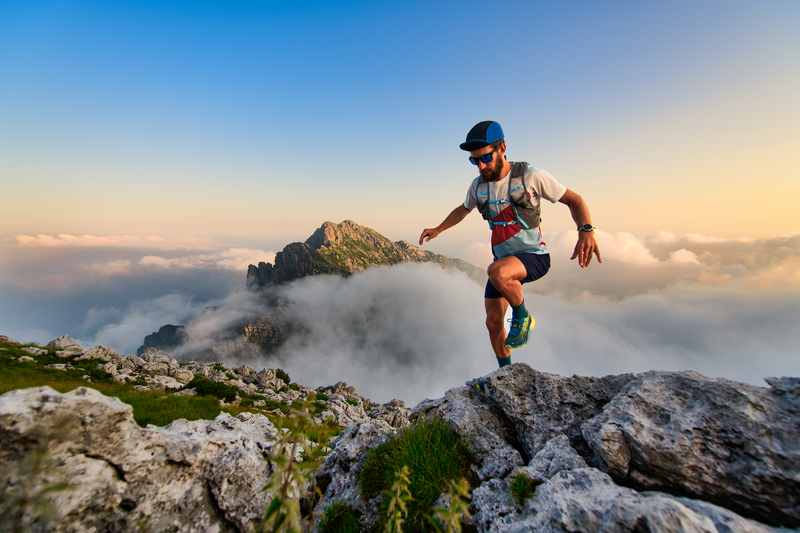
95% of researchers rate our articles as excellent or good
Learn more about the work of our research integrity team to safeguard the quality of each article we publish.
Find out more
REVIEW article
Front. Immunol. , 30 August 2022
Sec. B Cell Biology
Volume 13 - 2022 | https://doi.org/10.3389/fimmu.2022.988536
B cells secrete antibodies and mediate the humoral immune response, making them extremely important in protective immunity against SARS-CoV-2, which caused the coronavirus disease 2019 (COVID-19) pandemic. In this review, we summarize the positive function and pathological response of B cells in SARS-CoV-2 infection and re-infection. Then, we structure the immunity responses that B cells mediated in peripheral tissues. Furthermore, we discuss the role of B cells during vaccination including the effectiveness of antibodies and memory B cells, viral evolution mechanisms, and future vaccine development. This review might help medical workers and researchers to have a better understanding of the interaction between B cells and SARS-CoV-2 and broaden their vision for future investigations.
The new coronavirus, severe acute respiratory syndrome coronavirus 2 (SARS-CoV-2), have caused over 550 million infections and more than 6 million deaths till July 18, 2022. The Chinese Center for Disease Control and Prevention identified the virus as a novel coronavirus on January 7, 2020 and reported the results of pathogen identification to the World Health Organization (WHO) on January 9, 2020. Then, more importantly, the genome sequence of this novel virus was registered in the global influenza sharing database on January 12, 2020 (1). WHO declared this outbreak a public health emergency of international concern as an alarm to the countries around the world on January 23, 2020 and named the infectious coronavirus disease COVID-19 (2). Bats are the likely reservoir hosts for SARS-CoV-2, while other species are intermediate hosts that harbor the virus to allow the replication and thus the mutations required to become highly transmissible and pathogenic to humans (3, 4). At present, targeted and effective vaccines have been under production and inoculation in many countries, and facilitated the prevention and control of the pandemic.
SARS-CoV-2 belongs to the Coronaviridae family, in which 229E, HKU1, NL63, and OC43 have caused small endemic infections, as well as the unanticipated worldwide outbreaks of SAR-CoV and MERS-CoV (5, 6). The human coronaviruses that are responsible for numerous chronic or acute respiratory diseases ranging from the self-curable common cold to severe pneumonia, contain an envelope and have single-stranded positive RNA genomes (7, 8). The genome of SARS-CoV-2 encodes four structural proteins including membrane (M), envelope (E), nucleocapsid (N), and spike (S). The S protein allows the virus to infect cells and mutations on this protein help the virus escape from existing neutralizing antibodies. Additionally, nonstructural proteins (NSPs) are also necessary and important for virus infection (9, 10). The spike protein contains the receptor-binding domain (RBD) and along with the nucleocapsid, acting as effective antigens that elicit B cell-mediated antibody response (11). Studies on severe COVID-19 cases have reported a greater antibody response against the S and N protein and larger memory B cell response towards S protein, suggesting that the more severe COVID-19 infection might provide superior protection from re-infection with SARS-CoV-2 (12).
In this review, we discuss the function of B cells in SARS-CoV-2 viral infection and reinfection. From the antibodies B cells secrete to other subsets like memory B cells and regulatory B cells, from the role of antivirus response to pathological impairment, and from natural infection to vaccination.
In humans, there are two types of immune responses against infections that include the innate immune system and the adaptive immune system. B cells play a significant and irreplaceable role in the adaptive immune response, which contributes to the control, destruction, and clearance of invaders like viruses and bacteria. The role of B cells in virus infection is dynamic and far-ranging, involving cytokine production, antigen presentation, and antibody secretion.
B cells develop through the pro-B cells, pre-B cells, and immature B cells into mature B cells in the fetal liver before birth and afterwards in the bone marrow. Some mature B cell subpopulations, such as B-1, B-2, and regulatory B cells, are necessary components in antiviral B cell responses. B-1 cells derived from fetal liver could be divided into B-1a and B-1b subpopulations (13). B-2 cells originating from bone marrow contain follicular B (FO B) cells and marginal zone B (MZ B) cells. In general, MZ B cells and B-1 cells participate in T-independent responses to produce short-term immunity, while FO B cells are involved in T-dependent responses to produce long-standing protection against reinfection with the same pathogen (14). However, studies revealed that MZ B cells can not only elicit early-generated antibody responses but also play a role in germinal center (GC) formation with high similarity compared to FO-derived GCs except for a delay in T-dependent responses (15). Regulatory B cells function as immunosuppressive cells to support immunological tolerance by producing pro-inflammatory factors like interleukin-10 (IL-10) to inhibit the multiplication of T cells and other pro-inflammatory cells (16).
Humoral immune responses are the most effective and long-lasting immunological mechanisms for the clearance and prevention of reinfection and are critical for SARS-CoV-2 infected individuals. Limited humoral responses lead to ineffective clearance of SARS-CoV-2 in patients with immune deficiency, thus recidivation occurs (17).
The interaction of naïve B cells with the antigen and CD4+ T cells in GCs induces B cells to go through the process of proliferation, class-switch recombination (CSR) to affinity maturation and, eventually, differentiation into LLPCs and MBCs that produce antibodies (Abs) with high-affinity (Figure 1). After the T-B interaction, some B cells generate extrafollicular reactions in which its, rather than forming GCs, rapidly proliferate and differentiate into SLPCs that secrete low-affinity Abs and pre-GC MBCs that take part in the differentiation into plasmablasts or the initiation of secondary GC responses together with MBCs generated in GCs. T follicular helper (Tfh) cells activate and regulate the activity of B cells in the GC response (19) (Figure 1).
Figure 1 The role of B cells in COVID-19 infection and re-infection. Naïve B cells are activated via the help of folliculat T (Tfh) cells after the invasion of SARS-CoV-2 virus. Following T-B interaction, B cells go through rapid proliferation and differentiation, producing pre-germinal center memory B cells (pre-GC MBCs) and short-lived plasma cells (SLPCs) producing early antibodies with low affinity. Other B cells enter the germinal center (GC) to increase antibody affinity by clonal expansion and somatic hypermutation (SHM), and change the structure through class-switching recombination (CSR), resulting in long-lived plasma cells (LLPCs) and memory B cells (MBCs). If challenged with a second invasion or vaccination, both pre-GC MBCs and MBCs generated after GC reactions differentiate into plasma cells or generate a second GC in a short period of time to produce high-affinity antibodies. Those B cells and antibodies secreted enter the blood circulation and peripheral tissues like mucosa to defend against viral infection and re-infection. Figure adapted from (18).
After GC response, the LLPCs migrate and locate in bone marrow and could produce neutralizing Abs continuously to protect individuals from re-infection. One study has found that the SARS-CoV-2-specific LLPCs could exist 7 to 11months in bone marrow and provide effective protection in mild COVID-19 infections (20). Moreover, investigations show that mRNA vaccines could induce persistent germinal center reactions, resulting in the unremitting production of LLPCs and the neutralizing Abs (21, 22). These might be the mechanism of the long-lived immune memory in mild-infected cases. In severely infected individuals, the elevated antibody titers and MBCs response correlate with the robust extrafollicular response rather than GC response (23–25). This might be attribute to the failure in functional GC formation due to the significant decrease in the Tfh cell quantity, resulting in the defective LLPCs and MBCs generation from GC response and impaired long-term protection (26).
After activation, B cells differentiate into antibody-producing cells and secrete Abs as a powerful weapon against primary or secondary infection (27). In SARS-CoV-2 infection, data show that neutralizing antibodies are generated from naïve B cells and not from pre-existing memory B cells (28–31). The affinity of Abs improves by SHM and clonal evolution in GCs to increase the binding efficiency to pathogens (32). Abs are generated into different isotypes that have diversity in the structure of the heavy chain constant region (CH), including IgA, IgG, IgM, IgD and IgE. This transformation of isotype is achieved through CSR (33) (Figure 1). Activation-induced cytidine deaminase (AID) is critical in the genomic recombination for SHM and CSR (34) and AID-deficiency is associated with hyper-IgM syndrome in patients (35).
Antibodies of COVID-19 patients bind S proteins and inhibit entry of viruses into host cells by sterically blocking the interaction of the receptor-binding domain (RBD) with ACE2 (36–38). While RBD remains the dominating target for neutralizing antibodies, studies reveal that other epitopes on the S proteins, like the N-terminal domain (NTD), also interact with virus-neutralizing antibodies (39, 40). However, one study indicates that the N protein has little reactivity to neutralizing antibodies and that antibodies binding non-S proteins provide little protection against COVID-19 infection (31).
Generally, in virus infections, the generation of serum IgM is dominant over other antigen-specific antibodies including IgA and IgG (41). However, the serum antibody production of IgM, IgA, and IgG against SARS-CoV-2 S and N proteins occur almost simultaneously in COVID-19 patients (42, 43). Studies further illustrate that IgA contributed more in neutralizing viruses than IgG and dominated the humoral response specific to SARS-CoV-2 in the early stage of COVID-19 infection in mucosa (44).
