- 1Department of Ophthalmology, West China Hospital, Sichuan University, Chengdu, China
- 2Department of Ophthalmology, ShangjinNanfu Hospital, Chengdu, China
Exosomes are membrane-bound tiny particles that are released by all live cells that contain multiple signal molecules and extensively participate in numerous normal physical activities and pathologies. In glaucoma, the crucial role of exosome-based crosstalk has been primarily revealed in animal models and ex vivo cell studies in the recent decade. In the aqueous drainage system, exosomes derived from non-pigment ciliary epithelium act in an endocrine manner and specifically regulate the function of the trabecular meshwork to cope with persistent oxidative stress challenges. In the retina, a more complicated regulatory network among microglia, retinal neurons, retinal ganglial cells, retinal pigment epithelium, and other immune effector cells by exosomes are responsible for the elaborate modulation of tissue homeostasis under physical state and the widespread propagation of neuroinflammation and its consequent neurodegeneration in glaucoma pathogenesis. Accumulating evidence indicates that exosome-based crosstalk depends on numerous factors, including the specific cargos they carried (particularly micro RNA), concentration, size, and ionization potentials, which largely remain elusive. In this narrative review, we summarize the latest research focus of exosome-based crosstalk in glaucoma pathogenesis, the current research progress of exosome-based therapy for glaucoma and provide in-depth perspectives on its current research gap.
1 Introduction
Glaucoma is a multifactorial disease characterized by progressive damage to the retinal ganglial cells (RGCs) and consequent visual impairment (1). Many pathological processes, including oxidative stress, chronic neuroinflammation, mechanical damage with elevated intraocular pressure (IOP), mitochondrial dysfunction, impaired micro-circulation, and extracellular matrix remodeling have been found to contribute to its irreversible damage to the retina yet the associated mechanisms remain elusive (2–4). Due to the complexity, chronicity, and irreversibility of glaucoma, current medical and surgical treatment which is mainly based on IOP management fail to provide sufficient efficacy in a number of patients who progress to end-stage glaucoma and suffer from poor vision and chronic pain (5). In the recent decade, the focus of glaucoma research and management undergoes paradigm shifts from the previous focus on IOP-induced damage to prolonged neuroinflammation and neurodegeneration, with persistent efforts on regenerative therapy to reverse the damage of the retina (6–8). As discussed in detail in our previous reviews, there is accumulating evidence that the breakdown of the blood retinal barrier (BRB) and activation of immune effector cells, including microglia, T cells, plasma cells, macrophages, and glial cells participate in the entire process of glaucomatous neuroinflammation and precede the development of retinal damage (9, 10). However, how immune cells are synchronized and organized to induce neuroinflammation is poorly understood and remains the frontier of glaucoma research.
Conventionally, signal molecules, including cytokines, chemokines, growth factors, and hormones are found to be regulators of cell states and contribute to maintaining tissue homeostasis and their imbalance have been extensively studied in glaucoma pathogenesis. Recently, a new way of intercellular communication based on exosomes has been revealed to play a significant role in glaucoma (11–20). Exosomes are tiny membrane-bound nanovesicles with a size of 30 – 150 μm that are constitutively produced by all live cells (21). Exosomes contain several active biological materials, including proteins, messenger RNA (mRNA), micro RNA (miRNA), small interfering RNA (siRNA), and transcription factors that can regulate the function of recipient cells (22). Although exosomes are ubiquitous in the body, they demonstrate the selectivity of action which is achieved by the recognition and internalization through surface receptors (23, 24). Emerging evidence from animal studies and ex vivo cell experiments describes the central role of exosome-based crosstalk between trabecular meshwork (TM) and non-pigment ciliary epithelium (NPCE) in the modulation of aqueous drainage in reaction to oxidative stress and the crosstalk among microglia, retinal pigment epithelium (RPE), and retinal ganglial cells in the regulation of retinal inflammation and neurodegeneration (12, 13, 15, 25–27). In addition, exosome-based therapy has been primarily explored in animal models of glaucoma and shows promising results, despite numerous knowledge gaps to be explored (28–35). This review comprehensively summarizes the physical, pathological, and therapeutic role of exosomes associated with glaucoma, focusing on oxidative stress and retinal neuroinflammation.
2 Exosome-mediated crosstalk between NPCE and TM in the modulation of oxidative stress and extracellular matrix remodeling
The NPCE and TM are key components of the aqueous humor production and drainage system and play a vital role in the regulation of IOP. In patients with primary open-angle glaucoma (POAG), stiffened extracellular matrix builds up in the aqueous drainage system of the TM and Scheme’s canal, leading to their dysfunction (36). Exosomes as constitutive components in the aqueous humor have been found to play a vital role in the regulation of the homeostasis of the aqueous drainage system (37). The oriented outflow of the aqueous humor from NPCE to TM results in the basically one-way transmission of signals between the two tissues. Although theoretically exosomes can be secreted by all live cells, the binding of exosomes with receptor cells is relatively specific and exosomes originating from RPE have little effect on TM cells compared to NPCE-derived exosomes (14). The whole uptake process of NPCE-derived exosomes by TM starts with the recognition of specific surface receptors and ligands and involves the Dynamin 2-dependent route of transportation, which primarily depends on the endocytosis route for internalization (38). In the last decade, a number of ex vivo studies have explored the delicate crosstalk between NPCE and TM, which support the positive role of exosomes in maintaining the function of TM, especially under stress conditions (11–13, 15, 16, 39–42).
Intraocular tissues are consistently exposed to oxidative stress and rely on the antioxidant system to clear reactive oxygen species (43). Patients with chronic glaucoma present with dysfunction of mitochondria and deficiency of antioxidants, which results in the elevated production of reactive oxygen species in the aqueous humor and intraocular tissues (44). Higher levels of oxidative stress markers, such as malondialdehyde, are significantly enriched both in the aqueous humor and blood samples collected from glaucoma patients, particularly POAG, compared to healthy control (45). The chronic challenge of oxidative stress is detrimental to TM, which triggers an inflammatory cascade and activation of apoptosis and leads to the dysfunction and blockage of the aqueous drainage pathway (46). In addition, the antioxidant defense mechanisms of TM are compromised as the TM is not naturally exposed to the sunlight like cornea or iris, thus the TM is found to be particularly sensitive to oxidative damage (47). Exosomes produced by NPCE have been found to act as signal transmitters and break the vicious cycle of oxidative damage and TM dysfunction in glaucoma pathogenesis, as emerging evidence supports their role in the protection and modulation of TM metabolism, including improving their endurance under oxidative challenge and the ability to refresh the extracellular matrix (11–15). In the face of oxidative stress, exosomes from NPCE carry high levels of carbonylated proteins, which are end products of oxidized proteins and act as signal markers to evoke the activity of catalase and superoxide dismutase in TM cells (39). TM exposed to exosomes derived from hypoxia-challenged NPCE exhibits a significantly decreased level of oxidative stress due to the induction of major antioxidant genes (11). However, such activities are not observed when exosomes derived from non-stressed NPCE are used, suggesting their role in alerting target cells and improving their resistance under stressed conditions (39, 48).
The chronic stress condition of the TM eventually results in its dysfunction in the modulation of the extracellular matrix and deposition of collagen fibers, leading to partial or complete blockage of the aqueous drainage pathway (49). The canonical Wnt/β-catenin signaling pathway is a key signal cascade downstream of the oxidative damage and is found to play extensive roles in many physiological and pathological processes, including cell proliferation, embryogenesis, inflammation, apoptosis, cell migration, and most relevantly, extracellular matrix remodeling and fibrogenesis (50–52). Activation of the canonical Wnt/β-catenin signaling pathway activates downstream genes involved in extracellular matrix recycling and refreshing and is found to antagonize dexamethasone-induced glucocorticoid receptor signaling in the TM (53). Inhibition of the canonical Wnt/β-catenin signaling pathway and overactivation of its key negative regulator glycogen synthase kinase-3β (GSK-3β) is a signature of glaucoma patients and is associated with elevated IOP (54, 55). In the canonical Wnt/β-catenin signaling pathway, the Wnt ligand binds with its receptor low-density lipoprotein receptor-related protein 5/6 (LPR 5/6) and disrupts the formation of the β-catenin destruction complex, leading to the enrichment of cytoplasmic β-catenin. β-catenin translocates to the nucleus, binds with the T cell factor/lymphoid enhancer factor (TCF/LEF) receptor, and activates related genes. In contrast, GSK-3β as a key component of the β-catenin destruction complex leads to hyperphosphorylation of β-catenin and subsequently activates its proteolysis (Figure 1). Exosomes released from NPCE have been found to elaborately modulate the canonical Wnt/β-catenin signaling pathway and related modulators, which seems to be both dose-dependent and time-dependent. Accumulating data suggests that NPCE-derived exosomes up-regulate the Wnt/β-catenin signaling pathway after their specific internalization by TM cells. A more than 2-fold decrease of the cytosolic fraction of β-catenin and conversely a significant increase of its nuclear fraction are observed in TM cells after exposure to NPCE-derived exosomes in vivo, accompanied by the decreased expression and phosphorylation of its key inhibitor GSK-3β (12, 13). In addition, a bimodal response of TM to NPCE-derived exosomes is revealed, which shows decreased level of β-catenin and its nuclear receptor LEF-1 when exposed to low concentration of exosomes compared to high concentration, suggesting the concentration of exosomes may play a key role in its differential regulation of TM metabolism (14). The modulation effects of exosomes are also found to be much more prominent in NPCE cell lines compared to NPCE primary cells, although they demonstrate a similar tendency to activate the Wnt/β-catenin signaling pathway in TM cells. Exosomes derived from NPCE cell line have a significantly larger volume and carries 5 times more protein content compared to primary cells (56). Thus, for therapeutic considerations, the selection of optimal cells for the collection of exosomes is vital to improve their therapeutic effects.
