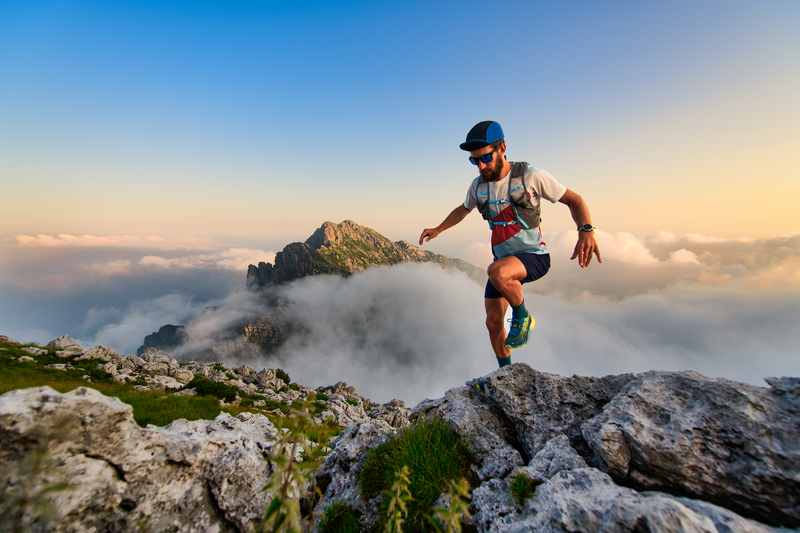
95% of researchers rate our articles as excellent or good
Learn more about the work of our research integrity team to safeguard the quality of each article we publish.
Find out more
REVIEW article
Front. Immunol. , 25 September 2023
Sec. Vaccines and Molecular Therapeutics
Volume 14 - 2023 | https://doi.org/10.3389/fimmu.2023.1278346
This article is part of the Research Topic Precision Vaccinology for Infectious Diseases View all 26 articles
Pneumococcal infections continue to pose a significant global health concern, necessitating the development of effective vaccines. Despite the progress shown by pneumococcal polysaccharide and conjugate vaccines, their limited coverage and the emergence of non-vaccine serotypes have highlighted the need for alternative approaches. Protein-based pneumococcal vaccines, targeting conserved surface proteins of Streptococcus pneumoniae, have emerged as a promising strategy. In this review, we provide an overview of the advancements made in the development of pneumococcal protein vaccines. We discuss the key protein vaccine candidates, highlight their vaccination results in animal studies, and explore the challenges and future directions in protein-based pneumococcal vaccine.
Pneumococcal infections, caused by the bacterium Streptococcus pneumoniae (S. pneumoniae), continue to be a major global health burden, particularly affecting vulnerable populations such as young children, the elderly, and immunocompromised individuals (1–4). Pneumococcal diseases encompass a spectrum of illnesses, including pneumonia, meningitis, sepsis, and otitis media, resulting in substantial morbidity and mortality worldwide (2, 4). Antibiotics have traditionally been the primary treatment for S. pneumoniae infections; however, the rise of antibiotic-resistant strains has posed significant challenges to effective therapy (5). Therefore, vaccines have emerged as a promising approach to prevent pneumococcal infections (6). Currently, based on a limited number of serotype-specific capsular polysaccharides, there are two types of pneumococcal vaccines are available (7). The first type is the 23-valent pneumococcal polysaccharide vaccines (PPVs) consisted with 23 different capsular polysaccharides. The second type is the multivalent pneumococcal conjugate vaccines (PCVs), which involve polysaccharides conjugated to a carrier protein (8, 9). These vaccines aim to elicit immune responses that protect against pneumococcal infections caused by specific serotypes (10). Immunization with these vaccines targeting the polysaccharide capsule of S. pneumoniae has resulted in significant reductions in pneumococcal disease burden, particularly in children (10–12). While PCVs have made remarkable strides in preventing pneumococcal infections, they do have certain limitations (13). Firstly, there are over 90 known pneumococcal serotypes, and PCVs primarily target a limited number of the most common ones. This leaves a significant proportion of pneumococcal strains unaddressed, leading to a phenomenon known as serotype replacement, whereby non-vaccine serotypes emerge and cause infections (14). Furthermore, the polysaccharide antigens used in PCVs have poor immunogenicity in very young children and immunocompromised individuals, limiting the vaccine’s efficacy in these populations (15).
Protein-based pneumococcal vaccines have emerged as a promising strategy to address the limitations of polysaccharide-based vaccines (16). Unlike polysaccharide capsules, which exhibit significant antigenic variation among different serotypes, certain surface proteins of S. pneumoniae are more conserved and shared across serotypes (17). By targeting these conserved proteins, protein-based vaccines have the capacity to offer broader protection against a wider range of pneumococcal strains, including those not covered by PCVs (18). The development of pneumococcal protein vaccines has been fueled by advancements in understanding the biology and pathogenesis of S. pneumoniae. Identification of key virulence factors and surface proteins involved in host-pathogen interactions has facilitated the selection of potential vaccine candidates (19). Pneumolysin (PLY), pneumococcal surface protein A (PspA), pneumococcal surface protein C (PspC), pneumococcal histidine triad proteins (Pht), pneumococcal surface antigen A (PsaA), pneumococcal iron uptake A (PiuA) and pneumococcal iron acquisition A (PiaA) are among the most extensively studied protein candidates, each with unique immunogenic properties and mechanisms of immune protection (Figure 1) (16, 20–22). In this review, we aim to comprehensively explore the progress made in pneumococcal protein vaccines, focusing on key protein candidates and future directions. By understanding the advancements and remaining gaps in this field, we can envision a future where protein-based pneumococcal vaccines play a crucial role in reducing the burden of pneumococcal diseases and protecting vulnerable populations.
Figure 1 The key protein vaccine candidates of S. pneumoniae, routes of vaccination, and elicited immune responses against challenge from S. pneumoniae.
PLY is a vital cholesterol-dependent cytolysin produced by S. pneumoniae (Table 1) (23). With 471 amino acids (53 kDa) and a distinct tertiary structure consisting of four domains, PLY binds to eukaryotic cell membranes’ cholesterol and creates membrane pores, leading to cellular destruction (20). Domains 1, 2, and 3 are essential for oligomerization and pore formation, while domain 4, located in the C-terminal region, facilitates cholesterol binding (20). PLY serves as a critical virulence factor in various stages of pneumococcal disease, including transmission, colonization, and infection (23). It exerts its effects through multiple mechanisms. PLY has the capacity to activate the classical complement pathway, trigger inflammation, induce apoptosis or necroptosis, and directly cause cell toxicity (23, 24). Furthermore, PLY can interact with the mannose receptor C type 1, resulting in the downregulation of inflammation and promoting bacterial survival in the airways (35). Significantly, PLY exhibits a high degree of conservation in its amino acid sequence across different pneumococcal serotypes, making it an attractive target for vaccine development (36). By targeting PLY, vaccines can potentially elicit immune responses that neutralize its cytolytic activity, prevent cellular damage, and limit the spread of pneumococcal infections.
It has been well-established that immunizing mice with purified PLY provides protection against highly virulent pneumococci challenges (37). It has reported that murine monoclonal antibodies targeting PLY have demonstrated a reduction in bacterial burden in the lungs and protection against invasive pneumococcal disease (38). Humans naturally develop an antibody response to PLY due to exposure to S. pneumoniae. The belief that human antibodies to PLY may be protective is supported by evidence showing that anti-PLY antibodies can delay pneumococcal carriage in high-risk infants and provide protection against pneumococcal infections in healthy individuals (39, 40). Although initial experiments with PLY immunization showed promise as it offered protection against multiple S. pneumoniae serotypes, PLY still retained its hemolytic activity in host cells (41). To address this issue, various derivatives of detoxified PLY mutants have been developed, such as recombinant pneumolysoid with the cytolytic functionality removed. This derivative has displayed reactivity to IgGs targeting critical PLY epitopes and has proven successful as an immunogen for specific serotypes in mice and rhesus macaques (38, 42, 43). Further, a self-biomineralized calcium phosphate (CaP)-pneumolysoid nanoparticle vaccine induces bone marrow-derived dendritic cells (BMDCs) and splenocytes production of cytokine, and elicits efficient humoral and cellular immune responses to protect mice from both pneumonia and sepsis infection (44).
