- 1Department of Neurology and Epileptology, Centre of Postgraduate Medical Education, Warsaw, Poland
- 2Department of Neurology, Medical University of Warsaw, Warsaw, Poland
- 3Department of Nuclear Medicine, Medical University of Warsaw, Warsaw, Poland
Misfolding protein aggregation inside or outside cells is the major pathological hallmark of several neurodegenerative diseases. Among proteinopathies are neurodegenerative diseases with atypical Parkinsonism and an accumulation of insoluble fibrillary alpha-synuclein (synucleinopathies) or hyperphosphorylated tau protein fragments (tauopathies). As there are no therapies available to slow or halt the progression of these disea ses, targeting the inflammatory process is a promising approach. The inflammatory biomarkers could also help in the differential diagnosis of Parkinsonian syndromes. Here, we review inflammation’s role in multiple systems atrophy pathogenesis, diagnosis, and treatment.
1 Introduction
Multiple system atrophy (MSA) is a rare, progressive proteinopathy with an accumulation of insoluble fibrillary alpha-synuclein (aSyn) that presents with various combinations of atypical Parkinsonian syndrome, autonomic failure, and cerebellar syndrome.
Most studies assessing the pathogenesis of MSA have focused on the mechanisms of aSyn accumulation in neurons and oligodendrocytes. The glial cytoplasmic inclusions (GCIs) in the oligodendrocytes are unique among synucleinopathies (1, 2).
2 Inflammation: effect or cause of neurodegeneration
Marked neuroinflammation is a suggested mechanism in MSA pathogenesis (3, 4). It remains uncertain if inflammation is a primary driver of protein accumulation, a response to the degeneration of neural tissue, or an aggravator of the process (5, 6). Increasing evidence suggests that misfolded aSyn triggers microglial activation and astrogliosis, which are thought to accelerate aSyn aggregation and oligodendroglial apoptosis (7–11). Although the initial stimulus in the MSA remains unknown, the inflammation mechanism is common (12). Extensive microglial activation and neuroinflammation with diffuse T-cell infiltration have been found in postmortem studies of MSA brains (8, 13–18). Activated microglia can either take up a pro-inflammatory or an anti-inflammatory phenotype. Depending on the type of activation, microglia release specific inflammatory cytokines, which can be detected in blood, cerebrospinal fluid (CSF), and the brain parenchyma (9) (Figure 1).
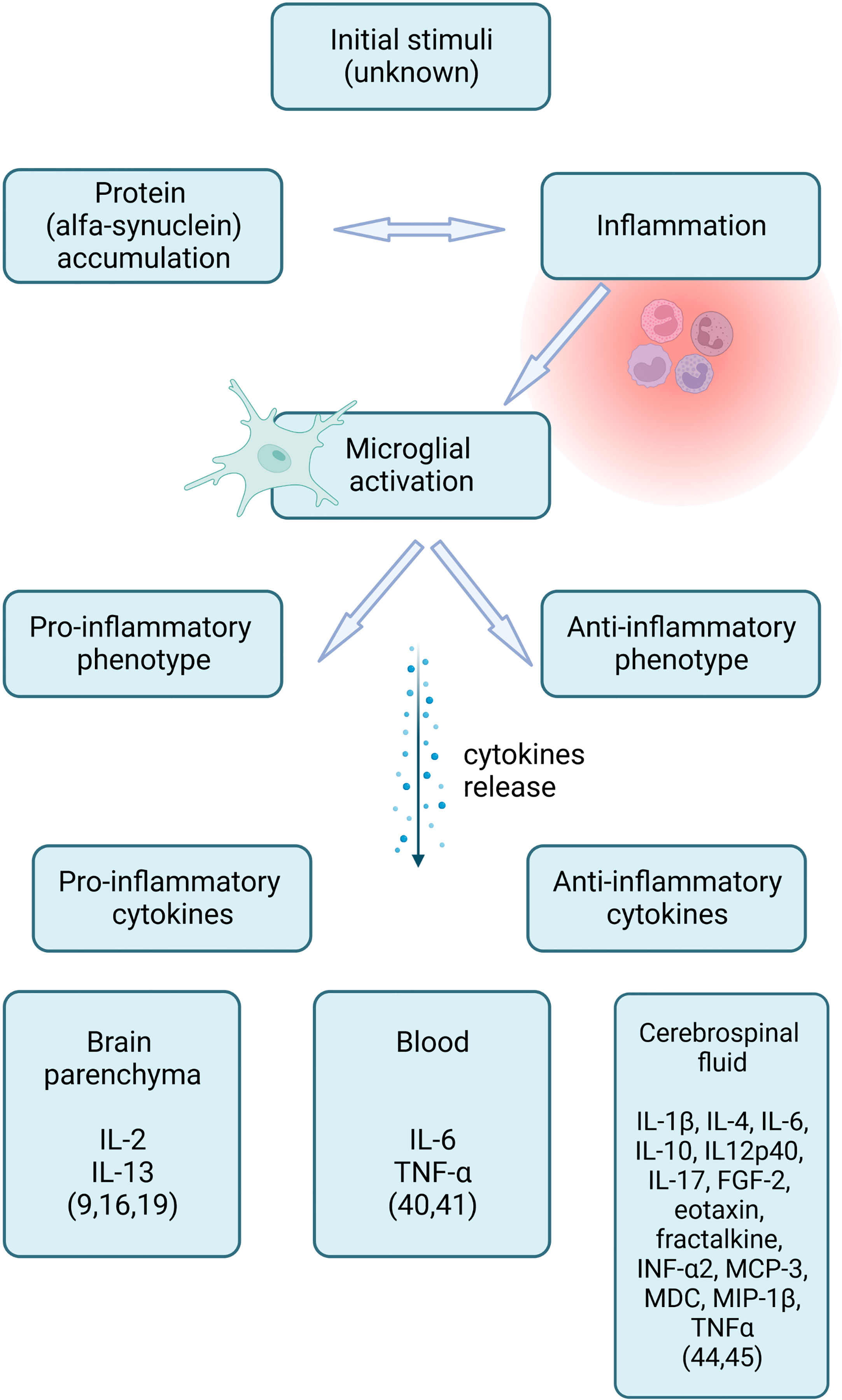
Figure 1 Inflammation and inflammatory cytokines in multiple system atrophy (created with BioRender.com).
3 Immune response in MSA brain parenchyma
Brain inflammatory responses can enhance neuronal excitability, injure cells and increase blood-brain barrier permeability (12). The increased microglia antigen presentation (MHC class II+cells) indicates a reactive immune response (9). CD3+, CD4+, CD8+T cells, and an increase in pro-inflammatory cytokines (IL-2, IL-13) were found in the MSA brain (9, 16, 19). Increased density of interferon stimulator genes, (STING)- and TANK-binding kinase 1 (TBK1)-immunopositive astrocytes, was reported. Continuous STING activation is assumed to contribute to chronic inflammation (20).