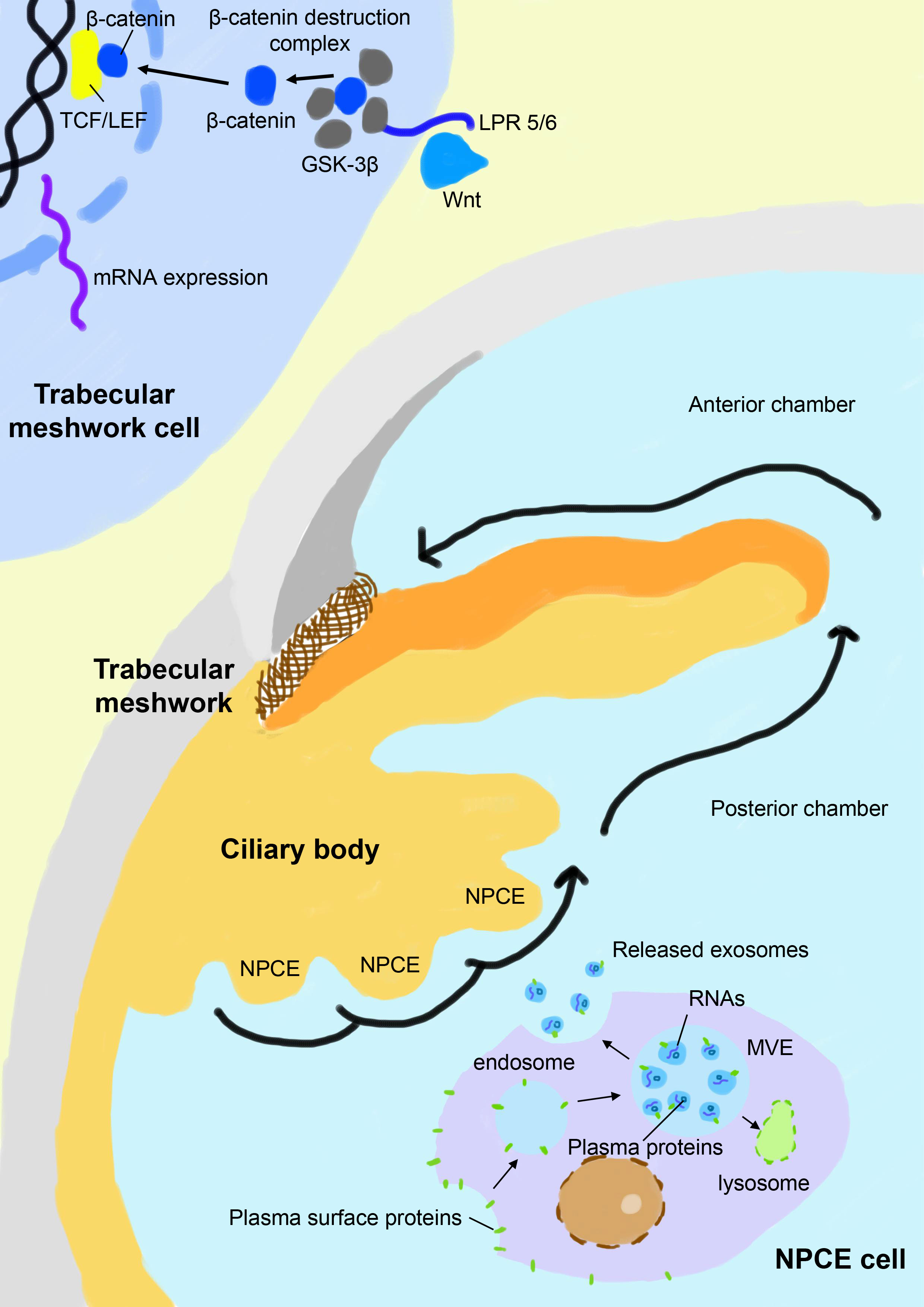
Figure 1 Schematic view of exosome-based crosstalk between NPCE and TM. In the NPCE cells, exosomes are constitutively formed from multivesicular bodies (MVE) after the inward budding of their membranes and are released with the fusion of MV with the plasma membrane. NPCE-derived exosomes specifically act on TM via oriented outflow of the aqueous humor to regulate its activities, particularly the canonical Wnt/β-catenin signaling pathway. Inhibition of the canonical Wnt/β-catenin signaling pathway in the TM is a signature of POAG, which is characterized by a reduced nuclear fraction of β-catenin and overexpression of its key negative regulator GSK-3β. Under the physical state, NPCE-derived exosomes positively regulate the Wnt/β-catenin signaling pathway in TM and promote the refreshing and phagocytosis of its extracellular matrix, which is compromised in patients with POAG. NPCE, non-pigment ciliary epithelium; GSK–3β, glycogen synthase kinase-3β; LPR 5/6, low-density lipoprotein receptor-related protein 5/6; TCF/LEF, T cell factor/lymphoid enhancer factor.
Dysregulation of extracellular matrix deposition is a hallmark of POAG, which increases the outflow resistance of the aqueous humor and leads to elevated IOP (57). Under physiological conditions, exosomes produced from TM and NPCE are found to participate in the opsonization and refreshing of its extracellular matrix, and this process is compromised in patients with POAG. Proteomic analysis reveals that exosomes derived from TM contain abundant proteins associated with extracellular matrix building and remodeling, including fibronectin, collagen, and integrin-binding ligand (15). TM secrete exosomes that assist the digestion of extracellular matrix components, including fibronectin and collagen type I (41, 42). For example, in a glucocorticoid-induced glaucoma model, excessive accumulation of fibronectin in the TM extracellular matrix results from reduced turnover by exosomes that bind significantly reduced amounts of fibronectin per exosome, which is consistent with a dramatic decrease of its binding proteins annexin A2 and A6 on its surface (58). Exosomes collected from non-glaucomatous individuals show a 55% increase in fibronectin-binding capacity compared to glaucoma patients, which can be further induced to improve by 63% after pre-conditioning with mechanical stretch, suggesting its regulatory role in reaction with stress conditions (15). In addition, exosomes secreted from NPCE demonstrate a positive role in the inhibition of TM extracellular matrix fibrosis by reducing the secretion of collagen type I, a key extracellular matrix component, by TM cells (40). Interestingly, TM exosomes from POAG patients are much smaller than healthy control due to the difference in membrane phospholipid content and phospholipid conversion enzymes, indicating decreased capacity to load extracellular matrix recycling proteins (59).
In addition to conditionally-expressed protein cargos, miRNA as another key component and regulatory factor of exosomes have been revealed to alter the gene expression of the TM and Schlemm’s canal, playing a significant role in glaucoma pathogenesis (16, 17). miRNAs are a group of non-coding RNAs that suppress the expression of their complementary mRNAs by cleavage, destabilization, or inhibition of their translation (60). As a core regulator in the post-translational process, a single miRNA may play multiple roles by interacting with numerous target mRNAs, and vice versa, which greatly diversifies the complicated regulatory network (61). As roughly estimated, more than 2,600 miRNAs have been identified in human since its first discovery in the 1990s (62, 63). Altered miRNA expression profile has been found to act as a signature of glaucoma in both experimental glaucoma models and patients (64–66). A panel of 20 circulating miRNAs have been identified to differentially express in glaucoma patients compared to healthy control, suggesting their role as potential biomarkers and pathogenic modifiers (67). Molecular analysis of exosomal cargos elucidates 584 micro RNAs (miRNAs) and 182 proteins associated with TM metabolism regulation (16). Accumulating evidence indicates that miRNAs as key cargos of exosomes regulate the response of TM to oxidative stress and alters the deposition of the extracellular matrix (16, 17). For example, tumor growth factor β2 (TGF-β2) is a key modulator of extracellular matrix remodeling and has been found to play significant roles in glaucoma pathogenesis (68, 69). TGF-β2-stimulated TM cells secreted exosomes that contain more than 2 folds the level of miR-7515 that promotes the expression of VEGFA, VEGFR2, PECAM1, and Tie2 in Schlemm’s canal endothelial cells (17). After culturing with TGF-β2 for 24 hours, human TM cells produced exosomes that showed an upregulation of 23 miRNAs and downregulation of 3 miRNAs (70). Among these miRNAs identified, miR-29b as a major downstream effector of TGF-β2 has been most extensively studied, which is a suppressor of many extracellular matrix proteins of the TM (71, 72). By inhibiting the binding of nuclear factor-like 2 (Nrf2) to the promoter region of miR-29b, TGF-β2 suppresses its expression and promotes tissue fibrosis (73). In addition to its association with the TGF-β2 pathway, miR-29b is also a key modulator of the canonical Wnt/β-catenin signaling pathway, another important pathway involved in extracellular matrix remodeling as mentioned in the previous text (74). Exosomes produced from NPCEs carry abundant levels of miR-29b, and significantly suppress the expression of collagen 3A1 (COL3A1) in the TM cells via the Wnt/β-catenin signaling pathway (16). Thus, collective evidence suggests that exosomes produced by NPCEs may employ miRNAs as intracellular messengers to communicate with target cells and result in cell reprogramming.
Taken together, exosomes are constitutively released from NPCE and TM and serve a physical role in maintaining their homeostasis in oxidative stress response, apoptosis, and extracellular matrix remodeling. In patients with glaucoma, elevated oxidative stress and compromised function of exosomes form a vicious cycle that leads to increased resistance of aqueous humor outflow and elevation of IOP. Currently, researchers are aiming to stimulate and reacquire the normal function of TM with the help of external exosomes collected from mesenchymal stem cells, which is discussed in detail in part 4.
