Po J, Xuan W, Scott KF, et al. Comparison of neutrophil to lymphocyte ratio and prognostic nutritional index with other clinical and molecular biomarkers for prediction of glioblastoma multiforme outcome. PloS One. (2021) 16:e0252614. doi: 10.1371/journal.pone.0252614
116. Sedef AM. Pre-treatment lymphopenia and NLR may have prognostic value in turkish high-grade glial tumor patients. Eurasian J Med Investig. (2021) 5(1):107–12. doi: 10.14744/ejmi.2021.12734
117. Templeton AJ, McNamara MG, Šeruga B, Vera-Badillo FE, Aneja P, Ocaña A, et al. Prognostic role of neutrophil-to-lymphocyte ratio in solid tumors: A systematic review and meta-analysis. J Natl Cancer Inst. (2014) 106:dju124. doi: 10.1093/jnci/dju124
118. Mei Z, Shi L, Wang B, Yang J, Xiao Z, Du P, et al. Prognostic role of pretreatment blood neutrophil-to-lymphocyte ratio in advanced cancer survivors: A systematic review and meta-analysis of 66 cohort studies. Cancer Treat Rev. (2017) 58:1–13. doi: 10.1016/j.ctrv.2017.05.005
119. McNamara MG, Lwin Z, Jiang H, Templeton AJ, Zadeh G, Bernstein M, et al. Factors impacting survival following second surgery in patients with glioblastoma in the temozolomide treatment era, incorporating neutrophil/lymphocyte ratio and time to first progression. J Neurooncol. (2014) 117:147–52. doi: 10.1007/s11060-014-1366-9
120. Kaya V, Yıldırım M, Yazıcı G, Yalçın AY, Orhan N, Güzel A. Prognostic significance of indicators of systemic inflammatory responses in glioblastoma patients. Asian Pac J Cancer Prev. (2017) 18:3287–91. doi: 10.22034/APJCP.2017.18.12.3287
121. Wang P-F, Song H-W, Cai H-Q, Kong L-W, Yao K, Jiang T, et al. Preoperative inflammation markers and IDH mutation status predict glioblastoma patient survival. Oncotarget. (2017) 8:50117–23. doi: 10.18632/oncotarget.15235
122. Wiencke JK, Koestler DC, Salas LA, Wiemels JL, Roy RP, Hansen HM, et al. Immunomethylomic approach to explore the blood neutrophil lymphocyte ratio (NLR) in glioma survival. Clin Epigenet. (2017) 9:10. doi: 10.1186/s13148-017-0316-8
123. Bao Y, Yang M, Jin C, Hou S, Shi B, Shi J, et al. Preoperative hematologic inflammatory markers as prognostic factors in patients with glioma. World Neurosurg. (2018) 119:e710–6. doi: 10.1016/j.wneu.2018.07.252
124. Coleman N, Michalarea V, Alken S, Rihawi K, Lopez RP, Tunariu N, et al. Safety, efficacy and survival of patients with primary Malignant brain tumours (PMBT) in phase I (Ph1) trials: The 12-year Royal Marsden experience. J Neurooncol. (2018) 139:107–16. doi: 10.1007/s11060-018-2847-z
125. Hao Y, Li X, Chen H, Huo H, Liu Z, Tian F, et al. A cumulative score based on preoperative neutrophil-lymphocyte ratio and fibrinogen in predicting overall survival of patients with glioblastoma multiforme. World Neurosurg. (2019) 128:e427–33. doi: 10.1016/j.wneu.2019.04.169
126. Lv Y, Zhang S, Liu Z, Tian Y, Liang N, Zhang J. Prognostic value of preoperative neutrophil to lymphocyte ratio is superior to systemic immune inflammation index for survival in patients with Glioblastoma. Clin Neurol Neurosurg. (2019) 181:24–7. doi: 10.1016/j.clineuro.2019.03.017
127. Maas SLN, Draaisma K, Snijders TJ, Senders JT, Berendsen S, Seute T, et al. Routine blood tests do not predict survival in glioblastoma patients – multivariable analysis of 497 patients. World Neurosurg. (2019) 126:e1081–91. doi: 10.1016/j.wneu.2019.03.053
128. Zhang Z-Y, Zhan Y-B, Zhang F-J, Yu B, Ji Y-C, Zhou J-Q, et al. Prognostic value of preoperative hematological markers combined with molecular pathology in patients with diffuse gliomas. Aging (Albany NY). (2019) 11:6252–72. doi: 10.18632/aging.102186
129. Yang C, Hu B-W, Tang F, Zhang Q, Quan W, Wang J, et al. Prognostic value of systemic immune-inflammation index (SII) in patients with glioblastoma: A comprehensive study based on meta-analysis and retrospective single-center analysis. J Clin Med. (2022) 11:7514. doi: 10.3390/jcm11247514
130. Duan X, Yang B, Zhao C, Tie B, Cao L, Gao Y. Prognostic value of preoperative hematological markers in patients with glioblastoma multiforme and construction of random survival forest model. BMC Cancer. (2023) 23:432. doi: 10.1186/s12885-023-10889-0
131. Lei Y, Li Y, Hu Q, Wang J, Sui A. Prognostic impact of neutrophil-to-lymphocyte ratio in gliomas: a systematic review and meta-analysis. World J Surg Oncol. (2019) 17:152. doi: 10.1186/s12957-019-1686-5
132. Guo X, Jiao H, Zhang T, Zhang Y. Pre-treatment and preoperative neutrophil-to-lymphocyte ratio predicts prognostic value of glioblastoma: A meta-analysis. Brain Sci. (2022) 12:675. doi: 10.3390/brainsci12050675
133. Jarmuzek P, Kozlowska K, Defort P, Kot M, Zembron-Lacny A. Prognostic values of systemic inflammatory immunological markers in glioblastoma: A systematic review and meta-analysis. Cancers (Basel). (2023) 15:3339. doi: 10.3390/cancers15133339
134. Saito T, Sugiyama K, Hama S, Yamasaki F, Takayasu T, Nosaka R, et al. Prognostic importance of temozolomide-induced neutropenia in glioblastoma, IDH-wildtype patients. Neurosurg Rev. (2018) 41(2):621–8. doi: 10.1007/s10143-017-0903-3
135. Haksoyler V, Besen A A, Koseci T, Olgun P, Bayram E, Topkan E. Neutrophil-to-lymphocyte ratio is prognostic in recurrent glioblastoma multiforme treated with bevacizumab plus irinotecan. biomark Med. (2021) 15:851–9. doi: 10.2217/bmm-2021-0271
136. Brenner A, Friger M, Geffen DB, Kaisman-Elbaz T, Lavrenkov K. The prognostic value of the pretreatment neutrophil/lymphocyte ratio in patients with glioblastoma multiforme brain tumors: A retrospective cohort study of patients treated with combined modality surgery, radiation therapy, and temozolomide chemotherapy. Oncology. (2019) 97:255–63. doi: 10.1159/000500926
137. Gao S, Tang W, Zuo B, Mulvihill L, Yu J, Yu Y. The predictive value of neutrophil-to-lymphocyte ratio for overall survival and pathological complete response in breast cancer patients receiving neoadjuvant chemotherapy. Front Oncol. (2023) 12:1065606. doi: 10.3389/fonc.2022.1065606
138. Kim J-Y, Jung EJ, Kim J-M, Lee HS, Kwag S-J, Park J-H, et al. Dynamic changes of neutrophil-to-lymphocyte ratio and platelet-to-lymphocyte ratio predicts breast cancer prognosis. BMC Cancer. (2020) 20:1206. doi: 10.1186/s12885-020-07700-9
139. Nemoto T, Endo S, Isohata N, Takayanagi D, Nemoto D, Aizawa M, et al. Change in the neutrophil−to−lymphocyte ratio during chemotherapy may predict prognosis in patients with advanced or metastatic colorectal cancer. Mol Clin Oncol. (2021) 14:107. doi: 10.3892/mco.2021.2269
