- 1Centre for Cell Factories and Biopolymers (CCFB), Griffith Institute for Drug Discovery, Griffith University, Nathan, QLD, Australia
- 2Menzies Health Institute Queensland (MHIQ), Griffith University, Gold Coast, QLD, Australia
Vaccines remain the best approach for the prevention of infectious diseases. Protein subunit vaccines are safe compared to live-attenuated whole cell vaccines but often show reduced immunogenicity. Subunit vaccines in particulate format show improved vaccine efficacy by inducing strong immune responses leading to protective immunity against the respective pathogens. Antigens with proper conformation and function are often required to induce functional immune responses. Production of such antigens requiring post-translational modifications and/or composed of multiple complex domains in bacterial hosts remains challenging. Here, we discuss strategies to overcome these limitations toward the development of particulate vaccines eliciting desired humoral and cellular immune responses. We also describe innovative concepts of assembling particulate vaccine candidates with complex antigens bearing multiple post-translational modifications. The approaches include non-covalent attachments (e.g. biotin-avidin affinity) and covalent attachments (e.g. SpyCatcher-SpyTag) to attach post-translationally modified antigens to particles.
Immunologic properties of soluble and particulate vaccines
Subunit vaccines contain selected immunogenic components of the pathogen to elicit an immune response (1, 2). Particulate vaccines involve the attachment of the antigens to microcarriers through chemical adsorption, encapsulation, conjugation, or biological self-assembly for enhanced delivery and induction of an efficient immune response (3, 4). Soluble vaccines are weakly immunogenic when compared to their insoluble counterparts such as when displayed on particulate carrier (5). Due to low immunogenicity, soluble vaccines often require the administration of multiple boosts (6, 7). The use of immunostimulatory adjuvants along with soluble vaccines would improve the immune responses but potentially increases the overall vaccine production cost (8). However, antigens immobilized on particulate carrier exhibit enhanced immunogenicity (9–11). Some particulate carriers themselves act as adjuvants resulting in enhanced and targeted immune responses. Particulate carrier also allow the codelivery of adjuvants and multiple antigens to the same antigen presenting cells (APCs) (12, 13). Therefore, particulate vaccines are promising and potent antigen delivery systems to overcome the low immunogenicity of soluble subunit vaccine formulations.
The proposed mechanism of antigen processing elicited by soluble and particulate vaccine formulations is illustrated in Figure 1. Soluble antigens can be internalized via endocytosis (14) and exclusively presented by major histocompatibility complex (MHC) II machinery in the endosomes, whereas particulate antigens above 500 nm can be phagocytosed by APCs into the phagosomes and presented by both MHC class I and II machineries in the cytosol (7). The maturation of the phagosome occurs after the formation of nascent phagosomes (pH 7.4) containing the engulfed particulate antigens which are then sequentially trafficked into progressive acidified compartments called early phagosome (pH 6), late phagosome (pH 5.5), and phagolysosomes (pH 5) (15). The efficient degradation of antigens into smaller peptides occurs in the phagolysosomes which contain a variety of digestive enzymes such as proteases, lipases, and glycosidases without degrading the epitopes (16). Further degradation of antigens into smaller polypeptides occurs in the cytosol by a protein complex called proteosomes (17). The degraded protein fragments will be transported into the endoplasmic reticulum, where the folding and assembly of the heavy and light chain of MHC molecules occurs and facilitates the MHC and peptide binding (18). Finally, the peptide-loaded MHC complex gets transported to the cell surface through the Golgi apparatus and attracts both CD4+ T cells and CD8+ T cells with specific receptors to mount a cell-mediated immune response eliminating the damaged/infected cells displaying corresponding peptide fragments (19). MHC class I and II pathways are usually involved presenting peptides from intracellular or extracellular pathogens, respectively. However, dendritic cells (DCs) possess the ability to divert peptides derived from extracellular pathogens to cytotoxic CD8+ T cells via the MHC class I presentation pathway (20). A phagosome is a key organelle in antigen cross-presentation which primarily induces cytotoxic CD8+ T cell responses. However, such responses mediated by the phagosome route are not achievable by endocytosed soluble antigens (21). In addition, B cell receptors (BCRs) are uniformly distributed on B cell surface in the absence of pathogen invasion. However, BCRs are brought together to bind multiple copies of antigens on the invading pathogen’s surface. This process is also called BCR cross-linking, required for B cell activation. Repetitive display of antigens on particles facilitates efficient recognition and BCR cross-linking, which allow strong B cell activation and antigen uptake for presentation to CD4+ T cells. It results in inducing higher levels of neutralizing antibodies and functional cellular immune responses than achieved by soluble antigens present in most subunit vaccines (22).
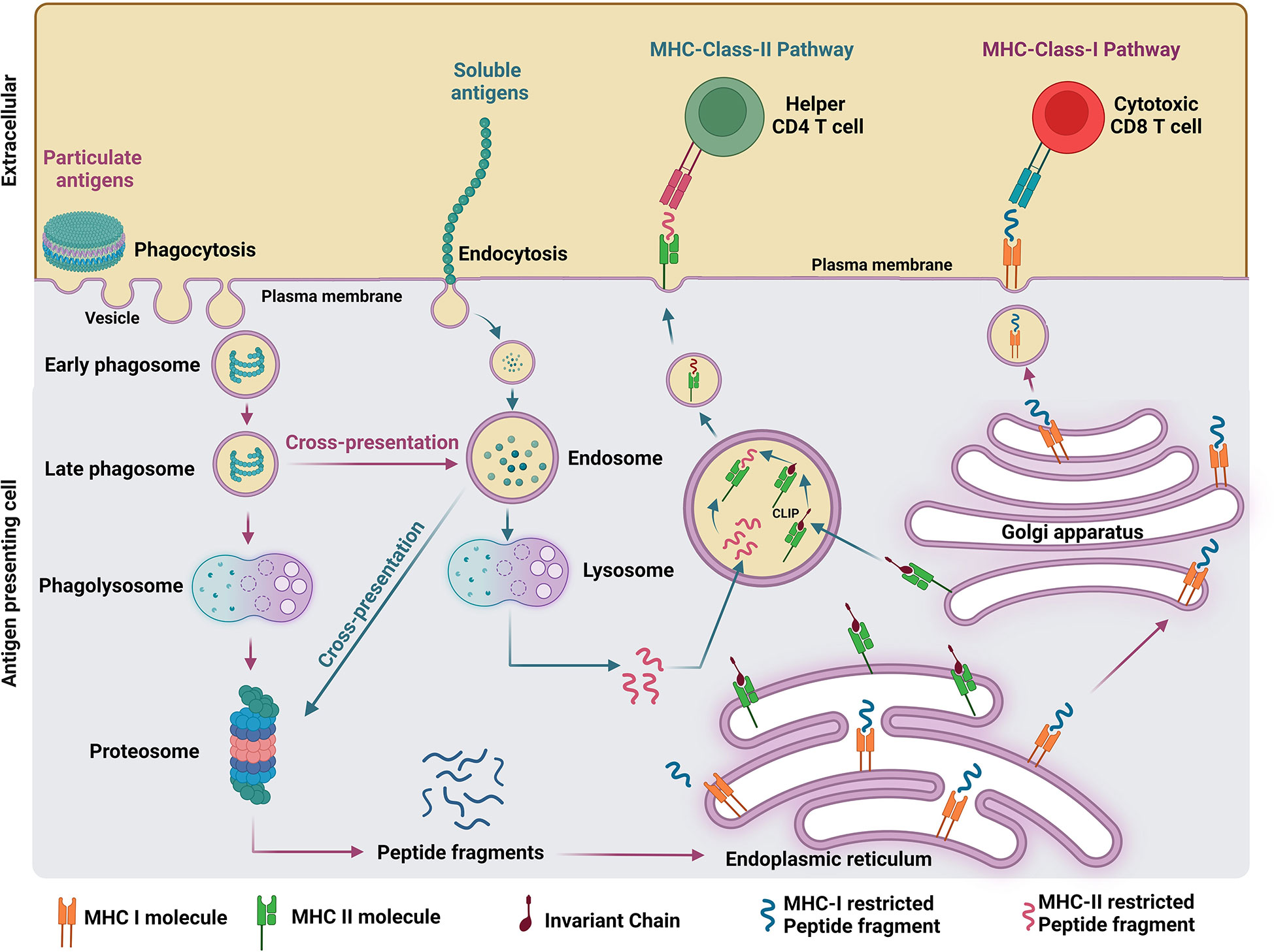
Figure 1 Comparison of antigen processing pathways elicited by soluble and particulate antigen formulations.