3 Role of microglia-derived exosomes in the spreading and modulation of glaucomatous neuroinflammation
For many years, RGC apoptosis by elevated intraocular pressure has been suggested to be the crucial etiology for irreversible visual impairment in glaucoma patients (75). However, in the recent decade, there is a paradigm shift that neuroinflammation induced primarily by microglia and T cells participates in the entire process of glaucomatous chronic neurodegeneration, which results in persistent and progressive damage to RGCs (9, 10). Microglia as constitutive housekeepers of neural tissues in the eye and the central nervous system are broadly distributed and serve many important physical functions in their quiescent form in maintaining the homeostasis of the neural tissue, including immune surveillance, control of neuronal excitability, organization of synapses, phagocytosis of cell debris, and secretion of trophic factors (76–78). When activated, microglia are highly heterogeneous and basically assume 2 different states in reaction to different physical conditions and stimuli, including the neurotoxic M1 microglia and neurotrophic M2 microglia (79, 80). Animal models of glaucoma reveal that microglia act as the earliest sensor and effector in glaucoma pathogenesis, which develop ahead of evident damage of RGCs (81–83). A pathogenic shift of microglial subtypes from M2-dominant to M1-dominant composition stimulated by pro-inflammatory markers such as interferon-γ (IFN-γ) is a hallmark of glaucoma neuroinflammation and is responsible for the synchronous activation of other immune effector cells, including cytotoxic T cells, plasma cells, and macrophages (see our previous reviews) (9, 10). Depletion of microglial activity by blockage of microglial adenosine A2A receptor generates neuroprotective effects by suppressing the spread of neuroinflammation and subsequent RGC death in a rat glaucoma model (84). Collective evidence points to the central role of retinal microglia in glaucoma neurodegeneration.
Traditionally, microglia are supposed to spread neuroinflammation by physically wandering across the blood-retinal barrier (BRB) and secretion of corresponding cytokines and chemokines (tumor necrosis factor-α, interleukin-1β, superoxides, and reactive oxygen species for M1 microglia and interleukin-4, interleukin-10, interleukin-13, and tumor growth factor β for M2 microglia, respectively) (85, 86). Recently, researchers find microglia also employ exosomes to spread inflammatory signals in a paracrine and endocrine manner and serve as a key pathological component in many neurodegenerative diseases, including glaucoma, Alzheimer’s disease, and Parkinson disease (87–90). In the retina, the crosstalk between microglia, RGCs, and RPE is highly complicated yet elaborately organized due to the specificity of exosomes in signal transduction. Exosomes carry miRNAs and exchange them between origin and receptor cells to regulate their physical activities (91, 92). Studies find that microglia can amplify inflammation by selectively exchanging exosomes with different cell types, including microglia, RGC, RPE, retinal vascular endothelium, pericytes, oligodendrocytes, and astrocytes (25–27). In a rat retinal degeneration model, neural exosomes released from neural stem/progenitor cells that are injected into the subretinal space are found to be taken up primarily by microglial cells and result in immune modulation and protection of the retina (93). RPE is able to propagate signals of oxidative stress by sending exosomes from its apical site that contains damaged mitochondrial DNA, which are internalized by retinal microglia and induce a proinflammatory phenotype by activation of its cytoplasmic receptor Z-DNA-binding protein 1 (94). Conversely, microglia are able to regulate the production of exosomes in different states and modulate the immune background. Activated proinflammatory microglia not only multiply the production of exosomes but also load proinflammatory or neurotoxic molecules (95). Consistent with the subgroups of microglia, exosomes secreted by M1 and M2 microglia also contain distinct state-associated proteins and miRNAs that may participate in intercellular communication and regulation of recipient cells (96). For example, exosomes derived from M2 microglia exhibit neuroprotective ability on mice brain ischemic stroke model via exosomal miR-124 and its downstream target ubiquitin-specific protease 14 (97). On the other hand, M1 microglia-derived exosomes contain a distinct group of miRNAs, with the pro-inflammatory miR-146a-5p as their dominant miRNA component (98). As suggested by the current evidence, microglia seem to serve as an exchanging hub of exosomes with other cell types, and this bidirectional communication via exosomes leads to the change of their phenotypes and the spreading of neuroinflammation (Figure 2).
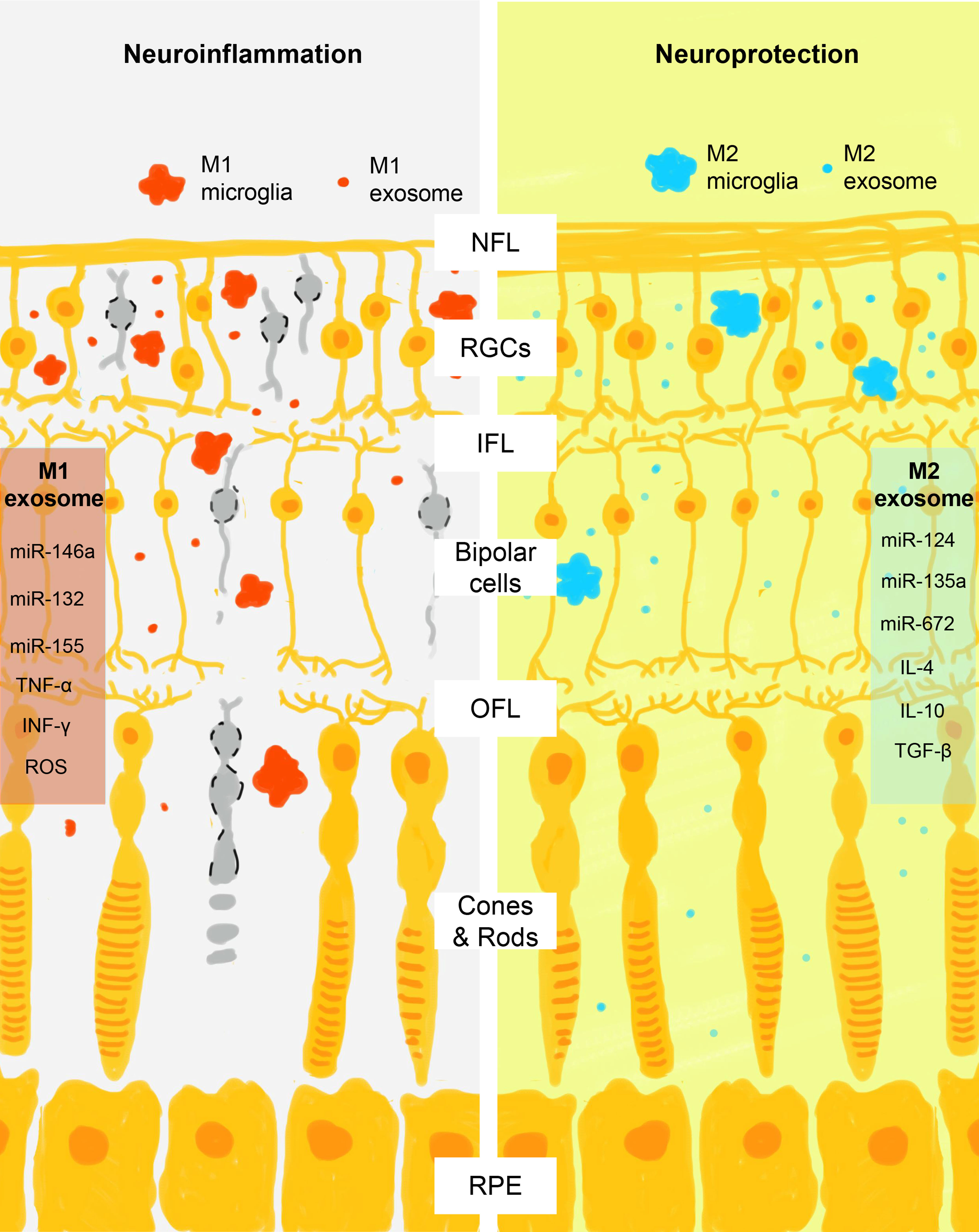
Figure 2 Schematic view of M1 and M2 microglia exosomes in the regulation of retinal neuroinflammation. After activation, microglia assume M1 or M2 phenotypes that act differently in the promotion and control of retinal neuroinflammation. Consistent with the action of M1 and M2 cells, their exosomes also contain phase-specific molecules, particularly miRNAs that regulate the activity of retinal neural cells through the complex crosstalk. M1 exosomes contain proinflammatory signals (miR-146a, miR-132, miR-155, tumor necrosis factor α, interferon γ, and reactive oxygen species) and M2 exosomes contain neuroprotective signals (miR-124, miR-135a, miR-672, interleukin 4, interleukin 10, and tumor growth factor β). NFL, nerve fiber layer; RGCs, retinal ganglial cells; IPL, inner plexiform layer; OPL, outer plexiform layer; RPE, retinal pigment epithelium.
In glaucoma pathogenesis, accumulating evidence indicates that the activation of microglia is the prelude of neuroinflammation that rapidly respond to ocular hypertension challenge and assume a proinflammatory M1 phenotype within 24 hours (99–101). Emerging evidence suggests that microglia-derived exosomes may participate in the rapid spreading of inflammatory signals to other microglia and result in RGC degeneration (18–20). Microglia cells exposed to elevated hydrostatic pressure produced double amounts of exosomes that activate naïve microglia in vivo and improve their proliferation, phagocytic capacity, and mobility (18, 19). In addition, injection of such exosomes into healthy mice vitreous bodies induces propagation of intraocular inflammation and widespread RGC death (20). Compared with exosomes generated from naïve microglia, exosomes from activated microglia induced by elevated hydrostatic pressure contain increased levels of major histocompatibility complex II (MHC II), inducible NO synthase (iNOS), TNF, and IL-1β. Interestingly, such effects are generally abolished when GW4869, a neutral sphingomyelinase inhibitor that impairs the formation of exosomes, is added into the system, reinforcing the crucial role of exosomes in the spreading of neuroinflammation (20).