140. Dan J, Tan J, Huang J, Zhang X, Guo Y, Huang Y, et al. The dynamic change of neutrophil to lymphocyte ratio is predictive of pathological complete response after neoadjuvant chemotherapy in breast cancer patients. Breast Cancer. (2020) 27:982–8. doi: 10.1007/s12282-020-01096-x
141. Cho K-M, Park H, Oh D-Y, Kim T-Y, Lee K-H, Han S-W, et al. Neutrophil-to-lymphocyte ratio, platelet-to-lymphocyte ratio, and their dynamic changes during chemotherapy is useful to predict a more accurate prognosis of advanced biliary tract cancer. Oncotarget. (2017) 8:2329–41. doi: 10.18632/oncotarget.13731
142. Tang Y, Cui Y, Li L, Guan Y, Feng D, Yin B, et al. Dynamics of early serum tumour markers and neutrophil-to-lymphocyte ratio predict response to PD-1/PD-L1 inhibitors in advanced non-small-cell lung cancer. Cancer Manag Res. (2021) 13:8241–55. doi: 10.2147/CMAR.S329963
143. Nindra U, Shahnam A, Stevens S, Pal A, Nagrial A, Lee J, et al. Elevated neutrophil-to-lymphocyte ratio ( NLR ) is associated with poorer progression-free survival in unresectable stage III NSCLC treated with consolidation durvalumab. Thorac Cancer. (2022) 13:3058–62. doi: 10.1111/1759-7714.14646
144. Liu D, Jin J, Zhang L, Li L, Song J, Li W. The neutrophil to lymphocyte ratio may predict benefit from chemotherapy in lung cancer. Cell Physiol Biochem. (2018) 46:1595–605. doi: 10.1159/000489207
145. Paramanathan A, Saxena A, Morris DL. A systematic review and meta-analysis on the impact of pre-operative neutrophil lymphocyte ratio on long term outcomes after curative intent resection of solid tumours. Surg Oncol. (2014) 23:31–9. doi: 10.1016/j.suronc.2013.12.001
146. Donskov F. Immunomonitoring and prognostic relevance of neutrophils in clinical trials. Semin Cancer Biol. (2013) 23:200–7. doi: 10.1016/j.semcancer.2013.02.001
147. Zhang J, Zhang S, Song Y, He M, Ren Q, Chen C, et al. Prognostic role of neutrophil lymphocyte ratio in patients with glioma. Oncotarget. (2017) 8:59217–24. doi: 10.18632/oncotarget.19484
148. Shaul ME, Fridlender ZG. Tumour-associated neutrophils in patients with cancer. Nat Rev Clin Oncol. (2019) 16:601–20. doi: 10.1038/s41571-019-0222-4
149. Sacdalan DB, Lucero JA, Sacdalan DL. Prognostic utility of baseline neutrophil-to-lymphocyte ratio in patients receiving immune checkpoint inhibitors: a review and meta-analysis. Onco Targets Ther. (2018) 11:955–65. doi: 10.2147/OTT.S153290
150. Cupp MA, Cariolou M, Tzoulaki I, Aune D, Evangelou E, Berlanga-Taylor AJ. Neutrophil to lymphocyte ratio and cancer prognosis: an umbrella review of systematic reviews and meta-analyses of observational studies. BMC Med. (2020) 18:360. doi: 10.1186/s12916-020-01817-1
151. Mjaess G, Chebel R, Karam A, Moussa I, Pretot D, Abi Tayeh G, et al. Prognostic role of neutrophil-to-lymphocyte ratio (NLR) in urological tumors: an umbrella review of evidence from systematic reviews and meta-analyses. Acta Oncol (Madr). (2021) 60:704–13. doi: 10.1080/0284186X.2021.1886323
152. Takenaka Y, Oya R, Takemoto N, Inohara H. Neutrophil-to-lymphocyte ratio as a prognostic marker for head and neck squamous cell carcinoma treated with immune checkpoint inhibitors: Meta-analysis. Head Neck. (2022) 44:1237–45. doi: 10.1002/hed.26997
153. Kang D, Liu S, Yuan X, Liu S, Zhang Z, He Z, et al. A systematic review and meta-analysis of prognostic indicators in patients with head and neck Malignancy treated with immune checkpoint inhibitors. J Cancer Res Clin Oncol. (2023) 149:18215–40. doi: 10.1007/s00432-023-05504-5
154. Zhang S, Qiu C, Yu H, Xu Y, Xu X. Prognostic value of neutrophil to lymphocyte ratio in gastric cancer patients receiving immune checkpoint inhibitors: a systematic review and meta-analysis. Front Oncol. (2023) 13:1070019. doi: 10.3389/fonc.2023.1070019
155. Li L, Pan L. Prognostic value of neutrophil-to-lymphocyte ratio in gastric cancer patients treated with immune checkpoint inhibitors: A meta-analysis. Kaohsiung J Med Sci. (2023) 39:842–52. doi: 10.1002/kjm2.12694
156. Zhang Y, Liu B, Kotenko S, Li W. Prognostic value of neutrophil-lymphocyte ratio and lactate dehydrogenase in melanoma patients treated with immune checkpoint inhibitors: A systematic review and meta-analysis. Med (Baltimore). (2022) 101:e29536. doi: 10.1097/MD.0000000000029536
157. Yanagisawa T, Mori K, Katayama S, Mostafaei H, Quhal F, Laukhtina E, et al. Hematological prognosticators in metastatic renal cell cancer treated with immune checkpoint inhibitors: A meta-analysis. Immunotherapy. (2022) 14:709–25. doi: 10.2217/imt-2021-0207
158. Chen X, Meng F, Jiang R. Neutrophil-to-lymphocyte ratio as a prognostic biomarker for patients with metastatic renal cell carcinoma treated with immune checkpoint inhibitors: A systematic review and meta-analysis. Front Oncol. (2021) 11:746976. doi: 10.3389/fonc.2021.746976
159. Wang Y, Lu J, Wu C, Fei F, Chu Z, Lu P. Clinical markers predict the efficacy of several immune checkpoint inhibitors in patients with non-small cell lung cancer in China. Front Immunol. (2023) 14:1276107. doi: 10.3389/fimmu.2023.1276107
160. Zhang L, Feng J, Kuang T, Chai D, Qiu Z, Deng W, et al. Blood biomarkers predict outcomes in patients with hepatocellular carcinoma treated with immune checkpoint Inhibitors: A pooled analysis of 44 retrospective sudies. Int Immunopharmacol. (2023) 118:110019. doi: 10.1016/j.intimp.2023.110019
161. Rugambwa TK, Abdihamid O, Zhang X, Peng Y, Cai C, Shen H, et al. Neutrophil–lymphocyte ratio and platelet–lymphocyte ratio as potential predictive markers of treatment response in cancer patients treated with immune checkpoint inhibitors: A systematic review and meta-analysis. Front Oncol. (2023) 13:1181248. doi: 10.3389/fonc.2023.1181248
162. Guo Y, Xiang D, Wan J, Yang L, Zheng C. Focus on the dynamics of neutrophil-to-lymphocyte ratio in cancer patients treated with immune checkpoint inhibitors: A meta-analysis and systematic review. Cancers (Basel). (2022) 14:5297. doi: 10.3390/cancers14215297
163. Zheng M. Systemic inflammation shapes clinical outcomes in response to immune checkpoint blockade treatment: moving toward optimizing antitumor immunity. J Immunother Cancer. (2023) 11:e006462. doi: 10.1136/jitc-2022-006462
164. Tashima Y, Kuwata T, Yoneda K, Hirai A, Mori M, Kanayama M, et al. Prognostic impact of PD-L1 expression in correlation with neutrophil-to-lymphocyte ratio in squamous cell carcinoma of the lung. Sci Rep. (2020) 10:1243. doi: 10.1038/s41598-019-57321-x