Soluble antigens are engulfed by APCs through endocytosis and get presented to endosomes. Consequently, endosomes fuse with lysosomes and degrade the antigens into peptide fragments restricted to both MHC class I and II. MHC class II specific peptides bind to MHC class II molecules by replacing the class II-associated invariant chain peptide (23). This peptide-MHC class II complex will get presented to the cell surface and activates naïve CD4+ T cells. However, if the peptides are MHC class I specific, they will get cross-presented to proteosomes and follows MHC class-I pathway to expand cytotoxic CD8+ T cells (14). Even though the cross-presentation of soluble antigen was detected in vivo, it is not very efficient in generating strong immune responses (24). However, unlike soluble antigens, particulate antigens are sustainably presented by APCs in large quantities for a prolonged time resulting in enhanced immunogenicity (25).
Antigen delivery platforms and their immunological properties
Virus-like particles (VLPs)
Virus-like particles (VLPs) are nanostructures made up of self-assembled virus proteins. VLPs do not contain viral genomes and thus they do not have the capacity of infecting the host cell (26). VLPs can be generated by using bacterial, yeast, insect, plant, and mammalian cells (27). It has been reported that VLPs can serve as carriers for the delivery of various biomaterials and nanomaterials including vaccines (Figure 2) (26, 28, 29). Currently, VLP-based vaccines against human papillomavirus, hepatitis B virus and malaria are commercially available (Table 1) (26, 30).
VLP-based vaccines are highly immunogenic and can induce both cellular and humoral immune responses and the use of adjuvants enhances their immunogenicity (32). VLPs were found to strongly activate DCs (32). DCs are well-known as the most effective APCs and have the ability to activate both naϊve and memory immune responses (52). Activation of DCs takes place owing to the binding of VLPs with the receptors that are present on the surface of DCs known as pattern recognition receptors (PRRs) such as Toll-like receptors (TLRs) (32, 53). VLP-based vaccines are then recognized and internalized by DCs and get presented to CD4+ helper T cells and cytotoxic CD8+ T cells via MHC class II and MHC class I molecules, respectively (32, 54). VLPs allow presentation of multiple copies of epitopes on their surface, which mediate cross-linking of B cell receptors and subsequent priming B cells toward generation of antibodies even without the help of CD4+ T helper cells (32). VLP-based vaccines are regarded safer as compared to attenuated or inactivated viruses. Nonetheless, instability of VLPs can compromise their immunogenicity and the production cost of some VLP-based vaccines can be high because of their low yields (28, 31).
Viral vector vaccines (VVVs)
Viral vectors are considered as an advanced approach for the development of vaccines. Viral vectors have been used to produce vaccines against multiple infectious diseases including SARS-CoV-2, Ebola, Malaria, and HIV (34, 55). Viral vector vaccines (VVVs) comprise a harmless, modified, and unrelated virus that delivers foreign genetic material (DNA) to human cells, which then produce the pathogen-specific antigens encoded by the DNA (33). Moreover, VVVs offer multiple advantages as compared to other vaccine platforms including long-lasting immune responses, high immunogenicity without the need for adjuvant co-administration, and strong cellular and antibody responses (34).
Viral vectors such as based on adenovirus, poxvirus, influenza viruses, and measles virus are currently widely considered for the development of VVVs (56). Among them, the adenoviral vector has been used extensively to develop COVID-19 vaccines (56, 57). VVVs can induce long-lasting and strong cellular responses to eliminate virus-infected cells. Cytotoxic CD8+ T cells rapidly proliferate and differentiate in response to antigens, while cell-mediated immunity induces natural killer cells and macrophages to eliminate intracellular pathogens (58). On the other hand, most of the VVVs can induce powerful humoral immunity, however it has been observed that immunogen design can affect VVV-mediated humoral immunogenicity (58). For example, Huang et al. (59) revealed that immunizing mice or hamsters with SARS-CoV-2 spike proteins lacking glycan shields induced potent and broadly reactive immune responses. Despite many advantages, the production process of VVVs is comparatively expensive and highly complex. Moreover, VVVs could pose a risk for the environment and human health (35).
Messenger RNA (mRNA) vaccines
Messenger RNA (mRNA) vaccines have already been found highly effective against various infectious diseases (36, 60). There are several advantages offered by mRNA vaccines including rapid development, and a cell-free manufacturing process (36). mRNA vaccine technology involves delivering a transcript of interest that encodes a target antigen (37). There are two categories of mRNA vaccines including self-amplifying and non-replicating mRNA vaccines (61). mRNA vaccines precisely encode the specific antigen of interest, and then cells that take up the mRNA can translate it into proteins (62, 63). Subsequently, the immune system mounts robust adaptive immune responses against the target proteins (63). mRNA vaccines are safer and more effective than DNA-based vaccines, since mRNA vaccines do not require nuclear entry and they do not pose a risk of integration into the host genome (38–40). In addition, since the outbreak of the COVID-19 pandemic, there is a growing research interest in mRNA vaccines because of their capacity to trigger strong immune responses, rapid development, and simple manufacturing process (64). Currently approved mRNA COVID-19 vaccines include Moderna (mRNA-1273) and Pfizer-BioNTech (BNT162b2) (41–43).
mRNA vaccines contain intrinsic self-adjuvant properties and exert potent cellular and humoral immune responses (65). Hence mRNA vaccines have the potential to induce both innate and adaptive immune responses. mRNA vaccines also generated strong immune responses in experimental animal models of various infectious diseases including rabies, Zika virus disease, and influenza (66, 67). Following the delivery of mRNA vaccines in the body, they are taken up by APCs such as DCs or macrophages. Subsequently, they escape the endosome and enter into the cytosol, where mRNA is translated into protein by the ribosome (68). In the lymph node, antigen derived peptides are presented via MHC class I or II to cytotoxic CD8+ T cells or CD4+ T cells, which ultimately results in the activation of cell-mediated and humoral immune responses (69). However, there are some drawbacks of mRNA vaccines that need to be addressed to develop more effective, stable, and safe mRNA vaccines. These drawbacks include limited transfection efficiency, degradation of free mRNA via nucleases, and degradation of exogenous naked mRNA in the endolysosomal compartments (44–46). In addition, rare evidence was found that severe anaphylaxis and myocarditis occurred after immunization with mRNA-containing lipid nanoparticles (mRNA-LNP). It is believed that the lipid component, polyethylene glycol (PEG) 2000, in the vaccine formulation causes anaphylactic shock, especially in those who already have pre-existing anti-PEG antibodies (70).