In addition to the pro-inflammatory effects of M1 microglia, M2 microglia may generate neuroprotective effects via their exosomes and are beneficial for immune modulation and protection of retinal cells. In a mice model of oxygen-induced retinopathy, microglia-derived exosomes injected into the vitreous body are selectively incorporated into photoreceptors and inhibit their apoptosis. These exosomes are found to contain high levels of miR-124-3p that inhibit hypoxia-induced activation of inositol-requiring enzyme 1α (IRE1α)-X-box binding protein 1 (XBP1) cascade in recipient cells (102). In addition, in the central nervous system, the protective effect of M2 microglia-derived exosomes has been extensively demonstrated in many different animal models, including Alzheimer’s disease (103), ischemic-reperfusion injury (97, 104), oxygen-glucose deprivation (92), and ischemic brain injury (105, 106). The neuroprotective effects are found to be basically induced by modulatory miRNAs carried in exosomes, such as miR-124, miR-137, miR-23a-5p, and miR-672 that target different singling pathways, including the Notch1, Olig3, and AIM2/ASC/caspase-1 pathways (97, 107–109). The activity of M2 microglia and their exosomes are vital to limit the intensity of neuroinflammation induced by M1 microglia and prevent extensive damage to the retina. However, in patients with glaucoma, chronic insult of elevated intraocular pressure, oxidative stress, excitotoxicity, and mitochondria dysfunction result in the imbalance of proinflammatory and anti-inflammatory microglia and their exosomes (110, 111). A shift of immune cells towards proinflammatory phenotypes is evidenced by autopsy studies (112–114) and peripheral blood samples collected from glaucoma patients (115, 116). As a result, researchers have proposed immunomodulatory therapy by applying regulatory exosomes, which are commonly collected from M2 microglia and stem cells, to shape microglia phenotypes (see part 4) (117).
4 Primary explorations of exosome-based therapy for glaucoma
Exosomes are crucial signal carriers that shape the state of recipient cells, and the imbalance of the production and malfunction of exosomes have been revealed to contribute to glaucoma pathogenesis both in the aqueous drainage system and the retina (16–20). In recent years, researchers have employed exosomes primarily originating from mesenchymal stem cells (MSCs) and induced pluripotent stem cells (iPSCs) to modulate the function of immune cells and achieve tissue regeneration in different animal models of glaucoma.
MSC-derived exosomes are currently the most extensively studied therapeutic exosomes in many medical fields, which have been promoted into clinical trials in patients with inflammatory bowel disease (118), chronic kidney disease (119), cerebral artery infarction (120), and demyelinating diseases (121). In the ocular system, exosomes as biological nano-sized particles have been primarily explored in several inflammatory and degenerative ocular disorders, including glaucoma, age-related macular degeneration, and anterior ischemic optic neuropathy (122–124). Compared with cell-based therapy, exosome-based therapy shows superiorities of great tissue compatibility, better penetration capacity, better immune tolerance, and the freedom to be modified with specific cargos to regulate target cells (125, 126). As with physical exosomes released by cells in the ocular system, external exosomes originating from MSCs are well recognized and free to distribute across the retina and taken up by microglia, RGCs, and retinal neural cells, resulting in the regulation of their activities (127). Exosomes show good retinal tropism after intravitreal injection and expand to diffused areas (128). When injected into the mice’s vitreous cavity, exosomes can efficiently reach the inner nuclear layer and outer plexiform layer, and to a lesser extent the outer nuclear layer as well (129). The rapid internalization of exosomes by the retina and microglia also attenuates their clearance and extends their effect time. Exosomes injected into the rat’s vitreous body remain detectable for as long as 4 weeks, with saturated binding to vitreous humor components (127). In addition, although MSCs are generally immune suppressive and show good tolerability, the immune-privileged intraocular tissues are highly sensitive, especially in diseased conditions, and the risk of inflammatory adverse events after MSC injection can’t be fully eliminated. As an example, intravitreal injection of 2 × 104 bone marrow-derived MSCs and human adipose-derived stem cells into rat vitreous body elicits retinal glial activation, pericytes apoptosis, regression of retinal vessels, and formation of cataract with elevated levels of interleukin-1β, complex 3, arginase 1, and heat shock protein 90 (130). In a clinical trial, patients with retinitis pigmentosa receiving a single intravitreal bone marrow-derived MSCs showed mild adverse events, including mild macular edema, localized choroidal detachment, and the development of pre-retinal fibrous membrane (131). In comparison, injection of exosomes into the vitreous cavity is generally safe and no adverse events have been reported in animal studies so far.
As a cutting-edge research focus for glaucoma, exosome-based therapy demonstrates potential benefits in 3 basic aspects: modulation of neuroinflammation, protection of RGCs and retinal neurons, and promotion of tissue regeneration (28–35). However, currently, all evidence comes from ex vivo studies and animal models, with no clinical data available (summarized in Table 1). MSC-derived exosomes basically exert therapeutic effects via miRNA-dependent mechanisms, which is consistent with physical exosomes produced by intraocular tissues as discussed in the previous parts of the review. Consequently, the knockdown of Argonaute-2, a key miRNA effector molecule, diminishes the beneficial effect of intravitreal injection of exosomes produced by marrow-derived MSCs in a rat optic nerve crush model (33).The neuroprotective effect is also absent when fibroblast-derived exosomes are used, reinforcing the importance of cargo carried by exosomes (30). Interestingly, when original cells are primed in advance with appropriate conditions, the therapeutic effects of exosomes can be enhanced. For instance, in a mice chronic glaucoma model, the monthly injection of exosomes collected from tumor necrosis factor α-primed bone marrow-derived MSCs shows better efficacy of RGC protection compared with naïve exosomes (30). In glaucomatous rats, injection of exosomes from hypoxic MSCs but not normoxic MSCs exhibits beneficial effects by recruiting pro-regenerative macrophages into the TM and activation of ocular progenitor cells (28). The mechanisms of priming the original cells are still poorly understood, but researchers need to keep in mind the application of appropriate stimuli during cell culture to achieve better therapeutic outcomes. In addition, exosomes are excellent natural carriers of small and large molecules that show better penetration and distribution property compared to artificial nanocarriers (132). Modification of exosomes by changing their surface ligands, cargos, and insertion of specific genetic materials has become readily feasible and has been explored in different medical fields. For example, to enhance the activation of canonical Wnt/β-catenin signaling pathway in TM cells, NPCE-derived exosomes are genetically modified and loaded with SMAD7 small-interfering RNA (siRNA), which achieves a 53% knockdown of SMAD7 and elevation of β-catenin and tumor growth factor β2 in TM cells after incubation in vivo (29). By conjugating Arg-Gly-Asp, a ligand for integrins that are highly expressed in choroidal neovascularization (CNV) tissues, exosomes derived from Müller glial cells or retina are able to selectively accumulate in areas of CNV after intravitreal injection and promote therapeutic effect (133). In considerations of glaucoma therapy, such surface modifications of MSC-derived exosomes may show potential benefits by enhancing their selectivity to target cells, such as microglia and RGCs, which need to be further explored and verified.
In the field of ophthalmology, exosome-based therapy is just in its infancy phase of development. To promote the clinical translation of exosome-based therapy, great efforts have been made to lift the hurdles of manufacturing exosomes that comply with good manufacturing practices (GMP) (134). Major challenges come from the upstream standardized development, validation, and culture of cell lines and the downstream purification, collection, storage, and quality control of exosomes (135). Currently, only a few contract manufacturing organizations worldwide are available to provide clinical-grade exosomes under GMD regulation and no global consensus on the validation, production, and evaluation of exosome-based therapy is available, which limits the use of exosomes in clinical trials (136, 137). From the view of ophthalmologists, exosomes used for intraocular injection should adhere to a stricter standard due to the immune-privileged state of the eye in its physical state, despite their good safety profile demonstrated in previous animal models (138–140). Another important consideration for exosome-based therapy is its relatively short duration of action due to the clearance of exosomes and degradation of their cargos. This becomes a particular problem in glaucoma patients as glaucoma is a chronic and progressive disease and patients rely on long-term therapy to control IOP and protect their retina. In animal models, exosomes basically have to be injected into the vitreous body monthly or even weekly to achieve durable effects (30, 33, 35). However, the exact pharmacokinetics and efficacy of exosomes after intravitreal injection is poorly understood, especially for larger animal eyes such as nonhuman primates. Thus, the appropriate time gap between injections in patients is still unknown based on the current evidence. In addition, besides remarkable structural differences between animal eyes and human eyes, another gap between clinical translation and current animal models is the chronic pathogenic mechanisms of glaucoma that can’t be fully mimicked in acute phase animal models, such as optic nerve crush, acute elevation of IOP, and retinal ischemic injury models (141, 142). Whether frequent intravitreal injection of exosome-based therapy provides additional benefits over the conventional non-invasive application of eye drops and anti-glaucoma surgeries needs to be further evaluated. Moreover, a practice regimen that achieves RGC protection with lower injection frequency, potentially by combining the sustained release delivery system or using other routes of administration (suprachoroidal injection or subretinal injection) should be another focus of future research to improve patients’ compliance (143, 144).
5 Summary and conclusions
In this review, we thoroughly summarize recent research findings of exosomes in glaucoma pathogenesis and treatment. As constitutive nano-sized biological particles in the eye, exosomes participate in maintaining the homeostasis of TM and retina through complicated crosstalk between origin and recipient cells. The relatively free movement of exosomes within intraocular aqueous compartments and across BRB boundaries confers their capacity to rapidly spread signals in a paracrine and endocrine manner and shape the immune background of glaucomatous chronic neuroinflammation. As a potential therapy for glaucoma, exosomes originating from MSCs have been explored in multiple animal models and demonstrate neuroprotective effects. However, there are still obstacles between these emerging findings of exosomes in animal studies and clinical application, including knowledge gaps in pharmacokinetics, long-term efficacy, safety, and clinical-grade manufacturing. However, with boosting applications of exosomes in clinical trials in several other fields, there is great potential that exosome-based therapy may become readily available for clinical translation in the near future.
Author contributions
Conception and design, LW and XW. Manuscript preparation, LW. Critical revisions, XW. All authors contributed to the article and approved the submitted version.
