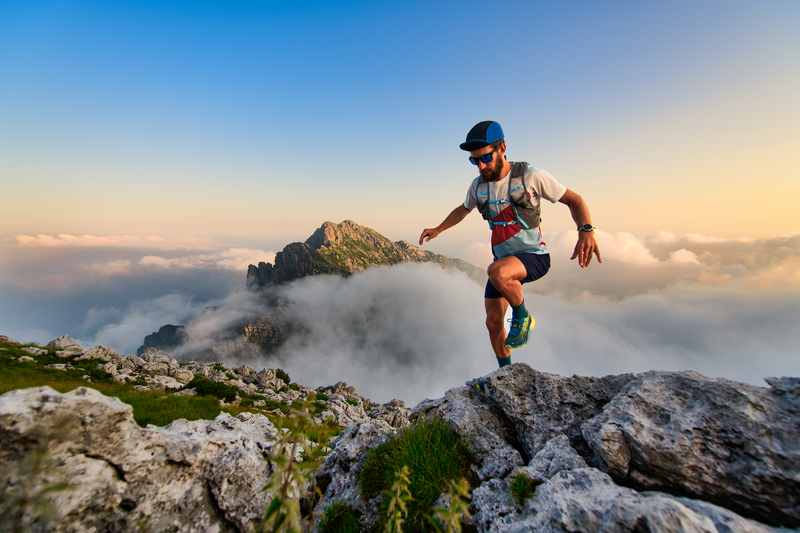
95% of researchers rate our articles as excellent or good
Learn more about the work of our research integrity team to safeguard the quality of each article we publish.
Find out more
REVIEW article
Front. Immunol. , 17 January 2023
Sec. Cancer Immunity and Immunotherapy
Volume 13 - 2022 | https://doi.org/10.3389/fimmu.2022.1093607
This article is part of the Research Topic Delivering Nucleic Acids to Immune and Non-Immune Cells View all 16 articles
Exosomes (Exos) as drug delivery vehicles have been widely used for cancer immunotherapy owing to their good biocompatibility, low toxicity, and low immunogenicity. Some Exos-based cancer immunotherapy strategies such as tuning of immunosuppressive tumor microenvironment, immune checkpoint blockades, and cancer vaccines have also been investigated in recent years, which all showed excellent therapeutic effects for malignant tumor. Furthermore, some Exos-based drug delivery systems (DDSs) for cancer immunotherapy have also undergone clinic trails, indicating that Exos are a promising drug delivery carrier. In this review, in order to promote the development of Exos-based DDSs in cancer immunotherapy, the biogenesis and composition of Exos, and Exos as drug delivery vehicles for cancer immunotherapy are summarized. Meanwhile, their clinical translation and challenges are also discussed. We hope this review will provide a good guidance for Exos as drug delivery vehicles for cancer immunotherapy.
Cancer has become a major cause of death worldwide. According to the latest statistiscs, there will be a total of more than 1.9 million new cancer patients and 600 thousand cancer deaths in the United States in 2022, suggesting that cancer has seriously threatened human health (1, 2). Although traditional therapeutics, including radiation, chemotherapy and surgery, have shown a certain tumoricidal ability, there are still some limitations (3, 4). These therapeutics often kill both cancer and normal cells, leading to severe side effects and drugs resistance (5). Therefore, it is critical to find an effective therapeutic approach with low or no side effects (6).
Cancer immunotherapy is a novel therapeutic approach that exploits the body’s own immune system to recognize and eradicate tumor cells (3, 7). In order to achieve sustained antitumor immune response, the cancer immunity cycle must be repeatedly initiated and expanded (3), as shown in Figure 1. Firstly, tumor cells release some tumor-specific immunogenic antigens, and then, antigen-presenting cells (APCs) including dendritic cells (DCs) and macrophages present antigens for the activation of certain lymphocytes via major histocompatibility complex I (MHC-I). After that, these antigens can be further recognized by T cells including CD4+ T cells and CD8+ T cells inside the lymph nodes, and thus resulting in specific immune responses to the cancer cells. In this case, cancer immunotherapy can specifically kill cancer cells with minimal effect to normal cells, and induce immunological memory to trigger long-term protection against tumor recurrence (5, 8). Therefore, cancer immunotherapy has attracted widespread attentions in the field of cancer therapy.
Figure 1 Immune actions in the cancer immunity cycle. APCs present antigens which are released by tumor cells to lymphocytes. And then, these antigens can be subsequently recognized by T cells located in the lymph nodes, thereby activating specific immune responses to the tumor sites.
Nowadays, a series of cancer immunotherapy approaches including nonspecific immune stimulation (9), immune checkpoint blockades (ICB) (10), and cancer vaccines (11, 12) have been evaluated to modulate immune responses. Moreover, some cancer immunotherapy drugs including cytotoxic T-lymphocyte-associated protein 4 (CTLA-4) inhibitors (10), programmed cell death 1 (PD-1) inhibitors and programmed cell death 1 ligand 1 (PD-L1) inhibitors have been authorized by the United States Food and Drug Administration (FDA) for clinical use (13, 14). Although these inhibitors have shown exciting outcomes, some shortcomings still exist. For instance, many malignant tumors have the ability of releasing different immunosuppressive molecules into the tumor microenvironment (TME), promoting their immune escape or suppressing immune reactions (15). Furthermore, their therapeutic effect is often diminished by off-targeting delivery, the induction of immune tolerance and evasion, and all these limit their applications (13, 16). In order to overcome these shortcomings, many researchers focus on the application of drug delivery systems (DDSs). DDSs can deliver payloads including immune checkpoint inhibitors (ICIs) and immunosuppressive regulatory molecules to the desired site and realize the sustained release of the drugs, thereby improving the efficiency of cancer immunotherapy. Currently, various DDSs, such as exosomes (Exos), liposomes, and nanoparticles, have been extensively studied and hold great promise in cancer immunotherapy.
Exos, one of drug delivery carriers, are 40–160 nm sized extracellular vesicles secreted by live cells and can be found in different types of biological fluids (e.g., serum, saliva, and urine) (17). They possess many advantages such as small size, good biocompatibility, low toxicity, and low immunogenicity (18). Meanwhile, Exos can protect cancer immunotherapeutic agents from degradation, thus increasing their circulation time and targeting ability (19). Unlike liposomes and other synthetic drug nanoparticle carriers, Exos are able to inherit the properties of parent cells and obtain some components of parent cells such as proteins, lipids and nucleic acids, which may endow them homing effect and the ability to activate immune responses (20). Moreover, Exos contain transmembrane and membrane anchored proteins, which may enhance target cells’ endocytosis and promote the delivery of their internal content (16). In addition, Exos could be easily engineered to improve drug-loading capacity and tissue-specific targeting (21). Therefore, Exos are recognized as a promising drug carrier.
In order to promote the development of Exos in cancer immunotherapy, in this review, we comprehensively summarized the application of Exos as smart drug delivery vehicles for cancer immunotherapy. First, the biogenesis and composition of Exos are introduced. Then, Exos as drug carrier for cancer immunotherapy are discussed. Finally, the clinical translation and challenges of Exos as drug delivery vehicles are presented.
The name “exosome” (Exo) first appeared in 1981. At that time, Trams et al. (22) extracted plasma membrane-derived vesicles with 5’-nucleotidase activity, and referred the vesicles as Exos. Exos are the important subset of extracellular vesicles, possessing 40–160 nm particle size (23). A large number of researches have proven that Exos can be actively secreted by most, if not all, organisms including bacteria (24) and almost all cell types (e.g., red blood cells (25), platelets (26), immune cells (27), fibroblasts (28), endothelial cells (29), epithelial cells (30) and tumor cells (31). Their secretion mechanism is simple, and the scheme is shown in Figure 2. It is generally recognized that the generation of Exos involves three major steps: invagination, multivesicular bodies generation, and secretion (32, 33). The generation of Exos begins with the inward budding of the plasma membrane and generates several endocytic vesicles which encapsulate proteins both on the surface of the plasma membrane and in the extracellular matrix (32). And then, early sorting endosomes (ESEs) are formed under the effect of the endocytic sorting complex and the proteins required for transport. After that, ESEs mature into late sorting endosomes, and continue inward invagination to form multivesicular bodies (MVBs). Finally, MVBs, which contain many intraluminal vesicles (ILVs), can either fuse with the cytoplasmic membrane to release Exos into the extracellular environment or fuse with lysosomes or autophagosomes to be degraded.
Figure 2 The biogenesis and composition of Exos. ER; Endoplasmic reticulum, MVB; Multivesicular Body.
It is generally believed that the biogenesis of Exos is a tightly controlled process. In brief, two potential mechanisms are involved in this process: endosomal sorting complexes required for transport (ESCRT) dependent mechanism and ESCRT-independent mechanism (34). Of which, ESCRT provides a crucial mechanism for the formation and sorting of the ILVs (35). ESCRT consists of a five-part protein complex with different roles including ESCRT-0, -I, -II, -III and the AAA ATPase Vps4. Specifically, ubiquitin-binding ESCRT-0 binds directly to specific structural domains of the endosomal membrane through the action of hepatocyte growth factor-regulated tyrosine kinase substrate (HRS) with endosomal-specific phosphatidylinositol 3-phosphate (PtdIns-3-P) (36). Then, ESCRT-I and ESCRT-II are recruited by the interaction between HRS and the ESCRT-I subunit TSG101 (37), and the complexes further recruit ESCRT-III which consists of various soluble coiled-coil-containing proteins Vps2, Vps20, Vps24, and Snf7 to form a protein complex which is involved in promoting the inward budding processes (38). The ESCRT-III complex drives vesicle division and is dissociated and recovered from the MVB membrane through the mediation of the AAA ATPase Vps4 (39). An increasing number of studies demonstrated that several ESCRT-related proteins can affect the secretion of Exos (40, 41). For example, the experimental results of twenty-three components of the ESCRT machinery in Exos biogenesis and related proteins in MHC II-expressing HeLa cells by RNA interference (RNAi) have shown that silencing of HRS, STAM1 and TSG101 can reduce secretion of Exos and decrease the expression of MHC II and CD63 proteins (40, 41). Meanwhile, silencing of VPS4B increased secretion of exosome marker proteins (CD63, MHC II, HSC70), and depletion of ALIX enhanced MHC II-expression on Exos and secreting cells (40). Another research also showed that ESCRT-III-associated protein ALIX interacts with cytoplasmic adaptor syntenin, thus promoting the intraluminal budding of endosomal membrane and Exos’ secretion (42). Likewise, the depletion of ESCRT-III and its associated proteins, including CHMP4C, VTA1, increased Exos’ secretion (42).
Exos are regarded as small “progeny” of parental cells, because it contains components of parental cells. They contain thousands of proteins, lipids and nucleic acids, and the scheme is presented in Figure 2. Typically, Exos contain a variety of non-specific proteins, including heat shock proteins (HSP70, HSP90), membrane transport proteins (such as annexins and flotillin), cytoskeletal proteins (myosin, actin and tubulin), MHC proteins (MHC I and MHC II) (43), adhesion molecules (CD11b and CD54) (44), and tetraspanins protein superfamily (CD9, CD63 and CD81) which is considered as the marker protein of Exos (45). Moreover, ALIX and TSG101 proteins aforementioned are also the important components of Exos. Cell type-specific proteins have also been discovered in Exos, such as the A33 protein secreted by the human colon tumor cell line LIM1215 (46), further suggesting that their composition is related to the type and physiological condition of the source cells. In addition, Exos also possess extensive lipids, cholesterol, sphingomyelin, glycosphingolipids and different patterns of RNAs including mRNAs and non-coding RNAs (e.g., miRNAs, circRNAs, lncRNAs, ribosomal RNAs (rRNAs) and transfer RNAs (tRNAs)) (47, 48). Of which, bioactive lipids play an important role in the stability and structural rigidity of Exos, cholesterol can regulate Exos’ secretion, and sphingomyelin triggers calcium influx (47). Meanwhile, exosomal miRNAs, such as miR-214, miR-29a, miR-1, miR-126, and miR-320, participate in angiogenesis, hematopoiesis, exocytosis, and tumorigenesis (48). Moreover, exosomal lncRNAs as an intercellular signaling are also involved in the development of oncogenesis and regulation of the TME.
Exos, especially these secreted from tumor cells and immune cells, may influence the phenotype and immune function of target cells (49). In order to better understand the source of Exos as drug delivery carrier, in this section, we summarized the characteristics of immune cell-derived and tumor cell-derived Exos (TEXs).
Immune cells mainly include DCs, macrophage, B lymphocytes, T lymphocyte cells, etc. Phagocytes (e.g., macrophages and neutrophils) and natural killer (NK) cells act as the first line of defense against pathogens, rapidly activating the innate immune response and killing pathogens; T cells, B cells and related cytokines can activate specialized humoral and cellular immune responses, respectively (50). However, Exos produced by immune cells are able to inherit the properties of parent cells and participate in the innate and adaptive immune responses (20). Therefore, a large number of researches have used immune cells-derived Exos as drug carriers for cancer immunotherapy.