Polymeric vaccines
There is a growing interest in polymer-based vaccines because of their several beneficial properties including induction of enhanced levels of antigen-specific antibodies, extended antigen circulation, co-loading of antigens, elevated level of cytokine release, and potent cellular immune responses (47). Polymer-based particles can be used as vaccine platforms to deliver numerous antigenic molecules including nucleic acids, carbohydrates, cell lysates, lipopeptides, peptides, and proteins (71). Polymer-based particles have great potential in the prevention and treatment of infectious diseases (51). Lipid- and polymer-based NPs have already been extensively studied in vaccine development. NPs have the capacity to induce strong cellular and humoral immune responses owing to their unique properties including their pathogen mimicking size, controllable lipophilicity/hydrophobicity, modifiable surfaces, and high surface-to-volume ratios (48).
There are two major groups of biodegradable polymers such as natural and synthetic polymers. A range of both synthetic and natural biodegradable polymers have already been studied for the development of vaccines. Naturally occurring biomaterials including various proteins including silk, fibrin, and collagen, and several polysaccharides including hyaluronic acid derivatives, chitosan, alginate, and starch have widely been studied to prepare particulate vaccine formulations (72). On the other hand, many synthetic biodegradable polymers including polyanhydrides, polyphosphazene, and various polyesters including poly(lactic-co-glycolic acid), polyglycolide, polycaprolactone, and polylactides have been studied as potential vaccine delivery systems (73).
Polyhydroxyalkanoates (PHAs) have been identified as biocompatible and biodegradable biopolymers and that are produced by a range of bacteria serving as energy and carbon storage materials (74). PHAs have drawn a lot of attention because of their potential as particulate vaccines in delivering various antigens from diverse pathogens (75). Furthermore, PHA particles displaying one or more antigens already exhibited improved cell-mediated and humoral immune responses when compared to the corresponding soluble subunit vaccines (49). Extensive animal trials have already confirmed that PHA particle vaccine candidates are safe and have the potential to induce robust and protective immune responses against bacterial and viral pathogens (Table 2) (11, 76, 78, 79, 85). Despite the great potential of polymeric nanostructured vaccines, further clinical studies are needed towards regulatory approval (51).
In a recent study, the immunogenic carrier protein, CRM197 (non-toxic variant diphtheria toxin) was engineered for the assembly of antigen-containing submicron-sized particles (86). Chen et al. (2022) developed a bioprocess for high-yield production via efficient assembly inside engineered endotoxin-free Escherichia coli. These particles were ambient-temperature stable and were precision engineered to serve as vaccine candidates that induce protective immunity against SARS-CoV-2, Mycobacterium tuberculosis and group A Streptococcus, respectively. The study demonstrated the utility and versatility of engineering immunogenic carrier proteins, that have been approved in vaccine formulation, into a synthetic particulate vaccine for induction of functional humoral and T cell-mediated immune responses (86).
Strategies to enhance immunogenicity of particulate vaccines
The ultimate aim of vaccination is to induce protective immunity against pathogens (10). The addition of adjuvants can further enhance and/or modulate the immunogenicity of particulate vaccines resulting in enhanced vaccine performance (1, 84). Induction of protective immunity requires effective priming of T and B cells (10, 59, 87). The activation and effector phases of T cell-mediated responses require two signals, “signal one” through T cell receptor recognition of peptide-MHC complexes and “signal two” via costimulatory receptors on T cells. Both signals are provided by APCs that encounter pathogens (1). Adjuvants can stimulate the activation and maturation of APCs, such as DCs, and thus enhance the expression of MHC and co-stimulatory molecules, which are essential for the induction of adaptive immunity (1, 10). Adjuvants are usually categorized as the delivery system (such as lipid vesicles) or immunostimulatory molecules (such as PRR ligands) based on their proposed mechanism. Most adjuvants possess both properties (88). For example, alum salts promote Th2-type immunity and B cell differentiation, leading to tremendous antibody production (78). Adjuvant System 04 (AS04) is a combination adjuvant, consisting of alum salts and TLR4 ligand monophosphoryl lipid A, which respectively upregulate potent humoral (Th2) and cellular (Th1) immune responses (Figure 2) (88).
As PRR ligands enable innate recognition by PRR, such as TLRs, present on DCs, the fabrication of particulate vaccines with PRR ligands can lead to targeted delivery to DCs and enhance the effectiveness of particulate vaccines (89). This enhancement is achieved by inducing “signal two” through the expression of co-stimulatory molecules on DCs, which augments T-cell responses (89). Thus coupling PRR ligands with antigens confer innate activation and antigenic stimulation to the same DC that uptakes the particulate vaccine. This lead to the generation of both signals, MHC-peptide complex and co-stimulatory signal, required for T-cell activation (1). In addition, targeted delivery to DCs can alternatively be achieved through the co-delivery of the CD40 ligand with antigen. The ligation of the CD40 ligand with the CD40 receptor on DCs serves as a key co-stimulator for DCs maturation and induction of CD4+ T cell responses (90).
The ability of DCs to present extracellular antigens in the context of MHC class I, a phenomenon called cross-presentation, can be an ideal target for the targeted delivery of vaccine antigens (20). Adjuvants such as MF59 and QS-21 in combination with TLR ligands can facilitate cytosolic or vacuole pathways of cross-presentation (91). In addition, cell-penetrating peptides (CPPs) also known as protein translocation domains or membrane translocating sequences, are small peptides with strong membrane permeability. CPPs are comprised of 6 to 30 amino acid residues and majority of them are basic amino acid residues, leading to an overall positive net charge (92–94). Recently the use of arginine- and lysine-rich CPPs in conjunction with particulate vaccines promotes the MHC class I pathway cross-presentation effectively against viral and tumor antigens (20, 92, 93). Moreover, DC targeting peptides (DCpep) are strongly targeted to DCs. They can improve vaccine capture efficiency, and promote DCs maturation, cytokine secretion, and T cell proliferation. Vaccines with DCpep can significantly induce stronger immune responses than the vaccines without DCpep (95). For example, Clec9A is produced on various DC subsets, such as mouse CD8a+ DCs and CD103+ DCs, and responsible for antigen cross-presentation. CBP-12 has high affinity with Clec9A on DCs. Vaccines with CBP-12 has been shown to elicit both strong cytotoxic CD8+ T cell and antibody responses (96, 97).
