- 1Department of Internal Medicine I, University Hospital Carl Gustav Carus, Technische Universität Dresden, Dresden, Germany
- 2Center for Regenerative Therapies (CRTD), Technische Universität Dresden, Dresden, Germany
- 3Department of Medicine, University of Cambridge, Cambridge, United Kingdom
T follicular helper cells comprise a specialized, heterogeneous subset of immune-competent T helper cells capable of influencing B cell responses in lymphoid tissues. In physiology, for example in response to microbial challenges or vaccination, this interaction chiefly results in the production of protecting antibodies and humoral memory. In the context of kidney transplantation, however, immune surveillance provided by T follicular helper cells can take a life of its own despite matching of human leukocyte antigens and employing the latest immunosuppressive regiments. This puts kidney transplant recipients at risk of subclinical and clinical rejection episodes with a potential risk for allograft loss. In this review, the current understanding of immune surveillance provided by T follicular helper cells is briefly described in physiological responses to contrast those pathological responses observed after kidney transplantation. Sensitization of T follicular helper cells with the subsequent emergence of detectable donor-specific human leukocyte antigen antibodies, non-human leukocyte antigen antibodies their implication for kidney transplantation and lessons learnt from other transplantation “settings” with special attention to antibody-mediated rejection will be addressed.
1 Introduction
T follicular helper (TFH) cells are a specialized CD4+ TH cell population critical for driving adaptive humoral immunity (1, 2), with B-cell lymphoma 6 (Bcl6) as a repressor of B-lymphocyte-induced maturation protein 1 (Blimp-1) driving their differentiation from CD4+ T helper cells (3–5). TFH cells instruct germinal center (GC) formation which is pivotal to T cell-dependent antibody responses and their affinity maturation (6, 7). Inducible T cell co-stimulator (ICOS), C-X-C motif chemokine receptor (CXCR)5, and programmed cell death protein 1 (PD-1) are distinguishing markers of TFH cells, which contribute to lymphoid tissue homing and B cell help (8–10). The expression of C-C motif chemokine receptor (CCR)7 instructs naïve T cell migration across high endothelial venules to locate to T cell zones. Upon activation by dendritic cells, a population of activated CD4+ T cells differentiates into TFH cells through the up-regulation of Bcl6 and CXCR5 with a concomitant down-regulation of CCR7 enabling them to advance toward the C-X-C motif ligand (CXCL)13-rich B cell area in lymphoid organs (11, 12). Here, Interleukin (IL)-21 and the strength of T cell antigen receptor binding determine a long-lasting antibody response through effector antibody-secreting cells (ASCs, plasma cells) and the emergence of memory B cell subsets residing in the bone marrow termed long-lived plasma cells (LLPCs) (13–18). To date, the family of TFH cell subtypes comprises virus-specific TFH-1 cells, centrocyte-stimulating TFH-2 cells, IL-17- and IL-21-producing TFH-17 cells, and T follicular regulatory (TFR) cells (Table 1) (27). TFH-13 cells were recently identified which appear to be associated to IgE production to food and airborne allergens (64, 65). Synergies between these TFH cell subsets with B cells provide unique and necessary cues that go beyond homeostatic B cell maturation and high-affinity antibody production (66, 67).
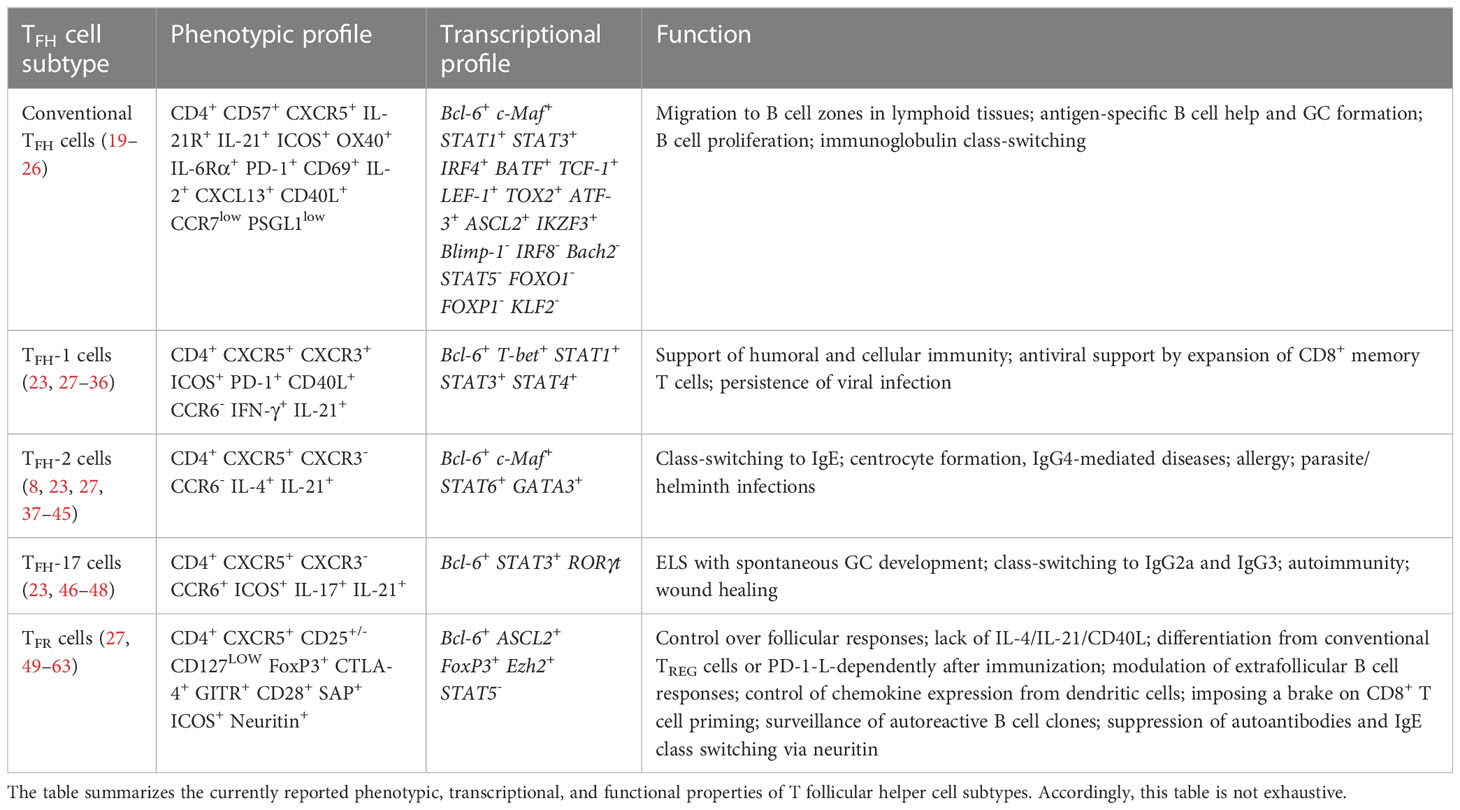
Table 1 A snapshot on T follicular cell subtypes and some aspects of their phenotypic, transcriptional, and functional characterization.
The TFH-B cell interaction influences TFH cells to maintain a lymph node migratory phenotype (68). Besides its auxiliary effects on B cells concerning isotype switching and the formation of GCs, IL-21 appears to support TFH cells in acquiring and maintaining TFH cell gene expression in vivo in an autocrine fashion (69–71). Under physiological conditions, the emergence of high-affinity, class-switched antibodies indicates a prosperous immune response aimed at facilitating the clearance of microbial invaders or are a means to measure successful seroconversion after vaccination (72, 73). Studies have shown that improved humoral responses were preceded by an enhanced ICOS expression on circulating TFH cells (74). As such, increased ICOS+CXCR3+CXCR5+CD4+ TFH cell numbers on day 7 after antigen stimulation appear to forecast a humoral response (75). Interestingly, serological response displays Cxcr5 and IL21 induction as early as the day of the antigenic challenge (day 0), instructs antigen-specific GC responses. A failing antigen-specific TFH cell response features increased expression of IL2 and STAT5 (76). Accordingly, spatiotemporal positioning between T and B cell zones within GCs appears pivotal. For example, FoxP3hiCD4+ regulatory T (TREG) cells are predominantly confined to extrafollicular areas, whereas both TFR cells and extrafollicular TREG cells in vaccinated children are reduced following vaccination suggesting a released break in both peripheral TH cell commitment towards TFH cells (extrafollicular response) and favored cognate B cell help by TFH cells within GCs (follicular response). Besides, the release of CXCL13 into the circulation reflects the ensuing GC reactivity and correlates between TFH cells and Ag-specific B cells in tonsils (77), which can be considered an early surrogate biomarker for an ensuing humoral response (77, 78).
For humoral immunity, the functionality of TFH cells is critical and can determine successful seroconversion. For example, during acute viral infections, fate commitment of a TFH cell-to-be is acquired as early as 24 to 48 hours after infection (79). If strong interactions with antigen-presenting dendritic cells (DCs) are maintained, TFH cell differentiation is facilitated permitting TFH cell lineage determination over TH-1 cell commitment via a balanced regulation of the transcription factors Bcl6, T-box expressed in T cells (T-bet), and Blimp-1 (2, 19, 79, 80). Also, Lee et al. have reported that the downregulation of Krüppel-like Factor 2 (KLF2) further promotes the inhibition of the TFH cell differentiation-opposing transcription factors Blimp1, T-bet, and GATA binding protein (GATA)3, while the inhibition of Cxcr5 transcription is withdrawn (81). IL-10 can affect the equipoise between TH-1 cell and TFH-1 cell commitment early after antigen challenge and can enhance the degree of CD4+ and CD8+ memory T cell generation and Bcl6 expression (82).
Lately, circulating CCR7loPD-1hi TFH cell subsets were suggested to serve as surrogate markers for an ensuing humoral response (83). Moreover, a failure to down-regulate T-bet in TFH cells was shown to maintain an IFN-stimulated gene signature, which can propagate antigen persistence (28, 29). Hence, antigen persistence results in TFH-1 cell differentiation, yet may result in less meticulous B cell selection by which the origination of antigen-unspecific and self-reactive B cells and hypergammaglobulinemia is promoted (84). As a consequence, T cell exhaustion may occur (85, 86).
Lastly, the strength of T cell receptor (TCR) ligation and TCR-independent T cell activation by glycolipid recognition via CD1 contributes to determining the outcome of TFH cell-driven humoral immunity (87, 88), where weak ligation maintains T-bet to produce B cell oligoclonality, antigen persistence, TFH cell expansion, and a failing GC response.
In the context of organ transplantation, seroconversion is an undesired phenomenon since memory LLPCs residing in the bone marrow and effector ASCs within draining lymph nodes are responsible for threatening transplant longevity by imposing endothelial injury (15–18, 89). To prevent the emergence of antibodies directed against donor-specific antigenic material, donor and recipient matching for human leukocyte antigens (HLA) molecules and subjecting the recipient to an intense, life-long immunosuppression post-transplant are vital. If not adequately controlled by the immunosuppressive medication or immunodynamic interactions, the risk to develop antibody-mediated rejection (AMR) of the transplant looms. Predisposing factors for AMR include acute and chronic trauma being on hand in the transplanted setting since they disclose autoantigens, which also permit non-HLA antibodies to be patterned.
In this review, I will look back at recent and past insights into immune surveillance by TFH cells following kidney transplantation, with an emphasis on clinical data. Focus will be dedicated to the involvement of TFH cells in the emergence of autoantibodies against non-HLA autoantigens and de novo donor-specific HLA antibodies (dnDSA). Highlighting the current advances and paradigms in TFH cell biology may hold the potential to stratify transplanted patients at risk for the emergence of antibodies and may open new avenues on how to treat episodes of AMR more successfully.
2 Kidney transplantation, organ donation after circulatory death, and the detrimental impact of ischemia reperfusion injury
Previous studies have primed our understanding of factors influencing allograft tolerance. Whilst donor age and HLA mismatches were demonstrated indicators for kidney allograft survival of deceased donors, the factor age did not apply to living donations (90). Intriguingly, the presence of dnDSA more adversely affects allograft survival in donations after circulatory death (DACD) (91). Since it is obvious that deceased donor transplants are more often subject to ischemia-reperfusion injury (IRI), organ preservation and recovery may not equally be off to a favorable start when rejection episodes, the transfer of pro-inflammatory cells, and ultimately allograft survival are concerned (92). Recently, various strategies are used in animals and humans to reduce the impact of inflammatory cells on allograft longevity. In that regard, targeting immune cell diapedesis by targeting the CD62/CD62L-axis has successfully demonstrated a reduction in the adherence of pro-inflammatory immune cells to the endothelial lining (93, 94). Similarly, a cease in T cell activation via the TIM-1-TIM-4 pathway was found to foster allograft survival after IRI in mice, which conceptually can impact on their fate commitment towards further differentiation trajectories (95).
2.1 Immunosuppressive drugs influence TFH cells after kidney transplantation
Besides the transfer of pro-inflammatory cells, which is heightened in DACDs, the relevance of immunosuppressive drugs on TFH cells after kidney transplantation and transplant longevity is being increasingly appreciated, too. Although CD4+ TFH cell subpopulations reciprocally orchestrate their preeminent transcriptional regulation, immunosuppressive drugs can have a profound effect in governing subset skewing (96). Thymoglobulin induction therapy (e.g., antithymoglobulin/ATG) was reported to deplete effector CD4+ and CD8+ T cells, whilst preserving allograft permissive FoxP3+ TFR cells (97, 98). This warrants further studies investigating possible therapies that draw on TFR cell-mediated effects. This could potentially enable long-term drug-free allograft permissiveness (96, 99). This appears to be of particular importance since kidney transplant patients who have suffered from prior AMR were found to display higher ratios of IL-21+ TFH cells whilst their TFR cell population was decreased both within the graft and in the circulation. Besides, sirolimus was also found to reduce the TFR cell population even further (100). Permissiveness may also be facilitated actively by B cells since B cell depletion or IL-10 deficiency were shown to skew the tolerogenic environment towards an increased IL-21+ TFH cell population decreasing TFR cells in follicles (Figure 1) (101). By extension, basic leucine zipper ATF-like transcription factor (BATF) inhibition, a transcription factor for TH-17 and TFH-17 cells alike, could be linked to enhanced FoxP3 levels coinciding with a reduction in retinoic-acid-receptor-related orphan nuclear receptor gamma (RORγt), IL-17A, and IL-4, thus, generating tolerance after transplantation (102). Calcineurin inhibitors like cyclosporine A also impact on TFH cells. Although no effect on TFH subtypes concerning TFH-1, TFH-2, and TFH-17 cells was observed in healthy volunteers under transient cyclosporine A medication, a profound reduction in the pro-inflammatory markers IFN-γ, IL-17A, and IL-21, produced by TFH-1 and TFH-17 cells, respectively, was observed (103). This poses the question how the commonly employed permanent triple immunosuppressive therapy comprising not only of calcineurin inhibitors, but also corticosteroids and inosine-5’-monophosphate dehydrogenase (IMPDH) inhibitors, all known suppressors of T cell functionality (103–105), can lead to TFH cell dysregulation culminating in alloantibody formation and potentially allograft loss.
