- 1Department of Orthodontics, Hospital of Stomatology, Tianjin Medical University, Tianjin, China
- 2Department of Spine Surgery, Tianjin Hospital, Tianjin University, Tianjin, China
- 3Institute of Disaster and Emergency Medicine, Tianjin University, Tianjin, China
As natural nanocarriers and intercellular messengers, extracellular vesicles (EVs) control communication among cells. Under physiological and pathological conditions, EVs deliver generic information including proteins and nucleic acids to recipient cells and exert regulatory effects. Macrophages help mediate immune responses, and macrophage-derived EVs may play immunomodulatory roles in the progression of chronic inflammatory diseases. Furthermore, EVs derived from various macrophage phenotypes have different biological functions. In this review, we describe the pathophysiological significance of macrophage-derived extracellular vesicles in the development of chronic inflammatory diseases, including diabetes, cancer, cardiovascular disease, pulmonary disease, and gastrointestinal disease, and the potential applications of these EVs.
Background
Extracellular vesicles (EVs) are natural phospholipid bilayer-derived particles expressing specific surface markers (e.g., tetraspanins, Alix, and TSG101) secreted by cells into the extracellular space (1). EVs have been isolated from various types of cells, tissues, and even bodily fluids (2). They are categorized mainly as exosomes (~40–160 nm diameter), microvesicles, and apoptotic bodies (~50 nm to 1 μm diameter) (3, 4). Initially, EVs were considered waste released by cells (5). More recently, their roles in cell–cell interactions have been identified (6, 7). Various cargos in the form of nucleic acids, proteins, lipids, and metabolites are transferred by EVs to recipient cells, thereby influencing the biological functions of those cells. EVs are highly heterogeneous and dynamic, depending on the parental cell source and microenvironment (3). Due to their unique characteristics and properties, EVs are critical mediators of various physiological and pathological processes including the immune response, cell proliferation and migration, tumor invasion, and metastasis (8–10). Furthermore, they are used as diagnostic tools and as therapeutic delivery systems carrying biological factors and/or drugs (11–13).
Macrophages (Mφ) are derived from monocytes in the bone marrow and are involved in specific and nonspecific immunity of the body. In nonspecific immunity, the biological functions of Mφ include removing dead cells and cellular debris, and presenting antigens for recognition. In specific immunity, activated Mφ have immunomodulatory functions by secreting cytokines. Moreover, they play a major role in antigen presentation and initiation of the immune response (14). Mφ are divided mainly into two phenotypes: classically activated M1 (M1Mφ) and alternatively activated M2 (M2Mφ) (15). Mφ play essential roles in the microenvironment and are also regulated by that microenvironment. Phenotypic polarization of Mφ has a dynamic influence on the balance between inflammation and tissue repair. Moreover, the functions and properties of EVs secreted from Mφ (Mφ-EVs) are influenced by Mφ polarization, with different phenotypes of Mφ-EVs being involved in diverse biological processes under various physiological and pathological conditions (16).
Inflammation is defined biologically as the response of the body’s immune system to a stimulus. Mainly caused by various pathogens and tissue damage, it plays an important role in tissue repair and is considered a protective response of the organism (17). Activated Mφ dominate the histopathology of chronic inflammation and can amplify the inflammatory response by mediating the release of inflammatory mediators (18). In 2010, the WHO stated that chronic diseases such as cardiovascular disease, diabetes, cancer, and chronic respiratory disease account for approximately two-thirds of global deaths (19). To date, several studies have confirmed the link between chronic inflammation and chronic disease, although the exact mechanisms are still not clear (20–22). Inflammation not only plays an important role in host defense mechanisms but also greatly contributes to the pathological process of chronic diseases. Therefore, targeting inflammation is a promising strategy for improving and treating chronic diseases.
In this review, we summarize the crucial role of Mφ-EVs in the pathogenic mechanisms of chronic diseases such as atherosclerosis, diabetes, cancer, lung-related disease, cardiovascular-related disease, and gastrointestinal-related disease. In addition, therapeutic strategies based on Mφ-EVs are discussed, as well as the challenges associated with their application.
Mφ-EVs
EVs are vesicles derived from the phospholipid bilayer released by cells (23), including various subtypes of nanoscale-to-microscale particles. They transmit information that helps regulate recipient cells (Table 1). Furthermore, they retain the biological properties of the parental cells (71). Thus, different phenotypes of Mφ-EVs play different roles in different pathological conditions (Figure 1).