Recent studies have shown that innate inflammatory processes cause the deteriorating course of chronic neurodegenerative diseases. Williams et al. proposed a hypothesis of inflammation in a mouse model of MSA, in which the accumulation of aSyn in oligodendrocytes leads to the upregulation of major histocompatibility class II antigen (MCHII) on microglia and promotes the infiltration of the CD4+T cells and monocytes from the blood. All pro-inflammatory cells promote the dysfunction of oligodendrocytes (19).
4 Inflammation-related gene expression
Changes in inflammation-related gene expression occur in the MSA brain, through both down- and up-regulation (9, 21, 22).
Reported changes in DNA methylation or the expression level of genes being more highly expressed in microglia/macrophages support the involvement of inflammatory processes in MSA (17, 23). The dysregulation of inflammation-related intracellular pathways results in aberrant mRNA levels (24).
Gene encoding inflammatory mediators are also an area of interest (11). Selected interleukins (IL-1A, IL-1B, IL-8) and TNF-α genetic polymorphism increased the risk of MSA, as well as variants of Triggering Receptor Expressed on Myeloid Cells 2 (TREM2) and nucleotide-binding oligomerisation domain containing 2 (NOD2) (25–29). Alpha-1-antichymotrypsin (ACT) gene polymorphism is a risk and modulating factor for MSA. Onset was earlier and the disease progressed faster in patients with the ACT-AA genotype (30).
An altered microRNA (miRNA) expression is associated with initiating and/or sustaining neuroinflammation. An increased brain expression of 214 miRNA was found in MSA, and of these dysregulated miRNA 83 were disease-specific (31). Abnormal levels of more than 60 miRNAs were found in the striatum of MSA patients, with miR-124-3p, miR-19a-3p, miR-27b-3p, and miR29c-3p as altered key regulators, mainly contributing to neuroinflammation (32).
Altered expression of miRNA was also found in the blood and plasma of MSA patients, both under- and over-expression. The results of the studies are divergent (33–35).
A study of CSF showed a difference between MSA and controls in four miRNAs (miR-19a, miR-19b, miR-24, miR34c) (36).
5 Activation of inflammatory pathways in MSA
5.1 Toll-like receptors signaling - pattern recognition receptor activation
TLRs have altered expression in the substantia nigra, the striatum, the cerebral cortex, and the nucleus dentatus (37). aSyn real-time quaking-induced conversion (RT-QuIC) products generated by the olfactory mucosa of MSA patients increase the transcription level of several inflammatory mediators, including TLR2 and tumour necrosis factor (TNF) receptor-associated factor 6 (TRAF6) (38). Preformed α-syn fibrils (PFF) increase the association between TLR2 and innate immune signal transduction adaptor MyD88, resulting in microglial activation (39).
5.2 Cytokines in blood and CSF
IL-6 and TNF-α serum levels are increased in MSA (40, 41). Levels of serum inflammatory markers might depend on the disease duration. Kim et al. reported no difference between MSA and controls in serum levels of IL-1β, IL-2, IL-6, IL-10, TNF-α, or high‐sensitivity C reactive protein (CRP). The authors postulated that peripheral inflammation might be vital to the initial pathogenesis of MSA (42).
Although the results are inconsistent, several cytokines and acute phase response (APR) protein levels altered in CSF. The levels of cytokines in MSA were similar to, or less than, the control (43). Compta et al. reported that 12 of the 38 tested cytokines were significantly increased (FGF-2, eotaxin, fractalkine, INF-α2, IL-10, MCP-3, IL12p40, MDC, IL-17, IL-6, MIP-1β, and TNFα) (44). Starhof et al. reported elevated CSF concentration of TFN-α, IL-1β, IL-4 and IL-6 (45).
5.3 Other peripheral inflammatory biomarkers
Some peripheral inflammatory biomarkers are elevated in MSA CSF: CRP, human serum amyloid A (SAA), ceruloplasmin, α1-antichymotrypsin, ferritin, and transthyretin (45–47). Folke et al. reported that anti-aSyn IgM nAbs plasma and CSF levels were reduced, and anti-aSyn IgG1, IgG2, and IgG3 CSF levels increased in MSA (48).
Higher serum monocyte to high-density lipoprotein ratio (MHR), neutrophil-to-lymphocyte ratio (NLR), and red cell distribution width to platelet ratio (RPR) was reported (49). An increase in CD3+ and CD4+ T-lymphocyte, the CD4+/CD8+ ratio, and a decrease in Ig concentration were found, especially in female patients (22).
6 Gut microbiome in MSA
Studies demonstrated altered gut microbiome composition and function with the presence of “pro-inflammatory” bacteria. The bacterial alterations differed between the studies and the studied populations (50). Engen et al. showed intestinal barrier dysfunction with a high relative abundance of gram-negative, putative “pro-inflammatory” bacteria. Patients had evidence of disrupted Zonula Occludens-1 (51). Some gut inflammation risk genes (LRRK2, NOD2) could also increase the risk of MSA, and “pro-inflammatory” bacteria may participate in stimulating MSA pathology (28, 52, 53).
7 Inflammation as a biomarker for MSA diagnosis
CSF inflammatory proteins (DNER, β-NGF) were reported as potential biomarkers, which could differentiate MSA from Parkinson’s Disease (PD) and controls. Both were down-regulated in MSA (54). CSF levels of TFN-α, IL-1β, IL-4, and IL-6 were significantly higher in MSA than in PD, and the best diagnostic discrimination was found with a combination of analytes (45).
MHR serum level was higher in MSA than in PD (49). The diagnostic value of serum miR-30c-5p in discriminating between MSA and PD was reported with a sensitivity of 82% and a specificity of 54% (55).
Two cytokines (MCP-3, MDC) were reported to discriminate MSA from non-MSA patients. In a respective binary logistic regression model combining age at inclusion and both these cytokines, younger age and increasing CSF levels of cytokines were predictors of MSA (44).
Ferritin and transthyretin CSF levels discriminated MSA patients from PD, progressive supranuclear palsy (PSP), and dementia with Lewy bodies (DLB) (47).
8 Inflammation as a biomarker for MSA progression
The more robust responses in the experimental viral oligodendroglial MSA model than in the PD model suggest that inflammation is responsible for the aggressive MSA clinical course (6). CSF CRP and IL-8 correlated with disease severity (46). Up-regulated serum miR-30c-5p levels are associated with disease duration (55). Recently CRP serum level was proposed as a prognostic biomarker related to higher mortality in MSA (56).
9 Challenges for future treatment
To date, there are no available therapies able to modify the course of MSA. Therefore, inflammation as a potential therapeutic target has been intensively studied (Table 1).
Repurposing existing drugs is one idea. Starhof et al. studied the use of anti-inflammatory drugs and the risk of MSA in a register-based case-control study. They observed a link between using non-aspirin non-steroidal anti-inflammatory drugs (NSAID) and the associated reduced risk of MSA (62).
Intriguingly, selective serotonin reuptake inhibitors may be disease-modifying, reducing inflammation-mediated neurodegenerative processes (63, 64).
Reduction of microglial activation may be the next promising future strategy for disease course modification.
Myeloperoxidase (MPO) inhibition in MSA mice in the early stage of the disease improves motor deficits more than in the advanced disease model (59, 60). This suggests time’s importance in MSA therapy when targeting microglial activation (69). The MPO inhibition was targeted in Phase III clinical trial with BHV-3241(verdiperstat). It was recently finished and reported that it failed to meet primary and key secondary endpoints (10, 65).