165. Zurlo IV, Schino M, Strippoli A, Calegari MA, Cocomazzi A, Cassano A, et al. Predictive value of NLR, TILs (CD4+/CD8+) and PD-L1 expression for prognosis and response to preoperative chemotherapy in gastric cancer. Cancer Immunol Immunother. (2022) 71:45–55. doi: 10.1007/s00262-021-02960-1
166. Xia L, Huang H, Xiao H, Wang D, Yang Z. Utilization of combined PD-L1 expression and neutrophil-to-lymphocyte ratio prior to surgery as a prognostic factor in non-small cell lung cancer with brain metastasis. Transl Cancer Res. (2019) 8:2864–77. doi: 10.21037/tcr.2019.11.08
167. Valero C, Lee M, Hoen D, Weiss K, Kelly DW, Adusumilli PS, et al. Pretreatment neutrophil-to-lymphocyte ratio and mutational burden as biomarkers of tumor response to immune checkpoint inhibitors. Nat Commun. (2021) 12:729. doi: 10.1038/s41467-021-20935-9
168. Kao C, Powers E, Wu Y, Datto MB, Green MF, Strickler JH, et al. Predictive value of combining biomarkers for clinical outcomes in advanced non-small cell lung cancer patients receiving immune checkpoint inhibitors. Clin Lung Cancer. (2021) 22:500–9. doi: 10.1016/j.cllc.2021.03.017
169. Cortellini A, Ricciuti B, Borghaei H, Naqash AR, D’Alessio A, Fulgenzi CAM, et al. Differential prognostic effect of systemic inflammation in patients with non–small cell lung cancer treated with immunotherapy or chemotherapy: A post hoc analysis of the phase 3 OAK trial. Cancer. (2022) 128:3067–79. doi: 10.1002/cncr.34348
170. Lu H-R, Zhu P-F, Deng Y-Y, Chen Z-L, Yang L. Predictive value of NLR and PLR for immune-related adverse events: a systematic review and meta-analysis. Clin Transl Oncol. (2023). doi: 10.1007/s12094-023-03313-3
171. Zhang W, Tan Y, Li Y, Liu J. Neutrophil to Lymphocyte ratio as a predictor for immune-related adverse events in cancer patients treated with immune checkpoint inhibitors: A systematic review and meta-analysis. Front Immunol. (2023) 14:1234142. doi: 10.3389/fimmu.2023.1234142
172. Fest J, Ruiter R, Ikram MA, Voortman T, van Eijck CHJ, Stricker BH. Reference values for white blood-cell-based inflammatory markers in the Rotterdam Study: A population-based prospective cohort study. Sci Rep. (2018) 8:10566. doi: 10.1038/s41598-018-28646-w
173. Quail DF, Amulic B, Aziz M, Barnes BJ, Eruslanov E, Fridlender ZG, et al. Neutrophil phenotypes and functions in cancer: A consensus statement. J Exp Med. (2022) 219. doi: 10.1084/jem.20220011
174. Sagiv JY, Michaeli J, Assi S, Mishalian I, Kisos H, Levy L, et al. Phenotypic diversity and plasticity in circulating neutrophil subpopulations in cancer. Cell Rep. (2015) 10:562–73. doi: 10.1016/j.celrep.2014.12.039
175. Mishalian I, Granot Z, Fridlender ZG. The diversity of circulating neutrophils in cancer. Immunobiology. (2017) 222:82–8. doi: 10.1016/j.imbio.2016.02.001
176. Vanhaver C, Aboubakar Nana F, Delhez N, Luyckx M, Hirsch T, Bayard A, et al. Immunosuppressive low-density neutrophils in the blood of cancer patients display a mature phenotype. Life Sci Alliance. (2024) 7:e202302332. doi: 10.26508/lsa.202302332
177. Messiaen J, Jacobs SA, De Smet F. The tumor micro-environment in pediatric glioma: friend or foe? Front Immunol. (2023) 14:1227126. doi: 10.3389/fimmu.2023.1227126
178. Atai NA, Bansal M, Lo C, Bosman J, Tigchelaar W, Bosch KS, et al. Osteopontin is up-regulated and associated with neutrophil and macrophage infiltration in glioblastoma. Immunology. (2011) 132:39–48. doi: 10.1111/j.1365-2567.2010.03335.x
179. Liang J, Piao Y, Holmes L, Fuller GN, Henry V, Tiao N, et al. Neutrophils promote the Malignant glioma phenotype through S100A4. Clin Cancer Res. (2014) 20:187–98. doi: 10.1158/1078-0432.CCR-13-1279
180. Zilio S, Serafini P. Neutrophils and granulocytic MDSC: The janus god of cancer immunotherapy. Vaccines. (2016) 4:31. doi: 10.3390/vaccines4030031
181. Khan F, Pang L, Dunterman M, Lesniak MS, Heimberger AB, Chen P. Macrophages and microglia in glioblastoma: Heterogeneity, plasticity, and therapy. J Clin Invest. (2023) 133. doi: 10.1172/JCI163446
182. Pinton L, Masetto E, Vettore M, Solito S, Magri S, D’Andolfi M, et al. The immune suppressive microenvironment of human gliomas depends on the accumulation of bone marrow-derived macrophages in the center of the lesion. J Immunother Cancer. (2019) 7:58. doi: 10.1186/s40425-019-0536-x
183. González-Tablas Pimenta M, Otero Á, Arandia Guzman DA, Pascual-Argente D, Ruíz Martín L, Sousa-Casasnovas P, et al. Tumor cell and immune cell profiles in primary human glioblastoma: Impact on patient outcome. Brain Pathol. (2021) 31:365–80. doi: 10.1111/bpa.12927
184. Karimi E, Yu MW, Maritan SM, Perus LJM, Rezanejad M, Sorin M, et al. Single-cell spatial immune landscapes of primary and metastatic brain tumours. Nature. (2023) 614:555–63. doi: 10.1038/s41586-022-05680-3
185. Buonfiglioli A, Hambardzumyan D. Macrophages and microglia: the cerberus of glioblastoma. Acta Neuropathol Commun. (2021) 9:54. doi: 10.1186/s40478-021-01156-z
186. Salacz M, Kast R, Saki N, Brüning A, Karpel-Massler G, Halatsch M-E. Toward a noncytotoxic glioblastoma therapy: Blocking MCP-1 with the MTZ Regimen. Onco Targets Ther. (2016) 9:2535. doi: 10.2147/OTT.S100407
187. Zeiner PS, Preusse C, Golebiewska A, Zinke J, Iriondo A, Muller A, et al. Distribution and prognostic impact of microglia/macrophage subpopulations in gliomas. Brain Pathol. (2019) 29:513–29. doi: 10.1111/bpa.12690
188. Poon CC, Sarkar S, Yong VW, Kelly JJP. Glioblastoma-associated microglia and macrophages: Targets for therapies to improve prognosis. Brain. (2017) 140:1548–60. doi: 10.1093/brain/aww355
189. Gieryng A, Pszczolkowska D, Walentynowicz KA, Rajan WD, Kaminska B. Immune microenvironment of gliomas. Lab Investig. (2017) 97:498–518. doi: 10.1038/labinvest.2017.19
190. Boussiotis VA, Charest A. Immunotherapies for Malignant glioma. Oncogene. (2018) 37(9):1121–41. doi: 10.1038/s41388-017-0024-z
191. Prosniak M, Harshyne LA, Andrews DW, Kenyon LC, Bedelbaeva K, Apanasovich TV, et al. Glioma grade is associated with the accumulation and activity of cells bearing M2 monocyte markers. Clin Cancer Res. (2013) 19:3776–86. doi: 10.1158/1078-0432.CCR-12-1940
192. Vidyarthi A, Agnihotri T, Khan N, Singh S, Tewari MK, Radotra BD, et al. Predominance of M2 macrophages in gliomas leads to the suppression of local and systemic immunity. Cancer Immunol Immunother. (2019) 68:1995–2004. doi: 10.1007/s00262-019-02423-8
193. Sørensen MD, Dahlrot RH, Boldt HB, Hansen S, Kristensen BW. Tumour-associated microglia/macrophages predict poor prognosis in high-grade gliomas and correlate with an aggressive tumour subtype. Neuropathol Appl Neurobiol. (2018) 44:185–206. doi: 10.1111/nan.12428