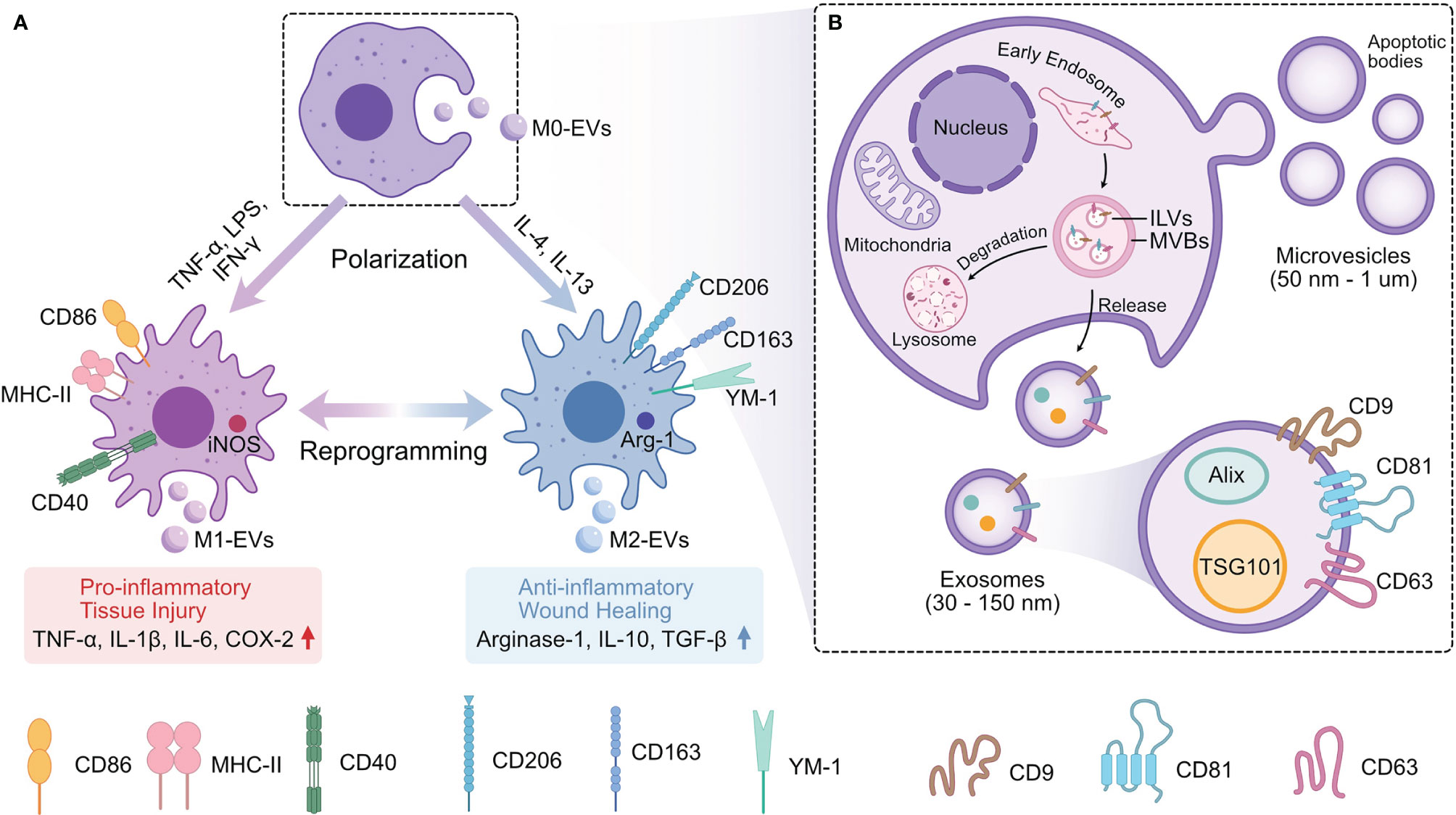
Figure 1 The Relation of Mφ and Mφ-EVs. (A) EVs derived from different phenotypes of Mφ (M0-EVs, M1-EVs, and M2-EVs) have different properties and biological functions. (B) EVs are released from Mφ by either secreting microvesicles and apoptotic bodies extracellularly through plasma membrane fusion or releasing exosomes by endosomal pathway. Mφ, Macrophages; EVs, Extracellular vesicles; Mφ-EVs, Macrophage-derived exosomes; TNF-α, Tumor necrosis factor alpha; LPS, Bacterial lipopolysaccharide; IFN-γ, Interferon gamma; IL-1β,4,6,10,13, Interleukin-1beta,4,6,10,13; CD40,86, 163,206, Surface markers in Mφ; MHC-I, Major histocompatibility complex II; iNOS, Inducible nitric oxide synthase; YM-1, Chitinase-like protein; Arg-1, Arginase-1; ILVs, Intraluminal vesicles; MVBs, Multivesicular bodies; Alix, Apoptosis-linked gene 2-interacting protein X; TSG101, Tumor susceptibility gene 101; CD9, 63,81, Tetraspanins; COX-2, Cyclooxygenase-2; TGF-β, Transforming growth factor-beta.