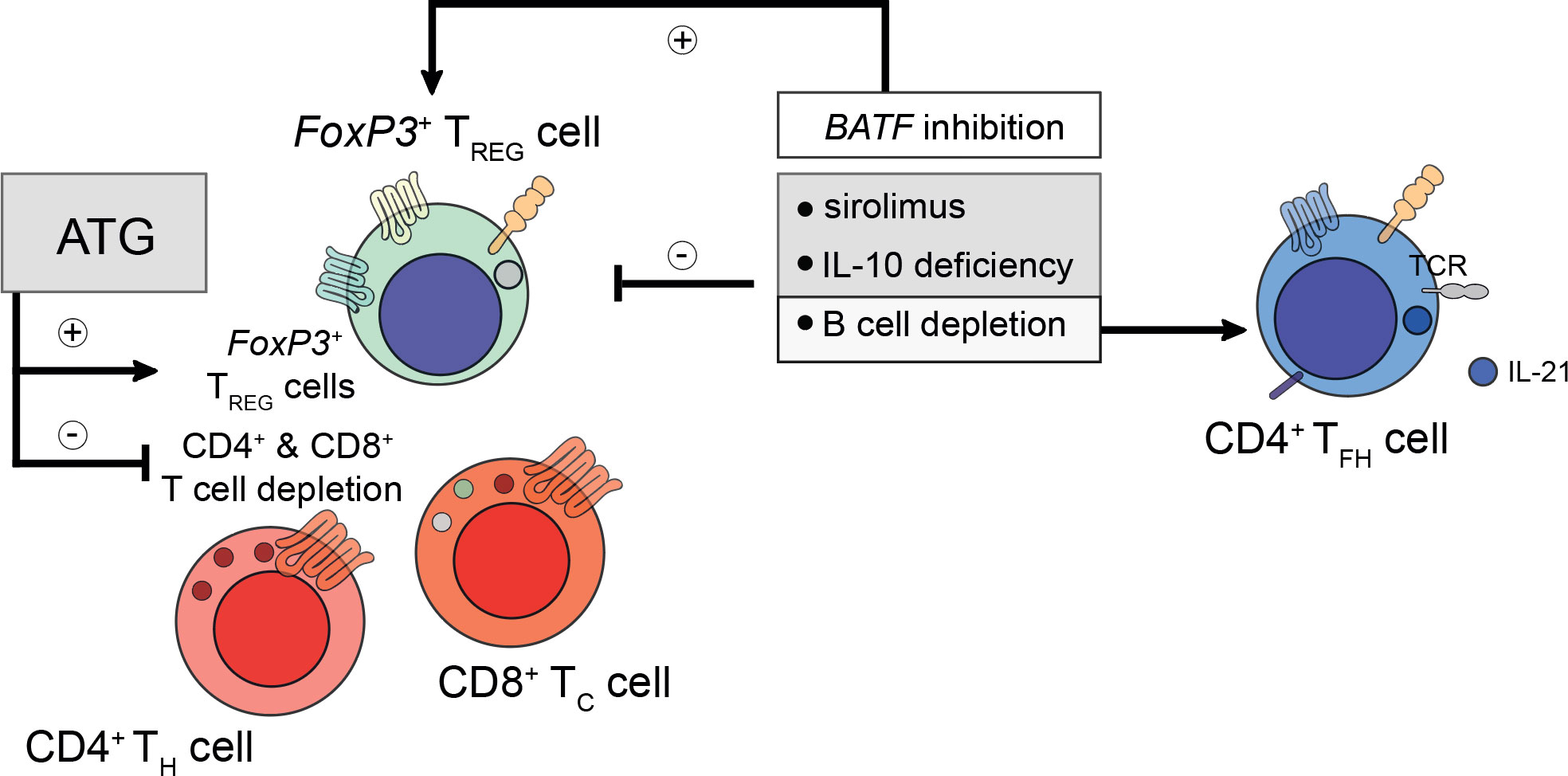
Figure 1 Considerations for prospective peri- and post-transplant immunosuppression. Peri- and post-transplant immunosuppressive regiments do confer differential regulatory phenotypes and need to be tailored to patient-specific demands. For example, pre-transplant administration of anti-thymocyte globulin (ATG) does deplete recipient CD4+ T helper (TH) and CD8+ cytotoxic T (TC) cells, whilst preserving the population of the recipient’s FoxP3+ regulatory T (TREG) cells. TREG cells are required to prevent the formation of de novo donor-specific human leukocyte antigen (HLA) antibodies. However, too ample TREG cell-signatures do prevent the mounting of a successful humoral immune response during microbial challenge. To understand these intricacies better, future studies are needed to elucidate the mechanisms underlying the refinement and control of TREG, TH, follicular TC, and T follicular helper (TFH) cells. To date, sirolimus and interleukin (IL)-10 deficiency were found to impair TREG cell activity. Likewise, B cell depletion (for example using rituximab) also disables TREG cell responses. Moreover, B cell depletion restores IL-21-producing TFH cell responses indirectly by alleviating the control imposed by follicular TFR cells. In contrast to sirolimus and rituximab, inhibition of basic zipper ATF-like transcription factor (BATF) does promote TREG cell responses by reducing the expression of IL-4, IL-17A, and RORγt.
Together, this argues for a better understanding of the effects of the lifelong post-transplant immunosuppressive regimen on the follicular and extrafollicular immune cell compartment to prevent sensitization to donor-specific HLA molecules or autologous non-HLA molecules with an eminent focus on the control of prevalent TFH cell responses whilst maintaining a strong TFR surveillance to induce allotolerance in kidney transplant recipients (KTxs).
2.2 Sequelae of persistent auto- and alloantigen stimulation of TFH cells
Under physiological circumstances, the evolution of a humoral memory (LLPCs) allows high-affinity antibodies to be established within a matter of days upon re-exposure of an antigen to protect us from microbial threats (106). However, auto- and alloantigenic settings constantly expose TFH cells to antigens and disturb modulating checkpoints provided by TREG cells, TFR cells, follicular cytotoxic T (TFC) cells, or even regulatory B cells. Hence, the excessive TFH cell stimulation may take a life of its own. Strategies to prevent this from happening exploit cytotoxic T-lymphocyte-associated protein 4 (CTLA-4)-specific immunoglobulin or IL-21 receptor (IL-21R) antagonists to prevent alloimmune responses (107, 108).
Hence, the surveillance of immune subtype compositions may turn out as a valuable tool in identifying KTxs at risk for the formation of auto- and alloantibodies and subsequent allograft rejection.
Considering the selective influence on TFH cells, studies on autoimmune diseases have shaped our understanding of persistent TFH cell stimulation resulting in dysregulated responses. For example, unrestricted clonal expansion of TFH cells allows the generation of ectopic lymphoid structures (ELS), which are unphysiological tertiary lymphoid follicles featuring stimulation and clonal expansion of antigen-specific B cells (109). These ELS are being established because of an antigen response yet to be cleared (110), and are formed by a regulated and well-orchestrated expression of the lymphoid chemokines C-C motif ligand (CCL)19, CCL21, and CXCL13 (111–113). Persistence of these lymphokines (with TFH cells being a major source of CXCL13) (9) allows PSGL-1LOWCD40L+ TFH-17 cells to maintain aggregates in an ICOS-dependent manner with B cells in extrafollicular ELS, inevitably preserving an immune response with a serious risk for the formation of autoantibodies of the IgG2a/IgG2b or IgG1/IgG3 type, tissue destruction, and ultimately the development of an autoantibody-mediated pathology (114–116). Autoimmune conditions and others have been shown to be associated with ELS, which are a major compartment for ample extrafollicular TFH cell accumulation (117). To date, several studies have implied TFH cells especially in the context of glomerulonephritis where IL-17 drives inflammation and autoantibody-induced kidney injury which can be considered to be key determinants for autoimmune disease activity (118–121), whilst a disrupted TFH cell response has been shown to reduction disease activity, respectively (122–124). It appears intuitive that especially endothelial injury and incessant inflammation with enhanced HLA class II up-regulation can expose autoantigens for TFH cell sensitization (125, 126). Despite the predominance of literature, particularly from human studies, on the importance of ELS for chronic inflammation caused by infections, autoimmune diseases, cancer, or transplantation (127), the contribution of derailed TFH cell activity in the induction of autoimmunity within secondary lymphoid tissues (SLOs) including the spleen, lymph nodes, and mucosal-associated lymphoid tissue (MALT) (128), may be equally important. For example, a murine study investigating the importance of TFH cells in the induction of rheumatoid arthritis found TFH cell frequencies to be significantly increased in the spleen and joint-draining lymph nodes following disease induction. Here, CXCR5-/- mice were protected from autoimmune arthritis by abrogating TFH-B cell interactions within SLOs stressing its importance in the induction of autoimmune inflammation (129). In fact, not only murine models show that immune responses in SLOs may not only precede the formation of ELS and tertiary lymphoid organs (TLOs) (130), but also maintain an active role during autoimmune conditions as is observed in patients suffering from rheumatoid arthritis, who feature follicular hyperplasia and active GC responses in SLOs (131–133).
Together, a skewed TFH cell response featuring lack of TFH-17 cell control can lead to the establishment of ELS enhancing the likelihood of TFH-17-B cell aggregates within these extrafollicular spaces for alloantibodies and alloreactive LLPCs to occur. Understanding how the disrupted TFH cell subset equipoise can be restored may hold therapeutic potential to circumvent the need for immunosuppressive therapies with its inherent adverse effects and risk for infections (134).
2.3 Donor-specific HLA antibodies and TFH cells: the feared couple to answer for antibody-mediated rejection
Although more and more details are being understood regarding characteristics of HLA epitopes to perform appropriate epitope matching in clinical settings, there remains much to be learned. To date, HLA-matching in kidney transplantation is only performed for HLA-A, -B, -C, -Bw4, -Bw6, -DR, -DR51/52/53, -DQA1, - DQB1, and -DPB1 antigens (135). Nevertheless, mismatches are mostly inevitable and enhance the risk of dnDSA formation (136). However, it appears that not every epitope is equally immunogenic. Aubert et al. have found that low-level donor-specific HLA antibodies remain controversial in terms of predicting the risk of graft failure (136, 137). Indeed, studies have proposed dnDSA directed at HLA-DQ to be a risk factor for late allograft failure that feature histological alterations in accordance with chronic AMR (138–140).
Although dnDSA appear to form at relatively low frequencies (~15%) and often only several years after transplantation, they commonly facilitate detrimental consequences (141, 142). Independent risk factors were reported to be HLA-DR mismatches and non-adherence to immunosuppressive therapy. Upon emergence, dnDSA could progress and show antibody-mediated graft injury without impaired graft function (142).
Evidence suggesting AMR to be a consequence of the presence of dnDSA effectively challenged the dogma of calcineurin inhibitor toxicity and chronic allograft nephropathy (141). In fact, capillaritis was found to forecast allograft dysfunction culminating in AMR (143–145). Not much later, Lefaucheur et al. identified four distinct patterns of kidney rejection with capillaritis in association with AMR illustrating the poorest outcomes (146). Not all dnDSA could be paired with ensuing AMR (147, 148). In that regard, dnDSA properties would matter with respect to MFI, complement-binding capacity, and IgG subclass composition (147, 149). Conversely, the clinical phenotype of AMR cannot be linked with the presence of circulating dnDSA in all cases challenging the criteria for diagnosis. In that regard, evidence comprised histological acute tissue injury, current antibody interaction with endothelium as in C4d deposition, and serological evidence of dnDSA detection or non-HLA donor-specific antibodies (150, 151). The identification of intragraft dnDSA has been proposed to enable clinicians to correctly diagnose AMR, which would not meet these criteria (151, 152). However, if serologically detectable, dnDSA presence has been attributed to a poor graft outcome imposed by AMR (139, 149, 153–155).
A clear link between AMR and TFH cells, particularly regarding IL-21 production, has been established (156). KTxs suffering from chronic allograft rejection were found to display distinctive increases in circulating TFH cells with impaired controllability given a reduced PD-1 expression (157). Accordingly, PD-1 expression fine-tunes TFH cell responses by suppressing follicular T cell recruitment, confining TFH cell localization within GCs, and increasing the stringency of GC affinity selection via suppressed phosphoinositide 3-kinase activity upon PD-L1 ligation (158). Another study found stable circulating TFH cell numbers with decreased IL-21 production. However, their ability to stimulate alloantigen-specific B cells to produce IgG was maintained (159). Stable numbers of TFH cells have also been found by Chen et al., however, indicating a skewing toward IL-21-producing TFH-17 and TFH-2 cell subpopulations in AMR (100). To control this dysregulation of TFH cells, Rodriguez-Barbosa et al. have found that whilst the CD40/L pathway could be used, the B and T lymphocyte attenuator (BTLA) pathway was dispensable (160). Exploiting the CD40/L axis revealed reduced clonal B cell expansion associated with curtailed GC-TFH cell numbers and a blunted IL-21 secretion (161). It appears that challenging cellular receptor-ligand interactions between TFH-B cells may alleviate the burden of AMR, which studies estimate to be responsible for 30-50% of allografts to fail (156). Likewise, protection from AMR could be mediated by strengthening the control of TFH cells and Ag-specific B cells by TFR cells (96, 100, 101, 155).
Therefore, TFH cells are not only capable of inducing dnDSA formation but can also further augment preexisting DSA levels following alloantigen recall (162). Conversely, TFR cells curtail TFH cell-directed B cell help by preventing dnDSA formation. Whilst lack of TFH cells control drives severe AMR, TFR cells are less involved in this process (162). These observations appear to translate to the human setting. Here, KTxs with immunogenic tolerance towards their allograft have disrupted TFH cell functionality characterized by lack of IL-21 production, although in humans TFR cells control AMR (100, 163). In a similar notion, IL-21+ TFH cells and activated B cell responses (ASCs, CD86+CD38+) together with serum IL-21 levels were proposed as biomarkers for AMR in KTxs (164, 165). A more profound understanding if certain TFH cell-related pathways predisposed to the formation of complement-fixing or non-complement fixing dnDSA, would help to develop non-invasive biomarker-guided risk stratification and molecular refinement tools to prevent the emergence of dnDSA altogether. In fact, Louis et al. recently demonstrated that TFR cells and transitional B cells were selectively reduced in KTxs with AMR (166). Characteristically, both populations comprising of CXCR5+ TFR cells and CD21- transitional B cells that had vanished expressed T-bet. Their loss coincided with enhanced inflammatory antibody responses, microvascular inflammation, and allograft failure (166). Previous studies have highlighted the importance of T-bet to act as a repressor of PD-1 and other inhibitory receptors such as LAG-3, CD160, and BTLA in adaptive immune cells (167), possibly identifying T-bet expression as a canonical immune checkpoint to drive alloreactivity in T cells (Figure 2) (168). However, it must be recognized that noncanonical pathways in the absence of TFH-B cell interactions need to be considered that may also contribute to the production of alloantibodies or certain subtypes of allospecific immunoglobulins such as IgG2c where TFH cells may be dispensable (169, 170).