194. Martinez-Lage M, Lynch TM, Bi Y, Cocito C, Way GP, Pal S, et al. Immune landscapes associated with different glioblastoma molecular subtypes. Acta Neuropathol Commun. (2019) 7:203. doi: 10.1186/s40478-019-0803-6
195. Mi Y, Guo N, Luan J, Cheng J, Hu Z, Jiang P, et al. The emerging role of myeloid-derived suppressor cells in the glioma immune suppressive microenvironment. Front Immunol. (2020) 11:737. doi: 10.3389/fimmu.2020.00737
196. Dubinski D, Wölfer J, Hasselblatt M, Schneider-Hohendorf T, Bogdahn U, Stummer W, et al. CD4+ T effector memory cell dysfunction is associated with the accumulation of granulocytic myeloid-derived suppressor cells in glioblastoma patients. Neuro Oncol. (2016) 18:807–18. doi: 10.1093/neuonc/nov280
197. da Silva LHR, Catharino LCC, da Silva VJ, Evangelista GCM, Barbuto JAM. The War Is on: The Immune System against Glioblastoma-How Can NK Cells Drive This Battle? Biomedicines. (2022) 10:400. doi: 10.3390/biomedicines10020400
198. Li Y, Sharma A, Maciaczyk J, Schmidt-Wolf IGH. Recent development in NKT-based immunotherapy of glioblastoma: From bench to bedside. Int J Mol Sci. (2022) 23:1311. doi: 10.3390/ijms23031311
199. Asl NS, Behfar M, Amiri RS, Mohseni R, Azimi M, Firouzi J, et al. Intra-lesion injection of activated Natural Killer (NK) cells in recurrent Malignant brain tumors. Int Immunopharmacol. (2023) 120:110345. doi: 10.1016/j.intimp.2023.110345
200. Burger MC, Forster M-T, Romanski A, Straßheimer F, Macas J, Zeiner PS, et al. Intracranial injection of NK cells engineered with a HER2-targeted chimeric antigen receptor in patients with recurrent glioblastoma. Neuro Oncol. (2023) 25(11):2058–71. doi: 10.1093/neuonc/noad087
201. Villar J, Segura E. Decoding the heterogeneity of human dendritic cell subsets. Trends Immunol. (2020) 41:1062–71. doi: 10.1016/j.it.2020.10.002
202. Gardam B, Gargett T, Brown MP, Ebert LM. Targeting the dendritic cell-T cell axis to develop effective immunotherapies for glioblastoma. Front Immunol. (2023) 14:1261257. doi: 10.3389/fimmu.2023.1261257
203. Han S, Zhang C, Li Q, Dong J, Liu Y, Huang Y, et al. Tumour-infiltrating CD4(+) and CD8(+) lymphocytes as predictors of clinical outcome in glioma. Br J Cancer. (2014) 110:2560–8. doi: 10.1038/bjc.2014.162
204. Heimberger AB, Abou-Ghazal M, Reina-Ortiz C, Yang DS, Sun W, Qiao W, et al. Incidence and prognostic impact of foxP3+ Regulatory T cells in human gliomas. Clin Cancer Res. (2008) 14:5166–72. doi: 10.1158/1078-0432.CCR-08-0320
205. Lohr J, Ratliff T, Huppertz A, Ge Y, Dictus C, Ahmadi R, et al. Effector T-cell infiltration positively impacts survival of glioblastoma patients and is impaired by tumor-derived TGF-β. Clin Cancer Res. (2011) 17:4296–308. doi: 10.1158/1078-0432.CCR-10-2557
206. El Andaloussi A, Lesniak MS. CD4+ CD25+ FoxP3+ T-cell infiltration and heme oxygenase-1 expression correlate with tumor grade in human gliomas. J Neurooncol. (2007) 83:145–52. doi: 10.1007/s11060-006-9314-y
207. Yu JS, Lee PK, Ehtesham M, Samoto K, Black KL, Wheeler CJ. Intratumoral T cell subset ratios and Fas ligand expression on brain tumor endothelium. J Neurooncol. (2003) 64:55–61. doi: 10.1007/BF02700020
208. Innocenti L, Ortenzi V, Scarpitta R, Montemurro N, Pasqualetti F, Asseri R, et al. The prognostic impact of gender, therapeutic strategies, molecular background, and tumor-infiltrating lymphocytes in glioblastoma: A still unsolved jigsaw. Genes (Basel). (2023) 14:501. doi: 10.3390/genes14020501
209. Rutledge WC, Kong J, Gao J, Gutman DA, Cooper LAD, Appin C, et al. Tumor-infiltrating lymphocytes in glioblastoma are associated with specific genomic alterations and related to transcriptional class. Clin Cancer Res. (2013) 19:4951–60. doi: 10.1158/1078-0432.CCR-13-0551
210. Yang I, Tihan T, Han SJ, Wrensch MR, Wiencke J, Sughrue ME, et al. CD8+ T-cell infiltrate in newly diagnosed glioblastoma is associated with long-term survival. J Clin Neurosci. (2010) 17:1381–5. doi: 10.1016/j.jocn.2010.03.031
211. Berghoff AS, Kiesel B, Widhalm G, Rajky O, Ricken G, Wöhrer A, et al. Programmed death ligand 1 expression and tumor-infiltrating lymphocytes in glioblastoma. Neuro Oncol. (2015) 17:1064–75. doi: 10.1093/neuonc/nou307
212. Tamura R, Ohara K, Sasaki H, Morimoto Y, Kosugi K, Yoshida K, et al. Difference in immunosuppressive cells between peritumoral area and tumor core in glioblastoma. World Neurosurg. (2018) 120:e601–10. doi: 10.1016/j.wneu.2018.08.133
213. Barcia C, Gómez A, Gallego-Sanchez JM, Perez-Vallés A, Castro MG, Lowenstein PR, et al. Infiltrating CTLs in human glioblastoma establish immunological synapses with tumorigenic cells. Am J Pathol. (2009) 175:786–98. doi: 10.2353/ajpath.2009.081034
214. Yang I, Han SJ, Sughrue ME, Tihan T, Parsa AT. Immune cell infiltrate differences in pilocytic astrocytoma and glioblastoma: Evidence of distinct immunological microenvironments that reflect tumor biology. J Neurosurg. (2011) 115:505–11. doi: 10.3171/2011.4.JNS101172
215. Waziri A, Killory B, Ogden AT, Canoll P, Anderson RCE, Kent SC, et al. Preferential in situ CD4+CD56+ T cell activation and expansion within human glioblastoma. J Immunol. (2008) 180:7673–80. doi: 10.4049/jimmunol.180.11.7673
216. Magri S, Musca B, Bonaudo C, Tushe A, Russo MG, Masetto E, et al. Sustained accumulation of blood-derived macrophages in the immune microenvironment of patients with recurrent glioblastoma after therapy. Cancers (Basel). (2021) 13:6178. doi: 10.3390/cancers13246178
217. Woroniecka K, Chongsathidkiet P, Rhodin KE, Kemeny HR, Dechant CA, Farber SH, et al. T cell exhaustion signatures vary with tumor type and are severe in glioblastoma. Clin Cancer Res. (2018) 24(17):4175–86. doi: 10.1158/1078-0432.CCR-17-1846
218. Brooks WH, Markesbery WR, Gupta GD, Roszman TL. Relationship of lymphocyte invasion and survival of brain tumor patients. Ann Neurol. (1978) 4:219–24. doi: 10.1002/ana.410040305
219. Palma L, Di Lorenzo N, Guidetti B. Lymphocytic infiltrates in primary glioblastomas and recidivous gliomas. Incidence fate relevance to prognosis 228 operated cases. J Neurosurg. (1978) 49:854–61. doi: 10.3171/jns.1978.49.6.0854
220. Schiffer D, Cavicchioli D, Giordana MT, Palmucci L, Piazza A. Analysis of some factors effecting survival in Malignant gliomas. Tumori. (1979) 65:119–25. doi: 10.1177/030089167906500114
221. Böker DK, Kalff R, Gullotta F, Weekes-Seifert S, Möhrer U. Mononuclear infiltrates in human intracranial tumors as a prognostic factor. Influence of preoperative steroid treatment. I. Glioblastoma. Clin Neuropathol. (1984) 3(4):143–7.