Suppression of microglial activation by early long-term minocycline treatment and protected dopaminergic neurons of the substantia nigra pars compacta in a transgenic mouse model was reported (61). A phase II randomised controlled trial failed to demonstrate motor improvement or neuroprotective effects (66).
Microglial development, survival, and maintenance depend on the colony-stimulating factor 1 receptor (CSF1R) (70). CSF1R selective inhibitor - PLX5622, which is available orally and crosses the blood-brain barrier, was administered to MSA mice. Reduced inflammatory signals, an extended lifespan, and delayed onset of neurologic symptoms were observed. Converse aggravation of motor deficits and alternation in neuronal and synaptic regulation in MBP29-hα-syn mice were reported. PLX5622 had no restorative effect on oligodendropathy (57).
Dutta et al. reported the intranasal administration of two peptides in preformed fibrils (PFF)-seeded mice. The wild-type TLR2-interaction domain of MyD88 (wtTIDM) peptide inhibits TLR2 activation, and the wild-type of NEMO-biding domain (wtNBD) peptide inhibits TLR2-mediated NF-κB activation. Disruption of TLR2-MyD88 interaction led to attenuation of microglial activation. Therefore wtTIDM and wtNBD might be the next therapeutic targets (39).
Inflammasomes are innate immune system receptors and sensors that regulate the activation of caspase-1 and induce inflammation. The regulation of microglial NLRP3-inflammasome activation was proposed as a potential therapeutic target (16).
In the mouse model, FTY720-Mitoxy reduces microglial activation in the cerebellum and has potent anti-inflammatory effects in vivo (58).
Both anti- and pro-inflammatory cytokines may be promising targets for potential MSA therapy. An anti-inflammatory cytokine, G-CSF induces neurogenesis and counteracts apoptosis. In MSA, a lower level of G-CSF was found. G-CSF was administrated to MSA patients subcutaneously in a minor clinical trial with inconclusive results (67).
The viral oligodendroglial MSA model has significant T-cell infiltration before any cell loss. That suggests the next therapeutic target, the blockade of T-cell infiltration (6, 19).
Intravenous immunoglobulin (IVIG) use in MSA patients was studied by Novak et al. in a small group of 7 patients. Unified Multiple System Atrophy Rating Scale (UMSARS) was improved in all, and UMSARS part II in 5 subjects (68). IgM levels gradually decrease during the disease course. This indicates that immunotherapy should start at the early stage of the disease (22).
Recent failures of single-drug therapies resulted in studies combining therapies targeting different aspects of the disease, with better results than each treatment alone (71).
10 Imaging inflammation in the brain
The main molecular targets in functional imaging of inflammatory processes are microglial cells (72–74) and the translocator protein (TSPO). TSPO (18 kDa) is a well-preserved and tryptophan-rich 169-amino-acid protein located on the outer side of the mitochondrial membrane. A characteristic feature of this protein is its ability to bind to benzodiazepines (72). The upregulation of TSPO is well documented in inflammatory processes.
Several radiopharmaceuticals have been developed that allow the assessment of TSPO expression using both Single-photon Emission Computed Tomography (SPECT) and positron emission tomography (PET) techniques. There are currently three generations of radiopharmaceuticals (75).
The first generation includes benzodiazepines and isoquinoline. [11C]Ro5-4864 belongs to the 4-chlorodiazepam family. It shows good binding affinity (Ki = 6 nM) for TSPO. The radiopharmaceutical is labeled with the C-11 isotope and can be used in the PET technique. This was the first tracer that specifically bound to TSPO but did not bind to the benzodiazepine subunit of the GABA receptor. Another radiopharmaceutical used is isoquinoline ([11C]-PK-11195). Isoquinoline binds to activated microglial cells and is an indicator of glial and macrophage activation.
The second generation belongs to different groups of chemical compounds. It is characterised by a much higher binding specificity to the TPSO protein and, thus a better signal-to-noise ratio (76, 77). An example is Phenoxy-phenylacetamide [11C]DAA1106 or [18F]DAA1106. However, these radiopharmaceuticals bind to microglial cells, astrocytes, and other cellular response cells. This ability to label other inflammatory cells limits the radiopharmaceutical use in studies on the role of microglial cells.
The third generation focused on the imaging of TSPO activity has almost eliminated the shortcomings of the existing tracers. They allow for better and more specific imaging of the activated microglia. Among others, this group includes tricyclic radiotracers like [18F]GE180, [11C]vinpocetine, or [18F]FEBMP and modified radiopharmaceuticals that had been developed previously: [18F]FE-DAA1106, [11C]DPA-713, [18F]DPA-714, [18F]PBR28, [18F]PBR111, [11C]SSR18075, [11C]CLINME, [123I]CLINDE (78–82).
Molecular imaging of the inflammatory processes is one of the essential tools in both research and clinical practice. Further progress in this field will allow us to approximate the pathomechanisms of several neurodegenerative diseases.
11 Inflammation in other atypical parkinsonism – tauopathies: similarities and differences
Inflammation in tauopathies is interpreted as a feature possibly coexisting with tau. However, additional mechanisms may interfere with the inflammatory-neurodegenerative relationship (83). The pathological protein tau in Alzheimer’s Disease (AD), corticobasal syndrome (CBS), and frontotemporal dementia (FTD) are considered initiatory points of inflammation leading to neurodegeneration (84). The accumulation of phosphorylated-tau is thought to be present before microglial activation (85). The activated microglia are observed around neurofibrillary tangles, which were described in the assessment with benzodiazepine receptor radiotracer in PET– [11C]PK11195 (86).
It was found that levels of double-stranded RNA are increased in astrocytes in PSP and AD (87).
Inflammatory genetic risk factors overlap between PD, AD, and FTD, but microglial-activated mechanisms may differ (88). The issue concerning inflammatory mechanisms in tauopathic atypical parkinsonism has not been widely described in the context of PSP. Some studies indicate oxidative stress as a likely significant feature in this context. One of the studies reported protein kinase p38SAPK as possibly affecting oxidative stress (89). In PSP, systemic inflammation is associated with cognitive decline (90). Information concerning CBS is relatively poor. It is affected by the lower incidence of the disease and more diverse pathologies, among which are PSP, CBD, FTD, AD, and, less commonly, vascular injury (91).