Additionally, chemically detoxified PLY derivatives have been explored, inducing an IgG response against PLY without causing tissue damage, as observed in histopathology examinations of host tissues. These derivatives have shown efficacy in protecting against intranasal challenges involving three distinct serotypes (45). Additionally, the protective effects induced by a PLY or pneumolysoid vaccine could potentially be enhanced through the incorporation of other antigens that elicit protection. One such antigen is PspA, which induces high levels of antibodies against each protein and confers protection to mice against invasive challenges, some of which have advanced to phase II trials (21, 46). In addition, immunization mice with pneumolysoid fused with pneumococcal SP0148 has shown to elicit high level of antibody in the serum and effective protection against pneumococcal challenge (47). Moreover, PLY has shown promise as a carrier protein in PCV formulations, especially when combined with CPS. Immunization studies in mice using pneumolysoid conjugated to type 19F CPS have demonstrated a robust and boostable antibody response against both the protein and CPS components. This immunization approach resulted in a high level of protection for infant mice when they were challenged with S. pneumoniae (48). Similar positive outcomes have been observed for conjugates of native PLY with type 18C CPS (49). Comparisons between tetravalent pneumolysoid-CPS conjugate vaccines and tetanus toxoid-CPS conjugate vaccines have revealed that pneumolysoid performs at least as well as tetanus toxoid as a carrier protein, and in some cases, such as with type 23F, it has shown superiority (50). These findings highlight the potential of such antigens to elicit a substantial immune response against CPS, as well as an immune response against virulence proteins, thereby offering comprehensive protection against pneumococcal disease in humans. Extensive animal studies and ongoing clinical trials have positioned PLY as a promising candidate for incorporation into multicomponent protein vaccines. The diverse range of detrimental effects exerted by PLY on the host highlights its significant potential as a valuable vaccine target.
PspA, one of the extensively studied choline-binding proteins, belongs to the major class of S. pneumoniae surface proteins (Table 1) (51, 52). It is widely expressed by all capsular serotypes of S. pneumoniae and serves as a crucial virulence factor that influences bacterium-host interactions by interfering with the fixation of complement C3 (52–54). PspA consists of three major domains: an α-helical domain at the N-terminus, a proline-rich domain in the central portion, and a choline-binding domain at the C-terminus (55). Its α-helical domain exhibits high variability between serotypes and strains. The sequence diversity of PspA has led to its classification into three families and six clades. Family 1 encompasses clades 1 and 2, family 2 comprises clades 3, 4, and 5, while family 3 includes clade 6 (55). Notably, a significant majority of pneumococcal isolates (ranging from 94 to 99%) belong to PspA families 1 and 2 (56), further highlighting its relevance in the global distribution of pneumococci (57). PspA plays pivotal roles in inhibiting complement component C3 deposition on the pneumococcal surface (25), reducing phagocytosis of pneumococci (58, 59), and providing substantial protection against bactericidal peptides of lactoferrin (26). Given its functional significance, PspA has been explored for over three decades as an immunogen and a potential vaccine candidate (22, 60, 61).
Immunization with recombinant family 1 PspA has demonstrated its immunogenicity in humans, with antibodies generated by PspA offering passive protection to mice against a pneumococcal challenge from serotypes 3, 6A, or 6B (62). Subcutaneous immunization with PspA has proven effective in protecting mice from fatal infections (63), while intranasal immunization with PspA has shown efficacy in protection against nasopharyngeal carriage in an adult mouse carriage model (64, 65). Furthermore, mucosal vaccination with PspA has successfully elicited both mucosal and systemic immune responses (66, 67). Initially, there were concerns that a vaccine targeting PspA might not provide adequate coverage due to its high variability (68). However, recent research has brought attention to the proline-rich domain of PspA, which has been found to be considerably more conserved (60). This discovery suggests that the proline-rich domain could be utilized to target uncommon strains beyond those belonging to family 1 or family 2, potentially enhancing the vaccine’s efficacy and widening its scope of protection (22, 68). Additionally, mice immunization with fusion proteins containing family 1 and family 2 PspA fragments could increase complement deposition and provide protection against pneumococcal infection with strains bearing PspA fragments from both families (69). Furthermore, a fusion protein vaccine combined PspA containing families 1 and 2 with other protein can potentially protect against a wide range of S. pneumoniae strains (70). Moreover, a PspA-based trivalent pneumococcal vaccine was formulated and the immunogenicity and protection efficacy were evaluated in macaques, which demonstrated the trivalent vaccine could target all families and clades of PspA and elicited high IgG titers and provided protection against pneumococcal intratracheal challenge (71). PspA has completed a phase I trial (NCT01033409). Further, PspA-based fusion protein vaccine or conjugate vaccine formulations with CPS induce high immune responses and protect mice against invasive challenge (21, 46, 72).
The conjugation of PspA with Vi CPS (Salmonella typhi CPS) has shown enhanced anti-PspA responses and elicited a T-cell dependent response to Vi (72). Additionally, immunization with a PspA fusion protein in combination with CpG oligonucleotides and aluminum hydroxide gel provided significant protection against pneumococcal challenge in mice (73). Moreover, intranasal immunization with a PspA-based vaccine, fused with a protein anchor to display PspA on the surface of bacterium-like particles or pneumolysin, efficiently induced both IgG in the serum and IgA in mucosal washes, ultimately providing complete protection against pneumococcal challenge in mice (74, 75). Despite the challenge posed by the variability in PspA, it remains a robust candidate with decades of research backing its potential as a valuable vaccine component.
PspC, also known as CbpA, SpsA, or Hic, is another choline-binding protein that was initially identified due to its homology with PspA (Table 1) (76). Similar to PspA, PspC features an N-terminal α-helical domain, a central proline-rich domain, and a choline-binding domain (77). However, a significant difference between the two proteins lies in the complexity of the α-helical domain of PspC, which exists in several distinct alleles with different combinations of functions (78). PspC exhibits binding capabilities to secretory IgA, complement component C3, and complement factor H, contributing to its potential roles in colonization, adherence, and invasion (27, 28). Notably, PspC can inhibit C3 deposition on the bacterial surface and mediate the translocation of pneumococci from the nasopharynx to sterile sites such as the lungs or bloodstream (28, 76). Due to its highly immunogenic nature, anti-PspC antibodies play a significant role in antibody immunity against S. pneumoniae (79). Numerous studies have demonstrated that PspC vaccines can induce robust immune responses and provide protection against carriage or invasive challenges in mice (80, 81).
PspC has exhibited promising potential as a vaccine candidate, capable of providing protection as the sole immunogen against pneumococcal infection and carriage (22, 80, 82). Nasal immunization with PspC has been shown to prime the immune system of mice, leading to faster immune responses and a reduction in pneumococcal colonization (83). Subcutaneous immunization with PspC has elicited cross-reactive antibodies with PspA, resulting in mice being protected against pneumococcal sepsis challenges (84). Moreover, intranasal immunization with PspC induced high level of anti-PspC antibodies, and the anti-PspC antiserum from intranasally immunized mice significantly inhibited the adhesion of S. pneumoniae to A549 cells (82). Although a study reported that immunization with PspC through nasal or subcutaneous route did not confer protection against specific pneumococcal challenges (85), PspC has shown to produce additive and longer-lasting immune responses and broaden the range of serotypes covered when combined with other pneumococcal immunogens (46, 86). These combination strategies aim to target multiple virulence factors of S. pneumoniae, thereby providing a more comprehensive and prolonged immune response against pneumococcal infections (86). Notably, one such combination involved fusing PspC with L460D-pneumolysoid as a fusion protein, which demonstrated broader immunogenicity compared to PspC alone. This fusion protein displayed protection against pneumococcal infections and the possibility of providing additional protection against other meningeal pathogens (87). Additionally, recent studies have identified the NEEK motif of PspC, which has the ability to bind to laminin receptors on the blood-brain barrier, suggesting its potential importance in eliciting protection against fatal pneumococcal infections, particularly in cases of meningitis (22, 88).
Studies investigating PspC-based vaccines in animal models have shown promising results, highlighting their immunogenicity and protective efficacy (22). However, the development of PspC-based vaccines still faces certain challenges that need to be addressed. Ongoing research is focused on optimizing vaccine formulations, identifying the most immunogenic PspC variants, and determining the ideal combination strategies with other antigens. By addressing these challenges, PspC-based vaccines hold great potential for providing effective protection against pneumococcal infections.