222. Safdari H, Hochberg FH, Richardson EP. Prognostic value of round cell (lymphocyte) infiltration in Malignant gliomas. Surg Neurol. (1985) 23:221–6. doi: 10.1016/0090-3019(85)90086-2
223. Rossi ML, Jones NR, Candy E, Nicoll JA, Compton JS, Hughes JT, et al. The mononuclear cell infiltrate compared with survival in high-grade astrocytomas. Acta Neuropathol. (1989) 78:189–93. doi: 10.1007/BF00688208
224. Kim Y-H, Jung T-Y, Jung S, Jang W-Y, Moon K-S, Kim I-Y, et al. Tumour-infiltrating T-cell subpopulations in glioblastomas. Br J Neurosurg. (2012) 26:21–7. doi: 10.3109/02688697.2011.584986
225. Yue Q, Zhang X, Ye H, Wang Y, Du Z, Yao Y, et al. The prognostic value of Foxp3+ tumor-infiltrating lymphocytes in patients with glioblastoma. J Neurooncol. (2014) 116:251–9. doi: 10.1007/s11060-013-1314-0
226. Madkouri R, Kaderbhai CG, Bertaut A, Truntzer C, Vincent J, Aubriot-Lorton MH, et al. Immune classifications with cytotoxic CD8(+) and Th17 infiltrates are predictors of clinical prognosis in glioblastoma. Oncoimmunology. (2017) 6:e1321186. doi: 10.1080/2162402X.2017.1321186
227. Orrego E, Castaneda CA, Castillo M, Bernabe LA, Casavilca S, Chakravarti A, et al. Distribution of tumor-infiltrating immune cells in glioblastoma. CNS Oncol. (2018) 7:CNS21. doi: 10.2217/cns-2017-0037
228. Wang R, Song Y, Hu T, Wang X, Jiang Y, Zhang D, et al. Decreased CD8+ Lymphocytic infiltration in multifocal and multicentric glioblastomas. Front Oncol. (2021) 11:748277. doi: 10.3389/fonc.2021.748277
229. Mauldin IS, Jo J, Wages NA, Yogendran LV, Mahmutovic A, Young SJ, et al. Proliferating CD8+ T cell infiltrates are associated with improved survival in glioblastoma. Cells. (2021) 10:3378. doi: 10.3390/cells10123378
230. Sobhani N, Bouchè V, Aldegheri G, Rocca A, D’Angelo A, Giudici F, et al. Analysis of PD-L1 and CD3 expression in glioblastoma patients and correlation with outcome: A single center report. Biomedicines. (2023) 11:311. doi: 10.3390/biomedicines11020311
231. Thomas AA, Fisher JL, Rahme GJ, Hampton TH, Baron U, Olek S, et al. Regulatory T cells are not a strong predictor of survival for patients with glioblastoma. Neuro Oncol. (2015) 17:801–9. doi: 10.1093/neuonc/nou363
232. Sayour EJ, McLendon P, McLendon R, De Leon G, Reynolds R, Kresak J, et al. Increased proportion of FoxP3+ regulatory T cells in tumor infiltrating lymphocytes is associated with tumor recurrence and reduced survival in patients with glioblastoma. Cancer Immunol Immunother. (2015) 64:419–27. doi: 10.1007/s00262-014-1651-7
233. Jacobs JFM, Idema AJ, Bol KF, Grotenhuis JA, de Vries IJM, Wesseling P, et al. Prognostic significance and mechanism of Treg infiltration in human brain tumors. J Neuroimmunol. (2010) 225:195–9. doi: 10.1016/j.jneuroim.2010.05.020
234. Geng Y, Shao Y, He W, Hu W, Xu Y, Chen J, et al. Prognostic role of tumor-infiltrating lymphocytes in lung cancer: A meta-analysis. Cell Physiol Biochem. (2015) 37:1560–71. doi: 10.1159/000438523
235. Ibrahim EM, Al-Foheidi ME, Al-Mansour MM, Kazkaz GA. The prognostic value of tumor-infiltrating lymphocytes in triple-negative breast cancer: A meta-analysis. Breast Cancer Res Treat. (2014) 148:467–76. doi: 10.1007/s10549-014-3185-2
236. Zheng X, Song X, Shao Y, Xu B, Hu W, Zhou Q, et al. Prognostic role of tumor-infiltrating lymphocytes in esophagus cancer: A meta-analysis. Cell Physiol Biochem. (2018) 45:720–32. doi: 10.1159/000487164
237. de Ruiter EJ, Ooft ML, Devriese LA, Willems SM. The prognostic role of tumor infiltrating T-lymphocytes in squamous cell carcinoma of the head and neck: A systematic review and meta-analysis. Oncoimmunology. (2017) 6:e1356148. doi: 10.1080/2162402X.2017.1356148
238. Li J, Wang J, Chen R, Bai Y, Lu X. The prognostic value of tumor-infiltrating T lymphocytes in ovarian cancer. Oncotarget. (2017) 8:15621–31. doi: 10.18632/oncotarget.14919
239. Shang B, Liu YY, Jiang S, Liu YY. Prognostic value of tumor-infiltrating FoxP3+ regulatory T cells in cancers: A systematic review and meta-analysis. Sci Rep. (2015) 5:15179. doi: 10.1038/srep15179
240. Barnes TA, Amir E. HYPE or HOPE: The prognostic value of infiltrating immune cells in cancer. Br J Cancer. (2017) 117:451–60. doi: 10.1038/bjc.2017.220
241. Kirilovsky A, Marliot F, El Sissy C, Haicheur N, Galon J, Pagès F. Rational bases for the use of the Immunoscore in routine clinical settings as a prognostic and predictive biomarker in cancer patients. Int Immunol. (2016) 28:373–82. doi: 10.1093/intimm/dxw021
242. Galon J, Mlecnik B, Bindea G, Angell HK, Berger A, Lagorce C, et al. Towards the introduction of the “Immunoscore” in the classification of Malignant tumours. J Pathol. (2014) 232:199–209. doi: 10.1002/path.4287
243. Jia Q, Yang Y, Wan Y. Tumor-infiltrating memory T-lymphocytes for prognostic prediction in cancer patients: A meta-analysis. Int J Clin Exp Med. (2015) 8:1803–13.
244. Simoni Y, Chng MHY, Li S, Fehlings M, Newell EW. Mass cytometry: A powerful tool for dissecting the immune landscape. Curr Opin Immunol. (2018) 51:187–96. doi: 10.1016/j.coi.2018.03.023
245. Alban TJ, Alvarado AG, Sorensen MD, Bayik D, Volovetz J, Serbinowski E, et al. Global immune fingerprinting in glioblastoma patient peripheral blood reveals immune-suppression signatures associated with prognosis. JCI Insight. (2018) 3. doi: 10.1172/jci.insight.122264
246. Yang Z-Z, Kim HJ, Villasboas JC, Price-Troska T, Jalali S, Wu H, et al. Mass cytometry analysis reveals that specific intratumoral CD4+ T cell subsets correlate with patient survival in follicular lymphoma. Cell Rep. (2019) 26:2178–2193.e3. doi: 10.1016/j.celrep.2019.01.085
247. Chevrier S, Levine JH, Zanotelli VRT, Silina K, Schulz D, Bacac M, et al. An immune atlas of clear cell renal cell carcinoma. Cell. (2017) 169:736–749.e18. doi: 10.1016/j.cell.2017.04.016
248. Saito T, Nishikawa H, Wada H, Nagano Y, Sugiyama D, Atarashi K, et al. Two FOXP3(+)CD4(+) T cell subpopulations distinctly control the prognosis of colorectal cancers. Nat Med. (2016) 22:679–84. doi: 10.1038/nm.4086
249. Li Z, Liu X, Guo R, Wang P. CD4+Foxp3- type 1 regulatory T cells in glioblastoma multiforme suppress T cell responses through multiple pathways and are regulated by tumor-associated macrophages. Int J Biochem Cell Biol. (2016) 81:1–9. doi: 10.1016/j.biocel.2016.09.013
250. Li X, Peng J, Pang Y, Yu S, Yu X, Chen P, et al. Identification of a FOXP3+CD3+CD56+ population with immunosuppressive function in cancer tissues of human hepatocellular carcinoma. Sci Rep. (2015) 5:14757. doi: 10.1038/srep14757
251. Agrawal L, Engel KB, Greytak SR, Moore HM. Understanding preanalytical variables and their effects on clinical biomarkers of oncology and immunotherapy. Semin Cancer Biol. (2018) 52:26–38. doi: 10.1016/j.semcancer.2017.12.008