However, there is no consensus on the specific markers of EVs subtypes according to the MISEV2018 (23). In this review, we primarily focus on EVs 150 nm or less in size.
Molecular Components of Mφ-EVs
The RNA molecules enclosed in Mφ-EVs comprise mainly mRNA (intact mRNA and mRNA fragments) (31, 43), miRNA (60), long non-coding RNA (46), and tRNA (1). Lee et al. obtained EVs from Mφ treated with Shiga toxin 2a toxoids and found that they express higher levels of mRNAs encoding the pro-inflammatory cytokines IL-1β and IL-8, thereby exacerbating inflammation (9). Zhu et al. evaluated the features of Mφ-EVs and EVs derived from tumor-associated Mφ (TAM-EVs) (72) and found that different RNA processing proteins resulted in different RNA profiles. These results indicate that EVs that transport mRNA may be internalized and translated. MiRNA incorporated in EVs could circulate in the blood without degradation from blood RNAse activity (3). In addition, Mφ-EV miRNAs participate in the immune response (51), induce mesenchymal stem cell differentiation (57), regulate the tumor-associated microenvironment (73, 74), and mediate cell proliferation and migration (52). However, to date, few studies have investigated the presence of DNA in EVs (3).
In recent years, proteomic studies have provided new insights into the protein components of Mφ-EVs. Yao et al. reported a number of differentially expressed proteins in IFN-α-treated Mφ-EVs (35), including 74 upregulated and 20 downregulated proteins involved in antiviral-related pathways. In another study, 22 upregulated proteins in LPS-induced Mφ-EVs activated the NOD-like receptor signaling pathway and the NLRP3 inflammasome in patients with acute liver injury (25). Huang et al. screened proteins from silica-exposed Mφ-EVs and identified 291 differentially expressed proteins; the SPP1 protein was found to play a critical role in the response to silicosis (36). These findings emphasize that the biological functions of EV proteins vary under different conditions.
EVs can also deliver soluble mediators such as cytokines and enzymes. Haque et al. investigated the role of Mφ-EVs exposed to cigarette smoke condensate in HIV patients and found an association with catalase upregulation (29). In colitis patients, EVs transmitting CC chemokine 1 directly interact with CCR8 to alleviate colon damage and relieve inflammation (12).
Lipids also play an important role in the functions of Mφ-EVs. Kadiu et al. applied lipidomic analysis to explore how Mφ-EVs facilitate HIV-1 infection (75) and found that MVs and exosomes derived from Mφ have unique lipid profiles. Specifically, viral membranes enriched with lipids, such as glycerophosphoserine, sphingomyelin, and dihydrosphingomyelin, were readily detected in the MV fraction; by contrast, phosphatidylethanolamine/ceramide was identified only in the exosome population (75). However, the complete lipid profiles of Mφ-EVs are poorly understood and require further study.