DCs are classical APCs that stimulate specific antigenic immune responses (23). DCs-derived Exos (DEXs), which mainly contain MHC-I, MHC-II, costimulatory molecules (CD80 and CD86), heat shock proteins (HSP70 and HSP90) and adhesion molecules (ICAM-1) (51), are the most widely used immune cells-derived drug carrier. They can activate T cells to kill cancer cells through directly binding of MHC-peptide complex and costimulatory molecules to T cell receptors (TCR) (51). Moreover, DEXs also can present the MHC-peptide complex to another DCs which is possibly an inactivated DC, thereby increasing the expression of the MHC-peptide complex, and subsequently leading to large-scale activation of T cells (52). In fact, DEXs have the same therapeutic effect as the parent DCs. For example, genetically modified DEXs contain Th2 cytokines (e.g., IL-4 and IL-10) and apoptotic proteins (e.g., FASL) to inhibit inflammation and ameliorate the extent of collagen-induced arthritis (53). In contrast, NK cells derived Exos contain NK markers like CD56, NKG2D, CD94, CD40L and killer proteins (e.g., FASL and perforin) (54). NK cells-derived Exos can induce tumor cells apoptosis by significant activation of caspase death pathways via perforin and FASL (55, 56). In addition to killer proteins, NK cell-derived Exos may also carry tumor suppressor miRNAs such as miR-186, and thus inhibiting tumor growth and TGFβ1-dependent immune escape, and all of which exhibited the therapeutic potential of NK cell-derived Exos (57).
Macrophage-derived Exos, another immune cells-derived Exos, exhibit pro-inflammatory and pro-tumor functions, which mainly depend on the phenotype of macrophages (M1 and M2 subtypes) (58, 59). For instance, M1 phenotype macrophages-derived Exos (M1-Exos) can activate NLRP3 inflammasomes to enhance the cytotoxicity of T cells and NK cells and thus inhibiting the growth of tumor (20). Moreover, they can also upregulate the expression of miRNAs (e.g., miR-146a, miR-146b and miR-21-3p) and resolve inflammation by inhibiting NF-κB and TLR signaling pathways (60, 61). Meanwhile, a study has proven that M1-Exos can repolarize M2 tumor associated macrophages (TAMs) to M1 macrophages, resulting in pro-inflammatory cytokines releasing and synergistic effects of anti-PD-L1 in tumor immunotherapy (62). In contrast, M2 phenotype macrophages derived Exos showed the ability to suppress T-cell function and participate in tumor proliferation, migration, angiogenesis, and facilitate tumor immune escape (63).
In addition, B lymphocytes and T lymphocyte cells are also immune cells used for Exos generation. B lymphocytes derived−Exos contain CD19, B cell-specific markers, and the immunogenic molecules (e.g., MHC-I, MHC-II, CD40, CD54 and CD86), which stimulate T lymphocytes proliferation, activation and T(H)2-like cytokine production (64–66). Meanwhile, T cell-derived Exos express TCR, adhesion factors and various markers including CD2, CD3, CD4, CD8, CD11c, CD25, CD69, LFA-1, CXCR4, FASL, GITR (67). In general, T lymphocyte cells are classified into two phenotypes: CD4+ T cells and CD8+ T cells (68). Depending on their functions and the expression of antigens, CD4+ T cells are further classified as regulatory T cells (Tregs), Th cells and follicular helper T cells (Tfhs) (67). The Exos secreted by different phenotypes T cells have distinct regulatory effects on immune cells and non-immune cells (67). For example, Exos purified from CD8+ T cells generate proliferation in autologous resting cells and produce a higher proportion of CD8+ T cells (69). CD8+ cytotoxic T lymphocyte (CTL)-derived Exos have a potent benefit when used as DDSs for tumor immunotherapy since the inherited CTL properties. Exos derived from IL-12-stimulated CTLs could directly activate naive CD8+ T cells in the absence of antigen, producing IFN-γ and granzyme B, and eliminating tumor cells (70, 71). Conversely, Treg-derived Exos contain specific molecular cargo (let-7b, let-7d, miRNA-155 and iNOS) and cooperate with cytokines (IL-10 and TGF-β) to perform immunosuppressive functions (72).
Furthermore, other immune cell-derived Exos as drug delivery carrier, including neutrophil-derived Exos (73), mast cell-derived Exos (74), eosinophils-derived Exos (75) and myeloid-derived suppressor cell-derived Exos (76), also showed an essential role in the immune microenvironment, participating in immune regulation, inflammatory responses, intercellular communication, etc. (77, 78).
In general, TEXs are rarely used as drug carriers for cancer immunotherapy, which mainly because they accurately reproduce the content of parent tumor cells (79–82), and transfer oncogenic signals including activated oncoproteins, transcripts, oncogenic DNA sequences and oncogenic micro-RNAs (83–85) to surrounding immune cells, stromal cells and other tumor cells, and induce various functional changes in the cells (86–89). However, TEXs also contain some immunostimulatory molecules, such as CD80, CD86, MHC complexes (90, 91). They can act as adjuvants and participate in antigen presentation, and thus stimulating the activation of immune response (92). For example, TEXs serve as effective carriers of the chemotherapeutic drug methotrexate and simultaneously act as immunomodulators, stimulating the recruitment of large quantity of neutrophils to the cholangiocarcinoma tumor region and activating the neutrophil anti-tumor response to alleviate obstructive extrahepatic cholangiocarcinoma (93). TEXs are also important mediators in intercellular communication and immune regulation, and the ability of TEXs to protect internal proteins or nucleic acids from degradation makes TEXs the most promising choice as diagnostic and prognostic biomarkers (94). Currently, TEXs are widely used as diagnostic biomarkers for non-small cell lung cancer (95), pancreatic cancer (96), colorectal cancer (97), and gastric cancer (98).
Various studies have suggested that exosome is a potential drug delivery carrier due to its high biocompatibility, low toxicity, low immunogenicity and the ability of crossing natural barriers (99). Various drug-loading strategies have been designed and developed, including incubation (100), physical loading techniques (e.g., electroporation, ultrasound and extrusion) (101, 102), and cell engineering techniques (103), etc. Their pros and cons are presented in Table 1.
Incubation is the simplest drug-loading method, where the drug diffuses into the exosome membrane or cell membrane according to a concentration gradient (99). Up to now, three incubation strategies have been developed: direct incubation, transfection reagent-mediated incubation, and source cell-mediated incubation (118). They present multiple advantages such as simple operation, no special equipment requirement, preservation of exosome integrity and with minimum damage to Exos and drugs, and these drug-loading strategies have been applied to load different types of drugs. For example, study showed that ExoCe6+R848 was constructed by simple co-incubation of HEK 293T cell-derived Exos with Chlorin e6 (Ce6) and immune adjuvant R848 to reprogram immunosuppressive M2-like phenotypic macrophages and restore the immune microenvironment (119).
Although some payloads cannot be loaded by co-incubation, commercial reagents with better transfection efficacy (e.g., lipofectamine and dharmaFECT3) have been applied to load drugs into Exos (120, 121). For instance, PD-L1 siRNA can be entrapped by Lipofectamine® 2000, and then, adding Exos inside can decrease its cytotoxicity and improve its targeting (120). In addition, source cell-mediated incubation was also utilized to obtain DDSs (122). Specifically, donor cells were co-incubated with drugs, causing the secretion of Exos loaded with active drug components (123). Although incubation presents many advantages, the variety of encapsulated drugs is limited and the drug-loading efficiency is relatively low (124).
Physical loading methods including electroporation, ultrasound and extrusion have also been widely applied to load drugs (125). Electroporation is a strategy that drugs are instantaneously loaded into Exos under an electrical impulse (126). In this situation, when the transmembrane potential reaches a certain threshold, a hydrophilic channel is formed in the membrane allowing small molecules hydrophilic nucleic acids to be rapidly loaded by the electric field, followed by self-healing of membranes, which can improve the drug-loading efficiency (127). In view of this, bone marrow mesenchymal stem cell (BM-MSC) Exos were loaded with galectin-9 siRNA by electroporation and modified with oxaliplatin (OXA) prodrug as an immunogenic cell death trigger to disrupt tumor immunosuppression by M2 TAMs and recruit cytotoxic T lymphocytes, achieving significant therapeutic efficacy in the immunotherapy of pancreatic ductal adenocarcinoma (128). In another study, it was indicated that the loading efficiency of electroporation was three times higher than that of normal incubation (129). Although electroporation showed high drug-loading efficiency, it may damage the intact structure of the Exos and cause cargo aggregation. Therefore, in order to solve these shortcomings, various innovative electroporation strategies were developed to load cargo into Exos (130, 131). Chang et al. (130) batch-produced a 3D NEP chip with a uniform and parallel nanochannel array. The results indicated that this chip showed a significant higher efficiency and transfection uniformity. In addition, our groups (131) developed a cellular nanoporation (CNP) biochip with 500 nm nanochannels, and the scheme is presented in Figure 3. In this work, pores were produced in the cell membrane under transient electrical pulses, and DNA plasmids were shuttled from the buffer into cells. The experimental results indicated that this approach causes less cellular damage and produces more than 50-fold Exos than that of conventional strategies. Moreover, more than 1000-fold mRNA transcripts were loaded inside compared to control. Different from this, the ultrasound method allows the drug to enter the Exos via disrupting the Exos membrane by mechanical shear (132). However, ultrasound can result in a degree of membrane damage (106). Extrusion is a technology that breaks the exosome membrane by external force, allowing the mixture of Exos and drugs to recombine into a new exosome (110). Though physical loading methods have been widely used, they also exist some limitations, such as damage the stability and integrity of Exos, specialized equipment requirement and limitation of production scale (118).
Figure 3 Schematic representation of CNP-generated EVs for targeted nucleic acid delivery (Reprinted with the permission from Ref. 131). Abbreviation: CNP: Cellular Nanoporation; BBB: Blood Brain Barrier; BBTB: Blood Brain Tumor Barrier.
In addition to above-mentioned approaches, cell engineering technology is also a drug-loading method. It is a technology that modify the donor cells through gene editing technology or other methods to secrete Exos with target proteins on the cell surface. This approach is the most well-established and complex method, and has been extensively applied to load cargo into Exos (133, 134). Yong et al. (117) developed Exosome-sheathed doxorubicin-loaded PSiNPs (DOX@E-PSiNPs) generated by exocytosis of the tumor cells after treatment with DOX-loaded porous silicon nanoparticles (PSiNPs), penetrating deep into the tumor and exhibiting significant tumor toxicity. In spite of the wide range of applications and greater scope for manipulation of cellular engineering modifications, there are still limitations, such as complicated operations and uncertainty about the cargo of Exos and the amount of cargo (135).
Exos as a promising drug carrier show the advantage of good biocompatibility, low toxicity, and low immunogenicity. Exos-based cancer immunotherapy strategies including tuning of immunosuppressive tumor microenvironment (ITME), ICB, and cancer vaccines have been widely applied, as shown in Table 2. In order to better understand these strategies, their research status was summarized below.
As is known to us, TME is very complex and comprised of multiple components including cytokines, inflammatory cytokines, extracellular matrix and blood vessels, etc. (3). It plays an important role in the recruitment of immune cells and tumor progression (143). However, some cancer cells may evade immune systems due to the downregulation of tumor associated antigens, high infiltration of multiple immunosuppressive cells such as TAMs, and low expression of antitumor cytokines (144). In addition, both the physicochemical properties of cancer cells (e.g., hypoxia and weak acidity) and the abnormal metabolic activities can also promote the immune escape of tumors, resulting in an ITME, which becomes one of the major obstacles in cancer immunotherapy (136). Therefore, tuning of ITME can efficiently enhance cancer immunotherapeutic effects.
TAMs, essential elements of the immune responses in TME, play a critical role in inhibiting tumor growth and metastasis (6). TAMs were divided into two phenotypes: tumor-suppressing M1 macrophages and tumor-promoting M2 macrophages. In general, TME promotes the functionality of TAMs into M2 phenotypes, and M2 macrophages produce immunosuppressive cytokines to facilitate tumor progression (145). In contrast, M1 macrophages are activated by pro-inflammatory cytokines, resulting in tumor suppression (146). Thus, regulation of macrophage polarization from M2 phenotypes to M1 phenotypes can efficiently inhibit cancer progression. In order to reactivate TME and enhance the efficiency of breast cancer therapy, Zhao et al. (6) designed and established exosome delivery system derived from M1 macrophage (DTX-M1-Exo). The results indicated that DTX-M1-Exo can promote the development of naïve macrophages into M1 phenotype. Meanwhile, M1 macrophages was long-term maintained by modulating mitochondrial function. DTX-M1-Exo showed a great antitumor therapeutic efficacy. Similarly, Zhou et al. (128) designed and developed a pancreatic-targeting Exos-based dual delivery biosystem (iEXO-OXA) for pancreatic immunotherapy, and the scheme is shown in Figure 4A). In their work, Exos were secreted from bone marrow mesenchymal stem cell. Galectin-9 siRNA was loaded inside Exos by electroporation method, and OXA was modified on the surface to trigger immunogenic cell death. The results indicated that iEXO-OXA promoted the polarization of M2 phenotype to M1 phenotype upon disrupting the combination of galectin-9 and dectin 1, and TME was reprogrammed, increasing anti-tumor immunity for pancreatic cancer. Moreover, researches showed that high molecular-weight folic acid could suppresses M1 macrophage polarization and enhance M2 polarization, resulting in immunosuppression (15). In order to modulate TME, Feng et al. (15) designed and fabricated folic acid modified exos with expressing of human hyaluronidase (PH20) drug delivery platform (Exos-PH20-FA) for cancer therapy. The results indicated that Exos-PH20-FA can degrade high molecular-weight folic acid to low-molecular-weight folic acid and polarize macrophages to the M1 type, thereby improving the efficiency of cancer therapy. Meanwhile, Exos-PH20-FA also reduced tumor cell metastasis, which provides a promising treatment for cancer.
Figure 4 (A) Pancreatic-targeting exosomes-based dual delivery biosystem for pancreatic immunotherapy and reprogramming tumor microenvironment (Reprinted with the permission from Ref. 128); (B) The Scheme of NEs-Exos system for glioma immunotherapy (Reprinted with the permission from Ref. 147); (C) The preparation scheme and therapeutic process of Exo@DOX–EPT1 for oral squamous cell carcinoma (Reprinted with the permission from Ref. 136). iEXO-OXA, Exosomes losding oxaliplatin; ICD, immunogenic cell death; EPT1, Endoperoxide derivative; NPs, Nanoparticles; DOX, Doxorubicin; ROS, reactive oxygen; Ce6, Chlorin e6.