The heterogeneity observed in patients’ disease severity, humoral immunity, B cell response and antibody titers is associated with age, gender and race (45–47). Meanwhile, the severity of the disease is also highly associated with the load of antigens and the corresponding neutralizing antibody (nAb) titers, with the sickest patients having high load of virus, inducing high nAb titers and the milder or asymptomatic individuals having low or no nAb titers because of low antigen load (48, 49). These results are also observed in SARS and MERS infection (50). This suggests that a high nAb titer does not necessarily mean better humoral protection against the virus. Moreover, the quick decline of SARS-CoV-2 specific nAb titers (within 2 months) among severe, mild and asymptomatic patients further indicates that the protection from the humoral response is not only time-limited, but it is also insufficient (51, 52). Furthermore, the inconsistency between antibody response and disease outcome shows that some individuals resolve the virus infection before antibody production, indicating that the innate immunity and early T cell response may be the factors in determining the duration and severity of primary SAR-CoV-2 infection (53).
Antibodies prevent pathogens from entering host cells and they also agglutinate free viruses and kill virus-infected cells (49). Although it remains to be shown in human COVID-19 cases, these significant mechanisms have been studied in mice (52, 54). These models have shown that antibodies with high Fc effector activity provide the most protection against SARS-CoV-2 infection (52), and that vaccination using RBD-Fc based activity was effective in fighting COVID-19 infection (54). Additionally, human patients that have died of COVID-19 produced antibodies with decreased Fc effector activity, which also resulted in deficient humoral induced immune responses (55). Altogether, these results provide a new approach in developing efficient and broad-spectrum vaccines involved in protection against SAR-CoV-2 re-infection. However, even minor changes to the Fc structure of antibodies, like IgG that contains fucosylated Fc glycans, may negatively influence Fc-related interaction. This could result in increased secretion of inflammatory cytokines and disease severity which resembles what has been observed in severely infected patients (56). Although the treatment of COVID-19 with antibody Fc appears to be a promising therapy, further studies are needed.
Apart from the function of antibody secretion, B cells also play an important role in inflammatory cytokines. The expression of several inflammatory cytokines such as TNF-α, Interleukin-2, Interleukin-6, monocyte chemoattractant proteins (MCPs), and IFN-γ-inducible protein 10 (IP-10) as well as relevant chemokines such as CXCL2 and CCL2 are elevated in COVID-19 patients, accounting for the cytokine storm observed in these patients (57–59). However, the mechanism of the cytokine storm formation and amplification remains to be unknown, and more investigations are needed to explore it in order to give proper suggestion on clinical treatment and therapy.
Memory B cells (MBCs) take an important part in deciding the occurrence and severity of COVID-19 re-infection, thus finding ways to elicit widely reactive MBCs is key in vaccine development. MBCs develop through either GC-dependent or GC-independent pathways (60) and are classified into different subsets with distinct functions and markers (61, 62). One atypical MBC is characterized by CD11c+ T-bet+, develops independently from GCs, and plays a protective role in COVID-19 infection (25, 63, 64). In general, MBCs that do not undergo GC response lack class switching and affinity maturation and are incapable of producing effective nAbs against viral variants (60, 65, 66). Additionally, CD80-PD-L2 double negative population of MBCs do not go through GC reaction and differentiate into antibody-secreting cells in a short period of time. However, they are able to re-enter GCs for activation and development into antibody secreting cells that produce nAbs with high-affinity (66, 67). Therefore, methods to promote the re-entrance of MBCs into GCs to generate protective nAbs are important for creating potential therapies against COVID-19.
During COVID-19 re-infection, MBCs circulating throughout the whole body are activated and quickly differentiate into Ab-secreting cells. However, if these circulating Abs are deficient or activated by a variant of the primary pathogen, the MBCs are induced to generate new GCs for producing higher affinity Abs (68). The antigen-specific Abs are lost in the large majority of SARS-CoV-infected patients. This may be similar to what is seen in SARs patients, where specific MBC responses are undetectable in 100% of the recovered individuals 6 years after infection (69). Overall, the protection offered by immune memory against reinfection of coronaviruses only persists for a short period of time (70).
There are limited human studies on the memory immune response in COVID-19 re-infection. The common method to evaluate B cell memory is monitoring the amount and duration of circulating antibodies in serum. One study indicates that the antigen-specific IgG titers keep steady for 3-4 months after infection, while IgA and IgG are maintained for an even shorter time (71, 72). In contrast, many other studies have shown durability in immune memory. In one cohort study, 25 patients grouped as mild, moderate, or severe were assessed for RBD or N-specific MBCs and it was found that all groups had increased MBCs from the beginning to 150 days after infection (73). Additionally, the RBD- or N-specific MBCs mainly contain IgM and IgG with various immunophenotypes, and the quantity of RBD-specific IgG MBCs also correlates with the number of Tfh cells. In the early stage of re-infection, IgM secreting MBCs represent the majority of MBCs, but they decrease after 20 days as IgG secreting MBCs steadily increase and become the dominant MBCs that are detectable between 120-240 days post-symptom onset (PSO). As for long term studies, a cohort evaluation of 188 cases found that circulating MBCs specific for RBD, N and S proteins could exist for more than 6 months and up to 8 months PSO. Additionally, a large proportion of RBD memory B cells that increased over time from 1 month to 6 months PSO secreted IgG (24). Another longitudinal study investigated the amount of MBCs in mild cases and found that IgG+ MBCs persisted and increased through 3 months after COVID-19 infection (74). RBD-specific MBCs that have undergone SHM in GCs and produce high affinity nAbs have frequencies that remain unchanged or even rise 6 months after infection (75, 76). Moreover, a recent study showed an encouraging discovery that functional IgG MBCs persist after 5-8 months in infected patients, while the level of serum SARS-CoV-2 specific IgG is eventually lost (77). This might suggest that the level of MBCs is more sensitive for detection of previous infection and prediction of long-term protection. Overall, these results show that the B cell-mediated humoral response, which produces short-term or long-term MBCs and antibodies, provides indispensable protection during reinfection. Clinical findings also support the research above, with nearly 1200 individuals showing no symptoms during COVID-19 reinfection (78). However, due to the limited re-infected populations and insufficient information, more researches are required to further investigate the time span and protective magnitude of B cell responses in reinfections.
Respiratory viruses like SARS-CoV and SARS-CoV-2 enter organisms mainly via the mucosal surfaces of the respiratory tract. Antibody and B cell responses are critical in controlling virus adherence and invasion effectively in the peripheral tissues. Collecting blood samples to assess immune responses is the most common method because it is the most direct and convenient way, but blood samples do not represent the immune cells and response in peripheral tissues, like mucosa (79). Thus, it is important to understand the relationship between B cells and antibody response not only in blood, but in tissues too.
Currently, less is known about mucosal immunity against SARS-CoV-2 compared to systemic immunity. Both IgA and IgG are produced in mucosal tissues. IgA dimers specific to SARS-CoV-2 are nearly 15 times more potent than IgA monomers, which play a major role in neutralizing viruses and preventing initial viral spread and amplification (80). One study detected the level of antibody responses in both serum and saliva and described that the IgG response was similar and lasted for several months while the titers of IgA and IgM declined rapidly (81). Further investigations revealed that there is a positive correlation of IgG and IgM responses between blood and mucosa, while the relation with IgA is less comparable (81, 82). In nasal samples collected from COVID-19 patients, severely infected individuals had higher IgG responses while those with mild or moderate symptoms showed higher IgA responses (83). These findings on mucosal immunity may not be universally applied in all cases, but they are evidences that B cells and antibody responses are playing a critical role in immune mechanisms against SARS-CoV-2 and may influence the outcome of infection.
Although existing antibodies in mucosal tissues can prevent humans from pathogen infections like COVID-19, MBCs play an important role during reinfection by responding locally to increase production of antigen-specific antibodies in a short period of time. MBCs are present in multiple human tissues, such as lung, spleen, lymph nodes and gut (84–87). MBCs in the lung enhance immune responses against viruses and provide cross-reactive antibodies through clonal selection in germinal centers (85–88). In the gut, MBCs enable continued clonal selection in mucosal to generate B cells which secrete antigen-specific antibodies with high affinity (75). MBCs in peripheral tissues, like lung, require tertiary lymphoid structures induced by inflammation to undergo reactivation and produce antibodies to clear viruses rapidly (89, 90).
The development of a vaccine that induces mucosal B cell responses to generate SARS-CoV-2 specific antibodies and relevant memory B cells are of great significance to prevent and protect against viruses. One investigation revealed that even individuals fully vaccinated with mRNA vaccines could still be infected by SARS-CoV-2 variants (91). The level of IgA and IgG in saliva is much lower than in serum, accounting for the ineffective protection in mucosal compartment induced by intramuscular vaccines (92). However, animal studies have shown that a single dose of the adenovirus-vectored SARS-CoV-2 vaccine had better protective effects in upper and lower respiratory tracts in mice and hamsters compared to other vaccines (93, 94). Further investigation confirmed that this adenovirus-vectored SARS-CoV-2 vaccine could provide general mucosal immunity against SARS-CoV-2 and the variants (95). A phase I/II clinical trial of a novel vaccine, NASVAC, was designed to measure its effectiveness in inducing mucosal immune response in COVID-19 patients via intranasal approach, declaring the safety and tolerance of this vaccine to infected patients (96). Other studies also found that an intranasal approach of vaccination and antibody therapies could be effective to protect individuals against COVID-19 infection (97, 98). Overall, the existence of memory B cells and secreted Abs in mucosal tissues provide important goals in vaccine development since the response of mucosal immunity measures the protective level in uninfected individuals and the disease outcome in infected patients (99).
The rapid spread and frightful mortality of SARS-CoV-2 sounded a global alarm and the research and development for an effective vaccine to control the epidemic became an emergency.
Several different types of vaccines have been used worldwide. At first, two inactivated vaccines including BBIBP-CorV and CoronaVac and one RBD-based protein subunit vaccine, ZF2001, were authorized for wide use (100–103). Three clinical trials in phases I and II, which included the candidate vaccine, CoronaVac, revealed effective production of neutralizing antibodies with low rates of adverse reactions in three healthy age groups of 3 to17-years old, 18 to 59-years old and more than 60 years old (100–102). Other two studies focusing on the safety and effectiveness of BBIBP-CorV revealed similar outcomes, with most individuals having a humoral response in 28 days post-vaccination with no severe adverse events (103, 104). However, one study that was randomized, double-blinded and placebo-controlled showed that ZF2001, the RBD-based protein subunit vaccine, caused more and worse side-effects compared to the other two vaccines, but still was beneficial in protection against COVID-19 (105).
