- 1Department of Radiotherapy, Second Hospital of Jilin University, Changchun, China
- 2National Health Commission (NHC) Key Laboratory of Radiobiology, School of Public Health, Jilin University, Changchun, China
Women worldwide are more likely to develop breast cancer (BC) than any other type of cancer. The treatment of BC depends on the subtype and stage of the cancer, such as surgery, radiotherapy, chemotherapy, and immunotherapy. Although significant progress has been made in recent years, advanced or metastatic BC presents a poor prognosis, due to drug resistance and recurrences. During embryonic development, myeloid-derived suppressor cells (MDSCs) develop that suppress the immune system. By inhibiting anti-immune effects and promoting non-immune mechanisms such as tumor cell stemness, epithelial-mesenchymal transformation (EMT) and angiogenesis, MDSCs effectively promote tumor growth and metastasis. In various BC models, peripheral tissues, and tumor microenvironments (TME), MDSCs have been found to amplification. Clinical progression or poor prognosis are strongly associated with increased MDSCs. In this review, we describe the activation, recruitment, and differentiation of MDSCs production in BC, the involvement of MDSCs in BC progression, and the clinical characteristics of MDSCs as a potential BC therapy target.
1 Introduction
Breast cancer (BC) is the primary driver of cancer-related mortality among females globally (1). BC can be categorized into four different subtypes based on the expression of the Human epidermal growth factor receptor (HER2/neu), the estrogen receptor (ER), and the progesterone receptor (PR): triple negative breast cancer (TNBC), Luminal A, Luminal B, and HER2 (+). This classification is the basis for most BC treatments, including surgery, radiotherapy (RT), chemotherapy, targeted therapy, and immunotherapy (2). Immunotherapy is becoming increasingly essential in treating other tumors, but only a tiny percentage of BC patients are aid from immunotherapy at present. In fact, the therapeutic relevance of immunotherapy is restricted to a minority of patients, and secondary resistance further limits the effect of immunotherapy to substantially better the prognosis of BC patients (3). Immunotherapies, particularly immune checkpoint inhibitors (ICIs), are ineffective as a sole treatment in most patients with BC. Tumor microenvironment (TME) is widely accepted as having an irreplaceable function in the drug resistance mechanism. Additionally, Myeloid-derived suppressor cells (MDSCs) serve an essential function in TME (3).
Myeloid-derived suppressor cells (MDSCs), a diverse group of immature myeloid cells (IMCs), serve an essential function in the immune cell network (4). MDSCs play a role in tumor development via a variety of immunosuppressive mechanisms, including metabolite depletion, upregulation of reactive oxygen species (ROS), and secretion of multiple cytokines, and kinds of non-immunosuppressive mechanisms, like epithelial-mesenchymal transformation (EMT), promoting tumor cell stemness, as well as tumor vascular production (5). Previous research has demonstrated a correlation between MDSCs and poor overall survival (OS) in patients (6). Consequently, cancer immunotherapy can be proven to enhance by fostering MDSCs elimination, inhibiting their recruitment, expansion, and function.
In this review, we summarize the immunosuppressive function of MDSCs, their role in BC, and strategies for targeting them in various cancer treatments. We explore the feasibility and prospective development of multiple targeted MDSCs detection methods and therapeutics for the treatment or prevention of BC.
2 MDSCs in breast cancer
2.1 Classification of MDSCs in breast cancer
MDSCs from BC patients are functionally and phenotypically similar to MDSCs that come from bone marrow, indicating that MDSCs emerge from myeloid precursors (4). Granulocytic (G)-MDSCs (CD11b+Ly6G+Ly6Clow) and monocytic (M)-MDSCs (CD11b+Ly6G-Ly6Chigh) could be precisely identified relying on their phenotypic and morphological characteristics in mice as research demonstrates (7). In contrast to mouse MDSCs, isolating and identifying human MDSCs is difficult due to their heterogeneity, proximity phenotypic and functional closeness to other cell subpopulations, and lack of definitive markers (7, 8). MDSCs are correspondingly classified into two categories in humans. CD11b+CD33+HLA-DR-/low CD14+CD15- identifies M-MDSCs, whereas CD11b+CD33+HLA-DR-/lowCD14-CD15+(or CD66b+) identifies G-MDSCs (6, 7). The identification of CD84 as MSDC-specific cell surface markers in BC was reported using a single-cell transcriptomic approach by Alshetaiwi et al. (9). In addition, a subpopulation of MDSCs in humans is comprised of undifferentiated progenitor cells known as e-MDSCs, identified as HLA-DR-CD33+Lin- (CD3/14/15/19/56) for which the mouse counterpart has not been discovered (7) (Table 1).
2.2 MDSCs production and activation in breast cancer
The development of MDSCs is governed by a complicated system of signals that can be categorized into two groups: those that promote the accumulation of IMCs and those that cause the activation of these cells (10). The signal transducer and activator of transcription (STAT) family, IFN regulators, Notch, adenosine receptor A2b, NLRP3, and many other signaling pathways and regulators are responsible for stimulating myelopoiesis, inhibiting the maturation and differentiation of progenitor cells, and promoting the expansion of the IMCs (9, 10). The other group is accountable for the pathological activation of immature cells that acquire an immunosuppressive phenotype, involving diverse signaling pathways and regulators such as NF-κB pathway, STAT1 pathway, STAT6 pathway, prostaglandin E2 (PGE2), and Cyclooxygenase-2 (COX-2) and ER stress response pathway (9, 10). These two groups of signals partially overlap but are controlled by various transcription factors and intermediates, both of which are necessary for the accumulation of MDSCs.
In BC, factors that contribute to the amplification and activation of MDSCs involve granulocyte-macrophage colony-stimulating factor (GM-CSF) (10, 11), granulocyte colony-stimulating factor (G-CSF) (11, 12), PGE2 (13, 14), vascular endothelial growth factor (VEGF) and interleukins (IL-1 (15), IL-6 (16), IL-13 (17), IL-17 (18), IL-20 (19), IL-33 (20), IL-34 (21)), macrophage migration inhibitory factor (MIF) (22), microRNAs (miRNAs) derived from tumor exosomes (23). It is interesting to note that higher levels of psychological stress in patients with BC might result in the production of stress-related hormones and cytokines (IL-1Rα, IP 10, G-CSF, and IL-6), which in turn stimulate the production and accumulation of MDSCs (24) (Figure 1).
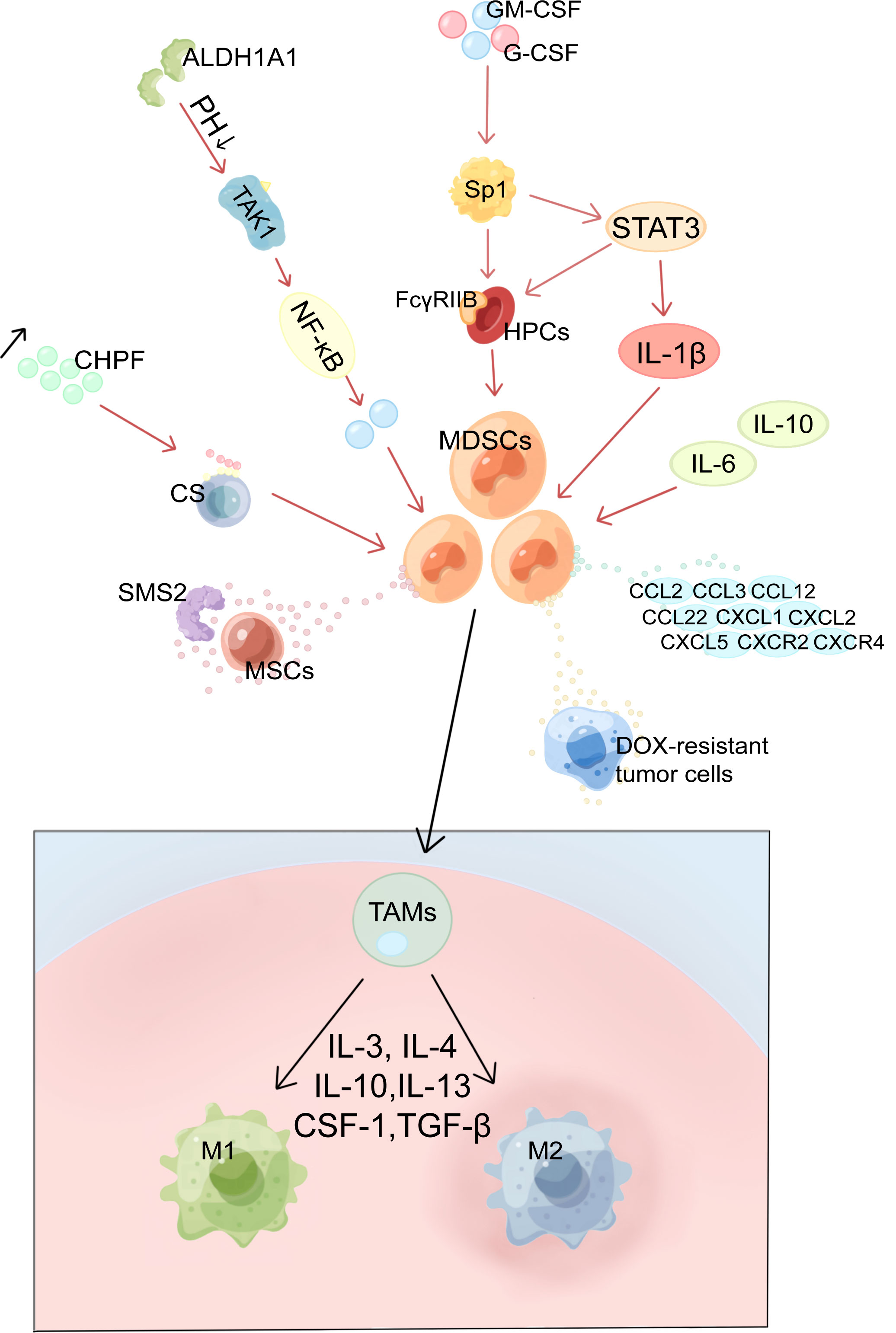
Figure 1 Mechanism of MDSCs production and activation, recruitment and differentiation in BC. MDSCs, myeloid-derived suppressor cells; HPCs, haematopoietic progenitor cells; Sp1, specific protein 1; GM-CSF, granulocyte-macrophage colony-stimulating factor; G-CSF, granulocyte colony-stimulating factor; ALDH1A1, aldehyde dehydrogenase 1A1; TAK1, TGF-β-activated kinase 1; CS, Chondroitin sulfate; CHPF, chondroitin polymerase factor; SMS2, Sphingosine synthase 2; MSCs, mesenchymal stem cells; TAMs, tumor-associated macrophages; M1, M1 macrophages; M2, M2 macrophages.
Tumor cell derived G-CSF and GM-CSF are key factors contributing to MDSCs accumulation (10, 11). CSF raises the levels of FcγRIIB on hematopoietic progenitor cells (HPCs) by activating specific protein 1 (Sp1), which then activates the STAT3 signaling pathway, which biases hematopoiesis toward the myeloid lineage spectrum and promotes MDSCs production by HPCs (10, 25). Moreover, chondroitin polymerase factor (CHPF), which is frequently highly expressed in BC tissues, facilitates the binding of G-CSF to cell surface Chondroitin sulfate (CS) and promotes the accumulation of MDSCs (26). In addition, aldehyde dehydrogenase 1A1 (ALDH1A1) relies on its enzymatic activity to reduce intracellular pH in BC cells in order to increase TGF-β-activated kinase 1(TAK1) phosphorylation and activate NF-κB pathways, which then induces GM-CSF secretion, thereby inducing MDSCs amplification and promoting BC progression (27).
With a diameter of 50–200 nm, exosomes are lipid bilayer structures that are important in the reprogramming of TME. IL-6, IL-10, and other cytokines released by BC cells (4T1) exosomes would boost the stimulation and expansion of MDSCs by promoting STAT3 phosphorylation in myeloid cells, which would, in turn, reduce myeloid proliferation and death and hasten their differentiation into MDSCs (23). Jiang M et al. used miRNA microarrays to identify miRNAs secreted by tumor exosomes; two of them, miR-9 and miR-181a, were shown to target protein inhibitors of activated STAT3 (PIAS3) and cytokine signaling protein 3 (SOCS3), respectively, therefore activating the JAK/STAT signaling cascade (28). Moreover, the accumulation of early-stage MDSCs was stimulated by prolonged SOCS3 suppression and aberrant upregulating of the JAK/STAT signaling pathway (29).
In addition, various pathway signaling molecules overlap with each other and can form loops to regulate each other. In BC cells Mammalian target of rapamycin (mTOR) signaling stimulates MDSCs accumulation by regulating G-CSF, and MDSCs were found to mutually increase Tumor-initiating cells frequency by activating Notch in tumor cells, which in turn promotes G-CSF secretion, forming a feed-forward loop leading to further MDSCs expansion (30). Furthermore, by inducing autocrine secretion of GM-CSF, IL-33 in the TME inhibits apoptosis and maintains MDSCs survival, resulting in a positive feedback cycle for MDSCs accumulation (20).
2.3 MDSCs recruitment in breast cancer
Many different variables can induce the recruitment of MDSCs into tumor tissue. These factors include chemokines, cytokines, and complements generated by tumor cells and normal cells. Chemokines are an essential component in the process of recruiting MDSCs (31).
BC regulates the production of chemokines by MDSCs via multiple pathways. Enhanced lung fibroblast CXCL1 secretion reduces the immunity of lung microenvironment by recruiting G-MDSCs and facilitates the formation of niches in the anterior lung metastases of BC (32). Inactivation of retinoblastoma enhances the secretion of the chemoattractant CCL2. Consequently, the activated CCL2-CCR2 axis in the TME promotes tumor angiogenesis and recruitment of tumor-associated macrophages (TAMs) and MDSCs to the TME (33). BC exosomes convey upregulated miR-200b-3p, taken by alveolar epithelial type II cells, and targets PTEN straightforwardly. Suppression of PTEN facilitates activation of the AKT/NF-κBp65 pathway, which increases CCL2 expression and MDSCs recruitment, thereby promoting pulmonary metastasis of BC (34). ERO1-α, a tumor-associated endoplasmic reticulum disulfide oxidase, facilitates the oxidative folding of G-CSF, CXCL1, and CXCL2 to produce and recruit G-MDSCs (35). TGF-β1 promotes miR-494 upregulation in MDSCs, and miR-494 expression enhances CXCR4-mediated MDSCs chemotaxis (36). The transcription factor ΔNp63 has been reported to directly regulate CXCL2 and CCL22 to promote MDSCs recruitment in TNBC (37).