Funding
This work was supported by grants from the Natural Science Foundation of China (No.82070954), the key project of the Science and Technology Department in Sichuan province (2023YFS0309), the Innovative Spark Grant of Sichuan University (No.2018SCUH0062), 1·3·5 Project for Disciplines of Excellence–Clinical Research Incubation Project, West China Hospital, Sichuan University (No.2021HXFH057), the Science & Technology Department of Sichuan Province (China) Funding Project (No.2021YFS0221), and the Postdoctoral Research Funding of West China Hospital, Sichuan University, China (No.2020HXBH044).
Conflict of interest
The authors declare that the research was conducted in the absence of any commercial or financial relationships that could be construed as a potential conflict of interest.
Publisher’s note
All claims expressed in this article are solely those of the authors and do not necessarily represent those of their affiliated organizations, or those of the publisher, the editors and the reviewers. Any product that may be evaluated in this article, or claim that may be made by its manufacturer, is not guaranteed or endorsed by the publisher.
References
1. Obara EA, Hannibal J, Heegaard S, Fahrenkrug J. Loss of melanopsin-expressing retinal ganglion cells in severely staged glaucoma patients. Invest Ophthalmol Vis Sci (2016) 57(11):4661–7. doi: 10.1167/iovs.16-19997
2. Guo Y, Huang S, Xu S, Zhong Y. Choroidal microvasculature dropout in glaucoma. Semin Ophthalmol (2023). doi: 10.1080/08820538.2023.2187258
3. Ozkan D, Altan C, Er MO, Gultekin F, Kuraş S, Artunay O. The role of oxidative status in the pathogenesis of primary open-angle glaucoma, pseudoexfolyation syndrome and glaucoma. Eur J Ophthalmol (2023) 33(1):352–60. doi: 10.1177/11206721221113199
4. Duarte JN. Neuroinflammatory mechanisms of mitochondrial dysfunction and neurodegeneration in glaucoma. J Ophthalmol (2021) 2021. doi: 10.1155/2021/4581909
5. Tharmathurai S, Huwaina AS, Azhany Y, Razak AA, Che-Hamzah J, Fazilawati Q, et al. Quality of life of older adults with primary open angle glaucoma using bahasa Malaysia version of glaucoma quality of life 36 questionnaire. Curr Aging Science. (2022) 15(2):147–62. doi: 10.2174/1874609814666210903155251
6. Baudouin C, Kolko M, Melik-Parsadaniantz S, Messmer EM. Inflammation in glaucoma: from the back to the front of the eye, and beyond. Prog Retinal Eye Res (2021) 83. doi: 10.1016/j.preteyeres.2020.100916
7. Hua ZQ, Liu H, Wang N, Jin ZB. Towards stem cell-based neuronal regeneration for glaucoma. Prog Brain Res (2020) 257:99–118. doi: 10.1016/bs.pbr.2020.05.026
8. Mallick S, Sharma M, Kumar A, Du Y. Cell-based therapies for trabecular meshwork regeneration to treat glaucoma. Biomolecules (2021) 11(9). doi: 10.3390/biom11091258
9. Wang L, Wei X. T Cell-mediated autoimmunity in glaucoma neurodegeneration. Front Immunol (2021) 12:803485. doi: 10.3389/fimmu.2021.803485
10. Wei X, Cho KS, Thee EF, Jager MJ, Chen DF. Neuroinflammation and microglia in glaucoma: time for a paradigm shift. J Neurosci Res (2019) 97(1):70–6. doi: 10.1002/jnr.24256
11. Lerner N, Chen I, Schreiber-Avissar S, Beit-Yannai E. Extracellular vesicles mediate anti-oxidative response–in vitro study in the ocular drainage system. Int J Mol Sci (2020) 21(17):1–17. doi: 10.3390/ijms21176105
12. Lerner N, Avissar S, Beit-Yannai E. Extracellular vesicles mediate signaling between the aqueous humor producing and draining cells in the ocular system. PloS One (2017) 12(2). doi: 10.1371/journal.pone.0171153
13. Lerner N. Trabecular meshwork wnt signaling pathway is altered by non-pigmented ciliary epithelium derived exosomes. Invest Ophthalmol Visual Science. (2016) 57(12):3014.
14. Beit-Yannai E, Schreiber-Avissar S, Lener N. How much exosomes will mimic physiological response in in vitro experiment? learning from extracellular vesicles mediate signaling in ocular system. J Extracellular Vesicles. (2017) 6:201–2. doi: 10.1080/20013078.2017.1310414
15. McDonnell FS, Riddick BJ, Roberts H, Skiba NP, Stamer DW. Trabecular meshwork exosomes and the extracellular matrix. Invest Ophthalmol Visual Science. (2022) 63(7):445.
16. Lerner N, Schreiber-Avissar S, Beit-Yannai E. Extracellular vesicle-mediated crosstalk between NPCE cells and TM cells result in modulation of wnt signalling pathway and ECM remodelling. J Cell Mol Med (2020) 24(8):4646–58. doi: 10.1111/jcmm.15129
17. Takahashi E, Saruwatari J, Fujimoto T, Tanoue Y, Fukuda T, Inoue T. The effects of exosomes derived from trabecular meshwork cells on schlemm's canal endothelial cells. Sci Rep (2021) 11(1):21942. doi: 10.1038/s41598-021-01450-9
18. Aires ID, Ribeiro-Rodrigues T, Boia R, Catarino S, Almeida D, Girão H, et al. Exosomes derived from microglia exposed to elevated pressure code for an inflammatory response: implications for retinal degeneration. GLIA (2019) 67:E89–90. doi: 10.1002/glia.23674
19. Santiago AR, Aires ID, Ribeiro-Rodrigues T, Boia R, Catarino S, Almeida D, et al. Extracellular vesicles released by microglia exposed to elevated hydrostatic pressure promote retinal neural cell loss and microglia reactivity. Invest Ophthalmol Visual Science. (2019) 60(9):2705–34.
20. Aires ID, Ribeiro-Rodrigues T, Boia R, Catarino S, Girão H, Ambrósio AF, et al. Exosomes derived from microglia exposed to elevated pressure amplify the neuroinflammatory response in retinal cells. GLIA (2020) 68(12):2705–24. doi: 10.1002/glia.23880
21. Staals RH, Pruijn GJ. The human exosome and disease. Adv Exp Med Biol (2011) 702:132–42. doi: 10.1007/978-1-4419-7841-7_11
22. Deng H, Sun C, Sun Y, Li H, Yang L, Wu D, et al. Lipid, protein, and MicroRNA composition within mesenchymal stem cell-derived exosomes. Cell Reprogram (2018) 20(3):178–86. doi: 10.1089/cell.2017.0047
23. Guidolin D, Tortorella C, Marcoli M, Cervetto C, Maura G, Agnati LF. Receptor-receptor interactions and microvesicle exchange as mechanisms modulating signaling between neurons and astrocytes. Neuropharmacology (2023) 231. doi: 10.1016/j.neuropharm.2023.109509
24. Figueroa A, Congrove NR, Sillik SA, Sadideen DT, Falk T, Rickman CB, et al. Exosome uptake is selective but not species or tissue-specific. Invest Ophthalmol Visual Science. (2018) 59(9).
25. Paolicelli RC, Bergamini G, Rajendran L. Cell-to-cell communication by extracellular vesicles: focus on microglia. Neuroscience (2019) 405:148–57. doi: 10.1016/j.neuroscience.2018.04.003
26. Beltramo E, Mazzeo A, Porta M. Extracellular vesicles derived from M1-activated microglia induce pro-retinopathic functional changes in microvascular cells. Eur J Ophthalmol (2022) 32. doi: 10.1177/11206721221092575
27. Zhang Y, Lian L, Fu R, Liu J, Shan X, Jin Y, et al. Microglia: the hub of intercellular communication in ischemic stroke. Front Cell Neurosci (2022) 16:889442. doi: 10.3389/fncel.2022.889442
28. Tebid CT, Lesk M, Dave V, Roy D. Exosomes/Evs: mesenchymal stromal cells secretome-induced trabecular meshwork regeneration for glaucoma therapy. Cytotherapy (2022) 24(5):S34. doi: 10.1016/S1465-3249(22)00140-2
29. Tabak S, Feinshtein V, Schreiber-Avissar S, Beit-Yannai E. Non-pigmented ciliary epithelium-derived extracellular vesicles loaded with smad7 sirna attenuate wnt signaling in trabecular meshwork cells in vitro. Pharmaceuticals (2021) 14(9). doi: 10.3390/ph14090858
30. Mead B, Zack DJ, Ahmed Z, Tomarev SI. Neuroprotection of retinal ganglion cells by BMSC-derived exosomes. Invest Ophthalmol Visual Science. (2019) 60(9).
31. Yu Z, Zhuo Y. TNF-α stimulation enhances the neuroprotective effects of gingival MSCs derived exosomes in retinal ischemia-reperfusion injury via the MEG3/miR-21a-5p axis. Invest Ophthalmol Visual Sci (2022) 63(7):2975–F0216. doi: 10.1016/j.biomaterials.2022.121484
32. Yu Z, Wen Y, Jiang N, Li Z, Guan J, Zhang Y, et al. TNF-α stimulation enhances the neuroprotective effects of gingival MSCs derived exosomes in retinal ischemia-reperfusion injury via the MEG3/miR-21a-5p axis. Biomaterials (2022) 284. doi: 10.1016/j.biomaterials.2022.121484
33. Mead B, Tomarev S. Bone marrow-derived mesenchymal stem cells-derived exosomes promote survival of retinal ganglion cells through mirna-dependent mechanisms. Stem Cells Trans Med (2017) 6(4):1273–85. doi: 10.1002/sctm.16-0428
34. Moisseiev E, Anderson JD, Oltjen S, Goswami M, Zawadzki RJ, Nolta JA, et al. Protective effect of intravitreal administration of exosomes derived from mesenchymal stem cells on retinal ischemia. Curr Eye Res (2017) 42(10):1358–67. doi: 10.1080/02713683.2017.1319491
35. Pan D, Chang X, Xu M, Zhang M, Zhang S, Wang Y, et al. UMSC-derived exosomes promote retinal ganglion cells survival in a rat model of optic nerve crush. J Chem Neuroanatomy. (2019) 96:134–9. doi: 10.1016/j.jchemneu.2019.01.006
36. Keller KE, Peters DM. Pathogenesis of glaucoma: extracellular matrix dysfunction in the trabecular meshwork-a review. Clin Exp Ophthalmol (2022) 50(2):163–82. doi: 10.1111/ceo.14027
37. Dismuke WM, Challa P, Navarro I, Stamer WD, Liu Y. Human aqueous humor exosomes. Exp eye Res (2015) 132:73–7. doi: 10.1016/j.exer.2015.01.019
38. Tabak S, Hadad U, Schreiber-Avissar S, Beit-Yannai E. Non-pigmented ciliary epithelium derived extracellular vesicles uptake mechanism by the trabecular meshwork. FASEB J (2021) 35(2). doi: 10.1096/fj.202002040R
39. Beit-Yannai E, Lerner N, Schreiber-Avissar S. Exosomes mediate anti-oxidative response, in-vitro study in ocular drainage system cells. Invest Ophthalmol Visual Science. (2020) 61(7).