The assessments of biochemical and laboratory features of tauopathic parkinsonian syndromes in contemporary literature provide moderately encouraging results in the context of differentiation. Neurofilament light chains, linked with inflammatory-driven neurodegeneration, are increased in CBS and other atypical parkinsonisms, including PSP and MSA, compared to PD (92). Recently a study evaluating inflammatory parameters in Parkinsonian syndromes revealed increased levels of haptoglobin in PSP and ferritin and transthyretin in MSA. The study did not show the significance of these parameters in CBS (47). In PSP and CBS, the possible overlaps in clinical manifestation result in the need to verify whether adequate links can be observed in the context of pathophysiological features. Recent work based on the evaluation of the easily accessible peripheral NLR parameter did not reveal significant differences between PSP and CBS (93). On the other hand, the same parameter showed significantly increased values when compared to PD (94). The peripheral stimulation of the inflammatory mechanism in these diseases may be partly explained by dyslipidemia, vascular risk factors, and oxidative stress, which are related to the pathomechanisms leading to the diseases (91, 95). The abnormalities of inflammatory factors were evaluated in various Parkinsonian syndromes. However, the data suggest that the deviations may not strictly correlate with the pathology. The evaluations show significantly increased levels of CRP, TNF-alpha, IL-1-beta, IL-4, and IL-6 in comparing PSP and PD and between MSA and PD (45). Evaluations based on the analysis of the CSF revealed a pronounced increase of microglial-derived cytokines in PSP (46). The search for links between inflammatory pathways and the risk of PSP was also explored in the context of genes affecting microglial activation – chemokine receptor type 4 (96). Similar observations were made in the context of CBS and FTD.
The links between inflammation, PSP, and CBS were also verified in the context of their correlations with infection. Recently it was described in the context of neurosyphilis. The patient showed clinical manifestation of PSP with a moderate response to antibacterial treatment and a lack of improvement based on levodopa therapy (97). Several previous papers identified cases of clinical manifestations of syphilis as PSP or CBS. They were generally based on single cases and did not provide an overview of the possible mechanism leading to clinical manifestations of tauopathic parkinsonian syndromes (98, 99). A case of progressive supranuclear palsy-like phenotype as one of the primary symptoms of HIV is noteworthy among other instances of PSP possibly related to prior infection (100).
The possible inflammatory aspect of PSP is known in the context of microbiota, as diverse profiles in parkinsonisms may be interpreted as a consequence of microbial communities. In PSP, increased levels of Lactobacillaceae were found, interestingly similar to MSA (101). The possible impact of neuroinflammatory parameters in PSP is a developed feature in pharmacotherapy, as faecal microbiota transplantation was found to improve motor and non-motor elements of clinical manifestation in PSP-Richardson Syndromes (102). The anti-inflammatory impact was also assessed in the context of NSAID. No association between the use of NSAID and the incidence of PSP was revealed however, the study was affected by several limitations: a low number of examined patients and the use of NSAID was verified by phone. Additionally, the criteria of diagnosis used in this study were not contemporary (103, 104). None of the studies indicated the possible significance of differences in inflammatory stimulation depending on the phenotype of PSP. Moreover, the potential anti-inflammatory impact of future drugs in PSP seems likely to be achievable mainly in the preclinical or early stages of the disease. This leads to the need to develop practical methods enabling earlier diagnosis of PSP than is currently possible.
12 Conclusion and remarks
Neuroinflammation is a proposed mechanism in the pathogenesis of MSA and other neurodegenerative diseases. It remains uncertain if inflammation is a primary driver, a response to degeneration, or aggravates the process. Inflammatory biomarkers are potential markers for MSA diagnosis and progression, and inflammation is a potential therapeutic target for disease course modification.
Author contributions
ML-M designed and reviewed the literature, adjusted the structure of the article, wrote drafts of the manuscript, supervised the work, and accepted the final version. PA reviewed the literature, wrote drafts of the manuscript, and accepted the final version. NM-A reviewed the literature, wrote drafts of the manuscript, and accepted the final version. UF supervision and final version acceptance. DK supervision and final version acceptance. LK reviewed the literature, wrote manuscript drafts, supervision, and final version acceptance. All authors contributed to the article and approved the submitted version.
Funding
This work was financially supported by the Centre of Postgraduate Medical Education, Warsaw, Poland (project number: 501-1-014-16-22.
Acknowledgments
Graphics were created with BioRender.com (agreement number: HY25FOQ4UH).
Conflict of interest
The authors declare that the research was conducted without any commercial or financial relationships that could be construed as a potential conflict of interest.
Publisher’s note
All claims expressed in this article are solely those of the authors and do not necessarily represent those of their affiliated organizations, or those of the publisher, the editors and the reviewers. Any product that may be evaluated in this article, or claim that may be made by its manufacturer, is not guaranteed or endorsed by the publisher.
References
1. Wenning GK, Stankovic I, Vignatelli L, Fanciulli A, Calandra-Buonaura G, Seppi K, et al. The movement disorder society criteria for the diagnosis of multiple system atrophy. Mov Disord (2022) 37(6):1131–48. doi: 10.1002/mds.29005