Pht proteins constitute a group of surface proteins identified in S. pneumoniae, comprising four members: PhtA, PhtB, PhtD, and PhtE (89). The discovery of the Pht family was based on the presence of hydrophobic leader sequences, suggesting their localization as cell surface proteins after transport across the cytoplasmic membrane (Table 1) (90). These proteins contain a distinctive polyhistidine motif, HXXHXH, which is repeated five times (PhtA, PhtB, and PhtD) or six times (PhtE) within their amino acid sequences (89). Ranging in size from 91.5 to 114.6 kDa, these four proteins exhibit a close relationship at the amino acid sequence level, with identities ranging from 32% to 87%. The N-terminal regions demonstrate the highest similarity among the four proteins, exhibiting 87% identity (89, 90). Pht proteins play a crucial role in the pathogenesis of S. pneumoniae infections and are considered multifunctional virulence factors (91). Although their precise functions are not fully understood, they are known to be involved in metal-ion binding, particularly zinc, which is vital for bacterial survival and growth (29). Additionally, Pht has been shown to interact with various host proteins, modulating the host immune response. For instance, Pht has been reported to inhibit the deposition of complement component C3 on the pneumococcal surface through the recruitment of factor H (30). Given their immunogenic properties, Pht has garnered interest as a potential vaccine candidate for non-serotype-dependent prevention of pneumococcal infections.
There is a high degree of protein sequence conservation among various Pht proteins across different serotypes of S. pneumoniae (92). Numerous studies have demonstrated the immunogenicity and protective efficacy of all Pht proteins in multiple mouse models of invasive disease, sepsis, and nasopharyngeal colonization when challenged with diverse S. pneumoniae strains (93–95). Immunizations with different portions of PhtA, including the N-terminal or C-terminal regions, as well as full-length PhtA, generated high levels of antibody titers (90). Full-length PhtA induced antibodies that protected mice against sepsis challenge with serotype 6A or serotype 6B (90). Immunization with either the N-terminal or C-terminal portion of PhtA also conferred protection against serotype 6B challenge, while only the N-terminal half of PhtA induced a protective response against serotype 6A challenge (90). Similarly, immunization with full-length PhtB also elicited high levels of antibody titers and protected mice against challenge with serotype 6B (90). Additionally, immunization with recombinant PhtB provided protection against serotype 3 intranasal pneumococcal challenge (96). Furthermore, immunization of mice with PhtD resulted in the highest levels of antibody titers among all Pht-based vaccines and protected mice against challenge with serotype 3 and 4. Intranasal immunization with PhtD induced robust serum antibody and CD4 Th1-biased immune memory, providing protection against pneumococcal colonization (97). Recent studies using PhtD and C-terminal fragment of PhtD with alum or outer-membrane vesicles as adjuvants elicited significantly high levels of antibodies and conferred protection against pneumococcal challenge (93, 98, 99). In addition, anti-PhtD antibodies were shown to protect against S. pneumoniae through complement- and macrophage-dependent opsonophagocytosis (100). Among the Pht proteins, PhtD is considered the most suitable candidate due to its superior efficacy in a nasopharyngeal colonization model and its high level of conservation among pneumococcal strains (92). PhtD has undergone phase I trials (NCT01767402 and NCT01444001) and failed in an otitis media clinical trial (101, 102). Additionally, Pht-based vaccines have been tested in combination with other protein antigens, such as PspA and PLY, to explore synergistic effects and broaden the spectrum of serotype coverage (43, 103). PhtD conjugated with PLY elicited significant protection that have made it up to phase II trials (Clinical Trial Number, NCT01262872 and NCT00896064). Despite these promising results, challenges remain in the development of Pht-based vaccines. Further studies are needed to identify the most immunogenic epitopes within Pht proteins and to optimize vaccine formulations that induce protective immune responses against multiple pneumococcal strains.
PsaA is an important protein found on the surface of S. pneumoniae, which plays a crucial role in the pathogenesis of pneumococcal infections (104). This surface-exposed lipoprotein has an approximate molecular weight of 37 kDa and is involved in vital functions, including oxidative stress resistance, bacterial adhesion, and acquisition of essential metal ions, particularly manganese and zinc, imperative for pneumococcal growth and viability (Table 1) (31, 32, 105, 106). By scavenging these metal ions from the host environment, PsaA aids the bacterium in evading the host immune response and establishing infections (107). Notably, PsaA exhibits a high degree of conservation across various serotypes of S. pneumoniae, making it an attractive target for vaccination strategies aimed at providing broad protection against multiple strains (108).
Utilized as an immunogen, PsaA has been shown to induce a strong immune response, making it an important target for eliciting protective immune responses through vaccination (37). Parenteral immunization of mice with PsaA using adjuvants has yielded substantial protection against challenge from type 3 pneumococcal strain WU2 (109). Similarly, oral or intranasal administration of PsaA has led to elevated titers of IgG anti-PsaA antibodies in serum and IgA antibodies in mucosal sites. These responses have correlated with a notable reduction in nasopharyngeal colonization following intranasal exposure to S. pneumoniae (110). However, in other study immunization with PsaA elicited antibody response, which cannot effectively protect mice from challenge with S. pneumoniae (111). This prompted the exploration of combinatory approaches, wherein PsaA was fused with other protein antigens to amplify immune responses and synergistic effects. Immunization with PsaA fused with PspA, for instance, triggered heightened antibody levels against both PsaA and PspA. This conferred reduced S. pneumoniae levels in the bloodstream and lungs, ultimately shielding against fatal challenges with the pathogen (112). Moreover, the fusion of PsaA with B lymphocyte stimulator has exhibited potent immune stimulation, marked by heightened serum antibodies specific to PsaA and elevated cytokine levels (113). Additionally, immunization with PasA effectively provides protection when combined with adjuvants in animal challenge (114). Notably, PsaA has also found utility as a carrier protein in glycoconjugate vaccines, displaying protective efficacy in animal models (115). These finding underscore that PsaA represents an appealing candidate for incorporation into pneumococcal vaccines, offering the potential to enhance protection against an extensive array of pneumococcal infections and reduce the burden of disease worldwide. PsaA included vaccine formulations with other proteins have completed or be active in clinical trials (NCT00873431, NCT03803202, and NCT04525599).
Pneumococcal iron uptake A (PiuA) and pneumococcal iron acquisition A (PiaA) are two important iron transport lipoproteins found in S. pneumoniae, which are part of the ATP-binding cassette (ABC) transporter system, a common mechanism used by bacteria to transport essential nutrients across the cell membrane (Table 1) (33, 116). These proteins play a critical role in the uptake of iron from the host environment and its subsequent utilization within the bacterial cell (117, 118). PiuA and PiaA play roles in iron acquisition, with PiuA responsible for transporting ferrichrome iron and PiaA involved in heme iron transport (33, 34). Given their essential role in iron uptake, PiuA and PiaA have been explored as potential targets for the development of antibacterial strategies. Disruption of these proteins or interference with their iron-binding capabilities could potentially impair bacterial survival and growth (118).
Additionally, PiuA and PiaA have undergone investigation as potential vaccine candidates due to their surface exposure, high conservation and immunogenic properties (116, 119, 120). Immunizing mice with recombinant PiuA and PiaA has led to the generation of robust antibody titers, resulting in enhanced complement-independent and -dependent opsonophagocytosis (121). This immune response has been shown to confer protection against systemic challenges posed by S. pneumoniae (119). Moreover, mucosal immunization of mice with PiuA and PiaA has demonstrated the induction of specific antibody responses in both serum and respiratory secretions. This antibody-mediated immune reaction proved effective in safeguarding mice against intranasal challenges from S. pneumoniae (120). In addition, serum antibody to PiuA and PiaA from patients with pneumococcal septicaemia was significantly higher in convalescent-phase than acute-phase, and cross-reacted in different serotypes (116). Further evidence of the immunogenicity of PiuA and PiaA comes from the detection of anti-PiuA and anti-PiaA antibodies in healthy seven-month-old infants, indicating that these proteins can elicit an immune response from a very early age (116). However, there are no clinical trials involving PiuA or PiaA.
Whole-cell vaccines are type of vaccines that use the entire cell of the bacterium S. pneumoniae and present all protein antigens without the need for individual protein purification to stimulate the immune system. Whole-cell vaccines can be developed from killed or live-attenuated whole-cell or by using genetically modified strains of pneumococcus to ensure safety while retaining immunogenicity. These vaccines approach aim to induce both humoral and cellular protective immune response against multiple antigens of the bacterial cell in animal models.
Killed whole-cell vaccines (WCVs) are typically composed of inactivated or killed S. pneumoniae cells. These vaccines can include a variety of strains or serotypes of the bacteria, allowing them to cover a broader spectrum of pneumococcal infections. A WCV was developed by deleting the autolysin gene (lytA) in S. pneumoniae strain RX1 and killed using ethanol. The vaccine was administered via intranasal route to elicit effective prevention of nasopharyngeal colonization with serotype 6B in mice and confer protection against illness and death in rats with serotype 3 strain (122). In addition, a RM200 WCV was constructed by replacing entire lytA gene with a kanamycin resistance gene in strain RX1E with a detoxified PLY mutation (123). The RM200 WCV was inactivated using beta-propiolactone and exhibited strong protective effects against nasopharyngeal colonization by serotype 6B strain and activated IL-17A priming (123, 124).