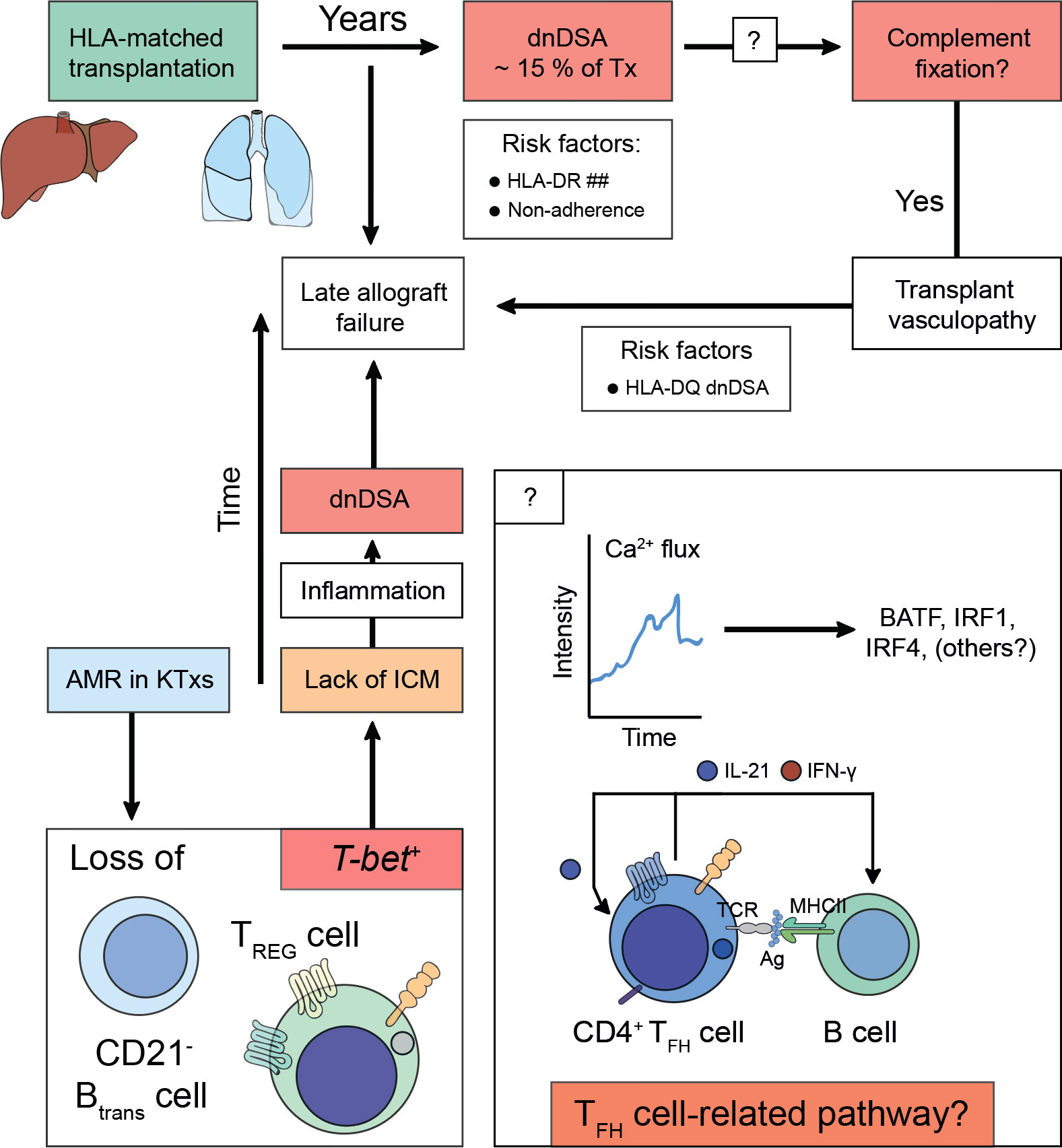
Figure 2 Alloimmunity following solid organ transplantation predispose for acquiring de novo donor-specific human leukocyte antigen antibodies and antibody-mediated allograft failure. Solid organ transplants (e.g., liver or lung) are matched for human leukocyte antigens (HLA)-A, -B, and -DR before the transplantation is undertaken. After transplantation, the constant exposure to non-HLA antigens, small antigens, or (unmatched) HLA antigens predispose for the acquisition of de novo Donor-specific HLA antibodies (dnDSA), which can be detected in ~ 15% of transplanted patients years after the transplantation was performed. Risk factors for the establishment of dnDSA are mismatches for HLA-DR (HLA-DR ##) or non-adherence to taking the life-long immunosuppressive treatment. The dnDSA can be classified into non-fixing or complement-fixing antibodies. Complement fixation is associated with a pro-inflammatory response, which is the cause for transplant vasculopathy. To date, it is unclear how complement-fixing abilities of dnDSA are determined (? in box), however, HLA-DQ dnDSA were found to be a risk factor. It is speculated that T follicular helper (TFH) cell-related pathways either on the level of fate commitment upon acquisition of antigen-specificity via T cell receptor engagement or during TFH cell – B cell interactions would imprint on complement-fixing abilities of dnDSA. Irrespective of whether dnDSA can be detected in a patient, which often only attach to the transplanted vascular tissue structures of the allograft and thus evade detection by conventional blood taking methods, transplant vasculopathy drives (sub)clinical rejection episodes, which confer the risk to acquire late allograft failure. During antibody-mediated rejection (AMR) T-bet expression is lost from transitional B cells (CD21- Btrans cell) and follicular regulatory T (TFR) cells. T-bet confers instructional cues for PD-1 acquisition, thus lack of immune checkpoint modulation (ICM) can be deduced that preserves an interferon-stimulated gene signature (ISGS) upon TFH cell – B cell encounters. Perpetuation of ISGS maintains inflammation and fosters the establishment of dnDSA if immune suppressive measurements are not engaged.
Subclinical rejection episodes, a sequelae of aberrant allosensitization possibly causing loss of TFR cells, are considered prognostic indicators for chronic AMR (171, 172). Adjusting signaling pathways culminating in the absence of IL-21 production in TFH cells prevents dnDSA formation due to lack of cognate B cell help and thus fosters allotolerance (163). Hence, defying allosensitization of TFH cells, e.g., via engagement of the co-stimulatory blockade receptor CTLA-4 (100, 173), enhanced mTOR immunosuppression (174, 175), blockade of the CD40/L axis (67, 160, 161), or antagonizing antibodies against the IL-21R (108, 156, 159), may allow to further refine or counteract chronic AMR in kidney allografts. This strategy may promote longevity of kidney transplants and the quality of life in transplanted patients. Mechanisms underlying this deleterious allosensitization of naïve T cells to becoming alloreactive TFH cells are conceivable. Comparing immunosuppression with tacrolimus against co-stimulation blockade of CD80 and CD86 using the CTLA-4-Ig belatacept conferred diminished seroconversion rates to influenza vaccination (176). Hence, understanding how to prevent the selection of alloreactive TFH cell effector and memory clones will be an important area of future investigations. For example, a recent cohort study in patients with a positive dnDSA status showed an enhanced alloreactive TFH cell pool in response to donor-specific HLA antigens. Despite continuous immunosuppression compared with healthy controls, an augmented IL-21 production and proliferative response in these TFH cells upon stimulation in vitro was reported suggesting either incessantly activated or more easily recruited signaling domains even years after kidney transplantation (103). Further studies are needed to better define how to circumvent unwanted allograft-directed immune responses, whilst maintaining T cell-mediated antigen-specific B cell help in the context of the host’s immune protection and response to vaccination. GC responses emerge as prominent areas to be more meticulously studied. For example, KTxs were shown to lack GC responses to mRNA vaccination against severe acute respiratory syndrome coronavirus type 2 (SARS-CoV-2), a feature well characterized to be associated with neutralizing antibodies against SARS-CoV-2 (177). A prominent feature of this missing GC response in this study was a blunted TFH cell signature, which is considered instrumental in orchestrating architecture and functionality of GCs (6–10). One may speculate that allograft-draining lymph nodes’ GCs may present a unique architecture in patients having developed dnDSA compared to other allograft-unassociated draining lymph nodes. Recent studies have shown that the trauma of the surgical intervention requires lymphatic vessels to heal, which in murine kidney transplantation showed lymphatic endothelial cells to abundantly release CCL21 by which stromal lymph node remodeling was fostered. Accordingly, dendritic cell enrichment was observed thus increasing the chance for successful alloreactive T cell priming. The causative role of the lymphatic system pertaining to the kidney allograft could be established in retrospective analyses in humans (178). Furthermore, the formation of TLOs within the allograft itself were observed in human kidneys undergoing chronic rejection (179, 180). Besides the formation of de novo lymphatic angiogenesis, the contribution of increased lymphatic flow may be another factor by which cellular trafficking, alloimmunity, and vasculopathy are being propagated, which in the context of heart transplantation found donor cell-trafficking to allograft-draining lymph nodes, increased lymphatic vessel are, and allograft infiltration of CD4+ and CD8+ T cells as well as CD68+ macrophages (181). In fact, draining lymph nodes and TLOs after small-bowel transplant rejection were enriched in CXCR3+ host T cells stimulated by donor-derived type 1 helper T cell-related chemokines (IP-10) suggesting their possible contribution also in other solid organ transplantation contexts such as the kidney (182). Since alloreactive T cells are susceptible toward inhibition by standard immunosuppressive drugs such as corticosteroids or calcineurin inhibitors, an advanced understanding of potentially derailed signaling pathways downstream of calcineurin in cases where patients are suspected of allograft rejection despite appropriate drug levels is needed (183).
Recent studies highlight the importance to better understand cytotoxic T cell responses in GCs. Some cytotoxic T cells can acquire CXCR5 expression (28), thus enabling them to enter GCs. Compared with STAT5+CXCR5+CD8+ TFC cells, PD-1+CXCR5+CD8+ TFC cells were found to be a biomarker for AMR. In this study, PD-1+CXCR5+CD8+ T cells were associated with chronic allograft dysfunction following kidney transplantation (184). Indeed, CXCR5+ CD8+ T cells with IFN-γ-producing abilities can be sampled from the peripheral blood in higher quantities in KTxs who remain DSA-free (185). Studies in a murine system of AMR using adoptive cell transfers in CCR5 KO mice equally show reduced frequencies of CXCR5+CD8+ TFC cells following AMR of the kidney transplant (186), which confer cytotoxicity towards alloprimed IgG+ B cells. This could be a means by which incessant adaptive immune cell activation in allograft-draining lymph nodes may be held in check in transplanted patients who remain dnDSA-free.
Together, these studies implicate both TFH cell and TFR cell subsets as relevant entities to risk-stratify patients concerning potential dnDSA formation (89). Further studies are needed to better define lineage-commitment trajectories of TFC cells to comprehend how to therapeutically intervene in cases of ongoing AMR. This may hold the potential to maintain allograft longevity even in cases of severe AMR.
2.4 Non-HLA antibodies: the underestimated reason for transplant morbidity caused by exuberant sensitization of TFH cells?
Having discussed the relevance of TFH cells and their cellular modulators for the formation of HLA-specific dnDSA and the importance of TFH cell subsets for the disclosure of autoantigens and autoimmune conditions to emerge, the relevance of non-HLA antibodies remains to be outlined.
Just over a decade ago, Dragun et al. reported refractory vascular rejection in KTxs caused by non-HLA autoantibodies against the angiotensin II type 1 receptor (AT1R) (187). Similarly, transplant glomerulopathy (TG) a consequence of autoantibody formation against perlecan and agrin, both compounds of the glomerular basement membrane, was described (188). It appeared that the risk for TG was even enhanced if both dnDSA and non-HLA antibodies were coincidentally present (189). To address these descriptions, a study conducted by Amico et al. found around 2.3% of patients of their cohort, who experienced early AMR, to be due to non-HLA antibodies (190). Progressive loss of self-tolerance to the autoantigens k-α-1 tubulin, collagen type V, and the collagen I was linked to increased risk of primary graft dysfunction in lung transplantation, which in turn would augment alloimmune responses inclining towards bronchiolitis obliterans syndrome (BOS) (191, 192). The importance of activating antibodies against AT1R is by far the most thoroughly studied. AT1R and the C-terminal fragment of perlecan (LG3) were studied in pregnant transplanted women and appeared to trigger allograft rejection. The mechanism of formation did not require allosensitization and a lack of correlation suggested different mechanisms of generation (193). It was suggested that renal ischemia, alterations to the intragraft microenvironment, and alloimmunity may be some of the various factors predisposing for AT1R antibodies, which augment the ischemic condition by further contraction of the renal vasculature (187, 194, 195). The exposure of other cryptic antigens culminates in the emergence of non-HLA antibodies, which was described for the antigens LG3, vimentin, the endothelin type A receptor (ETAR), and other extracellular proteins and intermediate filaments. But also, more recently, their ability to bind to antigens present on apoptotic cells activating complement was appreciated (Figure 3) (195–198).
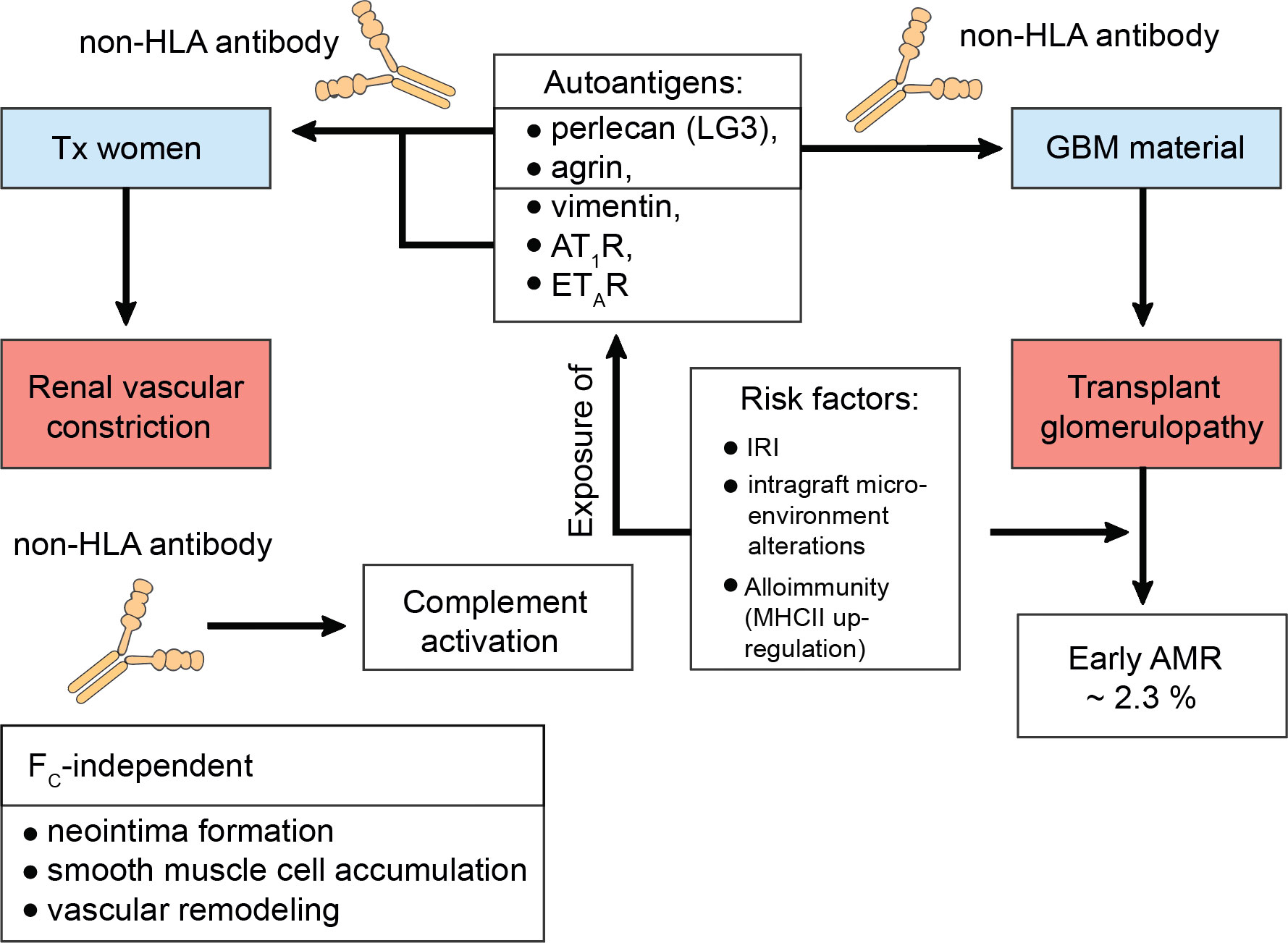
Figure 3 Transplant glomerulopathy in the context of non-human leukocyte antigen antibodies. Constant cellular turnover as occurs through programmed-cell death or damage to the transplanted organ predisposes for the disclosure of autoantigens. To date, the C-terminal fragment of perlecan (LG3), agrin, vimentin, the angiotensin II type 1 receptor (AT1R), the endothelin type A receptor (ETAR), k-α-1 tubulin, collagen type V, and collagen type I have been identified as serving as autoantigens with risk for progressive loss of self-tolerance. This loss of self-tolerance can result in their presentation by tissue-resident phagocytes and serological acquisition of non-human leukocyte antigen (non-HLA) antibodies without prior allosensitization. Interestingly, LG3 and agrin are composite material of the glomerular basement membrane (GBM) and can thus drive transplant glomerulopathy (TG) via renal vascular constriction, which was studies in pregnant kidney transplanted women. In this study by Hönger et al. AT1R was also identified to mediate TG. TG can cause early antibody-mediated rejection (AMR) and it was found that ~ 2.3% of these cases are due to non-HLA antibodies. Risk factors that mediate this process comprise ischemia-reperfusion injury (IRI), intragraft microenvironment alterations, or alloimmunity, which drives the up-regulation of major histocompatibility complex (MHC) class II (MHCII) on antigen-presenting cells, thereby upping the odds of successful cross-presentation to T helper cells. Studies have found that non-HLA antibodes can activate complement. Moreover, their pathology also entails FC-receptor-independent effects. For example, they can induce neointima formation, enhance the accumulation of smooth muscle cells, and promote vascular remodeling.
Evidence also suggests FC-independent effects of non-HLA antibodies like augmented neointima formation with an accumulation of smooth muscle cells, mesenchymal stem cells advocating for their regulatory function via ERK1/2 signaling and interactions with α2β1 integrins in obliterative vascular remodeling during rejection (199, 200). But also other conditions have seen the discovery of non-HLA autoantibodies including systemic sclerosis with or without associated pulmonary arterial hypertension (201, 202).
It was shown that apoptotic cells release exosome-like vesicles, which, mediated by the 20S proteasome, incite non-HLA antibody formation. This coincided with enhanced TFH and GC B cells (203). The conceptual framework of apoptosis taking place constantly may allow us to explain why some non-HLA autoantibodies may be present pre-transplant. In fact, a study by Nagele et al. reported ample naturally occurring immunoglobulin (Ig) G autoantibodies, which were influenced by age, gender, and disease (204).