Persistent inflammation is also another characteristic of TME (146). It can induce stromal destruction and normal tumor vasculature, and thus inhibiting tumor growth. Researches showed that the secretion of pro-inflammatory cytokines, such as TNF-α, can trigger the apoptosis of cancer cells in tumor site (148). Wang et al. (109) established DDSs based on M1-EXOs. The results indicated that the expression level of caspase-3 and pro-inflammatory cytokines were elevated when M1-Exos were exposed around macrophages. Macrophages were polarized to M1 phenotype, and thus enhancing antitumor activity. In addition, in recent years, inflammatory TME targeting has been recognized as a promising and attractive therapeutic strategy. Encouraged by these, Wang et al. (147) designed and developed a neutrophil-Exos (NEs-Exos) system to deliver DOX for glioma immunotherapy, and the scheme is shown in Figure 4B). First, they isolated Exos from neutrophil by ultracentrifugation technique. And then, DOX was loaded inside Exos by sonication. The cellular uptake and the effect of NEs-Exos in vitro were investigated. In addition, the tumor-targetability and anti-glioma effect of NEs-Exos were also examined in vivo. The results indicated that NEs-Exos not only present the ability of crossing blood brain barrier, but can also respond to inflammatory stimuli and move to inflamed glioma site.
In addition, owing to high glycolysis rate and increased production of lactate, weak acidity becomes another distinct hallmark of ITME, and it can induce irreversible tumor metastasis and promote the tumor growth (149). Therefore, many researchers were devoted to develop pH-responsive DDSs to target tumors and improve tumor therapy efficiency. Kim et al. (149) fabricated a pH-responsive DDSs based on i-motif-modified Exos (Exo-i-motif) to delivery DOX for anti-proliferation activity. The results indicated that Exo-i-motif showed significant anti-proliferation effect in MCF-7/MDR cells. Meanwhile, hypoxia is another feature of ITME and it can promote the tumor growth (149). In this situation, the reactive oxygen (ROS) secreted could correct hypoxia in TME and suppress cancer cells. Therefore, targeting acidic TME and correcting the hypoxic TME is also a promising approach for cancer therapy. Based on these, Zhang et al. (136) established a novel pH/light sensitive drug delivery platform using milk-Exos (Exo@DOX–EPT1) in squamous cell carcinoma therapy, and the scheme is shown in Figure 4C). In their work, DOX was conjugated to the membrane of Exos by a pH-cleavable bond which can target acidic microenvironment. Endoperoxides and Ce6 were both incorporated inside the Exos. The results indicated that Exo@DOX–EPT1 can be efficiently accumulated in tumor site and DOX was specifically released by acid environment stimulation. Ce6 could produce plasmonic heat upon NIR irradiating and ROS was effectively released to kill cancer cells.
ICB as an emerging cancer immunotherapy can block the regulatory receptors which are expressed on immune cells or tumor cells, and thus activating antitumor cytotoxic T-cell responses and improving cancer therapy efficiency (110, 150). In the past years, PD-1 and CTLA-4 inhibitory receptors were extensively studied and undergone clinic success (151). Despite ICB showed excellent cancer therapy effects, and some inhibitors including anti-CTLA-4 and PD-L1 monoclonal antibodies have been approved by FDA, however, some limitations still exist such as high off-target, low objective response rate and the risk of immune-related side effects (152). Therefore, in order to solve aforementioned drawbacks, many researchers focus on ICB inhibitors DDSs. For instance, Fan et al. (137) developed an Exos-based DDSs (named as cGAMP@dual-anti-Exos) in which anti-PD-L1 and anti-CD40 were all engineered on the surface of Exos for cancer immunotherapy, and the scheme is shown in Figure 5A). Firstly, lipophilic DSPE-PEG-anti-CD40 and DSPE-PEG-PLGVA-anti-PD-L1 were synthesized and applied to donor cells. Meanwhile, immune drug (2’-3’-cyclic guanosine monophosphate-adenosine monophosphate (cGAMP)) was also incubated with donor cells. And then, cGAMP@dual-anti-Exos was generated with these molecules loaded inside. The results indicated that cGAMP@dual-anti-Exos presented excellent targeting and anti-tumor effects, since PLGVA peptides could be cut off by the matrix metalloproteinase enzyme (MMP-2) inside the TME, and anti-PD-L1 was separated from Exos to achieve ICB (137). Similarly, Zhou et al. (153) designed and fabricated exosome-mimetic nanovesicles co-loading CD73 inhibitor (AB680) and PD-L1 antibodies (AB680@EMVs-aPDL1) to target bladder cancer, and the scheme is presented in Figure 5B). In this work, macrophage cell line (RAW264.7 cells) was chosen to secret exosome-mimetic nanovesicles and AB680 was loaded inside by coextrusion method. After that, PD-L1 antibodies was conjugated to the surface of the exosome-mimetic nanovesicles for ICB. The results suggested that AB680@EMVs-aPDL1 was conducive to drive the transition of CD8+ T-cells into effector cells owing to the existence of CD73 molecules. Moreover, a more efficient antitumor effect to PD-1 inhibition and better tumor regression were presented owing to a higher CD8+/CD4+ ratio in bladder cancer. In addition, the toxicity and biosafety in vivo were also evaluated, indicating that AB680@EMVs-aPDL1 was safe and had low toxicity. This work also provides a new and useful strategy for bladder cancer immunotherapy.
Figure 5 (A) The fabricated scheme of cGAMP@dual-anti-Exos and the process of cancer immunotherapy (Reprinted with the permission from Ref. 137). (B) The scheme of AB680@EMVs-aPDL1 for bladder cancer therapy (Reprinted with the permission from Ref. 153). (C) The mechanism of cRGD-Exo/siMix for colorectal cancer immunotherapy in vivo (Reprinted with the permission from Ref. 154). PD-1; Programmed cell death protein 1, PD-L1; Programmed cell death ligand; MMP-2; Matrix metalloproteinase enzyme; cGAMP; 2’-3’-cyclic guanosine monophosphate–adenosine monophosphate; AB680; CD73 inhibitor, EMVs; Exosome-mimetic nanovesicles, aPDL1; Monoclonal antibody targeting programmed cell death ligand 1; cRGD-Exo/siMix; a cyclic RGD peptide (cRGD)-modified exosome delivery system that simultaneously delivered FGL1 and TGFβ1 siRNAs.
CTLA-4, which belongs to the CD28 receptor family, is overexpressed on the activated T cells and Tregs (10). It interacts with CD80/CD86 molecules expressed on the APCs and impedes T-cell activation and downregulates immune responses (21). Therefore, blocking the interaction between CTLA-4 and CD80/CD86 molecules on the APCs can activate T cells and enhance tumor immunotherapeutic efficacy. Recently, many researchers focus on this therapeutic strategy. For example, Phung et al. (10) constructed an exosome-based drug delivery platform (EXO-OVA-mAb) in which Exos were secreted from DCs and anti-CTLA-4 antibody was modified on their surface. EXO-OVA-mAb presented stronger ability of activating T cells than others and increased the CTLs/Treg ratio within the tumor site, and this phenomenon may be attributed to the crucial role of anti-CTLA-4 antibody. Moreover, EXO-OVA-mAb also increased the level of IFN-γ and TNF-α in both serum and tumors, and thus enhancing cancer therapeutic effect.
CD47 as another immune checkpoint is also overexpressed on the most tumor cells, and it often interacts with signal regulatory protein α (SIRPα) on phagocytic cells, which activates “don’t eat me signal” of CD47 and leads tumor cells to escape from immune monitoring (16). Therefore, blocking the interaction between CD47 and SIRPα can enhance tumor therapeutic efficacy. Based on this strategy, Koh et al. (155) designed and developed SIRPα-Exos for interfering CD47-SIRPa interaction to enhance cancer immunotherapy. In their work, plasmid DNA encoding SIRPa variant was firstly constructed and cocultured with HEK293T cells. And then, engineered Exos with SIRPα proteins were obtained by ultracentrifuged method. Finally, the anti-tumor effect was evaluated in mouse model. The results indicated that SIRPα-Exos presented higher CD47 affinity than control Exos, and enhanced tumor cell phagocytosis in vitro and in vivo. In addition, the existence of SIRPα-Exos also improved the infiltration of CD8+ T cell, suggesting that SIRPα-Exos could efficiently induce tumor phagocytosis and lead to anti-tumor T cell response.
In addition, silencing the expression of tumor immune checkpoint is also another strategy for cancer immunotherapy. Pei et al. (154) established a cyclic RGD peptide (cRGD)-modified exosome co-loaded with siFGL1 and siTGF-β1 (cRGD-Exo/siMix) for colorectal cancer immunotherapy by ICB, and the scheme is shown in Figure 5C). cRGD-Exo/siMix can efficiently deliver siFGL1 to silence the expression of tumor immune checkpoint ligand FGL1, and T cells were significantly activated.
It is well known that cancer immunotherapy is largely dependent on the functions of APCs and T cell, because the cancer immunity cycle must be repeatedly initiated and expanded to achieve sustained cancer immune response. In view of this, many researchers focus on cancer immunotherapy via Exos-based therapeutic cancer vaccines.
DEXs have been widely used in therapeutic vaccines as an effective alternative to tumor antigens and have tremendous potential for cancer immunotherapy due to their features of long validity period and easily being engineered (156). DEXs express peptide/MHC-I and peptide/MHC-II complexes (pMHC I and pMHC II), heat-shock proteins (HSP), costimulatory molecules (CD80, CD86) and adhesion molecules, and they are involved in antigen uptake and presentation, and also activation of the antitumor response in CD4+ and CD8+ T cells (157), and the interaction mechanism is shown in Figure 6. Research found that Exos secreted from α-fetoprotein (AFP)-expressing DCs (DEXAFP) stimulated CD8+ T lymphocytes to express IFN-γ and secrete IL-2, which leaded to the reduced CD25+Foxp3+ Treg, IL-10 and TGF-β in the tumor microenvironment (158). DEXAFP elicited potent antigen-specific immune responses and was proved to be a cell-free vaccine for immunotherapy. Furthermore, a novel EXO-T vaccine was developed which converted the exhausted T cells into tumor-specific effector CTL via the CD40L signaling pathway of CD4+ T cells to stimulate a more massive CTL anti-tumor response (159). Moreover, HER2-specific exosome (EXO)-T vaccine was also developed to trigger the activation of immune responses and assist in the treatment against HER2-positive breast cancer (160).
Figure 6 Interaction of DEXs with immune cells. CTL; cytotoxic T lymphocyte, DCs; Dendritic cells, MFG-E8; milk fat globule EGF factor 8, iCAMs; intercellular cell adhesion molecules, BAG6; Bcl-2-associated athanogen-6, NKG2D-L; natural killer group 2, member D receptor ligands, Dex; DC-derived exosomes, MHC; major histocompatibility complex.
In general, TEXs can also interfere with the immune system by delivering tumor antigens to DCs. However, because TEXs have the dual role of immunosuppressive and immune activating effects, there is a concern that TEXs will block antigen processing and presentation in DCs (161–164). Study showed that TEXs could be used for vaccine with immunostimulatory effects because they have the same rejection antigens as tumor cells (165). Recently, TEXs containing tumor-specific antigens were extracted from autologous tumor tissue to regulate the Th1 immune response in melanoma, and they blocked tumor growth and metastasis (166). However, the immune response elicited by TEXs is relatively weak which results in the unsatisfactory antitumor effect, so efforts have been made to generate vaccine systems, such as artificially modified TEXs and TEXs-loaded DC, with higher immunogenicity, (167). Common strategies for TEXs modifications include genetic modification (168), external stimulation of donor cells (169), and incorporation of fusion proteins (170). CIITA (Class II transactivator) gene was transduced into B16F1 murine melanoma cell line (B16F1- CIITA) by genetic engineering, and the secreted Exos (CIITA- Exo) expressed high level of MHC-II as well as the tumor antigen TRP2. CIITA-Exo enhanced the splenocyte proliferation and IL-2 secretion, and induced inflammatory cytokines (such as TNF-α and IL-12) mRNA production, so that CIITA-Exo had a more potent anti-tumor immune response compared to control Exos (168). In addition, Morishita et al. (9) chose TEXs as tumor antigen carrier to establish a tumor antigens-adjuvant co-delivery system. In their work, firstly, murine melanoma B16BL6 tumor cells were engineered to produce Exos expressing SAV-LA, and then immunostimulatory CpG DNA was modified on the surface of Exos by SAV-biotin interaction (CpG-SAV-Exo), and the scheme is shown in Figure 7A). The results indicated that CpG-SAV-Exo could efficiently deliver CpG DNA to APC, showing a high antigens-presenting capacity. Meanwhile, CpG-SAV-Exo can efficiently activate T cells and present an excellent antitumor efficacy. Apart from genetic modification, to enrich Exos with more HSP70, external heat stimulation was applied to tumor cells, and the HSP70-enriched Exos (HS Exo) was shown to increase the expression of MHC-II and achieve higher productions of IgG2a and IFN-γ, resulting in strong Th1 immune responses and eliminating cancer cells (169). In addition, the incorporation of viral fusion proteins (such as the G protein of vesicular stomatitis virus (VSV-G)) into TEXs enhances their uptake, induces the maturation of DCs, and improves immunogenicity (172). Co-expression of antigen OVA and VSV-G on TEXs induced a specific CTL immune response in vivo, as exhibited with increased IgG2a antibody responses and amplification of antigen-specific CD8+ T cells (170). In addition, another strategy to enhance TEXs vaccine activity is the application of TEXs-loaded DCs, which is due to the advantage of efficient antigen processing and MHC I loading of DCs after co-incubation with TAA-TEXs. Therefore, in vitro activation and loading of TEXs into DCs initiate an effective antitumor response, which overcomes the immunosuppressive limitations of TEXs alone (165). TEXs-loaded DCs activate T lymphocytes to develop into antigen-specific CTLs and trigger specific CTL immune responses with strongly cytotoxicity to autologous tumor cells (173). In a similar study, DCs that loaded with Exos from the supernatant of HeLa cells (HeLa-TEXs) enhanced the proliferation and cytotoxic activity of CTLs, whereas HeLa-TEXs alone showed no effect (174). Before TEXs-loaded DCs were developed, DCs were also used to load tumor lysates, but there is no disputing that TEXs are a better source of TAA due to the better antigen processing and presentation (175). In a comparative study, TEXs-loaded DCs (DC-TEXs) was significantly superior to lysate-loaded DCs in vaccination efficacy. TEX is more effective than tumor lysates in inducing an appropriate anti-tumor immune response, avoiding potentially fatalities in inoculated mice, and providing more persistent antigen presentation and priority antigen processing (176). Overall, immunogenic Exos could serve as adjuvants for therapeutic cancer vaccines in the future.