Live attenuated WCVs contain weakened or attenuated strains of S. pneumoniae. These strains are modified so that they can still replicate within the body but are less virulent, causing only mild or no disease symptoms. A live attenuated WCV was achieved through the attenuation of S. pneumoniae D39, wherein the pep27 gene was removed (125). Mice immunized intranasally with the WCV exhibited high level of IgG and serotype-independent protection against lethal intranasal challenge (125). Additionally, to enhance the safety of this WCV, comD gene was also removed to constructed a WCV of Δpep27ΔcomD. Immunization of mice with Δpep27ΔcomD significantly increased the survival time after heterologous challenge and diminished colonization levels of independent of serotype, which indicated that the WCV of Δpep27ΔcomD appears to be a highly feasible and safe vaccine against pneumococcal infections (126). These vaccines are currently in clinical trial phase 1/2 (NCT02097472).
The progress in pneumococcal protein vaccines represents a significant advancement in the field of vaccine development against S. pneumoniae infections. Protein-based vaccines offer several advantages over traditional capsular polysaccharide-based vaccines, including the potential for broader serotype coverage and the ability to target conserved surface proteins that play critical roles in pneumococcal pathogenesis. Numerous protein candidates have been investigated for their potential as vaccine antigens, including PLY, PspA, PspC, Pht, PsaA, PiuA and PiaA. These proteins have shown promising immunogenicity and protective efficacy in animal studies, with some candidates demonstrating the ability to induce immune responses that inhibit bacterial adherence, colonization, and invasion. Furthermore, several proteins have been evaluated in early clinical trials, providing insights into their safety and immunogenicity in humans. The diversity of protein antigens under investigation highlights the multifaceted nature of pneumococcal pathogenesis and the need for a comprehensive vaccine approach. Combining multiple protein antigens in vaccine formulations, either as multicomponent vaccines or through the use of carrier proteins, holds the potential to broaden serotype coverage and enhance the protective immune response. However, challenges remain in the development of pneumococcal protein vaccines. The high degree of antigenic variability among pneumococcal strains necessitates the identification of conserved epitopes and the design of vaccines that provide broad protection against diverse serotypes. Optimization of vaccine formulations, including the selection of appropriate adjuvants and delivery systems, is crucial to ensure optimal immune responses and long-term efficacy. Furthermore, the evaluation of protein-based vaccines in larger-scale clinical trials is necessary to assess their efficacy in preventing pneumococcal infections and reducing disease burden. Continued surveillance and monitoring of pneumococcal strains are essential to detect any potential serotype replacement or emergence of new strains that may impact vaccine effectiveness.
SL: Supervision, Writing – original draft. HL: Writing – original draft. S-HZ: Writing – original draft. X-YY: Supervision, Writing – review & editing. ZG: Supervision, Writing – review & editing.
The authors declare financial support was received for the research, authorship, and/or publication of this article. This work was financially supported by the National Natural Science Foundation of China (32160232, to SL, 32160183, to X-YY), Guizhou Provincial Natural Science Foundation (QKH-ZK[2021]YB420 and QKH-[2018]5772-018, to SL), Beijing Normal University via the Youth Talent Strategic Program Project (310432102, to ZG).
The authors declare that the research was conducted in the absence of any commercial or financial relationships that could be construed as a potential conflict of interest.
All claims expressed in this article are solely those of the authors and do not necessarily represent those of their affiliated organizations, or those of the publisher, the editors and the reviewers. Any product that may be evaluated in this article, or claim that may be made by its manufacturer, is not guaranteed or endorsed by the publisher.
1. Bogaert D, De Groot R, Hermans PW. Streptococcus pneumoniae colonisation: the key to pneumococcal disease. Lancet Infect Dis (2004) 4:144–54. doi: 10.1016/s1473-3099(04)00938-7
2. van der Poll T, Opal SM. Pathogenesis, treatment, and prevention of pneumococcal pneumonia. Lancet (2009) 374:1543–56. doi: 10.1016/s0140-6736(09)61114-4
3. O’Brien KL, Wolfson LJ, Watt JP, Henkle E, Deloria-Knoll M, McCall N, et al. Burden of disease caused by Streptococcus pneumoniae in children younger than 5 years: global estimates. Lancet (2009) 374:893–902. doi: 10.1016/s0140-6736(09)61204-6
4. Said MA, Johnson HL, Nonyane BA, Deloria-Knoll M, O'Brien KL, Andreo F, et al. Estimating the burden of pneumococcal pneumonia among adults: a systematic review and meta-analysis of diagnostic techniques. PloS One (2013) 8:e60273. doi: 10.1371/journal.pone.0060273
5. Cherazard R, Epstein M, Doan TL, Salim T, Bharti S, Smith MA. Antimicrobial resistant streptococcus pneumoniae: prevalence, mechanisms, and clinical implications. Am J Ther (2017) 24:e361–9. doi: 10.1097/mjt.0000000000000551
6. Musher DM, Anderson R, Feldman C. The remarkable history of pneumococcal vaccination: an ongoing challenge. Pneumonia (Nathan) (2022) 14:5. doi: 10.1186/s41479-022-00097-y
7. El-Beyrouty C, Buckler R, Mitchell M, Phillips S, Groome S. Pneumococcal vaccination-A literature review and practice guideline update. Pharmacotherapy (2022) 42:724–40. doi: 10.1002/phar.2723
8. Pichichero ME, Khan MN, Xu Q. Next generation protein based Streptococcus pneumoniae vaccines. Hum Vaccin Immunother (2016) 12:194–205. doi: 10.1080/21645515.2015.1052198
9. Shafaghi M, Bahadori Z, Madanchi H, Ranjbar MM, Shabani AA, Mousavi SF. Immunoinformatics-aided design of a new multi-epitope vaccine adjuvanted with domain 4 of pneumolysin against Streptococcus pneumoniae strains. BMC Bioinf (2023) 24:67. doi: 10.1186/s12859-023-05175-6
10. Pilishvili T, Lexau C, Farley MM, Hadler J, Harrison LH, Bennett NM, et al. Sustained reductions in invasive pneumococcal disease in the era of conjugate vaccine. J Infect Dis (2010) 201:32–41. doi: 10.1086/648593
11. Maruyama T, Taguchi O, Niederman MS, Morser J, Kobayashi H, Kobayashi T, et al. Efficacy of 23-valent pneumococcal vaccine in preventing pneumonia and improving survival in nursing home residents: double blind, randomised and placebo controlled trial. Bmj (2010) 340:c1004. doi: 10.1136/bmj.c1004
12. Dorosti H, Eslami M, Negahdaripour M, Ghoshoon MB, Gholami A, Heidari R, et al. Vaccinomics approach for developing multi-epitope peptide pneumococcal vaccine. J Biomol Struct Dyn (2019) 37:3524–35. doi: 10.1080/07391102.2018.1519460
13. Micoli F, Romano MR, Carboni F, Adamo R, Berti F. Strengths and weaknesses of pneumococcal conjugate vaccines. Glycoconj J (2023) 40:135–48. doi: 10.1007/s10719-023-10100-3
14. Dagan R. Serotype replacement in perspective. Vaccine (2009) 27 Suppl 3:C22–24. doi: 10.1016/j.vaccine.2009.06.004
15. Käyhty H, O’Brien KL, Goldblatt D. Pneumococcal conjugate vaccines (PCV). Foreword. Vaccine (2013) 32:131–2. doi: 10.1016/j.vaccine.2013.05.066