Biogenesis of Mφ-EVs
The mechanisms of microvesicle biogenesis are associated with outward budding and fission of the plasma membrane. Apoptotic bodies are released as blebs in cells undergoing apoptosis (3). The biogenesis of exosomes is a complex and dynamic process. Invagination of the plasma membrane initiates the first step of exosome biogenesis and produces endocytic vesicles. The fusion of multiple endocytic vesicles leads to the formation of early endosomes (EEs). Many intercellular cargoes are encapsulated in such EEs in a clathrin- or caveolin-dependent or independent manner (76, 77). With the assistance of the Golgi complex, EEs invaginate and mature into late endosomes known as multivesicular bodies (MVBs) (2). Inward membrane budding results in the formation of intraluminal vesicles (ILVs), which are housed in MVBs (78). MVBs show two types of reversion: they may fuse with the plasma membrane and release ILVs into the extracellular space as exosomes, or they may fuse with lysosomes or autophagosomes and ultimately lead to degradation (4). Endosomal sorting complex required for transport (ESCRT) is the best-described mechanism underlying MVB formation and protein sorting in MVBs (79). ESCRT includes four different protein complexes, ESCRT-0, -I, -II, -III (80). The ESCRT-0, -I and ESCRT-II complexes form a recognition domain in the endosomal membrane that recognizes and ubiquitinates membrane proteins. The ESCRT-III complex is responsible for membrane budding and the release of ILVs (81). Other critical players include Sytenin-1, TSG101, ALIX, Rab GTPases, Pmel17, and tetraspanins (4). In addition to proteins and ceramides, lipids such as sphingomyelinases are also involved in the biogenesis of exosomes (82).
Furthermore, stimulation could influence Mφ-EV cargo sorting or ultimate release in the biogenesis of Mφ-EVs. Mφ exposed to irradiated apoptotic cancer cells activate peroxisome proliferator-activated receptor gamma (PPARγ) and increase the expression of phosphatase and tensin homolog (PTEN) in Mφ-EVs (83). Similarly, Mφ exposed to extracellular adenosine triphosphate (ATP) activate the P2X7 signaling pathway and increase calpain activity, ultimately leading to IL-1β expression and loading of unconventional proteins into Mφ-EVs (84, 85). Interestingly, the more released of Mφ-EVs would result from lipopolysaccharide (LPS) stimulation, and the mechanism is related to upregulation of Rab27a and Rab27b, while it is inhibited by IL-25 (86).
Relationship Between Mφ and Mφ-EVs
The description of the Mφ phenotype is widely accepted: classically activated or inflammatory M1Mφ are induced by IFN-γ, TNF-α or bacterial LPS, whereas alternatively activated or anti-inflammatory M2Mφ are polarized by IL-4 and IL-13 (87, 88). Plasticity is an important property of Mφ (89). Cytokines in the microenvironment can alter the phenotype of Mφ (90). Different phenotypes have different functions; for example, M1Mφ secrete higher levels of pro-inflammatory cytokines, exerting potent antimicrobial and antitumor activities that impair tissue regeneration and wound healing (91–93). By contrast, M2Mφ have anti-inflammatory effects that remove debris and apoptotic cells, promote angiogenesis, and facilitate fibrosis, tissue repair, and wound healing (94–96).
There are three major phenotypes of Mφ-EVs: unpolarized M0Mφ-derived EVs (M0-EVs), M1Mφ-derived EVs (M1-EVs), and M2Mφ-derived EVs (M2-EVs) (97). Their biological functions vary depending on the parental cell properties. For instance, in the pathogenesis of atherosclerosis (AS), M1-EVs containing a high level of miR-155 suppress the proliferation of fibroblasts and promote the development of AS (98), while M2-EVs deliver miR-1271-5p to suppress cardiomyocyte apoptosis and perform cardiac repair (67). Moradi-Chaleshtori et al. reported that M1-EVs polarize Mφ from the M2 to M1 phenotype and have antitumor effects by carrying miR-130 and miR-33 (69). M2-EVs promote cell invasion in breast cancer by transporting miR-223 to target the Mef2c/β-catenin pathway (99). Furthermore, each EV phenotype has polarization-specific control in the bone repair process. M0-EVs and M2-EVs enhance bone regeneration, while M1-EVs inhibit bone repair (100).
Immunomodulatory Effects of Mφ-EVs in Chronic Inflammatory Disease
Inflammation is the body’s defense response to stimuli such as infection or injury, and it can be divided into acute and chronic phases. Acute inflammation is rapid in onset and short in duration, mainly characterized by exudative lesions. Chronic inflammation can lead to pathological changes in tissues and organs, which in turn cause diverse chronic diseases, including diabetes, cancer, cardiovascular disease, respiratory disease, and gastrointestinal disease (101, 102).
