- Glycosciences and Nanomaterial Laboratory, Université du Québec à Montréal, Montréal, QC, Canada
Aberrant glycosylation patterns of glycoproteins and glycolipids have long been recognized as one the major hallmarks of cancer cells that has led to numerous glycoconjugate vaccine attempts. These abnormal glycosylation profiles mostly originate from the lack of key glycosyltransferases activities, mutations, over expressions, or modifications of the requisite chaperone for functional folding. Due to their relative structural simplicity, O-linked glycans of the altered mucin family of glycoproteins have been particularly attractive in the design of tumor associated carbohydrate-based vaccines. Several such glycoconjugate vaccine formulations have generated potent monoclonal anti-carbohydrate antibodies useful as diagnostic and immunotherapies in the fight against cancer. Paradoxically, glycoproteins related to enveloped viruses also express analogous N- and O-linked glycosylation patterns. However, due to the fact that viruses are not equipped with the appropriate glycosyl enzyme machinery, they need to hijack that of the infected host cells. Although the resulting N-linked glycans are very similar to those of normal cells, some of their O-linked glycan patterns often share the common structural simplicity to those identified on tumor cells. Consequently, given that both cancer cells and viral glycoproteins share both common N- and O-linked glycoepitopes, glycoconjugate vaccines could be highly attractive to generate potent immune responses to target both conditions.
1 Introduction
Tumor-associated carbohydrate antigens (TACAs) have long been recognized as key targets toward prophylactic vaccines against cancer (1–5). The corresponding glycoepitopes originate from either glycolipids and gangliosides in particular (6–8) or from their glycoprotein counterparts, in particular cell-surface mucins (9). They are all constitutive members of the glycan structures found on the extracellular membranes of cells where they orchestrate cellular recognition, adhesion, and signaling (10) as well as several other functions, not yet fully exploited (11). As such, carbohydrates add to the arsenal of encoded information analogous to those attributed to nucleotides and amino acids, now referred to as glycocodes (12). Therefore, and not surprisingly, the overall information encoded by carbohydrates holds great promises for cancer (13) and cancer-associated glycosylation for the discovery of effective new cancer drugs (14, 15). As a result, TACAs are important members of the prioritization of cancer antigens which have been included in the National Cancer Institute (NCI) pilot project for accelerated translational research (16). Unfortunately, the targeting of O-linked tumor glycans for generating anti-cancer vaccines has had limited successes in clinical Phase III trials (17) and a thorough analysis of the post mortem data might be useful to explain the numerous failures (18).
2 Glycan biosynthesis and structures of TACAs on tumor cells
As mentioned, the transformation from normal to malignant phenotype in human cancers is associated with aberrant cell surface glycosylation (Figure 1) (13–15). The biosynthesis of O-linked glycans is initiated and completed in the Golgi apparatus. In normal cells, mucin-type O-glycans are represented by structurally complex branched and linear arrangements of monosaccharides that are sequentially assembled by appropriate glycosyltransferases to glycoproteins on serine/threonine residues within the Golgi apparatus. The synthesis of mucin-type O-glycans is complex and depends on many factors. Alternatively, the mucin-type O-glycans are constituted by shorter saccharide sequences in cancer cells due to glycoenzymes mis-regulations.
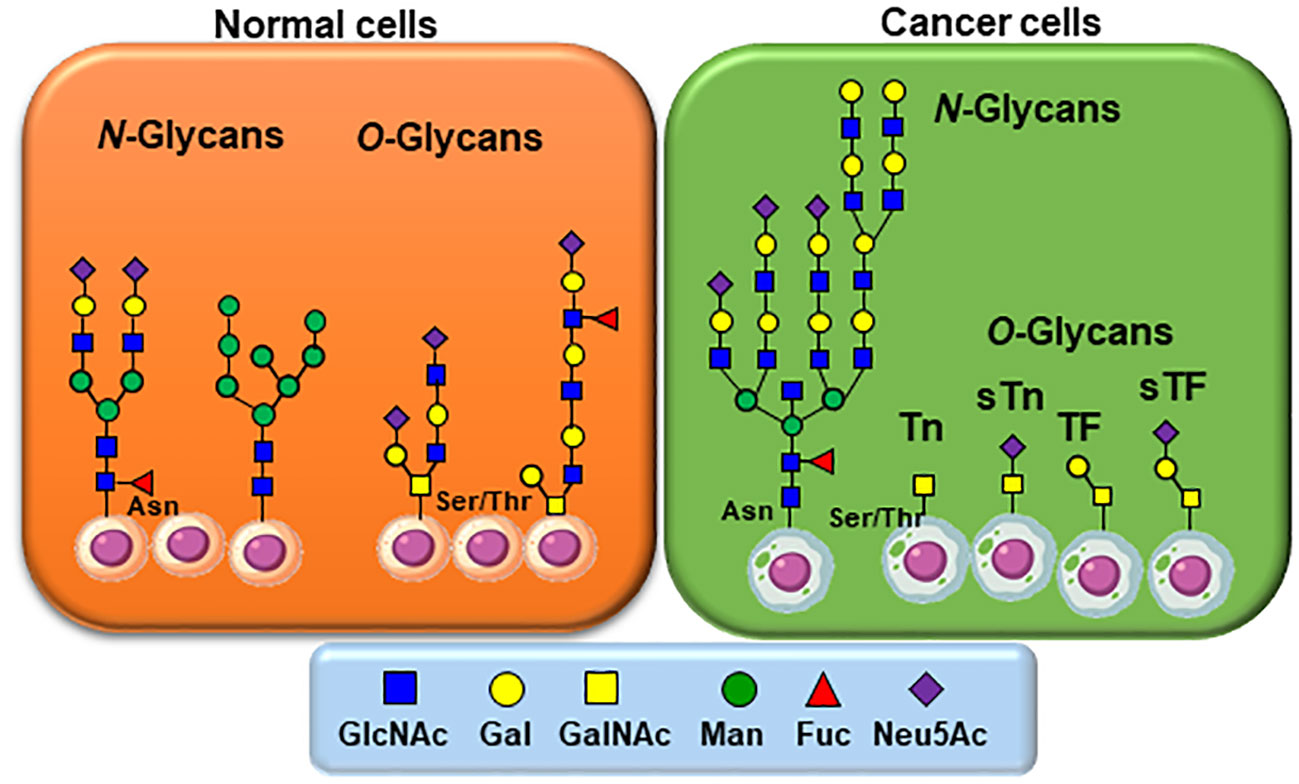
Figure 1 Typical glycan structures as they usually appear on normal and cancer cells. For N-linked glycans, altered and increased branching and/or capping by fucosylation and sialylation occurs. Of particular interest is the most simplified structural architectures of O-linked glycans in comparison to those identified on healthy cells. N-linked carbohydrates on glycoproteins are built from β-N-acetylglucosamine (GlcNAc) attached to asparagine (Asn) while O-linked glycoproteins are initiated by an α-N-acetylgalactosamine (GalNAc) to either Serine or Threonine (Ser/Thr).
N-linked carbohydrates on glycoproteins are built in three key steps from a lipid-linked (dolichol-phosphate) α-N-acetylglucosamine (GlcNAc) residue to which multiple saccharide chains are attached for transfer, followed by a transfer to nascent proteins through attachment to asparagine residues via an inverted β-GlcNAc linkage, and final processing of complex glycan chains (19, 20). Sialylated Lewis X, over sialylated glycans (21), together with the most common polylactosamine elongation, represent typical members of this class of TACAs (22).
The biosynthesis of O-linked glycans, pertinent to this report and their relation to viruses, are rather initiated by an α-N-acetylgalactosamine (GalNAc) linked to either Serine or Threonine (GalNAc-Ser/Thr) that is also a post-translational modification that occurs after the protein has been synthesized. Overall and in both cases, N-/O-glycosylation is the most commonly occurring post-translational protein modifications. A malfunctioning in glycan processing is due to the lack of key glycosyltransferases activities, mutations, over expressions, or modifications of the requisite chaperone for functional folding. Alteration of several types of O-glycan core structures has been widely implicated in multiple forms of cancers (Figure 2) (23–28). Approximately twenty GalNAc-transferase isoenzymes (GalNAc-Ts) have been identified in this initial key step (29). They are organized into nine subfamilies according to the similarity of their sequences. GalNAc-Ts select their sites of O-linked glycosylation depending on several structural differentiations of the peptide sequences. The biosynthesis of the ensuing abnormally O-glycosylated proteins is schematically illustrated in Figure 2. Elongation to core-2 glycans is prohibited by loss of an important β-GlcNAc transferase activity, resulting in buildup of the core-1 disaccharide, also known as the Thomsen Friedenreich (TF) antigen, Galβ1-3GalNAcα1-Ser/Thr. Biosynthesis of the TF antigen is through an essential C1βGal transferase, whose activity is dependent on the molecular chaperone called Cosmc. Mutation of Cosmc halts mucin type glycosylation at a single α-GalNAc residue, also known as the Thomsen nouveau or Tn antigen. Consequently, the buildup of the so-called O-linked monosaccharide Tn antigen (GalNAcα1-Ser/Thr) is overexpressed. The over expression of the ensuing Thomsen–Friedenreich disaccharide antigen (Galβ1-3GalNAcα1-Ser/Thr) (TF) is the consequence of other glycosyl transferases lack of integrity (30).