2. Monzio Compagnoni G, Di Fonzo A. Understanding the pathogenesis of multiple system atrophy: state of the art and future perspectives. Acta Neuropathol Commun (2019) 7(1):113. doi: 10.1186/s40478-019-0730-6
3. Jellinger KA. Neuropathology of multiple system atrophy: new thoughts about pathogenesis. Mov Disord (2014) 29(14):1720–41. doi: 10.1002/mds.26052
4. Hoffmann A, Ettle B, Battis K, Reiprich S, Schlachetzki JCM, Masliah E, et al. Oligodendroglial α-synucleinopathy-driven neuroinflammation in multiple system atrophy. Brain Pathol (2019) 29(3):380–96. doi: 10.1111/bpa.12678
5. Bourdenx M, Koulakiotis NS, Sanoudou D, Bezard E, Dehay B, Tsarbopoulos A. Protein aggregation and neurodegeneration in prototypical neurodegenerative diseases: examples of amyloidopathies, tauopathies and synucleinopathies. Prog Neurobiol (2017) 155:171–93. doi: 10.1016/j.pneurobio.2015.07.003
6. Harms AS, Kordower JH, Sette A, Lindestam Arlehamn CS, Sulzer D, Mach RH. Inflammation in experimental models of α-synucleinopathies. Mov Disord (2021) 36(1):37–49. doi: 10.1002/mds.28264
7. Vieira BD, Radford RA, Chung RS, Guillemin GJ, Pountney DL. Neuroinflammation in multiple system atrophy: response to and cause of α-synuclein aggregation. Front Cell Neurosci (2015) 9:437. doi: 10.3389/fncel.2015.00437
8. Refolo V, Stefanova N. Neuroinflammation and glial phenotypic changes in alpha-synucleinopathies. Front Cell Neurosci (2019) 13:263. doi: 10.3389/fncel.2019.00263
9. Rydbirk R, Elfving B, Andersen MD, Langbøl MA, Folke J, Winge K, et al. Cytokine profiling in the prefrontal cortex of parkinson's disease and multiple system atrophy patients. Neurobiol Dis (2017) 106:269–78. doi: 10.1016/j.nbd.2017.07.014
10. Sidoroff V, Bower P, Stefanova N, Fanciulli A, Stankovic I, Poewe W, et al. Disease-modifying therapies for multiple system atrophy: where are we in 2022? J Parkinsons Dis (2022) 12(5):1369–87. doi: 10.3233/JPD-223183
11. Tseng FS, Foo JQX, Mai AS, Tan EK. The genetic basis of multiple system atrophy. J Transl Med (2023) 21(1):104. doi: 10.1186/s12967-023-03905-1
12. Chen L, Deng H, Cui H, Fang J, Zuo Z, Deng J, et al. Inflammatory responses and inflammation-associated diseases in organs. Oncotarget. (2017) 9(6):7204–18. doi: 10.18632/oncotarget.23208
13. Schwarz J, Weis S, Kraft E, Tatsch K, Bandmann O, Mehraein P, et al. Signal changes on MRI and increases in reactive microgliosis, astrogliosis, and iron in the putamen of two patients with multiple system atrophy. J Neurol Neurosurg Psychiatry (1996) 60(1):98–101. doi: 10.1136/jnnp.60.1.98
14. Gerhard A, Banati RB, Goerres GB, Cagnin A, Myers R, Gunn RN, et al. [11C](R)-PK11195 PET imaging of microglial activation in multiple system atrophy. Neurology. (2003) 61(5):686–9. doi: 10.1212/01.wnl.0000078192.95645.e6
15. Ishizawa K, Komori T, Sasaki S, Arai N, Mizutani T, Hirose T. Microglial activation parallels system degeneration in multiple system atrophy. J Neuropathol Exp Neurol (2004) 63(1):43–52. doi: 10.1093/jnen/63.1.43
16. Li F, Ayaki T, Maki T, Sawamoto N, Takahashi R. NLRP3 inflammasome-related proteins are upregulated in the putamen of patients with multiple system atrophy. J Neuropathol Exp Neurol (2018) 77(11):1055–65. doi: 10.1093/jnen/nly090
17. Kiely AP, Murray CE, Foti SC, Benson BC, Courtney R, Strand C, et al. Immunohistochemical and molecular investigations show alteration in the inflammatory profile of multiple system atrophy brain. J Neuropathol Exp Neurol (2018) 77(7):598–607. doi: 10.1093/jnen/nly035
18. Kübler D, Wächter T, Cabanel N, Su Z, Turkheimer FE, Dodel R, et al. Widespread microglial activation in multiple system atrophy. Mov Disord (2019) 34(4):564–8. doi: 10.1002/mds.27620
19. Williams GP, Marmion DJ, Schonhoff AM, Jurkuvenaite A, Won WJ, Standaert DG, et al. T Cell infiltration in both human multiple system atrophy and a novel mouse model of the disease. Acta Neuropathol (2020) 139(5):855–74. doi: 10.1007/s00401-020-02126-w
20. Inoue Y, Ayaki T, Ishimoto T, Yamakado H, Maki T, Matsuzawa S, et al. The stimulator of interferon genes (STING) pathway is upregulated in striatal astrocytes of patients with multiple system atrophy. Neurosci Lett (2021) 757:135972. doi: 10.1016/j.neulet.2021.135972
21. Langerveld AJ, Mihalko D, DeLong C, Walburn J, Ide CF. Gene expression changes in postmortem tissue from the rostral pons of multiple system atrophy patients. Mov Disord (2007) 22(6):766–77. doi: 10.1002/mds.21259
22. Cao B, Chen X, Zhang L, Wei Q, Liu H, Feng W, et al. Elevated percentage of CD3+ T-cells and CD4+/CD8+ ratios in multiple system atrophy patients. Front Neurol (2020) 11:658. doi: 10.3389/fneur.2020.00658
23. Bettencourt C, Piras IS, Foti SC, Talboom J, Miki Y, Lashley T, et al. Epigenomics and transcriptomics analyses of multiple system atrophy brain tissue supports a role for inflammatory processes in disease pathogenesis. Acta Neuropathol Commun (2020) 8(1):71. doi: 10.1186/s40478-020-00946-1
24. Schafferer S, Khurana R, Refolo V, Venezia S, Sturm E, Piatti P, et al. Changes in the miRNA-mRNA regulatory network precede motor symptoms in a mouse model of multiple system atrophy: clinical implications. PloS One (2016) 11(3):e0150705. doi: 10.1371/journal.pone.0150705
25. Combarros O, Infante J, Llorca J, Berciano J. Interleukin-1A (-889) genetic polymorphism increases the risk of multiple system atrophy. Mov Disord (2003) 18(11):1385–6. doi: 10.1002/mds.10540
26. Infante J, Llorca J, Berciano J, Combarros O. Interleukin-8, intercellular adhesion molecule-1 and tumour necrosis factor-alpha gene polymorphisms and the risk for multiple system atrophy. J Neurol Sci (2005) 228(1):11–3. doi: 10.1016/j.jns.2004.09.023
27. Zhou X, Wang C, Chen Z, Peng Y, Peng H, Hou X, et al. Association of TNF-α rs1799964 and IL-1β rs16944 polymorphisms with multiple system atrophy in Chinese han population. Int J Neurosci (2018) 128(8):761–4. doi: 10.1080/00207454.2017.1418346
28. Cao B, Chen Y, Zhou Q, Zhang L, Ou R, Wei Q, et al. Functional variant rs3135500 in NOD2 increases the risk of multiple system atrophy in a Chinese population. Front Aging Neurosci (2018) 10:150. doi: 10.3389/fnagi.2018.00150
29. Ogaki K, Heckman MG, Koga S, Martens YA, Labbé C, Lorenzo-Betancor O, et al. Association study between multiple system atrophy and TREM2 p. R47H Neurol Genet (2018) 4(4):e257. doi: 10.1212/NXG.0000000000000257