Particulate vaccine fabrication strategies
Post-translational modifications (PTMs) regulate function of proteins including antigens and contribute to their immunological properties and stability (98). A study showed that PTMs are often required for antigens to induce functional immune responses (98). Over the last decade, an increasing number of PTMs have been detected and characterized in E. coli, and most PTMs are rarely found in bacterial antigens with the majority of modified antigens having a low sub-stoichiometric degree of modification (99). As a result, retaining the conformation and function of eukaryotic multi-domain antigens produced in E. coli is more challenging (100).
Although the E. coli expression system is commonly used to produce recombinant protein vaccines, mammalian or insect cells should be considered for antigens that require high levels of PTMs that are required for vaccine purposes (98, 101). These post-translationally modified complex antigens can then be incorporated into particulate platforms using a variety of methods (Figure 2). Non-covalent methods include the use of peptide tags like polyhistidine, protein tags such as maltose-binding protein and glutathione- S-transferase, DNA-directed immobilization, and the biotin–streptavidin pair (102). One of the most powerful non-covalent biological interactions known is the binding of biotin to avidin (103). Purified post-translationally modified complex antigens that have been biotin labelled are therefore highly effective for protein capture on particulate platforms for vaccine delivery, and the biotinylated antigens are subsequently recognized by avidin/streptavidin. Although chemical biotinylation frequently results in heterogeneous products with impaired function (104), enzymatic biotinylation with E. coli biotin ligase (BirA) attaches biotin to the 15 amino acid avidin tag (AviTag) peptide yielding a homogeneous product with a high yield (105). Streptavidin is used as a potent immunostimulant in less immunogenic antigen-based vaccines, most notably cancer vaccines, in addition to binding to the biotinylated antigen (106).
Although the interaction between streptavidin and biotin is strong, the binding can be destroyed by molecular motors (such as FtsK) in seconds (107) or shear forces in milliseconds (108), making it challenging to implement barriers or locks in cellular systems. Vaccine stability is required not just during storage but also following injection, where the lower concentration and other circumstances, such as endosomal pH and shear stress in the circulation, may mediate the dissociation of non-covalently attached antigens from particles (109). Therefore, covalent interaction between the target antigen and carriers is considered as a distinct, more robust, and long-lasting method of attaching antigens to the surface of an antigen carrier. Covalent linkage to peptide tags can be accomplished using SortaseA, SNAP-tag, split inteins, HaloTag, click reactions and Electrostatic Interaction Locks; however, SpyCatcher/SpyTag technology is most commonly used in vaccine delivery because SpyCatcher forms intermolecular isopeptide bonds selectively and spontaneously with SpyTag without the need for additional enzymes or chemical catalysis (102, 105). Furthermore, in comparison, SpyCatcher/SpyTag chemistry is reactive at the terminal and internal sites of a protein and can improve protein stability without changing its function (110). Thus, the bacterial production host can be bioengineered to produce vaccine particles displaying SpyCatcher to enable specific immobilization of SpyTag-fused target antigens with proper PTMs produced by such as mammalian or insect cell cultures (111, 112). However, several limitations typically may restrict the use of SpyCatcher/SpyTag in vaccine delivery. The final construct contains around 17 kDa molecular scar left by SpyCatcher/SpyTag coupling unlike the sortaseA with a smaller scar or split intein with no scar. Moreover, SpyCatcher/SpyTag is derived from Streptococcus pyogenes. The potential immunogenicity problem related to its bacterial origin may be an issue for vaccine design (113).
Conclusion and future perspectives
Antigens delivered in particulate form show superior immunological properties when compared to corresponding antigens in soluble forms. APCs, such as DCs, can cross-present antigens taken up in particulate form to potently activate both cytotoxic CD8+ and CD4+ T cells. Particulate vaccines are versatile as they can be formulated with adjuvants and/or bioengineered for the co-delivery of antigens with PRR ligands, CPPs, and DCpep for induction of protective immunity. Immobilizing antigens to particulate carriers significantly enhances their stability such as enabling the generation of ambient-temperature stable vaccine formulations. Bacterial production hosts are unable to produce complex antigens with high levels of PTMs. This review highlighted the advances of using various technologies via covalent and non-covalent attachments to incorporate antigens with PTMs on particles. Although particle vaccines possess a great promise to combat infectious diseases, there are still a number of unknowns. These include a profound understanding of how particle size, charge, and structure influence the induction of immune responses. Safety concerns, such as severe anaphylaxis and myocarditis, have also been raised due to the extensive use of some new nanoparticle vaccines, such as mRNA-LNP. Understanding the underlying mechanisms of nanoparticle vaccine properties and potential toxicity could significantly advance the rational design of prophylactic and therapeutic nanoparticle vaccines.
Author contributions
All authors contributed to the manuscript. SC and BR conceived and revised the manuscript. All authors contributed to the article and approved the submitted version.
Acknowledgments
The authors thank Katharina Gabriela Spaniol, at Griffith University, to provide Saranya Pounraj access to Biorender to draw a licensed figure. This work is supported by the Griffith Institute for Drug Discovery, Griffith University (Australia) and the Centre for Cell Factories and Biopolymers, Griffith University (Australia). BR acknowledges financial support from the award of the Australian Research Council (ARC) Discovery Project (DP200100874) and the ARC Linkage Infrastructure, Equipment and Facilities (LE200100140).
Conflict of interest
Author BHAR is an inventor of the biopolymer particle technology and co-founder/shareholder of Polybatics Ltd. BHAR and SC are inventors on a patent application describing the immunogenic carrier protein CRM197 vaccine platform and its uses.
The remaining authors declare that the research was conducted in the absence of any commercial or financial relationships that could be construed as a potential conflict of interest.
Publisher’s note
All claims expressed in this article are solely those of the authors and do not necessarily represent those of their affiliated organizations, or those of the publisher, the editors and the reviewers. Any product that may be evaluated in this article, or claim that may be made by its manufacturer, is not guaranteed or endorsed by the publisher.