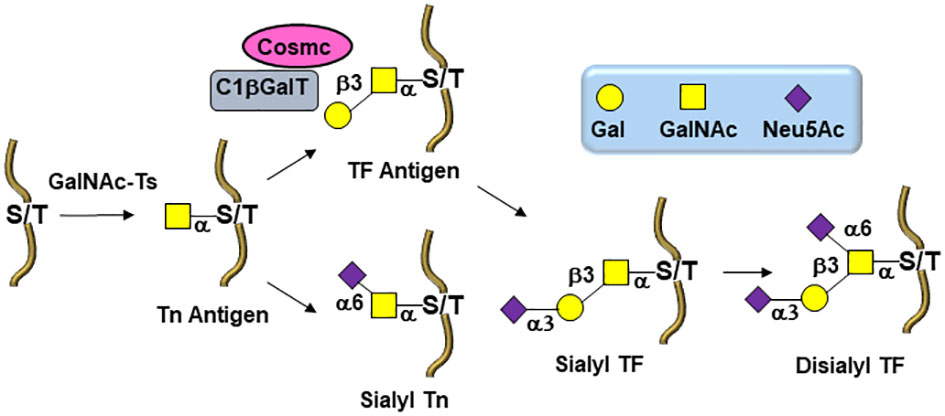
Figure 2 Biosynthetic pathways leading to O-linked tumor-associated carbohydrate antigens (TACAs) in tumor cells.
Moreover, overexpression of α2,6- and α2,3-sialyl transferases result with the accumulation of the sialylated forms of the above two antigens resulting in 5 predominantly expressed TACAs structures illustrated in Figure 3: Tn, TF, sTn, (2,3)-sTF, and (2,3)-, (2,6)-di-sTF. These TACAs are usually present on 90% of carcinomas (31) and have been proposed as key glycoepitopes in various forms of cancer (32–35).
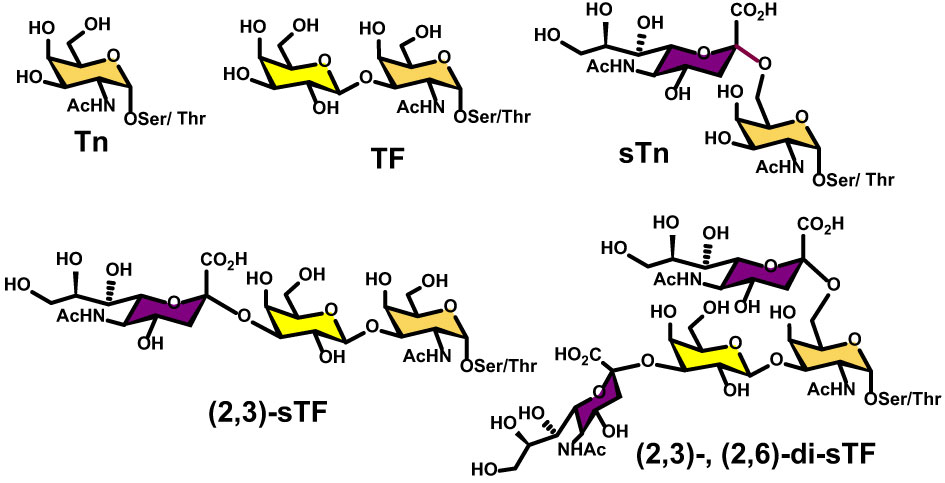
Figure 3 Structural details of the most common O-linked glycans present on both tumor cells and virus envelop glycoproteins.
3 Glycan structures on enveloped viruses
Comprehensive identification, structural characterization, location, and impact of glycosylation on virus biology triggered new strategies to treat viral infections and has an increasing contribution on widespread vaccine design (36, 37). Documentation of consensus glycoepitopes in native viral glycoproteins and tumor cells is particularly useful in the conception of shared vaccination approaches (38).
Viral protein glycosylation is a pervasive post-translational modification that is responsible for virus protective shielding, host cell targeting, adhesion, and spreading (37). As obligate parasites such as some plants, fungi, and bacteria, viruses also exploit host-cell metabolic machinery to glycosylate their own proteins during replication (Figure 4) (36–39). Viral envelope glycoproteins from a variety of human pathogens, including influenza virus (40, 41), HIV-1 (42–44), Lassa virus (45), coronavirus (46–48), Zika virus (49), Dengue virus (50, 51), Ebola virus (52, 53), human respiratory syncytial virus (hRSV) (54), and more recently SARS-CoV-2 (55–58), have progressed to have been shown to be broadly glycosylated by both N- and O-linked glycoepitopes. These host cell-derived glycoforms facilitate diverse structural and functional roles during the viral life-cycle mostly related to immune evasion since these extensive glycosylation sites usually mask peptide sequences that would be otherwise useful targets for vaccine design (38, 39, 59). Importantly, whether the envelope glycoproteins of most of these viruses are expressed in human cells, their expression into other vectors lead to identical glycoform patterns (60).
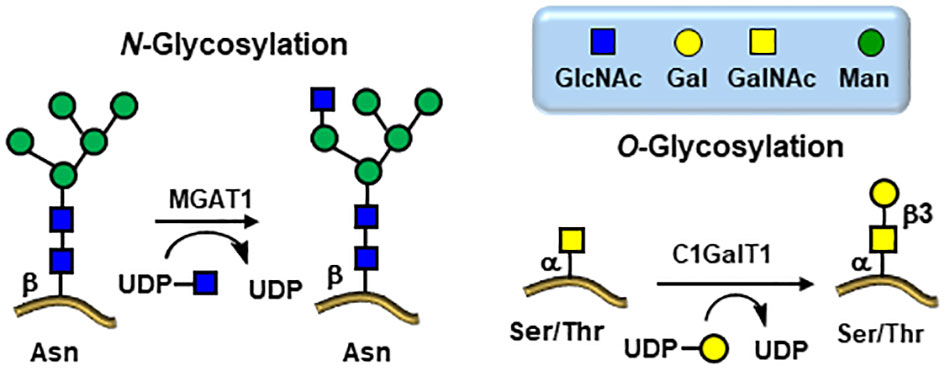
Figure 4 Viral glycosylation pathways hijacked from the infected host cells. Knock down MGAT1 and C1GalT1 glycosyltransferases inhibits viral entry.
Comprehensive structural investigations of the glycoepitopes of viral envelope glycoproteins have been investigated for a long time, particularly for N-linked glycans whose structural analysis were simpler (36–39). However, given the inherent technical difficulties encountered for the structural analysis of O-linked glycans, detailed studies of the latter have lagged behind (61, 62). Mass spectrometry has played and continues to play a vibrant role in chemical characterizations of both N- and O-linked glycans amongst glycomic and glycoproteomic analytical methodologies (62). As seen in Figure 5, N-glycans of enveloped viruses are so abundant that they clearly masked key amino acid epitopes (39), thus preventing the efficacy of several anti-peptide antibody approaches generated through common vaccinations of glycoproteins of enveloped viruses: human coronavirus: HKU1 S, SARS S, MERS S; Lassa virus glycoproteins (LASV GPC); influenza virus (H3N2); human immunodeficiency viruses (HIV-1; simian immunodeficiency viruses (SIV). Analogously to TACAs expressed on cancer cells, viral protein glycosylation patterns follow the same trends with typical fucosylation, sialylation, branching, and polylactosaminylation (22, 63, 64) in addition to their simplified O-linked glycan patterns.