16. Scott NR, Mann B, Tuomanen EI, Orihuela CJ. Multi-valent protein hybrid pneumococcal vaccines: A strategy for the next generation of vaccines. Vaccines (Basel) (2021) 9(3):209. doi: 10.3390/vaccines9030209
17. Converso TR, Assoni L, André GO, Darrieux M, Leite LCC. The long search for a serotype independent pneumococcal vaccine. Expert Rev Vaccines (2020) 19:57–70. doi: 10.1080/14760584.2020.1711055
18. Lagousi T, Basdeki P, Routsias J, Spoulou V. Novel protein-based pneumococcal vaccines: assessing the use of distinct protein fragments instead of full-length proteins as vaccine antigens. Vaccines (Basel) (2019) 7(1):9. doi: 10.3390/vaccines7010009
19. Masomian M, Ahmad Z, Gew LT, Poh CL. Development of next generation streptococcus pneumoniae vaccines conferring broad protection. Vaccines (Basel) (2020) 8(1):132. doi: 10.3390/vaccines8010132
20. Cima Cabal MD, Molina F, López-Sánchez JI, Pérez-Santín E, Del Mar García-Suárez M. Pneumolysin as a target for new therapies against pneumococcal infections: A systematic review. PloS One (2023) 18:e0282970. doi: 10.1371/journal.pone.0282970
21. Dos Santos TW, Gonçalves PA, Rodriguez D, Pereira JA, Martinez CAR, Leite LCC, et al. A fusion protein comprising pneumococcal surface protein A and a pneumolysin derivate confers protection in a murine model of pneumococcal pneumonia. PloS One (2022) 17:e0277304. doi: 10.1371/journal.pone.0277304
22. Aceil J, Avci FY. Pneumococcal surface proteins as virulence factors, immunogens, and conserved vaccine targets. Front Cell Infect Microbiol (2022) 12:832254. doi: 10.3389/fcimb.2022.832254
23. Nishimoto AT, Rosch JW, Tuomanen EI. Pneumolysin: pathogenesis and therapeutic target. Front Microbiol (2020) 11:1543. doi: 10.3389/fmicb.2020.01543
24. Riegler AN, Brissac T, Gonzalez-Juarbe N, Orihuela CJ. Necroptotic cell death promotes adaptive immunity against colonizing pneumococci. Front Immunol (2019) 10:615. doi: 10.3389/fimmu.2019.00615
25. Mukerji R, Mirza S, Roche AM, Widener RW, Croney CM, Rhee DK, et al. Pneumococcal surface protein A inhibits complement deposition on the pneumococcal surface by competing with the binding of C-reactive protein to cell-surface phosphocholine. J Immunol (2012) 189:5327–35. doi: 10.4049/jimmunol.1201967
26. Shaper M, Hollingshead SK, Benjamin WH Jr., Briles DE. PspA protects Streptococcus pneumoniae from killing by apolactoferrin, and antibody to PspA enhances killing of pneumococci by apolactoferrin [corrected]. Infect Immun (2004) 72:5031–40. doi: 10.1128/iai.72.9.5031-5040.2004
27. Rosenow C, Ryan P, Weiser JN, Johnson S, Fontan P, Ortqvist A, et al. Contribution of novel choline-binding proteins to adherence, colonization and immunogenicity of Streptococcus pneumoniae. Mol Microbiol (1997) 25:819–29. doi: 10.1111/j.1365-2958.1997.mmi494.x
28. Dave S, Carmicle S, Hammerschmidt S, Pangburn MK, McDaniel LS. Dual roles of PspC, a surface protein of Streptococcus pneumoniae, in binding human secretory IgA and factor H. J Immunol (2004) 173:471–7. doi: 10.4049/jimmunol.173.1.471
29. Plumptre CD, Hughes CE, Harvey RM, Eijkelkamp BA, McDevitt CA, Paton JC. Overlapping functionality of the Pht proteins in zinc homeostasis of Streptococcus pneumoniae. Infect Immun (2014) 82:4315–24. doi: 10.1128/iai.02155-14
30. Ogunniyi AD, Grabowicz M, Mahdi LK, Cook J, Gordon DL, Sadlon TA, et al. Pneumococcal histidine triad proteins are regulated by the Zn2+-dependent repressor AdcR and inhibit complement deposition through the recruitment of complement factor H. FASEB J (2009) 23:731–8. doi: 10.1096/fj.08-119537
31. Tseng HJ, McEwan AG, Paton JC, Jennings MP. Virulence of Streptococcus pneumoniae: PsaA mutants are hypersensitive to oxidative stress. Infect Immun (2002) 70:1635–9. doi: 10.1128/iai.70.3.1635-1639.2002
32. McAllister LJ, Tseng HJ, Ogunniyi AD, Jennings MP, McEwan AG, Paton JC, et al. Molecular analysis of the psa permease complex of Streptococcus pneumoniae. Mol Microbiol (2004) 53:889–901. doi: 10.1111/j.1365-2958.2004.04164.x
33. Yang XY, He K, Du G, Wu X, Yu G, Pan Y, et al. Integrated translatomics with proteomics to identify novel iron-transporting proteins in streptococcus pneumoniae. Front Microbiol (2016) 7:78. doi: 10.3389/fmicb.2016.00078
34. Tai SS, Yu C, Lee JK. A solute binding protein of Streptococcus pneumoniae iron transport. FEMS Microbiol Lett (2003) 220:303–8. doi: 10.1016/s0378-1097(03)00135-6
35. Subramanian K, Neill DR, Malak HA, Spelmink L, Khandaker S, Dalla Libera Marchiori G, et al. Pneumolysin binds to the mannose receptor C type 1 (MRC-1) leading to anti-inflammatory responses and enhanced pneumococcal survival. Nat Microbiol (2019) 4:62–70. doi: 10.1038/s41564-018-0280-x
36. Lock RA, Zhang QY, Berry AM, Paton JC. Sequence variation in the Streptococcus pneumoniae pneumolysin gene affecting haemolytic activity and electrophoretic mobility of the toxin. Microb Pathog (1996) 21:71–83. doi: 10.1006/mpat.1996.0044
37. Briles DE, Paton JC, Mukerji R, Swiatlo E, Crain MJ. Pneumococcal vaccines. Microbiol Spectr (2019) 7(6):10.1128. doi: 10.1128/microbiolspec.GPP3-0028-2018
38. García-Suárez Mdel M, Cima-Cabal MD, Flórez N, García P, Cernuda-Cernuda R, Astudillo A, et al. Protection against pneumococcal pneumonia in mice by monoclonal antibodies to pneumolysin. Infect Immun (2004) 72:4534–40. doi: 10.1128/iai.72.8.4534-4540.2004
39. Francis JP, Richmond PC, Pomat WS, Michael A, Keno H, Phuanukoonnon S, et al. Maternal antibodies to pneumolysin but not to pneumococcal surface protein A delay early pneumococcal carriage in high-risk Papua New Guinean infants. Clin Vaccine Immunol (2009) 16:1633–8. doi: 10.1128/cvi.00247-09
40. Huo Z, Spencer O, Miles J, Johnson J, Holliman R, Sheldon J, et al. Antibody response to pneumolysin and to pneumococcal capsular polysaccharide in healthy individuals and Streptococcus pneumoniae infected patients. Vaccine (2004) 22:1157–61. doi: 10.1016/j.vaccine.2003.09.025
41. Baba H, Kawamura I, Kohda C, Nomura T, Ito Y, Kimoto T, et al. Essential role of domain 4 of pneumolysin from Streptococcus pneumoniae in cytolytic activity as determined by truncated proteins. Biochem Biophys Res Commun (2001) 281:37–44. doi: 10.1006/bbrc.2001.4297
42. Salha D, Szeto J, Myers L, Claus C, Sheung A, Tang M, et al. Neutralizing antibodies elicited by a novel detoxified pneumolysin derivative, PlyD1, provide protection against both pneumococcal infection and lung injury. Infect Immun (2012) 80:2212–20. doi: 10.1128/iai.06348-11
43. Denoël P, Philipp MT, Doyle L, Martin D, Carletti G, Poolman JT. A protein-based pneumococcal vaccine protects rhesus macaques from pneumonia after experimental infection with Streptococcus pneumoniae. Vaccine (2011) 29:5495–501. doi: 10.1016/j.vaccine.2011.05.051
44. Wu J, Wu K, Xu W, Yuan T, Wang X, Zhang J, et al. Engineering detoxified pneumococcal pneumolysin derivative ΔA146PLY for self-biomineralization of calcium phosphate: Assessment of their protective efficacy in murine infection models. Biomaterials (2018) 155:152–64. doi: 10.1016/j.biomaterials.2017.11.018
45. Hermand P, Vandercammen A, Mertens E, Di Paolo E, Verlant V, Denoël P, et al. Preclinical evaluation of a chemically detoxified pneumolysin as pneumococcal vaccine antigen. Hum Vaccin Immunother (2017) 13:220–8. doi: 10.1080/21645515.2016.1234553
46. Chen A, Mann B, Gao G, Heath R, King J, Maissoneuve J, et al. Multivalent pneumococcal protein vaccines comprising pneumolysoid with epitopes/fragments of cbpA and/or pspA elicit strong and broad protection. Clin Vaccine Immunol (2015) 22:1079–89. doi: 10.1128/cvi.00293-15