Chronic diseases are driven by pathological inflammation, eventually leading to tissue damage (103). In short, disorders resulting from inflammation-related pathways are the primary mechanism leading to chronic disease.
EVs play a vital role in maintaining tissue homeostasis and regulating disease progression as another mode of cellular interaction (104, 105). Numerous studies have elucidated the impact of Mφ-EVs on chronic inflammation and disease. EVs derived from different Mφ have unique effects under various pathological conditions (106, 107) (Figure 2).
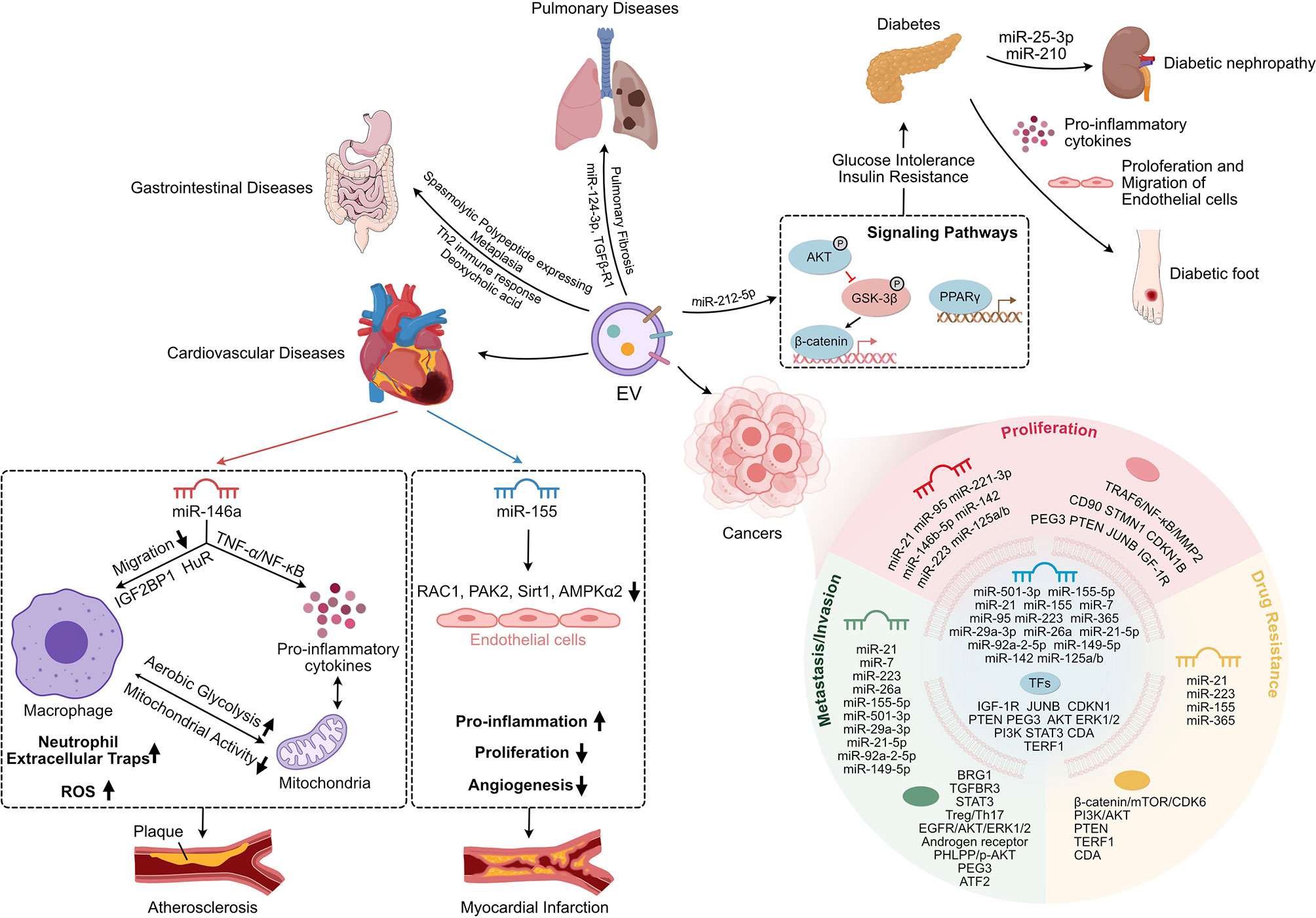
Figure 2 The Immuno-modulation Effect of Mφ-EVs in chronic inflammatory diseases. Mφ-EVs regulate the immune response and cell proliferation and migration and are involved in signaling pathways in the development of chronic inflammatory disease. Mφ-EVs, Macrophage-derived extracellular vesicles; Th2, CD4+ T helper 2 lymphocytes; AKT, Protein kinase B; GSK-3β, Glycogen synthase kinase-3beta; PPARγ, Peroxisome proliferator-activated receptor gamma; TNF-α, Tumor necrosis Factor alpha; NF-κB, Nuclear factor kappa-light-chain-enhancer of activated B cells; IGF2BP1, Insulin-like growth factor 2 mRNA-binding protein 1; HuR, Human antigen R; ROS, Reactive oxygen species; RAC1, RAS-related C3 botulinus toxin substrate 1; PAK2, p21-activated kinase 2; Sirt1, Sirtuin 1; AMPKα2, Adenosine monophosphate-activated protein kinas alpha 2; TRAF6, TNF receptor associated factor 6; MMP2, Matrix metalloproteinase 2; CD90, Cluster of differentiation 90; STMN1, Stathmin 1; CDKN1B, Cyclin-dependent kinase inhibitor 1B; PEG3, Paternally expressed gene 3; PTEN, Phosphatase and tensin homolog; JUNB, AP-1 transcription factor; IGF-1R, Insulin-like growth factor receptor; mTOR, Mammalian target of rapamycin; CDK6, Cyclin-dependent kinase 6; PI3K, Phosphatidylinositol-3-kinase; TERF1, Telomeric repeat binding factor 1; CDA, Cytidine deaminase; BRG1, Brahma-related gene 1; TGFBR3, TGF-beta type III receptor; STAT3, Signal Transducers and Activators of Transcription 3; Treg, regulatory T lymphocytes; Th17, IL-17-producing CD4+ T lymphocytes; EGFR, Epidermal growth factor receptor; ERK1/2, Extracellular signal-regulated kinase 1/2; PHLPP, PH domain leucine-rich-protein phosphatase; p-AKT, Phosphorylated-Akt; ATF2, Activating transcription factor 2.
Cardiovascular Disease