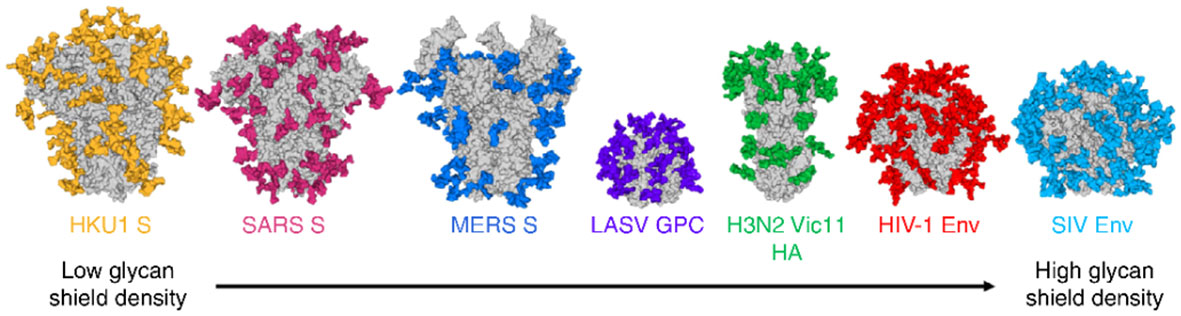
Figure 5 Typical high shielding effects of N-linked glycoepitopes (colored) of common viral envelope glycoproteins (the illustration shows mainly the N-glycosylation sites modeled on the Man5GlcNAc2 motif anchoring glycans). Reproduced with permission from reference (39).
Glycan-Binding Proteins (Lectins) in Antiviral Therapies: The Envelope Glycoproteins of Influenza Virus.
Lectins, i. e. carbohydrate-binding proteins of non-immune origin, play several key roles in biological processes, including innate immunity (65–67). In this regard, lectins act as pattern recognition receptors (PRRs) that can bind pathogen-associated molecular patterns (PAMPs). Consequently, like antibodies of the adaptative immune system, lectins greatly contribute to the protective immune responses. The major classes of lectins in innate immunity are represented by C-type lectin receptors (ex. Dectin-1, Langerin, Mincle, Ficolins, Dendritic Cell-Specific ICAM-3 Grabbing Integrin (DC-SIGN, L-SIGN, Mannose-Binding Protein (MBP), Galectins, and Siglecs (65–67). Alternatively, several leguminous lectins are also capable of binding glycans on both cancer cells and viruses (68).
The envelope glycoproteins of influenza viruses are mainly represented by their hemagglutinins (HA) and neuraminidases (NA) (69). Their N-glycosylation by the host machinery implies, like all other viruses, the attachment of oligosaccharides to the side-chain amide Asn at the Asn-X-Ser\Thr sequon, where X can be any amino acid except proline (70). The resulting glycan sequences involves high-mannose oligosaccharides, galactose and/or N-acetyl galactosamine/fucose (complex glycans) or a combination there of (hybrid glycans) (Figures 1, 5). Consequently, typical lectins implicated in the innate immunity can bind, trigger viral neutralization, and prime the immune system. Unlike most antibodies, these lectins can bind to a wide range of influenza strains, implying that they would be attractive candidates for antiviral (“immuno’’) therapies (65, 67, 68, 71).
As mentioned above, most enveloped viral glycoproteins express the typical glycan structures detected on cancer cells. As such, they are inherently recognized by the endogenous lectins of the innate immune system with mannosides, galactosides, and sialic acid-ending glycans being the major players. Several such viral glycoproteins can also bind to leguminous lectins (Table 1). Thus, in spite of historically inadequate analytical mass spectrometry tools, leguminous lectins, together with C-type lectins of the innate immunity system have played central roles in the structural elucidation of glycans on viruses. The high mannose oligosaccharide (Man9GlcNAc2) of HIV-1 gp120 glycoprotein is highly representative of noteworthy efforts directed at generating glycoconjugate anti-viral vaccines (79).
4 Detailed O-Linked glycans of the spike glycoproteins of SARS-CoV-2
The scarcity of O-linked glycans on viral glycoproteins coupled with early difficulties in detailed structural analytical methodologies have impeded the progress of their detailed compositional and positional analyses (61, 62). However, the global pandemic of the SARS-CoV-2 in 2019 (80) has triggered tremendous efforts toward the discoveries of a wide range of new therapeutics strategies leading to investigations toward several potential targets, including glycan structures, and particularly in the field of vaccines (81, 82). The virion particles of SARS-CoV-2 are 91 ± 11 nm wide and covered with approximately 24 highly glycosylated fusion S-proteins which contain the binding site for its host cell receptor (RBD) – the angiotensin-converting enzyme 2 (ACE2) (Figures 6, 7) (83). Further evidences for the key roles of proteins glycosylation on viruses were illustrated by the knocking out of two key enzymes responsible for the build-up of both N- and O-linked glycan biosynthesis (84). Yang et al. showed that the SARS-CoV-2 viral entry mechanism was inhibited by blocking α-1,3-mannosyl-glycoprotein 2-β-N-acetylglucosaminyl transferase (MGAT1) and the core 1 synthase, glycoprotein-N-acetylgalactosamine 3-β-galactosyltransferase 1 (C1GALT1) enzymes (Figure 4) using CRSP-Cas9 knockout cells (84).
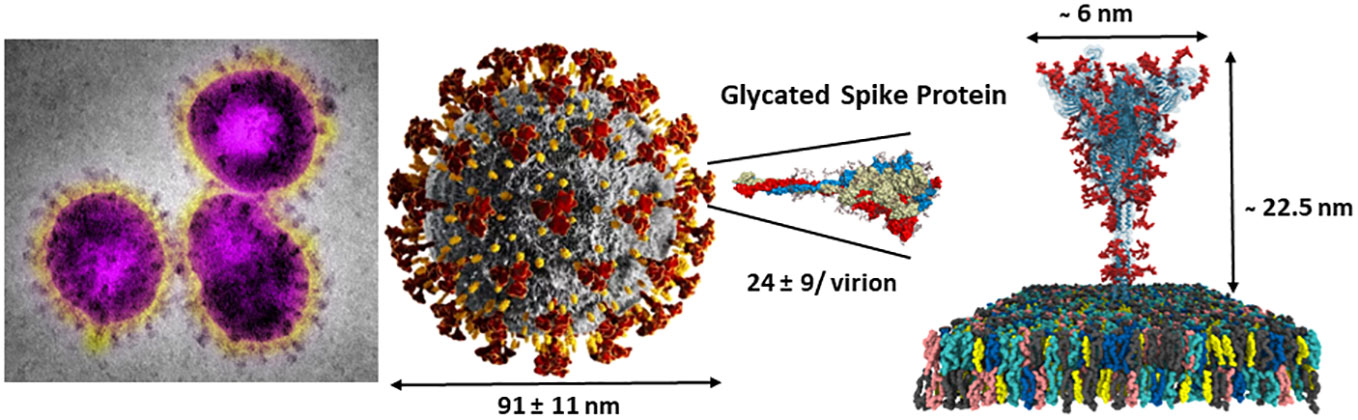
Figure 6 SARS-CoV-2 virus nanoparticles are coated by a large number of trimeric spike glycoproteins shielded by several N-linked glycans (red) and a few O-linked glycoepitopes (not shown) (see Figure 7 for details). Right adapted from: https://charmm-gui.org/?doc=archive&lib=covid19.
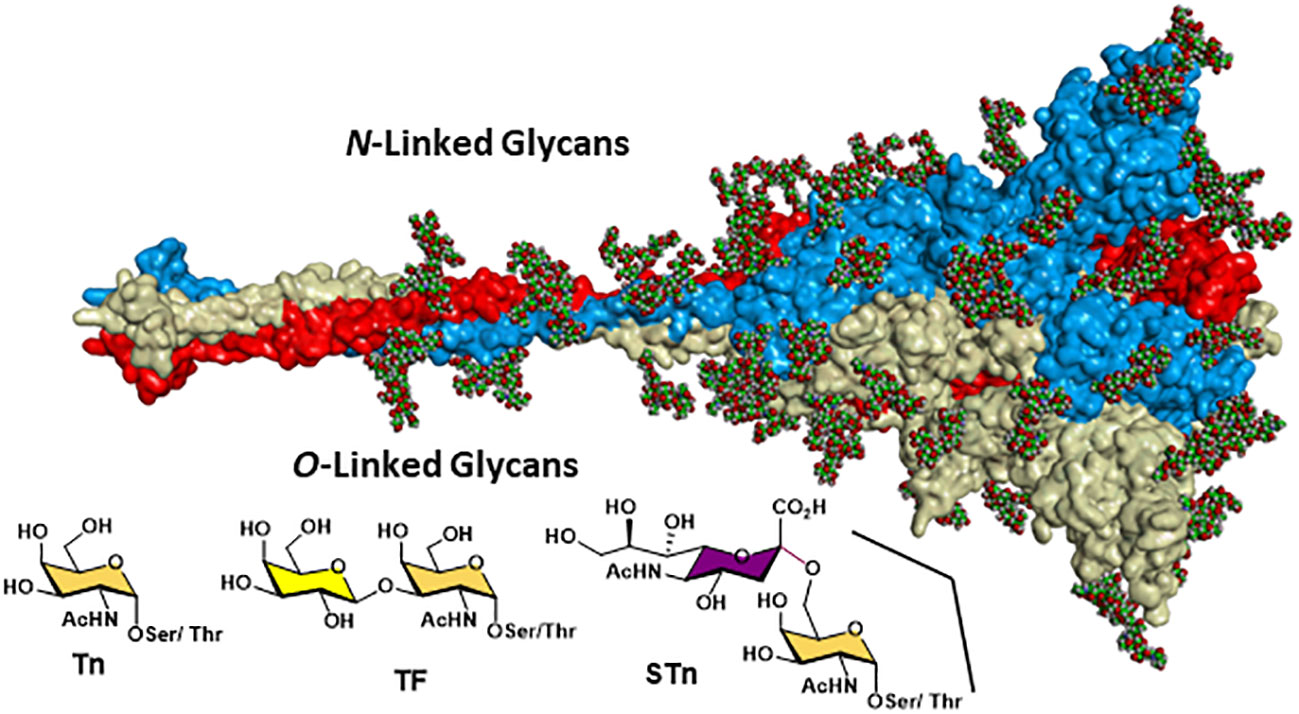
Figure 7 SARS-CoV-2 spike protein showing the dense shielding effect of the N-linked glycans (as CPK balls) and the region where the O-linked TACAs have been clearly identified using high-resolution LC-MS/MS 320VQPT*ES*IVR328, adapted from https://charmm-gui.org/?doc=archive&lib=covid19.