A diversity of other elements also influences the recruitment of MDSCs. S100A8/A9 proteins are chemotactic for MDSCs; for instance, the lung and liver can express high levels of S100A8, which promotes the recruitment of MDSCs at these sites, thereby promoting BC metastasis (38). Chen JY et al. found that BC-derived VEGF-C upregulates chemokines derived from lymphatic endothelial cells (LECs) to recruit MDSCs to TME and lymph nodes (LNs) via CXCR2 (39). There is experimental evidence that, in coordination with LECs, interstitial fluid flow promotes the spread of MDSCs at the existence of 4T1 cells and that inhibition of VEGFR 3 reduces the flow response of MDSCs and 4T1 cells (40). Moreover, epigenetic regulation is implicated. The epigenetic regulator Lysine acetyltransferase 6A (KAT6A) dependent SMAD3 protein acetylation promotes MDSCs recruitment and TNBC metastasis (41). In addition, complement activation and C5a signaling are involved in the recruitment of MDSCs into TME and in the inhibition of CD8+ T cell mediated tumor elimination, thereby inducing angiogenesis in the lungs of tumor-bearing mice, promoting BC lung metastasis (42). Interestingly, Cheng R et al. discovered that periodontal inflammation (PI) could upregulate CCL5, CXCL12, CCL2, and CCL5. These chemokines recruit MDSCs, which can generate pre-metastatic ecological niches at inflammation sites to promote the metastasis of BC (43).
2.4 MDSCs differentiation in breast cancer
MDSCs can differentiate under the regulation of multiple transcription factors (Figure 1). They differentiate into TAMs and inflammatory dendritic cells (inf-DCs). Since G-MDSCs are typically have a brief half-life and differentiate into tumor-associated neutrophils (TAN), the differentiation of M-MDSCs has been studied in more detail (44). In response to environmental stresses such as hypoxia, M-MDSCs can differentiate into TAMs after migrating to the target tissue and undergoing migration. In response to lipopolysaccharide (LPS), TNF-α, and IFN-γ, TAMs can polarize into either the M1 phenotype, which has pro-inflammatory and anti-tumor activities, or the M2 phenotype, which has pro-tumor activities (45).
Development and progression of BC are accompanied by a transition from MDSCs to TAMs. In MDSCs, IL-33 induces the expression of IL-13 while suppressing that of IL-12. As a result, M2 macrophages and Th2 cells may become polarized inside the tumor site, which is detrimental to anti-tumor immunity (20). Similarly both Sphingosine synthase 2 (SMS2) and exosomes secreted by mesenchymal stem cells (MSCs) can speed the progression of BC by inducing the differentiation of M-MDSCs into immunosuppressive M2-polarized macrophages (45, 46). Tumor cells resistant to doxorubicin (DOX) secrete PGE2, which stimulates the EP2-EP4/cAMP/PKA signaling pathway in MDSCs and, in turn, increases MDSCs growth and M2 polarization by inducing miR-10a expression (13). In addition, Natural killer T (NKT) cells have been shown to promote the transformation of CD11b+ HLA-DR-MDSCs into CD11b low HLA-DR dendritic cells (DCs) (47).
2.5 Identification and detection of MDSCs in breast cancer
The current methods commonly used for MDSCs detection are magnetic selective enrichment, sorting and purification, flow cytometry sorting, and density gradient separation (48). MDSCs identification and functional assays are aided by the secretion of downstream molecules and the determination of target cell effects. Recently several novel assays have been proposed. Hoffmann SHL et al. demonstrated that targeting MDSCs cell surface integrin CD11b with radionuclide-labeled monoclonal antibody (mAb) has little effect on cell viability and function, and can be used to image MDSCs in tumor-bearing mice using PET (49). Sceneay J et al. discovered that BM-MDSC (GM-CSF and IL-6 cultured bone marrow cells to produce MDSCs nearly identical to G-MDSCs) could be persistently tagged with a near-infrared fluorescent dye called DiD and monitored in vivo by optical imaging (OI), as well as repositioned and identified in vitro by flow cytometry. This technique permits the study of the distribution and destiny of DiD-labeled BM-MDSC in breast tumor-bearing mice as a way of finding MDSCs in BC that present new organ-specific changes (50). Although there have been many attempts of targeted detection methods for MDSCs, most of them can only stay in the laboratory stage and still have problems of complex methods and confusing detection. There is still a need to develop more rapid functional assays as well as definitive marker molecular assays.
3 Immunosuppressive effects and non-immune functions of MDSCs in the progression of breast cancer
MDSCs substantially suppress tumor-fighting T and B cells, particularly cytotoxic T lymphocytes (CTL) and pro-inflammatory cells like natural killer (NK) cells in TME. Additionally, MDSCs support cancer progression by inducing Tregs and Th17 cells, therefore modifying the microenvironment that fosters tumor development and driving cells to evade immune surveillance (51) (Figure 2). In addition, MDSCs also promote BC progression through non-immunosuppressive pathways including promotion of tumor stem cells, mediation of EMT, and promotion of angiogenesis. These functions are summarized in Figure 3.
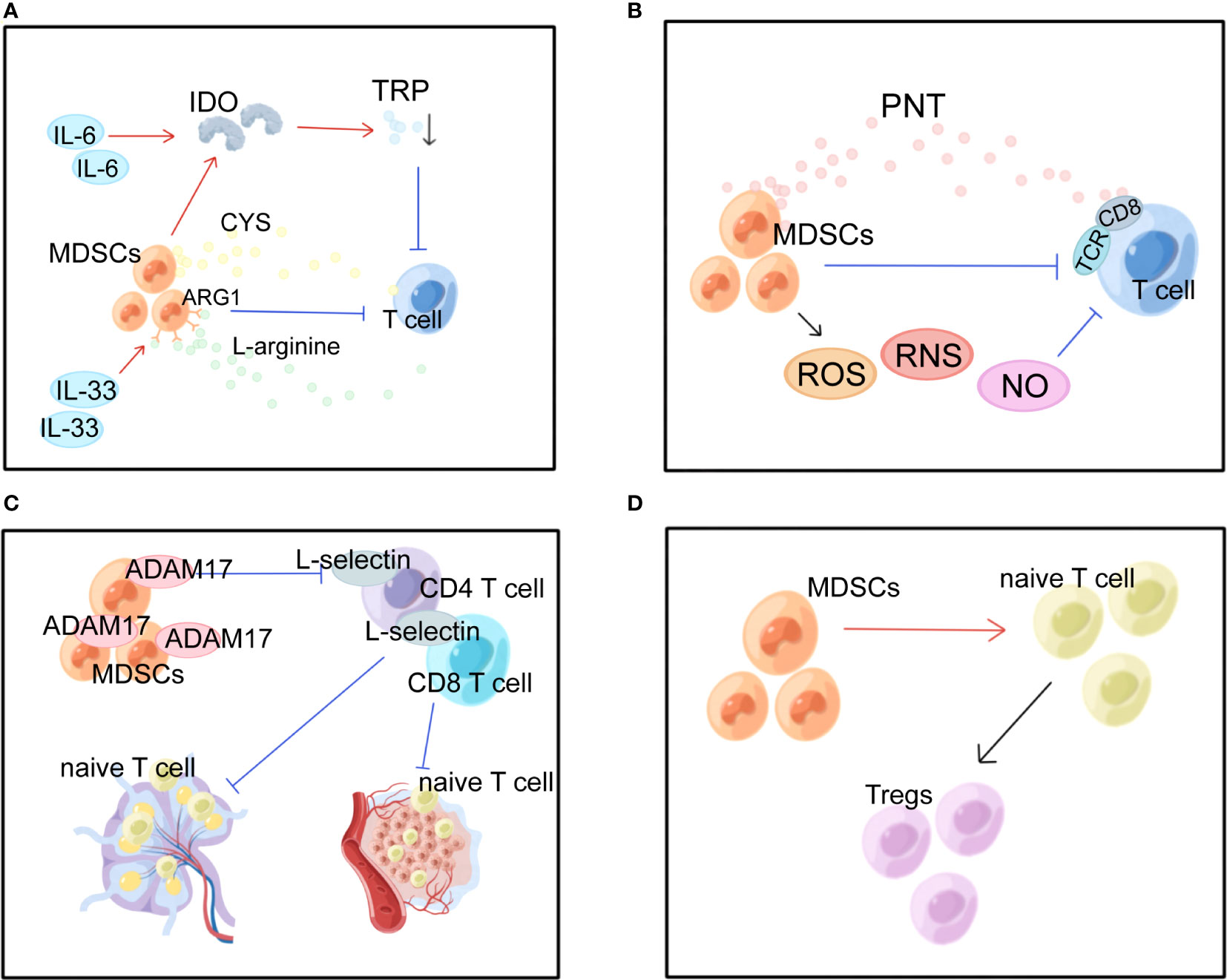
Figure 2 Multiple immunosuppressive effects of MDSCs in BC. MDSCs, myeloid-derived suppressor cells; IDO, indoleamine 2, 3-dioxygenase; TRP, tryptophan; ARG1, arginase 1; CYS, cysteine; PNT, peroxynitrite; TCR, T cell receptor; ROS, reactive oxygen species; RNS, reactive nitrogen species; ADAM17, a disintegrin and metalloproteinase domain 17. (A) MDSCs suppress T cells by depleting nutrients such as ARG, TRP, and CYS. (B) MDSCs produce ROS, RNS and NO causing oxidative stress and suppressing T cells. (C) MDSCs interfere with lymphocyte migration through direct contact and plasma membrane expression of ADAM17.(D) MDSCs promote the conversion of naive CD4+ T cells into Tregs.
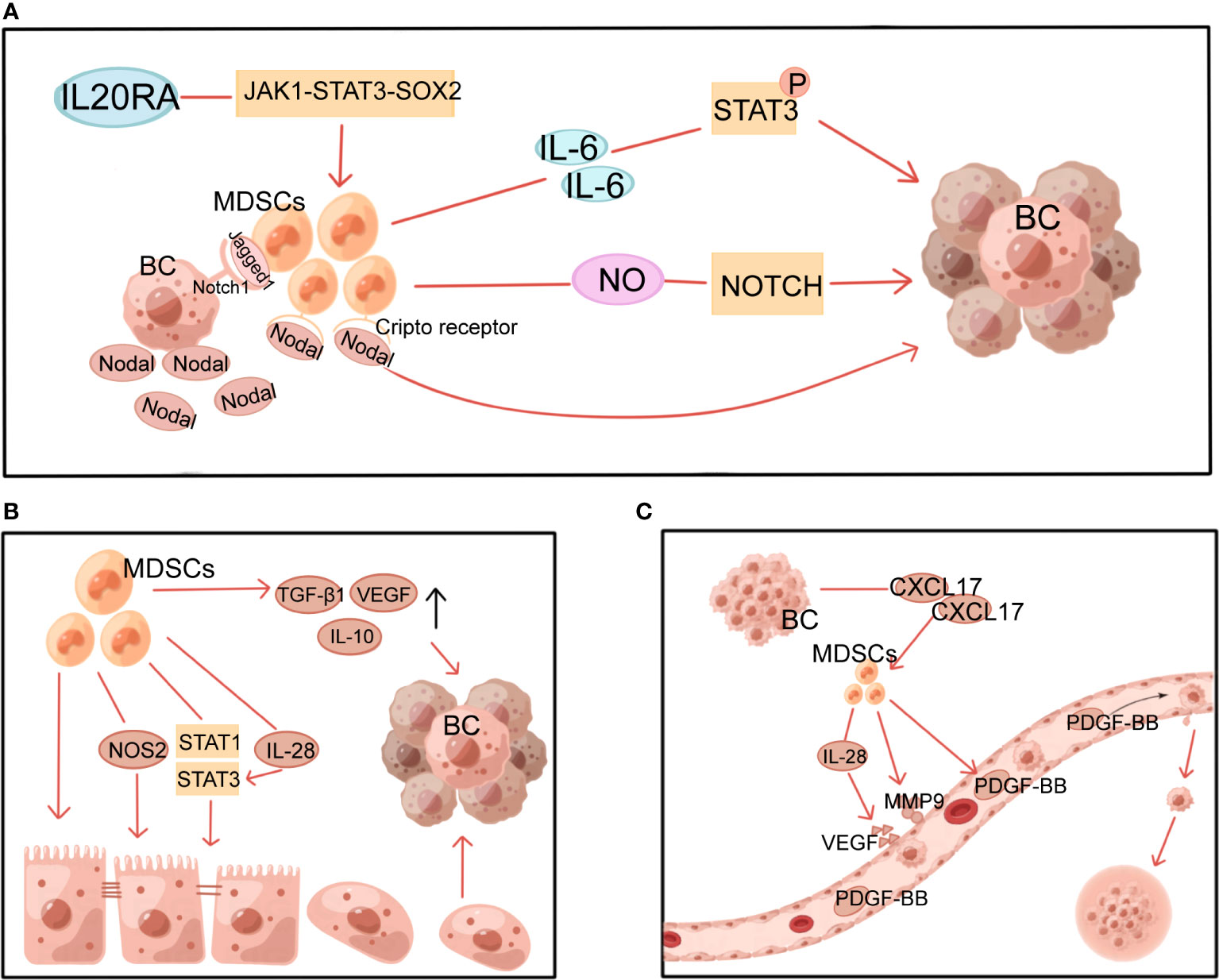
Figure 3 Multiple non-immune functions of MDSCs in BC. MDSCs, myeloid-derived suppressor cells; NOS2, nitric oxide synthase 2; VEGF, vascular endothelial growth factor; PDGF-BB, platelet-derived growth factor-BB; MMP9, matrix metalloproteinase 9; BC, breast cancer. (A) MDSCs induce and maintain tumor cell stemness through STAT3 and Notch pathways. (B) MDSCs induce EMT through multiple mechanisms. (C) MDSCs induce angiogenesis in tumors.