Later, mRNA-based BNT162b22 (Pfizer-BioNTech), mRNA-1273 (Moderna) and viral vector-based Johnson & Johnson Janssen vaccine were authorized by the Food and Drug Administration (FDA) for emergency use. In the individuals who were not infected before, mRNA-based vaccines induced B cell responses and antibody generation effectively, enabling acquired immunity against SARS-CoV-2 infection (53, 106, 107). Half of the vaccinated individuals developed detectable nAbs with one dose and the majority generated adequate antibodies and enhanced B cell memory after a second dose (106, 107). In addition, the first dose of both Pfizer-BioNTech and Moderna vaccines induced anti-S IgG titers that declined after 6 weeks, thus further confirming the necessity of a second dose (53). These two mRNA-based vaccines also promote a protective B cell immune response in individuals that recovered from COVID-19 infection (65, 107–109). With these individuals, after the first dose, vaccination successfully induced an enhanced memory B cell response with neutralizing antibodies specific to SARS-CoV-2 (108). Also, the number of existing memory B cells all increased after the vaccination and the degree of enhancement correlated with the number of memory B cells pre-existing in the SARS-CoV-2 infected individuals (65, 107). However, the second dose did not further increase the B cell memory antibody response, suggesting that only the first dose of the mRNA-based vaccines is helpful in reaching the peak of humoral immunity in recovered SARS-CoV-2 individuals (109). While the quantity of memory B cells in recovered individuals was higher than those in uninfected individuals at 3 months post-vaccination, the number of memory B cells at 6 months and the decline rate afterward remained similar (110). Furthermore, several studies showed that mRNA-based vaccines induce cross-reactive nAbs against SARS-CoV-2 variants in not only naïve but also recovered individuals (65, 111).
One article revealed that most vaccines are safe even in cancer patients, but a customized vaccination approach is preferred (112). While the vaccines above have been proven safe in clinical investigations, they are insufficient to provide enough protection for this universal outbreak, therefore, creating new vaccines with diverse protective mechanisms is necessary. A study of one influenza virus vaccine, which can alternatively induce stalk-reactive memory B cells in comparison with conventional influenza virus vaccines, has completed the phase I trial and has proven safe and efficient in generating a more prolonged and broader B cell and antibody response (62). Targeting the conserved and immunodominant antigen of hemagglutinin, this vaccine has a novel approach to vaccine production, but whether this finding can be applied to making SARS-CoV-2 vaccines is unclear because of the less protective cross-reaction between SARS-CoV-2 and seasonal coronaviruses (31).
Coronaviruses, like SARS-CoV-2, have a decreased mutation rate compared to other smaller RNA viruses because of their 3′-5′ exoribonuclease, which has an efficient proofreading function in mismatch correction (113, 114). However, various mutations with enhanced pathogenicity, transmissibility, or escaping capability from neutralizing antibodies have been reported in new waves of epidemics around the world.
An early study following the viral genome evolution of the Wuhan-Hu-1 strain after one year found 75 non-synonymous nucleotide mutations in 3,823 samples compared to the initial viral copy (EPI_ISL_402125) from January 2021 (115). The viral variant D614G, with a mutation in the nucleotide fragment encoding the spike protein, was more infectious and rapidly replaced the former Wuhan-Hu-1 during the early stage of the COVID-19 epidemic (116). Ever since then, several changes in the amino acid of the S protein have been identified and variants with increased spread and escape from vaccination and natural immunity are emerging (117). Studies also showed that the sensitivity of neutralizing antibodies to the spike proteins has decreased against several virus variants (9, 118–121).
Consequently, vaccination for inducing effective antivirus immunity including MBCs and nAbs have become significant in protecting against infection or reinfection by SARS-CoV-2 variants. One dose of mRNA vaccine is sufficient to elicit enhanced antibody responses with higher antibody titer specific to Alpha (B.1.1.7) and Beta (B.1.351) variants in previously infected patients (107). But there needs to be two doses to induce enough nAbs against the S protein of Alpha, Beta and Delta (B.1.617) variants (107, 108, 122). The mutation E484 was especially adept at escaping neutralization by antibodies in vaccinated individuals (123, 124). Interestingly, the titer of antibodies against Beta variants was not reduced in vaccinated individuals who were previously infected by SARS-CoV-2 (65, 107). Antibodies specific to both wild-type and viral variants from those vaccinated who were previously infected showed an increase in neutralizing titer in comparison to naïve individuals vaccinated (107, 125). No significant increase was found in the quantity of MBCs against variants post vaccination in previously infected individuals (65). These results suggest that vaccinated individuals with previous COVID-19 infections have more intensive humoral responses compared to those individuals who remained uninfected. This might also be due to clonal evolution increasing the breadth of B cell responses after infection (65, 126). Also, that could be an explanation of some breakthrough infection caused by delta variant with low frequencies of the memory B cells resulted from the impaired clonal evolution, neutralizing potency and neutralizing breadth (127). Memory B cells undergo clonal evolution persistently after at least one year of infection and secreting antibodies specific to Alpha, Beta and Delta variants were important in preventing reinfection and influencing disease outcomes in severely infected patients (65, 110). Consistent with the research above, there were 10 in 15 MBC clones at 12 months generating antibodies capable of neutralizing all tested variants while only 1 of 15 clones at 1.3 months (65), which further proves the critical role of MBCs in protective immunity mechanisms in infection and reinfection. The fifth SARS-CoV-2 variant, Omicron, was reported on Nov 25, 2021 and characterized by the high resistance against the antibodies induced by infection and vaccination (128, 129). In symptomatic infection caused by the omicron variant, two doses of BNT162b2 or ChAdOx1 nCoV-19 became mostly ineffective 6 months after vaccination (130, 131). Three doses of BNT162b2 might be more protective, however, antibody neutralization evasion still exist in omicron infected disease (129). The neutralizing Abs induced by mRNA vaccination in COVID-19 patients with primary antibody deficiency (PAD) were found to have limited protective function against Omicron variant (132). Studies also indicated that this newly generated variant, especially its spike protein, could evade most therapeutic antibodies (129). Therefore, improvement in vaccination appliance and clinical treatment is of great emergency.
The humoral response is important in defending against viral infections such as COVID-19, but the damage to the host by its own B cells also needs to be considered to have a better understanding of the immune system and the mechanism in COVID-19.
Double-negative (DN) B cells are peripheral B cells which have gone through maturation but lack of IgD and CD27 expression (133). Former studies have shown the association between DN B cells and rheumatoid arthritis, systemic lupus erythematosus (SLE), HIV, and other immunopathological diseases, in which DN B cells expand, release cytokines and produce autoimmune antibodies that enhance disease progression (134–136). Recently, one study showed that DN B cell subsets are associated with the severity of COVID-19 infection (137), but it is unclear the specific role of each subset and the mechanism of DN B cells in the SAR-CoV-2 outcome.
While antibodies secreted by B cells are great weapons to fight SARS-CoV-2 and other pathogens, they could be harmful in some cases. Previous studies have shown that SARS patients who respond to viral infection early and produce detectable antibodies within two weeks have higher mortality than patients that are later responders (138). This same outcome has been observed in COVID-19 infected patients too (139). An explanation for this is that a skewed macrophage response increases a pro-inflammatory M1 phenotype, which enhances inflammation and impairs tissues in patients who generate early antibodies, especially IgGs against spike protein (140). This is known as an antibody-dependent enhancement of disease (ADE), which also occurs in SARS-CoV and MERS-CoV infections (141, 142). ADE develops when early produced antibodies promote viral entry into macrophages and other innate and adaptive cells via binding to both the spike protein of viruses and the Fc receptor on cell surfaces to form a functional complex (142). The timing of an antibody response is significant in antibody-based therapies, in which the outcome for patients is influenced by the timing of treatment application. However, there are no methods to differentiate viral infections from ADE and the risk of ADE is unpredictable, therefore the possibility of ADE-antibodies occurring in vaccinated individuals is of concern (143). Interestingly, there is no evidence of ADE during infection of COVID-19 vaccinated individuals by escape variants.
Autoimmune antibodies have been reported in SARS-CoV-2 infected individuals. However, there is no conclusion whether these antibodies are common, short-lived types from anti-viral responses or pathogenic autoantibodies that cause autoimmune diseases like rheumatic heart disease (144). A particular B cell-mediated autoimmunity that lowers serum levels of IFNs by producing neutralizing IgG against type I IFNs was found in at least 10% of patients with life-threatening COVID-19 pneumonia (145). Additionally, a group of severe COVID-19 patients also suffered from worse symptoms due to an inborn error of type I IFNs (146). These two factors of lower IFN levels in patients cause devastating disease because of insufficient natural and acquired immunity, which indicates that the preexistence of autoimmune antibodies increase the severity of COVID-19 infection. Consistent with the hypotheses, these autoantibodies neutralizing type I IFN were also founded in nearly 20% COVID-19 deaths (147, 148). Samples from more than 34,000 uninfected individuals were analyzed and showed that the level of these autoimmune antibodies increased with age, which account for the risk of severe COVID-19 infection cases associated with age (147). Furthermore, a previous investigation on a yellow fever live attenuated vaccine revealed that more than 10% of vaccinated cases had a high titer of autoantibodies against type I IFNs, which explained the adverse effects in more than half of the life-threatening vaccine-associated disease (149). The connection between autoantibodies and vaccine side effects is a cause of concern in SARS-CoV-2 vaccination, thus further research is necessary to uncover the linkage and mechanisms involved.
Numerous case reports showed that de novo autoimmune disease would develop following COVID-19 infection, such as rheumatoid arthritis, psoriatic arthritis, and predict a worse disease outcome and prognosis (150–153). The most common antibodies are screened in SARS-CoV-2 patients, in which antinuclear antibodies, antineutrophil cytoplasmic antibodies, and ASCA immunoglobulin A antibodies showed great prevalence (153). Another functional antibody, antiphospholipid autoantibodies (aPLs), which cause abnormal coagulation along with microvascular and macrovascular thrombosis, is found in severe COVID-19 infected patients as well as Antiphospholipid syndrome (154, 155). Antiphospholipid syndrome is an autoimmune thromboinflammatory disease associated with aPLs, which stimulate endothelial cells and platelets activation and neutrophil extracellular traps (NETs) release from neutrophils (156–158). More than half of hospitalized SARS-CoV-2 infected patients had the presence of aPLs in serum samples. Moreover, the higher levels of aPLs have a positive association with neutrophil activation, NETs release, platelet quantity, and COVID-19 disease severity (144). All of these antibodies might be generated by a subpopulation of extrafollicular B cells, known as DN B cells which we have discussed before (25). These studies suggest that COVID-19 infection could trigger the autoantibody production, resulting in the de novo autoimmune disease and life-threatening infection.