Initially, it has been strongly speculated that sialic acid-ending residues acted as auxiliary co-receptor (85, 86), thus opening the door to sialic acid-based anti-adhesion inhibitors (87). More recently, sialylated glycolipids (gangliosides) have now been more accurately identified as the key natural host co-receptors (88). The heavily N-glycosylated spike protein of SARS-CoV-2 masked potentially immunogenic peptide sequences by forming dense shielding (see Figures 5, 6).
Detailed site-specific glycan profiling of the betacoronavirus SARS-CoV-2 spike (S) glycoprotein has unraveled 17 N-glycan chains per monomer out of the 22 potential sites (55–58) thus covering 42% of the surface potentially accessible toward antibodies, much less than the surface covered by the glycans on HIV-1. The complete N-glycoform mapping has been thoroughly investigated using high-resolution LC-MS/MS (58). The same investigation (58) unambiguously revealed that the O-glycoforms were clearly identified at sites Thr323 and Ser 325, near the hinge region of the receptor-binding domain (RBD) domain of the S1 protein. Remarkably, the usual O-linked TACAs identified on tumor cells were also identified (Tn, TF, sTn, (2,3)-sTF, and (2,3)-, (2,6)-di-sTF) (Figures 3, 7), together with some more elaborated chains. The presence of additional O-glycans at Ser673, Thr678, and Ser686 has also been postulated based on glycoinformatics (89). Deeper insights into the chemical details of the SARS-CoV-2 architectures with respect to the shielding of the glycans illustrate the effects of the carbohydrate glycoforms and the relative positioning of the TACAs of interest (90–94).
5 Joint strategies in the design of both TACAs and viruses therapeutic agents
As indicated, TACAs have long been identified on viral glycoproteins (36–39). Therefore, using viral carbohydrates as targets for neutralizing antibodies is offering several advantages for group-specific vaccine development since this approach could alleviate viral mutations commonly and rapidly occurring as it has been witnessed with the SARS-CoV-2 pandemic. One of the early reports of the presence of TACAs (sTn) on enveloped viruses has been reported on the gp120 of HIV isolates and anti-sTn antibodies were shown to neutralize infection of lymphocytes (95). Later, the same group also demonstrated that the related anti-Tn monoclonal antibodies (Mab) IgG2a (1E3) and an IgM (TKH6) inhibited both HIV-1 and HIV-2 infection in vitro (96). The antibodies showed a dose-dependent inhibition of viral infections together with syncytium formation in cultures inoculated with free virus. In addition, the infection was not prevented when synthetic α-GalNAc-Ser hapten was pre-incubated with the Mab 1E3 used to block the interaction. These findings are very relevant to our postulate since the presence of other O-linked glycans have been identified on other retroviral envelope glycoproteins (97).
Importantly, it is well documented that human serum contains substantial amounts of a wide range of anti-carbohydrate antibodies. These have been identified using modern glycan microarrays (98–100) and has been postulated to originate from prior exposures of humans to various pathogenic agents, including viruses (101) and their relative contents and specificities depends on age and ethnicity (102). Interestingly, anti-TACAs antibodies were identified in the sera of healthy individuals (103) and it has been hypothesized that such antibodies might confer protection against COVID-19, as shown with the anti-Tn glycoepitope mentioned above (104). The study showed that lower levels of anti-Tn antibodies were specifically low in COVID-19 patients in comparison to those of the healthy group. These findings are not without precedent since the αGal glycoantigen constitute one of most extensively studied example of a carbohydrate epitope that can lead to the elimination of viruses through natural antibodies (105, 106). This glycoepitope is expressed by many cell types in most mammalian species, but is lacking in humans due to the lack of the cognate galactosyl transferase. Overall, natural anti-Tn antibodies could benefit from a natural immunity conferred by these antibodies against COVID-19 (and likely other viral infections) and corresponding vaccines would offer an attractive prophylactic perspective (Figure 8).
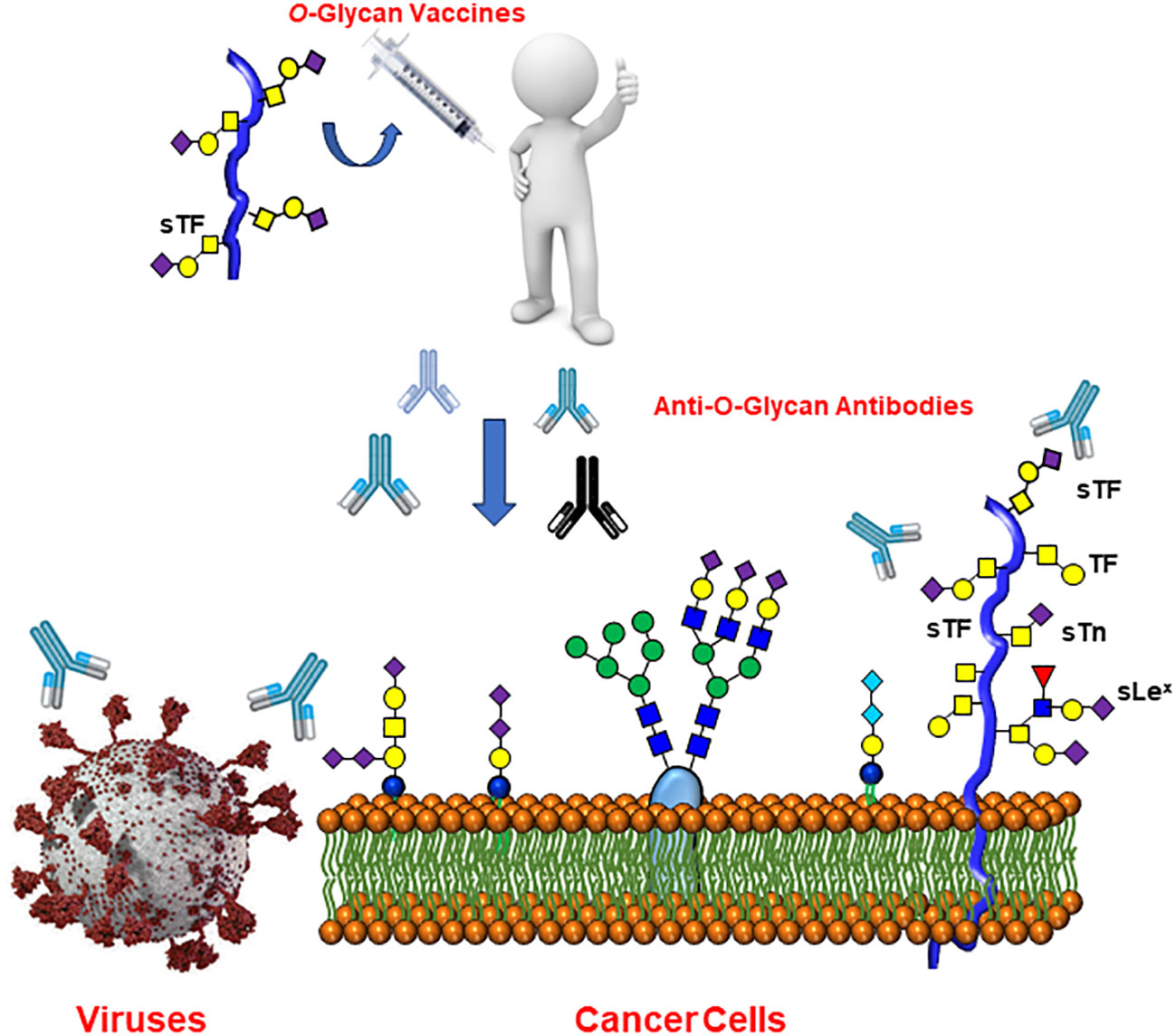
Figure 8 Raising anti-Tumor-Associated Carbohydrate Antigen (TACAs) antibodies may offer wide protection against both cancer and several forms of viral infections.