252. Pollard K, Lunny D, Holgate CS, Jackson P, Bird CC. Fixation, processing, and immunochemical reagent effects on preservation of T-lymphocyte surface membrane antigens in paraffin-embedded tissue. J Histochem Cytochem. (1987) 35:1329–38. doi: 10.1177/35.11.3309048
253. Tan WCC, Nerurkar SN, Cai HY, Ng HHM, Wu D, Wee YTF, et al. Overview of multiplex immunohistochemistry/immunofluorescence techniques in the era of cancer immunotherapy. Cancer Commun. (2020) 40:135–53. doi: 10.1002/cac2.12023
254. Sankowski R, Süß P, Benkendorff A, Böttcher C, Fernandez-Zapata C, Chhatbar C, et al. Multiomic spatial landscape of innate immune cells at human central nervous system borders. Nat Med. (2024) 30(1):186–98. doi: 10.1038/s41591-023-02673-1
255. Yin W, Ping Y, Li F, Lv S, Zhang X, Li X, et al. A map of the spatial distribution and tumour-associated macrophage states in glioblastoma and grade 4 IDH -mutant astrocytoma. J Pathol. (2022) 258:121–35. doi: 10.1002/path.5984
256. Richardson LG, Miller JJ, Kitagawa Y, Wakimoto H, Choi BD, Curry WT. Implications of IDH mutations on immunotherapeutic strategies for Malignant glioma. Neurosurg Focus. (2022) 52:E6. doi: 10.3171/2021.11.FOCUS21604
257. Galluzzi L, Kroemer G. Potent immunosuppressive effects of the oncometabolite R -2-hydroxyglutarate. Oncoimmunology. (2018) 7:e1528815. doi: 10.1080/2162402X.2018.1528815
258. Yan D, Li W, Liu Q, Yang K. Advances in immune microenvironment and immunotherapy of isocitrate dehydrogenase mutated glioma. Front Immunol. (2022) 13:914618. doi: 10.3389/fimmu.2022.914618
259. Berghoff AS, Kiesel B, Widhalm G, Wilhelm D, Rajky O, Kurscheid S, et al. Correlation of immune phenotype with IDH mutation in diffuse glioma. Neuro Oncol. (2017) 19:1460–8. doi: 10.1093/neuonc/nox054
260. Tang F, Wang D, Xi C, Yang J, Liu Z, Yu D, et al. Local and systemic effects of IDH mutations on primary glioma patients. Immunology. (2023) 169:503–14. doi: 10.1111/imm.13649
261. Kohanbash G, Carrera DA, Shrivastav S, Ahn BJ, Jahan N, Mazor T, et al. Isocitrate dehydrogenase mutations suppress STAT1 and CD8+ T cell accumulation in gliomas. J Clin Invest. (2017) 127:1425–37. doi: 10.1172/JCI90644
262. Richardson LG, Nieman LT, Stemmer-Rachamimov AO, Zheng XS, Stafford K, Nagashima H, et al. IDH-mutant gliomas harbor fewer regulatory T cells in humans and mice. Oncoimmunology. (2020) 9. doi: 10.1080/2162402X.2020.1806662
263. Klemm F, Maas RR, Bowman RL, Kornete M, Soukup K, Nassiri S, et al. Interrogation of the microenvironmental landscape in brain tumors reveals disease-specific alterations of immune cells. Cell. (2020) 181:1643–1660.e17. doi: 10.1016/j.cell.2020.05.007
264. Amankulor NM, Kim Y, Arora S, Kargl J, Szulzewsky F, Hanke M, et al. Mutant IDH1 regulates the tumor-associated immune system in gliomas. Genes Dev. (2017) 31:774–86. doi: 10.1101/gad.294991.116
265. Friedrich M, Hahn M, Michel J, Sankowski R, Kilian M, Kehl N, et al. Dysfunctional dendritic cells limit antigen-specific T cell response in glioma. Neuro Oncol. (2023) 25:263–76. doi: 10.1093/neuonc/noac138
266. Tyrakis PA, Palazon A, Macias D, Lee KL, Phan AT, Veliça P, et al. S-2-hydroxyglutarate regulates CD8+ T-lymphocyte fate. Nature. (2016) 540:236–41. doi: 10.1038/nature20165
267. Böttcher M, Renner K, Berger R, Mentz K, Thomas S, Cardenas-Conejo ZE, et al. D-2-hydroxyglutarate interferes with HIF-1α stability skewing T-cell metabolism towards oxidative phosphorylation and impairing Th17 polarization. Oncoimmunology. (2018) 7:e1445454. doi: 10.1080/2162402X.2018.1445454
268. Bunse L, Pusch S, Bunse T, Sahm F, Sanghvi K, Friedrich M, et al. Suppression of antitumor T cell immunity by the oncometabolite (R)-2-hydroxyglutarate. Nat Med. (2018) 24:1192–203. doi: 10.1038/s41591-018-0095-6
269. Notarangelo G, Spinelli JB, Perez EM, Baker GJ, Kurmi K, Elia I, et al. Oncometabolite d -2HG alters T cell metabolism to impair CD8 + T cell function. Sci (80- ). (2022) 377:1519–29. doi: 10.1126/science.abj5104
270. Zhang X, Rao A, Sette P, Deibert C, Pomerantz A, Kim WJ, et al. IDH mutant gliomas escape natural killer cell immune surveillance by downregulation of NKG2D ligand expression. Neuro Oncol. (2016) 18:1402–12. doi: 10.1093/neuonc/now061
271. Friedrich M, Sankowski R, Bunse L, Kilian M, Green E, Ramallo Guevara C, et al. Tryptophan metabolism drives dynamic immunosuppressive myeloid states in IDH-mutant gliomas. Nat Cancer. (2021) 2:723–40. doi: 10.1038/s43018-021-00201-z
272. Cao C, Zhang L, Sorensen MD, Reifenberger G, Kristensen BW, McIntyre TM, et al. D-2-hydroxyglutarate regulates human brain vascular endothelial cell proliferation and barrier function. J Neuropathol Exp Neurol. (2023) 82:921–33. doi: 10.1093/jnen/nlad072
273. Alshiekh Nasany R, de la Fuente MI. Therapies for IDH-mutant gliomas. Curr Neurol Neurosci Rep. (2023) 23:225–33. doi: 10.1007/s11910-023-01265-3
274. de la Fuente MI. Targeting IDH1/IDH2 mutations in gliomas. Curr Opin Neurol. (2022) 35:787–93. doi: 10.1097/WCO.0000000000001111
275. Mellinghoff IK, van den Bent MJ, Blumenthal DT, Touat M, Peters KB, Clarke J, et al. Vorasidenib in IDH1- or IDH2-mutant low-grade glioma. N Engl J Med. (2023) 389:589–601. doi: 10.1056/NEJMoa2304194
276. Phillips HS, Kharbanda S, Chen R, Forrest WF, Soriano RH, Wu TD, et al. Molecular subclasses of high-grade glioma predict prognosis, delineate a pattern of disease progression, and resemble stages in neurogenesis. Cancer Cell. (2006) 9:157–73. doi: 10.1016/j.ccr.2006.02.019
277. Verhaak RGW, Hoadley KA, Purdom E, Wang V, Qi Y, Wilkerson MD, et al. Integrated genomic analysis identifies clinically relevant subtypes of glioblastoma characterized by abnormalities in PDGFRA, IDH1, EGFR, and NF1. Cancer Cell. (2010) 17:98–110. doi: 10.1016/j.ccr.2009.12.020
278. Chen J-R, Yao Y, Xu H-Z, Qin Z-Y. Isocitrate dehydrogenase (IDH)1/2 mutations as prognostic markers in patients with glioblastomas. Med (Baltimore). (2016) 95:e2583. doi: 10.1097/MD.0000000000002583
279. Xia L, Wu B, Fu Z, Feng F, Qiao E, Li Q, et al. Prognostic role of IDH mutations in gliomas: A meta-analysis of 55 observational studies. Oncotarget. (2015) 6:17354–65. doi: 10.18632/oncotarget.4008
280. Zou P, Xu H, Chen P, Yan Q, Zhao L, Zhao P, et al. IDH1/IDH2 mutations define the prognosis and molecular profiles of patients with gliomas: A meta-analysis. PloS One. (2013) 8:e68782. doi: 10.1371/journal.pone.0068782
281. Noushmehr H, Weisenberger DJ, Diefes K, Phillips HS, Pujara K, Berman BP, et al. Identification of a cpG island methylator phenotype that defines a distinct subgroup of glioma. Cancer Cell. (2010) 17:510–22. doi: 10.1016/j.ccr.2010.03.017