References
1. Gonzalez-Miro M, Chen S, Gonzaga ZJ, Evert B, Wibowo D, Rehm BH. Polyester as antigen carrier toward particulate vaccines. Biomacromolecules (2019) 20(9):3213–32. doi: 10.1021/acs.biomac.9b00509
2. Tao P, Zhu J, Mahalingam M, Batra H, Rao VB. Bacteriophage T4 nanoparticles for vaccine delivery against infectious diseases. Adv Drug Deliv Rev (2019) 145:57–72. doi: 10.1016/j.addr.2018.06.025
3. Seyfoori A, Shokrollahi Barough M, Mokarram P, Ahmadi M, Mehrbod P, Sheidary A, et al. Emerging advances of nanotechnology in drug and vaccine delivery against viral associated respiratory infectious diseases (Varid). Int J Mol Sci (2021) 22(13):6937. doi: 10.3390/ijms22136937
4. Pielenhofer J, Sohl J, Windbergs M, Langguth P, Radsak MP. Current progress in particle-based systems for transdermal vaccine delivery. Front Immunol (2020) 11:266. doi: 10.3389/fimmu.2020.00266
5. Snapper CM. Distinct immunologic properties of soluble versus particulate antigens. Front Immunol (2018) 9:598. doi: 10.3389/fimmu.2018.00598
6. Jhaveri J, Raichura Z, Khan T, Momin M, Omri A. Chitosan nanoparticles-insight into properties, functionalization and applications in drug delivery and theranostics. Molecules (2021) 26(2):272. doi: 10.3390/molecules26020272
7. Gala RP, Bajaj L, Bansal A, Gomes KB, Joshi D, Menon I, et al. Oral vaccine delivery: The coming age of particulate vaccines to elicit mucosal immunity. Mucosal Deliv Drugs Biol Nanoparticles Springer (2020) . p:155–75. doi: 10.1007/978-3-030-35910-2_7
8. O’Hagan DT, Lodaya RN, Lofano G. The continued advance of vaccine adjuvants–’We can work it out’. In: Seminars in immunology. Elsevier (2020). doi: 10.1016/j.smim.2020.101426
9. Evert BJ, Chen S, McConville R, Steel RW, Healer J, Boddey JA, et al. Epitope-coated polymer particles elicit neutralising antibodies against plasmodium falciparum sporozoites. NPJ Vaccines (2021) 6(1):1–12. doi: 10.1038/s41541-021-00408-2
10. Wibowo D, Jorritsma SH, Gonzaga ZJ, Evert B, Chen S, Rehm BH. Polymeric nanoparticle vaccines to combat emerging and pandemic threats. Biomaterials (2021) 268:120597. doi: 10.1016/j.biomaterials.2020.120597
11. Chen S, Quan DH, Wang XT, Sandford S, Kirman JR, Britton WJ, et al. Particulate mycobacterial vaccines induce protective immunity against tuberculosis in mice. Nanomaterials (2021) 11(8):2060. doi: 10.3390/nano11082060
12. Tang S, Liu Z, Xu W, Li Q, Han T, Pan D, et al. Versatile functionalization of ferritin nanoparticles by intein-mediated trans-splicing for antigen/adjuvant co-delivery. Nano Lett (2019) 19(8):5469–75. doi: 10.1021/acs.nanolett.9b01974
13. Huang P, Wang X, Liang X, Yang J, Zhang C, Kong D, et al. Nano-, micro-, and macroscale drug delivery systems for cancer immunotherapy. Acta Biomater (2019) 85:1–26. doi: 10.1016/j.actbio.2018.12.028
14. Burgdorf S, Kurts C. Endocytosis mechanisms and the cell biology of antigen presentation. Curr Opin Immunol (2008) 20(1):89–95. doi: 10.1016/j.coi.2007.12.002
15. Blander JM. Regulation of the cell biology of antigen cross presentation. Annu Rev Immunol (2018) 36:717. doi: 10.1146/annurev-immunol-041015-055523
16. Taefehshokr N, Yin C, Heit B. Rab gtpases in the differential processing of phagocytosed pathogens versus efferocytosed apoptotic cells. Histol Histopathol (2021) 36:123–35. doi: 10.14670/HH-18-252
17. Desjardins M. Antigen cross-presentation: Proteasome location, location, location. EMBO J (2019) 38(16):e102799. doi: 10.15252/embj.2019102799
18. Fountain A, Inpanathan S, Alves P, Verdawala MB, Botelho RJ. Phagosome maturation in macrophages: Eat, digest, adapt, and repeat. Adv Biol Regul (2021) 82:100832. doi: 10.1016/j.jbior.2021.100832
19. Cruz FM, Colbert JD, Rock KL. The gtp ase Rab39a promotes phagosome maturation into mhc-I antigen-presenting compartments. EMBO J (2020) 39(2):e102020. doi: 10.15252/embj.2019102020
20. Wylie B, Ong F, Belhoul-Fakir H, Priebatsch K, Bogdawa H, Stirnweiss A, et al. Targeting cross-presentation as a route to improve the efficiency of peptide-based cancer vaccines. Cancers (Basel) (2021) 13(24). doi: 10.3390/cancers13246189
21. Donahue ND, Acar H, Wilhelm S. Concepts of nanoparticle cellular uptake, intracellular trafficking, and kinetics in nanomedicine. Adv Drug Deliv Rev (2019) 143:68–96. doi: 10.1016/j.addr.2019.04.008
22. Liu Z-H, Deng Z-F, Lu Y, Fang W-H, He F. A modular and self-adjuvanted multivalent vaccine platform based on porcine circovirus virus-like nanoparticles. J Nanobiotechnol (2022) 20(1):1–17. doi: 10.1186/s12951-022-01710-4
23. Roche PA, Furuta K. The ins and outs of mhc class ii-mediated antigen processing and presentation. Nat Rev Immunol (2015) 15(4):203–16. doi: 10.1038/nri3818
24. Shen H, Ackerman AL, Cody V, Giodini A, Hinson ER, Cresswell P, et al. Enhanced and prolonged cross-presentation following endosomal escape of exogenous antigens encapsulated in biodegradable nanoparticles. Immunology (2006) 117(1):78–88. doi: 10.1111/j.1365-2567.2005.02268.x
25. Singh B, Maharjan S, Cho K-H, Cui L, Park I-K, Choi Y-J, et al. Chitosan-based particulate systems for the delivery of mucosal vaccines against infectious diseases. Int J Biol Macromol (2018) 110:54–64. doi: 10.1016/j.ijbiomac.2017.10.101
26. Nooraei S, Bahrulolum H, Hoseini ZS, Katalani C, Hajizade A, Easton AJ, et al. Virus-like particles: Preparation, immunogenicity and their roles as nanovaccines and drug nanocarriers. J Nanobiotechnol (2021) 19(1):59. doi: 10.1186/s12951-021-00806-7
27. Mejía-Méndez JL, Vazquez-Duhalt R, Hernández LR, Sánchez-Arreola E, Bach H. Virus-like particles: Fundamentals and biomedical applications. Int J Mol Sci (2022) 23(15):8579. doi: 10.3390/ijms23158579
28. Tariq H, Batool S, Asif S, Ali M, Abbasi BH. Virus-like particles: Revolutionary platforms for developing vaccines against emerging infectious diseases. Front Microbiol (2021) 12:790121. doi: 10.3389/fmicb.2021.790121
29. Biela AP, Naskalska A, Fatehi F, Twarock R, Heddle JG. Programmable polymorphism of a virus-like particle. Commun Mater (2022) 3(1):7. doi: 10.1038/s43246-022-00229-3
30. Donaldson B, Lateef Z, Walker GF, Young SL, Ward VK. Virus-like particle vaccines: Immunology and formulation for clinical translation. Expert Rev Vaccines (2018) 17(9):833–49. doi: 10.1080/14760584.2018.1516552
31. Lomonossoff GP, Ponndorf D. Biotechnology approaches to modern vaccine design. In: Bamford DH, Zuckerman M, editors. Encyclopedia of virology, 4th ed. Oxford: Academic Press (2021). p. 662–70.