Together, copious amounts of studies highlight the importance of auto- and allosensitization of TFH cells in generating a permissive environment to stimulate cognate Ag-specific B cells toward the production of both non-HLA autoantibodies and dnDSA. Therefore, clinicians need to carefully monitor patients to identify derailed homeostatic interactions even in the absence of measurable auto- or allosensitization (205). It is noteworthy that compartments reflecting dysbalanced interactions (ELS, allograft draining lymph nodes) are not part of the regular clinical routine suggesting that further research is needed to define appropriate biomarkers that may reflect the current state of health of these compartments. Moreover, a more fundamental understanding about how these antibodies evolve from a TFH cell perspective may identify interventions potent to either decrease the intensity of the antibodies detected or even reverse their presence after all.
3 Lessons learnt on TFH cells from other solid organ transplantation settings
Besides kidney transplantation, TFH cell biology has also been studied in the context of other solid organ transplantations (Table 2). Although organ-specific biology considering epigenetic, molecular, cellular, and environmental effects cannot be ruled out in T cell biology, these findings can instruct experimental nephrologist to consider similar trends in kidney transplantation (215–217).
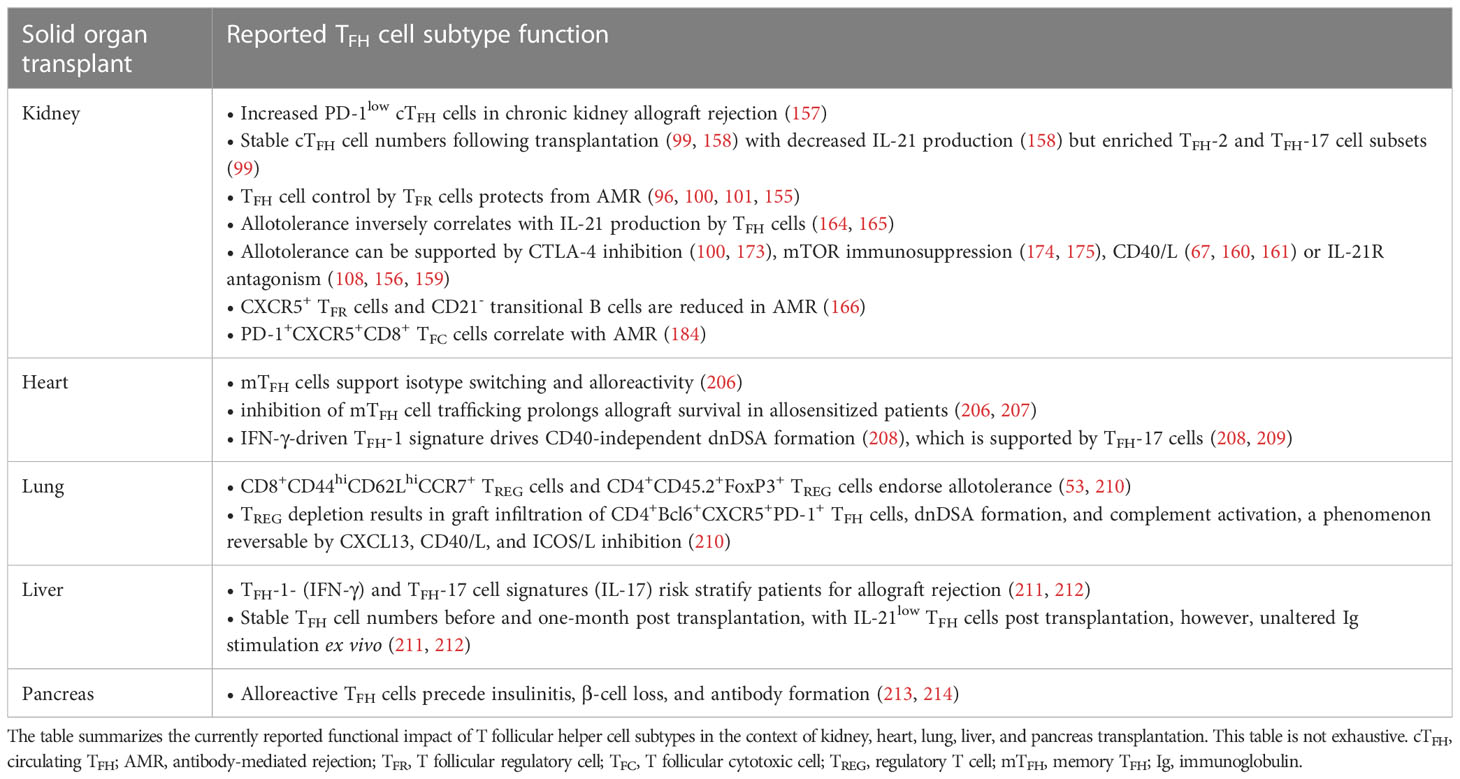
Table 2 A summary on the reported impact of T follicular cell subtypes in the context of solid organ transplantation settings.
Towards the turn of the millennium, MHC class I, mainly HLA-A, antibodies had been described to forecast the development of BOS (210, 218). Other reports reliably confirmed their detrimental impact on lung allograft longevity as they caused persistent-recurrent lung rejections and chronic allograft dysfunctions (219, 220). Moreover, dnDSA were found to occur more frequently as is the case in other solid organ transplantation settings (148). Despite their frequency, their full impact remains yet to be fully elucidated with intragraft dnDSA noticed to carry a higher risk for lung allograft loss (148). Formation of dnDSA after lung transplantation corresponds to a high risk of refractory acute cellular rejection, lymphocytic bronchiolitis (with an increased influx of lymphocytes and neutrophils in bronchoalveolar lavages) (221), and BOS (222). Lately, Krupnick et al. have described the importance of CD8+CD44hiCD62LhiCCR7+ TREG cells in a mouse lung transplant model, which patrolled the lung successfully to endorse allotolerance (223). Furthermore, Li et al. have recently demonstrated that depletion of CD4+CD45.2+FoxP3+ TREG cells induced the emergence of dnDSA resulting in AMR (155). According to the authors, TREG cell depletion allowed CXCL13-mediated graft infiltration of CD4+Bcl6+CXCR5+PD-1+ TFH cells and IgM dnDSA to be patterned. Ultimately, complement would deposit and destroy the airway epithelium, a process which could be reversed by CXCL13 blockade or utilization of CD40/L and ICOS/L pathway inhibition (Figure 4) (155). This suggests that even severe endothelial cell injury could be reversed if the right immunological setscrew was identified. These findings warrant similar research in the context of kidney transplantation. Bypassing both TFH cell or TREG cell influence on lung allograft longevity, a study has also successfully described B cell-targeted inhibition impeding follicle formation and eventually preventing BOS after lung transplantation (224).
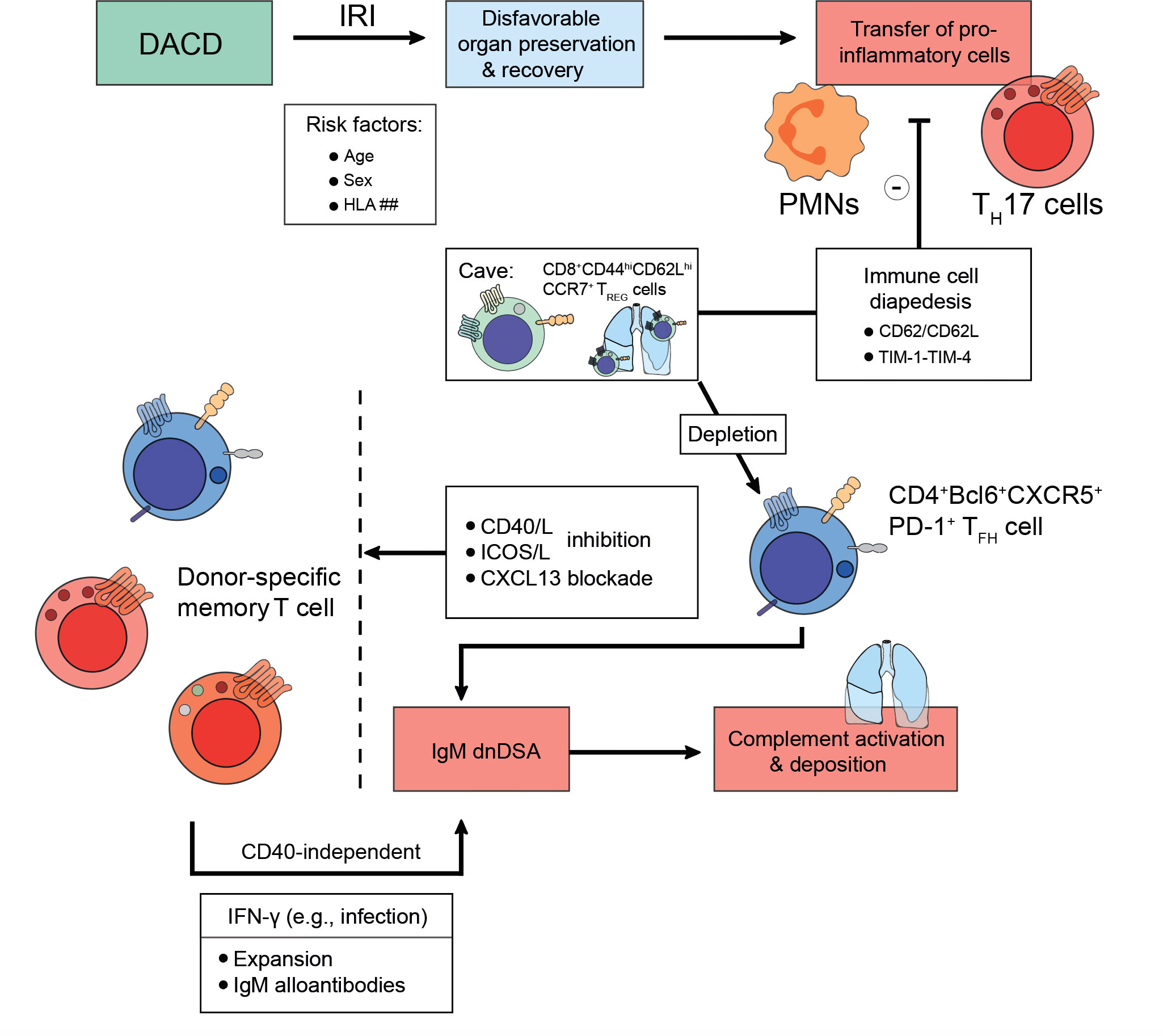
Figure 4 Strategies to foster transplant longevity for donations after circulatory death. Donations after circulatory death (DACD) comprise the largest fraction of organs available for transplantation. However, due to reduced circulation ischemia-reperfusion injury (IRI) has often occurred, which may be even exacerbated depending on factors including age, sex, or mismatches in the human leukocyte antigen (HLA) system. This disadvantages the length of organ preservation possible and potential chances for recovery upon transplantation. Moreover, the IRI does confer migratory effects to donor pro-inflammatory cells like polymorphonuclear neutrophils (PMNs) or IL-17A-producing T helper (TH17) cells, which are being transplanted jointly with the organ. However, to prevent incessant recipient pro-inflammatory immune cell recruitment towards the inflamed organ, several strategies are being explored evolving around the issue of disabling immune cell diapedesis. For example, the CD62-CD62L axis or the TIM-1-TIM-4 pathway can be targeted since CD8+CD44hiCD62LhiCCR7+ TREG cells patrol the lung to endorse alloimmunity after lung transplantation. Pending successful implementation, transplant longevity may also conceptually be fostered by employing co-stimulation inhibitory approaches for the CD40/CD40L-axis, the ICOS/ICOSL-pathway, or by CXCL13 blockade to deplete donor-specific memory T cells. Following an infection that drives interferon (IFN)-γ expression, donor-specific memory T cell populations can expand and contribute to an IgM alloantibody response in a CD40-independent manner. As above, these IgM alloantibodies activate compliment and drive microvasculature inflammation that up the odds for late allograft failure.
In heart transplantation (HTx), the importance of alloreactive memory TFH cells has been delineated previously. In that regard, memory TFH cells were shown to be the barrier to long-term allograft survival as they support antibody isotype switching and alloreactive effector TFH cell damage (206). Moreover, inhibiting memory CD4 TH cell trafficking was assessed by Zhang et al. and effectively described to prolong HTx survival in sensitized patients with alloreactive memory TFH cells, a cohort that is particularly prone to insufficient co-stimulation blockade (206, 207). By employing lymphoid sequestration, TREG cell differentiation was advocated (225). More recently, Gorbacheva et al. have found that CD40-independent alloantibody responses were facilitated by IFN-γ producing TH cells and may be the cause for deleterious alloantibody responses despite co-stimulation blockade (208). Especially TH-1 and TH-17 cells promoted dnDSA responses (208, 209), while also displaying CD40-independent help to alloreactive effector CD8+ T cells (209). Together, this suggests that follicular and extrafollicular alloreactive B cell responses need to be screened in experimental models to identify pathways by which this extrafollicular CD40-independent B cell help can be curtailed and rather host protective and allograft-permissive T cell biology can be regained.
In liver transplantation (LTx), cytokines related to TFH-1 and TFH-17 cells, IFN-γ and IL-17, have been identified to risk stratify patients regarding their risk of rejection (211, 212). More recently, TFH cell dynamics were studied by Zhang et al. one day before up to one-month post-transplant. In their study, the authors have shown an unchanged TFH cell frequency in the circulation, however, a reduced production of IL-21 one month after LTx was noticed. Despite the lack in IL-21 production, these TFH cell could provide cognate B cell help with unaltered production of immunoglobulins in ex vivo cultures (213).
Lastly, pancreas transplantation utilizes similar draining lymph nodes of the paracaval and paraaortic affiliation. There are essentially three forms of pancreas transplantations (PTx) currently in practice: simultaneous pancreas-kidney (SPK), isolated pancreas (IP), and islet cell transplantation. As with other forms of organ transplantation, the deleterious impact of alloreactive TFH cells was also investigated regarding tissue reactivity and alloantibody production. In a study conducted by Vendrame et al., tissue-reactive TH cells were described to precede insulinitis, β-cell loss, and hyperglycemia due to C-peptide loss mediated by the emergence of autoantibodies (214). These negative consequences could be attenuated by non-specific T cell depletion (214). The challenge that alloreactive TH cell pose to transplantation was delineated by another group (226). They described the serious adverse effects imposed on PTx survival by the emergence of dnDSA where, on average, 14.7% developed dnDSA, which was more frequent in IP (~ 19%) and showed an inferior outcome compared to non-donor HLA. This renders the detection of dnDSA as a strong independent predictor of pancreas allograft failure (226). Another independent study also reported in ~ 15.6% the appearance of dnDSA, predominantly class II-specific, whereas non-DSA were mostly of class I-specific nature (227). More recently, it was described that dnDSA would occur equally likely in PTx and islet cell transplantation, also being mostly class II-specific (228). Finally, more frequent, and severe rejection episodes were linked to the presence of dnDSA rather than non-DSA or antibody-negative patients (227).
4 Conclusions
TFH cells play a crucial role in the host’s immune response and allow humoral memory to be established and maintained. However, when sensitized, particularly in a setting of persistent antigen presentation, TFH cell responses are not well studied and may be surprising. Here, their responses may stretch from inabilities to mount an appropriate antibody response thus resulting in an inability to clear microbial threats or even overwhelming immune responses despite immunosuppression. The latter carries the risk of seroconversion of alloantibodies that can cause AMR. We have a lot to learn about TFH cells, their interaction with B cells, TREG cells, TFR cells, regulatory B cells, or tissue-resident (or recruited) myeloid cells including macrophages, dendritic cells, and neutrophils. Advances in drug availability may cause a skewing of subpopulations, which may constitute an advantage or disadvantage given the respective immunological context. Taken together, progress in TFH cells’ biology and unveiling their relation to CXCR5+CD8+ TFC cell subsets or TFR cells may hold the potential not only to risk stratify transplanted patients but moreover to regain control in cases of severe allograft injury and to reinforce allotolerance. This may bring about significant changes how we detect and treat AMR in KTxs. It is conceivable that based on an enhanced immunological understanding, AMR treatment by intensifying the immunosuppressive regimen to become a matter of the past. We should be confident that further studies can significantly improve transplant longevity, and ultimately the quality of life of transplanted patients.
Author contributions
The author confirms being the sole contributor of this work and has approved it for publication.
Funding
JS was funded by a scholarship from the German Academic Scholarship Foundation (Studienstiftung des deutschen Volkes) and by the German Research Foundation (DFG, Clinician Scientist position, SU 1360/1-1).