30. Furiya Y, Hirano M, Kurumatani N, Nakamuro T, Matsumura R, Futamura N, et al. Alpha-1-antichymotrypsin gene polymorphism and susceptibility to multiple system atrophy (MSA). Brain Res Mol Brain Res (2005) 138(2):178–81. doi: 10.1016/j.molbrainres.2005.04.011
31. Ubhi K, Rockenstein E, Kragh C, Inglis C, Spencer B, Michael S, et al. Widespread microRNA dysregulation in multiple system atrophy - disease-related alteration in miR-96. Eur J Neurosci (2014) 39(6):1026–41. doi: 10.1111/ejn.12444
32. Kim T, Valera E, Desplats P. Alterations in striatal microRNA-mRNA networks contribute to neuroinflammation in multiple system atrophy. Mol Neurobiol (2019) 56(10):7003–21. doi: 10.1007/s12035-019-1577-3
33. Vallelunga A, Ragusa M, Di Mauro S, Iannitti T, Pilleri M, Biundo R, et al. Identification of circulating microRNAs for the differential diagnosis of parkinson's disease and multiple system atrophy. Front Cell Neurosci (2014) 8:156. doi: 10.3389/fncel.2014.00156
34. Kume K, Iwama H, Deguchi K, Ikeda K, Takata T, Kokudo Y, et al. Serum microRNA expression profiling in patients with multiple system atrophy. Mol Med Rep (2018) 17(1):852–60. doi: 10.3892/mmr.2017.7995
35. Uwatoko H, Hama Y, Iwata IT, Shirai S, Matsushima M, Yabe I, et al. Identification of plasma microRNA expression changes in multiple system atrophy and parkinson's disease. Mol Brain (2019) 12(1):49. doi: 10.1186/s13041-019-0471-2
36. Marques TM, Kuiperij HB, Bruinsma IB, van Rumund A, Aerts MB, Esselink RAJ, et al. MicroRNAs in cerebrospinal fluid as potential biomarkers for parkinson's disease and multiple system atrophy. Mol Neurobiol (2017) 54(10):7736–45. doi: 10.1007/s12035-016-0253-0
37. Brudek T, Winge K, Agander TK, Pakkenberg B. Screening of toll-like receptors expression in multiple system atrophy brains. Neurochem Res (2013) 38(6):1252–9. doi: 10.1007/s11064-013-1020-5
38. De Luca CMG, Consonni A, Cazzaniga FA, Bistaffa E, Bufano G, Quitarrini G, et al. The alpha-synuclein RT-QuIC products generated by the olfactory mucosa of patients with parkinson's disease and multiple system atrophy induce inflammatory responses in SH-SY5Y cells. Cells. (2021) 11(1):87. doi: 10.3390/cells11010087
39. Dutta D, Jana M, Majumder M, Mondal S, Roy A, Pahan K. Selective targeting of the TLR2/MyD88/NF-κB pathway reduces α-synuclein spreading in vitro and in vivo. Nat Commun (2021) 12(1):5382. doi: 10.1038/s41467-021-25767-1
40. Brodacki B, Staszewski J, Toczyłowska B, Kozłowska E, Drela N, Chalimoniuk M, et al. Serum interleukin (IL-2, IL-10, IL-6, IL-4), TNFalpha, and INFgamma concentrations are elevated in patients with atypical and idiopathic parkinsonism. Neurosci Lett (2008) 441(2):158–62. doi: 10.1016/j.neulet.2008.06.040
41. Kaufman E, Hall S, Surova Y, Widner H, Hansson O, Lindqvist D. Proinflammatory cytokines are elevated in serum of patients with multiple system atrophy. PloS One (2013) 8(4):e62354. doi: 10.1371/journal.pone.0062354
42. Kim R, Kim HJ, Kim A, Jang M, Kim A, Kim Y, et al. Does peripheral inflammation contribute to multiple system atrophy? Parkinsonism Relat Disord (2019) 64:340–1. doi: 10.1016/j.parkreldis.2019.03.020
43. Csencsits-Smith K, Suescun J, Li K, Luo S, Bick DL, Schiess M. Serum lymphocyte-associated cytokine concentrations change more rapidly over time in multiple system atrophy compared to Parkinson disease. Neuroimmunomodulation. (2016) 23(5-6):301–8. doi: 10.1159/000460297
44. Compta Y, Dias SP, Giraldo DM, Pérez-Soriano A, Muñoz E, Saura J, et al. Cerebrospinal fluid cytokines in multiple system atrophy: a cross-sectional Catalan MSA registry study. Parkinsonism Relat Disord (2019) 65:3–12. doi: 10.1016/j.parkreldis.2019.05.040
45. Starhof C, Winge K, Heegaard NHH, Skogstrand K, Friis S, Hejl A. Cerebrospinal fluid pro-inflammatory cytokines differentiate parkinsonian syndromes. J Neuroinflammation (2018) 15(1):305. doi: 10.1186/s12974-018-1339-6
46. Hall S, Janelidze S, Surova Y, Widner H, Zetterberg H, Hansson O. Cerebrospinal fluid concentrations of inflammatory markers in parkinson's disease and atypical parkinsonian disorders. Sci Rep (2018) 8(1):13276. doi: 10.1038/s41598-018-31517-z
47. Ayton S, Hall S, Janelidze S, Kalinowski P, Palmqvist S, Belaidi AA, et al. The neuroinflammatory acute phase response in parkinsonian-related disorders. Mov Disord (2022) 37(5):993–1003. doi: 10.1002/mds.28958
48. Folke J, Rydbirk R, Løkkegaard A, Hejl AM, Winge K, Starhof C, et al. Cerebrospinal fluid and plasma distribution of anti-α-synuclein IgMs and IgGs in multiple system atrophy and parkinson's disease. Parkinsonism Relat Disord (2021) 87:98–104. doi: 10.1016/j.parkreldis.2021.05.001
49. Jiang L, Zhong Z, Huang J, Bian H, Huang W. Monocytohigh-density lipoprotein ratio has a high predictive value for the diagnosis of multiple system atrophy and the differentiation from parkinson's disease. Front Aging Neurosci (2022) 14:1035437. doi: 10.3389/fnagi.2022.1035437
50. Wan L, Zhou X, Wang C, Chen Z, Peng H, Hou X, et al. Alterations of the gut microbiota in multiple system atrophy patients. Front Neurosci (2019) 13:1102. doi: 10.3389/fnins.2019.01102
51. Engen PA, Dodiya HB, Naqib A, Forsyth CB, Green SJ, Voigt RM, et al. The potential role of gut-derived inflammation in multiple system atrophy. J Parkinsons Dis (2017) 7(2):331–46. doi: 10.3233/JPD-160991
52. Hor JW, Lim SY, Khor ES, Chong KK, Song SL, Ibrahim NM, et al. Fecal calprotectin in parkinson's disease and multiple system atrophy. J Mov Disord (2022) 15(2):106–14. doi: 10.14802/jmd.21085
53. Tan AH, Chong CW, Song SL, Teh CSJ, Yap IKS, Loke MF, et al. Altered gut microbiome and metabolome in patients with multiple system atrophy. Mov Disord (2018) 33(1):174–6. doi: 10.1002/mds.27203
54. Santaella A, Kuiperij HB, van Rumund A, Esselink RAJ, van Gool AJ, Bloem BR, et al. Inflammation biomarker discovery in parkinson's disease and atypical parkinsonisms. BMC Neurol (2020) 20(1):26. doi: 10.1186/s12883-020-1608-8
55. Vallelunga A, Iannitti T, Dati G, Capece S, Maugeri M, Tocci E, et al. Serum miR-30c-5p is a potential biomarker for multiple system atrophy. Mol Biol Rep (2019) 46(2):1661–6. doi: 10.1007/s11033-019-04614-z