47. Wang Y, Xia L, Wang G, Lu H, Wang H, Luo S, et al. Subcutaneous immunization with the fusion protein ΔA146Ply-SP0148 confers protection against Streptococcus pneumoniae infection. Microb Pathog (2022) 162:105325. doi: 10.1016/j.micpath.2021.105325
48. Lee CJ, Lock RA, Andrew PW, Mitchell TJ, Hansman D, Paton JC. Protection of infant mice from challenge with Streptococcus pneumoniae type 19F by immunization with a type 19F polysaccharide–pneumolysoid conjugate. Vaccine (1994) 12:875–8. doi: 10.1016/0264-410x(94)90028-0
49. Kuo J, Douglas M, Ree HK, Lindberg AA. Characterization of a recombinant pneumolysin and its use as a protein carrier for pneumococcal type 18C conjugate vaccines. Infect Immun (1995) 63:2706–13. doi: 10.1128/iai.63.7.2706-2713.1995
50. Michon F, Fusco PC, Minetti CA, Laude-Sharp M, Uitz C, Huang CH, et al. Multivalent pneumococcal capsular polysaccharide conjugate vaccines employing genetically detoxified pneumolysin as a carrier protein. Vaccine (1998) 16:1732–41. doi: 10.1016/s0264-410x(98)00225-4
51. Sempere J, Llamosí M, Del Río Menéndez I, López Ruiz B, Domenech M, González-Camacho F. Pneumococcal choline-binding proteins involved in virulence as vaccine candidates. Vaccines (Basel) (2021) 9(2):181. doi: 10.3390/vaccines9020181
52. Lane JR, Tata M, Briles DE, Orihuela CJ. A jack of all trades: the role of pneumococcal surface protein A in the pathogenesis of streptococcus pneumoniae. Front Cell Infect Microbiol (2022) 12:826264. doi: 10.3389/fcimb.2022.826264
53. Yother J, Handsome GL, Briles DE. Truncated forms of PspA that are secreted from Streptococcus pneumoniae and their use in functional studies and cloning of the pspA gene. J Bacteriol (1992) 174:610–8. doi: 10.1128/jb.174.2.610-618.1992
54. Tu AH, Fulgham RL, McCrory MA, Briles DE, Szalai AJ. Pneumococcal surface protein A inhibits complement activation by Streptococcus pneumoniae. Infect Immun (1999) 67:4720–4. doi: 10.1128/iai.67.9.4720-4724.1999
55. Hollingshead SK, Becker R, Briles DE. Diversity of PspA: mosaic genes and evidence for past recombination in Streptococcus pneumoniae. Infect Immun (2000) 68:5889–900. doi: 10.1128/iai.68.10.5889-5900.2000
56. Hotomi M, Togawa A, Kono M, Ikeda Y, Takei S, Hollingshead SK, et al. PspA family distribution, antimicrobial resistance and serotype of Streptococcus pneumoniae isolated from upper respiratory tract infections in Japan. PloS One (2013) 8:e58124. doi: 10.1371/journal.pone.0058124
57. Hollingshead SK, Baril L, Ferro S, King J, Coan P, Briles DE, et al. Pneumococcal surface protein A (PspA) family distribution among clinical isolates from adults over 50 years of age collected in seven countries. J Med Microbiol (2006) 55:215–21. doi: 10.1099/jmm.0.46268-0
58. Genschmer KR, Accavitti-Loper MA, Briles DE. A modified surface killing assay (MSKA) as a functional in vitro assay for identifying protective antibodies against pneumococcal surface protein A (PspA). Vaccine (2013) 32:39–47. doi: 10.1016/j.vaccine.2013.10.080
59. Kuroda E, Koizumi Y, Piao Z, Nakayama H, Tomono K, Oishi K, et al. Establishment of a modified opsonophagocytic killing assay for anti-pneumococcal surface protein A antibody. J Microbiol Methods (2023) 212:106804. doi: 10.1016/j.mimet.2023.106804
60. Afshari E, Cohan RA, Sotoodehnejadnematalahi F, Mousavi SF. In-silico design and evaluation of an epitope-based serotype-independent promising vaccine candidate for highly cross-reactive regions of pneumococcal surface protein A. J Transl Med (2023) 21:13. doi: 10.1186/s12967-022-03864-z
61. Alghamdi S, Sahibzada MUK, Shesha NT, Aslam A, Kabrah A, Atwah B, et al. Pneumococcal surface protein A: A promising candidate for the next generation of pneumococcal vaccines. Cell Mol Biol (Noisy-le-grand) (2022) 67:289–98. doi: 10.14715/cmb/2021.67.4.32
62. Briles DE, Hollingshead SK, King J, Swift A, Braun PA, Park MK, et al. Immunization of humans with recombinant pneumococcal surface protein A (rPspA) elicits antibodies that passively protect mice from fatal infection with Streptococcus pneumoniae bearing heterologous PspA. J Infect Dis (2000) 182:1694–701. doi: 10.1086/317602
63. Roche H, Ren B, McDaniel LS, Håkansson A, Briles DE. Relative roles of genetic background and variation in PspA in the ability of antibodies to PspA to protect against capsular type 3 and 4 strains of Streptococcus pneumoniae. Infect Immun (2003) 71:4498–505. doi: 10.1128/iai.71.8.4498-4505.2003
64. Briles DE, Ades E, Paton JC, Sampson JS, Carlone GM, Huebner RC, et al. Intranasal immunization of mice with a mixture of the pneumococcal proteins PsaA and PspA is highly protective against nasopharyngeal carriage of Streptococcus pneumoniae. Infect Immun (2000) 68:796–800. doi: 10.1128/iai.68.2.796-800.2000
65. Nagano H, Kawabata M, Sugita G, Tsuruhara A, Ohori J, Jimura T, et al. Transcutaneous immunization with pneumococcal surface protein A in mice. Laryngoscope (2018) 128:E91–e96. doi: 10.1002/lary.26971
66. Kong IG, Sato A, Yuki Y, Nochi T, Takahashi H, Sawada S, et al. Nanogel-based PspA intranasal vaccine prevents invasive disease and nasal colonization by Streptococcus pneumoniae. Infect Immun (2013) 81:1625–34. doi: 10.1128/iai.00240-13
67. Figueiredo DB, Kaneko K, Rodrigues TDC, MacLoughlin R, Miyaji EN, Saleem I, et al. Pneumococcal surface protein A-hybrid nanoparticles protect mice from lethal challenge after mucosal immunization targeting the lungs. Pharmaceutics (2022) 14(6):1238. doi: 10.3390/pharmaceutics14061238
68. Mukerji R, Hendrickson C, Genschmer KR, Park SS, Bouchet V, Goldstein R, et al. The diversity of the proline-rich domain of pneumococcal surface protein A (PspA): Potential relevance to a broad-spectrum vaccine. Vaccine (2018) 36:6834–43. doi: 10.1016/j.vaccine.2018.08.045
69. Darrieux M, Miyaji EN, Ferreira DM, Lopes LM, Lopes AP, Ren B, et al. Fusion proteins containing family 1 and family 2 PspA fragments elicit protection against Streptococcus pneumoniae that correlates with antibody-mediated enhancement of complement deposition. Infect Immun (2007) 75:5930–8. doi: 10.1128/iai.00940-07
70. Yu J, Chen X, Li B, Gu T, Meng X, Kong W, et al. A pneumococcal vaccine combination with two proteins containing PspA families 1 and 2 can potentially protect against a wide range of Streptococcus pneumoniae strains. Immunol Res (2018) 66:528–36. doi: 10.1007/s12026-018-9016-5
71. Nakahashi-Ouchida R, Uchida Y, Yuki Y, Katakai Y, Yamanoue T, Ogawa H, et al. A nanogel-based trivalent PspA nasal vaccine protects macaques from intratracheal challenge with pneumococci. Vaccine (2021) 39:3353–64. doi: 10.1016/j.vaccine.2021.04.069
72. Kothari N, Genschmer KR, Kothari S, Kim JA, Briles DE, Rhee DK, et al. Preparation and testing of a Vi conjugate vaccine using pneumococcal surface protein A (PspA) from Streptococcus pneumoniae as the carrier protein. Vaccine (2014) 32:5755–60. doi: 10.1016/j.vaccine.2014.08.041
73. Piao Z, Akeda Y, Takeuchi D, Ishii KJ, Ubukata K, Briles DE, et al. Protective properties of a fusion pneumococcal surface protein A (PspA) vaccine against pneumococcal challenge by five different PspA clades in mice. Vaccine (2014) 32:5607–13. doi: 10.1016/j.vaccine.2014.07.108
74. Wang D, Lu J, Yu J, Hou H, Leenhouts K, Van Roosmalen ML, et al. A novel pspA protein vaccine intranasal delivered by bacterium-like particles provides broad protection against pneumococcal pneumonia in mice. Immunol Invest (2018) 47:403–15. doi: 10.1080/08820139.2018.1439505