Acknowledgments
The author thanks Ricardo Lira Martinez for feedback on the manuscript. The Article Processing Charge (APC) were funded by the joint publication funds of the TU Dresden, including Carl Gustav Carus Faculty of Medicine, and the SLUB Dresden as well as the Open Access Publication Funding of the DFG.
Conflict of interest
The author declares that the research was conducted in the absence of any commercial or financial relationships that could be construed as a potential conflict of interest.
Publisher’s note
All claims expressed in this article are solely those of the authors and do not necessarily represent those of their affiliated organizations, or those of the publisher, the editors and the reviewers. Any product that may be evaluated in this article, or claim that may be made by its manufacturer, is not guaranteed or endorsed by the publisher.
Glossary
References
1. King C. New insights into the differentiation and function of T follicular helper cells. Nat Rev Immunol (2009) 9(11):757–66. doi: 10.1038/nri2644
2. Zou X, Sun G, Huo F, Chang L, Yang W. The role of dendritic cells in the differentiation of t follicular helper cells. J Immunol Res (2018) 2018:7281453. doi: 10.1155/2018/7281453
3. Johnston RJ, Poholek AC, DiToro D, Yusuf I, Eto D, Barnett B, et al. Bcl6 and blimp-1 are reciprocal and antagonistic regulators of T follicular helper cell differentiation. Science (1979) 325(5943):1006–10. doi: 10.1126/science.1175870
4. Nurieva RI, Chung Y, Martinez GJ, Yang XO, Tanaka S, Matskevitch TD, et al. Bcl6 mediates the development of T follicular helper cells. Science (2009) 325(5943):1001–5. doi: 10.1126/science.1176676
5. Yu D, Rao S, Tsai LM, Lee SK, He Y, Sutcliffe EL, et al. The transcriptional repressor bcl-6 directs T follicular helper cell lineage commitment. Immunity (2009) 31(3):457–68. doi: 10.1016/j.immuni.2009.07.002
6. Fukuda T, Yoshida T, Okada S, Hatano M, Miki T, Ishibashi K, et al. Disruption of the Bcl6 gene results in an impaired germinal center formation. J Exp Med (1997) 186(3):439–48. doi: 10.1084/jem.186.3.439
7. Dent AL, Shaffer AL, Yu X, Allman D, Staudt LM. Control of inflammation, cytokine expression, and germinal center formation by BCL-6. Science (1997) 276(5312):589–92. doi: 10.1126/science.276.5312.589
8. Morita R, Schmitt N, Bentebibel SE, Ranganathan R, Bourdery L, Zurawski G, et al. Human blood CXCR5+CD4+ T cells are counterparts of T follicular cells and contain specific subsets that differentially support antibody secretion. Immunity (2011) 34(1):108–21. doi: 10.1016/j.immuni.2010.12.012
9. Chevalier N, Jarrossay D, Ho E, Avery DT, Ma CS, Yu D, et al. CXCR5 expressing human central memory CD4 T cells and their relevance for humoral immune responses. J Immunol (2011) 186(10):5556–68. doi: 10.4049/jimmunol.1002828
10. Havenith SHC, Remmerswaal EBM, Idu MM, van Donselaar-van der Pant KAMI, van der Bom N, Bemelman FJ, et al. CXCR5+CD4+ follicular helper T cells accumulate in resting human lymph nodes and have superior b cell helper activity. Int Immunol (2014) 26(3):183–92. doi: 10.1093/intimm/dxt058
11. Hardtke S, Ohl L, Förster R. Balanced expression of CXCR5 and CCR7 on follicular T helper cells determines their transient positioning to lymph node follicles and is essential for efficient b-cell help. Blood (2005) 106(6):1924–31. doi: 10.1182/blood-2004-11-4494
12. Braun A, Worbs T, Moschovakis GL, Halle S, Hoffmann K, Bölter J, et al. Afferent lymph–derived T cells and DCs use different chemokine receptor CCR7–dependent routes for entry into the lymph node and intranodal migration. Nat Immunol (2011) 12(9):879–87. doi: 10.1038/ni.2085
13. King C, Tangye SG, Mackay CR. T Follicular helper (TFH) cells in normal and dysregulated immune responses. Annu Rev Immunol (2008) 26:741–66. doi: 10.1146/annurev.immunol.26.021607.090344
14. Fazilleau N, McHeyzer-Williams LJ, Rosen H, McHeyzer-Williams MG. The function of follicular helper T cells is regulated by the strength of T cell antigen receptor binding. Nat Immunol (2009) 10(4):375–84. doi: 10.1038/ni.1704
15. Ionescu L, Urschel S. Memory b cells and long-lived plasma cells. Transplantation (2019) 103(5):890–8. doi: 10.1097/TP.0000000000002594
16. Nguyen DC, Joyner CJ, Sanz I, Lee FEH. Factors affecting early antibody secreting cell maturation into long-lived plasma cells. Front Immunol (2019) 10. doi: 10.3389/fimmu.2019.02138
17. Chong AS, Sage PT, Alegre ML. Regulation of alloantibody responses. Front Cell Dev Biol (2021) 9:1710. doi: 10.3389/fcell.2021.706171
18. Khiew SHW, Jain D, Chen J, Yang J, Yin D, Young JS, et al. Transplantation tolerance modifies donor-specific b cell fate to suppress de novo alloreactive b cells. J Clin Invest (2020) 130(7):3453–66. doi: 10.1172/JCI132814
19. Vinuesa CG, Linterman MA, Yu D, Maclennan ICM. Follicular helper T cells. Annu Rev Immunol (2016) 34:335–68. doi: 10.1146/annurev-immunol-041015-055605
20. Choi J, Diao H, Faliti CE, Truong J, Rossi M, Bélanger S, et al. Bcl-6 is the nexus transcription factor of T follicular helper cells via repressor-of-repressor circuits. Nat Immunol (2020) 21(7):777–89. doi: 10.1038/s41590-020-0706-5
21. Crotty S. T Follicular helper cell biology: a decade of discovery and diseases. Immunity (2019) 50(5):1132–48. doi: 10.1016/j.immuni.2019.04.011
22. Liu X, Nurieva RI, Dong C. Transcriptional regulation of follicular T-helper (Tfh) cells. Immunol Rev (2013) 252(1):139–45. doi: 10.1111/imr.12040
23. Nurieva RI, Chung Y. Understanding the development and function of T follicular helper cells. Cell Mol Immunol (2010) 7:190–7. doi: 10.1038/cmi.2010.24
24. Ji LS, Sun XH, Zhang X, Zhou ZH, Yu Z, Zhu XJ, et al. Mechanism of follicular helper T cell differentiation regulated by transcription factors. J Immunol Res (2020) 2020:1826587. doi: 10.1155/2020/1826587
25. Xu W, Zhao X, Wang X, Feng H, Gou M, Jin W, et al. The transcription factor Tox2 drives T follicular helper cell development via regulating chromatin accessibility. Immunity (2019) 51(5):826–839.e5. doi: 10.1016/j.immuni.2019.10.006
26. Read KA, Jones DM, Pokhrel S, Hales EDS, Varkey A, Tuazon JA, et al. Aiolos represses CD4+ T cell cytotoxic programming via reciprocal regulation of TFH transcription factors and IL-2 sensitivity. Nat Commun (2023) 14(1):1652. doi: 10.1038/s41467-023-37420-0
27. Olatunde AC, Hale JS, Lamb TJ. Cytokine-skewed tfh cells: functional consequences for b cell help. Trends Immunol (2021) 42(6):536–50. doi: 10.1016/j.it.2021.04.006
28. Vella LA, Herati RS, Wherry EJ. CD4+ T cell differentiation in chronic viral infections: the tfh perspective. Trends Mol Med (2017) 23(12):1072–87. doi: 10.1016/j.molmed.2017.10.001
29. Crawford A, Angelosanto JM, Kao C, Doering TA, Odorizzi PM, Barnett BE, et al. Molecular and transcriptional basis of CD4+ T cell dysfunction during chronic infection. Immunity (2014) 40(2):289–302. doi: 10.1016/j.immuni.2014.01.005
30. Yu S, Jia L, Zhang Y, Zhong J, Yang B, Wu C. IL-12 induced the generation of IL-21- and IFN-γ-co-expressing poly-functional CD4+ T cells from human naive CD4+ T cells. Cell Cycle (2015) 14(21):3362–72. doi: 10.1080/15384101.2015.1093703
31. Powell MD, Read KA, Sreekumar BK, Jones DM, Oestreich KJ. IL-12 signaling drives the differentiation and function of a TH1-derived TFH1-like cell population. Sci Rep (2019) 9(1):13991. doi: 10.1038/s41598-019-50614-1
32. de Giovanni M, Cutillo V, Giladi A, Sala E, Maganuco CG, Medaglia C, et al. Spatiotemporal regulation of type I interferon expression determines the antiviral polarization of CD4+ T cells. Nat Immunol (2020) 21(3):321–30. doi: 10.1038/s41590-020-0596-6
33. Whitmire JK, Tan JT, Whitton JL. Interferon-γ acts directly on CD8 + T cells to increase their abundance during virus infection. J Exp Med (2005) 201(7):1053–9. doi: 10.1084/jem.20041463
34. Huang S, Hendriks W, Althage A, Hemmi S, Bluethmann H, Kamijo R, et al. Immune response in mice that lack the interferon-y receptor. Sci (1979). (1993) 259(5102):1742–5. doi: 10.1126/science.8456301
35. Weinstein JS, Laidlaw BJ, Lu Y, Wang JK, Schulz VP, Li N, et al. STAT4 and T-bet control follicular helper T cell development in viral infections. J Exp Med (2018) 215(1):337–55. doi: 10.1084/jem.20170457
36. Ma X, Nakayamada S, Kubo S, Sakata K, Yamagata K, Miyazaki Y, et al. Expansion of T follicular helper-T helper 1 like cells through epigenetic regulation by signal transducer and activator of transcription factors. Ann Rheum Dis (2018) 77(9):1354–61. doi: 10.1136/annrheumdis-2017-212652
37. Schmitt N, Bentebibel SE, Ueno H. Phenotype and functions of memory tfh cells in human blood. Trends Immunol (2014) 35(9):436–42. doi: 10.1016/j.it.2014.06.002
38. Xiao N, Eto D, Elly C, Peng G, Crotty S, Liu YC. The E3 ubiquitin ligase itch is required for the differentiation of follicular helper T cells. Nat Immunol (2014) 15(7):657–66. doi: 10.1038/ni.2912
39. Zhu J, Min B, Hu-Li J, Watson CJ, Grinberg A, Wang Q, et al. Conditional deletion of Gata3 shows its essential function in T(H)1-T(H)2 responses. Nat Immunol (2004) 5(11):1157–65. doi: 10.1038/ni1128
40. Takeda H, Tanaka T, Shi W, Matsumoto M, Minami M, Kashiwamura SI, et al. Essential role of Stat6 in IL-4 signalling. Nature (1996) 380(6575):627–30. doi: 10.1038/380627a0
41. Gonzalez DG, Cote CM, Patel JR, Smith CB, Zhang Y, Nickerson KM, et al. Nonredundant roles of IL-21 and IL-4 in the phased initiation of germinal center b cells and subsequent self-renewal transitions. J Immunol (2018) 201(12):3569–79. doi: 10.4049/jimmunol.1500497
42. Akiyama M, Yasuoka H, Yamaoka K, Suzuki K, Kaneko Y, Kondo H, et al. Enhanced IgG4 production by follicular helper 2 T cells and the involvement of follicular helper 1 T cells in the pathogenesis of IgG4-related disease. Arthritis Res Ther (2016) 18(1):167. doi: 10.1186/s13075-016-1064-4
43. Akiyama M, Yasuoka H, Yoshimoto K, Takeuchi T. Interleukin-4 contributes to the shift of balance of IgG subclasses toward IgG4 in IgG4-related disease. Cytokine (2018) 110:416–9. doi: 10.1016/j.cyto.2018.05.009
44. Cargill T, Makuch M, Sadler R, Lighaam LC, Peters R, van Ham M, et al. Activated T-follicular helper 2 cells are associated with disease activity in IgG4-related sclerosing cholangitis and pancreatitis. Clin Transl Gastroenterol (2019) 10(4):e00020. doi: 10.14309/ctg.0000000000000020
45. Bao K, Isik Can U, Miller MM, Brown IK, Dell’Aringa M, Dooms H, et al. A bifurcated role for c-maf in Th2 and Tfh2 cells during helminth infection. Mucosal Immunol (2023) 16(3):357–72. doi: 10.1016/j.mucimm.2023.04.002
46. Bauquet AT, Jin H, Paterson AM, Mitsdoerffer M, Ho IC, Sharpe AH, et al. The costimulatory molecule ICOS regulates the expression of c-maf and IL-21 in the development of follicular T helper cells and TH-17 cells. Nat Immunol (2009) 10(2):167–75. doi: 10.1038/ni.1690
47. Hsu HC, Yang PA, Wang J, Wu Q, Myers R, Chen J, et al. Interleukin 17-producing T helper cells and interleukin 17 orchestrate autoreactive germinal center development in autoimmune BXD2 mice. Nat Immunol (2008) 9(2):166–75. doi: 10.1038/ni1552
48. Mitsdoerffer M, Lee Y, Jäger A, Kim HJ, Korn T, Kolls JK, et al. Proinflammatory T helper type 17 cells are effective b-cell helpers. Proc Natl Acad Sci U.S.A. (2010) 107(32):14292–7. doi: 10.1073/pnas.1009234107
49. Linterman MA, Pierson W, Lee SK, Kallies A, Kawamoto S, Rayner TF, et al. Foxp3+ follicular regulatory T cells control the germinal center response. Nat Med (2011) 17(8):975–82. doi: 10.1038/nm.2425
50. Sage PT, Paterson AM, Lovitch SB, Sharpe AH. The coinhibitory receptor CTLA-4 controls b cell responses by modulating T follicular helper, T follicular regulatory, and T regulatory cells. Immunity (2014) 41(6):1026–39. doi: 10.1016/j.immuni.2014.12.005
51. Aloulou M, Carr EJ, Gador M, Bignon A, Liblau RS, Fazilleau N, et al. Follicular regulatory T cells can be specific for the immunizing antigen and derive from naive T cells. Nat Commun (2016) 7:10579. doi: 10.1038/ncomms10579
52. Wollenberg I, Agua-Doce A, Hernández A, Almeida C, Oliveira VG, Faro J, et al. Regulation of the germinal center reaction by Foxp3+ follicular regulatory T cells. J Immunol (2011) 187(9):4553–60. doi: 10.4049/jimmunol.1101328
53. Chung Y, Tanaka S, Chu F, Nurieva RI, Martinez GJ, Rawal S, et al. Follicular regulatory T cells expressing Foxp3 and bcl-6 suppress germinal center reactions. Nat Med (2011) 17(8):983–8. doi: 10.1038/nm.2426
54. Jandl C, Liu SM, Cañete PF, Warren J, Hughes WE, Vogelzang A, et al. IL-21 restricts T follicular regulatory T cell proliferation through bcl-6 mediated inhibition of responsiveness to IL-2. Nat Commun (2017) 8:14647. doi: 10.1038/ncomms14647
55. Botta D, Fuller MJ, Marquez-Lago TT, Bachus H, Bradley JE, Weinmann AS, et al. Dynamic regulation of T follicular regulatory cell responses by interleukin 2 during influenza infection. Nat Immunol (2017) 18(11):1249–60. doi: 10.1038/ni.3837
56. Wing JB, Kitagawa Y, Locci M, Hume H, Tay C, Morita T, et al. A distinct subpopulation of CD25– T-follicular regulatory cells localizes in the germinal centers. Proc Natl Acad Sci U.S.A. (2017) 114(31):E6400–9. doi: 10.1073/pnas.1705551114
57. Liu X, Chen X, Zhong B, Wang A, Wang X, Chu F, et al. Transcription factor achaete-scute homologue 2 initiates follicular T-helper-cell development. Nature (2014) 507(7493):513–8. doi: 10.1038/nature12910
58. Pace L, Tempez A, Arnold-Schrauf C, Lemaitre F, Bousso P, Fetler L, et al. Regulatory T cells increase the avidity of primary CD8+ T cell responses and promote memory. Science (2012) 338(6106):532–6. doi: 10.1126/science.1227049
59. Morlacchi S, Dal Secco V, Soldani C, Glaichenhaus N, Viola A, Sarukhan A. Regulatory T cells target chemokine secretion by dendritic cells independently of their capacity to regulate T cell proliferation. J Immunol (2011) 186(12):6807–14. doi: 10.4049/jimmunol.1003265