282. Pal S, Bi Y, Macyszyn L, Showe LC, O’Rourke DM, Davuluri RV. Isoform-level gene signature improves prognostic stratification and accurately classifies glioblastoma subtypes. Nucleic Acids Res. (2014) 42:e64–4. doi: 10.1093/nar/gku121
283. Turcan S, Rohle D, Goenka A, Walsh LA, Fang F, Yilmaz E, et al. IDH1 mutation is sufficient to establish the glioma hypermethylator phenotype. Nature. (2012) 483:479–83. doi: 10.1038/nature10866
284. Brennan CW, Verhaak RGW, McKenna A, Campos B, Noushmehr H, Salama SR, et al. The somatic genomic landscape of glioblastoma. Cell. (2013) 155:462–77. doi: 10.1016/j.cell.2013.09.034
285. Wiestler B, Capper D, Sill M, Jones DTW, Hovestadt V, Sturm D, et al. Integrated DNA methylation and copy-number profiling identify three clinically and biologically relevant groups of anaplastic glioma. Acta Neuropathol. (2014) 128:561–71. doi: 10.1007/s00401-014-1315-x
286. Mur P, Mollejo M, Ruano Y, de Lope ÁR, Fiaño C, García JF, et al. Codeletion of 1p and 19q determines distinct gene methylation and expression profiles in IDH-mutated oligodendroglial tumors. Acta Neuropathol. (2013) 126:277–89. doi: 10.1007/s00401-013-1130-9
287. Ceccarelli M, Barthel FP, Malta TM, Sabedot TS, Salama SR, Murray BA, et al. Molecular profiling reveals biologically discrete subsets and pathways of progression in diffuse glioma. Cell. (2016) 164:550–63. doi: 10.1016/j.cell.2015.12.028
288. Malta TM, de Souza CF, Sabedot TS, Silva TC, Mosella MS, Kalkanis SN, et al. Glioma CpG island methylator phenotype (G-CIMP): biological and clinical implications. Neuro Oncol. (2018) 20:608–20. doi: 10.1093/neuonc/nox183
289. Malta TM, Sabedot TS, Morosini NS, Datta I, Garofano L, Vallentgoed WR, et al. The epigenetic evolution of glioma is determined by the IDH1 mutation status and treatment regimen. Cancer Res. (2023). doi: 10.1158/0008-5472.CAN-23-2093
290. Li KK-W, Shi Z-F, Malta TM, Chan AK-Y, Cheng S, Kwan JSH, et al. Identification of subsets of IDH-mutant glioblastomas with distinct epigenetic and copy number alterations and stratified clinical risks. Neuro-Oncology Adv. (2019) 1. doi: 10.1093/noajnl/vdz015
291. Weller M, Felsberg J, Hentschel B, Gramatzki D, Kubon N, Wolter M, et al. Improved prognostic stratification of patients with isocitrate dehydrogenase-mutant astrocytoma. Acta Neuropathol. (2024) 147:11. doi: 10.1007/s00401-023-02662-1
292. Wang Q, Hu B, Hu X, Kim H, Squatrito M, Scarpace L, et al. Tumor evolution of glioma-intrinsic gene expression subtypes associates with immunological changes in the microenvironment. Cancer Cell. (2017) 32:42–56.e6. doi: 10.1016/j.ccell.2017.06.003
293. Teo W-Y, Sekar K, Seshachalam P, Shen J, Chow W-Y, Lau CC, et al. Relevance of a TCGA-derived glioblastoma subtype gene-classifier among patient populations. Sci Rep. (2019) 9:7442. doi: 10.1038/s41598-019-43173-y
294. White K, Connor K, Meylan M, Bougoüin A, Salvucci M, Bielle F, et al. Identification, validation and biological characterisation of novel glioblastoma tumour microenvironment subtypes: Implications for precision immunotherapy. Ann Oncol. (2023) 34:300–14. doi: 10.1016/j.annonc.2022.11.008
295. Doucette T, Rao G, Rao A, Shen L, Aldape K, Wei J, et al. Immune heterogeneity of glioblastoma subtypes: Extrapolation from the cancer genome atlas. Cancer Immunol Res. (2013) 1:112–22. doi: 10.1158/2326-6066.CIR-13-0028
296. Kaffes I, Szulzewsky F, Chen Z, Herting CJ, Gabanic B, Velázquez Vega JE, et al. Human Mesenchymal glioblastomas are characterized by an increased immune cell presence compared to Proneural and Classical tumors. Oncoimmunology. (2019) 8:e1655360. doi: 10.1080/2162402X.2019.1655360
297. Prins RM, Soto H, Konkankit V, Odesa SK, Eskin A, Yong WH, et al. Gene expression profile correlates with T-cell infiltration and relative survival in glioblastoma patients vaccinated with dendritic cell immunotherapy. Clin Cancer Res. (2011) 17:1603–15. doi: 10.1158/1078-0432.CCR-10-2563
298. Frederico SC, Darling C, Bielanin JP, Dubinsky AC, Zhang X, Hadjipanayis CG, et al. Neoadjuvant immune checkpoint inhibition in the management of glioblastoma: Exploring a new frontier. Front Immunol. (2023) 14:1057567. doi: 10.3389/fimmu.2023.1057567
299. Ogino H, Taylor JW, Nejo T, Gibson D, Watchmaker PB, Okada K, et al. Randomized trial of neoadjuvant vaccination with tumor-cell lysate induces T cell response in low-grade gliomas. J Clin Invest. (2022) 132. doi: 10.1172/JCI151239
300. Waqar M, Trifiletti DM, McBain C, O’Connor J, Coope DJ, Akkari L, et al. Early therapeutic interventions for newly diagnosed glioblastoma: Rationale and review of the literature. Curr Oncol Rep. (2022) 24:311–24. doi: 10.1007/s11912-021-01157-0
301. Moffet JJD, Fatunla OE, Freytag L, Kriel J, Jones JJ, Roberts-Thomson SJ, et al. Spatial architecture of high-grade glioma reveals tumor heterogeneity within distinct domains. Neuro-Oncology Adv. (2023) 5. doi: 10.1093/noajnl/vdad142
302. Wirsching H-G, Felsberg J, Prummer M, Moisoiu V, Lourman R, Hertler C, et al. Spatial immune profiling of glioblastoma identifies an inflammatory, perivascular phenotype associated with longer survival. Acta Neuropathol. (2023) 146:647–9. doi: 10.1007/s00401-023-02617-6
303. Devine RD, Behbehani GK. Mass cytometry, imaging mass cytometry, and multiplexed ion beam imaging use in a clinical setting. Clin Lab Med. (2021) 41:297–308. doi: 10.1016/j.cll.2021.03.008
304. Liu CC, Bosse M, Kong A, Kagel A, Kinders R, Hewitt SM, et al. Reproducible, high-dimensional imaging in archival human tissue by multiplexed ion beam imaging by time-of-flight (MIBI-TOF). Lab Investig. (2022) 102:762–70. doi: 10.1038/s41374-022-00778-8
305. Ptacek J, Locke D, Finck R, Cvijic M-E, Li Z, Tarolli JG, et al. Multiplexed ion beam imaging (MIBI) for characterization of the tumor microenvironment across tumor types. Lab Investig. (2020) 100:1111–23. doi: 10.1038/s41374-020-0417-4
306. Wang XQ, Danenberg E, Huang C-S, Egle D, Callari M, Bermejo B, et al. Spatial predictors of immunotherapy response in triple-negative breast cancer. Nature. (2023) 621:868–76. doi: 10.1038/s41586-023-06498-3
307. Hartmann FJ, Babdor J, Gherardini PF, Amir E-AD, Jones K, Sahaf B, et al. Comprehensive immune monitoring of clinical trials to advance human immunotherapy. Cell Rep. (2019) 28:819–831.e4. doi: 10.1016/j.celrep.2019.06.049
308. Sahaf B, Pichavant M, Lee BH, Duault C, Thrash EM, Davila M, et al. Immune profiling mass cytometry assay harmonization: Multicenter experience from CIMAC-CIDC. Clin Cancer Res. (2021) 27:5062–71. doi: 10.1158/1078-0432.CCR-21-2052
309. Sidiropoulos DN, Stein-O’Brien GL, Danilova L, Gross NE, Charmsaz S, Xavier S, et al. Integrated T cell cytometry metrics for immune-monitoring applications in immunotherapy clinical trials. JCI Insight. (2022) 7. doi: 10.1172/jci.insight.160398