3.1 Immunosuppressive effects of MDSCs
In the BC microenvironment, MDSCs exert immunosuppressive effects in several ways. The most prominent of these is the suppression of T-cell activity. The first mechanism is nutrient depletion (Figure 2A). MDSCs have been demonstrated to conduct their suppressive function by stimulating the formation of indoleamine 2, 3-dioxygenase (IDO), which diminishes local tryptophan (TRP) and creates cytotoxic metabolites such as kynurenine in the TME and lymphatic drainage areas, which causes a rise in Tregs, inhibition of antigen-specific immune responses, and suppression of tumor-specific CTLs (52). Overexpression of IDO is maintained by STAT3-dependent NF-κB activation by IL-6 (16, 52). Moreover, IL-33 strengthened the suppressive action of MDSCs against T cells arginase 1 (ARG1)-dependent L-arginine depletion (19, 50). The cysteine (CYS) that T cells need for activation and proper functioning is depleted because MDSCs devour CYS but do not return CYS to their surroundings (53).
The second mechanism is oxidative stress generation (Figure 2B). MDSCs can inhibit T cells in the TME via ROS, reactive nitrogen species (RNS), and nitric oxide (NO). MDSCs induce tolerance in T cells through nitration/nitrosylation of T cell receptor (TCR) and CD8+ molecules on the cell surface and production of the free radical peroxynitrite (PNT) (54). Stiff A et al. found that MDSCs also create NO to inhibit NK cell FcR-mediated activity, which lowers the effectiveness of mAb treatment and hinders anti-tumor immunity (55).
The third mechanism is the one that inhibits lymphocyte migration (Figure 2C). In peripheral lymphoid organs, MDSCs reduce immunological response and aggregate in sentinel LNs, where they block CD3/CD28-induced T cell reproduction in a contact-dependent way. And this facilitates tumor development and metastasis (50, 55). Hanson EM et al. discovered that by downregulating L-selectin expression on the surface of CD4+ and CD8+ T cells via plasma membrane production of ADAM17 (a disintegrin and metalloproteinase domain 17), MDSCs in BC restrict the activation and entrance of naive T cells into LNs and the transport to tumors, ultimately suppressing anti-tumor immunity (56).
The fourth is the expansion mechanism and activation of Tregs. MDSCs promote the expansion and transformation of naive CD4+ T cells into Tregs (Figure 2D). However, the mechanism is not entirely comprehended. Tumor-infiltrating Tregs are important factors in antitumor immunosuppression. Inducible interleukin-34 (IL-34) causes the conversion of bone marrow stem cells (BMSCs) into M-MDSCs, which indirectly attenuates the immune response by producing CCL22-recruiting Tregs in TME, causing chemoresistance (21). In addition, BC-induced MDSCs can induce effector T cells and convert them into Tregs through the IDO mechanism (52). It has been established that T cells and MDSCs have a retaliatory interaction, with MDSCs suppressing T cell activation while stimulated T cells mediating MDSC death via the Fas-FasL pathway (57).
MDSCs in BC can also act by suppressing other immune cells. MDSCs can act through contact-dependent mechanisms and also indirectly by secreting NO, ARG, and IL-1 to suppress anti-tumor B cell responses (58). MDSCs can convert normal B cells into immunomodulatory B cell (Bregs) subtypes that suppress T cell responses (59). Furthermore, the expression of programmed cell death protein 1 (PD-1) is substantially upregulated in MDSCs, and studies have implicated various mediators in TME, like LPS, in inducing PD-1 expression in MDSCs in BC (60). Via the PD-1 and programmed cell death ligand 1 (PD-L1) axis, MDSCs have been demonstrated to increase PD-1/PD-L1 Bregs-mediated immune evasion by activating the phosphatidylinositol 3-kinase (PI3K)/protein kinase B (AKT)/NF-κB signaling pathway in B cells (61). In addition, medroxyprogesterone acetate, a progesterone analog, promotes MDSCs in the 4T1 tumor model to inhibit NK cell degranulation and IFN-γ production by inducing IDO production (62). BC cells grown under hypoxia release kinds of cytokines such as monocyte chemotactic protein-1, attract MDSCs and decrease the cytotoxic effects of NK cells, all of which foster the metastasis of cancer (63). Nevertheless, NKT cells may rescue suppressed T cells by transforming CD11b+ HLA-DR− MDSCs into CD11blow HLA-DR DCs through an NKG2D-dependent signaling mechanism (47). C5aR signaling contributes to MDSCs function by regulating CD4+ T cells are polarized to Th2 type in the lungs of tumor-bearing mice (42). In the lungs of tumor-bearing mice, treatment with DOX was shown to increase miR-126 exosomes from MDSCs, which in turn contributed to MDSCs mediated inhibition of Th1 cell activation, suppression of T cell activity, and induction of Th2 cell responses (64).
3.2 Non-immune functions of MDSCs
3.2.1 Induction and maintenance of stemness in tumor cells
By generating and sustaining cancer stem cells(CSCs), MDSCs provide a non-immune role that promotes tumor development and metastasis. This process involves multiple mechanisms, with STAT3 and Notch playing a significant role (Figure 3A). It has been demonstrated that MDSCs-derived IL-6 initiates STAT3 phosphorylation and that MDSCs-derived NO activates NOTCH, which then cooperates with IL-6 to cause sustained STAT3 activation (65). This suggests that MDSCs, via interacting with IL-6/STAT3 and NO/Notch, could contribute to activating and sustaining the pool of CSCs (65). In addition, the Janus kinase 1 (JAK1)-STAT3-SOX2 pathway was also discovered to be involved in the effects of IL-20RA on BC cell stemness and the promotion of more MDSCs (19). Sprouse ML et al. found that through activating the nuclear factor E2-related factor 2 (NRF2)-antioxidant response element axis, G-MDSCs increase ROS generation, which in turn increases Notch1 receptor expression in circulating tumor cells (CTCs). Direct cell contact between Jagged1-expressing G-MDSCs and Notch1 receptors on CTCs promotes Nodal secretion in Notch-activated CTCs. Nodal induces pro-tumor differentiation of G-MDSCs by binding to Cripto receptors abundantly expressed in G-MDSCs, thereby promoting CTCs survival and proliferation and BC metastasis (66). Furthermore, there is experimental evidence that MDSCs express pro-metastatic factors, including chitinase 3-like 1 and matrix metalloproteinase 9 (MMP9) in a ΔNp63-dependent manner to promote CSCs function in TNBC and facilitate TNBC metastasis (37).
3.2.2 Epithelial-mesenchymal transition
EMT is the process through which epithelial cells shed their adherent phenotypes and take on more mesenchymal features increasing cell mobility. This process has been demonstrated to be essential in tumor progression, from initiation to metastasis. MDSCs can facilitate this process (Figure 3B). It has been demonstrated that surgical stress shortens the OS of mice and raises the number of MDSCs in the TME, which induce EMT in tumor cells and increase BC metastasis via upregulating TGF-β1, VEGF, and IL-10 (67). Furthermore, through activating the PI3K-AKT-mTOR pathway, MDSCs promote EMT and boost the production of matrix MMPs in cancer cells, which may boost the invasive and metastatic potential of BC cells (68). M-MDSC from 4T1 tumor-bearing mice with induced tumor site infiltration has been reported to promote tumor metastasis by inducing nitric oxide synthase 2 (NOS2) production and activating STAT1 and STAT3 signaling pathways in tumor cells to induce EMT and CSCs phenotypes (69). Moreover, in an in vitro experiment with dog BC cells, MDSCs-secreted IL-28 stimulated STAT3 in tumor cells, inducing EMT and promoting tumor cell migration (70).
In contrast, G-MDSCs from the lung inhibited the EMT and CSCs phenotypes and promoted tumor cell proliferation (69). In a mouse model of spontaneous BC, the investigators found that MDSCs were recruited to the pre-metastatic lung. These MDSCs promoted mesenchymal-epithelial transformation (MET) of metastatic tumor cells within the lung metastases by expressing versican, which blocked TGF-β-Smad2 mother against decapentaplegic 2 (Smad2) pathway-mediated EMT, and in turn, promoted the growth of tumor lesions at the metastatic sites (71).
3.2.3 Angiogenesis
Through stimulating blood vessel growth, MDSCs help contribute to tumor growth, persistence, and dissemination (Figure 3C). CXCL17 released by BC cells upregulates MDSCs, which in turn secretes platelet-derived growth factor-BB and promotes the progression and metastasis of BC by establishing angiogenesis in the lung microenvironment (72). Similarly, by secreting IL-28, MDSCs promote tumor cell STAT3 activation and VEGF upregulation, leading to endothelial cell-induced angiogenesis (70). Other studies in mice with revealed that MDSCs produced a large amount of MMP9 (73) and reduced the production of platelet factor 4 (74), resulting in increased permeability and abnormal barrier function of newly generated vessels, which facilitated adhesion of peripheral CTCs to the vessel wall and leakage from the vessel to the metastases.
4 Clinical significance of MDSCs in breast cancer
4.1 Staging and prognosis
Higher levels of MDSCs in BC patients are associated with poor clinical outcomes. META analysis showed a significant association between high levels of MDSCs and poorer estimated OS in BC (6).. MDSCs levels can fluctuate following treatment. Experiments on mice with surgically excised primary cancer demonstrate that surgical stress promotes MDSCs recruitment into lung and tumor tissue (67). Moreover, neoadjuvant chemotherapy (NAT) influences the MDSCs count in TME (74, 75). In women with operable BC treated with NAT, circulating G-MDSCs increased during DOX and cyclophosphamide chemotherapy, declined sharply during paclitaxel, and returned to levels near baseline by the end of chemotherapy (Figure 4A). Levels of M-MDSCs were significantly lower, accounting for 1% or less of peripheral blood mononuclear cells, and did not differ significantly over time (76). Similar results were obtained in an experiment in 2022 (75). In a study of BC patients presenting with metastasis or recurrence, M-MDSCs have been observed to greatly expand in the peripheral circulation and associated with greater metastases to LNs and other organs, according to experiments that specifically targeted M-MDSCs. Patients with high levels of M-MDSCs suffer from a more severe condition and an inferior prognosis (77). Tracking M-MDSCs levels in patients with BC could serve as a fascinating and straightforward biomarker for monitoring cancer progression.
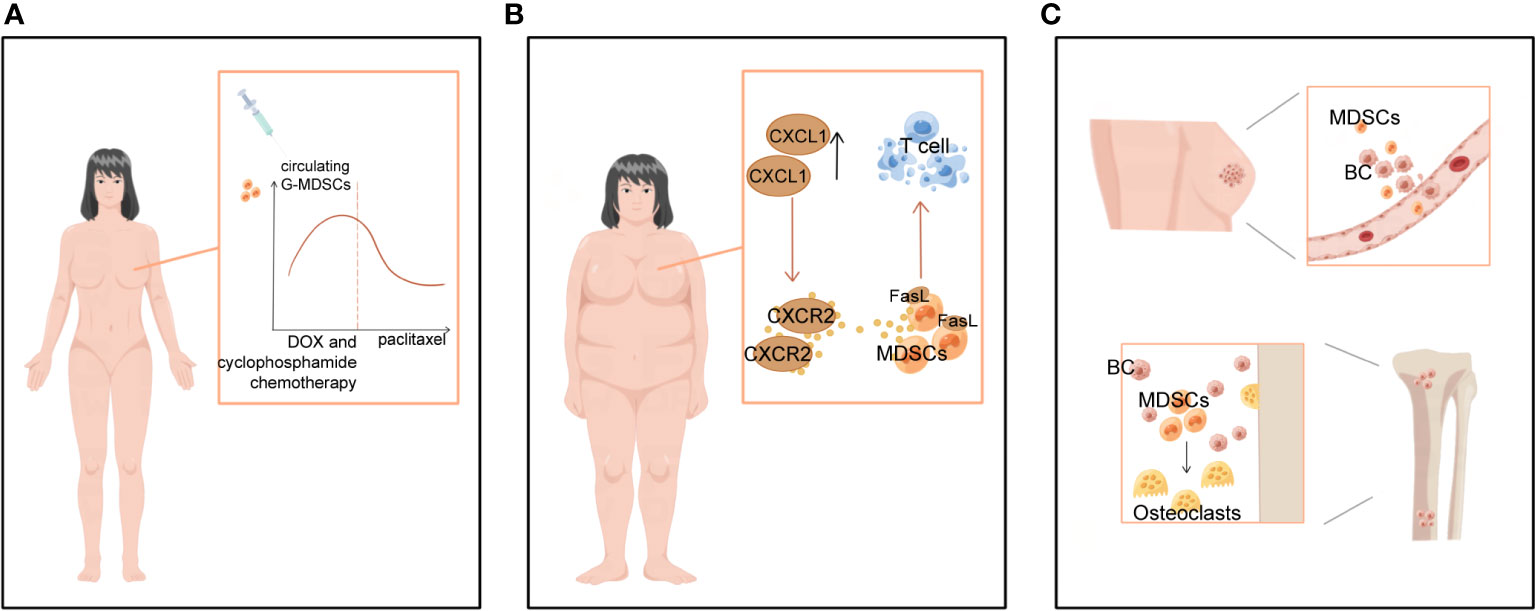
Figure 4 Clinical significance of MDSCs in BC-related staging and prognosis, obesity and bone metastases. (A) MDSCs levels varied with treatment progression. In women with operable breast cancer treated with NAT, circulating G-MDSCs increased during DOX and cyclophosphamide chemotherapy, decreased sharply during paclitaxel chemotherapy, and reached near baseline levels at the end of chemotherapy. (B) BC-related high-risk factors for obesity contribute to more MDSCs. (C) MDSCs not only promote breast cancer bone metastasis, but also differentiate into osteoclasts and cause bone destruction.
4.2 MDSCs and breast cancer-related high-risk factors: obesity
Obesity has been linked to an increased incidence of BC, especially in postmenopausal women, and a poorer prognosis of BC disease in all age groups of women patients (78). Obesity and a high-fat diet substantially boost the number of MDSCs in TME, thus further promoting tumor progression (78, 79). Obesity has been reported to increase CXCL1 concentrations in the BC microenvironment, contributing to CXCR2-mediated chemotaxis and the accumulation of G-MDSCs expressing the Fas ligand (FasL). In vitro and in vivo studies revealed that G-MDSCs cause immunotherapy tolerance by inducing Fas/FasL-mediated apoptosis of T cells (80) (Figure 4B). In two mouse models of TNBC, 4T1 and Py8119, glycolytic restriction suppresses G-CSF and GM-CSF expression and decreases MDSCs (81). Furthermore, exercise has been shown to slow the tumors from developing by inhibiting the collection of MDSCs (80–82). This indicates that a healthy diet, weight management, and increased physical activity can also prevent BC by preventing an increase in MDSCs.