56. Shin JH, Kim HJ, Lee CY, Chang HJ, Woo KA, Jeon B. Laboratory prognostic factors for the long-term survival of multiple system atrophy. NPJ Parkinsons Dis (2022) 8(1):141. doi: 10.1038/s41531-022-00413-9
57. Battis K, Florio JB, Mante M, Lana A, Naumann I, Gauer C, et al. CSF1R-mediated myeloid cell depletion prolongs lifespan but aggravates distinct motor symptoms in a model of multiple system atrophy. J Neurosci (2022) 42(40):7673–88. doi: 10.1523/JNEUROSCI.0417-22.2022
58. Vidal-Martinez G, Segura-Ulate I, Yang B, Diaz-Pacheco V, Barragan JA, De-Leon Esquivel J, et al. FTY720-mitoxy reduces synucleinopathy and neuroinflammation, restores behavior and mitochondria function, and increases GDNF expression in multiple system atrophy mouse models. Exp Neurol (2020) 325:113120. doi: 10.1016/j.expneurol.2019.113120
59. Stefanova N, Georgievska B, Eriksson H, Poewe W, Wenning GK. Myeloperoxidase inhibition ameliorates multiple system atrophy-like degeneration in a transgenic mouse model. Neurotox Res (2012) 21(4):393–404. doi: 10.1007/s12640-011-9294-3
60. Kaindlstorfer C, Sommer P, Georgievska B, Mather RJ, Kugler AR, Poewe W, et al. Failure of neuroprotection despite microglial suppression by delayed-start myeloperoxidase inhibition in a model of advanced multiple system atrophy: clinical implications. Neurotox Res (2015) 28(3):185–94. doi: 10.1007/s12640-015-9547-7
61. Stefanova N, Reindl M, Neumann M, Kahle PJ, Poewe W, Wenning GK. Microglial activation mediates neurodegeneration related to oligodendroglial alpha-synucleinopathy: implications for multiple system atrophy. Mov Disord (2007) 22(15):2196–203. doi: 10.1002/mds.21671
62. Starhof C, Hejl AM, Korbo L, Winge K, Friis S. Risk of multiple system atrophy and the use of anti-inflammatory drugs: a Danish register-based case-control study. Neuroepidemiology. (2020) 54(1):58–63. doi: 10.1159/000503003
63. Zhang F, Zhou H, Wilson BC, Shi JS, Hong JS, Gao HM. Fluoxetine protects neurons against microglial activation-mediated neurotoxicity. Parkinsonism Relat Disord (2012) 18 Suppl 1(0 1):S213–7. doi: 10.1016/S1353-8020(11)70066-9
64. Grossauer A, Sidoroff V, Heim B, Seppi K. Symptomatic care in multiple system atrophy: state of the art. Cerebellum. (2022) 22(3):433–446. doi: 10.1007/s12311-022-01411-6
65. Przewodowska D, Marzec W, Madetko N. Novel therapies for parkinsonian syndromes-recent progress and future perspectives. Front Mol Neurosci (2021) 14:720220. doi: 10.3389/fnmol.2021.720220
66. Dodel R, Spottke A, Gerhard A, Reuss A, Reinecker S, Schimke N, et al. Minocycline 1-year therapy in multiple-system-atrophy: effect on clinical symptoms and [(11)C] (R)-PK11195 PET (MEMSA-trial). Mov Disord (2010) 25(1):97–107. doi: 10.1002/mds.22732
67. Pezzoli G, Tesei S, Canesi M, Sacilotto G, Vittorio M, Mizuno Y, et al. The effect of repeated administrations of granulocyte colony stimulating factor for blood stem cells mobilization in patients with progressive supranuclear palsy, corticobasal degeneration and multiple system atrophy. Clin Neurol Neurosurg (2010) 112(1):65–7. doi: 10.1016/j.clineuro.2009.08.023
68. Novak P, Williams A, Ravin P, Zurkiya O, Abduljalil A, Novak V. Treatment of multiple system atrophy using intravenous immunoglobulin. BMC Neurol (2012) 12:131. doi: 10.1186/1471-2377-12-131
69. Heras-Garvin A, Stefanova N. MSA: from basic mechanisms to experimental therapeutics. Parkinsonism Relat Disord (2020) 73:94–104. doi: 10.1016/j.parkreldis.2020.01.010
70. Stanley ER, Chitu V. CSF-1 receptor signaling in myeloid cells. Cold Spring Harb Perspect Biol (2014) 6(6):a021857. doi: 10.1101/cshperspect.a021857
71. Valera E, Spencer B, Fields JA, Trinh I, Adame A, Mante M, et al. Combination of alpha-synuclein immunotherapy with anti-inflammatory treatment in a transgenic mouse model of multiple system atrophy. Acta Neuropathol Commun (2017) 5(1):2. doi: 10.1186/s40478-016-0409-1
72. Papadopoulos V, Baraldi M, Guilarte TR, Knudsen TB, Lacapère JJ, Lindemann P, et al. Translocator protein (18 kDa): new nomenclature for the peripheral-type benzodiazepine receptor based on its structure and molecular function. Trends Pharmacol Sci (2006) 27:402–9. doi: 10.1016/j.tips.2006.06.005
73. Casellas P, Galiegue S, Basile AS. Peripheral benzodiazepine receptors and mitochondrial function. Neurochem Int (2002) 40:475–86. doi: 10.1016/s0197-0186(01)00118-8
74. Perry VH, Nicoll JA. Holmes C microglia in neurodegenerative disease. Nat Rev Neurol (2010) 6:193–201. doi: 10.1038/nrneurol.2010.17
75. Singh P, Adhikari A, Singh D, Gond Ch, Tiwari AK. The 18-kDa translocator protein PET tracers as a diagnostic marker for neuroinflammation: development and current standing. ACS Omega (2022) 18:14412–29. doi: 10.1021/acsomega.2c00588
76. Grimm A, Lejri I, Hallé F, Schmitt M, Götz J, Bihel F, et al. Mitochondria modulatory effects of new TSPO ligands in a cellular model of tauopathies. Neuroendocrinol. (2020) 32(1):e12796. doi: 10.1111/jne.12796
77. Taliani S, Pugliesi I, Da Settimo F. Structural requirements to obtain highly potent and selective 18 kDa translocator protein (TSPO) ligands. Curr Top Med Chem (2011) 11(7):860–86. doi: 10.2174/156802611795165142
78. Wadsworth H, Jones PA, Chau WF, Durrant C, Fouladi N, Passmore J, et al. [¹⁸F]GE-180: a novel fluorine-18 labelled PET tracer for imaging translocator protein 18 kDa (TSPO). Bioorg Med Chem Lett (2012) 22(3):1308–13. doi: 10.1016/j.bmcl.2011.12.084
79. Boutin H, Murray K, Pradillo J, Maroy R, Smigova A, Gerhard A, et al. 18F-GE-180: a novel TSPO radiotracer compared to 11C-R-PK11195 in a preclinical model of stroke. Eur J Nucl Med Mol Imaging (2015) 42(3):503–11. doi: 10.1007/s00259-014-2939-8
80. Chau WF, Black AM, Clarke A, Durrant C, Gausemel I, Khan I, et al. Exploration of the impact of stereochemistry on the identification of the novel translocator protein PET imaging agent [(18)F]GE-180. Nucl Med Biol (2015) 42(9):711–9. doi: 10.1016/j.nucmedbio.2015.05.004
81. Fan Z, Calsolaro V, Atkinson RA, Femminella GD, Waldman A, Buckley C, et al. Flutriciclamide (18F-GE180) PET: first-in-Human PET study of novel third-generation In vivo marker of human translocator protein. J Nucl Med (2016) 57(11):1753–9. doi: 10.2967/jnumed.115.169078