32. Zepeda-Cervantes J, Ramírez-Jarquín JO, Vaca L. Interaction between virus-like particles (Vlps) and pattern recognition receptors (Prrs) from dendritic cells (Dcs): Toward better engineering of vlps. Front Immunol (2020) 11:1100. doi: 10.3389/fimmu.2020.01100
33. Chavda VP, Bezbaruah R, Athalye M, Parikh PK, Chhipa AS, Patel S, et al. Replicating viral vector-based vaccines for covid-19: Potential avenue in vaccination arena. Viruses (2022) 14(4). doi: 10.3390/v14040759
34. Travieso T, Li J, Mahesh S, Mello JDFRE, Blasi M. The use of viral vectors in vaccine development. NPJ Vaccines (2022) 7(1):75. doi: 10.1038/s41541-022-00503-y
35. Rauch S, Jasny E, Schmidt KE, Petsch B. New vaccine technologies to combat outbreak situations. Front Immunol (2018) 9:1963. doi: 10.3389/fimmu.2018.01963
36. Zhang C, Maruggi G, Shan H, Li J. Advances in mrna vaccines for infectious diseases. Front Immunol (2019) 10:594. doi: 10.3389/fimmu.2019.00594
37. Park JW, Lagniton PNP, Liu Y, Xu RH. Mrna vaccines for covid-19: What, why and how. Int J Biol Sci (2021) 17(6):1446–60. doi: 10.7150/ijbs.59233
38. Rosa SS, Prazeres DMF, Azevedo AM, Marques MPC. Mrna vaccines manufacturing: Challenges and bottlenecks. Vaccine (2021) 39(16):2190–200. doi: 10.1016/j.vaccine.2021.03.038
39. Kim J, Eygeris Y, Gupta M, Sahay G. Self-assembled mrna vaccines. Adv Drug Delivery Rev (2021) 170:83–112. doi: 10.1016/j.addr.2020.12.014
40. Domazet-Lošo T. Mrna vaccines: Why is the biology of retroposition ignored? Genes (Basel) (2022) 13(5). doi: 10.3390/genes13050719
41. Barda N, Dagan N, Cohen C, Hernán MA, Lipsitch M, Kohane IS, et al. Effectiveness of a third dose of the Bnt162b2 mrna covid-19 vaccine for preventing severe outcomes in Israel: An observational study. Lancet (2021) 398(10316):2093–100. doi: 10.1016/s0140-6736(21)02249-2
42. Tang P, Hasan MR, Chemaitelly H, Yassine HM, Benslimane FM, Al Khatib HA, et al. Bnt162b2 and mrna-1273 covid-19 vaccine effectiveness against the sars-Cov-2 delta variant in Qatar. Nat Med (2021) 27(12):2136–43. doi: 10.1038/s41591-021-01583-4
43. Walter EB, Talaat KR, Sabharwal C, Gurtman A, Lockhart S, Paulsen GC, et al. Evaluation of the Bnt162b2 covid-19 vaccine in children 5 to 11 years of age. N Engl J Med (2022) 386(1):35–46. doi: 10.1056/NEJMoa2116298
44. Chen M, Hu X, Liu S. Next-generation nonviral vectors for mrna vaccine delivery. Macromol Chem Phys (2022) 223(5):2100443. doi: 10.1002/macp.202100443
45. Esteban I, Pastor-Quiñones C, Usero L, Plana M, García F, Leal L. In the era of mrna vaccines, is there any hope for hiv functional cure? Viruses (2021) 13(3). doi: 10.3390/v13030501
46. Fan Y-N, Li M, Luo Y-L, Chen Q, Wang L, Zhang H-B, et al. Cationic lipid-assisted nanoparticles for delivery of mrna cancer vaccine. Biomater Sci (2018) 6(11):3009–18. doi: 10.1039/C8BM00908B
47. Pippa N, Gazouli M, Pispas S. Recent advances and future perspectives in polymer-based nanovaccines. Vaccines (Basel) (2021) 9(6). doi: 10.3390/vaccines9060558
48. Milovanovic M, Arsenijevic A, Milovanovic J, Kanjevac T, Arsenijevic N. Chapter 14 - nanoparticles in antiviral therapy. In: Grumezescu AM, editor. Antimicrobial nanoarchitectonics. Elsevier (2017). p. 383–410. doi: 10.1016/B978-0-323-52733-0.00014-8
49. Rehm BHA. Bioengineering towards self-assembly of particulate vaccines. Curr Opin Biotechnol (2017) 48:42–53. doi: 10.1016/j.copbio.2017.03.018
50. Han J, Zhao D, Li D, Wang X, Jin Z, Zhao K. Polymer-based nanomaterials and applications for vaccines and drugs. Polymers (Basel) (2018) 10(1). doi: 10.3390/polym10010031
51. Guo S, Fu D, Utupova A, Sun D, Zhou M, Jin Z, et al. Applications of polymer-based nanoparticles in vaccine field. Nanotechnol Rev (2019) 8(1):143–55. doi: 10.1515/ntrev-2019-0014
52. Sabado RL, Bhardwaj N. Directing dendritic cell immunotherapy towards successful cancer treatment. Immunotherapy (2010) 2(1):37–56. doi: 10.2217/imt.09.43
53. Sartorius R, Trovato M, Manco R, D’Apice L, De Berardinis P. Exploiting viral sensing mediated by toll-like receptors to design innovative vaccines. NPJ Vaccines (2021) 6(1):127. doi: 10.1038/s41541-021-00391-8
54. Keikha R, Daliri K, Jebali A. The use of nanobiotechnology in immunology and vaccination. Vaccines (Basel) (2021) 9(2). doi: 10.3390/vaccines9020074
55. Vanaparthy R, Mohan G, Vasireddy D, Atluri P. Review of covid-19 viral vector-based vaccines and covid-19 variants. Infez Med (2021) 29(3):328–38. doi: 10.53854/liim-2903-3
56. Humphreys IR, Sebastian S. Novel viral vectors in infectious diseases. Immunology (2018) 153(1):1–9. doi: 10.1111/imm.12829
57. Lee CS, Bishop ES, Zhang R, Yu X, Farina EM, Yan S, et al. Adenovirus-mediated gene delivery: Potential applications for gene and cell-based therapies in the new era of personalized medicine. Genes Dis (2017) 4(2):43–63. doi: 10.1016/j.gendis.2017.04.001
58. Deng S, Liang H, Chen P, Li Y, Li Z, Fan S, et al. Viral vector vaccine development and application during the covid-19 pandemic. Microorganisms (2022) 10(7):1450. doi: 10.3390/microorganisms10071450
59. Huang HY, Liao HY, Chen X, Wang SW, Cheng CW, Shahed-Al-Mahmud M, et al. Vaccination with sars-Cov-2 spike protein lacking glycan shields elicits enhanced protective responses in animal models. Sci Transl Med (2022) 14(639):eabm0899. doi: 10.1126/scitranslmed.abm0899
60. Hogan MJ, Pardi N. Mrna vaccines in the covid-19 pandemic and beyond. Annu Rev Med (2022) 73:17–39. doi: 10.1146/annurev-med-042420-112725
61. Heine A, Juranek S, Brossart P. Clinical and immunological effects of mrna vaccines in malignant diseases. Mol Cancer (2021) 20(1):52. doi: 10.1186/s12943-021-01339-1
62. Rijkers GT, Weterings N, Obregon-Henao A, Lepolder M, Dutt TS, van Overveld FJ, et al. Antigen presentation of mrna-based and virus-vectored sars-Cov-2 vaccines. Vaccines (Basel) (2021) 9(8). doi: 10.3390/vaccines9080848
63. Bettini E, Locci M. Sars-Cov-2 mrna vaccines: Immunological mechanism and beyond. Vaccines (Basel) (2021) 9(2). doi: 10.3390/vaccines9020147