Viruses have developed multiple ways to escape the immune system, including SARS-CoV-2, which is likely to escape early neutralizing antibodies via antibody-driven evolution and other mechanisms that have not been discovered yet. One mutation, D614G, enhanced furin-mediated spike cleavage to increase syncytium formation and viral titer, which caused the virus to become more infectious with higher viral loads compared to the former viruses (159–161). Other recent variants, for example, B.1.351, B.1.1.7 and P1, became resistant to neutralizing antibodies by changing infected host cell reactions to escape immune clearance, which allowed for re-infection of recovered individuals and new waves of COVID-19 (121, 162). However, specific mutations that may have the greatest antigenicity and pathogenicity remain unclear. Therefore, understanding viral evolution mechanisms and preferences is extremely significant in vaccine design.
Antigen presentation is the initiation process for adaptive immune response (163). The downregulation of major histocompatibility complex (MHC) I and II molecules and pathways in antigen presenting cells, such dendritic cells and B cells, suggested that the antigen presentation was inhibited by SARS-CoV-2, leading to the inhibition of T cell-mediated immune response (164, 165). T cells not only participate in T cell immune response against SARS-CoV-2 (166), but also various B cell-associated immune responses, such the T-B interaction and cytokine production (167). Therefore, the impaired T cell activity would result in the less protective humoral and cellular response against SARS-CoV-2 as well as viral evasion from adaptive immunity.
Multiple studies have been focusing on questions about the humoral response and the function of antibody-generating B cell and memory B cell responses. Several key problems still need answers such as: 1) the duration of antibodies and memory B cells in serum and mucosa, 2) the difference between the titers of neutralizing antibody and population of memory B cells in vaccination and infection, and 3) the factors influencing the outcome of disease in infected patients. Whether and how vaccination could induce an effective B cell response in mucosa requires further investigation. Current research has revealed that vaccination provides effective protection for naïve and previously infected individuals. However, with the ongoing viral evolution, vaccines also need to be continually updated and new approaches to defend against viral invasion are also urgently required for vaccine development, such as inducing effective mucosal immunity of dimeric IgA and memory B cells specific to SARS-CoV-2. Additionally, cross-reactive antibodies induced by infection or vaccination, which protects against not only COVID-19 but also other coronaviruses infections are important areas of study.
SC wrote the article. FG, FC, KB, NOSC, AH, LJ, JL, HM, and MK revised the draft. CL and QN organized and revised the draft. All authors contributed to the article and approved the submitted version.
This work was supported by HUST Academic Frontier Youth Team (2018QYTD10).
The authors declare that the research was conducted in the absence of any commercial or financial relationships that could be construed as a potential conflict of interest.
All claims expressed in this article are solely those of the authors and do not necessarily represent those of their affiliated organizations, or those of the publisher, the editors and the reviewers. Any product that may be evaluated in this article, or claim that may be made by its manufacturer, is not guaranteed or endorsed by the publisher.
1. Hu B, Guo H, Zhou P, Shi ZL. Characteristics of SARS-CoV-2 and COVID-19. Nat Rev Microbiol (2021) 19:141–54. doi: 10.1038/s41579-020-00459-7
2. Adhikari SP, Meng S, Wu YJ, Mao YP, Ye RX, Wang QZ, et al. Epidemiology, causes, clinical manifestation and diagnosis, prevention and control of coronavirus disease (COVID-19) during the early outbreak period: A scoping review. Infect Dis Poverty (2020) 9:29. doi: 10.1186/s40249-020-00646-x
3. Zhang YZ, Holmes EC. A genomic perspective on the origin and emergence of SARS-CoV-2. Cell (2020) 181:223–27. doi: 10.1016/j.cell.2020.03.035
4. Zhou P, Yang XL, Wang XG, Hu B, Zhang L, Zhang W, et al. A pneumonia outbreak associated with a new coronavirus of probable bat origin. Nature (2020) 579:270–73. doi: 10.1038/s41586-020-2012-7
5. Coronaviridae Study Group of the International Committee on Taxonomy of, Viruses. The species severe acute respiratory syndrome-related coronavirus: Classifying 2019-nCoV and naming it SARS-CoV-2. Nat Microbiol (2020) 5:536–44. doi: 10.1038/s41564-020-0695-z
6. de Wit E, van Doremalen N, Falzarano D, Munster VJ. SARS and MERS: Recent insights into emerging coronaviruses. Nat Rev Microbiol (2016) 14:523–34. doi: 10.1038/nrmicro.2016.81
7. Masters PS. The molecular biology of coronaviruses. Adv in Virus Res (2006) 66:193–292. doi: 10.1016/S0065-3527(06)66005-3
8. Pene F, Merlat A, Vabret A, Rozenberg F, Buzyn A, Dreyfus F, et al. Coronavirus 229E-related pneumonia in immunocompromised patients. Clin Infect Dis (2003) 37:929–32. doi: 10.1086/377612
9. Weisblum Y, Schmidt F, Zhang F, DaSilva J, Poston D, Lorenzi JC, et al. Escape from neutralizing antibodies by SARS-CoV-2 spike protein variants. Elife (2020) 9:e61312. doi: 10.7554/eLife.61312
10. Rohaim MA, El Naggar RF, Clayton E, Munir M. Structural and functional insights into non-structural proteins of coronaviruses. Microb Pathog (2021) 150:104641. doi: 10.1016/j.micpath.2020.104641
11. Robbiani DF. Convergent antibody responses to SARS-CoV-2 in convalescent individuals. Nature (2020) 584:437–42. doi: 10.1038/s41586-020-2456-9
12. Guthmiller JJ. SARS-CoV-2 infection severity is linked to superior humoral immunity against the spike. Mbio (2021) 12:e02940-20. doi: 10.1128/mBio.02940-20
13. Hardy RR. B-1 b cells: Development, selection, natural autoantibody and leukemia. Curr Opin Immunol (2006) 18:547–55. doi: 10.1016/j.coi.2006.07.010
14. Martin F, Oliver AM, Kearney JF. Marginal zone and B1 b cells unite in the early response against T-independent blood-borne particulate antigens. Immunity (2001) 14:617–29. doi: 10.1016/S1074-7613(01)00129-7
15. Song H, Cerny J. Functional heterogeneity of marginal zone b cells revealed by their ability to generate both early antibody-forming cells and germinal centers with hypermutation and memory in response to a T-dependent antigen. J Exp Med (2003) 198:1923–35. doi: 10.1084/jem.20031498
16. Rosser EC, Mauri C. Regulatory b cells: origin, phenotype, and function. Immunity (2015) 42:607–12. doi: 10.1016/j.immuni.2015.04.005
17. Ye X, Xiao X, Li B, Zhu W, Li Y, Wu J, et al. Low humoral immune response and ineffective clearance of SARS-Cov-2 in a COVID-19 patient with CLL during a 69-day follow-up. Front Oncol (2020) 10:1272. doi: 10.3389/fonc.2020.01272
18. Roltgen K, Boyd SD. Antibody and B cell responses to SARS-CoV-2 infection and vaccination. Cell Host Microbe (2021) 29:1063–75. doi: 10.1016/j.chom.2021.06.00
19. Juno JA, Tan HX, Lee WS, Reynaldi A, Kelly HG, Wragg K, et al. Humoral and circulating follicular helper T cell responses in recovered patients with COVID-19. Nat Med (2020) 26:1428–34. doi: 10.1038/s41591-020-0995-0
20. Turner JS, Kim W, Kalaidina E, Goss CW, Rauseo AM, Schmitz AJ, et al. SARS-CoV-2 infection induces long-lived bone marrow plasma cells in humans. Nature (2021) 595:421–25. doi: 10.1038/s41586-021-03647-4
21. Turner JS, O'Halloran JA, Kalaidina E, Kim W, Schmitz AJ, Zhou JQ, et al. SARS-CoV-2 mRNA vaccines induce persistent human germinal centre responses. Nature (2021) 596:109–13. doi: 10.1038/s41586-021-03738-2
22. Kim W, Zhou JQ, Horvath SC, Schmitz AJ, Sturtz AJ, Lei T, et al. Germinal centre-driven maturation of b cell response to mRNA vaccination. Nature (2022) 604:141–45. doi: 10.1038/s41586-022-04527-1
23. Kuri-Cervantes L, Pampena MB, Meng W, Rosenfeld AM, Ittner CAG, Weisman AR, et al. Comprehensive mapping of immune perturbations associated with severe COVID-19. Sci Immunol (2020) 5:eabd7114. doi: 10.1126/sciimmunol.abd7114
24. Dan JM, Mateus J, Kato Y, Hastie KM, Yu ED, Faliti CE, et al. Immunological memory to SARS-CoV-2 assessed for up to 8 months after infection. Science (2021) 371:eabf4063. doi: 10.1126/science.abf4063