Figure 7 (A) The scheme of CpG-SAV-exo to deliver APCs (Reprinted with the permission from Ref. 9); (B) The scheme of MHC-I positive exosomes for activating anti-tumor responses (Reprinted with the permission from Ref. 171). SAV; Streptavidin, SAV-LA; N-terminal secretion signal of lactadherin (LA) and C1C2 domain of LA.
In addition, a novel strategy for directly activating T cells was also introduced in recently years. Zhao et al. (171) designed and developed a microfluidic device to produce antigenic Exos modified with peptide complex (e.g., gp-100, MART-1, and MAGE-A3) on demand. They also designed magnetic-nanoparticles with functionalized photo-cleavable and peptide affinity probe for capturing antigenic Exos via a light trigger. Meanwhile, the antitumor capability of antigenic Exos was also evaluated in vitro and in vivo, and the scheme is shown in Figure 7B). The results indicated that Exos which were modified with melanoma tumor peptides including gp-100, MART-1 and MAGE-A3 enhanced the ability of antigen presentation and T cell activation. This is because MHC-I and tumor peptides can form MHC-I/peptide binding complex which can be presented to cytotoxic T cells and thus triggering an immediate response from the immune system (3, 171). Moreover, conjugating cytokine-loaded Exos to T cells surfaces is also another strategy that can enhance adaptive T cell therapy. This approach is simple and can minimize systemic side effects of adjuvant drugs (21).
The development of immunotherapy has yielded remarkable results in recent years. Currently, various ICIs have been approved by FDA as single agents for cancer treatment, however, the response rate for ICIs is only 10-35% (177–179). The effectiveness of immunotherapy is directly dependent on the state of the tumor microenvironment, while TME mostly presents an immunosuppressed condition with lack of T-cell infiltration or dysfunction, poor immunogenicity. Moreover, multiple mechanisms of drug resistance also contribute to the low efficiency in immunotherapy (180). Therefore, new alternative treatment strategies are being explored, and combination therapy containing two or three anti-tumor approaches (including chemotherapy, radiotherapy, photodynamic therapy, targeted therapy, vaccines, oncolytic viruses, ICB, ACT etc.) to achieve higher efficacy is under evaluation (181, 182). Chemotherapeutic agents [such as anthracyclines, cyclophosphamide, oxaliplatin and paclitaxel (183)] are highly cytotoxic. However, chemotherapeutic drugs can trigger immunogenic cell death to act as adjuvants for immunotherapy by releasing damage-associated molecular patterns and activating apoptosis which make tumors more sensitive to immunotherapy (184). TEX-loaded DC vaccine in combination with chemotherapy could effectively suppress tumor-infiltrating MDSCs, inhibite tumor cell migration and promote greater T-cell activation, resulting in a longer survival time compared to DCs-TEX vaccinated only mice (185). Likewise, radiotherapy can enhance the antitumor effects of immunotherapy by increasing tumor antigenicity through multiple approaches. Radiation has an abscopal effect allowing for systemic tumor control (186) and can trigger the cGAS/STING pathway and stimulate innate and adaptive immune responses through DNA damage and ROS production (187). Short-burst radiation treatment significantly enhanced the delivery efficiency of PD-L1siRNA-loaded targeted Exos, altered the immune environment, sensitized poorly immunogenic glioblastomas to ICB, inhibited tumor growth, and prolonged the survival of tumor-bearing mice (188, 189). Otherwise, photodynamic therapy in synergy with immunotherapy has become a focus of research to overcome the low efficacy of immunotherapy for primary tumors and to monitor the drug delivery status at the target site (190). TEXs loaded with the photosensitizer Ce6 have been used as vehicles for photoacoustic-guided photodynamic therapy and as tumor antigens to stimulate the immune system to activate anti-tumor responses (191), and the scheme is illustrated in Figure 8A. The lack of tumor infiltration in ICB can also be addressed by oncolytic viruses, which provide a critical switch for the immune system. Oncolytic viruses invade tumor cells and replicate extensively inside, leading to tumor cell lysis (194), and on the other hand recruiting TILs into the damaged tumor, initiating the release of tumor antigens and pro-inflammatory cytokines and promoting the activation of the immune system (195). The scheme is shown in Figure 8B, and it was demonstrated that VSVΔ51 oncolytic viruses loaded with artificial amiRNA-4, when co-targeted with Exos carrying amiRNA-4 and PD-L1 shRNA cargoes, upregulated PD-L1 expression, sensitized tumors to CTLA4 and PD-1 immune checkpoint inhibition, enhanced death of tumor cells, and prolonged overall survival in mice (192). Chimeric antigen receptor T (CAR T) cell therapy has achieved remarkable results in hematologic malignancies, but the results in solid tumors have been less than satisfactory. TDC-Exo, a DC-secreted exosome stimulated by tumor antigen carrying MHC-antigen complexes and CD86, was developed as the “CAR” portion of CAR-T, activating T cells and cooperating with anti-CD3 and anti-EGFR and immune checkpoint inhibitory antibodies anti-PD-L1, further enhancing the efficacy of the CAR-T cell therapy mimetic platform for solid tumor treatment (193). The scheme is demonstrated in Figure 8C.
Figure 8 (A) Schematic diagram of photoacoustic imaging-guided combined photodynamic and immunotherapy for Ce6-R-Exo treatment (Reprinted with the permission from Ref. 191) (B) Schematic illustration of VSVΔ51-amiR-4-shPD-L1 exerting enhanced T cell-mediated cancer cell death (Reprinted with the permission from Ref. 192) (C) The above diagram is a schematic view of the construction of the engineered tDC-Exo (Exo-OVA-aCD3/aEGFR) with anti-CD3 and anti-EGFR antibodies. The bottom diagram shows the simulated CAR-T treatment process (Reprinted with the permission from Ref. 193). R-Exo; re-assembled exosome, Ce6-R-Exo; chlorin e6-loaded R-Exo, IMT; immunotherapy, PDT; photodynamic therapy, PA; photoacoustic, VSVΔ51-amiR-4-shPD-L1; VSVΔ51 oncolytic viruses- artificial microRNA-4- shPD-L1, MVB; multivesicular bodies, SEVs; small extracellular vesicles, DC; dendritic cells, tDC-Exo;tumor antigen-stimulated dendritic cell-derived exosomes, aEGFR; anti-epidermal growth factor receptors antibodies, OVA; ovalbumin, CAR; chimeric antigen receptor, TCR; T cell receptor, MHC; major histocompatibility complex.
Currently, several cancers therapeutic strategies-based Exos DDSs have undergone clinic trials, and the relevant data which was obtained from https://clinicaltrials.gov/ are present in Table 3. As shown in Table 3, only few of Exos-based DDSs for cancer therapy have entered into clinical trials. Moreover, all of them are in the early clinical stage, suggesting that they still face many challenges.
Firstly, the stable mass production of Exos is the primary challenge. As is known to all, the selection and culture of donor cells are one of significantly important factors. In recent years, mesenchymal stromal cells and cardiac progenitor cells have been proved to provide stable Exos production during scale-up culture. Moreover, cell culture technologies have also been improved and up to 20,000 L of cells can also be cultured via stainless-steel bioreactors. In spite of this, the clinical translation of Exos is still difficult. The main reason is that the scaling-up process is relatively expensive. Furthermore, the conditions of cell culture also need to be meticulous, because improper operation may cause cell contamination, which can result in cell subtypes and variation. Therefore, strictly controlling and maintaining the genetic stability of donor cells are difficult.
Secondly, the isolation and purification of Exos are another challenge. Currently, the extraction technologies including ultracentrifugation, tangential flow fractionation, exclusion chromatography and commercial extraction kits have been extensively employed to isolate Exos. Of which, tangential flow fractionation is often used in the mass production of Exos in the clinical trials. However, the purity of Exos obtained by this separation method is low, thereby limiting its application. Although high purity of Exos can be obtained by ultracentrifugation, its features of low throughput and high cost limit the mass production of Exos. Currently, there is no standard procedures for large-scale Exos separation. Therefore, it is urgent to develop an advanced technique with high efficiency, high quality and low cost to separate Exos for DDSs.
Furthermore, the surface modification of Exos is also one of important factors because it affects the targeting functions and biological effects of DDSs. In general, two methods including chemical modification and genetic engineering can be used for the surface modification of Exos. Genetic engineering is highly effective for surface modification by fusing the gene sequence of targeting protein with exosomal membrane protein. However, this approach is limited to genetically encoded targeting motifs. Chemical modification often affects the structure and function of Exos, and thus limiting their application. Meanwhile, there is no standard strategies for loading drugs. Recently, many drug-loading strategies including incubation, electroporation, ultrasound, and cell engineering techniques have been applied. They all have their limitations to some extent. For instance, incubation is the simplest drug-loading method. It does not require special equipment, and the structure of Exos is rarely damaged. However, low drug-loading efficiency was presented in this loading method. Although ultrasound and electroporation can improve drug-loading efficiency, the membrane of exosome maybe damaged and aggregation of Exos may be caused by these methods. Meanwhile, the operation of cell engineering techniques is too complicated though it is considered as well-established operating strategy.
In addition to these limitations mentioned above, the storage conditions of purified Exos also play critical role in clinical translation of Exos. An increasing number of researches suggested that Exos derived from different sources require different storage conditions, because the storage temperature and storage solution (e.g., saline, PBS, cell culture media, etc.) all affected the particle size and protein content of Exos. Therefore, further researches should take the influences of storge conditions into consideration for Exos as drug delivery carriers.
In this review, some relevant knowledges including the biogenesis and composition of Exos, the source of Exos for DDSs, drug-loading strategies, cancer immunotherapy strategies, and their clinical translation and challenges were discussed. Exos are mainly divided into immune cell-derived and tumor cell-derived Exos. They can inherit the properties of donor cells and participate in the innate and adaptive immune responses, thus promoting extensive applications of immune cells-derived Exos as drug delivery carrier. Meanwhile, various drug-loading strategies of Exos-based DDSs including incubation, physical loading techniques (e.g., electroporation, ultrasound and extrusion), and cell engineering techniques have been designed and developed. In addition, Exos-based cancer immunotherapy strategies (e.g., tuning of ITME, ICB, cancer vaccines, etc.) have been extensively applied, they all presented excellent therapeutic effects.
To our delight, nowadays, several cancers immunotherapeutic strategies-based Exos DDSs have undergone clinic trials. In spite of this, they still face many challenges including their stable mass production, their isolation and purification, their surface modification, and their storage conditions. Therefore, cell culture technologies should be further improved and related bioreactors should also be designed and developed to scale up the Exos production in the future. In addition, it is urgent to develop an advanced technique with high efficiency, high quality and low cost to separate and purify Exos which can be used in DDSs. Meanwhile, the storage conditions of purified Exos from different cell sources should be further explored. Overall, although Exos as drug delivery vesicles still exist some challenges, they provide an excellent platform for cancer immunotherapy.
All authors listed have made a substantial, direct, and intellectual contribution to the work and approved it for publication.
This review paper was supported by the projects of National Natural Science Foundation of China (82073784(LT) and 21HAA01203(ZY)), and Forestry Science and Technology Innovation Project of Guangdong Province (No. 2021KJCX010(ZX))
We acknowledged the support of BioRender, Figure 1, Figure 2 and Figure 6 were created with BioRender.com.
The authors declare that the research was conducted in the absence of any commercial or financial relationships that could be construed as a potential conflict of interest.
All claims expressed in this article are solely those of the authors and do not necessarily represent those of their affiliated organizations, or those of the publisher, the editors and the reviewers. Any product that may be evaluated in this article, or claim that may be made by its manufacturer, is not guaranteed or endorsed by the publisher.