There are several other potential sources of carbohydrate-binding proteins (lectins) that could also be used against viral infections. Naturally occurring lectins from various organisms have been shown to inhibit viral entry mechanisms. These lectins are present in bacteria, plants, and marine algae (68). Lectins can block replications of viruses through binding with viral envelope glycoproteins. The molecular interactions rely on monosaccharides as well as complex branched glycans with a particular activity against high-mannose oligosaccharides as found on viral N-linked glycoproteins (Figure 4). However, the challenge in using heterologous lectins in clinical settings is limited by the complications of administrating foreign proteins into human system. Indeed, not only can they be immunogenic on their own but several lectins have displayed toxicity and mitogenic activities (107). Fortunately, a recent investigation demonstrated that a single amino acid substitution in a banana lectin (BanLec), replacing histidine 84 with a threonine, had significantly reduced its mitogenicity while preserving its broad-spectrum antiviral potency in HIV isolates (75). Interestingly, BanLec is known to be a mannose-specific lectin. In addition, studies have shown that BanLec can inhibit HIV-1 reverse transcriptase activity, suppress influenza viral fusion, and provide protective activity against herpes simplex virus (HSV) type 1 (108). Additionally, it has been observed to suppress cancer cell proliferation (109). Furthermore, and in line with the above relationships between TACAs on cancer cells and the ability of leguminous lectins to recognize tumors, a recent report described the use of BanLec CAR-T cells to target pancreatic tumors (110).
Most significantly, human innate immunity, conferred by the family of mannose-binding lectins (MBLs) such as the ficolins and the membrane-bound CD209 (DC-SIGN) similarly constitute protective activities against viral infections (67). The complement cascade of activation is also playing a key role in the initial defense mechanism against viral infections (111). These mannose-binding lectins are usually abundant in healthy human serum (1340 ng/mL) (112). Humans deficient in their serum concentrations of MBLs were shown to be more susceptible to SARS-CoV-2 infections (113).
Interestingly, non-peptidic carbohydrate binding agents (CBAs) such as pradimicin A and benanomicin A family of antibiotics produced in the actinomycetes strain Actinomadura hibisca and Actinomodura spadix, respectively, known to bind mannose-containing oligosaccharides, have also been shown to be potent inhibitors of viral infections (114, 115). They can also inhibit syncytia formation as well as DC-SIGN-mediated transmission of HIV to lymphocytes.
6 Conclusion
There are an increasing number of evidences clearly pointing toward common glycoepitopes present on both cancer cells and viral envelope glycoproteins. Several studies have established beneficial effects of monoclonal anti-carbohydrate antibodies against tumor-associated carbohydrate antigens (TACAs) as well as specific carbohydrate-binding lectins to protect entry and viral replications from a wide-range of envelope viruses. This report illustrated numerous examples wherein leguminous lectins were used to identify the presence of O-linked glycans (TACAs) on a wide range of viruses. However, further evidences are required to demonstrate that antibodies equivalent to lectin-recognition domains would be as efficacious as immunoprophylactics. Therefore, this report highlights the necessity to deepen our understanding on the vaccinal applications of existing MAbs against TACAs that can be potentially and equally useful as anti-viral vaccine prophylactics (Figure 8).
Author contributions
RR: Writing – review & editing.
Funding
The author(s) declare financial support was received for the research, authorship, and/or publication of this article. This review was supported by a Discovery grant from the Natural Sciences and Engineering Research Council of Canada (NSERC, grant. No. RGPIN-2018-05570) and by the Programme de recherche CNRST-FRQ (Maroc-Québec) No. 327250.
Acknowledgments
The author acknowledged the skillful proof reading of this manuscript by Dr. Leila Mousavifar and Dr. Mukul R. Gupta.
Conflict of interest
The author declares that the research was conducted in the absence of any commercial or financial relationships that could be construed as a potential conflict of interest.
Publisher’s note
All claims expressed in this article are solely those of the authors and do not necessarily represent those of their affiliated organizations, or those of the publisher, the editors and the reviewers. Any product that may be evaluated in this article, or claim that may be made by its manufacturer, is not guaranteed or endorsed by the publisher.
References
1. Roy R, Mousavifar L. Carrier diversity and chemical ligations in the toolbox for designing tumor-associated carbohydrate antigens (TACAs) as synthetic vaccine candidates. Chem Soc Rev. (2023) 52:3353–96. doi: 10.1039/D2CS01032A.
2. Roy R. New trends in carbohydrate-based Vaccines. Drug Discovery Today: Technol. (2004) 1:327–36. doi: 10.1016/j.ddtec.2004.10.005.
3. Guo Z, Wang Q. Recent development in carbohydrate-based cancer vaccines. Curr Opin Chem Biol. (2009) 13:608–17. doi: 10.1016/j.cbpa.2009.08.010
4. Buskas T, Thompson P, Boons G-J. Immunotherapy for cancer: synthetic carbohydrate-based vaccines. Chem Commun. (2009), 5335–49. doi: 10.1039/b908664c.
5. Hevey R, Ling C-C. Recent advances in developing synthetic carbohydrate-based vaccines for cancer immunotherapies. Future Med Chem. (2012) 4:545–84. doi: 10.4155/fmc.11.193
6. Hakomori S-I. Tumor-associated carbohydrate antigens. Ann Rev Immunol. (1984) 2:103–26. doi: 10.1146/annurev.iy.02.040184.000535.
7. Hakomori S-I. Aberrant glycosylation in cancer cell membranes as focused on glycolipids: overview and perspectives. Cancer Res. (1985) 45:2405–14.
8. Groux-Degroote S, Guérardel Y, Delannoy P. Gangliosides: structures, biosynthesis, analysis, and roles in cancer. ChemBioChem. (2017) 18:1146–54. doi: 10.1002/cbic.201600705.
9. Springer GF. T and Tn, general carcinoma antigens. Science. (1984) 224:1198–206. doi: 10.1126/science.6729450.
10. Cummings RD. Stuck on sugars – how carbohydrates regulate cell adhesion, recognition, and signaling. Glycoconjugate J. (2019) 36:241–57. doi: 10.1007/s10719-019-09876-0.
12. Gabius H-J, Cudic M, Diercks T, Kaltner K, Kopitz J, Mayo KH, et al. What is the sugar code? ChemBioChem. (2022) 23:e202100327. doi: 10.1002/cbic.202100327.
13. Beckwith DM, Cudic M. Tumor-associated O-glycans of MUC1: Carriers of the glyco-code and targets for cancer vaccine design. Semin Immunol. (2020) 47:101389. doi: 10.1016/j.smim.2020.101389.
14. Costa AF, Campos D, Reis CA, Gomes C. Targeting glycosylation: A new road for cancer drug discovery. Trends Cancer. (2020) 6:757–66. doi: 10.1016/j.trecan.2020.04.002
15. da Costa V, Freire T. Advances in the immunomodulatory properties of glycoantigens in cancer. Cancers. (2022) 14:1854. doi: 10.3390/cancers14081854
16. Cheever MA, Allison JP, Ferris AS, Finn OJ, Hastings BM, Hecht TT, et al. The prioritization of cancer antigens: A national cancer institute pilot project for the acceleration of translational research. Clin Cancer Res. (2009) 15:5323–37. doi: 10.1158/1078-0432.CCR-09-0737.
17. Berois N, Pittini A, Osinaga E. Targeting tumor glycans for cancer therapy: successes, limitations, and perspectives. Cancers. (2022) 14:645. doi: 10.3390/cancers14030645
18. Julien S, Videira PA, Delannoy P. Sialyl-tn in cancer: (How) did we miss the target? Biomolecules. (2012) 2:435–66. doi: 10.3390/biom2040435.
19. Burda P, Aebi M. The dolichol pathway of N-linked glycosylation. Biochim Biophys Acta. (1999) 1426:239–57. doi: 10.1016/S0304-4165(98)00127-5.
20. Imperiali B, O’Connor SE. Effect of N-linked glycosylation on glycopeptide and glycoprotein structure. Curr Opin Chem Biol. (1999) 3:643–9. doi: 10.1016/S1367-5931(99)00021-6.