40. Tabak S, Schreiber-Avissar S, Beit-Yannai E. Trabecular meshwork's collagen network formation is inhibited by non-pigmented ciliary epithelium-derived extracellular vesicles. J Cell Mol Med (2021) 25(7):3339–47. doi: 10.1111/jcmm.16408
41. Michael Dismuke W, Daniel Stamer W. Trabecular meshwork exosomes: fibronectin binding and ECM homeostasis. Invest Ophthalmol Visual Science. (2015) 56(7):4852.
42. Dismuke WM, Stamer WD. Trabecular meshwork exosomes enhance cellular collagen uptake. Invest Ophthalmol Visual Science. (2014) 55(13):3025.
43. Sun R, Zhang S, Liang Y. Oxidative injury on trabecular meshwork. Chin J Exp Ophthalmol (2016) 34(4):375–9. doi: 10.3760/cma.j.issn.2095-0160.2016.04.019
44. Chrysostomou V, Rezania F, Trounce IA, Crowston JG. Oxidative stress and mitochondrial dysfunction in glaucoma. Curr Opin Pharmacol (2013) 13(1):12–5. doi: 10.1016/j.coph.2012.09.008
45. Benoist d'Azy C, Pereira B, Chiambaretta F, Dutheil F. Oxidative and anti-oxidative stress markers in chronic glaucoma: a systematic review and meta-analysis. PloS One (2016) 11(12). doi: 10.1371/journal.pone.0166915
46. Saccà SC, Pulliero A, Izzotti A. The dysfunction of the trabecular meshwork during glaucoma course. J Cell Physiol (2015) 230(3):510–25. doi: 10.1002/jcp.24826
47. Izzotti A, Saccà SC, Longobardi M, Cartigl C. Sensitivity of ocular anterior chamber tissues to oxidative damage and its relevance to the pathogenesis of glaucoma. Invest Ophthalmol Visual Science. (2009) 50(11):5251–8. doi: 10.1167/iovs.09-3871
48. Lerner N, Schreiber-Avissar S, Beit-Yannai E. Oxidative stress alert by extracellular vesicles, in vitro study in ocular drainage system. J Extracellular Vesicles. (2019) 8:176–7. doi: 10.1080/20013078.2019.1593587
49. Tabak S, Schreiber-Avissar S, Beit-Yannai E. Crosstalk between microrna and oxidative stress in primary open-angle glaucoma. Int J Mol Sci (2021) 22(5):1–17. doi: 10.3390/ijms22052421
50. Shanbagh S, Kumar RS, Tejwani S, Machiraju P, Shetty R, Ghosh A. Altered expression of aquaporins in glaucomatous trabecular meshwork correlates to fibrotic genes and is regulated by WNT signalling. Invest Ophthalmol Visual Science. (2018) 59(9).
51. Eisenmann DM. Wnt signaling. WormBook Online Rev C elegans Biol (2005) 1-17. doi: 10.1895/wormbook.1.7.1
52. Mao W, Sugali CK, Rayana NP, Dai J. β-catenin/canonical wnt signaling negatively regulates glucocorticoid receptor signaling in the trabecular meshwork. Invest Ophthalmol Visual Science. (2020) 61(7).
53. Sugali CK, Rayana NP, Dai J, Peng M, Harris SL, Webber HC, et al. Activation of canonical wnt signaling pathway inhibits dexamethasone-induced glucocorticoid receptor signaling in the trabecular meshwork. Invest Ophthalmol Visual Science. (2021) 62(8).
54. Wang WH, McNatt LG, Pang IH, Millar JC, Hellberg PE, Hellberg MH, et al. Increased expression of the WNT antagonist sFRP-1 in glaucoma elevates intraocular pressure. J Clin Invest (2008) 118(3):1056–64. doi: 10.1172/JCI33871
55. Morgan JT, Raghunathan VK, Chang YR, Murphy CJ, Russell P. Wnt inhibition induces persistent increases in intrinsic stiffness of human trabecular meshwork cells. Exp eye Res (2015) 132:174–8. doi: 10.1016/j.exer.2015.01.025
56. Beit-Yannai E, Schreiber-Avissar S, Lerner N. Exosomes derived from human primary nonpigmented ciliary epithelium have the same tendency as cell line derived exosomes, but different potency to attenuate trabecular meshwork canonical WNT signaling pathway. Invest Ophthalmol Visual Science. (2018) 59(9).
57. Vranka JA, Kelley MJ, Acott TS, Keller KE. Extracellular matrix in the trabecular meshwork: intraocular pressure regulation and dysregulation in glaucoma. Exp eye Res (2015) 133:112–25. doi: 10.1016/j.exer.2014.07.014
58. Dismuke WM, Klingeborn M, Stamer WD. Mechanism of fibronectin binding to human trabecular meshwork exosomes and its modulation by dexamethasone. PloS One (2016) 11(10). doi: 10.1371/journal.pone.0165326
59. Bhattacharya SK, Edwards G, Ziebarth N, Liu Y, Lee RK. Phospholipid composition and their interconversion enzymes differences in glaucomatous human trabecular meshwork compared to controls. Invest Ophthalmol Visual Science. (2021) 62(8).
60. Filipowicz W, Bhattacharyya SN, Sonenberg N. Mechanisms of post-transcriptional regulation by microRNAs: are the answers in sight? Nat Rev Genet (2008) 9(2):102–14. doi: 10.1038/nrg2290
61. Lim LP, Lau NC, Garrett-Engele P, Grimson A, Schelter JM, Castle J, et al. Microarray analysis shows that some microRNAs downregulate large numbers of target mRNAs. Nature (2005) 433(7027):769–73. doi: 10.1038/nature03315
62. Hammond SM. An overview of microRNAs. Adv Drug Delivery Rev (2015) 87:3–14. doi: 10.1016/j.addr.2015.05.001
63. Lee RC, Feinbaum RL, Ambros V. The c. elegans heterochronic gene lin-4 encodes small RNAs with antisense complementarity to lin-14. Cell (1993) 75(5):843–54. doi: 10.1016/0092-8674(93)90529-y
64. Kosior-Jarecka E, Czop M, Gasińska K, Wróbel-Dudzińska D, Zalewski DP, Bogucka-Kocka A, et al. MicroRNAs in the aqueous humor of patients with different types of glaucoma. Graefes Arch Clin Exp Ophthalmol (2021) 259(8):2337–49. doi: 10.1007/s00417-021-05214-z
65. Luna C, Li G, Huang J, Qiu J, Wu J, Yuan F, et al. Regulation of trabecular meshwork cell contraction and intraocular pressure by miR-200c. PloS One (2012) 7(12):e51688. doi: 10.1371/journal.pone.0051688
66. Greene KM, Stamer WD, Liu Y. The role of microRNAs in glaucoma. Exp Eye Res (2022) 215:108909. doi: 10.1016/j.exer.2021.108909
67. Hindle AG, Thoonen R, Jasien JV, Grange RMH, Amin K, Wise J, et al. Identification of candidate miRNA biomarkers for glaucoma. Invest Ophthalmol Vis Sci (2019) 60(1):134–46. doi: 10.1167/iovs.18-24878
68. Fuchshofer R, Tamm ER. The role of TGF-β in the pathogenesis of primary open-angle glaucoma. Cell Tissue Res (2012) 347(1):279–90. doi: 10.1007/s00441-011-1274-7
69. Tsukamoto T, Kajiwara K, Nada S, Okada M. Src mediates TGF-β-induced intraocular pressure elevation in glaucoma. J Cell Physiol (2019) 234(2):1730–44. doi: 10.1002/jcp.27044
70. Zhang J, Wang Y. Altered expression of extracellular vesicles miRNAs from primary human trabecular meshwork cells induced by transforming growth factor-β2. DNA Cell Biol (2021) 40(7):988–97. doi: 10.1089/dna.2020.6298
71. Villarreal G Jr., Oh DJ, Kang MH, Rhee DJ. Coordinated regulation of extracellular matrix synthesis by the microRNA-29 family in the trabecular meshwork. Invest Ophthalmol Vis Sci (2011) 52(6):3391–7. doi: 10.1167/iovs.10-6165
72. Luna C, Li G, Qiu J, Epstein DL, Gonzalez P. Cross-talk between miR-29 and transforming growth factor-betas in trabecular meshwork cells. Invest Ophthalmol Vis Sci (2011) 52(6):3567–72. doi: 10.1167/iovs.10-6448
73. Ran W, Zhu D, Feng Q. TGF-β2 stimulates tenon's capsule fibroblast proliferation in patients with glaucoma via suppression of miR-29b expression regulated by Nrf2. Int J Clin Exp Pathol (2015) 8(5):4799–806.