1. Siegel RL, Miller KD, Fuchs HE, Jemal A. Cancer statistics 2022. CA Cancer J Clin (2022) 72:7–33. doi: 10.3322/caac.21708
2. Wang X, Zhao YR, Dong SY, Lee RJ, Yang DS, Zhang H, et al. Cell-penetrating peptide and transferrin co-modified liposomes for targeted therapy of glioma. Molecules (2019) 24:3540. doi: 10.3390/molecules24193540
3. Yang Z, Ma Y, Zhao H, Yuan Y, Kim BYS. Nanotechnology platforms for cancer immunotherapy. Wiley Interdiscip Rev Nanomed Nanobiotechnol (2020) 12:e1590. doi: 10.1002/wnan.1590
4. Cai GS, Wang SM, Zhao L, Sun YT, Yang DS, Lee RJ, et al. Thiophene derivatives as anticancer agents and their delivery to tumor cells using albumin nanoparticles. Molecules (2019) 24:192. doi: 10.3390/molecules24010192
5. Thakur A, Parra DC, Motallebnejad P, Brocchi M, Chen HJ. Exosomes: Small vesicles with big roles in cancer, vaccine development, and therapeutics. Bioactive Mater (2022) 10:281–94. doi: 10.1016/j.bioactmat.2021.08.029
6. Zhao Y, Zheng Y, Zhu Y, Li H, Zhu H, Liu T. Docetaxel-loaded M1 macrophage-derived exosomes for a safe and efficient chemoimmunotherapy of breast cancer. J Nanobiotechnol (2022) 20:359. doi: 10.1186/s12951-022-01526-2
7. Tan M, Chen Y, Guo Y, Yang C, Liu M, Guo D, et al. A low-intensity focused ultrasound-assisted nanocomposite for advanced triple cancer therapy: Local chemotherapy, therapeutic extracellular vesicles and combined immunotherapy. Biomater Sci (2020) 8:6703–17. doi: 10.1039/d0bm00804d
8. Ma XB, Yang SC, Zhang T, Wang S, Yang QC, Xiao Y, et al. Bioresponsive immune-booster-based prodrug nanogel for cancer immunotherapy. Acta Pharm Sin B (2022) 12:451–66. doi: 10.1016/j.apsb.2021.05.0162211-3835
9. Morishita M, Takahashi Y, Matsumoto A, Nishikawa M, Takakura Y. Exosome-based tumor antigens-adjuvant co-delivery utilizing genetically engineered tumor cell-derived exosomes with immunostimulatory CpG DNA. Biomaterials (2016) 111:55–65. doi: 10.1016/j.biomaterials.2016.09.031
10. Phung CD, Pham TT, Nguyen HT, Nguyen TT, Ou W, Jeong JH, et al. Anti-CTLA-4 antibody-functionalized dendritic cell-derived exosomes targeting tumor-draining lymph nodes for effective induction of antitumor T-cell responses. Acta Biomater (2020) 115:371–82. doi: 10.1016/j.actbio.2020.08.008
11. Rasihashemi SZ, Sahrai H, Rezazadeh-Gavgani E, Yazdani Y, Khalaji A, Lotfinejad P. Exosomes carrying immune checkpoints, a promising therapeutic approach in cancer treatment. Med Oncol (2022) 39:183. doi: 10.1007/s12032-022-01781-1
12. Tran TH, Mattheolabakis G, Aldawsari H, Amiji M. Exosomes as nanocarriers for immunotherapy of cancer and inflammatory diseases. Clin Immunol (2015) 160:46–58. doi: 10.1016/j.clim.2015.03.021
13. Francis DM, Thomas SN. Progress and opportunities for enhancing the delivery and efficacy of checkpoint inhibitors for cancer immunotherapy. Adv Drug Delivery Rev (2017) 114:33–42. doi: 10.1016/j.addr.2017.04.011
14. Choi JU, Park IK, Lee YK, Hwang SR. The biological function and therapeutic potential of exosomes in cancer: Exosomes as efficient nanocommunicators for cancer therapy. Int J Mol Sci (2020) 21:7363. doi: 10.3390/ijms21197363
15. Feng C, Xiong Z, Wang C, Xiao W, Xiao H, Xie K, et al. Folic acid-modified exosome-PH20 enhances the efficiency of therapy via modulation of the tumor microenvironment and directly inhibits tumor cell metastasis. Bioactive Mater (2021) 6:963–74. doi: 10.1016/j.bioactmat.2020.09.014
16. Kamerkar S, LeBleu VS, Sugimoto H, Yang S, Ruivo CF, Melo SA, et al. Exosomes facilitate therapeutic targeting of oncogenic KRAS in pancreatic cancer. Nature (2017) 546:498–503. doi: 10.1038/nature22341
17. Fang Z, Ding Y, Xue Z, Li P, Li J, Li F. Roles of exosomes as drug delivery systems in cancer immunotherapy: a mini-review. Discovery Oncol (2022) 13:74. doi: 10.1007/s12672-022-00539-5
18. Chen R, Xu X, Qian Z, Zhang C, Niu Y, Wang Z, et al. The biological functions and clinical applications of exosomes in lung cancer. Cell Mol Life Sci (2019) 76:4613–33. doi: 10.1007/s00018-019-03233-y
19. Bell BM, Kirk ID, Hiltbrunner S, Gabrielsson S, Bultema JJ. Designer exosomes as next-generation cancer immunotherapy. Nanomedicine (2016) 12:163–9. doi: 10.1016/j.nano.2015.09.011
20. Hazrati A, Soudi S, Malekpour K, Mahmoudi M, Rahimi A, Hashemi SM, et al. Immune cells-derived exosomes function as a double-edged sword: role in disease progression and their therapeutic applications. biomark Res (2022) 10:30. doi: 10.1186/s40364-022-00374-4
21. Jo SD, Nam G-H, Kwak G, Yang Y, Kwon IC. Harnessing designed nanoparticles: Current strategies and future perspectives in cancer immunotherapy. Nano Today (2017) 17:23–37. doi: 10.1016/j.nantod.2017.10.008
22. Trams EG, Lauter CJ, Salem N Jr., Heine U. Exfoliation of membrane ecto-enzymes in the form of micro-vesicles. Biochim Biophys Acta (1981) 645:63–70. doi: 10.1016/0005-2736(81)90512-5
23. Marar C, Starich B, Wirtz D. Extracellular vesicles in immunomodulation and tumor progression. Nat Immunol (2021) 22:560–70. doi: 10.1038/s41590-021-00899-0
24. Guo S, Zhao L, Tao S, Zhang C. Research progress on the role of extracellular vesicles in bacterial pathogenesis. Zhongguo Xiu Fu Chong Jian Wai Ke Za Zhi (2018) 32:1597–604. doi: 10.7507/1002-1892.201805075
25. Danesh A, Inglis HC, Jackman RP, Wu S, Deng X, Muench MO, et al. Exosomes from red blood cell units bind to monocytes and induce proinflammatory cytokines, boosting T-cell responses in vitro. Blood (2014) 123:687–96. doi: 10.1182/blood-2013-10-530469
26. Aatonen MT, Ohman T, Nyman TA, Laitinen S, Grönholm M, Siljander PR. Isolation and characterization of platelet-derived extracellular vesicles. J Extracell Vesicles (2014) 3:24692. doi: 10.3402/jev.v3.24692
27. Yu X, Huang C, Song B, Xiao Y, Fang M, Feng J, et al. CD4+CD25+ regulatory T cells-derived exosomes prolonged kidney allograft survival in a rat model. Cell Immunol (2013) 285:62–8. doi: 10.1016/j.cellimm.2013.06.010
28. Luga V, Wrana JL. Tumor-stroma interaction: Revealing fibroblast-secreted exosomes as potent regulators of wnt-planar cell polarity signaling in cancer metastasis. Cancer Res (2013) 73:6843–7. doi: 10.1158/0008-5472.Can-13-1791
29. Ju R, Zhuang ZW, Zhang J, Lanahan AA, Kyriakides T, Sessa WC, et al. Angiopoietin-2 secretion by endothelial cell exosomes: regulation by the phosphatidylinositol 3-kinase (PI3K)/Akt/endothelial nitric oxide synthase (eNOS) and syndecan-4/syntenin pathways. J Biol Chem (2014) 289:510–9. doi: 10.1074/jbc.M113.506899
30. Riches A, Campbell E, Borger E, Powis S. Regulation of exosome release from mammary epithelial and breast cancer cells-a new regulatory pathway. Eur J Cancer (2014) 50:1025–34. doi: 10.1016/j.ejca.2013.12.019
31. Kim SM, Yang Y, Oh SJ, Hong Y, Seo M, Jang M. Cancer-derived exosomes as a delivery platform of CRISPR/Cas9 confer cancer cell tropism-dependent targeting. J Control Release (2017) 266:8–16. doi: 10.1016/j.jconrel.2017.09.013
32. Kalluri R, LeBleu VS. The biology, function, and biomedical applications of exosomes. Science (2020) 367:640. doi: 10.1126/science.aau6977
33. Johnstone RM, Adam M, Hammond JR, Orr L, Turbide C. Vesicle formation during reticulocyte maturation. association of plasma membrane activities with released vesicles (exosomes). J Biol Chem (1987) 262:9412–20. doi: 10.1016/S0021-9258(18)48095-7
34. Katzmann DJ, Babst M, Emr SD. Ubiquitin-dependent sorting into the multivesicular body pathway requires the function of a conserved endosomal protein sorting complex, ESCRT-I. Cell (2001) 106:145–55. doi: 10.1016/s0092-8674(01)00434-2
35. Saksena S, Emr SD. ESCRTs and human disease. Biochem Soc Trans (2009) 37:167–72. doi: 10.1042/bst0370167
36. Raiborg C, Bremnes B, Mehlum A, Gillooly DJ, D'Arrigo A, Stang E, et al. FYVE and coiled-coil domains determine the specific localisation of hrs to early endosomes. J Cell Sci (2001) 114:2255–63. doi: 10.1242/jcs.114.12.2255
37. Lu Q, Hope LW, Brasch M, Reinhard C, Cohen SN. TSG101 interaction with HRS mediates endosomal trafficking and receptor down-regulation. Proc Natl Acad Sci U.S.A. (2003) 100:7626–31. doi: 10.1073/pnas.0932599100
38. Babst M, Katzmann DJ, Estepa-Sabal EJ, Meerloo T, Emr SD. Escrt-III: An endosome-associated heterooligomeric protein complex required for mvb sorting. Dev Cell (2002) 3:271–82. doi: 10.1016/s1534-5807(02)00220-4
39. Babst M, Wendland B, Estepa EJ, Emr SD. The Vps4p AAA ATPase regulates membrane association of a vps protein complex required for normal endosome function. EMBO J (1998) 17:2982–93. doi: 10.1093/emboj/17.11.2982
40. Colombo M, Moita C, van Niel G, Kowal J, Vigneron J, Benaroch P, et al. Analysis of ESCRT functions in exosome biogenesis, composition and secretion highlights the heterogeneity of extracellular vesicles. J Cell Sci (2013) 126:5553–65. doi: 10.1242/jcs.128868
41. Tamai K, Tanaka N, Nakano T, Kakazu E, Kondo Y, Inoue J, et al. Exosome secretion of dendritic cells is regulated by hrs, an ESCRT-0 protein. Biochem Biophys Res Commun (2010) 399:384–90. doi: 10.1016/j.bbrc.2010.07.083
42. Baietti MF, Zhang Z, Mortier E, Melchior A, Degeest G, Geeraerts A, et al. Syndecan-syntenin-ALIX regulates the biogenesis of exosomes. Nat Cell Biol (2012) 14:677–85. doi: 10.1038/ncb2502
43. McAndrews KM, Kalluri R. Mechanisms associated with biogenesis of exosomes in cancer. Mol Cancer (2019) 18:52. doi: 10.1186/s12943-019-0963-9
44. Cho JA, Yeo DJ, Son HY, Kim HW, Jung DS, Ko JK, et al. Exosomes: a new delivery system for tumor antigens in cancer immunotherapy. Int J Cancer (2005) 114:613–22. doi: 10.1002/ijc.20757
45. Zhang Y, Liu Y, Liu H, Tang WH. Exosomes: biogenesis, biologic function and clinical potential. Cell Biosci (2019) 9:19. doi: 10.1186/s13578-019-0282-2
46. Mathivanan S, Lim JW, Tauro BJ, Ji H, Moritz RL, Simpson RJ. Proteomics analysis of A33 immunoaffinity-purified exosomes released from the human colon tumor cell line LIM1215 reveals a tissue-specific protein signature. Mol Cell Proteomics (2010) 9:197–208. doi: 10.1074/mcp.M900152-MCP200
47. Skotland T, Sandvig K, Llorente A. Lipids in exosomes: current knowledge and the way forward. Prog Lipid Res (2017) 66:30–41. doi: 10.1016/j.plipres.2017.03.001
48. Xie Y, Dang W, Zhang S, Yue W, Yang L, Zhai X, et al. The role of exosomal noncoding RNAs in cancer. Mol Cancer (2019) 18:37. doi: 10.1186/s12943-019-0984-4
49. Valcz G, Újvári B, Buzás EI, Krenács T, Spisák S, Kittel Á., et al. Small extracellular vesicle DNA-mediated horizontal gene transfer as a driving force for tumor evolution: Facts and riddles. Front Oncol (2022) 12:945376. doi: 10.3389/fonc.2022.945376
50. Xu M, Yang Q, Sun X, Wang Y. Recent advancements in the loading and modification of therapeutic exosomes. Front Bioeng Biotechnol (2020) 8:586130. doi: 10.3389/fbioe.2020.586130
51. Viaud S, Théry C, Ploix S, Tursz T, Lapierre V, Lantz O, et al. Dendritic cell-derived exosomes for cancer immunotherapy: What's next? Cancer Res (2010) 70:1281–5. doi: 10.1158/0008-5472.Can-09-3276
52. Théry C, Duban L, Segura E, Véron P, Lantz O, Amigorena S. Indirect activation of naïve CD4+ T cells by dendritic cell-derived exosomes. Nat Immunol (2002) 3:1156–62. doi: 10.1038/ni854