4.3 Bone metastases and bone destruction in breast cancer
Bone is a significant target for BC metastases, and bone metastases are also a major cause of BC death, usually manifesting as excessive osteoclast-mediated osteolysis, causing fractures and pain in patients (83). During this process, MDSCs suppress the immune system and induce osteolysis in patients with BC by developing into osteoclasts (83, 84) (Figure 4C). In particular, MDSCs could only differentiate into osteoclasts under the local microenvironmental conditions of BC bone metastases. In contrast, MDSCs isolated from mice without bone metastases did not undergo such differentiation (83, 85). Studies have revealed that MDSCs cause osteolysis in BC bone metastases by acting as BC osteoclast progenitors and maturing into viable osteoclasts through a NO-dependent mechanism (84, 86). Kun Z et al. found that the tumor-derived integrin ligand epidermal growth factor-like repeat and disc-like structural domain 3 (EDIL3) suppresses tumor-induced MDSCs differentiation into osteoblasts in vitro and lowers tumor-induced MDSCs proliferation in vivo (86). Thus, treatments that aim specifically at MDSCs may be able to diminish not only their immunosuppressive activity but also MDSCs-mediated osteolysis and the management of BC sequelae.
5 MDSCs as a target for breast cancer treatment
As mentioned previously, MDSCs play an irreplaceable role in the progression of BC. Therefore, MDSCs have been identified as a target for tumor immunotherapy. Clinical trials of targeted MDSCs related to BC are summarized in (Supplementary Table 1).
Currently, targeting MDSCs includes four main approaches (Supplementary Table 2): (1) depletion of MDSCs; (2) blocking the recruitment of MDSCs; (3) suppressing the immunosuppressive function of MDSCs; (4) differentiation of MDSCs to a non-suppressive immune state.
5.1 Depletion of MDSCs
First strategy for targeting MDSCs: removal of circulating and tumor-infiltrating MDSCs. It has been demonstrated that low-dose chemotherapy can eradicate MDSCs populations in tumor-bearing rodents; chemotherapies such as gemcitabine, 5-fluorouracil (5-FU) (87), Docetaxel (88) and paclitaxel (89) deplete MDSCs and enhance anti - tumor immune function. However, gemcitabine and 5FU activate the thermal protein structural domain of the NOD-like receptor family containing the 3-protein-dependent inflammasome complex in MDSCs, resulting in the generation of IL-1β. MDSCs-derived IL-1β further stimulates CD4+T cell IL-17 production and impairs their anti-tumor activity (87).
Inhibition of VEGF receptor signaling resulted in reduced infiltration of MDSCs. R84 is an anti-VEGF suppressant that blocks the creation of cytokines and chemokines in tumors, especially CXCL1, IL-6 and IL-1β (90). R84 specifically suppresses VEGF binding to VEGFR2, which leads to efficient control of tumorigenesis and suppression of suppressive immune cell (MDSCs, Tregs, macrophages) infiltration while boosting the number of mature DCs (90). Anti-angiogenic treatment with the hypoxia-inducible factor-1 (HIF-1) dimerization inhibitor acridine flavin in combination with sunitinib reduced MDSCs accumulation in the spleen, significantly reduced the production of VEGF and TGF-β and retarded the growth of tumors in a mouse model (91). Based on the preceding, we hypothesized that the number of MDSCs could be effectively reduced by targeting VEGF.
Other methods have also been demonstrated to decrease MDSCs. DKN-01 is an IgG4 antibody that specifically neutralizes human and mouse DKK1, a secreted Wnt signaling regulator, and causes a reduction in MDSCs in tumors and spleens and upregulation of PD-L1 on MDSCs in a mice BC model (92). Zonneville et al. found that pharmacological p38 inhibitors (p38i) substantially reduce MDSCs within the TME in TNBC, while depleting MDSCs and simultaneously reducing the expression of chemokines derived from tumors and stroma that are thought to contribute to the recruitment of pro-tumor bone marrow populations (93). Moreover, type I interferon reduces MDSCs in both bone marrow and blood and dramatically reduces bone metastases and metastasis-free survival (94). In addition, a pharmacotherapy liver X nuclear receptor (LXR) agonism decreased the number of MDSCs in a mouse model and in the first human dose-escalation phase 1 trial (95). It has been found that MDSCs have elevated levels of TNF-related apoptosis-inducing ligand receptors (TRAIL-Rs); thus, DS-8273a, a new agonistic TRAIL-R antibody, can induce MDSCs apoptosis and decrease the quantity of MDSCs in the peripheral circulation of BC patients (NCT02076451) (96). In addition, a unique strategy of using spherical nucleic acids encapsulated with antigens encapsulated in lysates containing TNBC cells reduced the frequency of MDSCs in TME, significantly inhibited tumor growth, and prolonged life span (97).
In addition, a number of extensively utilized clinical treatments have been proven to decrease MDSCs. A clinical study showed that resection of the primary tumor in metastatic BC reduced the frequency of MDSCs and improved patient prognosis (98). However, another study showed that resection of the primary tumor in 4T1-bearing mice certainly reduced the number of MDSCs, but did not affect the eventual progression of metastatic BC (99). Furthermore, Habibi M et al. demonstrated that intratumoral injection of IL-7 and IL-15 into mammary carcinoma-bearing mice after radiofrequency thermal ablation reduces MDSCs, induces an immune response to the tumor, and inhibits tumor progression and lung metastasis (100). It has been proven that NKT cell activation therapy could reduce the number and inhibitory activity of MDSCs. However, NKT cell activation in conjunction with either gemcitabine or cyclophosphamide did not further reduce the number of MDSCs (101).
5.2 Blocking MDSCs recruitment
The second strategy for targeting MDSCs: blocking the recruitment of MDSCs. As mentioned above, the production and recruitment of MDSCs is regulated by multiple pathways, and targeting and blocking some of these pathways, such as chemokines and CSF, will achieve the goal.
5.2.1 Chemokine
Chemokines serve an essential role in MDSCs recruitment, and a variety of drugs targeting chemokines are currently under investigation. Therefore, MDSCs accumulation can be diminished by inhibiting cytokine or chemokine receptors on MDSCs. In a mouse model of BC, the poly (ADP-ribose) polymerase inhibitor (PARPi) olaparib was shown to inhibit MDSCs migration via the SDF1a/CXCR4 axis (102). In addition, MDSCs express large amounts of CXCR2. ΔNp63 promotes MDSCs recruitment to primary and metastatic sites through transcriptional activation of chemokines, such as CXCL2 and CCL2. The use of inhibitors of CXCR2 (SB 225002) or CCR4 inhibitors (Tocris) or the reduction of transcription factor ΔNp63 levels greatly reduces MDSCs recruitment and the angiogenesis and metastasis it causes (37). Another study demonstrated thatlow dosages of entinostat (ENT) and 5-azacytidine decreased the recruitment of MDSCs by downregulating CCR2 and CXCR2, correspondingly, and extended the OS of mice following surgical excision of the primary tumor (103). Similarly, Silibinin, a natural flavonoid from Sylibum marianum seeds, can down-regulate tumor-specific homing of MDSCs by decreasing CCR2 expression on MDSCs (104). Moreover, neutralization of CXCL1 with antibodies was observed to prevent the recruitment of senescent lung fibroblast-mediated G-MDSCs in the MMTV-PyVT recombinant mice (32). Indeed, by targeting the CCR5-CCL5 interaction, the CCR5 antagonist Maraviroc reduced lung metastases in a preclinical mouse model of BC (105). Moreover, AMD3100 is an antagonist for the CXCR4 receptor that diminishes the impact of SDF-1α on MDSCs recruitment, whereas gemcitabine is a drug that eliminates MDSCs directly. The accumulation of MDSCs in the TME is promoted by estrogen-induced production of SDF-1α, an effect which can be inhibited by AMD3100 or gemcitabinem (106)..
Notably, Chinese medicine can also block MDSCs recruitment through various mechanisms. The XIAOPI formula led to a substantial reduction in the quantity of MDSCs in lung tissue by reducing CXCL1 expression in a mouse model of BC (107). Baoyuan Jiedu decoction is a traditional Chinese medicine that inhibits MDSCs recruitment in pre-metastatic niches in the lung via the TGF-β/CCL9 pathway in BC (108).
5.2.2 CSF
CSF-1R is another major target to inhibit MDSCs recruitment to tumor sites to constrain tumorigenesis. Mice with p53N-C tumors had fewer MDSCs after treatment with the mTOR inhibitor rapamycin. Mechanistically, the accumulation of MDSCs is mediated by G-CSF, a downstream target gene of the mTOR pathway. Blocking G-CSF therefore may inhibit MDSCs accumulation (30). In addition, BMP4, a constituent of the TGF-β family, inhibits NF-κB function in human and mouse BC to reduce G-CSF expression (109). Kumar V et al. found that both TAMs and G-MDSCs were diminished at the tumor site by the combination of CSF1R and CXCR2 inhibitors. In contrast to the absence of antitumor activity exhibited by each inhibitor alone, combination therapy drastically decreased tumor growth (110). However, depleting MDSCs with anti-Gr1 or anti-G-CSF antibodies reduced the growth and proliferation of IL-17A-expressing 4T1 tumors but had no impact on control 4T1 tumors (111).
5.2.3 Interleukin
The interleukin family also plays a crucial role in MDSCs recruitment. In TNBC, Bo Yu et al. proved that IL-6 knockdown inhibited SMAD3 K20/117Q -induced MDSCs recruitment (41). In addition, Anakinra, an IL-1 receptor antagonist, was found to reduce MDSCs and M2 macrophage recruitment in a mouse model of mammary tumors with PI, but had no significant effect on M1 macrophages (43). Dominguez C et al. proved that when combined with docetaxel, a clinical-stage mAb that neutralizes the chemokine IL-8 (HuMax-IL-8) was shown to reduce the recruitment of G-MDSCs at tumor sites (112). Curcumin, a substance with IL-6 inhibiting function, reduces the production of IL-6 in BC, thus reducing the number of MDSCs (113).
5.2.4 Others
In addition to the drugs mentioned above, a variety of drugs can inhibit MDSCs recruitment. Leukadherin-1, a allosteric regulatory agent of CD11b, leads to reduced tumor CD11b+ MDSCs (114). In addition, AZD4547, a small molecule inhibitor of tyrosine kinases targeting fibroblast growth factor receptors (FGFRs), reduces MDSCs accumulation in carcinoma and lung tissue and suppresses tumor development and lung metastasis (115). The Apelin/Apelin receptor signaling pathway, which is independent from VEGFR signaling pathway, is associated with developmental angiogenesis. It was found that genetic and pharmacological inhibition of Apelin led to a massive decrease in G-MDSCs in tumors, remodeling of TME, reduction of angiogenesis, and effective inhibition of tumor growth (116). Moreover, MIF inhibitor sulforaphane also inhibits MDSCs formation (22). F1 antibody to aspartate protease cathepsin D (cath-D) blocks recruitment of immunosuppressive tumor-associated macrophages M2 and MDSCs and inhibits tumor growth in two TNBC xenografts (117). In a mouse model of BC, PI-3065, an inhibitor of phosphatidylinositol 3-kinase δ (PI3Kδ) signaling enzyme, was able to indirectly inhibit MDSCs recruitment in the TME (118). Atovaquone, an antiprotozoal medication that used treat malaria, decreases the production of ribosomal protein S19 (RPS19) in tumors, thereby decreasing the number of MDSCs and Tregs (119). Markiewski MM et al. discovered that C5aR1 inhibitor (C5aRA) therapy reduced tumor-infiltrating MDSCs and peripheral blood MDSCs in FVB/N Her2/neu transgenic mice (120). Analysis revealed that inhibiting C3a signaling in conjunction with DOX therapy substantially reduced G-MDSCs and eosinophils recruitment, while reducing M-MDSCs recruitment (121).
Above all, the recruitment of MDSCs can be inhibited by a wide range of medications, according to an extensive corpus of research on BC. This indicates that research aimed at inhibiting the recruitment of MDSCs is promising. However, most are still in the experimental phase, and their clinical efficacy remains unclear.
5.3 Suppressing the immunosuppressive function of MDSCs
The third strategy for targeting MDSCs: inhibition of the immunosuppressive function of MDSCs. Studies on the suppression of MDSCs activity are plentiful in malignant melanoma and ovarian cancers, but few in BC (122). DC101, an anti-VEGFR-2 antibody, partially diminishes the suppressive effect of M-MDSCs on T-cell propagation and decreases the number of Tregs in both the primary tumor and lung metastases. Remarkably, ARG-1 can be induced by treatment with DC101. The ARG inhibitor Nω-hydroxy-nor-Arginine (Nor-NOHA) reduced the inhibition activity of MDSCs on T-cell proliferation and diminished the number and extent of lung metastases, but the combination with DC101 had little additional effect (123). Chaib M et al. proved that protein kinase C (PKC) agonists inhibited the expansion of MDSCs in HPCs and stimulated the differentiation of M-MDSCs into an APC-like phenotype expressing CDC1-related markers by activating the p38 mitogen-activated protein kinase (MAPK) pathway. Functionally, PKC agonists diminished the inhibitory activity of MDSCs and increased their cross-stimulatory capacity (124).
5.4 Differentiation of MDSCs to a non-suppressive immune state
A fourth strategy for targeting MDSCs: promoting the differentiation of MDSCs into non-immunosuppressive cells. Effective concentrations of all-trans retinoic acid (ATRA) can eliminate IMCs by neutralizing high ROS production in cells, and induce the differentiation of MDSCs (125). In BC, blocking MDSC expansion with ATRA improves the efficacy of anti-angiogenic therapy (126). Mechanistically, ATRA significantly and specifically upregulates glutathione synthase (GSS) and accumulates glutathione (GSH) in MDSCs, thereby neutralizing the high ROS production in these cells to induce MDSCs differentiation (125). In addition, the application of other chemotherapeutic agents also promotes the differentiation of MDSCs into new cell types that lack immunosuppressive functions. For example, paclitaxel promotes the differentiation of MDSCs into DCs in vitro (89); docetaxel inhibits STAT3 phosphorylation and differentiates MDSCs to M1-type macrophages (88).