82. Sokias R, Werry EL, Alison Cheng HW, Lloyd JH, Sohler G, Danon JJ, et al. Tricyclic heterocycles display diverse sensitivity to the A147T TSPO polymorphism. Eur J Med Chem (2020) 207:112725. doi: 10.1016/j.ejmech.2020.112725
83. Malpetti M, Passamonti L, Rittman T, Jones PS, Vázquez Rodríguez P, Bevan-Jones WR, et al. Neuroinflammation and tau colocalize in vivo in progressive supranuclear palsy. Ann Neurol (2020) 88(6):1194–204. doi: 10.1002/ana.25911
84. Shin J, Kim HJ, Jeon B. Immunotherapy targeting neurodegenerative proteinopathies: α-synucleinopathies and tauopathies. J Mov Disord (2020) 13(1):11–9. doi: 10.14802/jmd.19057
85. van Olst L, Verhaege D, Franssen M, Kamermans A, Roucourt B, Carmans S, et al. Microglial activation arises after aggregation of phosphorylated-tau in a neuron-specific P301S tauopathy mouse model. Neurobiol Aging (2020) 89:89–98. doi: 10.1016/j.neurobiolaging.2020.01.003
86. Gerhard A, Trender-Gerhard I, Turkheimer F, Quinn NP, Bhatia KP, Brooks DJ. In vivo imaging of microglial activation with [11C](R)-PK11195 PET in progressive supranuclear palsy. Mov Disord (2006) 21(1):89–93. doi: 10.1002/mds.20668
87. Ochoa E, Ramirez P, Gonzalez E, De Mange J, Ray WJ, Bieniek KF, et al. Pathogenic tau-induced transposable element-derived dsRNA drives neuroinflammation. Sci Adv (2023) 9(1):eabq5423. doi: 10.1126/sciadv.abq5423
88. Rexach JE, Polioudakis D, Yin A, Swarup V, Chang TS, Nguyen T, et al. Tau pathology drives dementia risk-associated gene networks toward chronic inflammatory states and immunosuppression. Cell Rep (2020) 33(7):108398. doi: 10.1016/j.celrep.2020.108398
89. Alausa A, Ogundepo S, Olaleke B, Adeyemi R, Olatinwo M, Ismail A. Chinese Nutraceuticals and physical activity; their role in neurodegenerative tauopathies. Chin Med (2021) 16(1):1. doi: 10.1186/s13020-020-00418-7
90. Bäckström D, Granåsen G, Mo SJ, Riklund K, Trupp M, Zetterberg H, et al. Prediction and early biomarkers of cognitive decline in Parkinson disease and atypical parkinsonism: a population-based study. Brain Commun (2022) 4(2):fcac040. doi: 10.1093/braincomms/fcac040
91. Dunalska A, Pikul J, Schok K, Wiejak KA, Alster P. The significance of vascular pathogenesis in the examination of corticobasal syndrome. Front Aging Neurosci (2021) 13:668614. doi: 10.3389/fnagi.2021.668614
92. Bridel C, van Wieringen WN, Zetterberg H, Tijms BM, Teunissen CE, the NFL Group, et al. Diagnostic value of cerebrospinal fluid neurofilament light protein in neurology: a systematic review and meta-analysis. JAMA Neurol (2019) 76(9):1035–48. doi: 10.1001/jamaneurol.2019.1534
93. Alster P, Madetko N, Friedman A. Neutrophil-to-lymphocyte ratio (NLR) at boundaries of progressive supranuclear palsy syndrome (PSPS) and corticobasal syndrome (CBS). Neurol Neurochir Pol (2021) 55(1):97–101. doi: 10.5603/PJNNS.a2020.0097
94. Inci I, Kusbeci OY, Eskut N. The neutrophil-to-lymphocyte ratio as a marker of peripheral inflammation in progressive supranuclear palsy: a retrospective study. Neurol Sci (2020) 41(5):1233–7. doi: 10.1007/s10072-019-04208-4
95. Madetko-Alster N, Alster P, Bartošová T, Klempíř J, Migda B, Przewodowska D, et al. Could hyperlipidemia be a risk factor for corticobasal syndrome? - a pilot study. Neurol Neurochir Pol (2022) 57(2):117–182. doi: 10.5603/PJNNS.a2022.0078
96. Yokoyama JS, Karch CM, Fan CC, Bonham LW, Kouri N, Ross OA, et al. Shared genetic risk between corticobasal degeneration, progressive supranuclear palsy, and frontotemporal dementia. Acta Neuropathol (2017) 133:825–37. doi: 10.1007/s00401-017-1693-y
97. Rissardo JP, Caprara AL F. Parkinsonism-plus syndrome secondary to neurosyphilis: case report and literature review. Neurol India (2022) 70(4):1635–8. doi: 10.4103/0028-3886.355119
98. Benito-León J, Alvarez-Linera J, Louis ED. Neurosyphilis masquerading as corticobasal degeneration. Mov Disord (2004) 19:1367–70. doi: 10.1002/mds.20221
99. Murialdo A, Marchese R, Abbruzzese G, Tabaton M, Michelozzi G, Schiavoni S. Neurosyphilis presenting as progressive supranuclear palsy. Mov Disord (2000) 15:730–1. doi: 10.1002/1531-8257(200007)15:4<730::aid-mds1020>3.0.co;2-o
100. Jang W, Kim JS, Ahn JY, Kim HT. Reversible progressive supranuclear palsy-like phenotype as an initial manifestation of HIV infection. Neurol Sci (2012) 33(5):1169–71. doi: 10.1007/s10072-011-0875-y
101. Jabbari E, Zetterberg H, Morris HR. Tracking and predicting disease progression in progressive supranuclear palsy: CSF and blood biomarkers. J Neurol Neurosurg Psychiatry (2017) 88(10):883–8. doi: 10.1136/jnnp-2017-315857
102. Tian H, Wang J, Feng R, Zhang R, Liu H, Qin C, et al. Efficacy of faecal microbiota transplantation in patients with progressive supranuclear palsy-richardson's syndrome: a phase 2, single centre, randomised clinical trial. EClinicalMedicine. (2023) 58:101888. doi: 10.1016/j.eclinm.2023.101888
103. Marras C, Cunningham CR, Hou J, Proudfoot J, Standaert DG, Juncos J, et al. Anti-inflammatory drug use and progressive supranuclear palsy. Parkinsonism Relat Disord (2018) 48:89–92. doi: 10.1016/j.parkreldis.2017.11.346
Keywords: multiple system atrophy (MSA), neurodegeneration, inflammation, synucleinopathies, tauopathies
Citation: Leńska-Mieciek M, Madetko-Alster N, Alster P, Królicki L, Fiszer U and Koziorowski D (2023) Inflammation in multiple system atrophy. Front. Immunol. 14:1214677. doi: 10.3389/fimmu.2023.1214677
Received: 30 April 2023; Accepted: 06 June 2023;
Published: 22 June 2023.
Edited by:
Maryna Skok, Palladin Institute of Biochemistry (NAS Ukraine), UkraineReviewed by:
Wojciech Ambrosius, Poznan University of Medical Sciences, PolandCopyright © 2023 Leńska-Mieciek, Madetko-Alster, Alster, Królicki, Fiszer and Koziorowski. This is an open-access article distributed under the terms of the Creative Commons Attribution License (CC BY). The use, distribution or reproduction in other forums is permitted, provided the original author(s) and the copyright owner(s) are credited and that the original publication in this journal is cited, in accordance with accepted academic practice. No use, distribution or reproduction is permitted which does not comply with these terms.
*Correspondence: Marta Leńska-Mieciek, bWxlbnNrYUBjbWtwLmVkdS5wbA==