60. Dal Secco V, Soldani C, Debrat C, Asperti-Boursin F, Donnadieu E, Viola A, et al. Tunable chemokine production by antigen presenting dendritic cells in response to changes in regulatory T cell frequency in mouse reactive lymph nodes. PloS One (2009) 4(11):e7696. doi: 10.1371/journal.pone.0007696
61. Lu Y, Craft J. T Follicular regulatory cells: choreographers of productive germinal center responses. Front Immunol (2021) 12:2267. doi: 10.3389/fimmu.2021.679909
62. Gonzalez-Figueroa P, Roco JA, Papa I, Núñez Villacís L, Stanley M, Linterman MA, et al. Follicular regulatory T cells produce neuritin to regulate b cells. Cell (2021) 184(7):1775–1789.e19. doi: 10.1016/j.cell.2021.02.027
63. Hou S, Clement RL, Diallo A, Blazar BR, Rudensky AY, Sharpe AH, et al. FoxP3 and Ezh2 regulate tfr cell suppressive function and transcriptional program. J Exp Med (2019) 216(3):605–20. doi: 10.1084/jem.20181134
64. Gowthaman U, Chen JS, Zhang B, Flynn WF, Lu Y, Song W, et al. Identification of a T follicular helper cell subset that drives anaphylactic IgE. Science (2019) 365(6456):eaaw6433. doi: 10.1126/science.aaw6433
65. Chen JS, Grassmann JDS, Gowthaman U, Olyha SJ, Simoneau T, Berin MC, et al. Flow cytometric identification of Tfh13 cells in mouse and human. J Allergy Clin Immunol (2021) 147(2):470. doi: 10.1016/j.jaci.2020.04.063
66. Ma CS, Deenick EK, Batten M, Tangye SG. The origins, function, and regulation of T follicular helper cells. J Exp Med (2012) 209(7):1241–53. doi: 10.1084/jem.20120994
67. Ise W, Fujii K, Shiroguchi K, Ito A, Kometani K, Takeda K, et al. T Follicular helper cell-germinal center b cell interaction strength regulates entry into plasma cell or recycling germinal center cell fate. Immunity (2018) 48(4):702–715.e4. doi: 10.1016/j.immuni.2018.03.027
68. Ebert LM, Horn MP, Lang AB, Moser B. B cells alter the phenotype and function of follicular-homing CXCR5+ T cells. Eur J Immunol (2004) 34(12):3562–71. doi: 10.1002/eji.200425478
69. Vogelzang A, McGuire HM, Yu D, Sprent J, Mackay CR, King C. A fundamental role for interleukin-21 in the generation of T follicular helper cells. Immunity (2008) 29(1):127–37. doi: 10.1016/j.immuni.2008.06.001
70. Nurieva RI, Chung Y, Hwang D, Yang XO, Kang HS, Ma L, et al. Generation of T follicular helper cells is mediated by interleukin-21 but independent of T helper 1, 2, or 17 cell lineages. Immunity (2008) 29(1):138–49. doi: 10.1016/j.immuni.2008.05.009
71. Nurieva R, Yang XO, Martinez G, Zhang Y, Panopoulos AD, Ma L, et al. Essential autocrine regulation by IL-21 in the generation of inflammatory T cells. Nature (2007) 448(7152):480–3. doi: 10.1038/nature05969
72. Locci M, Havenar-Daughton C, Landais E, Wu J, Kroenke MA, Arlehamn CL, et al. Human circulating PD-1+CXCR3-CXCR5+ memory tfh cells are highly functional and correlate with broadly neutralizing HIV antibody responses. Immunity (2013) 39(4):758–69. doi: 10.1016/j.immuni.2013.08.031
73. Boyd SD, Jackson KJL. Predicting vaccine responsiveness. Cell Host Microbe (2015) 17(3):301–7. doi: 10.1016/j.chom.2015.02.015
74. Pilkinton MA, Nicholas KJ, Warren CM, Smith RM, Yoder SM, Talbot HK, et al. Greater activation of peripheral T follicular helper cells following high dose influenza vaccine in older adults forecasts seroconversion. Vaccine (2017) 35(2):329–36. doi: 10.1016/j.vaccine.2016.11.059
75. Pitisuttithum P, Boonnak K, Chamnanchanunt S, Puthavathana P, Luvira V, Lerdsamran H, et al. Safety and immunogenicity of a live attenuated influenza H5 candidate vaccine strain A/17/turkey/Turkey/05/133 H5N2 and its priming effects for potential pre-pandemic use: a randomised, double-blind, placebo-controlled trial. Lancet Infect Dis (2017) 17(8):833–42. doi: 10.1016/S1473-3099(17)30240-2
76. de Armas LR, Cotugno N, Pallikkuth S, Pan L, Rinaldi S, Sanchez MC, et al. Induction of IL21 in peripheral T follicular helper cells is an indicator of influenza vaccine response in a previously vaccinated HIV-infected pediatric cohort. J Immunol (2017) 198(5):1995–2005. doi: 10.4049/jimmunol.1601425
77. Amodio D, Cotugno N, Macchiarulo G, Rocca S, Dimopoulos Y, Castrucci MR, et al. Quantitative multiplexed imaging analysis reveals a strong association between immunogen-specific b cell responses and tonsillar germinal center immune dynamics in children after influenza vaccination. J Immunol (2018) 200(2):538–50. doi: 10.4049/jimmunol.1701312
78. Havenar-Daughton C, Lindqvist M, Heit A, Wu JE, Reiss SM, Kendric K, et al. CXCL13 is a plasma biomarker of germinal center activity. Proc Natl Acad Sci U.S.A. (2016) 113(10):2702–7. doi: 10.1073/pnas.1520112113
79. Huang Q, Hu J, Tang J, Xu L, Ye L. Molecular basis of the differrentiation and function of virus specific follicular helper CD4+ T cells. Front Immunol (2019) 10(FEB):249. doi: 10.3389/fimmu.2019.00249
80. Qi H. T Follicular helper cells in space-time. Nat Rev Immunol (2016) 16(10):612–25. doi: 10.1038/nri.2016.94
81. Lee JY, Skon CN, Lee YJ, Oh S, Taylor JJ, Malhotra D, et al. The transcription factor KLF2 restrains CD4+ T follicular helper cell differentiation. Immunity (2015) 42(2):252–64. doi: 10.1016/j.immuni.2015.01.013
82. Tian Y, Mollo SB, Harrington LE, Zajac AJ. IL-10 regulates memory T cell development and the balance between Th1 and follicular Th cell responses during an acute viral infection. J Immunol (2016) 197(4):1308–21. doi: 10.4049/jimmunol.1502481
83. He J, Tsai LM, Leong YA, Hu X, Ma CS, Chevalier N, et al. Circulating precursor CCR7loPD-1hi CXCR5+ CD4+ T cells indicate tfh cell activity and promote antibody responses upon antigen reexposure. Immunity (2013) 39(4):770–81. doi: 10.1016/j.immuni.2013.09.007
84. Greczmiel U, Oxenius A. The janus face of follicular T helper cells in chronic viral infections. Front Immunol (2018) 9(MAY):1162. doi: 10.3389/fimmu.2018.01162
85. Kahan SM, Wherry EJ, Zajac AJ. T Cell exhaustion during persistent viral infections. Virology (2015) 479–480:180–93. doi: 10.1016/j.virol.2014.12.033
86. Greczmiel U, Kräutler NJ, Pedrioli A, Bartsch I, Agnellini P, Bedenikovic G, et al. Sustained T follicular helper cell response is essential for control of chronic viral infection. Sci Immunol (2017) 2(18):eaam8686. doi: 10.1126/sciimmunol.aam8686
87. de Jong A. Activation of human T cells by CD1 and self-lipids. Immunol Rev (2015) 267(1):16–29. doi: 10.1111/imr.12322
88. Hinks TSC, Zhang XW. MAIT cell activation and functions. Front Immunol (2020) 11. doi: 10.3389/fimmu.2020.01014
89. Farkash EA, Colvin RB. Pathology: diagnostic challenges in chronic antibody-mediated rejection. Nat Rev Nephrol (2012) 8(5):255–7. doi: 10.1038/nrneph.2012.61
90. Foster BJ, Dahhou M, Zhang X, Platt RW, Hanley JA. Relative importance of HLA mismatch and donor age to graft survival in young kidney transplant recipients. Transplantation (2013) 96(5):469–75. doi: 10.1097/TP.0b013e318298f9db
91. Kamburova EG, Wisse BW, Joosten I, Allebes WA, van der Meer A, Hilbrands LB, et al. Differential effects of donor-specific HLA antibodies in living versus deceased donor transplant. Am J Transplant (2018) 18(9):2274–84. doi: 10.1111/ajt.14709
92. Bon D, Chatauret N, Giraud S, Thuillier R, Favreau F, Hauet T. New strategies to optimize kidney recovery and preservation in transplantation. Nat Rev Nephrol (2012) 8(6):339–47. doi: 10.1038/nrneph.2012.83
93. Cheadle C, Watkins T, Ehrlich E, Barnes K, Osama Gaber A, Hemmerich S, et al. Effects of anti-adhesive therapy on kidney biomarkers of ischemia reperfusion injury in human deceased donor kidney allografts. Clin Transplant (2011) 25(5):766–75. doi: 10.1111/j.1399-0012.2010.01365.x
94. Jayle C, Milinkevitch S, Favreau F, Doucet C, Richer JP, Deretz S, et al. Protective role of selectin ligand inhibition in a large animal model of kidney ischemia-reperfusion injury. Kidney Int (2006) 69(10):1749–55. doi: 10.1038/sj.ki.5000335
95. Rong S, Park JK, Kirsch T, Yagita H, Akiba H, Boenisch O, et al. The TIM-1:TIM-4 pathway enhances renal ischemia-reperfusion injury. J Am Soc Nephrol (2011) 22(3):484–95. doi: 10.1681/ASN.2010030321
96. Askar M. T Helper subsets & regulatory T cells: rethinking the paradigm in the clinical context of solid organ transplantation. Int J Immunogenet (2014) 41(3):185–94. doi: 10.1111/iji.12106
97. Tang Q, Leung J, Melli K, Lay K, Chuu EL, Liu W, et al. Altered balance between effector T cells and FOXP3+ HELIOS+ regulatory T cells after thymoglobulin induction in kidney transplant recipients. Transpl Int (2012) 25(12):1257–67. doi: 10.1111/j.1432-2277.2012.01565.x
98. Krystufkova E, Sekerkova A, Striz I, Brabcova I, Girmanova E, Viklicky O. Regulatory T cells in kidney transplant recipients: the effect of induction immunosuppression therapy. Nephrol Dial Transplant (2012) 27(6):2576–82. doi: 10.1093/ndt/gfr693
99. Chen M, Lin X, Li C, Olsen N, He X, Zheng SG. Advances in T follicular helper and T follicular regulatory cells in transplantation immunity. Transplant Rev (Orlando) (2018) 32(4):187–93. doi: 10.1016/j.trre.2018.07.002
100. Chen W, Bai J, Huang H, Bi L, Kong X, Gao Y, et al. Low proportion of follicular regulatory T cell in renal transplant patients with chronic antibody-mediated rejection. Sci Rep (2017) 7(1):1322. doi: 10.1038/s41598-017-01625-3
101. Lal G, Kulkarni N, Nakayama Y, Singh AK, Sethi A, Burrell BE, et al. IL-10 from marginal zone precursor b cells controls the differentiation of Th17, tfh and tfr cells in transplantation tolerance. Immunol Lett (2016) 170:52–63. doi: 10.1016/j.imlet.2016.01.002
102. Yang B, He F, Dai C, Tan R, Ma D, Wang Z, et al. BATF inhibition prevent acute allograft rejection after cardiac transplantation. Am J Transl Res (2016) 8(8):3603.
103. Subburayalu J, Dolff S, Xu S, Sun M, Lindemann M, Heinold A, et al. Characterization of follicular T helper cells and donor-specific T helper cells in renal transplant patients with de novo donor-specific HLA-antibodies. Clin Immunol (2021) 226(May 2021):108698. doi: 10.1016/j.clim.2021.108698
104. Davis TE, Kis-Toth K, Szanto A, Tsokos GC. Glucocorticoids suppress T cell function by up-regulating microRNA-98. Arthritis Rheum (2013) 65(7):1882–90. doi: 10.1002/art.37966
105. Jonsson CA, Carlsten H. Inosine monophosphate dehydrogenase (IMPDH) inhibition in vitro suppresses lymphocyte proliferation and the production of immunoglobulins, autoantibodies and cytokines in splenocytes from MRLlpr/lpr mice. Clin Exp Immunol (2001) 124(3):486–91. doi: 10.1046/j.1365-2249.2001.01545.x
106. Wang Y, Shi J, Yan J, Xiao Z, Hou X, Lu P, et al. Germinal-center development of memory b cells driven by IL-9 from follicular helper T cells. Nat Immunol (2017) 18(8):921–30. doi: 10.1038/ni.3788
107. Kim I, Wu G, Chai N-N, Klein AS, Jordan SC. Immunological characterization of de novo and recall alloantibody suppression by CTLA4Ig in a mouse model of allosensitization. Transpl Immunol (2016) 38:84–92. doi: 10.1016/j.trim.2016.08.001
108. de Leur K, Dor FJMF, Dieterich M, van der Laan LJW, Hendriks RW, Baan CC. IL-21 receptor antagonist inhibits differentiation of b cells toward plasmablasts upon alloantigen stimulation. Front Immunol (2017) 8(MAR):306. doi: 10.3389/fimmu.2017.00306
109. Corsiero E, Nerviani A, Bombardieri M, Pitzalis C. Ectopic lymphoid structures: powerhouse of autoimmunity. Front Immunol (2016) 7(OCT):430. doi: 10.3389/fimmu.2016.00430
110. Jones GW, Jones SA, Building T, Jones GW. Ectopic lymphoid follicles: inducible centres for generating antigen-specific immune responses within tissues. Immunology (2016) 147(2):141–51. doi: 10.1111/imm.12554
111. Cyster JC. Chemokines and cell migration in secondary lymphoid organs. Science (1999) 286(5447):2098–102. doi: 10.1126/science.286.5447.2098
112. Cyster JG. Chemokines and the homing of dendritic cells to the T cell areas of lymphoid organs. J Exp Med (1999) 189(3):447–50. doi: 10.1084/jem.189.3.447
113. Rangel-Moreno J, Moyron-Quiroz JE, Hartson L, Kusser K, Randall TD. Pulmonary expression of CXC chemokine ligand 13, CC chemokine ligand 19, and CC chemokine ligand 21 is essential for local immunity to influenza. Proc Natl Acad Sci U.S.A. (2007) 104(25):10577–82. doi: 10.1073/pnas.0700591104
114. Ludwig RJ, Vanhoorelbeke K, Leypoldt F, Kaya Z, Bieber K, McLachlan SM, et al. Mechanisms of autoantibody-induced pathology. Front Immunol (2017) 8(MAY). doi: 10.3389/fimmu.2017.00603
115. Odegard JM, Marks BR, Diplacido LD, Poholek AC, Kono DH, Dong C, et al. ICOS-dependent extrafollicular helper T cells elicit IgG production via IL-21 in systemic autoimmunity. J Exp Med (2008) 205(12):2873–86. doi: 10.1084/jem.20080840
116. Buhre JS, Becker M, Ehlers M. IgG subclass and fc glycosylation shifts are linked to the transition from pre- to inflammatory autoimmune conditions. Front Immunol (2022) 13:6717. doi: 10.3389/fimmu.2022.1006939
117. Moyron-Quiroz JE, Rangel-Moreno J, Kusser K, Hartson L, Sprague F, Goodrich S, et al. Role of inducible bronchus associated lymphoid tissue (iBALT) in respiratory immunity. Nat Med (2004) 10(9):927–34. doi: 10.1038/nm1091
118. Singh D, Henkel M, Sendon B, Feng J, Fabio A, Metes D, et al. Analysis of CXCR5+Th17 cells in relation to disease activity and TNF inhibitor therapy in rheumatoid arthritis. Sci Rep (2016) 6:39474. doi: 10.1038/srep39474
119. Zhang L, Li W, Cai Y, Liu X, Peng Q, Liang L. Aberrant expansion of circulating CD4+ CXCR5+ CCR7lo PD1hi tfh precursor cells in idiopathic inflammatory myopathy. Int J Rheum Dis (2020) 23(3):397–405. doi: 10.1111/1756-185X.13782
120. Deng J, Fan C, Gao X, Zeng Q, Guo R, Wei Y, et al. Signal transducer and activator of transcription 3 hyperactivation associates with follicular helper T cell differentiation and disease activity in rheumatoid arthritis. Front Immunol (2018) 9(JUN). doi: 10.3389/fimmu.2018.01226
121. Paquissi FC, Abensur H. The Th17/IL-17 axis and kidney diseases, with focus on lupus nephritis. Front Med (Lausanne) (2021) 8:1432. doi: 10.3389/fmed.2021.654912
122. Wang N, Gao C, Cui S, Qin Y, Zhang C, Yi P, et al. Induction therapy downregulates the expression of Th17/Tfh cytokines in patients with active lupus nephritis. Am J Clin Exp Immunol (2018) 7(4):67–75.