25. Woodruff MC, Ramonell RP, Nguyen DC, Cashman KS, Saini AS, Haddad NS, et al. Extrafollicular b cell responses correlate with neutralizing antibodies and morbidity in COVID-19. Nat Immunol (2020) 21:1506–16. doi: 10.1038/s41590-020-00814-z
26. Kaneko N, Kuo HH, Boucau J, Farmer JR, Allard-Chamard H, Mahajan VS, et al. Loss of bcl-6-Expressing T follicular helper cells and germinal centers in COVID-19. Cell (2020) 183:143–57.e13. doi: 10.1016/j.cell.2020.08.025
27. Shi W, Liao Y, Willis SN, Taubenheim N, Inouye M, Tarlinton DM, et al. Transcriptional profiling of mouse b cell terminal differentiation defines a signature for antibody-secreting plasma cells. Nat Immunol (2015) 16:663–73. doi: 10.1038/ni.3154
28. Nguyen-Contant P. S protein-reactive IgG and memory b cell production after human SARS-CoV-2 infection includes broad reactivity to the S2 subunit. Mbio (2020) 11:e01991–20. doi: 10.1128/mBio.01991-20
29. Song G, He WT, Callaghan S, Anzanello F, Huang D, Ricketts J, et al. Cross-reactive serum and memory b-cell responses to spike protein in SARS-CoV-2 and endemic coronavirus infection. Nat Commun (2021) 12:2938. doi: 10.1038/s41467-021-23074-3
30. Ng KW, Faulkner N, Cornish GH. Preexisting and de novo humoral immunity to SARS-CoV-2 in humans. Science (2020) 370:1339–43. doi: 10.1126/science.abe1107
31. Anderson EM, Goodwin EC, Verma A, Arevalo CP, Bolton MJ, Weirick ME, et al. Seasonal human coronavirus antibodies are boosted upon SARS-CoV-2 infection but not associated with protection. Cell (2021) 184:1858–64.e10. doi: 10.1016/j.cell.2021.02.010
32. Tas JMJ, Mesin L, Pasqual G, Targ S, Jacobsen JT, Mano YM, et al. Visualizing antibody affinity maturation in germinal centers. Science (2016) 351:1048–54. doi: 10.1126/science.aad3439
33. Roco JA, Mesin L, Binder SC, Nefzger C, Gonzalez-Figueroa P, Canete PF, et al. Class-switch recombination occurs infrequently in germinal centers. Immunity (2019) 51:337–50.e7. doi: 10.1016/j.immuni.2019.07.001
34. Muramatsu M. Class switch recombination and hypermutation require activation-induced cytidine deaminase (AID), a potential RNA editing enzyme. Cell (2000) 102:553–63. doi: 10.1016/S0092-8674(00)00078-7
35. Kuraoka M. Activation-induced cytidine deaminase expression and activity in the absence of germinal centers: insights into hyper-IgM syndrome. J Immunol (2009) 183:3237–48. doi: 10.4049/jimmunol.0901548
36. Hoffmann M, Kleine-Weber H, Schroeder S, Kruger N, Herrler T, Erichsen S, et al. SARS-CoV-2 cell entry depends on ACE2 and TMPRSS2 and is blocked by a clinically proven protease inhibitor. Cell (2020) 181:271–80.e8. doi: 10.1016/j.cell.2020.02.052
37. Walls AC, Park YJ, Tortorici MA, Wall A, McGuire AT, Veesler D. Structure, function, and antigenicity of the SARS-CoV-2 spike glycoprotein. Cell (2020) 181:281–92.e6. doi: 10.1016/j.cell.2020.02.058
38. Ju B, Zhang Q, Ge J, Wang R, Sun J, Ge X, et al. Human neutralizing antibodies elicited by SARS-CoV-2 infection. Nature (2020) 584:115–19. doi: 10.1038/s41586-020-2380-z
39. Chi X, Yan R, Zhang J. A neutralizing human antibody binds to the n-terminal domain of the spike protein of SARS-CoV-2. Science (2020) 369:650–5. doi: 10.1126/science.abc6952
40. Poh CM, Carissimo G, Wang B, Amrun SN, Lee CY, Chee RS, et al. Two linear epitopes on the SARS-CoV-2 spike protein that elicit neutralising antibodies in COVID-19 patients. Nat Commun (2020) 11:2806. doi: 10.1038/s41467-020-16638-2
41. Ravichandran S, Hahn M, Belaunzaran-Zamudio PF, Ramos-Castaneda J, Najera-Cancino G, Caballero-Sosa S, et al. Differential human antibody repertoires following zika infection and the implications for serodiagnostics and disease outcome. Nat Commun (2019) 10:1943. doi: 10.1038/s41467-019-09914-3
42. Long QX, Liu BZ, Deng HJ, Wu GC, Deng K, Chen YK, et al. Antibody responses to SARS-CoV-2 in patients with COVID-19. Nat Med (2020) 26:845–48. doi: 10.1038/s41591-020-0897-1
43. Rogers TF, Zhao F, Huang D, Beutler N. Isolation of potent SARS-CoV-2 neutralizing antibodies and protection from disease in a small animal model. Science (2020) 369:956–63. doi: 10.1126/science.abc7520
44. Sterlin D, Mathian A, Miyara M, Mohr A, Anna F. IgA dominates the early neutralizing antibody response to SARS-CoV-2. Sci Transl Med (2021) 13:eabd2223. doi: 10.1126/scitranslmed.abd2223
45. Rydyznski Moderbacher C, Ramirez SI, Dan JM, Grifoni A, Hastie KM, Weiskopf D, et al. Antigen-specific adaptive immunity to SARS-CoV-2 in acute COVID-19 and associations with age and disease severity. Cell (2020) 183:996–1012.e19. doi: 10.1016/j.cell.2020.09.038
46. Bunders MJ, Altfeld M. Implications of sex differences in immunity for SARS-CoV-2 pathogenesis and design of therapeutic interventions. Immunity (2020) 53:487–95. doi: 10.1016/j.immuni.2020.08.003
47. Yehia BR, Winegar A, Fogel R, Fakih M, Ottenbacher A, Jesser C, et al. Association of race with mortality among patients hospitalized with coronavirus disease 2019 (COVID-19) at 92 US hospitals. JAMA Netw Open (2020) 3:e2018039. doi: 10.1001/jamanetworkopen.2020.18039
48. Long QX, Tang XJ, Shi QL, Li Q, Deng HJ, Yuan J, et al. Clinical and immunological assessment of asymptomatic SARS-CoV-2 infections. Nat Med (2020) 26:1200–04. doi: 10.1038/s41591-020-0965-6
49. Legros V, Denolly S, Vogrig M, Boson B, Siret E, Rigaill J, et al. A longitudinal study of SARS-CoV-2-infected patients reveals a high correlation between neutralizing antibodies and COVID-19 severity. Cell Mol Immunol (2021) 18:318–27. doi: 10.1038/s41423-020-00588-2
50. Sariol A, Perlman S. Lessons for COVID-19 immunity from other coronavirus infections. Immunity (2020) 53:248–63. doi: 10.1016/j.immuni.2020.07.005
51. Seow J, Graham C, Merrick B, Acors S, Pickering S, Steel KJA, et al. Longitudinal observation and decline of neutralizing antibody responses in the three months following SARS-CoV-2 infection in humans. Nat Microbiol (2020) 5:1598–607. doi: 10.1038/s41564-020-00813-8
52. Ibarrondo FJ, Fulcher JA, Goodman-Meza D, Elliott J, Hofmann C, Hausner MA, et al. Rapid decay of anti-SARS-CoV-2 antibodies in persons with mild covid-19. N Engl J Med (2020) 383:1085–87. doi: 10.1056/NEJMc2025179
53. Röltgen K, Powell AE, Wirz OF, Stevens BA. Defining the features and duration of antibody responses to SARS-CoV-2 infection associated with disease severity and outcome. Sci Immunol (2020) 5:eabe0240. doi: 10.1126/sciimmunol.abe0240
54. Liu Z, Xu W, Xia S, Gu C, Wang X, Wang Q, et al. RBD-fc-based COVID-19 vaccine candidate induces highly potent SARS-CoV-2 neutralizing antibody response. Signal Transduct Target Ther (2020) 5:282. doi: 10.1038/s41392-020-00402-5
55. Zohar T, Loos C, Fischinger S, Atyeo C, Wang C, Slein MD, et al. Compromised humoral functional evolution tracks with SARS-CoV-2 mortality. Cell (2020) 183:1508–19.e12. doi: 10.1016/j.cell.2020.10.052
56. Chakraborty S, Gonzalez J, Edwards K, Mallajosyula V, Buzzanco AS, Sherwood R, et al. Proinflammatory IgG fc structures in patients with severe COVID-19. Nat Immunol (2021) 22:67–73. doi: 10.1038/s41590-020-00828-7
57. Coperchini F, Chiovato L, Rotondi M. Interleukin-6, CXCL10 and infiltrating macrophages in COVID-19-Related cytokine storm: Not one for all but all for one! Front Immunol (2021) 12:668507. doi: 10.3389/fimmu.2021.668507
58. Chen S, Yang L, Nilsson-Payant B, Han Y, Jaffre F, Zhu J, et al. SARS-CoV-2 infected cardiomyocytes recruit monocytes by secreting CCL2. Res Sq (2020) 16:2274–88. doi: 10.21203/rs.3.rs-94634/v1
59. Santa Cruz A, Mendes-Frias A, Oliveira AI, Dias L, Matos AR, Carvalho A, et al. Interleukin-6 is a biomarker for the development of fatal severe acute respiratory syndrome coronavirus 2 pneumonia. Front Immunol (2021) 12:613422. doi: 10.3389/fimmu.2021.613422
60. Viant C, Wirthmiller T, ElTanbouly MA, Chen ST, Cipolla M, Ramos V, et al. Germinal center-dependent and -independent memory b cells produced throughout the immune response. J Exp Med (2021) 218:e20202489. doi: 10.1084/jem.20202489
61. Laidlaw BJ, Duan L, Xu Y, Vazquez SE, Cyster JG. The transcription factor hhex cooperates with the corepressor Tle3 to promote memory b cell development. Nat Immunol (2020) 21:1082–93. doi: 10.1038/s41590-020-0713-6
62. Nachbagauer R, Feser J, Naficy A, Bernstein DI, Guptill J, Walter EB, et al. A chimeric hemagglutinin-based universal influenza virus vaccine approach induces broad and long-lasting immunity in a randomized, placebo-controlled phase I trial. Nat Med (2021) 27:106–14. doi: 10.1038/s41591-020-1118-7