21. Taniguchi N, Kizuka Y. Glycans and cancer: role of N-glycans in cancer biomarker, progression and metastasis, and therapeutics. Adv Cancer Res. (2015) 126:11–51. doi: 10.1016/bs.acr.2014.11.001
22. Kadirvelraj R, Yang J-Y, Kim HW, Sanders JH, Moremen KW, Wood ZA. Comparison of human poly-N-acetyl-lactosamine synthase structure with GT-A fold glycosyltransferases supports a modular assembly of catalytic subsites. J Biol Chem. (2021) 296:100110. doi: 10.1074/jbc.RA120.015305
23. Kudelka MR, Ju T, Heimburg-Molinaro J, Cummings RD. Simple sugars to complex disease-mucin-type O-glycans in cancer. Glycosylation and cancer. Adv Cancer Res. (2015) 126:53–135. doi: 10.1016/bs.acr.2014.11.002
24. Brockhausen I. Pathways of O-glycan biosynthesis in cancer cells. Biochim Biophys Acta. (1999) 1473:67–95. doi: 10.1016/S0304-4165(99)00170-1.
25. Gupta R, Leon F, Rauth S, Batra SK, Ponnusamy MPA. Systematic review on the implications of O-linked glycan branching and truncating enzymes on cancer progression and metastasis. Cells. (2020) 9:446. doi: 10.3390/cells9020446
26. Remmers N, Anderson JM, Linde EM, DiMaio DJ, Lazenby AJ, Wandall HH, et al. Aberrant expression of mucin core proteins and O-linked glycans associated with progression of pancreatic cancer. Clin Cancer Res. (2013) 19:1981–93. doi: 10.1158/1078-0432.CCR-12-2662
27. Munkley J. The glycosylation landscape of pancreatic cancer (Review). Oncol Lett. (2019) 17:2569–75. doi: 10.3892/ol.2019.9885
28. Cervoni GE, Cheng JJ, Stackhouse KA, Heimburg-Molinaro J. Cummings, R. D. O-glycan recognition and function in mice and human cancers. Biochem J. (2020) 477:1541–64. doi: 10.1042/BCJ20180103
29. Daniel EJP, de las Rivas M, Lira-Navarrete F, García-García A, Hurtado-Guerrero R, Clausen H, et al. Ser and Thr acceptor preferences of the GalNAc-Ts vary among isoenzymes to modulate mucin-type O-glycosylation. Glycobiology. (2020) 30:910–22. doi: 10.1093/glycob/cwaa036
30. MacLean GD, Longenecker BM. Clinical significance of the Thomsen-Friedenreich antigen. Semin Cancer Biol. (1991) 2:433–9.
31. Kurtenkov O. Profiling of naturally occurring antibodies to the Thomsen-Friedenreich antigen in health and cancer: the diversity and clinical potential. Biomed Res Int. (2020), 9747040. doi: 10.1155/2020/9747040
32. Steentoft C, Vakhrushev SY, Joshi HJ, Kong Y, Vester-Christensen MB, Schjoldager K. T.-BJ., et al. Precision mapping of the human O-GalNAc glycoproteome through Simple Cell technology. EMBO J. (2013) 32:1478–88. doi: 10.1038/emboj.2013.79.
33. Bagdonaite I, Pallesen EM-H, Ye Z, Vakhrushev SY, Marinova IN, Nielsen MI, et al. Wandall, H. H. O-glycan initiation directs distinct biological pathways and controls epithelial differentiation. EMBO Rep. (2020) 21:e48885. doi: 10.15252/embr.201948885.
34. Wandall HH, Nielsen MAI, King-Smith S, de Haan N, Bagdonaite I. Global functions of O-glycosylation: promises and challenges in O-glycobiology. FEBS J. (2021) 288:7183–212. doi: 10.1111/febs.16148.
35. Ju T, Otto VI, Cummings RD. The Tn antigen—Structural simplicity and biological complexity. Angew Chem Int Ed. (2011) 50:1770–91. doi: 10.1002/anie.201002313.
36. Bagdonaite I, Wandall HH. Global aspects of viral glycosylation. Glycobiology. (2018) 28:443–67. doi: 10.1093/glycob/cwy021
37. Watanabe Y, Bowden TA, Wilson IA, Crispin M. Exploitation of glycosylation in enveloped virus pathobiology. BBA Gen Subj. (2019) 1863:1480–97. doi: 10.1016/j.bbagen.2019.05.012
38. Bagdonaite I, Vakhrushev SY, Joshi HJ, Wandall HH. Viral glycoproteomes: technologies for characterization and outlook for vaccine design. FEBS Lett. (2018) 592:3898–920. doi: 10.1002/1873-3468.13177.
39. Watanabe Y, Berndsen ZT, Raghwani J, Seabright GE, Allen JD, Pybus OG, et al. Vulnerabilities in coronavirus glycan shields despite extensive glycosylation. Nat Commun. (2020) 11:2688. doi: 10.1038/s41467-020-16567-0
40. Kobayashi Y, Suzuki Y. Evidence for N-glycan shielding of antigenic sites during evolution of human influenza A virus hemagglutinin. J Virol. (2012) 86:3446–51. doi: 10.1128/JVI.06147-11
41. Lee PS, Ohshima N, Stanfield RL, Yu W, Iba Y, Okuno Y, et al. Receptor mimicry by antibody F045-092 facilitates universal binding to the H3 subtype of influenza virus. Nat Commun. (2014) 5:3614. doi: 10.1038/ncomms4614
42. Cao L, Pauthner M, Andrabi R, Rantalainen K, Berndsen Z, Diedrich JK, et al. Differential processing of HIV envelope glycans on the virus and soluble recombinant trimer. Nat Commun. (2018) 9:3693. doi: 10.1038/s41467-018-06121-4
43. Struwe WB, Chertova E, Allen JD, Seabright GE, Watanabe Y, Harvey DJ, et al. Site-specific glycosylation of virion-derived HIV-1 Env is mimicked by a soluble trimeric immunogen. Cell Rep. (2018) 24:1958–1966.e5. doi: 10.1016/j.celrep.2018.07.080
44. Panico M, Bouche L, Binet D, O'Connor M-J, Rahman D, Pang P-C, et al. Mapping the complete glycoproteome of virion derived HIV-1 gp120 provides insights into broadly neutralizing antibody binding. Sci Rep. (2016) 6:32956. doi: 10.1038/srep32956
45. Sommerstein R, Flatz L, Remy MM, Malinge P, Magistrelli G, Fischer N, et al. Arenavirus glycan shield promotes neutralizing antibody evasion and protracted infection. PloS Pathog. (2015) 11:e1005276. doi: 10.1371/journal.ppat.1005276e1005276
46. Walls AC, Tortorici MA, Frenz B, Snijder J, Li W, Rey FA, et al. Glycan shield and epitope masking of a coronavirus spike protein observed by cryo-electron microscopy. Nat Struct Mol Biol. (2016) 23:899–905. doi: 10.1038/nsmb.3293
47. Xiong X, Tortorici MA, Snijder J, Yoshioka C, Walls AC, Li W, et al. Glycan shield and fusion activation of a delta coronavirus spike glycoprotein fine-tuned for enteric infections. J Virol. (2018) 92:e01628-17. doi: 10.1128/JVI.01628-17
48. Ritchie G, Harvey DJ, Feldmann F, Stroeher U, Feldmann H, Royle L, et al. Identification of N-linked carbohydrates from severe acute respiratory syndrome (SARS) spike glycoprotein. Virology. (2010) 399:257–69. doi: 10.1016/j.virol.2009.12.020
49. Fontes-Garfias CR, Shan C, Luo H, Muruato AE, Medeiros DBA, Mays E, et al. Functional analysis of glycosylation of Zika virus envelope protein. Cell Rep. (2017) 21:1180–90. doi: 10.1016/j.celrep.2017.10.016
50. Flamand M, Megret F, Mathieu M, Lepault J, Rey FA, Deubel V. Dengue virus type 1 nonstructural glycoprotein NS1 is secreted from mammalian cells as a soluble hexamer in a glycosylation-dependent fashion. J Virol. (1999) 73:6104–10. doi: 10.1128/JVI.73.7.6104-6110.1999.
51. Mondotte JA, Lozach P-Y, Amara A, Gamarnik AV. Essential role of dengue virus envelope protein N glycosylation at asparagine-67 during viral propagation. J Virol. (2007) 81:7136–48. doi: 10.1128/JVI.00116-07
52. Ritchie G, Harvey DJ, Stroeher U, Feldmann F, Feldmann H, Wahl-Jensen V, et al. Identification of N-glycans from Ebola virus glycoproteins by matrix-assisted laser desorption/ionization time-of-flight and negative ion electrospray tandem mass spectrometry. Rapid Commun Mass Spectrom. (2010) 24:571–85. doi: 10.1002/rcm.4410
53. Lee JE, Fusco ML, Hessell AJ, Oswald WB, Burton DR, Saphire EO. Structure of the Ebola virus glycoprotein bound to an antibody from a human survivor. Nature. (2008) 454:177–82. doi: 10.1038/nature07082
54. McLellan JS, Ray WC, Peeples ME. Structure and function of RSV surface glycoproteins. Curr Top Microbiol Immunol. (2013) 372:83–104. doi: 10.1007/978-3-642-38919-1_4
55. Watanabe Y, Allen JD, Wrapp D, McLellan JS, Crispin M. Site-specific glycan analysis of the SARS-CoV-2 spike. Science. (2020) 369:330–33. doi: 10.1126/science.abb9983
56. Grant OC, Montgomery D, Ito K, Woods RJ. 3D Models of glycosylated SARS-CoV-2 spike protein suggest challenges and opportunities for vaccine development. doi: 10.1101/2020.04.07.030445.