53. Bianco NR, Kim SH, Morelli AE, Robbins PD. Modulation of the immune response using dendritic cell-derived exosomes. Methods Mol Biol (2007) 380:443–55. doi: 10.1007/978-1-59745-395-0_28
54. Shoae-Hassani A, Hamidieh AA, Behfar M, Mohseni R, Mortazavi-Tabatabaei SA, Asgharzadeh S. NK cell-derived exosomes from NK cells previously exposed to neuroblastoma cells augment the antitumor activity of cytokine-activated NK cells. J Immunother (2017) 40:265–76. doi: 10.1097/cji.0000000000000179
55. Federici C, Shahaj E, Cecchetti S, Camerini S, Casella M, Iessi E, et al. Natural-killer-derived extracellular vesicles: immune sensors and interactors. Front Immunol (2020) 11:262. doi: 10.3389/fimmu.2020.00262
56. Fais S. NK cell-released exosomes: Natural nanobullets against tumors. Oncoimmunology (2013) 2:e22337. doi: 10.4161/onci.22337
57. Neviani P, Wise PM, Murtadha M, Liu CW, Wu CH, Jong AY, et al. Natural killer-derived exosomal miR-186 inhibits neuroblastoma growth and immune escape mechanisms. Cancer Res (2019) 79:1151–64. doi: 10.1158/0008-5472.Can-18-0779
58. McDonald MK, Tian Y, Qureshi RA, Gormley M, Ertel A, Gao R, et al. Functional significance of macrophage-derived exosomes in inflammation and pain. Pain (2014) 155:1527–39. doi: 10.1016/j.pain.2014.04.029
59. Cheng L, Wang Y, Huang L. Exosomes from M1-polarized macrophages potentiate the cancer vaccine by creating a pro-inflammatory microenvironment in the lymph node. Mol Ther (2017) 25:1665–75. doi: 10.1016/j.ymthe.2017.02.007
60. Taganov KD, Boldin MP, Chang KJ, Baltimore D. NF-kappaB-dependent induction of microRNA miR-146, an inhibitor targeted to signaling proteins of innate immune responses. Proc Natl Acad Sci U.S.A. (2006) 103:12481–6. doi: 10.1073/pnas.0605298103
61. Wang G, Jin S, Ling X, Li Y, Hu Y, Zhang Y, et al. Proteomic profiling of LPS-induced macrophage-derived exosomes indicates their involvement in acute liver injury. Proteomics (2019) 19:e1800274. doi: 10.1002/pmic.201800274
62. Choo YW, Kang M, Kim HY, Han J, Kang S, Lee JR, et al. M1 macrophage-derived nanovesicles potentiate the anticancer efficacy of immune checkpoint inhibitors. ACS Nano (2018) 12:8977–93. doi: 10.1021/acsnano.8b02446
63. Arteaga-Blanco LA, Mojoli A, Monteiro RQ, Sandim V, Menna-Barreto RFS, Pereira-Dutra FS, et al. Characterization and internalization of small extracellular vesicles released by human primary macrophages derived from circulating monocytes. PloS One (2020) 15:e0237795. doi: 10.1371/journal.pone.0237795
64. Admyre C, Bohle B, Johansson SM, Focke-Tejkl M, Valenta R, Scheynius A, et al. B cell-derived exosomes can present allergen peptides and activate allergen-specific T cells to proliferate and produce TH2-like cytokines. J Allergy Clin Immunol (2007) 120:1418–24. doi: 10.1016/j.jaci.2007.06.040
65. Klinker MW, Lizzio V, Reed TJ, Fox DA, Lundy SK. Human b cell-derived lymphoblastoid cell lines constitutively produce fas ligand and secrete MHCII(+)FasL(+) killer exosomes. Front Immunol (2014) 5:144. doi: 10.3389/fimmu.2014.00144
66. Wubbolts R, Leckie RS, Veenhuizen PT, Schwarzmann G, Möbius W, Hoernschemeyer J, et al. Proteomic and biochemical analyses of human b cell-derived exosomes. potential implications for their function and multivesicular body formation. J Biol Chem (2003) 278:10963–72. doi: 10.1074/jbc.M207550200
67. Lu J, Wu J, Tian J, Wang S. Role of T cell-derived exosomes in immunoregulation. Immunol Res (2018) 66:313–22. doi: 10.1007/s12026-018-9000-0
68. Geltink RIK, Kyle RL, Pearce EL. Unraveling the complex interplay between t cell metabolism and function. Annu Rev Immunol (2018) 36:461–88. doi: 10.1146/annurev-immunol-042617-053019
69. Wahlgren J, Karlson Tde L, Glader P, Telemo E, Valadi H. Activated human T cells secrete exosomes that participate in IL-2 mediated immune response signaling. PloS One (2012) 7:e49723. doi: 10.1371/journal.pone.0049723
70. Li L, Jay SM, Wang Y, Wu SW, Xiao Z. IL-12 stimulates CTLs to secrete exosomes capable of activating bystander CD8(+) T cells. Sci Rep (2017) 7:13365. doi: 10.1038/s41598-017-14000-z
71. Mittelbrunn M, Gutiérrez-Vázquez C, Villarroya-Beltri C, González S, Sánchez-Cabo F, González M, et al. Unidirectional transfer of microRNA-loaded exosomes from T cells to antigen-presenting cells. Nat Commun (2011) 2:282. doi: 10.1038/ncomms1285
72. Okoye IS, Coomes SM, Pelly VS, Czieso S, Papayannopoulos V, Tolmachova T, et al. MicroRNA-containing T-regulatory-cell-derived exosomes suppress pathogenic T helper 1 cells. Immunity (2014) 41:503. doi: 10.1016/j.immuni.2014.08.008
73. Allen ER, Lempke SL, Miller MM, Bush DM, Braswell BG, Estes CL, et al. Effect of extracellular vesicles from s. aureus-challenged human neutrophils on macrophages. J Leukoc Biol (2020) 108:1841–50. doi: 10.1002/jlb.3ab0320-156r
74. Li F, Wang Y, Lin L, Wang J, Xiao H, Li J, et al. Mast cell-derived exosomes promote Th2 cell differentiation via OX40L-OX40 ligation. J Immunol Res (2016) 2016:3623898. doi: 10.1155/2016/3623898
75. Cañas JA, Sastre B, Mazzeo C, Fernández-Nieto M, Rodrigo-Muñoz JM, González-Guerra A, et al. Exosomes from eosinophils autoregulate and promote eosinophil functions. J Leukoc Biol (2017) 101:1191–9. doi: 10.1189/jlb.3AB0516-233RR
76. Zöller M. Janus-faced myeloid-derived suppressor cell exosomes for the good and the bad in cancer and autoimmune disease. Front Immunol (2018) 9:137. doi: 10.3389/fimmu.2018.00137
77. Zhu D, Tian J, Wu X, Li M, Tang X, Rui K, et al. G-MDSC-derived exosomes attenuate collagen-induced arthritis by impairing Th1 and Th17 cell responses. Biochim Biophys Acta Mol Basis Dis (2019) 1865:165540. doi: 10.1016/j.bbadis.2019.165540
78. Skokos D, Botros HG, Demeure C, Morin J, Peronet R, Birkenmeier G, et al. Mast cell-derived exosomes induce phenotypic and functional maturation of dendritic cells and elicit specific immune responses in vivo. J Immunol (2003) 170:3037–45. doi: 10.4049/jimmunol.170.6.3037
79. Wieckowski EU, Visus C, Szajnik M, Szczepanski MJ, Storkus WJ, Whiteside TL. Tumor-derived microvesicles promote regulatory T cell expansion and induce apoptosis in tumor-reactive activated CD8+ T lymphocytes. J Immunol (2009) 183:3720–30. doi: 10.4049/jimmunol.0900970
80. Whiteside TL. The effect of tumor-derived exosomes on immune regulation and cancer immunotherapy. Future Oncol (2017) 13:2583–92. doi: 10.2217/fon-2017-0343
81. Ren W, Zhang X, Li W, Feng Q, Feng H, Tong Y, et al. Exosomal miRNA-107 induces myeloid-derived suppressor cell expansion in gastric cancer. Cancer Manag Res (2019) 11:4023–40. doi: 10.2147/cmar.S198886
82. Choi D, Spinelli C, Montermini L, Rak J. Oncogenic regulation of extracellular vesicle proteome and heterogeneity. Proteomics (2019) 19:e1800169. doi: 10.1002/pmic.201800169
83. Zhang Y, Pfannenstiel LW, Bolesta E, Montes CL, Zhang X, Chapoval AI, et al. Interleukin-7 inhibits tumor-induced CD27-CD28- suppressor T cells: implications for cancer immunotherapy. Clin Cancer Res (2011) 17:4975–86. doi: 10.1158/1078-0432.Ccr-10-3328
84. Tauro BJ, Mathias RA, Greening DW, Gopal SK, Ji H, Kapp EA, et al. Oncogenic h-ras reprograms madin-Darby canine kidney (MDCK) cell-derived exosomal proteins following epithelial-mesenchymal transition. Mol Cell Proteomics (2013) 12:2148–59. doi: 10.1074/mcp.M112.027086
85. Whiteside TL. Exosomes and tumor-mediated immune suppression. J Clin Invest (2016) 126:1216–23. doi: 10.1172/jci81136
86. Groh V, Wu J, Yee C, Spies T. Tumour-derived soluble MIC ligands impair expression of NKG2D and T-cell activation. Nature (2002) 419:734–8. doi: 10.1038/nature01112
87. Qu JL, Qu XJ, Zhao MF, Teng YE, Zhang Y, Hou KZ, et al. Gastric cancer exosomes promote tumour cell proliferation through PI3K/Akt and MAPK/ERK activation. Dig Liver Dis (2009) 41:875–80. doi: 10.1016/j.dld.2009.04.006
88. Melo SA, Sugimoto H, O'Connell JT, Kato N, Villanueva A, Vidal A, et al. Cancer exosomes perform cell-independent microRNA biogenesis and promote tumorigenesis. Cancer Cell (2014) 26:707–21. doi: 10.1016/j.ccell.2014.09.005
89. de la Torre Gomez C, Goreham RV, Bech Serra JJ, Nann T, Kussmann M. "Exosomics"-a review of biophysics, biology and biochemistry of exosomes with a focus on human breast milk. Front Genet (2018) 9:92. doi: 10.3389/fgene.2018.00092
90. Whiteside TL. Tumor-derived exosomes and their role in cancer progression. Adv Clin Chem (2016) 74:103–41. doi: 10.1016/bs.acc.2015.12.005
91. Whiteside TL. The potential of tumor-derived exosomes for noninvasive cancer monitoring: an update. Expert Rev Mol Diagn (2018) 18:1029–40. doi: 10.1080/14737159.2018.1544494
92. Zhao H, Yang L, Baddour J, Achreja A, Bernard V, Moss T, et al. Tumor microenvironment derived exosomes pleiotropically modulate cancer cell metabolism. Elife (2016) 5:e10250. doi: 10.7554/eLife.10250
93. Gao Y, Zhang H, Zhou N, Xu P, Wang J, Gao Y, et al. Methotrexate-loaded tumour-cell-derived microvesicles can relieve biliary obstruction in patients with extrahepatic cholangiocarcinoma. Nat BioMed Eng (2020) 4:743–53. doi: 10.1038/s41551-020-0583-0
94. Nedaeinia R, Manian M, Jazayeri MH, Ranjbar M, Salehi R, Sharifi M, et al. Circulating exosomes and exosomal microRNAs as biomarkers in gastrointestinal cancer. Cancer Gene Ther (2017) 24:48–56. doi: 10.1038/cgt.2016.77
95. Jakobsen KR, Paulsen BS, Bæk R, Varming K, Sorensen BS, Jørgensen MM. Exosomal proteins as potential diagnostic markers in advanced non-small cell lung carcinoma. J Extracell Vesicles (2015) 4:26659. doi: 10.3402/jev.v4.26659
96. An M, Lohse I, Tan Z, Zhu J, Wu J, Kurapati H, et al. Quantitative proteomic analysis of serum exosomes from patients with locally advanced pancreatic cancer undergoing chemoradiotherapy. J Proteome Res (2017) 16:1763–72. doi: 10.1021/acs.jproteome.7b00024
97. Jia S, Zhang R, Li Z, Li J. Clinical and biological significance of circulating tumor cells, circulating tumor DNA, and exosomes as biomarkers in colorectal cancer. Oncotarget (2017) 8:55632–45. doi: 10.18632/oncotarget.17184
98. Fu H, Yang H, Zhang X, Wang B, Mao J, Li X, et al. Exosomal TRIM3 is a novel marker and therapy target for gastric cancer. J Exp Clin Cancer Res (2018) 37:162. doi: 10.1186/s13046-018-0825-0
99. Didiot MC, Hall LM, Coles AH, Haraszti RA, Godinho BM, Chase K, et al. Exosome-mediated delivery of hydrophobically modified siRNA for huntingtin mRNA silencing. Mol Ther (2016) 24:1836–47. doi: 10.1038/mt.2016.126
100. Zhuang X, Xiang X, Grizzle W, Sun D, Zhang S, Axtell RC, et al. Treatment of brain inflammatory diseases by delivering exosome encapsulated anti-inflammatory drugs from the nasal region to the brain. Mol Ther (2011) 19:1769–79. doi: 10.1038/mt.2011.164
101. Alvarez-Erviti L, Seow Y, Yin H, Betts C, Lakhal S, Wood MJ. Delivery of siRNA to the mouse brain by systemic injection of targeted exosomes. Nat Biotechnol (2011) 29:341–5. doi: 10.1038/nbt.1807
102. Kobayashi M, Sawada K, Miyamoto M, Shimizu A, Yamamoto M, Kinose Y, et al. Exploring the potential of engineered exosomes as delivery systems for tumor-suppressor microRNA replacement therapy in ovarian cancer. Biochem Biophys Res Commun (2020) 527:153–61. doi: 10.1016/j.bbrc.2020.04.076