63. Mathew D, Giles JR, Baxter AE, Oldridge DA, Greenplate AR, Wu JE, et al. Deep immune profiling of COVID-19 patients reveals distinct immunotypes with therapeutic implications. Science (2020) 369:e20202489. doi: 10.1126/science.abc8511
64. Winslow GM, Papillion AM, Kenderes KJ, Levack RC. CD11c+ T-bet+ memory b cells: Immune maintenance during chronic infection and inflammation? Cell Immunol (2017) 321:8–17. doi: 10.1016/j.cellimm.2017.07.006
65. Wang Z, Muecksch F, Schaefer-Babajew D, Finkin S, Viant C, Gaebler C, et al. Naturally enhanced neutralizing breadth against SARS-CoV-2 one year after infection. Nature (2021) 595:426–31. doi: 10.1038/s41586-021-03696-9
66. Zuccarino-Catania GV, Sadanand S, Weisel FJ, Tomayko MM, Meng H, Kleinstein SH, et al. CD80 and PD-L2 define functionally distinct memory b cell subsets that are independent of antibody isotype. Nat Immunol (2014) 15:631–7. doi: 10.1038/ni.2914
67. He JS, Subramaniam S, Narang V, Srinivasan K, Saunders SP, Carbajo D, et al. IgG1 memory b cells keep the memory of IgE responses. Nat Commun (2017) 8:641. doi: 10.1038/s41467-017-00723-0
68. Quast I, Tarlinton D. B cell memory: understanding COVID-19. Immunity (2021) 54:205–10. doi: 10.1016/j.immuni.2021.01.014
69. Tang F, Quan Y, Xin ZT, Wrammert J, Ma MJ, Lv H, et al. Lack of peripheral memory b cell responses in recovered patients with severe acute respiratory syndrome: a six-year follow-up study. J Immunol (2011) 186:7264–8. doi: 10.4049/jimmunol.0903490
70. Edridge AWD, Kaczorowska J, Hoste ACR, Bakker M, Klein M, Loens K, et al. Seasonal coronavirus protective immunity is short-lasting. Nat Med (2020) 26:1691–93. doi: 10.1038/s41591-020-1083-1
71. Gudbjartsson DF, Norddahl GL, Melsted P, Gunnarsdottir K, Holm H, Eythorsson E, et al. Humoral immune response to SARS-CoV-2 in iceland. N Engl J Med (2020) 383:1724–34. doi: 10.1056/NEJMoa2026116
72. Iyer AS, Jones FK, Nodoushani A. Persistence and decay of human antibody responses to the receptor binding domain of SARS-CoV-2 spike protein in COVID-19 patients. Sci Immunol (2020) 5:eabe0367. doi: 10.1126/sciimmunol.abe0367
73. Hartley GE, Edwards E, Aui PM, Varese N, Stojanovic S, McMahon J, et al. Rapid generation of durable b cell memory to SARS-CoV-2 spike and nucleocapsid proteins in COVID-19 and convalescence. Sci Immunol (2020) 5:eabf8891. doi: 10.1126/sciimmunol.abf8891
74. Rodda LB, Netland J, Shehata L, Pruner KB, Morawski PA, Thouvenel CD, et al. Functional SARS-CoV-2-Specific immune memory persists after mild COVID-19. Cell (2021) 184:169–83.e17. doi: 10.1016/j.cell.2020.11.029
75. Gaebler C, Wang Z, Lorenzi JCC, Muecksch F, Finkin S, Tokuyama M, et al. Evolution of antibody immunity to SARS-CoV-2. Nature (2021) 591:639–44. doi: 10.1038/s41586-021-03207-w
76. Sokal A, Chappert P, Barba-Spaeth G, Roeser A, Fourati S, Azzaoui I, et al. Maturation and persistence of the anti-SARS-CoV-2 memory b cell response. Cell (2021) 184:1201–13.e14. doi: 10.1016/j.cell.2021.01.050
77. Winklmeier S, Eisenhut K, Taskin D, Rubsamen H, Gerhards R, Schneider C, et al. Persistence of functional memory b cells recognizing SARS-CoV-2 variants despite loss of specific IgG. iScience (2022) 25:103659. doi: 10.1016/j.isci.2021.103659
78. Lumley SF, O'Donnell D, Stoesser NE, Matthews PC, Howarth A, Hatch SB, et al. Antibody status and incidence of SARS-CoV-2 infection in health care workers. N Engl J Med (2021) 384:533–40. doi: 10.1056/NEJMoa2034545
79. Masopust D, Soerens AG. Tissue-resident T cells and other resident leukocytes. Annu Rev Immunol (2019) 37:521–46. doi: 10.1146/annurev-immunol-042617-053214
80. Wang Z, Lorenzi JCC, Muecksch F, Finkin S, Viant C, Gaebler C, et al. Enhanced SARS-CoV-2 neutralization by dimeric IgA. Sci Transl Med (2021) 13:eabf1555. doi: 10.1126/scitranslmed.abf1555
81. Isho B, Abe KT, Zuo M, Jamal AJ, Rathod B, Wang JH, et al. Persistence of serum and saliva antibody responses to SARS-CoV-2 spike antigens in COVID-19 patients. Sci Immunol (2020) 5:eabe5511. doi: 10.1126/sciimmunol.abe5511
82. Cervia C, Nilsson J, Zurbuchen Y, Valaperti A, Schreiner J, Wolfensberger A, et al. Systemic and mucosal antibody responses specific to SARS-CoV-2 during mild versus severe COVID-19. J Allergy Clin Immunol (2021) 147:545–57.e9. doi: 10.1016/j.jaci.2020.10.040
83. Butler SE, Crowley AR, Natarajan H, Xu S, Weiner JA, Bobak CA, et al. Distinct features and functions of systemic and mucosal humoral immunity among SARS-CoV-2 convalescent individuals. Front Immunol (2020) 11:618685. doi: 10.3389/fimmu.2020.618685
84. Weisel NM, Weisel FJ, Farber DL, Borghesi LA, Shen Y, Ma W, et al. Comprehensive analyses of b-cell compartments across the human body reveal novel subsets and a gut-resident memory phenotype. Blood (2020) 136:2774–85. doi: 10.1182/blood.2019002782
85. Allie SR, Bradley JE, Mudunuru U, Schultz MD, Graf BA, Lund FE, et al. The establishment of resident memory b cells in the lung requires local antigen encounter. Nat Immunol (2019) 20:97–108. doi: 10.1038/s41590-018-0260-6
86. Sathaliyawala T, Kubota M, Yudanin N, Turner D, Camp P, Thome JJ, et al. Distribution and compartmentalization of human circulating and tissue-resident memory T cell subsets. Immunity (2013) 38:187–97. doi: 10.1016/j.immuni.2012.09.020
87. Poon MML, Rybkina K, Kato Y, Kubota M, Matsumoto R, Bloom NI, et al. SARS-CoV-2 infection generates tissue-localized immunological memory in humans. Sci Immunol (2021) 6:eabl9105. doi: 10.1126/sciimmunol.abl9105
88. Adachi Y, Onodera T, Yamada Y, Daio R, Tsuiji M, Inoue T, et al. Distinct germinal center selection at local sites shapes memory b cell response to viral escape. J Exp Med (2015) 212:1709–23. doi: 10.1084/jem.20142284
89. Onodera T, Takahashi Y, Yokoi Y, Ato M, Kodama Y, Hachimura S, et al. Memory b cells in the lung participate in protective humoral immune responses to pulmonary influenza virus reinfection. Proc Natl Acad Sci U.S.A. (2012) 109:2485–90. doi: 10.1073/pnas.1115369109
90. GeurtsvanKessel CH, Willart MA, Bergen IM, van Rijt LS, Muskens F, Elewaut D, et al. Dendritic cells are crucial for maintenance of tertiary lymphoid structures in the lung of influenza virus-infected mice. J Exp Med (2009) 206:2339–49. doi: 10.1084/jem.20090410
91. Brown CM, Vostok J, Johnson H, Burns M, Gharpure R, Sami S, et al. Outbreak of SARS-CoV-2 infections, including COVID-19 vaccine breakthrough infections, associated with Large public gatherings - barnstable county, Massachusetts, July 2021. MMWR Morb Mortal Wkly Rep (2021) 70:1059–62. doi: 10.15585/mmwr.mm7031e2
92. Azzi L, Dalla Gasperina D, Veronesi G, Shallak M, Ietto G, Iovino D, et al. Mucosal immune response in BNT162b2 COVID-19 vaccine recipients. Ebiomedicine (2022) 75:103788. doi: 10.1016/j.ebiom.2021.103788
93. Bricker TL, Darling TL, Hassan AO, Harastani HH, Soung A, Jiang X, et al. A single intranasal or intramuscular immunization with chimpanzee adenovirus-vectored SARS-CoV-2 vaccine protects against pneumonia in hamsters. Cell Rep (2021) 36:109400. doi: 10.1016/j.celrep.2021.109400
94. Hassan AO, Kafai NM, Dmitriev IP, Fox JM, Smith BK, Harvey IB, et al. A single-dose intranasal ChAd vaccine protects upper and lower respiratory tracts against SARS-CoV-2. Cell (2020) 183:169–84.e13. doi: 10.1016/j.cell.2020.08.026
95. Afkhami S, D'Agostino MR, Zhang A, Stacey HD, Marzok A, Kang A, et al. Respiratory mucosal delivery of next-generation COVID-19 vaccine provides robust protection against both ancestral and variant strains of SARS-CoV-2. Cell (2022) 185:896–915.e19. doi: 10.1016/j.cell.2022.02.005
96. Fleites YA, Aguiar J, Cinza Z, Bequet M, Marrero E, Vizcaino M, et al. HeberNasvac, a therapeutic vaccine for chronic hepatitis b, stimulates local and systemic markers of innate immunity: Potential use in SARS-CoV-2 postexposure prophylaxis. Euroasian J Hepatogastroenterol (2021) 11:59–70. doi: 10.5005/jp-journals-10018-1344
97. van Doremalen N, Purushotham JN, Schulz JE, Holbrook MG, Bushmaker T, Carmody A, et al. Intranasal ChAdOx1 nCoV-19/AZD1222 vaccination reduces viral shedding after SARS-CoV-2 D614G challenge in preclinical models. Sci Transl Med (2021) 13:eabh0755. doi: 10.1126/scitranslmed.abh0755
98. Ku Z, Xie X, Hinton PR, Liu X, Ye X, Muruato AE, et al. Nasal delivery of an IgM offers broad protection from SARS-CoV-2 variants. Nature (2021) 595:718–23. doi: 10.1038/s41586-021-03673-2
99. Lavelle EC, Ward RW. Mucosal vaccines - fortifying the frontiers. Nat Rev Immunol (2021) 22:263–50. doi: 10.1038/s41577-021-00583-2