310. Glasson Y, Chépeaux L-A, Dumé A-S, Lafont V, Faget J, Bonnefoy N, et al. Single-cell high-dimensional imaging mass cytometry: one step beyond in oncology. Semin Immunopathol. (2023) 45(1):17–28. doi: 10.1007/s00281-022-00978-w
311. Glasson Y, Chépeaux L-A, Dumé A-S, Jay P, Pirot N, Bonnefoy N, et al. A 31-plex panel for high-dimensional single-cell analysis of murine preclinical models of solid tumors by imaging mass cytometry. Front Immunol. (2023) 13:1011617. doi: 10.3389/fimmu.2022.1011617
312. Iyer A, Hamers AAJ, Pillai AB. CyTOF® for the masses. Front Immunol. (2022) 13:815828. doi: 10.3389/fimmu.2022.815828
313. Kole AJ, Park HS, Yeboa DN, Rutter CE, Corso CD, Aneja S, et al. Concurrent chemoradiotherapy versus radiotherapy alone for “biopsy-only” glioblastoma multiforme. Cancer. (2016) 122:2364–70. doi: 10.1002/cncr.30063
314. Trifiletti DM, Alonso C, Grover S, Fadul CE, Sheehan JP, Showalter TN. Prognostic implications of extent of resection in glioblastoma: Analysis from a large database. World Neurosurg. (2017) 103:330–40. doi: 10.1016/j.wneu.2017.04.035
315. Harlay V, Loundou A, Boucard C, Petrirena G, Barrie M, Campello C, et al. NCOG-08. biopsy-only glioblastomA (BO-GBM) As A Heterogeneous Group Of PatIENTS. Neuro Oncol. (2021) 23:vi153–3. doi: 10.1093/neuonc/noab196.599
316. Bagley SJ, Kothari S, Rahman R, Lee EQ, Dunn GP, Galanis E, et al. Glioblastoma clinical trials: Current landscape and opportunities for improvement. Clin Cancer Res. (2022) 28:594–602. doi: 10.1158/1078-0432.CCR-21-2750
317. Kim Y, Armstrong TS, Gilbert MR, Celiku O. A critical analysis of neuro-oncology clinical trials. Neuro Oncol. (2023) 25:1658–71. doi: 10.1093/neuonc/noad036
318. Getzler I, Bahouth Z, Nativ O, Rubinstein J, Halachmi S. Preoperative neutrophil to lymphocyte ratio improves recurrence prediction of non-muscle invasive bladder cancer. BMC Urol. (2018) 18:90. doi: 10.1186/s12894-018-0404-x
319. Johnson PJ, Dhanaraj S, Berhane S, Bonnett L, Ma YT. The prognostic and diagnostic significance of the neutrophil-to-lymphocyte ratio in hepatocellular carcinoma: a prospective controlled study. Br J Cancer. (2021) 125:714–6. doi: 10.1038/s41416-021-01445-3
320. Novin K, Fadavi P, Mortazavi N, Sanei M, Khoshbakht Ahmadi H, Barahman M, et al. Neutrophil-to-lymphocyte ratio (NLR) as a poor predictive biomarker for pathological response to neoadjuvant chemoradiation in locally advanced rectal cancer: A prospective study. Asian Pacific J Cancer Prev. (2023) 24:61–7. doi: 10.31557/APJCP.2023.24.1.61
321. Zhang Y, Chen S, Chen H, Chen S, Li Z, Feng E, et al. Prognostic value and risk factors of treatment-related lymphopenia in Malignant glioma patients treated with chemoradiotherapy: A systematic review and meta-analysis. Front Neurol. (2021) 12:726561. doi: 10.3389/fneur.2021.726561
322. Bispo RG, Bastos Siqueira IF, de Oliveira BFS, Moreira Fernandes CE, Figueiredo LA, Cintra LP, et al. Prognostic value of the platelet-lymphocyte ratio for glioblastoma: A systematic review. World Neurosurg. (2023) 175:137–141.e1. doi: 10.1016/j.wneu.2023.04.086
323. Wang Y, Xu C, Zhang Z. Prognostic value of pretreatment lymphocyte-to-monocyte ratio in patients with glioma: A meta-analysis. BMC Med. (2023) 21:486. doi: 10.1186/s12916-023-03199-6
324. Zhang S, Ni Q. Prognostic role of the pretreatment systemic immune-inflammation index in patients with glioma: A meta-analysis. Front Neurol. (2023) 14:1094364. doi: 10.3389/fneur.2023.1094364
325. Topkan E, Kucuk A, Ozdemir Y, Mertsoylu H, Besen AA, Sezen D, et al. Systemic inflammation response index predicts survival outcomes in glioblastoma multiforme patients treated with standard stupp protocol. J Immunol Res. (2020) 2020:1–10. doi: 10.1155/2020/8628540
326. He Q, Li L, Ren Q. The prognostic value of preoperative systemic inflammatory response index (SIRI) in patients with high-grade glioma and the establishment of a nomogram. Front Oncol. (2021) 11:671811. doi: 10.3389/fonc.2021.671811
327. Wang Z, Li J, Yuan Y, Li T, Zuo M, Liu Y. Prognostic significance of preoperative systemic inflammation response index in newly diagnosed glioblastoma patients underwent gross total resection: A propensity score matching analysis. World J Surg Oncol. (2022) 20:137. doi: 10.1186/s12957-022-02588-0
328. Ahn S, Park J-S, Kim H, Heo M, Sung YC, Jeun S-S. Compassionate use of recombinant human IL-7-hyFc as a salvage treatment for restoring lymphopenia in patients with recurrent glioblastoma. Cancer Med. (2023) 12:6778–87. doi: 10.1002/cam4.5467
329. Parks KR, Davis JM. Epinephrine, cortisol, endotoxin, nutrition, and the neutrophil. Surg Infect (Larchmt). (2012) 13:300–6. doi: 10.1089/sur.2012.161
330. Benschop RJ, Rodriguez-Feuerhahn M, Schedlowski M. Catecholamine-induced leukocytosis: Early observations, current research, and future directions. Brain Behav Immun. (1996) 10:77–91. doi: 10.1006/brbi.1996.0009
331. Ince LM, Weber J, Scheiermann C. Control of leukocyte trafficking by stress-associated hormones. Front Immunol. (2019) 9:3143. doi: 10.3389/fimmu.2018.03143
Keywords: glioblastoma, glioma, lymphopenia, macrophages, myeloid-derived suppressor cells, natural killer cells, neutrophil-to-lymphocyte ratio, regulatory T cells
Citation: Stepanenko AA, Sosnovtseva AO, Valikhov MP, Chernysheva AA, Abramova OV, Pavlov KA and Chekhonin VP (2024) Systemic and local immunosuppression in glioblastoma and its prognostic significance. Front. Immunol. 15:1326753. doi: 10.3389/fimmu.2024.1326753
Received: 23 October 2023; Accepted: 06 February 2024;
Published: 28 February 2024.
Edited by:
Dorota Lubanska, University of Windsor, CanadaReviewed by:
Mohamed Soliman, Cairo University, EgyptCopyright © 2024 Stepanenko, Sosnovtseva, Valikhov, Chernysheva, Abramova, Pavlov and Chekhonin. This is an open-access article distributed under the terms of the Creative Commons Attribution License (CC BY). The use, distribution or reproduction in other forums is permitted, provided the original author(s) and the copyright owner(s) are credited and that the original publication in this journal is cited, in accordance with accepted academic practice. No use, distribution or reproduction is permitted which does not comply with these terms.
*Correspondence: Aleksei A. Stepanenko, YS5hLnN0ZXBhbmVua29AZ21haWwuY29t
Disclaimer: All claims expressed in this article are solely those of the authors and do not necessarily represent those of their affiliated organizations, or those of the publisher, the editors and the reviewers. Any product that may be evaluated in this article or claim that may be made by its manufacturer is not guaranteed or endorsed by the publisher.
Research integrity at Frontiers
Learn more about the work of our research integrity team to safeguard the quality of each article we publish.