74. Subramanian M, Rao SR, Thacker P, Chatterjee S, Karunagaran D. MiR-29b downregulates canonical wnt signaling by suppressing coactivators of β-catenin in human colorectal cancer cells. J Cell Biochem (2014) 115(11):1974–84. doi: 10.1002/jcb.24869
75. Ofri R. Intraocular pressure and glaucoma. Vet Clin North Am Exot Anim Pract (2002) 5(2):391–406. doi: 10.1016/s1094-9194(01)00004-4
76. Kabba JA, Xu Y, Christian H, Ruan W, Chenai K, Xiang Y, et al. Microglia: housekeeper of the central nervous system. Cell Mol Neurobiol (2018) 38(1):53–71. doi: 10.1007/s10571-017-0504-2
77. Kaur G, Han SJ, Yang I, Crane C. Microglia and central nervous system immunity. Neurosurg Clinics North America. (2010) 21(1):43–51. doi: 10.1016/j.nec.2009.08.009
78. Marinelli S, Basilico B, Marrone MC, Ragozzino D. Microglia-neuron crosstalk: signaling mechanism and control of synaptic transmission. Semin Cell Dev Biol (2019) 94:138–51. doi: 10.1016/j.semcdb.2019.05.017
79. Tang Y, Le W. Differential roles of M1 and M2 microglia in neurodegenerative diseases. Mol Neurobiol (2016) 53(2):1181–94. doi: 10.1007/s12035-014-9070-5
80. Fan YY, Huo J. A1/A2 astrocytes in central nervous system injuries and diseases: angels or devils? . Neurochem Int (2021) 148:599–620. doi: 10.1016/j.neuint.2021.105080
81. Bosco A, Steele MR, Vetter ML. Early microglia activation in a mouse model of chronic glaucoma. J Comp Neurol (2011) 519(4):599–620. doi: 10.1002/cne.22516
82. Hu X, Zhao GL, Xu MX, Zhou H, Li F, Miao Y, et al. Interplay between müller cells and microglia aggravates retinal inflammatory response in experimental glaucoma. J Neuroinflammation. (2021) 18(1):303. doi: 10.1186/s12974-021-02366-x
83. Miao Y, Zhao GL, Cheng S, Wang Z, Yang XL. Activation of retinal glial cells contributes to the degeneration of ganglion cells in experimental glaucoma. Prog Retin Eye Res (2023) 93:101169. doi: 10.1016/j.preteyeres.2023.101169
84. Aires ID, Boia R, Rodrigues-Neves AC, Madeira MH, Marques C, Ambrósio AF, et al. Blockade of microglial adenosine A2A receptor suppresses elevated pressure-induced inflammation, oxidative stress, and cell death in retinal cells. GLIA (2019) 67(5):896–914. doi: 10.1002/glia.23579
85. Varnum MM, Ikezu T. The classification of microglial activation phenotypes on neurodegeneration and regeneration in alzheimer's disease brain. Archivum immunologiae therapiae experimentalis. (2012) 60(4):251–66. doi: 10.1007/s00005-012-0181-2
86. Wei X, Cho KS, Thee EF, Jager MJ, Chen DF. Neuroinflammation and microglia in glaucoma: time for a paradigm shift. J Neurosci Res (2019) 97(1):70–6. doi: 10.1002/jnr.24256
87. Brites D. Microglia, neuroinflammation, and neurodegeneration. GLIA (2019) 67:E6–7. doi: 10.1002/glia.23674
88. Gui Y, Liu H, Zhang L, Lv W, Hu X. Altered microRNA profiles in cerebrospinal fluid exosome in Parkinson disease and Alzheimer disease. Oncotarget (2015) 6(35):37043–53. doi: 10.18632/oncotarget.6158
89. Xiao T, Zhang W, Jiao B, Pan CZ, Liu X, Shen L. The role of exosomes in the pathogenesis of alzheimer' disease. Trans Neurodegeneration. (2017) 6(1). doi: 10.1186/s40035-017-0072-x
90. Badanjak K, Fixemer S, Smajić S, Skupin A, Grünewald A. The contribution of microglia to neuroinflammation in parkinson's disease. Int J Mol Sci (2021) 22(9). doi: 10.3390/ijms22094676
91. Morris DR, Bounds SE, Liu H, Ding WQ, Chen Y, Liu Y, et al. Exosomal MiRNA transfer between retinal microglia and RPE. Int J Mol Sci (2020) 21(10). doi: 10.3390/ijms21103541
92. Song Y, Feng Y, Qu M, He T, Yuan F, Li W, et al. Exosomes shed from M2 microglia protect neuron from hypoxia. J Cereb Blood Flow Metab (2017) 37(1):274–5. doi: 10.1177/0271678X17695987
93. Bian B, Zhao C, He X, Gong Y, Ren C, Ge L, et al. Exosomes derived from neural progenitor cells preserve photoreceptors during retinal degeneration by inactivating microglia. J Extracellular Vesicles. (2020) 9(1). doi: 10.1080/20013078.2020.1748931
94. Saada J, McAuley RJ, Marcatti M, Tang TZ, Motamedi M, Szczesny B. Oxidative stress induces z-DNA-binding protein 1-dependent activation of microglia via mtDNA released from retinal pigment epithelial cells. J Biol Chem (2022) 298(1). doi: 10.1016/j.jbc.2021.101523
95. Eom AY, Bondarenko V, Roque RS. Activated retinal microglia secrete exosomes containing galectin-3. FASEB J (2020) 34(SUPPL 1). doi: 10.1096/fasebj.2020.34.s1.06633
96. Santiago JV, Rayaprolu S, Xiao H, Seyfried NT, Rangaraju S. Identification of state-specific proteomic characteristics of microglia-derived exosomes. Alzheimer's dementia J Alzheimer's Assoc (2021) 17:e058665. doi: 10.1002/alz.058665
97. Song Y, Li Z, He T, Qu M, Jiang L, Li W, et al. M2 microglia-derived exosomes protect the mouse brain from ischemia-reperfusion injury via exosomal miR-124. Theranostics (2019) 9(10):2910–23. doi: 10.7150/thno.30879
98. Luo C, Tang X, Liu J, Tang S, Xu H. MiRNA profiles in exosomes from activated microglia-implication in retinal neuroinflammation. Invest Ophthalmol Visual Science. (2019) 60(9).
99. de Hoz R, Ramírez AI, González-Martín R, Ajoy D, Rojas B, Salobrar-Garcia E, et al. Bilateral early activation of retinal microglial cells in a mouse model of unilateral laser-induced experimental ocular hypertension. Exp eye Res (2018) 171:12–29. doi: 10.1016/j.exer.2018.03.006
100. Ramírez AI, Salazar JJ, de Hoz R, Rojas B, Gallego BI, Salobrar-García E, et al. Macro- and microglial responses in the fellow eyes contralateral to glaucomatous eyes. Prog Brain Res (2015) 220:155–72. doi: 10.1016/bs.pbr.2015.05.003
101. Ramírez AI, de Hoz R, Fernández-Albarral JA, Salobrar-Garcia E, Rojas B, Valiente-Soriano FJ, et al. Time course of bilateral microglial activation in a mouse model of laser-induced glaucoma. Sci Rep (2020) 10(1):4890. doi: 10.1038/s41598-020-61848-9
102. Xu W, Wu Y, Hu Z, Sun L, Dou G, Zhang Z, et al. Exosomes from microglia attenuate photoreceptor injury and neovascularization in an animal model of retinopathy of prematurity. Mol Ther - Nucleic Acids (2019) 16:778–90. doi: 10.1016/j.omtn.2019.04.029
103. Li N, Shu J, Yang X, Wei W, Yan A. Exosomes derived from M2 microglia cells attenuates neuronal impairment and mitochondrial dysfunction in alzheimer’s disease through the PINK1/Parkin pathway. Front Cell Neurosci (2022) 16:874102. doi: 10.3389/fncel.2022.874102
104. Li Z, Song Y, He T, Li W, Wen R, Tang Y, et al. M2 microglial derived exosome improves functional recovery via reducing glial scar formation after ischemic stroke in mice. J Cereb Blood Flow Metab (2019) 39(1):67–8. doi: 10.1177/0271678X19850985
105. Song Y, Li Z, Li W, Wang Y, Zhang Z, Tang Y, et al. M2 microglia derived exosome improves neurobehavioral recovery through promoting neurogenesis in a mouse model of middle cerebral artery occlusion. J Cereb Blood Flow Metab (2019) 39(1):390–1. doi: 10.1177/0271678X19851020
106. Liu Y, Li YP, Xiao LM, Chen LK, Zheng SY, Zeng EM, et al. Extracellular vesicles derived from M2 microglia reduce ischemic brain injury through microRNA-135a-5p/TXNIP/NLRP3 axis. Lab Invest (2021) 101(7):837–50. doi: 10.1038/s41374-021-00545-1
107. Zhang D, Cai G, Liu K, Zhuang Z, Jia K, Pei S, et al. Microglia exosomal miRNA-137 attenuates ischemic brain injury through targeting Notch1. Aging (Albany NY). (2021) 13(3):4079–95. doi: 10.18632/aging.202373
108. Zhou Z, Li C, Bao T, Zhao X, Xiong W, Luo C, et al. Exosome-shuttled miR-672-5p from anti-inflammatory microglia repair traumatic spinal cord injury by inhibiting AIM2/ASC/Caspase-1 signaling pathway mediated neuronal pyroptosis. J Neurotrauma. (2022) 39(15-16):1057–74. doi: 10.1089/neu.2021.0464
109. Li Y, Liu Z, Song Y, Pan JJ, Jiang Y, Shi X, et al. M2 microglia-derived extracellular vesicles promote white matter repair and functional recovery via miR-23a-5p after cerebral ischemia in mice. Theranostics (2022) 12(7):3553–73. doi: 10.7150/thno.68895
110. Weinreb RN, Aung T, Medeiros FA. The pathophysiology and treatment of glaucoma: a review. Jama (2014) 311(18):1901–11. doi: 10.1001/jama.2014.3192
111. Greco A, Rizzo MI, De Virgilio A, Gallo A, Fusconi M, de Vincentiis M. Emerging concepts in glaucoma and review of the literature. Am J Med (2016) 129(9):1000.e7–1000.e13. doi: 10.1016/j.amjmed.2016.03.038