100. Wu Z, Hu Y, Xu M, Chen Z, Yang W, Jiang Z, et al. Safety, tolerability, and immunogenicity of an inactivated SARS-CoV-2 vaccine (CoronaVac) in healthy adults aged 60 years and older: a randomised, double-blind, placebo-controlled, phase 1/2 clinical trial. Lancet Infect Dis (2021) 21:803–12. doi: 10.1016/S1473-3099(20)30987-7
101. Zhang Y, Zeng G, Pan H, Li C, Hu Y, Chu K, et al. Safety, tolerability, and immunogenicity of an inactivated SARS-CoV-2 vaccine in healthy adults aged 18-59 years: a randomised, double-blind, placebo-controlled, phase 1/2 clinical trial. Lancet Infect Dis (2021) 21:181–92. doi: 10.1016/S1473-3099(20)30843-4
102. Xia S, Zhang Y, Wang Y, Wang H, Yang Y, Gao GF, et al. Safety and immunogenicity of an inactivated SARS-CoV-2 vaccine, BBIBP-CorV: a randomised, double-blind, placebo-controlled, phase 1/2 trial. Lancet Infect Dis (2021) 21:39–51. doi: 10.1016/S1473-3099(20)30831-8
103. Han B, Song Y, Li C, Yang W, Ma Q, Jiang Z, et al. Safety, tolerability, and immunogenicity of an inactivated SARS-CoV-2 vaccine (CoronaVac) in healthy children and adolescents: a double-blind, randomised, controlled, phase 1/2 clinical trial. Lancet Infect Dis (2021) 21:1645–53. doi: 10.1016/S1473-3099(21)00319-4
104. Xia S, Zhang Y, Wang Y, Wang H, Yang Y, Gao GF, et al. Safety and immunogenicity of an inactivated COVID-19 vaccine, BBIBP-CorV, in people younger than 18 years: a randomised, double-blind, controlled, phase 1/2 trial. Lancet Infect Dis (2022) 22:196–208. doi: 10.1016/S1473-3099(21)00462-X
105. Yang S, Li Y, Dai L, Wang J, He P, Li C, et al. Safety and immunogenicity of a recombinant tandem-repeat dimeric RBD-based protein subunit vaccine (ZF2001) against COVID-19 in adults: two randomised, double-blind, placebo-controlled, phase 1 and 2 trials. Lancet Infect Dis (2021) 21:1107–19. doi: 10.1016/S1473-3099(21)00127-4
106. Wang Z, Schmidt F, Weisblum Y, Muecksch F, Barnes CO, Finkin S, et al. mRNA vaccine-elicited antibodies to SARS-CoV-2 and circulating variants. Nature (2021) 592:616–22. doi: 10.1038/s41586-021-03324-6
107. Goel RR, Apostolidis SA, Painter MM, Mathew D, Pattekar A, Kuthuru O, et al. Distinct antibody and memory b cell responses in SARS-CoV-2 naive and recovered individuals following mRNA vaccination. Sci Immunol (2021) 6. doi: 10.1101/2021.03.03.21252872
108. Reynolds CJ, Pade C, Gibbons JM, Butler DK, Otter AD, Menacho K, et al. Prior SARS-CoV-2 infection rescues b and T cell responses to variants after first vaccine dose. Science (2021) 37:1418–23. doi: 10.1126/science.abh1282
109. Marc GP, Alvarez-Paggi D, Polack FP. Mounting evidence for immunizing previously infected subjects with a single dose of SARS-CoV-2 vaccine. J Clin Invest (2021) 131:e150135. doi: 10.1172/JCI150135
110. Goel RR, Painter MM, Apostolidis SA, Mathew D, Meng W, Rosenfeld AM, et al. mRNA vaccines induce durable immune memory to SARS-CoV-2 and variants of concern. Science (2021) 374:abm0829. doi: 10.1126/science.abm0829
111. Stamatatos L, Czartoski J, Wan YH, Homad LJ, Rubin V, Glantz H, et al. mRNA vaccination boosts cross-variant neutralizing antibodies elicited by SARS-CoV-2 infection. Science (2021) 372:1413–8. doi: 10.1101/2021.02.05.21251182
112. Corti C, Antonarelli G, Scotte F, Spano JP, Barriere J, Michot JM, et al. Seroconversion rate after vaccination against COVID-19 in cancer patients-a systematic review. Ann Oncol (2021) 33:158–68. doi: 10.1016/j.annonc.2021.10.014
113. Smith EC, Blanc H, Surdel MC, Vignuzzi M, Denison MR. Coronaviruses lacking exoribonuclease activity are susceptible to lethal mutagenesis: Evidence for proofreading and potential therapeutics. PloS Pathog (2013) 9:e1003565. doi: 10.1371/journal.ppat.1003565
114. Ferron F, Subissi L, Silveira De Morais AT, Le NTT, Sevajol M, Gluais L, et al. Structural and molecular basis of mismatch correction and ribavirin excision from coronavirus RNA. Proc Natl Acad Sci U.S.A. (2018) 115:E162–E71. doi: 10.1073/pnas.1718806115
115. Wu A, Wang L, Zhou HY, Ji CY, Xia SZ, Cao Y, et al. One year of SARS-CoV-2 evolution. Cell Host Microbe (2021) 29:503–07. doi: 10.1016/j.chom.2021.02.017
116. Yurkovetskiy L, Wang X, Pascal KE, Tomkins-Tinch C, Nyalile TP, Wang Y, et al. Structural and functional analysis of the D614G SARS-CoV-2 spike protein variant. Cell (2020) 183:739–51.e8. doi: 10.1016/j.cell.2020.09.032
117. Plante JA, Mitchell BM, Plante KS, Debbink K, Weaver SC, Menachery VD. The variant gambit: COVID-19's next move. Cell Host Microbe (2021) 29:508–15. doi: 10.1016/j.chom.2021.02.020
118. Andreano E, Piccini G, Licastro D, Casalino L, Johnson NV, Paciello I, et al. SARS-CoV-2 escape in vitro from a highly neutralizing COVID-19 convalescent plasma. bioRxiv (2020) 118. doi: 10.1101/2020.12.28.424451
119. Cele S, Gazy I, Jackson L, Hwa SH, Tegally H, Lustig G, et al. Escape of SARS-CoV-2 501Y.V2 from neutralization by convalescent plasma. Nature (2021) 593:142–46. doi: 10.1038/s41586-021-03471-w
120. Ho D, Wang P, Liu L, Iketani S, Luo Y, Guo Y, et al. Increased resistance of SARS-CoV-2 variants B.1.351 and B.1.1.7 to antibody neutralization. Res Sq (2021). doi: 10.21203/rs.3.rs-155394/v1
121. Wang P, Nair MS, Liu L, Iketani S, Luo Y, Guo Y, et al. Antibody resistance of SARS-CoV-2 variants B.1.351 and B.1.1.7. Nature (2021) 593:130–35. doi: 10.1038/s41586-021-03398-2
122. Planas D, Veyer D, Baidaliuk A, Staropoli I, Guivel-Benhassine F, Rajah MM, et al. Reduced sensitivity of SARS-CoV-2 variant delta to antibody neutralization. Nature (2021) 596:276–80. doi: 10.1038/s41586-021-03777-9
123. Garcia-Beltran WF, Lam EC, St Denis K, Nitido AD, Garcia ZH, Hauser BM, et al. Multiple SARS-CoV-2 variants escape neutralization by vaccine-induced humoral immunity. Cell (2021) 184:2372–83.e9. doi: 10.1016/j.cell.2021.03.013
124. Jangra S, Ye C, Rathnasinghe R, Stadlbauer D, Krammer F, Simon V, et al. The E484K mutation in the SARS-CoV-2 spike protein reduces but does not abolish neutralizing activity of human convalescent and post-vaccination sera. medRxiv (2021). doi: 10.1101/2021.01.26.21250543
125. Lucas C, Vogels CBF, Yildirim I, Rothman JE, Lu P, Monteiro V, et al. Impact of circulating SARS-CoV-2 variants on mRNA vaccine-induced immunity. Nature (2021) 600:523–29. doi: 10.1038/s41586-021-04085-y
126. Cho A, Muecksch F, Schaefer-Babajew D, Wang Z, Finkin S, Gaebler C, et al. Anti-SARS-CoV-2 receptor-binding domain antibody evolution after mRNA vaccination. Nature (2021) 600:517–22. doi: 10.1038/s41586-021-04060-7
127. Tay MZ, Rouers A, Fong SW, Goh YS, Chan YH, Chang ZW, et al. Decreased memory b cell frequencies in COVID-19 delta variant vaccine breakthrough infection. EMBO Mol Med (2022) 14:e15227. doi: 10.15252/emmm.202115227
128. Karim SSA, Karim QA. Omicron SARS-CoV-2 variant: a new chapter in the COVID-19 pandemic. Lancet (2021) 398:2126–28. doi: 10.1016/S0140-6736(21)02758-6
129. Hoffmann M, Kruger N, Schulz S, Cossmann A, Rocha C, Kempf A, et al. The omicron variant is highly resistant against antibody-mediated neutralization: Implications for control of the COVID-19 pandemic. Cell (2022) 185:447–56.e11. doi: 10.1016/j.cell.2021.12.032
130. Andrews N, Stowe J, Kirsebom F, Toffa S, Rickeard T, Gallagher E, et al. Covid-19 vaccine effectiveness against the omicron (B.1.1.529) variant. N Engl J Med (2022) 386:1532–46. doi: 10.1056/NEJMoa2119451
131. Burki TK. Omicron variant and booster COVID-19 vaccines. Lancet Respir Med (2022) 10:e17. doi: 10.1016/S2213-2600(21)00559-2
132. Zimmerman O, Altman Doss AM, Kaplonek P, Liang CY, VanBlargan LA, Chen RE, et al. mRNA vaccine boosting enhances antibody responses against SARS-CoV-2 omicron variant in individuals with antibody deficiency syndromes. Cell Rep Med (2022) 3:100653. doi: 10.1016/j.xcrm.2022.100653
133. Matthias P, Rolink AG. Transcriptional networks in developing and mature b cells. Nat Rev Immunol (2005) 5:497–508. doi: 10.1038/nri1633
134. Bernard NJ. Double-negative b cells. Nat Rev Rheumatol (2018) 14:684. doi: 10.1038/s41584-018-0113-6
135. Jenks SA, Cashman KS, Zumaquero E, Marigorta UM, Patel AV, Wang X, et al. Distinct effector b cells induced by unregulated toll-like receptor 7 contribute to pathogenic responses in systemic lupus erythematosus. Immunity (2020) 52:203. doi: 10.1016/j.immuni.2019.12.005
136. Mahmood Z, Muhammad K, Schmalzing M, Roll P, Dorner T, Tony HP. CD27-IgD- memory b cells are modulated by in vivo interleukin-6 receptor (IL-6R) blockade in rheumatoid arthritis. Arthritis Res Ther (2015) 17:61. doi: 10.1186/s13075-015-0580-y