123. Liu C, Wang D, Lu S, Xu Q, Zhao L, Zhao J, et al. Increased circulating follicular treg cells are associated with lower levels of autoantibodies in patients with rheumatoid arthritis in stable remission. Arthritis Rheumatol (2018) 70(5):711–21. doi: 10.1002/art.40430
124. Xu B, Wang S, Zhou M, Huang Y, Fu R, Guo C, et al. The ratio of circulating follicular T helper cell to follicular T regulatory cell is correlated with disease activity in systemic lupus erythematosus. Clin Immunol (2017) 183:46–53. doi: 10.1016/j.clim.2017.07.004
125. Turesson C. Endothelial expression of MHC class II molecules in autoimmune disease. Curr Pharm Des (2004) 10(2):129–43. doi: 10.2174/1381612043453414
126. Cross AR, Lion J, Poussin K, Glotz D, Mooney N. Inflammation determines the capacity of allogenic endothelial cells to regulate human treg expansion. Front Immunol (2021) 12:2724. doi: 10.3389/fimmu.2021.666531
127. Pitzalis C, Jones GW, Bombardieri M, Jones SA. Ectopic lymphoid-like structures in infection, cancer and autoimmunity. Nat Rev Immunol (2014) 14(7):447–62. doi: 10.1038/nri3700
128. Nerviani A, Pitzalis C. Role of chemokines in ectopic lymphoid structures formation in autoimmunity and cancer. J Leukoc Biol (2018) 104(2):333–41. doi: 10.1002/JLB.3MR0218-062R
129. Moschovakis GL, Bubke A, Friedrichsen M, Falk CS, Feederle R, Förster R. T Cell specific Cxcr5 deficiency prevents rheumatoid arthritis. Sci Rep (2017) 7(1):8933. doi: 10.1038/s41598-017-08935-6
130. Wengner AM, Höpken UE, Petrow PK, Hartmann S, Schurigt U, Bräuer R, et al. CXCR5- and CCR7-dependent lymphoid neogenesis in a murine model of chronic antigen-induced arthritis. Arthritis Rheum (2007) 56(10):3271–83. doi: 10.1002/art.22939
131. Manzo A, Bombardieri M, Humby F, Pitzalis C. Secondary and ectopic lymphoid tissue responses in rheumatoid arthritis: from inflammation to autoimmunity and tissue damage/remodeling. Immunol Rev (2010) 233(1):267–85. doi: 10.1111/j.0105-2896.2009.00861.x
132. Nosanchuk JS, Schnitzer B. Follicular hyperplasia in lymph nodes from patients with rheumatoid arthritis. A clinicopathol study Cancer (1969) 24(2):243–54. doi: 10.1002/1097-0142(196908)24:2<343::aid-cncr2820240217>3.0.co;2-c
133. Imai Y, Sato T, Yamakawa M, Kasajima T, Suda A, Watanabe Y. A morphological and immunohistochemical study of lymphoid germinal centers in synovial and lymph node tissues from rheumatoid arthritis patients with special reference to complement components and their receptors. Acta Pathol Jpn (1989) 39(2):127–34. doi: 10.1111/j.1440-1827.1989.tb01490.x
134. Yang L, Xie H, Liu Z, Chen Y, Wang J, Zhang H, et al. Risk factors for infectious complications of ANCA-associated vasculitis: a cohort study. BMC Nephrol (2018) 19(1):138. doi: 10.1186/s12882-018-0933-2
135. Philogene MC, Brennan DC. Kidney transplantation in adults: HLA matching and outcomes (2021). Available at: https://www.uptodate.com/contents/kidney-transplantation-in-adults-hla-matching-and-outcomes?search=Kidney%20transplantation%20in%20adults:%20HLA%20matching%20and%20outcomes&source=search_result&selectedTitle=1~150&usage_type=default&display_rank=1.
136. Kramer CSM, Roelen DL, Heidt S, Claas FHJ. Defining the immunogenicity and antigenicity of HLA epitopes is crucial for optimal epitope matching in clinical renal transplantation. HLA (2017) 90(1):5–16. doi: 10.1111/tan.13038
137. Aubert V, Venetz JP, Pantaleo G, Pascual M. Low levels of human leukocyte antigen donor-specific antibodies detected by solid phase assay before transplantation are frequently clinically irrelevant. Hum Immunol (2009) 70(8):580–3. doi: 10.1016/j.humimm.2009.04.011
138. Lee H, Min JW, Kim J, Moon IS, Park KH, Yang CW, et al. Clinical significance of HLA-DQ antibodies in the development of chronic antibody-mediated rejection and allograft failure in kidney transplant recipients. Medicine (2016) 95(11):e3094. doi: 10.1097/MD.0000000000003094
139. Cole RT, Gandhi J, Bray RA, Gebel HM, Morris A, McCue A, et al. De novo DQ donor-specific antibodies are associated with worse outcomes compared to non-DQ de novo donor-specific antibodies following heart transplantation. Clin Transplant (2017) 31(4):e12924. doi: 10.1111/ctr.12924
140. Tikkanen JM, Singer LG, Kim SJ, Li Y, Binnie M, Chaparro C, et al. De novo DQ donor-specific antibodies are associated with chronic lung allograft dysfunction after lung transplantation. Am J Respir Crit Care Med (2016) 194(5):596–606. doi: 10.1164/rccm.201509-1857OC
141. Loupy A, Hill GS, Jordan SC. The impact of donor-specific anti-HLA antibodies on late kidney allograft failure. Nat Rev Nephrol (2012) 8(6):348–57. doi: 10.1038/nrneph.2012.81
142. Wiebe C, Gibson IW, Blydt-Hansen TD, Karpinski M, Ho J, Storsley LJ, et al. Evolution and clinical pathologic correlations of de novo donor-specific HLA antibody post kidney transplant. Am J Transplant (2012) 12(5):1157–67. doi: 10.1111/j.1600-6143.2012.04013.x
143. Farkash EA, Colvin RB. Diagnostic challenges in chronic antibody-mediated rejection. Nat Rev Nephrol (2012) 8(5):255–7. doi: 10.1038/nrneph.2012.61
144. Sis B, Jhangri GS, Bunnag S, Allanach K, Kaplan B, Halloran PF. Endothelial gene expression in kidney transplants with alloantibody indicates antibody-mediated damage despite lack of C4d staining. Am J Transplant (2009) 9(10):2312–23. doi: 10.1111/j.1600-6143.2009.02761.x
145. Gloor JM, Sethi S, Stegall MD, Park WD, Moore SB, DeGoey S, et al. Transplant glomerulopathy: subclinical incidence and association with alloantibody. Am J Transplant (2007) 7(9):2124–32. doi: 10.1111/j.1600-6143.2007.01895.x
146. Lefaucheur C, Loupy A, Vernerey D, Duong-Van-Huyen JP, Suberbielle C, Anglicheau D, et al. Antibody-mediated vascular rejection of kidney allografts: a population-based study. Lancet (2013) 381(9863):313–9. doi: 10.1016/S0140-6736(12)61265-3
147. Lefaucheur C, Viglietti D, Mangiola M, Loupy A, Zeevi A. From humoral theory to performant risk stratification in kidney transplantation. J Immunol Res (2017) 2017:5201098. doi: 10.1155/2017/5201098
148. Visentin J, Chartier A, Massara L, Linares G, Guidicelli G, Blanchard E, et al. Lung intragraft donor-specific antibodies as a risk factor for graft loss. J Heart Lung Transplant (2016) 35(12):1418–26. doi: 10.1016/j.healun.2016.06.010
149. Loupy A, Lefaucheur C, Vernerey D, Prugger C, van Huyen JPD, Mooney N, et al. Complement-binding anti-HLA antibodies and kidney-allograft survival. N Engl J Med (2013) 369(13):1215–26. doi: 10.1056/NEJMoa1302506
150. Djamali A, Kaufman DB, Ellis TM, Zhong W, Matas A, Samaniego M. Diagnosis and management of antibody-mediated rejection: current status and novel approaches. Am J Transplant (2014) 14(2):255–71. doi: 10.1111/ajt.12589
151. Nakamura T, Ushigome H, Watabe K, Imanishi Y, Masuda K, Matsuyama T, et al. Graft immunocomplex capture fluorescence analysis to detect donor-specific antibodies and HLA antigen complexes in the allograft. Immunol Invest (2017) 46(3):295–304. doi: 10.1080/08820139.2016.1258711
152. Einecke G, Sis B, Reeve J, Mengel M, Campbell PM, Hidalgo LG, et al. Antibody-mediated microcirculation injury is the major cause of late kidney transplant failure. Am J Transplant (2009) 9(11):2520–31. doi: 10.1111/j.1600-6143.2009.02799.x
153. Castro A, Malheiro J, Tafulo S, Dias L, Martins LS, Fonseca I, et al. Role of de novo donor-specific anti-HLA antibodies in kidney graft failure: a case-control study. HLA (2017) 90(5):267–75. doi: 10.1111/tan.13111
154. Haas M, Mirocha J, Reinsmoen NL, Vo AA, Choi J, Kahwaji JM, et al. Differences in pathologic features and graft outcomes in antibody-mediated rejection of renal allografts due to persistent/recurrent versus de novo donor-specific antibodies. Kidney Int (2017) 91(3):729–37. doi: 10.1016/j.kint.2016.10.040
155. Li W, Gauthier JM, Higashikubo R, Hsiao HM, Tanaka S, Vuong L, et al. Bronchus-associated lymphoid tissue-resident Foxp3+ T lymphocytes prevent antibody-mediated lung rejection. J Clin Invest (2019) 129(2):556–68. doi: 10.1172/JCI122083
156. Baan CC, de Graav GN, Boer K. T Follicular helper cells in transplantation: the target to attenuate antibody-mediated allogeneic responses? Curr Transplant Rep (2014) 1(3):166–72. doi: 10.1007/s40472-014-0019-4
157. Shi J, Luo F, Shi Q, Xu X, He X, Xia Y. Increased circulating follicular helper T cells with decreased programmed death-1 in chronic renal allograft rejection. BMC Nephrol (2015) 16(1):182. doi: 10.1186/s12882-015-0172-8
158. Shi J, Hou S, Fang Q, Liu X, Liu X, Qi H. PD-1 controls follicular T helper cell positioning and function. Immunity (2018) 49(2):264–274.e4. doi: 10.1016/j.immuni.2018.06.012
159. de Graav GN, Dieterich M, Hesselink DA, Boer K, Clahsen-van Groningen MC, Kraaijeveld R, et al. Follicular T helper cells and humoral reactivity in kidney transplant patients. Clin Exp Immunol (2015) 180(2):329–40. doi: 10.1111/cei.12576
160. Rodriguez-Barbosa JI, Fernandez-Renedo C, Moral AMB, Bühler L, Del Rio ML. T Follicular helper expansion and humoral-mediated rejection are independent of the HVEM/BTLA pathway. Cell Mol Immunol (2017) 14(6):497–510. doi: 10.1038/cmi.2015.101
161. Kim EJ, Kwun J, Gibby AC, Hong JJ, Farris AB, Iwakoshi NN, et al. Costimulation blockade alters germinal center responses and prevents antibody-mediated rejection. Am J Transplant (2014) 14(1):59–69. doi: 10.1111/ajt.12526
162. Mohammed MT, Cai S, Hanson BL, Zhang H, Clement RL, Daccache J, et al. Follicular T cells mediate donor specific antibody and rejection after solid organ transplantation. Am J Transplant (2021) 21(5):1893. doi: 10.1111/ajt.16484
163. Chenouard A, Chesneau M, Bui Nguyen L, le Bot S, Cadoux M, Dugast E, et al. Renal operational tolerance is associated with a defect of blood tfh cells that exhibit impaired b cell help. Am J Transplant (2017) 17(6):1490–501. doi: 10.1111/ajt.14142
164. Louis K, Macedo C, Bailly E, Lau L, Ramaswami B, Marrari M, et al. Coordinated circulating T follicular helper and activated b cell responses underlie the onset of antibody-mediated rejection in kidney transplantation. J Am Soc Nephrol (2020) 31(10):2457–74. doi: 10.1681/ASN.2020030320
165. Liu J, Tang T, Qu Z, Wang L, Si R, Wang H, et al. Elevated number of IL-21+ TFH and CD86+CD38+ b cells in blood of renal transplant recipients with AMR under conventional immuno-suppression. Int J Immunopathol Pharmacol (2022) 36:2058738421104802. doi: 10.1177/20587384211048027?icid=int.sj-abstract.similar-articles.9
166. Louis K, Fadakar P, Macedo C, Yamada M, Lucas M, Gu X, et al. Concomitant loss of regulatory T and b cells is a distinguishing immune feature of antibody-mediated rejection in kidney transplantation. Kidney Int (2022) 101(5):1003–16. doi: 10.1016/j.kint.2021.12.027
167. Kao C, Oestreich KJ, Paley MA, Crawford A, Angelosanto JM, Ali MAA, et al. Transcription factor T-bet represses expression of the inhibitory receptor PD-1 and sustains virus-specific CD8+ T cell responses during chronic infection. Nat Immunol (2011) 12(7):663–71. doi: 10.1038/ni.2046
168. Fu J, Wang D, Yu Y, Heinrichs J, Wu Y, Schutt S, et al. T-Bet is critical for the development of acute graft-versus-host disease through controlling T cell differentiation and function. J Immunol (2015) 194(1):388–97. doi: 10.4049/jimmunol.1401618
169. Chen JS, Chow RD, Song E, Mao T, Israelow B, Kamath K, et al. High-affinity, neutralizing antibodies to SARS-CoV-2 can be made without T follicular helper cells. Sci Immunol (2022) 7(68):eabl5652. doi: 10.1101/2021.06.10.447982
170. Mendoza A, Yewdell WT, Hoyos B, Schizas M, Bou-Puerto R, Michaels AJ, et al. Assembly of a spatial circuit of T-bet-expressing T and b lymphocytes is required for antiviral humoral immunity. Sci Immunol (2021) 6(60):eabi4710. doi: 10.1126/sciimmunol.abi4710
171. Moreso F, Carrera M, Goma M, Hueso M, Sellares J, Martorell J, et al. Early subclinical rejection as a risk factor for late chronic humoral rejection. Transplantation (2012) 93(1):41–6. doi: 10.1097/TP.0b013e31823bb647
172. Bestard O, Cruzado JM, Rama I, Torras J, Gomà M, Serón D, et al. Presence of FoxP3+ regulatory T cells predicts outcome of subclinical rejection of renal allografts. J Am Soc Nephrol (2008) 19(10):2020–6. doi: 10.1681/ASN.2007111174
173. Leibler C, Thiolat A, Hénique C, Samson C, Pilon C, Tamagne M, et al. Control of humoral response in renal transplantation by belatacept depends on a direct effect on b cells and impaired T follicular helper-b cell crosstalk. J Am Soc Nephrol (2018) 29(3):1049–62. doi: 10.1681/ASN.2017060679
174. Yang J, Lin X, Pan Y, Wang J, Chen P, Huang H, et al. Critical roles of mTOR complex 1 and 2 for T follicular helper cell differentiation and germinal center responses. Elife (2016) 5:e17936. doi: 10.7554/eLife.17936
175. Li YM, Li Y, Shi YY, Yan L, Wu XJ, Tang JT, et al. Impact of immunosuppressive drugs on circulating tfh cells in kidney transplant recipients: a pilot study. Transpl Immunol (2018) 46:1–7. doi: 10.1016/j.trim.2017.09.005
176. Pernin V, Meneghini M, Torija A, Jouve T, Del Bello A, Sanz-Muñoz I, et al. Impaired antigen-specific b-cell responses after influenza vaccination in kidney transplant recipients receiving co-stimulation blockade with belatacept. Front Immunol (2022) 13. doi: 10.3389/fimmu.2022.918887
177. Lederer K, Bettini E, Parvathaneni K, Painter MM, Agarwal D, Lundgreen KA, et al. Germinal center responses to SARS-CoV-2 mRNA vaccines in healthy and immunocompromised individuals. Cell (2022) 185(6):1008–1024.e15. doi: 10.1016/j.cell.2022.01.027