75. Zane L, Kraschowetz S, Trentini MM, Alves VDS, Araujo SC, Goulart C, et al. Peptide linker increased the stability of pneumococcal fusion protein vaccine candidate. Front Bioeng Biotechnol (2023) 11:1108300. doi: 10.3389/fbioe.2023.1108300
76. Darrieux M, Goulart C, Briles D, Leite LC. Current status and perspectives on protein-based pneumococcal vaccines. Crit Rev Microbiol (2015) 41:190–200. doi: 10.3109/1040841x.2013.813902
77. Briles DE, Hollingshead SK, Swiatlo E, Brooks-Walter A, Szalai A, Virolainen A, et al. PspA and PspC: their potential for use as pneumococcal vaccines. Microb Drug Resist (1997) 3:401–8. doi: 10.1089/mdr.1997.3.401
78. Iannelli F, Oggioni MR, Pozzi G. Allelic variation in the highly polymorphic locus pspC of Streptococcus pneumoniae. Gene (2002) 284:63–71. doi: 10.1016/s0378-1119(01)00896-4
79. Georgieva M, Kagedan L, Lu YJ, Thompson CM, Lipsitch M. Antigenic variation in streptococcus pneumoniae pspC promotes immune escape in the presence of variant-specific immunity. mBio (2018) 9(2):e00264–18. doi: 10.1128/mBio.00264-18
80. Balachandran P, Brooks-Walter A, Virolainen-Julkunen A, Hollingshead SK, Briles DE. Role of pneumococcal surface protein C in nasopharyngeal carriage and pneumonia and its ability to elicit protection against carriage of Streptococcus pneumoniae. Infect Immun (2002) 70:2526–34. doi: 10.1128/iai.70.5.2526-2534.2002
81. Ogunniyi AD, Woodrow MC, Poolman JT, Paton JC. Protection against Streptococcus pneumoniae elicited by immunization with pneumolysin and CbpA. Infect Immun (2001) 69:5997–6003. doi: 10.1128/iai.69.10.5997-6003.2001
82. Cao J, Gong Y, Li D, Yin N, Chen T, Xu W, et al. CD4(+) T lymphocytes mediated protection against invasive pneumococcal infection induced by mucosal immunization with ClpP and CbpA. Vaccine (2009) 27:2838–44. doi: 10.1016/j.vaccine.2009.02.093
83. Hernani Mde L, Ferreira PC, Ferreira DM, Miyaji EN, Ho PL, Oliveira ML. Nasal immunization of mice with Lactobacillus casei expressing the pneumococcal surface protein C primes the immune system and decreases pneumococcal nasopharyngeal colonization in mice. FEMS Immunol Med Microbiol (2011) 62:263–72. doi: 10.1111/j.1574-695X.2011.00809.x
84. Brooks-Walter A, Briles DE, Hollingshead SK. The pspC gene of Streptococcus pneumoniae encodes a polymorphic protein, PspC, which elicits cross-reactive antibodies to PspA and provides immunity to pneumococcal bacteremia. Infect Immun (1999) 67:6533–42. doi: 10.1128/iai.67.12.6533-6542.1999
85. Ferreira DM, Darrieux M, Silva DA, Leite LC, Ferreira JM Jr., Ho PL, et al. Characterization of protective mucosal and systemic immune responses elicited by pneumococcal surface protein PspA and PspC nasal vaccines against a respiratory pneumococcal challenge in mice. Clin Vaccine Immunol (2009) 16:636–45. doi: 10.1128/cvi.00395-08
86. Ogunniyi AD, Grabowicz M, Briles DE, Cook J, Paton JC. Development of a vaccine against invasive pneumococcal disease based on combinations of virulence proteins of Streptococcus pneumoniae. Infect Immun (2007) 75:350–7. doi: 10.1128/iai.01103-06
87. Mann B, Thornton J, Heath R, Wade KR, Tweten RK, Gao G, et al. Broadly protective protein-based pneumococcal vaccine composed of pneumolysin toxoid-CbpA peptide recombinant fusion protein. J Infect Dis (2014) 209:1116–25. doi: 10.1093/infdis/jit502
88. Orihuela CJ, Mahdavi J, Thornton J, Mann B, Wooldridge KG, Abouseada N, et al. Laminin receptor initiates bacterial contact with the blood brain barrier in experimental meningitis models. J Clin Invest (2009) 119:1638–46. doi: 10.1172/jci36759
89. Plumptre CD, Ogunniyi AD, Paton JC. Polyhistidine triad proteins of pathogenic streptococci. Trends Microbiol (2012) 20:485–93. doi: 10.1016/j.tim.2012.06.004
90. Adamou JE, Heinrichs JH, Erwin AL, Walsh W, Gayle T, Dormitzer M, et al. Identification and characterization of a novel family of pneumococcal proteins that are protective against sepsis. Infect Immun (2001) 69:949–58. doi: 10.1128/iai.69.2.949-958.2001
91. Huang J, Gingerich AD, Royer F, Paschall AV, Pena-Briseno A, Avci FY, et al. Broadly reactive human monoclonal antibodies targeting the pneumococcal histidine triad protein protect against fatal pneumococcal infection. Infect Immun (2021) 89(5):e00747–20. doi: 10.1128/iai.00747-20
92. Rioux S, Neyt C, Di Paolo E, Turpin L, Charland N, Labbé S, et al. Transcriptional regulation, occurrence and putative role of the Pht family of Streptococcus pneumoniae. Microbiol (Reading) (2011) 157:336–48. doi: 10.1099/mic.0.042184-0
93. Malekan M, Siadat SD, Aghasadeghi M, Shahrokhi N, Afrough P, Behrouzi A, et al. Evaluation of protective immunity responses against pneumococcal PhtD and its C-terminal in combination with outer-membrane vesicles as adjuvants. J Med Microbiol (2020) 69:465–77. doi: 10.1099/jmm.0.001103
94. Kawaguchiya M, Urushibara N, Aung MS, Shinagawa M, Takahashi S, Kobayashi N. Prevalence of various vaccine candidate proteins in clinical isolates of streptococcus pneumoniae: characterization of the novel pht fusion proteins phtA/B and phtA/D. Pathogens (2019) 8(4):162. doi: 10.3390/pathogens8040162
95. Plumptre CD, Ogunniyi AD, Paton JC. Surface association of Pht proteins of Streptococcus pneumoniae. Infect Immun (2013) 81:3644–51. doi: 10.1128/iai.00562-13
96. Zhang Y, Masi AW, Barniak V, Mountzouros K, Hostetter MK, Green BA. Recombinant PhpA protein, a unique histidine motif-containing protein from Streptococcus pneumoniae, protects mice against intranasal pneumococcal challenge. Infect Immun (2001) 69:3827–36. doi: 10.1128/iai.69.6.3827-3836.2001
97. Khan MN, Pichichero ME. CD4 T cell memory and antibody responses directed against the pneumococcal histidine triad proteins PhtD and PhtE following nasopharyngeal colonization and immunization and their role in protection against pneumococcal colonization in mice. Infect Immun (2013) 81:3781–92. doi: 10.1128/iai.00313-13
98. André GO, Borges MT, Assoni L, Ferraz LFC, Sakshi P, Adamson P, et al. Protective role of PhtD and its amino and carboxyl fragments against pneumococcal sepsis. Vaccine (2021) 39:3626–32. doi: 10.1016/j.vaccine.2021.04.068
99. André GO, Assoni L, Rodriguez D, Leite LCC, Dos Santos TEP, Ferraz LFC, et al. Immunization with PhtD truncated fragments reduces nasopharyngeal colonization by Streptococcus pneumoniae. Vaccine (2020) 38:4146–53. doi: 10.1016/j.vaccine.2020.04.050
100. Visan L, Rouleau N, Proust E, Peyrot L, Donadieu A, Ochs M. Antibodies to PcpA and PhtD protect mice against Streptococcus pneumoniae by a macrophage- and complement-dependent mechanism. Hum Vaccin Immunother (2018) 14:489–94. doi: 10.1080/21645515.2017.1403698
101. Ferreira DM, Jambo KC, Gordon SB. Experimental human pneumococcal carriage models for vaccine research. Trends Microbiol (2011) 19:464–70. doi: 10.1016/j.tim.2011.06.003
102. Seiberling M, Bologa M, Brookes R, Ochs M, Go K, Neveu D, et al. Safety and immunogenicity of a pneumococcal histidine triad protein D vaccine candidate in adults. Vaccine (2012) 30:7455–60. doi: 10.1016/j.vaccine.2012.10.080
103. Bologa M, Kamtchoua T, Hopfer R, Sheng X, Hicks B, Bixler G, et al. Safety and immunogenicity of pneumococcal protein vaccine candidates: monovalent choline-binding protein A (PcpA) vaccine and bivalent PcpA-pneumococcal histidine triad protein D vaccine. Vaccine (2012) 30:7461–8. doi: 10.1016/j.vaccine.2012.10.076