In addition, various studies have been conducted to promote MDSCs differentiation in BC. Inhibiting the differentiation of M-MDSCs by utilizing ER inhibitors or anti-IL-34 monoclonal antibodies can suppress tumor growth by inhibiting M-MDSCs accumulation and restore tumor chemical sensitivity by promoting G-MDSCs accumulation (21). Svoronos N et al. found that the Jak1/2 inhibitor Ruxolitinib significantly inhibited M-MDSCs differentiation and G-MDSCs expansion, and these inhibitory effects were significantly enhanced by concomitant use of the ERα antagonist methylpiperidino pyrazole (MPP) (127). Moreover, serine/threonine protein kinase CK2 inhibitors, which cause differentiation arrest, significantly reduced the number of G-MDSCs and TAMs (128). Cyclic di-Guanosine (c-di-GMP) is a ligand for interferon gene stimulator (STING). Chandra D et al. demonstrated that low concentrations of c-di-GMP can promote MDSCs maturation to eliminate MDSCs. The antitumor activity of CD8+ T cells is enhanced by a Listeria monocytogenes (LM) vaccine expressing the tumor-associated antigen Mage-b (LM-Mb). The combination of LM-Mb and c-di-GMP reduces the effect of MDSCs on CD8+ cells and enhances anti-tumor immunotherapy further (129).
Reprogramming the epigenome is an innovative strategy for targeting tumor-promoting properties of MDSCs. In combination with anti-PD-1, anti-CTLA-4, or both, the histone deacetylase inhibitor ENT significantly reduced the suppressive effect of G-MDSCs in the TME, increased CD8+ T-effector cells, and ultimately improved tumor-free survival in a mouse model of HER2/neu transgenic BC (130). However, the clinical trial of ENCORE 602 (NCT02708680), which included ENT for TNBC, failed to increase progression-free survival.
As mentioned previously, MDSCs have been shown to be associated with bone metastasis from BC. It has been demonstrated that MDSCs isolated from the tumor-bone microenvironment differentiate into functional osteoclasts both in vitro and in vivo, with NO signaling as a key regulatory pathway. NG-monomethyl-L-arginine acetate (L-NMMA), an inducible NO synthase (iNOS) inhibitor, inhibited NO signaling and blocked MDSCs differentiation into osteoclasts (85). Interestingly, bisphosphonates, a drug that has been used in BC bone metastases, were discovered to decrease to reduce the number of bone marrow and peripheral blood MDSCs (131). Additionally, the combination of anti-Gr1-mediated depletion of G-MDSCs and zoledronic acid (ZA)-induced osteoclast blockade inhibited the development of established skeletal metastases (132). Therefore, targeted inhibition of MDSCs can not only slow down the course of the primary tumor, but also prevent or delay BC bone metastases simultaneously. Thus, further appropriate clinical studies to back up this conclusion are much anticipated.
5.5 Novel drugs
A novel therapy targeting MDSCs based on traditional drugs is currently under development. The new therapy has various advantages, such as more efficient drug delivery and lower incidence of adverse reactions. The relevant new therapies are summarized in Table 2.
5.6 Targeted MDSCs in combination with other antitumor therapies
5.6.1 Immunotherapy
Immunotherapy is playing an increasingly important role in cancer treatment. And MDSCs are the essential immunosuppressive cells. Therefore, targeting MDSCs in combination with immunotherapy shows great potential.
Gene-engineered tumor cell derived exosome-like vaccine (eNVs-FAP) developed by Hu S et al. inhibits tumor progression by reducing the number of MDSCs, reprogramming TME and promoting tumor ferrocytosis (142). Another study has shown that a vaccine against fibroblast growth factor (FGF)-2 also reduced the number of MDSCs in mice bearing 4T1 mammary tumors (143). However, an epitope gene vaccine against fibroblast activating protein (FAP) α enhanced the antitumor immune response in BC models, but no improvement in antitumor effects was observed, which may be related to the vaccine-induced elevation of MDSCs (144). Moreover, the development of monoclonal antibodies targeting MUC1 has been reported to counteract the oncogenic effects of MUC1, eliminating MDSCs in TME and ultimately slowing the progression of BC (145). Researches along these lines have a promising future as a tumor vaccine.
Combining tumor vaccines with other therapies has also yielded positive results in research. A triple therapy combines T-cell inducible vaccine with PD-1 antagonist and CD40 agonist mAb leads to a reduction in tumor-infiltrating G-MDSCs and Tregs, further promoting an active CTL response (146). Geng F et al. developed a tumor vaccine targeting both the tumor stromal antigen FAPα and the tumor cell antigen Survivin in BC, which can effectively inhibit tumor progression, and the combination with low-dose DOX to clear induced peripheral MDSCs can further promote the anti-tumor activity of this vaccine (147). Similar results were obtained in another study combining a liposomal vaccine containing E75 (a HER-2/neu-derived peptide) with liposomal DOX (148). Tumor vaccines in combination with chemotherapy, notably DOX, to eliminate the impact of MDSCs on efficacy is, therefore, bright and promising for clinical translation.
5.6.2 RT in combination with other treatments
RT is presently one of the primary cancer therapeutic approaches. RT activates local anti-tumor immunity and non-irradiated, distant site anti-tumor immunity (distant compartment response) (149). Immunosuppressive cell types, such as Tregs, M2-like TAMs, and MDSCs, may be efficiently recruited and expanded by RT.
It is widely established that MDSCs express PD-L1, and RT has been shown to elevate PD-L1 expression in MDSCs. Together, RT and anti-PD-L1 treatment were able to diminish MDSCs accumulation (150). In one trial, triple therapy with RT, PD-1 blockade and PI3Kαδ inhibitors slowed tumor growth by reducing G-MDSCs and increasing cytotoxic CD8+ T cells, converting TME to PD-1 blockade or other conditions favourable to ICIs (151). In addition, group V immunomodulatory receptor (VSIR, most widely known as VISTA) is a co-inhibitory receptor, and VISTA blockade depleted MDSCs in 4T1 tumor TME when used with RT, whereas VISTA blockade alone had no such effect. Furthermore, cyclophosphamide plus RT and dual PD-1/VISTA blockade had a superior therapeutic effect. This was connected with the activation of tumor-infiltrating CD8+ T cells as well as the reduction of G-MDSCs inside the tumor (152).
Studies have been conducted to explore the effects of RT in combination with a variety of other treatments, but more trials are still required. Ruiz-Fernández de Córdoba B et al. found that using intratumoral α-irradiation ablation in the presence of immunosuppression and CpG inhibitors inhibited the development of mammary carcinoma in mice (153). In an immunocompetent model, leukadherin-1 therapy resulted in a decrease in tumor MDSCs and demonstrated improved antitumor capacity in combination with RT or paclitaxel (114). Moreover, a tyrosine kinase inhibitor, cabozantinib, was found to reduce the number of MDSCs. However, in a preclinical 4T1 BC model, the combination of cabozantinib and RT did not benefit tumor growth control (154).
6 Conclusions
These proofs support the theory that the presence of MDSCs is increased in BC patients and that they have a vital position in the immunological resistance phenotype of the disease. Due to the heterogeneity of MDSCs, it is necessary to develop assays that can more accurately characterize subpopulations of MDSCs in BC patients and to measure MDSCs in peripheral circulation and TME at different stages of BC progression in different subtypes to better understand their production, amplification, and function in peripheral blood and TME, and to observe the relationship between MDSCs and BC stage progression. This review will be utilized to support the practical application of research findings and serve as a foundation for the diagnosis and treatment of targeted MDSCs. Through a complex mechanism, MDSCs in BC promote tumor progression and metastasis. Not only do they exert immunosuppressive effects, weakening anti-tumor immunity to promote BC growth and metastasis, but they also diminish the efficacy of other therapeutic approaches. Diverse MDSCs-targeted therapies (as immunotherapy or in combination with conventional therapies, including chemotherapy and RT) are presently being evaluated for their efficacy in BC preclinically in order to maximize its anti-tumor effects. We believe that an improved understanding of the clinical relevance of MDSCs will encourage the research and development of MDSCs-targeted therapies that will enhance the prognosis of BC patients.
Author contributions
HL, ZW, YZ and YY all contributed to the writing and editing of the manuscript. All authors contributed to the article and approved the submitted version.
Funding
This work was supported by Science and technology Development Plan of Jilin (20210402026GH); Key Laboratory of Metrology and calibration technology (JLKG2021001C004).
Conflict of interest
The authors declare that the research was conducted in the absence of any commercial or financial relationships that could be construed as a potential conflict of interest.
Publisher’s note
All claims expressed in this article are solely those of the authors and do not necessarily represent those of their affiliated organizations, or those of the publisher, the editors and the reviewers. Any product that may be evaluated in this article, or claim that may be made by its manufacturer, is not guaranteed or endorsed by the publisher.
Supplementary material
The Supplementary Material for this article can be found online at: https://www.frontiersin.org/articles/10.3389/fimmu.2023.1199273/full#supplementary-material
References
1. Wild CP, Weiderpass E, Stewart BW. World cancer report: cancer research for cancer prevention . Available at: https://publications.iarc.fr/Non-Series-Publications/World-Cancer-Reports/World-Cancer-Report-Cancer-Research-For-Cancer-Prevention-2020.
2. Gradishar WJ, Moran MS, Abraham J, Aft R, Agnese D, Allison KH, et al. Breast cancer, version 3.2022, NCCN clinical practice guidelines in oncology. J Natl Compr Canc Netw (2022) 20(6):691–722. doi: 10.6004/jnccn.2022.0030
3. Salemme V, Centonze G, Cavallo F, Defilippi P, Conti L. The crosstalk between tumor cells and the immune microenvironment in breast cancer: implications for immunotherapy. Front Oncol (2021) 11:610303. doi: 10.3389/fonc.2021.610303
4. Cha YJ, Koo JS. Role of tumor-associated myeloid cells in breast cancer. Cells (2020) 9(8):1785. doi: 10.3390/cells9081785
5. Li K, Shi H, Zhang B, Ou X, Ma Q, Chen Y, et al. Myeloid-derived suppressor cells as immunosuppressive regulators and therapeutic targets in cancer. Signal Transduct Target Ther (2021) 6:362. doi: 10.1038/s41392-021-00670-9
6. Wang PF, Song SY, Wang TJ, Ji WJ, Li SW, Liu N, et al. Prognostic role of pretreatment circulating MDSCs in patients with solid malignancies: a meta-analysis of 40 studies. Oncoimmunology (2018) 7(10):e1494113. doi: 10.1080/2162402X.2018.1494113
7. Bronte V, Brandau S, Chen SH, Colombo MP, Frey AB, Greten TF, et al. Recommendations for myeloid-derived suppressor cell nomenclature and characterization standards. Nat Commun (2016) 7(1):12150. doi: 10.1038/ncomms12150
8. Cassetta L, Baekkevold ES, Brandau S, Bujko A, Cassatella MA, Dorhoi A, et al. Deciphering myeloid-derived suppressor cells: isolation and markers in humans, mice and non-human primates. Cancer Immunol Immunother (2019) 68(4):687–97. doi: 10.1007/s00262-019-02302-2
9. Alshetaiwi H, Pervolarakis N, McIntyre LL, Ma D, Nguyen Q, Rath JA, et al. Defining the emergence of myeloid-derived suppressor cells in breast cancer using single-cell transcriptomics. Sci Immunol (2020) 5(44):eaay6017. doi: 10.1126/sciimmunol.aay6017
10. Condamine T, Mastio J, Gabrilovich DI. Transcriptional regulation of myeloid-derived suppressor cells. J Leukoc Biol (2015) 98(6):913–22. doi: 10.1189/jlb.4RI0515-204R
11. Millrud CR, Bergenfelz C, Leandersson K. On the origin of myeloid-derived suppressor cells. Oncotarget (2016) 8(2):3649–65. doi: 10.18632/oncotarget.12278
12. Sun HW, Wu WC, Chen HT, Xu YT, Yang YY, Chen J, et al. Glutamine deprivation promotes the generation and mobilization of MDSCs by enhancing expression of G-CSF and GM-CSF. Front Immunol (2021) 11:616367. doi: 10.3389/fimmu.2020.616367
13. Rong Y, Yuan CH, Qu Z, Zhou H, Guan Q, Yang N, et al. Doxorubicin resistant cancer cells activate myeloid-derived suppressor cells by releasing PGE2. Sci Rep (2016) 6(1):1–11. doi: 10.1038/srep23824
14. Ching MM, Reader J, Fulton AM. Eicosanoids in cancer: prostaglandin E2 receptor 4 in cancer therapeutics and immunotherapy. Front Pharmacol (2020) 11:819. doi: 10.3389/fphar.2020.00819
15. Pradhan AK, Maji S, Bhoopathi P, Talukdar S, Mannangatti P, Guo C, et al. Pharmacological inhibition of MDA-9/Syntenin blocks breast cancer metastasis through suppression of IL-1β. Proc Natl Acad Sci U S A (2021) 118(21):e2103180118. doi: 10.1073/pnas.2103180118
16. Jiang M, Chen J, Zhang W, Zhang R, Ye Y, Liu P, et al. Interleukin-6 trans-signaling pathway promotes immunosuppressive myeloid-derived suppressor cells via suppression of suppressor of cytokine signaling 3 in breast cancer. Front Immunol (2017) 8:1840. doi: 10.3389/fimmu.2017.01840
17. Zhao N, Zhu W, Wang J, Liu W, Kang L, Yu R, et al. Group 2 innate lymphoid cells promote TNBC lung metastasis via the IL-13-MDSC axis in a murine tumor model. Int Immunopharmacol (2021) 99:107924. doi: 10.1016/j.intimp.2021.107924
18. Welte T, Zhang XHF. Interleukin-17 could promote breast cancer progression at several stages of the disease. Mediators Inflamm (2015) 2015:804347. doi: 10.1155/2015/804347
19. Gao W, Wen H, Liang L, Dong X, Du R, Zhou W, et al. IL20RA signaling enhances stemness and promotes the formation of an immunosuppressive microenvironment in breast cancer. Theranostics (2021) 11(6):2564–80. doi: 10.7150/thno.45280
20. Xiao P, Wan X, Cui B, Liu Y, Qiu C, Rong J, et al. Interleukin 33 in tumor microenvironment is crucial for the accumulation and function of myeloid-derived suppressor cells. Oncoimmunology (2015) 5(1):e1063772. doi: 10.1080/2162402X.2015.1063772
21. Kajihara N, Kobayashi T, Otsuka R, Nio-Kobayashi J, Oshino T, Takahashi M, et al. Tumor-derived interleukin-34 creates an immunosuppressive and chemoresistant tumor microenvironment by modulating myeloid-derived suppressor cells in triple-negative breast cancer. Cancer Immunol Immunother (2022) 72:851–64. doi: 10.1007/s00262-022-03293-3
22. Simpson KD, Templeton DJ, Cross JV. Macrophage migration inhibitory factor promotes tumor growth and metastasis by inducing myeloid-derived suppressor cells in the tumor microenvironment. J Immunol Baltim Md (2012) 189(12):5533–40. doi: 10.4049/jimmunol.1201161
23. Liu Q, Chen Y, Li J, Xiao L, Zhang W, Zhao J, et al. Bone marrow cells are differentiated into MDSCs by BCC-ex through down-regulating the expression of CXCR4 and activating STAT3 signalling pathway. J Cell Mol Med (2021) 25(12):5497–510. doi: 10.1111/jcmm.16559
24. Mundy-Bosse BL, Thornton LM, Yang HC, Andersen BL, Carson WE. Psychological stress is associated with altered levels of myeloid-derived suppressor cells in breast cancer patients. Cell Immunol (2011) 270(1):80–7. doi: 10.1016/j.cellimm.2011.04.003
25. Wu L, Xu Y, Zhao H, Zhou Y, Chen Y, Yang S, et al. FcγRIIB potentiates differentiation of myeloid-derived suppressor cells to mediate tumor immunoescape. Theranostics (2022) 12(2):842–58. doi: 10.7150/thno.66575
26. Liao WC, Yen HR, Chen CH, Chu YH, Song YC, Tseng TJ, et al. CHPF promotes malignancy of breast cancer cells by modifying syndecan-4 and the tumor microenvironment. Am J Cancer Res (2021) 11(3):812–26.