137. Cervantes-Diaz R, Sosa-Hernandez VA, Torres-Ruiz J, Romero-Ramirez S, Canez-Hernandez M, Perez-Fragoso A, et al. Severity of SARS-CoV-2 infection is linked to double-negative (CD27(-) IgD(-)) b cell subset numbers. Inflammation Res (2022) 71:131–40. doi: 10.1007/s00011-021-01525-3
138. Ho MS, Chen WJ, Chen HY, Lin SF, Wang MC, Di J, et al. Neutralizing antibody response and SARS severity. Emerg Infect Dis (2005) 11:1730–7. doi: 10.3201/eid1111.040659
139. Arvin AM, Fink K, Schmid MA, Cathcart A, Spreafico R, Havenar-Daughton C, et al. A perspective on potential antibody-dependent enhancement of SARS-CoV-2. Nature (2020) 584:353–63. doi: 10.1038/s41586-020-2538-8
140. Liu L, Wei Q, Lin Q, Fang J, Wang H, Kwok H, et al. Anti-spike IgG causes severe acute lung injury by skewing macrophage responses during acute SARS-CoV infection. JCI Insight (2019) 4:e123158. doi: 10.1172/jci.insight.123158
141. Jaume M, Yip MS, Cheung CY, Leung HL, Li PH, Kien F, et al. Anti-severe acute respiratory syndrome coronavirus spike antibodies trigger infection of human immune cells via a pH- and cysteine protease-independent FcgammaR pathway. J Virol (2011) 85:10582–97. doi: 10.1128/JVI.00671-11
142. Wan Y, Shang J, Sun S, Tai W, Chen J, Geng Q, et al. Molecular mechanism for antibody-dependent enhancement of coronavirus entry. J Virol (2020) 94:e02015-19. doi: 10.1128/JVI.02015-19
143. Tseng CT, Sbrana E, Iwata-Yoshikawa N, Newman PC, Garron T, Atmar RL, et al. Immunization with SARS coronavirus vaccines leads to pulmonary immunopathology on challenge with the SARS virus. PloS One (2012) 7:e35421. doi: 10.1371/journal.pone.0035421
144. Zuo Y, Estes SK, Ali RA, Gandhi AA, Yalavarthi S, Shi H, et al. Prothrombotic autoantibodies in serum from patients hospitalized with COVID-19. Sci Transl Med (2020) 12:eabd3876. doi: 10.1126/scitranslmed.abd3876
145. Bastard P, Rosen LB, Zhang Q, Michailidis E, Hoffmann HH, Zhang Y, et al. Autoantibodies against type I IFNs in patients with life-threatening COVID-19. Science (2020) 370:eabd4585. doi: 10.1126/science.abd4585
146. Zhang Q, Bastard P, Liu Z, Le Pen J, Moncada-Velez M, Chen J, et al. Inborn errors of type I IFN immunity in patients with life-threatening COVID-19. Science (2020) 370:eabd4570. doi: 10.1126/science.abd4570
147. Bastard P, Gervais A, Le Voyer T, Rosain J, Philippot Q, Manry J, et al. Autoantibodies neutralizing type I IFNs are present in ~4% of uninfected individuals over 70 years old and account for ~20% of COVID-19 deaths. Sci Immunol (2021) 6:eabl4340. doi: 10.1126/sciimmunol.abl4340
148. Chauvineau-Grenier A, Bastard P, Servajean A, Gervais A, Rosain J, Jouanguy E, et al. Autoantibodies neutralizing type I interferons in 20% of COVID-19 deaths in a French hospital. Res Sq (2021) 42:459–70. doi: 10.1007/s10875-021-01203-3
149. Bastard P, Michailidis E, Hoffmann HH, Chbihi M, Le Voyer T, Rosain J, et al. Auto-antibodies to type I IFNs can underlie adverse reactions to yellow fever live attenuated vaccine. J Exp Med (2021) 218:e20202486. doi: 10.1084/jem.20202486
150. Liew IY, Mak TM, Cui L, Vasoo S, Lim XR. A case of reactive arthritis secondary to coronavirus disease 2019 infection. J Clin Rheumatol (2020) 26:233. doi: 10.1097/RHU.0000000000001560
151. De Stefano L, Rossi S, Montecucco C, Bugatti S. Transient monoarthritis and psoriatic skin lesions following COVID-19. Ann Rheum Dis (2020). doi: 10.1136/annrheumdis-2020-218520
152. Talarico R, Stagnaro C, Ferro F, Carli L, Mosca M. Symmetric peripheral polyarthritis developed during SARS-CoV-2 infection. Lancet Rheumatol (2020) 2:e518–e19. doi: 10.1016/S2665-9913(20)30216-2
153. Sacchi MC, Tamiazzo S, Stobbione P, Agatea L, De Gaspari P, Stecca A, et al. SARS-CoV-2 infection as a trigger of autoimmune response. Clin Transl Sci (2021) 14:898–907. doi: 10.1111/cts.12953
154. Colling ME, Kanthi Y. COVID-19-associated coagulopathy: An exploration of mechanisms. Vasc Med (2020) 25:471–78. doi: 10.1177/1358863X20932640
155. Wu C, Chen X, Cai Y, Xia J, Zhou X, Xu S, et al. Risk factors associated with acute respiratory distress syndrome and death in patients with coronavirus disease 2019 pneumonia in wuhan, china. JAMA Intern Med (2020) 180:934–43. doi: 10.1001/jamainternmed.2020.0994
156. Yalavarthi S, Gould TJ, Rao AN, Mazza LF, Morris AE, Nunez-Alvarez C, et al. Release of neutrophil extracellular traps by neutrophils stimulated with antiphospholipid antibodies: a newly identified mechanism of thrombosis in the antiphospholipid syndrome. Arthritis Rheumatol (2015) 67:2990–3003. doi: 10.1002/art.39247
157. Meng H, Yalavarthi S, Kanthi Y, Mazza LF, Elfline MA, Luke CE, et al. In vivo role of neutrophil extracellular traps in antiphospholipid antibody-mediated venous thrombosis. Arthritis Rheumatol (2017) 69:655–67. doi: 10.1002/art.39938
158. Thalin C, Hisada Y, Lundstrom S, Mackman N, Wallen H. Neutrophil extracellular traps: Villains and targets in arterial, venous, and cancer-associated thrombosis. Arterioscler Thromb Vasc Biol (2019) 39:1724-38. doi: 10.1161/ATVBAHA.119.312463
159. Korber B, Fischer WM, Gnanakaran S, Yoon H, Theiler J, Abfalterer W, et al. Tracking changes in SARS-CoV-2 spike: Evidence that D614G increases infectivity of the COVID-19 virus. Cell (2020) 182:812–27.e19. doi: 10.1016/j.cell.2020.06.043
160. Volz E, Hill V, McCrone JT, Price A, Jorgensen D, O'Toole A, et al. Evaluating the effects of SARS-CoV-2 spike mutation D614G on transmissibility and pathogenicity. Cell (2021) 184:64–75.e11. doi: 10.1016/j.cell.2020.11.020
161. Cheng YW, Chao TL, Li CL, Wang SH, Kao HC, Tsai YM, et al. D614G substitution of SARS-CoV-2 spike protein increases syncytium formation and virus titer via enhanced furin-mediated spike cleavage. Mbio (2021) 12:e0058721. doi: 10.1128/mBio.00587-21
162. Hoffmann M, Arora P, Gross R, Seidel A, Hornich BF, Hahn AS, et al. SARS-CoV-2 variants B.1.351 and P.1 escape from neutralizing antibodies. Cell (2021) 184:2384–93.e12. doi: 10.1016/j.cell.2021.03.036
163. Paces J, Strizova Z, Smrz D, Cerny J. COVID-19 and the immune system. Physiol Res (2020) 69:379–88. doi: 10.33549/physiolres.934492
164. Saichi M, Ladjemi MZ, Korniotis S, Rousseau C, Ait Hamou Z, Massenet-Regad L, et al. Single-cell RNA sequencing of blood antigen-presenting cells in severe COVID-19 reveals multi-process defects in antiviral immunity. Nat Cell Biol (2021) 23:538–51. doi: 10.1038/s41556-021-00681-2
165. Yoo JS, Sasaki M, Cho SX, Kasuga Y, Zhu B, Ouda R, et al. SARS-CoV-2 inhibits induction of the MHC class I pathway by targeting the STAT1-IRF1-NLRC5 axis. Nat Commun (2021) 12:6602. doi: 10.1038/s41467-021-26910-8
166. Moss P. The T cell immune response against SARS-CoV-2. Nat Immunol (2022) 23:186–93. doi: 10.1038/s41590-021-01122-w
Keywords: B cells, antibody, COVID-19, SARS-CoV-2, memory B cells, vaccination
Citation: Chen S, Guan F, Candotti F, Benlagha K, Camara NOS, Herrada AA, James LK, Lei J, Miller H, Kubo M, Ning Q and Liu C (2022) The role of B cells in COVID-19 infection and vaccination. Front. Immunol. 13:988536. doi: 10.3389/fimmu.2022.988536
Received: 07 July 2022; Accepted: 26 July 2022;
Published: 30 August 2022.
Edited by:
Liwei Lu, The University of Hong Kong, Hong Kong SAR, ChinaReviewed by:
Kun Li, Cleveland Clinic, United StatesCopyright © 2022 Chen, Guan, Candotti, Benlagha, Camara, Herrada, James, Lei, Miller, Kubo, Ning and Liu. This is an open-access article distributed under the terms of the Creative Commons Attribution License (CC BY). The use, distribution or reproduction in other forums is permitted, provided the original author(s) and the copyright owner(s) are credited and that the original publication in this journal is cited, in accordance with accepted academic practice. No use, distribution or reproduction is permitted which does not comply with these terms.
*Correspondence: Chaohong Liu, Y2hhb2hvbmdsaXU4MEAxMjYuY29t; Qin Ning, cW5pbmdAdmlwLnNpbmEuY29t
Disclaimer: All claims expressed in this article are solely those of the authors and do not necessarily represent those of their affiliated organizations, or those of the publisher, the editors and the reviewers. Any product that may be evaluated in this article or claim that may be made by its manufacturer is not guaranteed or endorsed by the publisher.
Research integrity at Frontiers
Learn more about the work of our research integrity team to safeguard the quality of each article we publish.