64. Fang E, Liu X, Li M, Zhang Z, Song L, Zhu B, et al. Advances in covid-19 mrna vaccine development. Signal Transduct Target Ther (2022) 7(1):94. doi: 10.1038/s41392-022-00950-y
65. Linares-Fernández S, Lacroix C, Exposito J-Y, Verrier B. Tailoring mrna vaccine to balance Innate/Adaptive immune response. Trends Mol Med (2020) 26(3):311–23. doi: 10.1016/j.molmed.2019.10.002
66. Nitika, Wei J, Hui A-M. The development of mrna vaccines for infectious diseases: Recent updates. Infect Drug Resistance (2021) 14:5271–85. doi: 10.2147/idr.s341694
67. Le T, Sun C, Chang J, Zhang G, Yin X. Mrna vaccine development for emerging animal and zoonotic diseases. Viruses (2022) 14(2):401. doi: 10.3390/v14020401
68. Chaudhary N, Weissman D, Whitehead KA. Mrna vaccines for infectious diseases: Principles, delivery and clinical translation. Nat Rev Drug Discovery (2021) 20(11):817–38. doi: 10.1038/s41573-021-00283-5
69. Kowalski PS, Rudra A, Miao L, Anderson DG. Delivering the messenger: Advances in technologies for therapeutic mrna delivery. Mol Ther (2019) 27(4):710–28. doi: 10.1016/j.ymthe.2019.02.012
70. Vu MN, Kelly HG, Kent SJ, Wheatley AK. Current and future nanoparticle vaccines for covid-19. EBioMedicine (2021) 74:103699. doi: 10.1016/j.ebiom.2021.103699
71. Lambricht L, Peres C, Florindo H, Préat V, Vandermeulen G. Chapter ten - polymer-based nanoparticles as modern vaccine delivery systems. In: Skwarczynski M, Toth I, editors. Micro and nanotechnology in vaccine development. William Andrew Publishing (2017). p. 185–203. doi: 10.1016/B978-0-323-39981-4.00010-5
72. Song R, Murphy M, Li C, Ting K, Soo C, Zheng Z. Current development of biodegradable polymeric materials for biomedical applications. Drug Design Dev Ther (2018) 12:3117–45. doi: 10.2147/dddt.s165440
73. Bose RJ, Kim M, Chang JH, Paulmurugan R, Moon JJ, Koh WG, et al. Biodegradable polymers for modern vaccine development. J Ind Eng Chem (2019) 77:12–24. doi: 10.1016/j.jiec.2019.04.044
74. Miu DM, Eremia MC, Moscovici M. Polyhydroxyalkanoates (Phas) as biomaterials in tissue engineering: Production, isolation, characterization. Mater (Basel) (2022) 15(4). doi: 10.3390/ma15041410
75. Lee JW, Parlane NA, Wedlock DN, Rehm BH. Bioengineering a bacterial pathogen to assemble its own particulate vaccine capable of inducing cellular immunity. Sci Rep (2017) 7:41607. doi: 10.1038/srep41607
76. Gonzaga ZJC, Chen S, Lehoux M, Segura M, Rehm BHA. Engineering antigens to assemble into polymer particle vaccines for prevention of streptococcus suis infection. Vaccines (2021) 9(12):1386. doi: 10.3390/vaccines9121386
77. Evert BJ, Chen S, McConville R, Steel RWJ, Healer J, Boddey JA, et al. Epitope-coated polymer particles elicit neutralising antibodies against plasmodium falciparum sporozoites. NPJ Vaccines (2021) 6(1):141. doi: 10.1038/s41541-021-00408-2
78. Chen S, Evert B, Adeniyi A, Salla-Martret M, Lua LH-L, Ozberk V, et al. Ambient temperature stable, scalable covid-19 polymer particle vaccines induce protective immunity. Adv Healthcare Mater (2022) 11(3):2102089. doi: 10.1002/adhm.202102089
79. Gonzaga ZJC, Merakou C, DiGiandomenico A, Priebe GP, Rehm BHA. A pseudomonas aeruginosa-derived particulate vaccine protects against p. aeruginosa infection. Vaccines (2021) 9(7):803. doi: 10.3390/vaccines9070803
80. Gonzaga ZJC, Zhang J, Rehm BH. Intranasal delivery of antigen-coated polymer particles protects against pseudomonas aeruginosa infection. ACS Infect Dis (2022) 8(4):744–56. doi: 10.1021/acsinfecdis.1c00434
81. Parlane NA, Grage K, Lee JW, Buddle BM, Denis M, Rehm BHA. Production of a particulate hepatitis c vaccine candidate by an engineered lactococcus lactis strain. Appl Environ Microbiol (2011) 77(24):8516–22. doi: 10.1128/AEM.06420-11
82. Parlane NA, Grage K, Mifune J, Basaraba RJ, Wedlock DN, Rehm BHA, et al. Vaccines displaying mycobacterial proteins on biopolyester beads stimulate cellular immunity and induce protection against tuberculosis. Clin Vaccine Immunol (2012) 19(1):37–44. doi: 10.1128/CVI.05505-11
83. González-Miro M, Rodríguez-Noda L, Fariñas-Medina M, García-Rivera D, Vérez-Bencomo V, Rehm BHA. Self-assembled particulate psaa as vaccine against streptococcus pneumoniae infection. Heliyon (2017) 3(4):e00291. doi: 10.1016/j.heliyon.2017.e00291
84. González-Miró M, Rodríguez-Noda LM, Fariñas-Medina M, Cedré-Marrero B, Madariaga-Zarza S, Zayas-Vignier C, et al. Bioengineered polyester beads Co-displaying protein and carbohydrate-based antigens induce protective immunity against bacterial infection. Sci Rep (2018) 8(1):1888. doi: 10.1038/s41598-018-20205-7
85. Sheffee NS, Rubio-Reyes P, Mirabal M, Calero R, Carrillo-Calvet H, Chen S, et al. Engineered mycobacterium tuberculosis antigen assembly into core-shell nanobeads for diagnosis of tuberculosis. Nanomed: Nanotechnol Biol Med (2021) 34:102374. doi: 10.1016/j.nano.2021.102374
86. Chen S, Quan DH, Sam G, Ozberk V, Wang XT, Halfmann P, et al. Assembly of immunogenic protein particles toward advanced synthetic vaccines. Small (2022). doi: 10.1002/smll.202205819
87. Singh A. Eliciting b cell immunity against infectious diseases using nanovaccines. Nat Nanotechnol (2021) 16(1):16–24. doi: 10.1038/s41565-020-00790-3
88. Pulendran B, Arunachalam P S, O’Hagan DT. Emerging concepts in the science of vaccine adjuvants. Nat Rev Drug Discovery (2021) 20(6):454–75. doi: 10.1038/s41573-021-00163-y
89. Kaur A, Baldwin J, Brar D, Salunke DB, Petrovsky N. Toll-like receptor (Tlr) agonists as a driving force behind next-generation vaccine adjuvants and cancer therapeutics. Curr Opin Chem Biol (2022) 70:102172. doi: 10.1016/j.cbpa.2022.102172
90. Sharma PK, Dmitriev IP, Kashentseva EA, Raes G, Li L, Kim SW, et al. Development of an adenovirus vector vaccine platform for targeting dendritic cells. Cancer Gene Ther (2018) 25(1):27–38. doi: 10.1038/s41417-017-0002-1