AS plays a prominent role in coronary heart disease, cerebral infarction, and peripheral vascular disease (108). It is a multifactorial disease, and its exact pathogenesis has not been elucidated. Hypertension, hyperlipidemia, obesity, smoking, and diabetes are all risk factors for its development (109). AS is characterized by lesions in affected arteries starting from the intima, with lesions involving mainly large and medium arteries. It can lead to deposits of lipids and complex sugars, hemorrhage, thrombus formation, and many other conditions that eventually thicken and stiffen the arterial wall and narrow the vessel lumen. AS plaques are composed primarily of immune, foam, and inflamed smooth muscle cells (110).
AS is an inflammatory response caused by the retention of cholesterol-rich, B-type lipoproteins in susceptible areas of medium and large arteries (111). Dysregulation of the balance between cellular and systemic cholesterol promotes the deposition of such lipoproteins in the arterial wall (109).
The development of AS is closely associated with endothelial cell damage, vascular inflammation, and massive accumulation of Mφ. The imbalance between Mφ recruited to plaques and Mφ migration from plaques to regional lymph nodes causes deposition of lipid-laden Mφ in the arterial wall, further promoting the inflammatory progression of AS. Thus, Mφ migration regulates the development of AS (108, 112). EVs mediate intercellular communication in atherosclerotic plaques (113). Furthermore, activated monocytes or Mφ-EVs can propagate inflammatory signals and modulate AS development via various pathways (114–116). Nguyen et al. reported that EVs secreted from Mφ loaded with oxidized low density lipoprotein are taken up by naive Mφ and inhibit Mφ migration in vitro and in vivo (116). In addition, Mφ-EVs can block the migration of Mφ to the chemokine stimulator CCL2, and EVs transport miR-146a, which suppresses the expression of genes in Mφ related to cell migration, such as HuR and IGF2BP1 (116). Mφ may also promote the progression of AS via activation of the NF-κB pathway. The modulatory effect of Mφ-EVs on AS is further evidenced by their ability to regulate hematopoietic stem cells differentiation and necrosis by suppressing the TNF-α/NF-κB signaling pathway (49). Furthermore, Zhu et al. isolated EVs from nicotine-treated Mφ and illustrated that they aggravate the development of AS by transporting the exosomal miR-21-3p target PTEN, which enhances vascular smooth muscle cell migration and proliferation (52).