178. Lin J, Chen Y, Zhu H, Cheng K, Wang H, Yu X, et al. Lymphatic reconstruction in kidney allograft aggravates chronic rejection by promoting alloantigen presentation. Front Immunol (2021) 12. doi: 10.3389/fimmu.2021.796260
179. Thaunat O, Field AC, Dai J, Louedec L, Patey N, Bloch MF, et al. Lymphoid neogenesis in chronic rejection: evidence for a local humoral alloimmune response. Proc Natl Acad Sci U.S.A. (2005) 102(41):14723–8. doi: 10.1073/pnas.0507223102
180. Thaunat O, Patey N, Caligiuri G, Gautreau C, Mamani-Matsuda M, Mekki Y, et al. Chronic rejection triggers the development of an aggressive intragraft immune response through recapitulation of lymphoid organogenesis. J Immunol (2010) 185(1):717–28. doi: 10.4049/jimmunol.0903589
181. Edwards LA, Nowocin AK, Jafari NV, Meader LL, Brown K, Sarde A, et al. Chronic rejection of cardiac allografts is associated with increased lymphatic flow and cellular trafficking. Circulation (2018) 137(5):488–503. doi: 10.1161/CIRCULATIONAHA.117.028533
182. Zhang Z, Kaptanoglu L, Tang Y, Ivancic D, Rao SM, Luster A, et al. IP-10-induced recruitment of CXCR3 host T cells is required for small bowel allograft rejection. Gastroenterology (2004) 126(3):809–18. doi: 10.1053/j.gastro.2003.12.014
183. Fischer M, Leyking S, Schäfer M, Elsäßer J, Janssen M, Mihm J, et al. Donor-specific alloreactive T cells can be quantified from whole blood, and may predict cellular rejection after renal transplantation. Eur J Immunol (2017) 47(7):1220–31. doi: 10.1002/eji.201646826
184. Fan J-W, Yan L, Wang X-Q, Li Y-M, Bai Y-J, Ou X-Q, et al. The diagnostic role of PD-1+ CXCR5+ follicular helper CD8+ T cell in renal allograft dysfunction. J Clin Lab Anal (2022) 36(2):e24200. doi: 10.1002/jcla.24200
185. Zimmerer JM, Basinger MW, Ringwald BA, Abdel-Rasoul M, Pelletier RP, Rajab A, et al. Inverse association between the quantity of human peripheral blood CXCR5+IFN-γ+CD8+ T cells with de Novo DSA production in the first year after kidney transplant. Transplantation (2020) 104(11):2424–34. doi: 10.1097/TP.0000000000003151
186. Zimmerer JM, Han JL, Peterson CM, Zeng Q, Ringwald BA, Cassol C, et al. Antibody-suppressor CXCR5+CD8+ T cellular therapy ameliorates antibody-mediated rejection following kidney transplant in CCR5 KO mice. Am J Transplant (2022) 22(6):1550–63. doi: 10.1111/ajt.16988
187. Dragun D, Müller DN, Bräsen JH, Fritsche L, Nieminen-Kelhä M, Dechend R, et al. Angiotensin II type 1-receptor activating antibodies in renal-allograft rejection. N Engl J Med (2005) 352(6):558–69. doi: 10.1056/NEJMoa035717
188. Joosten SA, Sijpkens YWJ, Van Ham V, Trouw LA, van der Vlag J, Van Den Heuvel B, et al. Antibody response against the glomerular basement membrane protein agrin in patients with transplant glomerulopathy. Am J Transplant (2005) 5(2):383–93. doi: 10.1111/j.1600-6143.2005.00690.x
189. Angaswamy N, Klein C, Tiriveedhi V, Gaut J, Anwar S, Rossi A, et al. Immune responses to collagen-IV and fibronectin in renal transplant recipients with transplant glomerulopathy. Am J Transplant (2014) 14(3):685–93. doi: 10.1111/ajt.12592
190. Amico P, Hönger G, Bielmann D, Lutz D, Garzoni D, Steiger J, et al. Incidence and prediction of early antibody-mediated rejection due to non-human leukocyte antigen-antibodies. Transplantation (2008) 85(11):1557–63. doi: 10.1097/TP.0b013e31816f612a
191. Bharat A, Saini D, Steward N, Hachem R, Trulock EP, Patterson GA, et al. Antibodies to self-antigens predispose to primary lung allograft dysfunction and chronic rejection. Ann Thorac Surg (2010) 90(4):1094–101. doi: 10.1016/j.athoracsur.2010.06.009
192. Subramanian V, Ramachandran S, Banan B, Bharat A, Wang X, Benshoff N, et al. Immune response to tissue-restricted self-antigens induces airway inflammation and fibrosis following murine lung transplantation. Am J Transplant (2014) 14(10):2359–66. doi: 10.1111/ajt.12908
193. Hönger G, Cardinal H, Dieudé M, Buser A, Hösli I, Dragun D, et al. Human pregnancy and generation of anti-angiotensin receptor and anti-perlecan antibodies. Transpl Int (2014) 27(5):467–74. doi: 10.1111/tri.12282
194. Lukitsch I, Kehr J, Chaykovska L, Wallukat G, Nieminen-Kelhä M, Batuman V, et al. Renal ischemia and transplantation predispose to vascular constriction mediated by angiotensin II type 1 receptor-activating antibodies. Transplantation (2012) 94(1):8–13. doi: 10.1097/TP.0b013e3182529bb7
195. Dragun D, Catar R, Philippe A. Non-HLA antibodies in solid organ transplantation: recent concepts and clinical relevance. Curr Opin Organ Transplant (2013) 18(4):430–5. doi: 10.1097/MOT.0b013e3283636e55
196. Cardinal H, Dieudé M, Hébert MJ. The emerging importance of non-HLA autoantibodies in kidney transplant complications. J Am Soc Nephrol (2017) 28(2):400–6. doi: 10.1681/ASN.2016070756
197. Rose ML. Role of anti-vimentin antibodies in allograft rejection. Hum Immunol (2013) 74(11):1459–62. doi: 10.1016/j.humimm.2013.06.006
198. Zhang Q, Reed EF. The importance of non-HLA antibodies in transplantation. Nat Rev Nephrol (2016) 12(8):484–95. doi: 10.1038/nrneph.2016.88
199. Soulez M, Pilon EA, Dieudé M, Cardinal H, Brassard N, Qi S, et al. The perlecan fragment LG3 is a novel regulator of obliterative remodeling associated with allograft vascular rejection. Circ Res (2012) 110(1):94–104. doi: 10.1161/CIRCRESAHA.111.250431
200. Pilon EA, Dieudé M, Qi S, Hamelin K, Pomerleau L, Beillevaire D, et al. The perlecan fragment LG3 regulates homing of mesenchymal stem cells and neointima formation during vascular rejection. Am J Transplant (2015) 15(5):1205–18. doi: 10.1111/ajt.13119
201. Becker MO, Kill A, Kutsche M, Guenther J, Rose A, Tabeling C, et al. Vascular receptor autoantibodies in pulmonary arterial hypertension associated with systemic sclerosis. Am J Respir Crit Care Med (2014) 190(7):808–17. doi: 10.1164/rccm.201403-0442OC
202. Kill A, Tabeling C, Undeutsch R, Kühl AA, Günther J, Radic M, et al. Autoantibodies to angiotensin and endothelin receptors in systemic sclerosis induce cellular and systemic events associated with disease pathogenesis. Arthritis Res Ther (2014) 16(1):R29. doi: 10.1186/ar4457
203. Dieudé M, Bell C, Turgeon J, Beillevaire D, Pomerleau L, Yang B, et al. The 20S proteasome core, active within apoptotic exosome-like vesicles, induces autoantibody production and accelerates rejection. Sci Transl Med (2015) 7(318):318ra200. doi: 10.1126/scitranslmed.aac9816
204. Nagele EP, Han M, Acharya NK, DeMarshall C, Kosciuk MC, Nagele RG. Natural IgG autoantibodies are abundant and ubiquitous in human sera, and their number is influenced by age, gender, and disease. PloS One (2013) 8(4):e60726. doi: 10.1371/journal.pone.0060726
205. Padet L, Dieudé M, Karakeussian-Rimbaud A, Yang B, Turgeon J, Cailhier JF, et al. New insights into immune mechanisms of antiperlecan/LG3 antibody production: importance of T cells and innate B1 cells. Am J Transplant (2019) 19(3):699–712. doi: 10.1111/ajt.15082
206. Chen Y, Heeger PS, Valujskikh A. In vivo helper functions of alloreactive memory CD4+ T cells remain intact despite donor-specific transfusion and anti-CD40 ligand therapy. J Immunol (2004) 172(9):5456–66. doi: 10.4049/jimmunol.172.9.5456
207. Zhang Q, Chen Y, Fairchild RL, Heeger PS, Valujskikh A. Lymphoid sequestration of alloreactive memory CD4 T cells promotes cardiac allograft survival. J Immunol (2006) 176(2):770–7. doi: 10.4049/jimmunol.176.2.770
208. Gorbacheva V, Fan R, Wang X, Baldwin WM, Fairchild RL, Valujskikh A. IFN-γ production by memory helper T cells is required for CD40-independent alloantibody responses. J Immunol (2015) 194(3):1347–56. doi: 10.4049/jimmunol.1401573
209. Gorbacheva V, Ayasoufi K, Fan R, Baldwin WM, Valujskikh A. B cell activating factor (BAFF) and a proliferation inducing ligand (APRIL) mediate CD40-independent help by memory CD4 T cells. Am J Transplant (2015) 15(2):346–57. doi: 10.1111/ajt.12984
210. Sundaresan S, Mohanakumar T, Smith MA, Trulock EP, Lynch J, Phelan D, et al. HLA-a locus mismatches and development of antibodies to HLA after lung transplantation correlate with the development of bronchiolitis obliterans syndrome. Transplantation (1998) 65(5):648–53. doi: 10.1097/00007890-199803150-00008
211. Fábrega E, López-Hoyos M, San Segundo D, Casafont F, Pons-Romero F. Changes in the serum levels of interleukin-17/interleukin-23 during acute rejection in liver transplantation. Liver Transpl (2009) 15(6):629–33. doi: 10.1002/lt.21724
212. Millán O, Rafael-Valdivia L, San Segundo D, Boix F, Castro-Panete MJ, López-Hoyos M, et al. Should IFN-γ, IL-17 and IL-2 be considered predictive biomarkers of acute rejection in liver and kidney transplant? results of a multicentric study. Clin Immunol (2014) 154(2):141–54. doi: 10.1016/j.clim.2014.07.007
213. Zhang K, Sun YL, Yang F, Shi YC, Jin L, Liu ZW, et al. A pilot study on the characteristics of circulating T follicular helper cells in liver transplant recipients. Transpl Immunol (2018) 47:32–6. doi: 10.1016/j.trim.2018.01.003
214. Vendrame F, Pileggi A, Laughlin E, Allende G, Martin-Pagola A, Molano RD, et al. Recurrence of type 1 diabetes after simultaneous pancreas-kidney transplantation, despite immunosuppression, is associated with autoantibodies and pathogenic autoreactive CD4 T-cells. Diabetes (2010) 59(4):947–57. doi: 10.2337/db09-0498
215. Liston A, Lesage S, Wilson J, Peltonen L, Goodnow CC. Aire regulates negative selection of organ-specific T cells. Nat Immunol (2003) 4(4):350–4. doi: 10.1038/ni906
216. Bluestone JA, Bour-Jordan H, Cheng M, Anderson M. T Cells in the control of organ-specific autoimmunity. J Clin Invest (2015) 125(6):2250. doi: 10.1172/JCI78089
217. Gratz IK, Campbell DJ. Organ-specific and memory treg cells: specificity, development, function, and maintenance. Front Immunol (2014) 5(JUL):333. doi: 10.3389/fimmu.2014.00333
218. Jaramillo A, Smith MA, Phelan D, Sundaresan S, Trulock EP, Lynch JP, et al. Development of ELISA-detected anti-HLA antibodies precedes the development of bronchiolitis obliterans syndrome and correlates with progressive decline in pulmonary function after lung transplantation. Transplantation (1999) 67(8):1155–61. doi: 10.1097/00007890-199904270-00012
219. Girnita AL, McCurry KR, Iacono AT, Duquesnoy R, Corcoran TE, Awad M, et al. HLA-specific antibodies are associated with high-grade and persistent-recurrent lung allograft acute rejection. J Heart Lung Transplant (2004) 23(10):1135–41. doi: 10.1016/j.healun.2003.08.030
220. Girnita AL, Duquesnoy R, Yousem SA, Iacono AT, Corcoran TE, Buzoianu M, et al. HLA-specific antibodies are risk factors for lymphocytic bronchiolitis and chronic lung allograft dysfunction. Am J Transplant (2005) 5(1):131–8. doi: 10.1111/j.1600-6143.2004.00650.x
221. Vanaudenaerde BM, Dupont LJ, Wuyts WA, Verbeken EK, Meyts I, Bullens DM, et al. The role of interleukin-17 during acute rejection after lung transplantation. Eur Respir J (2006) 27(4):779–87. doi: 10.1183/09031936.06.00019405
222. Hachem R. Antibody-mediated lung transplant rejection. Curr Respir Care Rep (2012) 1(3):157–61. doi: 10.1007/s13665-012-0019-8
223. Krupnick AS, Lin X, Li W, Higashikubo R, Zinselmeyer BH, Hartzler H, et al. Central memory CD8+ T lymphocytes mediate lung allograft acceptance. J Clin Invest (2014) 124(3):1130–43. doi: 10.1172/JCI71359
224. Smirnova NF, Conlon TM, Morrone C, Dorfmuller P, Humbert M, Stathopoulos G, et al. Inhibition of b cell-dependent lymphoid follicle formation prevents lymphocytic bronchiolitis after lung transplantation. JCI Insight (2019) 4(3):e123971. doi: 10.1172/jci.insight.123971
225. Ochando JC, Yopp AC, Yang Y, Garin A, Li Y, Boros P, et al. Lymph node occupancy is required for the peripheral development of alloantigen-specific Foxp3+ regulatory T cells. J Immunol (2005) 174(11):6993–7005. doi: 10.4049/jimmunol.174.11.6993
226. Mittal S, Page SL, Friend PJ, Sharples EJ, Fuggle SV. De novo donor-specific HLA antibodies: biomarkers of pancreas transplant failure. Am J Transplant (2014) 14(7):1664–71. doi: 10.1111/ajt.12750
227. Cantarovich D, De Amicis S, Akl A, Devys A, Vistoli F, Karam G, et al. Posttransplant donor-specific anti-HLA antibodies negatively impact pancreas transplantation outcome. Am J Transplant (2011) 11(12):2737–46. doi: 10.1111/j.1600-6143.2011.03729.x
Keywords: HLA antigens, T follicular helper cell, transplantation immunology, solid organ transplantation, kidney transplantation, donor-specific HLA antibody, non-HLA antibody, antibody-mediated rejection
Citation: Subburayalu J (2023) Immune surveillance and humoral immune responses in kidney transplantation – A look back at T follicular helper cells. Front. Immunol. 14:1114842. doi: 10.3389/fimmu.2023.1114842
Received: 02 December 2022; Accepted: 22 June 2023;
Published: 12 July 2023.
Edited by:
Helong Dai, Central South University, ChinaReviewed by:
Yoshiko Matsuda, National Center for Child Health and Development (NCCHD), JapanGowthaman Uthaman, University of Massachusetts Medical School, United States
Copyright © 2023 Subburayalu. This is an open-access article distributed under the terms of the Creative Commons Attribution License (CC BY). The use, distribution or reproduction in other forums is permitted, provided the original author(s) and the copyright owner(s) are credited and that the original publication in this journal is cited, in accordance with accepted academic practice. No use, distribution or reproduction is permitted which does not comply with these terms.
*Correspondence: Julien Subburayalu, anVsaWVuLnN1YmJ1cmF5YWx1QHR1LWRyZXNkZW4uZGU=
†ORCID: Julien Subburayalu, orcid.org/0000-0001-9243-0558