91. Mosca F, Tritto E, Muzzi A, Monaci E, Bagnoli F, Iavarone C, et al. Molecular and cellular signatures of human vaccine adjuvants. Proc Natl Acad Sci (2008) 105(30):10501–6. doi: 10.1073/pnas.0804699105
92. Backlund CM, Holden RL, Moynihan KD, Garafola D, Farquhar C, Mehta NK, et al. Cell-penetrating peptides enhance peptide vaccine accumulation and persistence in lymph nodes to drive immunogenicity. Proc Natl Acad Sci (2022) 119(32):e2204078119. doi: 10.1073/pnas.2204078119
93. Silva S, Almeida AJ, Vale N. Combination of cell-penetrating peptides with nanoparticles for therapeutic application: A review. Biomolecules (2019) 9(1):22. doi: 10.3390/biom9010022
94. Hao M, Zhang L, Chen P. Membrane internalization mechanisms and design strategies of arginine-rich cell-penetrating peptides. Int J Mol Sci (2022) 23(16):9038. doi: 10.3390/ijms23169038
95. Xia T, Yang H, Guo Y, Guo T, Xin L, Jiang Y, et al. Human dendritic cell targeting peptide can be targeted to porcine dendritic cells to improve antigen capture efficiency to stimulate stronger immune response. Front Immunol (2022) 13:950597. doi: 10.3389/fimmu.2022.950597
96. Gou S, Wang S, Liu W, Chen G, Zhang D, Du J, et al. Adjuvant-free peptide vaccine targeting Clec9a on dendritic cells can induce robust antitumor immune response through Syk/Il-21 axis. Theranostics (2021) 11(15):7308. doi: 10.7150/thno.56406
97. Yan Z, Wu Y, Du J, Li G, Wang S, Cao W, et al. A novel peptide targeting Clec9a on dendritic cell for cancer immunotherapy. Oncotarget (2016) 7(26):40437. doi: 10.18632/oncotarget.9624
98. Ojha R, Prajapati VK. Cognizance of posttranslational modifications in vaccines: A way to enhanced immunogenicity. J Cell Physiol (2021) 236(12):8020–34. doi: 10.1002/jcp.30483
99. Macek B, Forchhammer K, Hardouin J, Weber-Ban E, Grangeasse C, Mijakovic I. Protein post-translational modifications in bacteria. Nat Rev Microbiol (2019) 17(11):651–64. doi: 10.1038/s41579-019-0243-0
100. Rosano GL, Ceccarelli EA. Recombinant protein expression in escherichia coli: Advances and challenges. Front Microbiol (2014) 5:172/BIBTEX(APR). doi: 10.3389/FMICB.2014.00172/BIBTEX
101. Nascimento IP, Leite LCC. Recombinant vaccines and the development of new vaccine strategies. Braz J Med Biol Res (2012) 45(12):1102. doi: 10.1590/S0100-879X2012007500142
102. Meldal M, Schoffelen S. Recent advances in covalent, site-specific protein immobilization. F1000Research (2016) 5. doi: 10.12688/F1000RESEARCH.9002.1
103. De Boer E, Rodriguez P, Bonte E, Krijgsveldt J, Katsantoni E, Heckt A, et al. Efficient biotinylation and single-step purification of tagged transcription factors in mammalian cells and transgenic mice. Proc Natl Acad Sci United States America (2003) 100(13):7480–5. doi: 10.1073/PNAS.1332608100/SUPPL_FILE/2608FIG5NEW.JPG
104. Predonzani A, Arnoldi F, López-Requena A, Burrone OR. In vivo site-specific biotinylation of proteins within the secretory pathway using a single vector system. BMC Biotechnol (2008) 8(1):1–11. doi: 10.1186/1472-6750-8-41/FIGURES/6
105. Fairhead M, Howarth M. Site-specific biotinylation of purified proteins using bira. Methods Mol Biol (Clifton NJ) (2015) 1266:171. doi: 10.1007/978-1-4939-2272-7_12
106. Jain A, Cheng K. The principles and applications of avidin-based nanoparticles in drug delivery and diagnosis. J Controlled Release (2017) 245:27–40. doi: 10.1016/j.jconrel.2016.11.016
107. Chivers CE, Crozat E, Chu C, Moy VT, Sherratt DJ, Howarth M. A streptavidin variant with slower biotin dissociation and increased mechanostability. Nat Methods (2010) 7(5):391–3. doi: 10.1038/nmeth.1450
108. Perumal SK, Raney KD, Benkovic SJ. Analysis of the DNA translocation and unwinding activities of T4 phage helicases. Methods (2010) 51(3):277–88. doi: 10.1016/j.ymeth.2010.02.011
109. Brune KD, Howarth M. New routes and opportunities for modular construction of particulate vaccines: Stick, click, and glue. Front Immunol (2018) 9:1432. doi: 10.3389/fimmu.2018.01432
110. Zakeri B, Fierer JO, Celik E, Chittock EC, Schwarz-Linek U, Moy VT, et al. Peptide tag forming a rapid covalent bond to a protein, through engineering a bacterial adhesin. Proc Natl Acad Sci (2012) 109(12):E690–E7. doi: 10.1073/pnas.1115485109
111. Lin Z, Lin Q, Li J, Pistolozzi M, Zhao L, Yang X, et al. Spy chemistry-enabled protein directional immobilization and protein purification. Biotechnol Bioengineering (2020) 117(10):2923–32. doi: 10.1002/BIT.27460
112. Tian J, Jia R, Wenge D, Sun H, Wang Y, Chang Y, et al. One-step purification and immobilization of recombinant proteins using Spytag/Spycatcher chemistry. Biotechnol Lett (2021) 43(5):1075–87. doi: 10.1007/S10529-021-03098-X
Keywords: particulate vaccine, cross-presentation, post-translational modification, dendritic cells, protective immunity, infectious diseases
Citation: Chen S, Pounraj S, Sivakumaran N, Kakkanat A, Sam G, Kabir MT and Rehm BHA (2023) Precision-engineering of subunit vaccine particles for prevention of infectious diseases. Front. Immunol. 14:1131057. doi: 10.3389/fimmu.2023.1131057
Received: 24 December 2022; Accepted: 23 January 2023;
Published: 03 February 2023.
Edited by:
Mahbuba Rahman, McMaster University, CanadaReviewed by:
Moza Alkowari, Hamad Medical Corporation, QatarSeetharama Konduru, Sidra Medicine, Qatar
Santosh Kumar Yadav, Johns Hopkins Medicine, United States
Copyright © 2023 Chen, Pounraj, Sivakumaran, Kakkanat, Sam, Kabir and Rehm. This is an open-access article distributed under the terms of the Creative Commons Attribution License (CC BY). The use, distribution or reproduction in other forums is permitted, provided the original author(s) and the copyright owner(s) are credited and that the original publication in this journal is cited, in accordance with accepted academic practice. No use, distribution or reproduction is permitted which does not comply with these terms.
*Correspondence: Bernd H. A. Rehm, b.rehm@griffith.edu.au; Shuxiong Chen, Shuxiong.chen@griffith.edu.au