57. Grant OC, Montgomery D, Ito K, Woods RJ. Analysis of the SARS−CoV−2 spike protein glycan shield reveals implications for immune recognition. Sci Rep. (2020) 10:14991. doi: 10.1038/s41598-020-71748-7
58. Shajahan A, Supekar NT, Gleinich AS, Azadi P. Deducing the N- and O- glycosylation profile of the spike protein of novel coronavirus SARS-CoV-2. Glycobiology. (2020) 30:981–8. doi: 10.1093/glycob/cwaa042
59. Li Y, Liu D, Wang Y, Su W, Liu G, Dong W. The importance of glycans of viral and host proteins in enveloped virus infection. Front Immunol. (2021) 12:638573. doi: 10.3389/fimmu.2021.638573
60. Bagdonaite I, Thompson AJ, Wang X, Søgaard M, Fougeroux C, Frank M, et al. Site-specific O-glycosylation analysis of SARS-CoV-2 spike protein produced in insect and human cells. Viruses. (2021) 13:551. doi: 10.3390/v13040551
61. Harvey DJ. Proteomic analysis of glycosylation: structural determination of N- and O-linked glycans by mass spectrometry. Expert Rev Proteomics. (2005) 2:87–101. doi: 10.1586/14789450.2.1.87
62. North SJ, Hitchen PG, Haslam S, Dell A. Mass spectrometry in the analysis of N- and O-linked glycans. Curr Opin Struct Biol. (2009) 19:498–506. doi: 10.1016/j.sbi.2009.05.005
63. Grzesik K, Janik M, Hoja-Łukowicz D. The hidden potential of glycomarkers: Glycosylation studies in the service of cancer diagnosis and treatment. BBA – Rev Cancer. (2023) 1878:188889. doi: 10.1016/j.bbcan.2023.188889.
64. Kremsreiter SM, Kroell A-SH, Weinberger K, Boehm H. Glycan–lectin interactions in cancer and viral infections and how to disrupt them. Int J Mol Sci. (2021) 22:10577. doi: 10.3390/ijms221910577.
65. Mayer S, Raulf M-K. Lepenies, B. C-type lectins: their network and roles in pathogen recognition and immunity. Histochem Cell Biol. (2017) 147:223–37. doi: 10.1007/s00418-016-1523-7.
66. Lepenies B, Lang R. Editorial: lectins and their ligands in shaping immune responses. Front Immunol. (2019) 10:2379. doi: 10.3389/fimmu.2019.02379.
67. Mason CP, Tarr AW. Human lectins and their roles in viral infections. Molecules. (2015) 20:2229–71. doi: 10.3390/molecules20022229
68. Mitchell CA, Ramessar K, O'Keefe BR. Antiviral lectins: Selective inhibitors of viral entry. Antiviral Res. (2017) 142:37e54. doi: 10.1016/j.antiviral.2017.03.007
69. Swarts BM, Guo Z. Carbohydrate-based antiviral vaccines. In: Guo Z, Boons G-J, editors. Carbohydrate-Based Vaccines and Immunotherapies, New York, USA: J. Wiley & Sons Inc., (2009). p. 167–93. doi: 10.1002/9780470473283.ch5
70. Ward CW, Dopheide TA. Amino acid sequence and oligosaccharide distribution of the haemagglutinin from an early Hong Kong influenza virus variant A/Aichi/2/68 (X-31). Biochem J. (1991) 193:953–62. doi: 10.1042/bj1930953.
71. York IA, Stevens J, Alymova IV. Influenza virus N-linked glycosylation and innate immunity. Biosci Rep. (2018) 39:BSR20171505. doi: 10.1042/BSR20171505.
72. Rawling J, Melero J. The use of monoclonal antibodies and lectins to identify changes in viral glycoproteins that are influenced by glycosylation: the case of human respiratory syncytial virus attachment (G) glycoprotein. Methods Mol Biol. (2007) 379:109–25. doi: 10.1073/pnas.0712326105
73. Collins PL, Mottet G. Oligomerization and post-translational processing of glycoprotein G of human respiratory syncytial virus: altered O-glycosylation in the presence of brefeldin A. J Gen Virol. (1992) 73:849–63. doi: 10.1099/0022-1317-73-4-849.
74. Vanover D, Smith DV, Blanchard EL, Alonas E, Kirschman JL, Lifland AW, et al. RSV glycoprotein and genomic RNA dynamics reveal filament assembly prior to the plasma membrane. Nat Commun. (2017) 8:667. doi: 10.1099/0022-1317-73-4-849
75. Swanson MD, Boudreaux DM, Salmon L, Chugh J, Winter HC, Meagher JL, et al. Engineering a therapeutic lectin by uncoupling mitogenicity from antiviral activity. Cell. (2015) 163:746–58. doi: 10.1016/j.cell.2015.09.056
76. Liu L, Bastien N, Li Y. Intracellular processing, glycosylation, and cell surface expression of human metapneumovirus attachment glycoprotein. J Virol. (2007) 81:13435–43. doi: 10.1128/JVI.01469-07
77. Lenza MP, Oyenarte I, Diercks T, Quintana JI, Gimeno A, Coelho H, et al. Structural characterization of N-linked glycans in the receptor binding domain of the SARS-CoV-2 spike protein and their interactions with human lectins. Angew Chem Int Ed Eng. (2020) 59:23763–71. doi: 10.1002/anie.202011015.
78. Casals C, Campanero-Rhodes MA, García-Fojeda B, Solís D. The role of collectins and galectins in lung innate immune defense. Front Immunol. (2018) 9:1998. doi: 10.3389/fimmu.2018.01998
79. Wang S-K, Liang P-H, Astronomo RD, Hsu T-L, Hsieh S-L, Burton DR, et al. Targeting the carbohydrates on HIV-1: Interaction of oligomannose dendrons with human monoclonal antibody 2G12 and DC-SIGN. Proc Natl Acad Sci USA. (2008) 105:3690–5. doi: 10.1073/pnas.0712326105.
80. Hozhabri H, Sparascio FP, Sohrabi H, Mousavifar L, Roy R, Scribano D, et al. The global emergency of novel coronavirus (SARS-CoV-2): an update of the current status and forecasting. Int J Environ Res Public Health. (2020) 17:5648. doi: 10.3390/ijerph17165648
81. Tenchov R, Bird R, Curtze AE, Zhou Q. Lipid nanoparticles-from liposomes to mRNA vaccine delivery, a landscape of research diversity and advancement. ACS Nano. (2021) 15:16982–7015. doi: 10.1021/acsnano.1c04996.
82. Feng T, Zhang J, Chen Z, Pan W, Chen Z, Yan Y, et al. Glycosylation of viral proteins: Implication in virus–host interaction and virulence. Virulence. (2022) 13:670–83. doi: 10.1080/21505594.2022.2060464
83. Ke Z, Oton J, Qu K, Cortese M, Zila V, McKeane L, et al. Structures and distributions of SARS-CoV-2 spike proteins on intact virions. Structures and distributions of SARS-CoV-2 spike proteins on intact virions. Nature. (2020) 588:498–502. doi: 10.1038/s41586-020-2665-2
84. Yang Q, Hughes TA, Kelkar A, Yu X, Cheng K, Park S, et al. Inhibition of SARS-CoV-2 viral entry upon blocking N- and O-glycan elaboration. eLife. (2020) 9:e61552. doi: 10.7554/eLife.61552
85. Sun X-L. The role of cell surface sialic acids for SARS-CoV-2 infection. Glycobiology. (2021) 31:1245–53. doi: 10.1093/glycob/cwab032
86. Li B, Wang L, Ge H, Zhang X, Ren P, Guo Y, et al. Identification of potential binding sites of sialic acids on the RBD domain of SARS-CoV-2 spike protein. Front Chem. (2021) 9:65976. doi: 10.3389/fchem.2021.65976
87. Petitjean SJL, Chen W, Koehler M, Jimmidi R, Yang J, Mohammed D, et al. Multivalent 9-O-Acetylated-sialic acid glycoclusters as potent inhibitors for SARS-CoV-2 infection. Nat Commun. (2022) 13:2564. doi: 10.1038/s41467-022-30313-8
88. Nguyen L, McCord KA, Bui DT, Bouwman KM, Kitova EN, Elaish M, et al. Sialic acid-containing glycolipids mediate binding and viral entry of SARS-CoV-2. Nat Chem Biol. (2022) 18:81–90. doi: 10.1038/s41589-021-00924-1.