27. Liu C, Qiang J, Deng Q, Xia J, Deng L, Zhou L, et al. ALDH1A1 activity in tumor-initiating cells remodels myeloid-derived suppressor cells to promote breast cancer progression. Cancer Res (2021) 81(23):5919–34. doi: 10.1158/0008-5472.CAN-21-1337
28. Jiang M, Zhang W, Zhang R, Liu P, Ye Y, Yu W, et al. Cancer exosome-derived miR-9 and miR-181a promote the development of early-stage MDSCs via interfering with SOCS3 and PIAS3 respectively in breast cancer. Oncogene (2020) 39(24):4681–94. doi: 10.1038/s41388-020-1322-4
29. Zhang W, Jiang M, Chen J, Zhang R, Ye Y, Liu P, et al. SOCS3 suppression promoted the recruitment of CD11b+Gr-1–F4/80–MHCII– early-stage myeloid-derived suppressor cells and accelerated interleukin-6-Related tumor invasion via affecting myeloid differentiation in breast cancer. Front Immunol (2018) 9:1699. doi: 10.3389/fimmu.2018.01699
30. Welte T, Kim IS, Tian L, Gao X, Wang H, Li J, et al. Oncogenic mTOR signalling recruits myeloid-derived suppressor cells to promote tumour initiation. Nat Cell Biol (2016) 18(6):632–44. doi: 10.1038/ncb3355
31. Ozga AJ, Chow MT, Luster AD. Chemokines and the immune response to cancer. Immunity (2021) 54(5):859–74. doi: 10.1016/j.immuni.2021.01.012
32. Huang YC, Hou MF, Tsai YM, Pan YC, Tsai PH, Lin YS, et al. Involvement of ACACA (acetyl-CoA carboxylase α) in the lung pre-metastatic niche formation in breast cancer by senescence phenotypic conversion in fibroblasts. Cell Oncol (2023) 46:643–60. doi: 10.1007/s13402-022-00767-5
33. Li F, Kitajima S, Kohno S, Yoshida A, Tange S, Sasaki S, et al. Retinoblastoma inactivation induces a protumoral microenvironment via enhanced CCL2 secretion. Cancer Res (2019) 79(15):3903–15. doi: 10.1158/0008-5472.CAN-18-3604
34. Gu P, Sun M, Li L, Yang Y, Jiang Z, Ge Y, et al. Breast tumor-derived exosomal MicroRNA-200b-3p promotes specific organ metastasis through regulating CCL2 expression in lung epithelial cells. Front Cell Dev Biol (2021) 9:657158. doi: 10.3389/fcell.2021.657158
35. Tanaka T, Kajiwara T, Torigoe T, Okamoto Y, Sato N, Tamura Y. Cancer-associated oxidoreductase ERO1-α drives the production of tumor-promoting myeloid-derived suppressor cells via oxidative protein folding. J Immunol (2015) 194(4):2004–10. doi: 10.4049/jimmunol.1402538
36. Liu Y, Lai L, Chen Q, Song Y, Xu S, Ma F, et al. MicroRNA-494 is required for the accumulation and functions of tumor-expanded myeloid-derived suppressor cells via targeting of PTEN. J Immunol (2012) 188(11):5500–10. doi: 10.4049/jimmunol.1103505
37. Kumar S, Wilkes DW, Samuel N, Blanco MA, Nayak A, Alicea-Torres K, et al. ΔNp63-driven recruitment of myeloid-derived suppressor cells promotes metastasis in triple-negative breast cancer. J Clin Invest (2018) 128(11):5095–109. doi: 10.1172/JCI99673
38. Vrakas CN, O’Sullivan RM, Evans SE, Ingram DA, Jones CB, Phuong T, et al. The measure of DAMPs and a role for S100A8 in recruiting suppressor cells in breast cancer lung metastasis. Immunol Invest (2015) 44(2):174–88. doi: 10.3109/08820139.2014.952818
39. Chen JY, Lai YS, Chu PY, Chan SH, Wang LH, Hung WC. Cancer-derived VEGF-c increases chemokine production in lymphatic endothelial cells to promote CXCR2-dependent cancer invasion and MDSC recruitment. Cancers (2019) 11(8):1120. doi: 10.3390/cancers11081120
40. Roberts LM, Perez MJ, Balogh KN, Mingledorff G, Cross JV, Munson JM. Myeloid derived suppressor cells migrate in response to flow and lymphatic endothelial cell interaction in the breast tumor microenvironment. Cancers (2022) 14(12):3008. doi: 10.3390/cancers14123008
41. Yu B, Luo F, Sun B, Liu W, Shi Q, Cheng S, et al. KAT6A acetylation of SMAD3 regulates myeloid-derived suppressor cell recruitment, metastasis, and immunotherapy in triple-negative breast cancer. Adv Sci (2021) 8(20):e2100014. doi: 10.1002/advs.202100014
42. Vadrevu SK, Chintala NK, Sharma SK, Sharma P, Cleveland C, Riediger L, et al. Complement c5a receptor facilitates cancer metastasis by altering T-cell responses in the metastatic niche. Cancer Res (2014) 74(13):3454–65. doi: 10.1158/0008-5472.CAN-14-0157
43. Cheng R, Billet S, Liu C, Haldar S, Choudhury D, Tripathi M, et al. Periodontal inflammation recruits distant metastatic breast cancer cells by increasing myeloid-derived suppressor cells. Oncogene (2020) 39(7):1543–56. doi: 10.1038/s41388-019-1084-z
44. Tcyganov E, Mastio J, Chen E, Gabrilovich DI. Plasticity of myeloid-derived suppressor cells in cancer. Curr Opin Immunol (2018) 51:76–82. doi: 10.1016/j.coi.2018.03.009
45. Mehta AK, Kadel S, Townsend MG, Oliwa M, Guerriero JL. Macrophage biology and mechanisms of immune suppression in breast cancer. Front Immunol (2021) 12:643771. doi: 10.3389/fimmu.2021.643771
46. Biswas S, Mandal G, Roy Chowdhury S, Purohit S, Payne KK, Anadon C, et al. Exosomes produced by mesenchymal stem cells drive differentiation of myeloid cells into immunosuppressive M2-polarized macrophages in breast cancer. J Immunol (2019) 203(12):3447–60. doi: 10.4049/jimmunol.1900692
47. Payne KK, Zoon CK, Wan W, Marlar K, Keim RC, Kenari MN, et al. Peripheral blood mononuclear cells of patients with breast cancer can be reprogrammed to enhance anti-HER-2/neu reactivity and overcome myeloid-derived suppressor cells. Breast Cancer Res Treat (2013) 142(1):45–57. doi: 10.1007/s10549-013-2733-5
48. Bruger AM, Dorhoi A, Esendagli G, Barczyk-Kahlert K, van der Bruggen P, Lipoldova M, et al. How to measure the immunosuppressive activity of MDSC: assays, problems and potential solutions. Cancer Immunol Immunother (2019) 68(4):631–44. doi: 10.1007/s00262-018-2170-8
49. Hoffmann SHL, Reck DI, Maurer A, Fehrenbacher B, Sceneay JE, Poxleitner M, et al. Visualization and quantification of in vivo homing kinetics of myeloid-derived suppressor cells in primary and metastatic cancer. Theranostics (2019) 9(20):5869–85. doi: 10.7150/thno.33275
50. Sceneay J, Griessinger CM, Hoffmann SHL, Wen SW, Wong CSF, Krumeich S, et al. Tracking the fate of adoptively transferred myeloid-derived suppressor cells in the primary breast tumor microenvironment. PloS One (2018) 13(4):e0196040. doi: 10.1371/journal.pone.0196040
51. Gabrilovich DI, Ostrand-Rosenberg S, Bronte V. Coordinated regulation of myeloid cells by tumours. Nat Rev Immunol (2012) 12(4):253–68. doi: 10.1038/nri3175
52. Li F, Zhao Y, Wei L, Li S, Liu J. Tumor-infiltrating treg, MDSC, and IDO expression associated with outcomes of neoadjuvant chemotherapy of breast cancer. Cancer Biol Ther (2018) 19(8):695–705. doi: 10.1080/15384047.2018.1450116
53. Srivastava MK, Sinha P, Clements VK, Rodriguez P, Ostrand-Rosenberg S. Myeloid-derived suppressor cells inhibit T cell activation by depleting cystine and cysteine. Cancer Res (2010) 70(1):68–77. doi: 10.1158/0008-5472.CAN-09-2587
54. Lu T, Ramakrishnan R, Altiok S, Youn JI, Cheng P, Celis E, et al. Tumor-infiltrating myeloid cells induce tumor cell resistance to cytotoxic T cells in mice. J Clin Invest (2011) 121(10):4015–29. doi: 10.1172/JCI45862
55. Stiff A, Trikha P, Mundy-Bosse B, McMichael E, Mace TA, Benner B, et al. Nitric oxide production by myeloid derived suppressor cells plays a role in impairing fc receptor-mediated natural killer cell function. Clin Cancer Res Off J Am Assoc Cancer Res (2018) 24(8):1891–904. doi: 10.1158/1078-0432.CCR-17-0691
56. Hanson EM, Clements VK, Sinha P, Ilkovitch D, Ostrand-Rosenberg S. Myeloid-derived suppressor cells down-regulate l-selectin expression on CD4+ and CD8+ T cells. J Immunol Baltim Md (2009) 183(2):937–44. doi: 10.4049/jimmunol.0804253
57. Sinha P, Chornoguz O, Clements VK, Artemenko KA, Zubarev RA, Ostrand-Rosenberg S. Myeloid-derived suppressor cells express the death receptor fas and apoptose in response to T cell–expressed FasL. Blood (2011) 117(20):5381–90. doi: 10.1182/blood-2010-11-321752
58. Lelis FJN, Jaufmann J, Singh A, Fromm K, Teschner AC, Pöschel S, et al. Myeloid-derived suppressor cells modulate b-cell responses. Immunol Lett (2017) 188:108–15. doi: 10.1016/j.imlet.2017.07.003
59. Shen M, Wang J, Yu W, Zhang C, Liu M, Wang K, et al. A novel MDSC-induced PD-1–PD-L1+ b-cell subset in breast tumor microenvironment possesses immuno-suppressive properties. Oncoimmunology (2018) 7(4):e1413520. doi: 10.1080/2162402X.2017.1413520
60. Nam S, Lee A, Lim J, Lim JS. Analysis of the expression and regulation of PD-1 protein on the surface of myeloid-derived suppressor cells (MDSCs). Biomol Ther (2019) 27(1):63–70. doi: 10.4062/biomolther.2018.201
61. Liu M, Wei F, Wang J, Yu W, Shen M, Liu T, et al. Myeloid-derived suppressor cells regulate the immunosuppressive functions of PD-1–PD-L1+ bregs through PD-L1/PI3K/AKT/NF-κB axis in breast cancer. Cell Death Dis (2021) 12(5):465. doi: 10.1038/s41419-021-03745-1
62. Spallanzani RG, Dalotto-Moreno T, Raffo Iraolagoitia XL, Ziblat A, Domaica CI, Avila DE, et al. Expansion of CD11b+Ly6G+Ly6Cint cells driven by medroxyprogesterone acetate in mice bearing breast tumors restrains NK cell effector functions. Cancer Immunol Immunother (2013) 62(12):1781–95. doi: 10.1007/s00262-013-1483-x
63. Sceneay J, Chow MT, Chen A, Halse HM, Wong CSF, Andrews DM, et al. Primary tumor hypoxia recruits CD11b+/Ly6Cmed/Ly6G+ immune suppressor cells and compromises NK cell cytotoxicity in the premetastatic niche. Cancer Res (2012) 72(16):3906–11. doi: 10.1158/0008-5472.CAN-11-3873
64. Deng Z, Rong Y, Teng Y, Zhuang X, Samykutty A, Mu J, et al. Exosomes miR-126a released from MDSC induced by DOX treatment promotes lung metastasis. Oncogene (2017) 36(5):639–51. doi: 10.1038/onc.2016.229
65. Peng D, Tanikawa T, Li W, Zhao L, Vatan L, Szeliga W, et al. Myeloid-derived suppressor cells endow stem-like qualities to breast cancer cells through IL-6/STAT3 and NO/NOTCH cross-talk signaling. Cancer Res (2016) 76(11):3156–65. doi: 10.1158/0008-5472.CAN-15-2528
66. Sprouse ML, Welte T, Boral D, Liu HN, Yin W, Vishnoi M, et al. PMN-MDSCs enhance CTC metastatic properties through reciprocal interactions via ROS/Notch/Nodal signaling. Int J Mol Sci (2019) 20(8):1916. doi: 10.3390/ijms20081916
67. Ma X, Wang M, Yin T, Zhao Y, Wei X. Myeloid-derived suppressor cells promote metastasis in breast cancer after the stress of operative removal of the primary cancer. Front Oncol (2019) 9:855. doi: 10.3389/fonc.2019.00855
68. Luo A, Meng M, Wang G, Han R, Zhang Y, Jing X, et al. Myeloid-derived suppressor cells recruited by chemokine (C-c motif) ligand 3 promote the progression of breast cancer via phosphoinositide 3-Kinase-Protein kinase b-mammalian target of rapamycin signaling. J Breast Cancer (2020) 23(2):141–61. doi: 10.4048/jbc.2020.23.e26
69. Ouzounova M, Lee E, Piranlioglu R, El Andaloussi A, Kolhe R, Demirci MF, et al. Monocytic and granulocytic myeloid derived suppressor cells differentially regulate spatiotemporal tumour plasticity during metastatic cascade. Nat Commun (2017) 8(1):14979. doi: 10.1038/ncomms14979
70. Mucha J, Majchrzak K, Taciak B, Hellmén E, Król M. MDSCs mediate angiogenesis and predispose canine mammary tumor cells for metastasis via IL-28/IL-28RA (IFN-λ) signaling. PloS One (2014) 9(7):e103249. doi: 10.1371/journal.pone.0103249
71. Gao D, Joshi N, Choi H, Ryu S, Hahn M, Catena R, et al. Myeloid progenitor cells in the premetastatic lung promote metastases by inducing mesenchymal to epithelial transition. Cancer Res (2012) 72(6):1384–94. doi: 10.1158/0008-5472.CAN-11-2905