104. Morrison KE, Lake D, Crook J, Carlone GM, Ades E, Facklam R, et al. Confirmation of psaA in all 90 serotypes of Streptococcus pneumoniae by PCR and potential of this assay for identification and diagnosis. J Clin Microbiol (2000) 38:434–7. doi: 10.1128/jcm.38.1.434-437.2000
105. Oliveira ML, Monedero V, Miyaji EN, Leite LC, Lee Ho P, Pérez-Martínez G. Expression of Streptococcus pneumoniae antigens, PsaA (pneumococcal surface antigen A) and PspA (pneumococcal surface protein A) by Lactobacillus casei. FEMS Microbiol Lett (2003) 227:25–31. doi: 10.1016/s0378-1097(03)00645-1
106. Hu Y, Park N, Seo KS, Park JY, Somarathne RP, Olivier AK, et al. Pneumococcal surface adhesion A protein (PsaA) interacts with human Annexin A2 on airway epithelial cells. Virulence (2021) 12:1841–54. doi: 10.1080/21505594.2021.1947176
107. Dintilhac A, Alloing G, Granadel C, Claverys JP. Competence and virulence of Streptococcus pneumoniae: Adc and PsaA mutants exhibit a requirement for Zn and Mn resulting from inactivation of putative ABC metal permeases. Mol Microbiol (1997) 25:727–39. doi: 10.1046/j.1365-2958.1997.5111879.x
108. Pimenta FC, Miyaji EN, Arêas AP, Oliveira ML, de Andrade AL, Ho PL, et al. Intranasal immunization with the cholera toxin B subunit-pneumococcal surface antigen A fusion protein induces protection against colonization with Streptococcus pneumoniae and has negligible impact on the nasopharyngeal and oral microbiota of mice. Infect Immun (2006) 74:4939–44. doi: 10.1128/iai.00134-06
109. Talkington DF, Brown BG, Tharpe JA, Koenig A, Russell H. Protection of mice against fatal pneumococcal challenge by immunization with pneumococcal surface adhesin A (PsaA). Microb Pathog (1996) 21:17–22. doi: 10.1006/mpat.1996.0038
110. Wang S, Li Y, Shi H, Scarpellini G, Torres-Escobar A, Roland KL, et al. Immune responses to recombinant pneumococcal PsaA antigen delivered by a live attenuated Salmonella vaccine. Infect Immun (2010) 78:3258–71. doi: 10.1128/iai.00176-10
111. Gor DO, Ding X, Briles DE, Jacobs MR, Greenspan NS. Relationship between surface accessibility for PpmA, PsaA, and PspA and antibody-mediated immunity to systemic infection by Streptococcus pneumoniae. Infect Immun (2005) 73:1304–12. doi: 10.1128/iai.73.3.1304-1312.2005
112. Lu J, Sun T, Wang D, Dong Y, Xu M, Hou H, et al. Protective immune responses elicited by fusion protein containing psaA and pspA fragments. Immunol Invest (2015) 44:482–96. doi: 10.3109/08820139.2015.1037956
113. Gor DO, Ding X, Li Q, Sultana D, Mambula SS, Bram RJ, et al. Enhanced immunogenicity of pneumococcal surface adhesin A (PsaA) in mice via fusion to recombinant human B lymphocyte stimulator (BLyS). Biol Direct (2011) 6:9. doi: 10.1186/1745-6150-6-9
114. Olafsdottir TA, Lingnau K, Nagy E, Jonsdottir I. Novel protein-based pneumococcal vaccines administered with the Th1-promoting adjuvant IC31 induce protective immunity against pneumococcal disease in neonatal mice. Infect Immun (2012) 80:461–8. doi: 10.1128/iai.05801-11
115. Chen Z, Guo R, Xu J, Qiu C. Immunogenicity and protective immunity against otitis media caused by pneumococcus in mice of Hib conjugate vaccine with PsaA protein carrier. Front Med (2016) 10:490–8. doi: 10.1007/s11684-016-0470-y
116. Whalan RH, Funnell SG, Bowler LD, Hudson MJ, Robinson A, Dowson CG. PiuA and PiaA, iron uptake lipoproteins of Streptococcus pneumoniae, elicit serotype independent antibody responses following human pneumococcal septicaemia. FEMS Immunol Med Microbiol (2005) 43:73–80. doi: 10.1016/j.femsim.2004.07.010
117. Zhang L, Li N, Cao K, Yang XY, Zeng G, Sun X, et al. Crucial residue Trp158 of lipoprotein PiaA stabilizes the ferrichrome-PiaA complex in Streptococcus pneumoniae. J Inorg Biochem (2017) 167:150–6. doi: 10.1016/j.jinorgbio.2016.08.015
118. Brown JS, Gilliland SM, Holden DW. A Streptococcus pneumoniae pathogenicity island encoding an ABC transporter involved in iron uptake and virulence. Mol Microbiol (2001) 40:572–85. doi: 10.1046/j.1365-2958.2001.02414.x
119. Brown JS, Ogunniyi AD, Woodrow MC, Holden DW, Paton JC. Immunization with components of two iron uptake ABC transporters protects mice against systemic Streptococcus pneumoniae infection. Infect Immun (2001) 69:6702–6. doi: 10.1128/iai.69.11.6702-6706.2001
120. Jomaa M, Terry S, Hale C, Jones C, Dougan G, Brown J. Immunization with the iron uptake ABC transporter proteins PiaA and PiuA prevents respiratory infection with Streptococcus pneumoniae. Vaccine (2006) 24:5133–9. doi: 10.1016/j.vaccine.2006.04.012
121. Jomaa M, Yuste J, Paton JC, Jones C, Dougan G, Brown JS, et al. Antibodies to the iron uptake ABC transporter lipoproteins PiaA and PiuA promote opsonophagocytosis of Streptococcus pneumoniae. Infect Immun (2005) 73:6852–9. doi: 10.1128/iai.73.10.6852-6859.2005
122. Malley R, Lipsitch M, Stack A, Saladino R, Fleisher G, Pelton S, et al. Intranasal immunization with killed unencapsulated whole cells prevents colonization and invasive disease by capsulated pneumococci. Infect Immun (2001) 69:4870–3. doi: 10.1128/iai.69.8.4870-4873.2001
123. Lu YJ, Yadav P, Clements JD, Forte S, Srivastava A, Thompson CM, et al. Options for inactivation, adjuvant, and route of topical administration of a killed, unencapsulated pneumococcal whole-cell vaccine. Clin Vaccine Immunol (2010) 17:1005–12. doi: 10.1128/cvi.00036-10
124. Hogenesch H, Dunham A, Hansen B, Anderson K, Maisonneuve JF, Hem SL. Formulation of a killed whole cell pneumococcus vaccine - effect of aluminum adjuvants on the antibody and IL-17 response. J Immune Based Ther Vaccines (2011) 9:5. doi: 10.1186/1476-8518-9-5
125. Kim EH, Choi SY, Kwon MK, Tran TD, Park SS, Lee KJ, et al. Streptococcus pneumoniae pep27 mutant as a live vaccine for serotype-independent protection in mice. Vaccine (2012) 30:2008–19. doi: 10.1016/j.vaccine.2011.11.073
Keywords: Streptococcus pneumoniae, Pneumococcal protein vaccine, pneumolysin, pneumococcal surface proteins, pneumococcal histidine triad proteins
Citation: Li S, Liang H, Zhao S-H, Yang X-Y and Guo Z (2023) Recent progress in pneumococcal protein vaccines. Front. Immunol. 14:1278346. doi: 10.3389/fimmu.2023.1278346
Received: 16 August 2023; Accepted: 07 September 2023;
Published: 25 September 2023.
Edited by:
Mahbuba Rahman, McMaster University, CanadaReviewed by:
Elaine I. Tuomanen, St. Jude Children’s Research Hospital, United StatesCopyright © 2023 Li, Liang, Zhao, Yang and Guo. This is an open-access article distributed under the terms of the Creative Commons Attribution License (CC BY). The use, distribution or reproduction in other forums is permitted, provided the original author(s) and the copyright owner(s) are credited and that the original publication in this journal is cited, in accordance with accepted academic practice. No use, distribution or reproduction is permitted which does not comply with these terms.
*Correspondence: Zhong Guo, ei5ndW9AYm51LmVkdS5jbg==
Disclaimer: All claims expressed in this article are solely those of the authors and do not necessarily represent those of their affiliated organizations, or those of the publisher, the editors and the reviewers. Any product that may be evaluated in this article or claim that may be made by its manufacturer is not guaranteed or endorsed by the publisher.
Research integrity at Frontiers
Learn more about the work of our research integrity team to safeguard the quality of each article we publish.