103. Pascucci L, Coccè V, Bonomi A, Ami D, Ceccarelli P, Ciusani E, et al. Paclitaxel is incorporated by mesenchymal stromal cells and released in exosomes that inhibit in vitro tumor growth: a new approach for drug delivery. J Control Release (2014) 192:262–70. doi: 10.1016/j.jconrel.2014.07.042
104. Haney MJ, Klyachko NL, Zhao Y, Gupta R, Plotnikova EG, He Z, et al. Exosomes as drug delivery vehicles for parkinson's disease therapy. J Control Release (2015) 207:18–30. doi: 10.1016/j.jconrel.2015.03.033
105. Lin Y, Wu J, Gu W, Huang Y, Tong Z, Huang L, et al. Exosome-liposome hybrid nanoparticles deliver CRISPR/Cas9 system in MSCs. Adv Sci (Weinh) (2018) 5:1700611. doi: 10.1002/advs.201700611
106. Salarpour S, Forootanfar H, Pournamdari M, Ahmadi-Zeidabadi M, Esmaeeli M, Pardakhty A. Paclitaxel incorporated exosomes derived from glioblastoma cells: Comparative study of two loading techniques. Daru (2019) 27:533–9. doi: 10.1007/s40199-019-00280-5
107. Kim MS, Haney MJ, Zhao Y, Mahajan V, Deygen I, Klyachko NL, et al. Development of exosome-encapsulated paclitaxel to overcome MDR in cancer cells. Nanomedicine (2016) 12:655–64. doi: 10.1016/j.nano.2015.10.012
108. Li X, Li X, Lin J, Sun X, Ding Q. Exosomes derived from low-intensity pulsed ultrasound-treated dendritic cells suppress tumor necrosis factor-induced endothelial inflammation. J Ultrasound Med (2019) 38:2081–91. doi: 10.1002/jum.14898
109. Wang P, Wang H, Huang Q, Peng C, Yao L, Chen H, et al. Exosomes from M1-polarized macrophages enhance paclitaxel antitumor activity by activating macrophages-mediated inflammation. Theranostics (2019) 9:1714–27. doi: 10.7150/thno.30716
110. Yang Z, Xie J, Zhu J, Kang C, Chiang C, Wang X, et al. Functional exosome-mimic for delivery of siRNA to cancer: In vitro and in vivo evaluation. J Control Release (2016) 243:160–71. doi: 10.1016/j.jconrel.2016.10.008
111. Sun L, Fan M, Huang D, Li B, Xu R, Gao F, et al. Clodronate-loaded liposomal and fibroblast-derived exosomal hybrid system for enhanced drug delivery to pulmonary fibrosis. Biomaterials (2021) 271:120761. doi: 10.1016/j.biomaterials.2021.120761
112. Zhou W, Chen X, Zhou Y, Shi S, Liang C, Yu X, et al. Exosomes derived from immunogenically dying tumor cells as a versatile tool for vaccination against pancreatic cancer. Biomaterials (2022) 280:121306. doi: 10.1016/j.biomaterials.2021.121306
113. Li L, He D, Guo Q, Zhang Z, Ru D, Wang L, et al. Exosome-liposome hybrid nanoparticle codelivery of TP and miR497 conspicuously overcomes chemoresistant ovarian cancer. J Nanobiotechnol (2022) 20:50. doi: 10.1186/s12951-022-01264-5
114. Jia G, Han Y, An Y, Ding Y, He C, Wang X, et al. NRP-1 targeted and cargo-loaded exosomes facilitate simultaneous imaging and therapy of glioma in vitro and in vivo. Biomaterials (2018) 178:302–16. doi: 10.1016/j.biomaterials.2018.06.029
115. Suetsugu A, Honma K, Saji S, Moriwaki H, Ochiya T, Hoffman RM. Imaging exosome transfer from breast cancer cells to stroma at metastatic sites in orthotopic nude-mouse models. Adv Drug Delivery Rev (2013) 65:383–90. doi: 10.1016/j.addr.2012.08.007
116. Tian Y, Li S, Song J, Ji T, Zhu M, Anderson GJ, et al. A doxorubicin delivery platform using engineered natural membrane vesicle exosomes for targeted tumor therapy. Biomaterials (2014) 35:2383–90. doi: 10.1016/j.biomaterials.2013.11.083
117. Yong T, Zhang X, Bie N, Zhang H, Zhang X, Li F, et al. Tumor exosome-based nanoparticles are efficient drug carriers for chemotherapy. Nat Commun (2019) 10:3838. doi: 10.1038/s41467-019-11718-4
118. Mehryab F, Rabbani S, Shahhosseini S, Shekari F, Fatahi Y, Baharvand H, et al. Exosomes as a next-generation drug delivery system: An update on drug loading approaches, characterization, and clinical application challenges. Acta Biomater (2020) 113:42–62. doi: 10.1016/j.actbio.2020.06.036
119. Wang D, Wan Z, Yang Q, Chen J, Liu Y, Lu F, et al. Sonodynamical reversion of immunosuppressive microenvironment in prostate cancer via engineered exosomes. Drug Delivery (2022) 29:702–13. doi: 10.1080/10717544.2022.2044937
120. Lv H, Wang T, Ma F, Zhang K, Gao T, Pei R, et al. Aptamer-functionalized targeted siRNA delivery system for tumor immunotherapy. BioMed Mater (2022) 17:024108. doi: 10.1088/1748-605X/ac5415
121. Dong SXM, Caballero R, Ali H, Roy DLF, Cassol E, Kumar A. Transfection of hard-to-transfect primary human macrophages with bax siRNA to reverse resveratrol-induced apoptosis. RNA Biol (2020) 17:755–64. doi: 10.1080/15476286.2020.1730081
122. Fu W, Li T, Chen H, Zhu S, Zhou C. Research progress in exosome-based nanoscale drug carriers in tumor therapies. Front Oncol (2022) 12:919279. doi: 10.3389/fonc.2022.919279
123. Wang J, Yeung BZ, Cui M, Peer CJ, Lu Z, Figg WD, et al. Exosome is a mechanism of intercellular drug transfer: Application of quantitative pharmacology. J Control Release (2017) 268:147–58. doi: 10.1016/j.jconrel.2017.10.020
124. Agrawal AK, Aqil F, Jeyabalan J, Spencer WA, Beck J, Gachuki BW, et al. Milk-derived exosomes for oral delivery of paclitaxel. Nanomedicine (2017) 13:1627–36. doi: 10.1016/j.nano.2017.03.001
125. Lee J, Lee JH, Chakraborty K, Hwang J, Lee YK. Exosome-based drug delivery systems and their therapeutic applications. RSC Adv (2022) 12:18475–92. doi: 10.1039/d2ra02351b
126. Familtseva A, Jeremic N, Tyagi SC. Exosomes: cell-created drug delivery systems. Mol Cell Biochem (2019) 459:1–6. doi: 10.1007/s11010-019-03545-4
127. Shi J, Ma Y, Zhu J, Chen Y, Sun Y, Yao Y, et al. A review on electroporation-based intracellular delivery. Molecules (2018) 23:3044. doi: 10.3390/molecules23113044
128. Zhou W, Zhou Y, Chen X, Ning T, Chen H, Guo Q, et al. Pancreatic cancer-targeting exosomes for enhancing immunotherapy and reprogramming tumor microenvironment. Biomaterials (2021) 268:120546. doi: 10.1016/j.biomaterials.2020.120546
129. Toffoli G, Hadla M, Corona G, Caligiuri I, Palazzolo S, Semeraro S, et al. Exosomal doxorubicin reduces the cardiac toxicity of doxorubicin. Nanomed (Lond) (2015) 10:2963–71. doi: 10.2217/nnm.15.118
130. Chang L, Bertani P, Gallego-Perez D, Yang Z, Chen F, Chiang C, et al. 3D nanochannel electroporation for high-throughput cell transfection with high uniformity and dosage control. Nanoscale (2016) 8:243–52. doi: 10.1039/c5nr03187g
131. Yang Z, Shi J, Xie J, Wang Y, Sun J, Liu T, et al. Large-Scale generation of functional mRNA-encapsulating exosomes via cellular nanoporation. Nat BioMed Eng (2020) 4:69–83. doi: 10.1038/s41551-019-0485-1
132. Liao W, Du Y, Zhang C, Pan F, Yao Y, Zhang T, et al. Exosomes: The next generation of endogenous nanomaterials for advanced drug delivery and therapy. Acta Biomater (2019) 86:1–14. doi: 10.1016/j.actbio.2018.12.045
133. Armstrong JP, Holme MN, Stevens MM. Re-engineering extracellular vesicles as smart nanoscale therapeutics. ACS Nano (2017) 11:69–83. doi: 10.1021/acsnano.6b07607
134. Liang Y, Xu X, Li X, Xiong J, Li B, Duan L, et al. Chondrocyte-targeted MicroRNA delivery by engineered exosomes toward a cell-free osteoarthritis therapy. ACS Appl Mater Interfaces (2020) 12:36938–47. doi: 10.1021/acsami.0c10458
135. Xu Z, Zeng S, Gong Z, Yan Y. Exosome-based immunotherapy: a promising approach for cancer treatment. Mol Cancer (2020) 19:160. doi: 10.1186/s12943-020-01278-3
136. Zhang Q, Xiao Q, Yin H, Xia C, Pu Y, He Z, et al. Milk-exosome based pH/light sensitive drug system to enhance anticancer activity against oral squamous cell carcinoma. RSC Adv (2020) 10:28314–23. doi: 10.1039/d0ra05630h
137. Fan Y, Zhou Y, Lu M, Si H, Li L, Tang B. Responsive dual-targeting exosome as a drug carrier for combination cancer immunotherapy. Res (Wash D C) (2021) 2021:9862876. doi: 10.34133/2021/9862876
138. Cheng Q, Dai Z, Smbatyan G, Epstein AL, Lenz HJ, Zhang Y. Eliciting anti-cancer immunity by genetically engineered multifunctional exosomes. Mol Ther (2022) 30:3066–77. doi: 10.1016/j.ymthe.2022.06.013
139. Ji P, Yang Z, Li H, Wei M, Yang G, Xing H, et al. Smart exosomes with lymph node homing and immune-amplifying capacities for enhanced immunotherapy of metastatic breast cancer. Mol Ther Nucleic Acids (2021) 26:987–96. doi: 10.1016/j.omtn.2021.10.009
140. Zhou X, Miao Y, Wang Y, He S, Guo L, Mao J, et al. Tumour-derived extracellular vesicle membrane hybrid lipid nanovesicles enhance siRNA delivery by tumour-homing and intracellular freeway transportation. J Extracell Vesicles (2022) 11:e12198. doi: 10.1002/jev2.12198
141. Yang P, Cao X, Cai H, Feng P, Chen X, Zhu Y, et al. The exosomes derived from CAR-T cell efficiently target mesothelin and reduce triple-negative breast cancer growth. Cell Immunol (2021) 360:104262. doi: 10.1016/j.cellimm.2020.104262
142. Shi X, Cheng Q, Hou T, Han M, Smbatyan G, Lang JE, et al. Genetically engineered cell-derived nanoparticles for targeted breast cancer immunotherapy. Mol Ther (2020) 28:536–47. doi: 10.1016/j.ymthe.2019.11.020
143. Chen JM, Fei XF, Wang JL, Cai ZJ. Tumor-derived extracellular vesicles: Regulators of tumor microenvironment and the enlightenment in tumor therapy. Pharmacol Res (2020) 159:105041. doi: 10.1016/j.phrs.2020.105041
144. Taghikhani A, Farzaneh F, Sharifzad F, Mardpour S, Ebrahimi M, Hassan ZM. Engineered tumor-derived extracellular vesicles: potentials in cancer immunotherapy. Front Immunol (2020) 11:221. doi: 10.3389/fimmu.2020.00221
145. Donoso-Meneses D, Figueroa-Valdes AI, Georges N, Tobar HE, Alcayaga-Miranda F. Turning adversity into opportunity: Small extracellular vesicles as nanocarriers for tumor-associated macrophages re-education. Bioeng Trans Med (2022) e10349. doi: 10.1002/btm2.10349
146. Rezaie J, Ahmadi M, Ravanbakhsh R, Mojarad B, Mahbubfam S, Shaban SA, et al. Tumor-derived extracellular vesicles: The metastatic organotropism drivers. Life Sci (2022) 289:120216. doi: 10.1016/j.lfs.2021.120216
147. Wang J, Tang W, Yang M, Yin Y, Li H, Hu F, et al. Inflammatory tumor microenvironment responsive neutrophil exosomes-based drug delivery system for targeted glioma therapy. Biomaterials (2021) 273:120784. doi: 10.1016/j.biomaterials.2021.120784
148. Rincon-Riveros A, Lopez L, Villegas EV, Rodriguez JA. Regulation of antitumor immune responses by exosomes derived from tumor and immune cells. Cancers (2021) 13:847. doi: 10.3390/cancers13040847
149. Kim JY, Song J, Jung H, Mok H. I-Motif-coated exosomes as a pH-sensitive carrier for anticancer drugs. Appl Biol Chem (2018) 61:599–606. doi: 10.1007/s13765-018-0394-0
150. Yong TY, Wei ZH, Gan L, Yang XL. Extracellular-vesicle-based drug delivery systems for enhanced antitumor therapies through modulating the cancer-immunity cycle. Adv Mater (2022) doi. doi: 10.1002/adma.202201054
151. Nam GH, Choi Y, Kim GB, Kim S, Kim SA, Kim IS. Emerging prospects of exosomes for cancer treatment: From conventional therapy to immunotherapy. Advanced Mater (2020) 32:2002440. doi: 10.1002/adma.202002440
152. Zocchi MR, Tosetti F, Benelli R, Poggi A. Cancer nanomedicine special issue review anticancer drug delivery with nanoparticles: Extracellular vesicles or synthetic nanobeads as therapeutic tools for conventional treatment or immunotherapy. Cancers (2020) 12:1886. doi: 10.3390/cancers12071886