72. Hsu YL, Yen MC, Chang WA, Tsai PH, Pan YC, Liao SH, et al. CXCL17-derived CD11b+Gr-1+ myeloid-derived suppressor cells contribute to lung metastasis of breast cancer through platelet-derived growth factor-BB. Breast Cancer Res BCR (2019) 21:23. doi: 10.1186/s13058-019-1114-3
73. Yan HH, Pickup M, Pang Y, Gorska AE, Li Z, Chytil A, et al. Gr-1+CD11b+ myeloid cells tip the balance of immune protection to tumor promotion in the premetastatic lung. Cancer Res (2010) 70(15):6139–49. doi: 10.1158/0008-5472.CAN-10-0706
74. Jian J, Pang Y, Yan HH, Min Y, Achyut BR, Hollander MC, et al. Platelet factor 4 is produced by subsets of myeloid cells in premetastatic lung and inhibits tumor metastasis. Oncotarget (2016) 8(17):27725–39. doi: 10.18632/oncotarget.9486
75. Urueña C, Lasso P, Bernal-Estevez D, Rubio D, Salazar AJ, Olaya M, et al. The breast cancer immune microenvironment is modified by neoadjuvant chemotherapy. Sci Rep (2022) 12:7981. doi: 10.1038/s41598-022-12108-5
76. Wesolowski R, Duggan MC, Stiff A, Markowitz J, Trikha P, Levine KM, et al. Circulating myeloid-derived suppressor cells increase in patients undergoing neo-adjuvant chemotherapy for breast cancer. Cancer Immunol Immunother (2017) 66(11):1437–47. doi: 10.1007/s00262-017-2038-3
77. Bergenfelz C, Roxå A, Mehmeti M, Leandersson K, Larsson AM. Clinical relevance of systemic monocytic-MDSCs in patients with metastatic breast cancer. Cancer Immunol Immunother (2020) 69(3):435–48. doi: 10.1007/s00262-019-02472-z
78. Picon-Ruiz M, Morata-Tarifa C, Valle-Goffin JJ, Friedman ER, Slingerland JM. Obesity and adverse breast cancer risk and outcome: mechanistic insights and strategies for intervention. CA Cancer J Clin (2017) 67(5):378–97. doi: 10.3322/caac.21405
79. Pingili AK, Chaib M, Sipe LM, Miller EJ, Teng B, Sharma R, et al. Immune checkpoint blockade reprograms systemic immune landscape and tumor microenvironment in obesity-associated breast cancer. Cell Rep (2021) 35(12):109285. doi: 10.1016/j.celrep.2021.109285
80. Gibson JT, Orlandella RM, Turbitt WJ, Behring M, Manne U, Sorge RE, et al. Obesity-associated myeloid-derived suppressor cells promote apoptosis of tumor-infiltrating CD8 T cells and immunotherapy resistance in breast cancer. Front Immunol (2020) 11:590794. doi: 10.3389/fimmu.2020.590794
81. Li W, Tanikawa T, Kryczek I, Xia H, Li G, Wu K, et al. Aerobic glycolysis controls myeloid-derived suppressor cells and tumor immunity via a specific CEBPB isoform in triple-negative breast cancer. Cell Metab (2018) 28(1):87–103.e6. doi: 10.1016/j.cmet.2018.04.022
82. Garritson J, Krynski L, Haverbeck L, Haughian JM, Pullen NA, Hayward R. Physical activity delays accumulation of immunosuppressive myeloid-derived suppressor cells. PloS One (2020) 15(6):e0234548. doi: 10.1371/journal.pone.0234548
83. Zhang Y, Ma B, Fan Q. Mechanisms of breast cancer bone metastasis. Cancer Lett (2010) 292(1):1–7. doi: 10.1016/j.canlet.2009.11.003
84. Danilin S, Merkel AR, Johnson JR, Johnson RW, Edwards JR, Sterling JA. Myeloid-derived suppressor cells expand during breast cancer progression and promote tumor-induced bone destruction. Oncoimmunology (2012) 1(9):1484–94. doi: 10.4161/onci.21990
85. Sawant A, Deshane J, Jules J, Lee CM, Harris BA, Feng X, et al. Myeloid-derived suppressor cells function as novel osteoclast progenitors enhancing bone loss in breast cancer. Cancer Res (2013) 73(2):672–82. doi: 10.1158/0008-5472.CAN-12-2202
86. Kun Z, Xin G, Tao W, Chenglong Z, Dongsheng W, Liang T, et al. Tumor derived EDIL3 modulates the expansion and osteoclastogenesis of myeloid derived suppressor cells in murine breast cancer model. J Bone Oncol (2019) 16:100238. doi: 10.1016/j.jbo.2019.100238
87. Bruchard M, Mignot G, Derangère V, Chalmin F, Chevriaux A, Végran F, et al. Chemotherapy-triggered cathepsin b release in myeloid-derived suppressor cells activates the Nlrp3 inflammasome and promotes tumor growth. Nat Med (2013) 19(1):57–64. doi: 10.1038/nm.2999
88. Kodumudi KN, Woan K, Gilvary DL, Sahakian E, Wei S, Djeu JY. A novel chemoimmunomodulating property of docetaxel: suppression of myeloid-derived suppressor cells in tumor bearers. Clin Cancer Res (2010) 16(18):4583–94. doi: 10.1158/1078-0432.CCR-10-0733
89. Michels T, Shurin GV, Naiditch H, Sevko A, Umansky V, Shurin MR. Paclitaxel promotes differentiation of myeloid-derived suppressor cells into dendritic cells in vitro in a TLR4-independent manner. J Immunotoxicol (2012) 9(3):292–300. doi: 10.3109/1547691X.2011.642418
90. Roland CL, Lynn KD, Toombs JE, Dineen SP, Udugamasooriya DG, Brekken RA, et al. Cytokine levels correlate with immune cell infiltration after anti-VEGF therapy in preclinical mouse models of breast cancer. PloS One (2009) 4(11):e7669. doi: 10.1371/journal.pone.0007669
91. Yin T, He S, Shen G, Wang Y. HIF-1 dimerization inhibitor acriflavine enhances antitumor activity of sunitinib in breast cancer model. Oncol Res Featur Preclin Clin Cancer Ther (2015) 22(3):139–45. doi: 10.3727/096504014X13983417587366
92. Haas MS, Kagey MH, Heath H, Schuerpf F, Rottman JB, Newman W. mDKN-01, a novel anti-DKK1 mAb, enhances innate immune responses in the tumor microenvironment. Mol Cancer Res MCR (2021) 19(4):717–25. doi: 10.1158/1541-7786.MCR-20-0799
93. Zonneville J, Colligan S, Grant S, Miller A, Wallace P, Abrams SI, et al. Blockade of p38 kinase impedes the mobilization of protumorigenic myeloid populations to impact breast cancer metastasis. Int J Cancer (2020) 147(8):2279–92. doi: 10.1002/ijc.33050
94. Slaney CY, Möller A, Hertzog PJ, Parker BS. The role of type I interferons in immunoregulation of breast cancer metastasis to the bone. OncoImmunology (2013) 2(1):e22339. doi: 10.4161/onci.22339
95. Tavazoie MF, Pollack I, Tanqueco R, Ostendorf BN, Reis BS, Gonsalves FC, et al. LXR/ApoE activation restricts innate immune suppression in cancer. Cell (2018) 172(4):825–840.e18. doi: 10.1016/j.cell.2017.12.026
96. Dominguez GA, Condamine T, Mony S, Hashimoto A, Wang F, Liu Q, et al. Selective targeting of myeloid-derived suppressor cells in cancer patients using DS-8273a, an agonistic TRAIL-R2 antibody. Clin Cancer Res Off J Am Assoc Cancer Res (2017) 23(12):2942–50. doi: 10.1158/1078-0432.CCR-16-1784
97. Callmann CE, Cole LE, Kusmierz CD, Huang Z, Horiuchi D, Mirkin CA. Tumor cell lysate-loaded immunostimulatory spherical nucleic acids as therapeutics for triple-negative breast cancer. Proc Natl Acad Sci U S A (2020) 117(30):17543–50. doi: 10.1073/pnas.2005794117
98. Rashid OM, Nagahashi M, Ramachandran S, Graham L, Yamada A, Spiegel S, et al. Resection of the primary tumor improves survival in metastatic breast cancer by reducing overall tumor burden. Surgery (2013) 153(6):771–8. doi: 10.1016/j.surg.2013.02.002
99. Ghochikyan A, Davtyan A, Hovakimyan A, Davtyan H, Poghosyan A, Bagaev A, et al. Primary 4T1 tumor resection provides critical “window of opportunity” for immunotherapy. Clin Exp Metastasis (2014) 31(2):185–98. doi: 10.1007/s10585-013-9619-0
100. Habibi M, Kmieciak M, Graham L, Morales JK, Bear HD, Manjili MH. Radiofrequency thermal ablation of breast tumors combined with intralesional administration of IL-7 and IL-15 augments anti-tumor immune responses and inhibits tumor development and metastasis. Breast Cancer Res Treat (2009) 114(3):423–31. doi: 10.1007/s10549-008-0024-3
101. Gebremeskel S, Lobert L, Tanner K, Walker B, Oliphant T, Clarke LE, et al. Natural killer T-cell immunotherapy in combination with chemotherapy-induced immunogenic cell death targets metastatic breast cancer. Cancer Immunol Res (2017) 5(12):1086–97. doi: 10.1158/2326-6066.CIR-17-0229
102. Sun R, Luo H, Su J, Di S, Zhou M, Shi B, et al. Olaparib suppresses MDSC recruitment via SDF1α/CXCR4 axis to improve the anti-tumor efficacy of CAR-T cells on breast cancer in mice. Mol Ther J Am Soc Gene Ther (2021) 29(1):60–74. doi: 10.1016/j.ymthe.2020.09.034
103. Lu Z, Zou J, Li S, Topper MJ, Tao Y, Zhang H, et al. Epigenetic therapy inhibits metastases by disrupting premetastatic niches. Nature (2020) 579(7798):284–90. doi: 10.1038/s41586-020-2054-x
104. Forghani P, Khorramizadeh MR, Waller EK. Silibinin inhibits accumulation of myeloid-derived suppressor cells and tumor growth of murine breast cancer. Cancer Med (2014) 3(2):215–24. doi: 10.1002/cam4.186
105. Velasco-Velázquez M, Jiao X, de la Fuente M, Pestell TG, Ertel A, Lisanti MP, et al. CCR5 antagonist blocks metastasis of basal breast cancer cells. Cancer Res (2012) 72(15):3839–50. doi: 10.1158/0008-5472.CAN-11-3917
106. Ouyang L, Chang W, Fang B, Qin J, Qu X, Cheng F. Estrogen-induced SDF-1α production promotes the progression of ER-negative breast cancer via the accumulation of MDSCs in the tumor microenvironment. Sci Rep (2016) 6:39541. doi: 10.1038/srep39541
107. Zheng Y, Wang N, Wang S, Yang B, Situ H, Zhong L, et al. XIAOPI formula inhibits the pre-metastatic niche formation in breast cancer via suppressing TAMs/CXCL1 signaling. Cell Commun Signal CCS (2020) 18(1):48. doi: 10.1186/s12964-020-0520-6
108. Tian S, Song X, Wang Y, Wang X, Mou Y, Chen Q, et al. Chinese Herbal medicine baoyuan jiedu decoction inhibits the accumulation of myeloid derived suppressor cells in pre-metastatic niche of lung via TGF-β/CCL9 pathway. BioMed Pharmacother Biomed Pharmacother (2020) 129:110380. doi: 10.1016/j.biopha.2020.110380
109. Cao Y, Slaney CY, Bidwell BN, Parker BS, Johnstone CN, Rautela J, et al. BMP4 inhibits breast cancer metastasis by blocking myeloid-derived suppressor cell activity. Cancer Res (2014) 74(18):5091–102. doi: 10.1158/0008-5472.CAN-13-3171
110. Kumar V, Donthireddy L, Marvel D, Condamine T, Wang F, Lavilla-Alonso S, et al. Cancer-associated fibroblasts neutralize the anti-tumor effect of CSF1 receptor blockade by inducing PMN-MDSC infiltration of tumors. Cancer Cell (2017) 32(5):654–668.e5. doi: 10.1016/j.ccell.2017.10.005
111. Dawod B, Liu J, Gebremeskel S, Yan C, Sappong A, Johnston B, et al. Myeloid-derived suppressor cell depletion therapy targets IL-17A-expressing mammary carcinomas. Sci Rep (2020) 10:13343. doi: 10.1038/s41598-020-70231-7
112. Dominguez C, McCampbell KK, David JM, Palena C. Neutralization of IL-8 decreases tumor PMN-MDSCs and reduces mesenchymalization of claudin-low triple-negative breast cancer. JCI Insight (2017) 2(21):94296. doi: 10.1172/jci.insight.94296
113. Singh M, Ramos I, Asafu-Adjei D, Quispe-Tintaya W, Chandra D, Jahangir A, et al. Curcumin improves the therapeutic efficacy of listeria(at)-mage-b vaccine in correlation with improved T-cell responses in blood of a triple-negative breast cancer model 4T1. Cancer Med (2013) 2(4):571–82. doi: 10.1002/cam4.94
114. Schmid MC, Khan SQ, Kaneda MM, Pathria P, Shepard R, Louis TL, et al. Integrin CD11b activation drives anti-tumor innate immunity. Nat Commun (2018) 9(1):5379. doi: 10.1038/s41467-018-07387-4
115. Liu L, Ye TH, Han YP, Song H, Zhang YK, Xia Y, et al. Reductions in myeloid-derived suppressor cells and lung metastases using AZD4547 treatment of a metastatic murine breast tumor model. Cell Physiol Biochem (2014) 33(3):633–45. doi: 10.1159/000358640
116. Uribesalgo I, Hoffmann D, Zhang Y, Kavirayani A, Lazovic J, Berta J, et al. Apelin inhibition prevents resistance and metastasis associated with anti-angiogenic therapy. EMBO Mol Med (2019) 11(8):e9266. doi: 10.15252/emmm.201809266