112. Yuan L, Neufeld AH. Activated microglia in the human glaucomatous optic nerve head. J Neurosci Res (2001) 64(5):523–32. doi: 10.1002/jnr.1104
113. Yang X, Luo C, Cai J, Powell DW, Yu D, Kuehn MH, et al. Neurodegenerative and inflammatory pathway components linked to TNF-α/TNFR1 signaling in the glaucomatous human retina. Invest Ophthalmol Visual science. (2011) 52(11):8442–54. doi: 10.1167/iovs.11-8152
114. Luo C, Yang X, Kain AD, Powell DW, Kuehn MH, Tezel G. Glaucomatous tissue stress and the regulation of immune response through glial toll-like receptor signaling. Invest Ophthalmol Visual science. (2010) 51(11):5697–707. doi: 10.1167/iovs.10-5407
115. Guo C, Wu N, Niu X, Wu Y, Chen D, Guo W. Comparison of T helper cell patterns in primary open-angle glaucoma and normal-pressure glaucoma. Med Sci monitor Int Med J Exp Clin Res (2018) 24:1988–96. doi: 10.12659/msm.904923
116. Wong M, Huang P, Li W, Li Y, Zhang SS, Zhang C. T-helper1/T-helper2 cytokine imbalance in the iris of patients with glaucoma. PloS One (2015) 10(3):e0122184. doi: 10.1371/journal.pone.0122184
117. Jin N, Sha W, Gao L. Shaping the microglia in retinal degenerative diseases using stem cell therapy: practice and prospects. Front Cell Dev Biol (2021) 9:741368. doi: 10.3389/fcell.2021.741368
118. Nazari H, Alborzi F, Heirani-Tabasi A, Hadizadeh A, Asbagh RA, Behboudi B, et al. Evaluating the safety and efficacy of mesenchymal stem cell-derived exosomes for treatment of refractory perianal fistula in IBD patients: clinical trial phase i. Gastroenterol Rep (2022) 10. doi: 10.1093/gastro/goac075
119. Li Q, Zhang L, Chen X, Shi R, Wang X. Mesenchymal stem cells-derived extracellular vesicles in the treatment and repair of acute and chronic renal injuries. Chin J Tissue Eng Res (2022) 26(31):5069–75. doi: 10.12307/2022.729
120. Dehghani L, Khojasteh A, Soleimani M, Oraee Yazdani S. Safety intraparenchymal injection of allogenic placenta mesenchymal stem cells derived exosome in patients underwent decompressive craniectomy following malignant middle cerebral artery infarct, a randomized clinical trial. Cytotherapy (2021) 23(5):S115. doi: 10.1016/S1465324921004576
121. Esmaeilizade Z, Mohammadi B, Omrani MD, Ghaderian SMH, Rajabibazl M, Fazeli Z. Preclinical studies and clinical trials with mesenchymal stem cell for demyelinating diseases: a systematic review. Curr Stem Cell Res Ther (2021) 16(8):1005–17. doi: 10.2174/1574888X16666210208162318
122. Chow LC, Mead B. Extracellular vesicles as a potential therapeutic for age-related macular degeneration. Neural Regeneration Res (2023) 18(9):1876–80. doi: 10.4103/1673-5374.367835
123. Yu C, Chen P, Xu J, Liu Y, Li H, Wang L, et al. hADSCs derived extracellular vesicles inhibit NLRP3inflammasome activation and dry eye. Sci Rep (2020) 10(1):14521. doi: 10.1038/s41598-020-71337-8
124. Li D, Gong Y. A promising strategy for non-arteritic anterior ischemic optic neuropathy: intravitreal mesenchymal stem cell exosome. Curr Stem Cell Res Ther (2020) 16(2):109–14. doi: 10.2174/1574888X15666200814121849
125. Patel VB, Fedak PWM. Commentary: cell therapy goes subcellular [Editorial]. J Thorac Cardiovasc Surgery. (2022) 164(6):e386–7. doi: 10.1016/j.jtcvs.2021.09.004
126. Cai Y, Liu W, Lian L, Xu Y, Bai X, Xu S, et al. Stroke treatment: is exosome therapy superior to stem cell therapy? Biochimie (2020) 179:190–204. doi: 10.1016/j.biochi.2020.09.025
127. Mathew B, Ravindran S, Liu X, Torres L, Chennakesavalu M, Huang CC, et al. Mesenchymal stem cell-derived extracellular vesicles and retinal ischemia-reperfusion. Biomaterials (2019) 197:146–60. doi: 10.1016/j.biomaterials.2019.01.016
128. Carvalho LS, György B, Mu D, Shah S, Vandenberghe LH, Maguire CA. Retinal tropism of exosome-associated aav vector via intravitreal delivery. Mol Ther (2015) 23:S61. doi: 10.1038/mt.2015.74
129. Wassmer SJ, Carvalho LS, György B, Vandenberghe LH, Maguire CA. Exosome-associated AAV2 vector mediates robust gene delivery into the murine retina upon intravitreal injection. Sci Rep (2017) 7:45329. doi: 10.1038/srep45329
130. Huang H, Kolibabka M, Eshwaran R, Chatterjee A, Schlotterer A, Willer H, et al. Intravitreal injection of mesenchymal stem cells evokes retinal vascular damage in rats. FASEB J (2019) 33(12):14668–79. doi: 10.1096/fj.201901500R
131. Tuekprakhon A, Sangkitporn S, Trinavarat A, Pawestri AR, Vamvanij V, Ruangchainikom M, et al. Intravitreal autologous mesenchymal stem cell transplantation: a non-randomized phase I clinical trial in patients with retinitis pigmentosa. Stem Cell Res Ther (2021) 12(1). doi: 10.1186/s13287-020-02122-7
132. György B, Maguire CA. Extracellular vesicles: nature's nanoparticles for improving gene transfer with adeno-associated virus vectors. Wiley Interdiscip Reviews: Nanomedicine Nanobiotechnol (2018) 10(3). doi: 10.1002/wnan.1488
133. Pollalis D, Nair GKG, Nanda AV, Kang C, Kim D, Lee SY. Exosome delivery to retina and active targeting of CNV by RGD-conjugated exosomes. Invest Ophthalmol Visual Sci (2022) 63(7):303–F0106.
134. Arjmand B, Alavi-Moghadam S, Rezaei-Tavirani M, Kokabi-Hamidpour S, Arjmand R, Gilany K, et al. GMP-compliant mesenchymal stem cell-derived exosomes for cell-free therapy in cancer. Methods Mol Biol (Clifton NJ) (2022). doi: 10.1007/7651_2022_467
135. Lui PPY, Leung YT. Practical considerations for translating mesenchymal stromal cell-derived extracellular vesicles from bench to bed. Pharmaceutics (2022) 14(8). doi: 10.3390/pharmaceutics14081684
136. Ahn SH, Ryu SW, Choi H, You S, Park J, Choi C. Manufacturing therapeutic exosomes: from bench to industry. Molecules Cells (2022) 45(5):284–90. doi: 10.14348/molcells.2022.2033
137. Chen YS, Lin EY, Chiou TW, Harn HJ. Exosomes in clinical trial and their production in compliance with good manufacturing practice. Tzu Chi Med J (2020) 32(2):113–20. doi: 10.4103/tcmj.tcmj_182_19
138. Moisseiev E, Anderson JD, Oltjen S, Goswami M, Zawadzki RJ, Nolta JA, et al. Protective effect of intravitreal administration of exosomes derived from mesenchymal stem cells on retinal ischemia. Curr Eye Res (2017) 42(10):1358–67. doi: 10.1080/02713683.2017.1319491
139. Ha DH, Kim SD, Lee J, Kwon HH, Park GH, Yang SH, et al. Toxicological evaluation of exosomes derived from human adipose tissue-derived mesenchymal stem/stromal cells. Regul Toxicol Pharmacol (2020) 115:104686. doi: 10.1016/j.yrtph.2020.104686
140. Weiss JN, Levy S. Stem cell ophthalmology treatment study: bone marrow derived stem cells in the treatment of retinitis pigmentosa. Stem Cell Investig (2018) 5:18. doi: 10.21037/sci.2018.04.02
141. Quigley HA. Use of animal models and techniques in glaucoma research: introduction. Methods Mol Biol (2018) 1695:1–10. doi: 10.1007/978-1-4939-7407-8_1
142. Iglesias AI, Springelkamp H, Ramdas WD, Klaver CC, Willemsen R, van Duijn CM. Genes, pathways, and animal models in primary open-angle glaucoma. Eye (Lond). (2015) 29(10):1285–98. doi: 10.1038/eye.2015.160
143. Wang L, Zhou MB, Zhang H. The emerging role of topical ocular drugs to target the posterior eye. Ophthalmol Ther (2021) 10(3):465–94. doi: 10.1007/s40123-021-00365-y
Keywords: exosome, glaucoma, trabecular meshwork, neuroinflammation, microglia, oxidative stress
Citation: Wang L and Wei X (2023) Exosome-based crosstalk in glaucoma pathogenesis: a focus on oxidative stress and neuroinflammation. Front. Immunol. 14:1202704. doi: 10.3389/fimmu.2023.1202704
Received: 09 April 2023; Accepted: 03 July 2023;
Published: 17 July 2023.
Edited by:
Wenru Su, Sun Yat-sen University, ChinaReviewed by:
Padmanabhan Paranji Pattabiraman, Indiana University-Purdue University Indianapolis, United StatesSanming Li, University of Texas Health Science Center at Houston, United States
Copyright © 2023 Wang and Wei. This is an open-access article distributed under the terms of the Creative Commons Attribution License (CC BY). The use, distribution or reproduction in other forums is permitted, provided the original author(s) and the copyright owner(s) are credited and that the original publication in this journal is cited, in accordance with accepted academic practice. No use, distribution or reproduction is permitted which does not comply with these terms.
*Correspondence: Xin Wei, weixin_1982@163.com