153. Zhou Q, Ding W, Qian Z, Zhu Q, Sun C, Yu Q, et al. Immunotherapy strategy targeting programmed cell death ligand 1 and CD73 with macrophage-derived mimetic nanovesicles to treat bladder cancer. Mol Pharm (2021) 18:4015–28. doi: 10.1021/acs.molpharmaceut.1c00448
154. Pei X, Zhang X, Zhang L, Yuan M, Sun L, Yu F, et al. Targeted exosomes for co-delivery of siFGL1 and siTGF-β1 trigger combined cancer immunotherapy by remodeling immunosuppressive tumor microenvironment. Chem Eng J (2021) 421:129774. doi: 10.1016/j.cej.2021.129774
155. Koh E, Lee EJ, Nam GH, Hong Y, Cho E, Yang Y, et al. Exosome-SIRPα, a CD47 blockade increases cancer cell phagocytosis. Biomaterials (2017) 121:121–9. doi: 10.1016/j.biomaterials.2017.01.004
156. Pitt JM, André F, Amigorena S, Soria JC, Eggermont A, Kroemer G, et al. Dendritic cell-derived exosomes for cancer therapy. J Clin Invest (2016) 126:1224–32. doi: 10.1172/jci81137
157. Lindenbergh MFS, Stoorvogel W. Antigen presentation by extracellular vesicles from professional antigen-presenting cells. Annu Rev Immunol (2018) 36:435–59. doi: 10.1146/annurev-immunol-041015-055700
158. Lu Z, Zuo B, Jing R, Gao X, Rao Q, Liu Z, et al. Dendritic cell-derived exosomes elicit tumor regression in autochthonous hepatocellular carcinoma mouse models. J Hepatol (2017) 67:739–48. doi: 10.1016/j.jhep.2017.05.019
159. Wang R, Xu A, Zhang X, Wu J, Freywald A, Xu J, et al. Novel exosome-targeted T-cell-based vaccine counteracts T-cell anergy and converts CTL exhaustion in chronic infection via CD40L signaling through the mTORC1 pathway. Cell Mol Immunol (2017) 14:529–45. doi: 10.1038/cmi.2016.23
160. Li R, Chibbar R, Xiang J. Novel EXO-T vaccine using polyclonal CD4(+) T cells armed with HER2-specific exosomes for HER2-positive breast cancer. Onco Targets Ther (2018) 11:7089–93. doi: 10.2147/ott.S184898
161. Morse MA, Garst J, Osada T, Khan S, Hobeika A, Clay TM, et al. A phase I study of dexosome immunotherapy in patients with advanced non-small cell lung cancer. J Transl Med (2005) 3:9. doi: 10.1186/1479-5876-3-9
162. Escudier B, Dorval T, Chaput N, André F, Caby MP, Novault S, et al. Vaccination of metastatic melanoma patients with autologous dendritic cell (DC) derived-exosomes: results of thefirst phase I clinical trial. J Transl Med (2005) 3:10. doi: 10.1186/1479-5876-3-10
163. Dai S, Wei D, Wu Z, Zhou X, Wei X, Huang H, et al. Phase I clinical trial of autologous ascites-derived exosomes combined with GM-CSF for colorectal cancer. Mol Ther (2008) 16:782–90. doi: 10.1038/mt.2008.1
164. Dunand-Sauthier I, Santiago-Raber ML, Capponi L, Vejnar CE, Schaad O, Irla M, et al. Silencing of c-fos expression by microRNA-155 is critical for dendritic cell maturation and function. Blood (2011) 117:4490–500. doi: 10.1182/blood-2010-09-308064
165. Wolfers J, Lozier A, Raposo G, Regnault A, Théry C, Masurier C, et al. Tumor-derived exosomes are a source of shared tumor rejection antigens for CTL cross-priming. Nat Med (2001) 7:297–303. doi: 10.1038/85438
166. Lee EY, Park KS, Yoon YJ, Lee J, Moon HG, Jang SC, et al. Therapeutic effects of autologous tumor-derived nanovesicles on melanoma growth and metastasis. PloS One (2012) 7:e33330. doi: 10.1371/journal.pone.0033330
167. Liu Y, Gu Y, Cao X. The exosomes in tumor immunity. Oncoimmunology (2015) 4:e1027472. doi: 10.1080/2162402x.2015.1027472
168. Lee YS, Kim SH, Cho JA, Kim CW. Introduction of the CIITA gene into tumor cells produces exosomes with enhanced anti-tumor effects. Exp Mol Med (2011) 43:281–90. doi: 10.3858/emm.2011.43.5.029
169. Cho JA, Lee YS, Kim SH, Ko JK, Kim CW. MHC independent anti-tumor immune responses induced by Hsp70-enriched exosomes generate tumor regression in murine models. Cancer Lett (2009) 275:256–65. doi: 10.1016/j.canlet.2008.10.021
170. Temchura VV, Tenbusch M, Nchinda G, Nabi G, Tippler B, Zelenyuk M, et al. Enhancement of immunostimulatory properties of exosomal vaccines by incorporation of fusion-competent G protein of vesicular stomatitis virus. Vaccine (2008) 26:3662–72. doi: 10.1016/j.vaccine.2008.04.069
171. Zhao Z, McGill J, Gamero-Kubota P, He M. Microfluidic on-demand engineering of exosomes towards cancer immunotherapy. Lab Chip (2019) 19:1877–86. doi: 10.1039/c8lc01279b
172. Marsac D, Loirat D, Petit C, Schwartz O, Michel ML. Enhanced presentation of major histocompatibility complex class I-restricted human immunodeficiency virus type 1 (HIV-1) gag-specific epitopes after DNA immunization with vectors coding for vesicular stomatitis virus glycoprotein-pseudotyped HIV-1 gag particles. J Virol (2002) 76:7544–53. doi: 10.1128/jvi.76.15.7544-7553.2002
173. Bu N, Wu H, Sun B, Zhang G, Zhan S, Zhang R, et al. Exosome-loaded dendritic cells elicit tumor-specific CD8+ cytotoxic T cells in patients with glioma. J Neurooncol (2011) 104:659–67. doi: 10.1007/s11060-011-0537-1
174. Ren G, Wang Y, Yuan S, Wang B. Dendritic cells loaded with HeLa-derived exosomes simulate an antitumor immune response. Oncol Lett (2018) 15:6636–40. doi: 10.3892/ol.2018.8126
175. Mahaweni NM, Kaijen-Lambers ME, Dekkers J, Aerts JG, Hegmans JP. Tumour-derived exosomes as antigen delivery carriers in dendritic cell-based immunotherapy for malignant mesothelioma. J Extracell Vesicles (2013) 2:22492. doi: 10.3402/jev.v2i0.22492
176. Gu X, Erb U, Büchler MW, Zöller M. Improved vaccine efficacy of tumor exosome compared to tumor lysate loaded dendritic cells in mice. Int J Cancer (2015) 136:E74–84. doi: 10.1002/ijc.29100
177. Ungefroren H. Blockade of TGF-β signaling: a potential target for cancer immunotherapy? Expert Opin Ther Targets (2019a) 23:679–93. doi: 10.1080/14728222.2019.1636034
178. Ungefroren H. TGF-β signaling in cancer: control by negative regulators and crosstalk with proinflammatory and fibrogenic pathways. Cancers (Basel) (2019b) 11:384. doi: 10.3390/cancers11030384
179. Hellmann MD, Friedman CF, Wolchok JD. Combinatorial cancer immunotherapies. Adv Immunol (2016) 130:251–77. doi: 10.1016/bs.ai.2015.12.005
180. Zhu S, Zhang T, Zheng L, Liu H, Song W, Liu D, et al. Combination strategies to maximize the benefits of cancer immunotherapy. J Hematol Oncol (2021) 14:156. doi: 10.1186/s13045-021-01164-5
181. Griffith JW, Sokol CL, Luster AD. Chemokines and chemokine receptors: positioning cells for host defense and immunity. Annu Rev Immunol (2014) 32:659–702. doi: 10.1146/annurev-immunol-032713-120145
182. Klein C, Waldhauer I, Nicolini VG, Freimoser-Grundschober A, Nayak T, Vugts DJ, et al. Cergutuzumab amunaleukin (CEA-IL2v), a CEA-targeted IL-2 variant-based immunocytokine for combination cancer immunotherapy: Overcoming limitations of aldesleukin and conventional IL-2-based immunocytokines. Oncoimmunology (2017) 6:e1277306. doi: 10.1080/2162402x.2016.1277306
183. Galon J, Bruni D. Approaches to treat immune hot, altered and cold tumours with combination immunotherapies. Nat Rev Drug Discovery (2019) 18:197–218. doi: 10.1038/s41573-018-0007-y
184. Garg AD, Galluzzi L, Apetoh L, Baert T, Birge RB, Bravo-San Pedro JM, et al. Molecular and translational classifications of DAMPs in immunogenic cell death. Front Immunol (2015) 6:588. doi: 10.3389/fimmu.2015.00588
185. Xiao L, Erb U, Zhao K, Hackert T, Zöller M. Efficacy of vaccination with tumor-exosome loaded dendritic cells combined with cytotoxic drug treatment in pancreatic cancer. Oncoimmunology (2017) 6:e1319044. doi: 10.1080/2162402x.2017.1319044
186. Whiteside TL, Demaria S, Rodriguez-Ruiz ME, Zarour HM, Melero I. Emerging opportunities and challenges in cancer immunotherapy. Clin Cancer Res (2016) 22:1845–55. doi: 10.1158/1078-0432.Ccr-16-0049
187. Woo SR, Fuertes MB, Corrales L, Spranger S, Furdyna MJ, Leung MY, et al. STING-dependent cytosolic DNA sensing mediates innate immune recognition of immunogenic tumors. Immunity (2014) 41:830–42. doi: 10.1016/j.immuni.2014.10.017
188. Colak S, Ten Dijke P. Targeting TGF-β signaling in cancer. Trends Cancer (2017) 3:56–71. doi: 10.1016/j.trecan.2016.11.008
189. Tian T, Liang R, Erel-Akbaba G, Saad L, Obeid PJ, Gao J, et al. Immune checkpoint inhibition in GBM primed with radiation by engineered extracellular vesicles. ACS Nano (2022) 16:1940–53. doi: 10.1021/acsnano.1c05505
190. Lee H, Han J, Shin H, Han H, Na K, Kim H. Combination of chemotherapy and photodynamic therapy for cancer treatment with sonoporation effects. J Control Release (2018) 283:190–9. doi: 10.1016/j.jconrel.2018.06.008
191. Jang Y, Kim H, Yoon S, Lee H, Hwang J, Jung J, et al. Exosome-based photoacoustic imaging guided photodynamic and immunotherapy for the treatment of pancreatic cancer. J Control Release (2021) 330:293–304. doi: 10.1016/j.jconrel.2020.12.039
192. Wedge ME, Jennings VA, Crupi MJF, Poutou J, Jamieson T, Pelin A, et al. Virally programmed extracellular vesicles sensitize cancer cells to oncolytic virus and small molecule therapy. Nat Commun (2022) 13:1898. doi: 10.1038/s41467-022-29526-8
193. Fan M, Liu H, Yan H, Che R, Jin Y, Yang X, et al. A CAR T-inspiring platform based on antibody-engineered exosomes from antigen-feeding dendritic cells for precise solid tumor therapy. Biomaterials (2022) 282:121424. doi: 10.1016/j.biomaterials.2022.121424
194. Zamarin D, Holmgaard RB, Subudhi SK, Park JS, Mansour M, Palese P, et al. Localized oncolytic virotherapy overcomes systemic tumor resistance to immune checkpoint blockade immunotherapy. Sci Transl Med (2014) 6:226ra232. doi: 10.1126/scitranslmed.3008095
Keywords: exosomes, immunotherapy, drug delivery system, immune checkpoint blockade, tumor immune microenvironment
Citation: Zhang H, Wang S, Sun M, Cui Y, Xing J, Teng L, Xi Z and Yang Z (2023) Exosomes as smart drug delivery vehicles for cancer immunotherapy. Front. Immunol. 13:1093607. doi: 10.3389/fimmu.2022.1093607
Received: 10 November 2022; Accepted: 23 December 2022;
Published: 17 January 2023.
Edited by:
Gang Zhou, Augusta University, United StatesReviewed by:
Andrea Nicolini, University of Pisa, ItalyCopyright © 2023 Zhang, Wang, Sun, Cui, Xing, Teng, Xi and Yang. This is an open-access article distributed under the terms of the Creative Commons Attribution License (CC BY). The use, distribution or reproduction in other forums is permitted, provided the original author(s) and the copyright owner(s) are credited and that the original publication in this journal is cited, in accordance with accepted academic practice. No use, distribution or reproduction is permitted which does not comply with these terms.
*Correspondence: Zhifang Xi, eHpmMTk4MDA3QDE2My5jb20=; Zhaogang Yang, emhhb2dhbmd5YW5nQGpsdS5lZHUuY24=
†These authors have contributed equally to this work
Disclaimer: All claims expressed in this article are solely those of the authors and do not necessarily represent those of their affiliated organizations, or those of the publisher, the editors and the reviewers. Any product that may be evaluated in this article or claim that may be made by its manufacturer is not guaranteed or endorsed by the publisher.
Research integrity at Frontiers
Learn more about the work of our research integrity team to safeguard the quality of each article we publish.