117. Ashraf Y, Mansouri H, Laurent-Matha V, Alcaraz LB, Roger P, Guiu S, et al. Immunotherapy of triple-negative breast cancer with cathepsin d-targeting antibodies. J Immunother Cancer (2019) 7(1):29. doi: 10.1186/s40425-019-0498-z
118. Lauder SN, Smart K, Bart VMT, Pires A, Scott J, Milutinovic S, et al. Treg-driven tumour control by PI3Kδ inhibition limits myeloid-derived suppressor cell expansion. Br J Cancer (2022) 127(9):1595–602. doi: 10.1038/s41416-022-01917-0
119. Gupta N, Gaikwad S, Kaushik I, Wright SE, Markiewski MM, Srivastava SK. Atovaquone suppresses triple-negative breast tumor growth by reducing immune-suppressive cells. Int J Mol Sci (2021) 22(10):5150. doi: 10.3390/ijms22105150
120. Markiewski MM, Vadrevu SK, Sharma SK, Chintala NK, Ghouse S, Cho JH, et al. The ribosomal protein S19 suppresses antitumor immune responses via the complement C5a receptor 1. J Immunol Baltim Md (2017) 198(7):2989–99. doi: 10.4049/jimmunol.1602057
121. Monteran L, Ershaid N, Doron H, Zait Y, Scharff Y, Ben-Yosef S, et al. Chemotherapy-induced complement signaling modulates immunosuppression and metastatic relapse in breast cancer. Nat Commun (2022) 13(1):5797. doi: 10.1038/s41467-022-33598-x
122. Liu Y, Wei G, Cheng WA, Dong Z, Sun H, Lee VY, et al. Targeting myeloid-derived suppressor cells for cancer immunotherapy. Cancer Immunol Immunother CII (2018) 67(8):1181–95. doi: 10.1007/s00262-018-2175-3
123. Secondini C, Coquoz O, Spagnuolo L, Spinetti T, Peyvandi S, Ciarloni L, et al. Arginase inhibition suppresses lung metastasis in the 4T1 breast cancer model independently of the immunomodulatory and anti-metastatic effects of VEGFR-2 blockade. Oncoimmunology (2017) 6(6):e1316437. doi: 10.1080/2162402X.2017.1316437
124. Chaib M, Sipe LM, Yarbro JR, Bohm MS, Counts BR, Tanveer U, et al. PKC agonism restricts innate immune suppression, promotes antigen cross-presentation and synergizes with agonistic CD40 antibody therapy to activate CD8(+) T cells in breast cancer. Cancer Lett (2022) 531:98–108. doi: 10.1016/j.canlet.2022.01.017
125. Nefedova Y, Fishman M, Sherman S, Wang X, Beg AA, Gabrilovich DI. Mechanism of all- Trans retinoic acid effect on tumor-associated myeloid-derived suppressor cells. Cancer Res (2007) 67(22):11021–8. doi: 10.1158/0008-5472.CAN-07-2593
126. Bauer R, Udonta F, Wroblewski M, Ben-Batalla I, Santos IM, Taverna F, et al. Blockade of myeloid-derived suppressor cell expansion with all- Trans retinoic acid increases the efficacy of antiangiogenic therapy. Cancer Res (2018) 78(12):3220–32. doi: 10.1158/0008-5472.CAN-17-3415
127. Svoronos N, Perales-Puchalt A, Allegrezza MJ, Rutkowski MR, Payne KK, Tesone AJ, et al. Tumor cell–independent estrogen signaling drives disease progression through mobilization of myeloid-derived suppressor cells. Cancer Discovery (2017) 7(1):72–85. doi: 10.1158/2159-8290.CD-16-0502
128. Hashimoto A, Gao C, Mastio J, Kossenkov A, Abrams SI, Purandare AV, et al. Inhibition of casein kinase 2 disrupts differentiation of myeloid cells in cancer and enhances the efficacy of immunotherapy in mice. Cancer Res (2018) 78(19):5644–55. doi: 10.1158/0008-5472.CAN-18-1229
129. Chandra D, Quispe-Tintaya W, Jahangir A, Asafu-Adjei D, Ramos I, Sintim HO, et al. STING ligand c-di-GMP improves cancer vaccination against metastatic breast cancer. Cancer Immunol Res (2014) 2(9):901–10. doi: 10.1158/2326-6066.CIR-13-0123
130. Christmas BJ, Rafie CI, Hopkins AC, Scott BA, Ma HS, Cruz KA, et al. Entinostat converts immune-resistant breast and pancreatic cancers into checkpoint-responsive tumors by reprogramming tumor-infiltrating MDSCs. Cancer Immunol Res (2018) 6(12):1561–77. doi: 10.1158/2326-6066.CIR-18-0070
131. Melani C, Sangaletti S, Barazzetta FM, Werb Z, Colombo MP. Amino-Biphosphonate–mediated MMP-9 inhibition breaks the tumor-bone marrow axis responsible for myeloid-derived suppressor cell expansion and macrophage infiltration in tumor stroma. Cancer Res (2007) 67(23):11438–46. doi: 10.1158/0008-5472.CAN-07-1882
132. Capietto AH, Lee S, Clever D, Eul E, Ellis H, Ma CX, et al. Effective treatment of established bone metastases can be achieved by combinatorial osteoclast blockade and depletion of granulocytic subsets. Cancer Immunol Res (2021) 9(12):1400–12. doi: 10.1158/2326-6066.CIR-21-0232
133. Zang X, Song J, Yi X, Piyu J. Polymeric indoximod based prodrug nanoparticles with doxorubicin entrapment for inducing immunogenic cell death and improving the immunotherapy of breast cancer. J Mater Chem B (2022) 10(12):2019–27. doi: 10.1039/D2TB00197G
134. Liu Y, Li C, Xia H, Bi J, Guan R, Du X, et al. An injectable superior depot of telratolimod inhibits post-surgical tumor recurrence and distant metastases. Acta Biomater (2022) 141:132–9. doi: 10.1016/j.actbio.2022.01.013
135. Revuri V, Rajendrakumar SK, Park MS, Mohapatra A, Uthaman S, Mondal J, et al. Heat-confined tumor-docking reversible thermogel potentiates systemic antitumor immune response during near-infrared photothermal ablation in triple-negative breast cancer. Adv Healthc Mater (2021) 10(21):e2100907. doi: 10.1002/adhm.202100907
136. Lu Z, Liu H, Ma L, Ren K, He Z, Li M, et al. Micellar nanoparticles inhibit breast cancer and pulmonary metastasis by modulating the recruitment and depletion of myeloid-derived suppressor cells. Nanoscale (2022) 14(46):17315–30. doi: 10.1039/D2NR03880C
137. Lu Z, Long Y, Li J, Li J, Ren K, Zhao W, et al. Simultaneous inhibition of breast cancer and its liver and lung metastasis by blocking inflammatory feed-forward loops. J Control Release Off J Control Release Soc (2021) 338:662–79. doi: 10.1016/j.jconrel.2021.08.047
138. Liu Y, Tiruthani K, Wang M, Zhou X, Qiu N, Xiong Y, et al. Tumor-targeted gene therapy with lipid nanoparticles inhibits tumor-associated adipocytes and remodels the immunosuppressive tumor microenvironment in triple-negative breast cancer. Nanoscale Horiz (2021) 6(4):319–29. doi: 10.1039/D0NH00588F
139. Phuengkham H, Song C, Um SH, Lim YT. Implantable synthetic immune niche for spatiotemporal modulation of tumor-derived immunosuppression and systemic antitumor immunity: postoperative immunotherapy. Adv Mater Deerfield Beach Fla (2018) 30(18):e1706719. doi: 10.1002/adma.201706719
140. Zhang R, Wan Y, Lv H, Li F, Lee CS. DTX@VTX NPs synergy PD-L1 immune checkpoint nanoinhibitor to reshape immunosuppressive tumor microenvironment for enhancing chemo-immunotherapy. J Mater Chem B (2021) 9(36):7544–56. doi: 10.1039/D1TB00269D
141. Li S, Wang Q, Shen Y, Hassan M, Shen J, Jiang W, et al. Pseudoneutrophil cytokine sponges disrupt myeloid expansion and tumor trafficking to improve cancer immunotherapy. Nano Lett (2020) 20(1):242–51. doi: 10.1021/acs.nanolett.9b03753
142. Hu S, Ma J, Su C, Chen Y, Shu Y, Qi Z, et al. Engineered exosome-like nanovesicles suppress tumor growth by reprogramming tumor microenvironment and promoting tumor ferroptosis. Acta Biomater (2021) 135:567–81. doi: 10.1016/j.actbio.2021.09.003
143. Shu C, Sun P, Xie H, Huang W, Qi J, Ma Y. Virus-like particles presenting the FGF-2 protein or identified antigenic peptides promoted antitumor immune responses in mice. Int J Nanomed (2020) 15:1983–96. doi: 10.2147/IJN.S237182
144. Zhang FF, Qiao Y, Xie Y, Liu C, Wu H, Wu JX, et al. Epitope-based minigene vaccine targeting fibroblast activation protein α induces specific immune responses and anti-tumor effects in 4 T1 murine breast cancer model. Int Immunopharmacol (2022) 112:109237. doi: 10.1016/j.intimp.2022.109237
145. Abdoli Shadbad M, Hajiasgharzadeh K, Baradaran B. Cross-talk between myeloid-derived suppressor cells and Mucin1 in breast cancer vaccination: on the verge of a breakthrough. Life Sci (2020) 258:118128. doi: 10.1016/j.lfs.2020.118128
146. Ma HS, Poudel B, Torres ER, Sidhom JW, Robinson TM, Christmas B, et al. A CD40 agonist and PD-1 antagonist antibody reprogram the microenvironment of nonimmunogenic tumors to allow T-cell-Mediated anticancer activity. Cancer Immunol Res (2019) 7(3):428–42. doi: 10.1158/2326-6066.CIR-18-0061
147. Geng F, Bao X, Dong L, Guo QQ, Guo J, Xie Y, et al. Doxorubicin pretreatment enhances FAPα/survivin co-targeting DNA vaccine anti-tumor activity primarily through decreasing peripheral MDSCs in the 4T1 murine breast cancer model. Oncoimmunology (2020) 9(1):1747350. doi: 10.1080/2162402X.2020.1747350
148. Zamani P, Navashenaq JG, Teymouri M, Karimi M, Mashreghi M, Jaafari MR. Combination therapy with liposomal doxorubicin and liposomal vaccine containing E75, an HER-2/neu-derived peptide, reduces myeloid-derived suppressor cells and improved tumor therapy. Life Sci (2020) 252:117646. doi: 10.1016/j.lfs.2020.117646
149. Rodriguez-Ruiz ME, Rodriguez I, Garasa S, Barbes B, Solorzano JL, Perez-Gracia JL, et al. Abscopal effects of radiotherapy are enhanced by combined immunostimulatory mAbs and are dependent on CD8 T cells and crosspriming. Cancer Res (2016) 76(20):5994–6005. doi: 10.1158/0008-5472.CAN-16-0549
150. Deng L, Liang H, Burnette B, Beckett M, Darga T, Weichselbaum RR, et al. Irradiation and anti-PD-L1 treatment synergistically promote antitumor immunity in mice. J Clin Invest (2014) 124(2):687–95. doi: 10.1172/JCI67313
151. Chang WI, Han MG, Kang MH, Park JM, Kim EE, Bae J, et al. PI3Kαδ inhibitor combined with radiation enhances the antitumor immune effect of anti-PD1 in a syngeneic murine triple-negative breast cancer model. Int J Radiat Oncol Biol Phys (2021) 110(3):845–58. doi: 10.1016/j.ijrobp.2021.01.025
152. Pilones KA, Hensler M, Daviaud C, Kraynak J, Fucikova J, Galluzzi L, et al. Converging focal radiation and immunotherapy in a preclinical model of triple negative breast cancer: contribution of VISTA blockade. Oncoimmunology (2020) 9(1):1830524. doi: 10.1080/2162402X.2020.1830524
153. Confino H, Schmidt M, Efrati M, Hochman I, Umansky V, Kelson I, et al. Inhibition of mouse breast adenocarcinoma growth by ablation with intratumoral alpha-irradiation combined with inhibitors of immunosuppression and CpG. Cancer Immunol Immunother CII (2016) 65(10):1149–58. doi: 10.1007/s00262-016-1878-6
Keywords: myeloid-derived suppressor cells, breast cancer, immunosuppression, immunotherapy, tumor microenvironments
Citation: Liu H, Wang Z, Zhou Y and Yang Y (2023) MDSCs in breast cancer: an important enabler of tumor progression and an emerging therapeutic target. Front. Immunol. 14:1199273. doi: 10.3389/fimmu.2023.1199273
Received: 03 April 2023; Accepted: 19 June 2023;
Published: 03 July 2023.
Edited by:
Robert Wesolowski, The Ohio State University, United StatesReviewed by:
Ik-Hwan Han, Kyung Hee University, Republic of KoreaRodwell Mabaera, Dartmouth Hitchcock Medical Center, United States
Copyright © 2023 Liu, Wang, Zhou and Yang. This is an open-access article distributed under the terms of the Creative Commons Attribution License (CC BY). The use, distribution or reproduction in other forums is permitted, provided the original author(s) and the copyright owner(s) are credited and that the original publication in this journal is cited, in accordance with accepted academic practice. No use, distribution or reproduction is permitted which does not comply with these terms.
*Correspondence: Yanming Yang, yym180@163.com