89. Zhang Y, Zhao W, Mao Y, Chen Y, Zheng S, Cao W, et al. O-glycosylation landscapes of SARS-CoV-2 spike proteins. Front Chem. (2021) 9:689521. doi: 10.3389/fchem.2021.689521
90. Woo H, Park S-J, Choi YK, Park T, Tanveer M, Cao Y, et al. Developing a fully glycosylated full-length SARS-CoV−2 spike protein model in a viral membrane. J Phys Chem B. (2020) 124:7128–37. doi: 10.1021/acs.jpcb.0c04553
91. Cao Y, Choi YK, Frank M, Woo H, Park S-J, Yeom MS, et al. Dynamic interactions of fully glycosylated SARS-CoV-2 spike protein with various antibodies. J Chem Theory Comput. (2021) 17:6559–69. doi: 10.1021/acs.jctc.1c00552.
92. Joshi HJ, Jørgensen A, Schjoldager KT, Halim A, Dworkin LA, Steentoft C, et al. GlycoDomainViewer: a bioinformatics tool for contextual exploration of glycoproteomes. Glycobiology. (2018) 28:131–6. doi: 10.1093/glycob/cwx104
93. Yao H, Song Y, Chen Y, Wu N, Xu J, Sun C, et al. Molecular architecture of the SARS-CoV-2 virus. Cell. (2020) 183:730–8. doi: 10.1016/j.cell.2020.09.018.
94. The readers are invited to consult the following COVID-19 protein library for building models from which Figure 7 was generated. Available online at: https://charmm-gui.org/?doc=archive&lib=covid19.
95. Hansen J-ES, Clausen H, Nielsen C, Teglbjierg LS, Hansen LL, Nielsen CM, et al. Inhibition of human immunodeficiency virus (HIV) infection in vitro by anti-carbohydrate monoclonal antibodies: peripheral glycosylation of HIV envelope glycoprotein gpl20 may be a target for virus neutralization. J Virol. (1990) 64:2833–40. doi: 10.1128/jvi.64.6.2833-2840.1990.
96. Hansen J-ES, Nielsen C, Arendrup M, Olofsson S, Mathiesen L, Nielsen JO, et al. Broadly neutralizing antibodies targeted to mucin-type carbohydrate epitopes of human immunodeficiency virus. J Virol. (1991) 65:6461–7. doi: 10.1128/jvi.65.12.6461-6467.1991.
97. Pinter A, Honnen WJ. O-linked glycosylation of retroviral envelope gene products. J Virol. (1988) 62:1016–21. doi: 10.1128/jvi.62.3.1016-1021.1988.
98. Park S, Gildersleeve JC, Blixt O, Shin I. Carbohydrate microarrays. Chem Soc Rev. (2013) 42:4310–26. doi: 10.1039/C2CS35401B
99. Durbin SV, Wright WS, Gildersleeve JC. Development of a multiplex glycan microarray assay and comparative analysis of human serum anti-glycan IgA, IgG, and IgM repertoires. ACS Omega. (2018) 3:16882–91. doi: 10.1021/acsomega.8b02238.
100. Mehta AY, Heimburg-Molinaro J, Cummings RD. Tools for generating and analyzing glycan microarray data. Beilstein J Org Chem. (2020) 16:2260–71. doi: 10.3762/bjoc.16.187
101. Muthana SM, Gildersleeve JC. Glycan microarrays: powerful tools for biomarker discovery. Cancer Biomarkers. (2014) 14:29–41. doi: 10.3233/CBM-130383
102. Luetscher RND, Mckitrick TR, Gao C, Mehta AY, Mcquillan AM, Kardish R, et al. Heimburg-Molinaro, J.; von Gunten, S.; Alter, G.; Cummings, R. D. Unique repertoire of anti-carbohydrate antibodies in individual human serum. Sci Rep. (2020) 10:15436. doi: 10.1038/s41598-020-71967-y.
103. Huflejt ME, Vuskovic M, Vasiliu D, Xu H, Obukhova P, Shilova N, et al. Anti-carbohydrate antibodies of normal sera: Findings, surprises and challenges. Mol Immunol. (2009) 46:3037–49. doi: 10.1016/j.molimm.2009.06.010.
104. Breiman A, Ruvoën-Clouet N, Deleers M, Beauvais T, Jouand N, Rocher J, et al. Low levels of natural anti-a-N-acetylgalactosamine (Tn) antibodies are associated with COVID-19. Front Microbiol. (2021) 12:641460. doi: 10.3389/fmicb.2021.641460
105. Galili U. Evolution in primates by “Catastrophic-selection” interplay between enveloped virus epidemics, mutated genes of enzymes synthesizing carbohydrate antigens, and natural anticarbohydrate antibodies. Am J Phys Anthropol. (2019) 168:352–63. doi: 10.1002/ajpa.23745
106. Galili U. Human natural antibodies to mammalian carbohydrate antigens as unsung heroes protecting against past, present, and future viral infections. Antibodies (Basel). (2020) 9:25. doi: 10.3390/antib9020025
107. Kilpatrick DC. Mechanisms and assessment of lectin-mediated mitogenesis. Mol Biotechnol. (1999) 11:55–65. doi: 10.1007/bf02789176
108. Hessel SS, Dwivany FM, Zainuddin IM, Wikantika K, Celik I, Emran TB, et al. A computational simulation appraisal of banana lectin as a potential anti-SARS-CoV-2 candidate by targeting the receptor-binding domain. J Genet Eng Biotechnol. (2023) 21:148. doi: 10.1186/s43141-023-00569-8
109. Srinivas BK, Shivamadhu MC, Jayarama S. Musa acuminata lectin exerts anti-cancer effects on HeLa and EAC cells via activation of caspase and inhibitions of Akt, Erk, and Jnk pathway expression and suppresses the neoangiogenesis in in-vivo models. Int J Biol Macromol. (2020) 166:1173–87. doi: 10.1016/j.ijbiomac.2020.10.27
110. McKenna MK, Ozcan A, Brenner D, Watanabe N, Legendre M, Thomas DG, et al. Novel banana lectin CAR-T cells to target pancreatic tumors and tumor-associated stroma. J ImmunoTher Cancer. (2023) 11:e005891. doi: 10.1136/jitc-2022-005891
111. Sarma JV, Ward PA. The complement system. Cell Tissue Res. (2011) 343:227–35. doi: 10.1007/s00441-010-1034-0.
112. Harrison E, Singh A, Morris J, Smith NL, Fraczek MG, Moore CB, et al. Mannose binding lectin genotype and serum levels in patients with chronic and allergic pulmonary aspergillosis. Int J Immunogenet. (2012) 39:224–32. doi: 10.1111/j.1744-313X.2011.01078.x
113. Gupta A, Gupta GS. Status of mannose-binding lectin (MBL) and complement system in COVID-19 patients and therapeutic applications of antiviral plant MBLs. Molec Cell Biochem. (2021) 476:2917–42. doi: 10.1007/s11010-021-04107-3
114. Balzarini J. Carbohydrate-binding agents: a potential future cornerstone for the chemotherapy of enveloped viruses? Antivir Chem Chemother. (2007) 18:1–11. doi: 10.1177/095632020701800101.
Keywords: O-glycans, vaccines, cancer, viruses, glycobiology, glycoepitopes, tumor associated carbohydrate antigens (TACAs), SARS-CoV-2
Citation: Roy R (2024) Cancer cells and viruses share common glycoepitopes: exciting opportunities toward combined treatments. Front. Immunol. 15:1292588. doi: 10.3389/fimmu.2024.1292588
Received: 11 September 2023; Accepted: 06 February 2024;
Published: 01 March 2024.
Edited by:
Tao Jiang, Shanghai Pulmonary Hospital, ChinaReviewed by:
Bernd Lepenies, University of Veterinary Medicine Hannover, GermanyPumtiwitt McCarthy, Morgan State University, United States
Joseph John Barchi, National Cancer Institute (NIH), United States
Copyright © 2024 Roy. This is an open-access article distributed under the terms of the Creative Commons Attribution License (CC BY). The use, distribution or reproduction in other forums is permitted, provided the original author(s) and the copyright owner(s) are credited and that the original publication in this journal is cited, in accordance with accepted academic practice. No use, distribution or reproduction is permitted which does not comply with these terms.
*Correspondence: René Roy, cm95LnJlbmVAdXFhbS5jYQ==