Oxidative stress and inflammation are closely related, and they form a feed-forward loop that promotes the development of AS. During AS, Mφ produce significant levels of reactive oxygen species (ROS) via mitochondrial metabolism (109). M1Mφ enhance aerobic glycolysis and reduce mitochondrial activity. In contrast, M2Mφ mediate mitochondrial oxidative phosphorylation (117). Bouchareychas et al. isolated EVs from mouse bone marrow-derived Mφ treated with IL-4 and applied these EVs to naive BMDMs, which resulted in enhanced ATP production and cellular respiration. These results indicate that EVs can effectively regulate cellular reprogramming by improving energy metabolism (49). Mφ-derived EVs treated with superoxide dismutase 2, a target of oxidized low density lipoprotein, showed increased ROS production and the release of neutrophil extracellular traps via miR-146a (53).
Mφ modulation plays an essential role in immunomodulatory processes during cardiac repair and in remodeling post-myocardial infarction (118). Hypoxia/serum deprivation-induced M1-EVs transport miR-222, which promotes apoptosis and inhibits viability in bone marrow mesenchymal stem cells by targeting B-cell lymphoma-2 (62). miR-155 is predominantly expressed in Mφ and cardiac fibroblasts and is one of the most abundant miRNAs in M1-EVs (119). The expression of miR-155 is upregulated in Mφ-EVs from the hearts of mice after acute myocardial infarction (AMI). EVs enriched with miR-155 inhibit the proliferation of fibroblasts and promote the inflammatory response; a deficiency of miR-155 decreases the incidence of cardiac rupture after AMI and improves cardiac function (98). Previous studies have observed angiogenesis-inhibiting effects of M1Mφ, but the mechanism of action is not entirely understood (120). M1-EVs transfer miR-155 to endothelial cells, reducing their angiogenic ability by downregulating miR-155 target genes including RAC1, PAK2, Sirt1, and AMPKα2 (48). Thus, inhibition of miR-155 expression may be a novel method for the clinical treatment of MI. By contrast, M2-EVs transport miR-1271-5p, which suppresses apoptosis in cardiomyocytes and performs a cardiac repair function in AMI by targeting SOX6 (67).
Generally, ischemia–reperfusion is applied to restore coronary artery blood flow and relieve disease progression. However, numerous studies have demonstrated that ischemia–reperfusion injury (IRI) can lead to cardiac dysfunction due to calcium overload and overproduction of free radicals such as ROS (121). Induction of hypoxia–reoxygenation is the main method for establishing IRI in animal models (64). Wang et al. demonstrated that Mφ subjected to hypoxia–reoxygenation are polarized toward M1Mφ and derived EV miR-29a to promote cardiomyocyte pyroptosis by targeting myeloid cell leukemia-1 (64). M2-EVs alleviate cardiac dysregulation and Ca2+ overload, which relieves IRI. miR-148a within M2-EVs suppresses the expression of thioredoxin-interacting protein and inactivates the TLR4/NF-κB/NLRP3 signaling pathway (63).
Diabetes Mellitus
The incidence of type 2 diabetes has risen sharply over the past few decades, with a global prevalence of over 300 million people (122). Type 2 diabetes accounts for approximately 95% of diabetes cases and is characterized by hyperglycemia due to insulin resistance (IR) and relative insulin deficiency (123, 124). Obesity is a risk factor for type 2 diabetes. Moreover, chronic low-grade inflammation is a leading cause of obesity-induced IR (125).
The massive accumulation of pro-inflammatory Mφ in adipose tissue and the liver is a distinguishing feature of obesity-induced chronic inflammation in tissues (126). Mφ could be the ultimate effector cells that secrete the major cytokines responsible for IR. Mφ in normal adipose tissue express CD206 receptors and release Arg-1, but those in inflamed tissues are polarized toward the M1 phenotype (127). In a previous study, administering EVs derived from adipose tissue Mφ (ATM-EVs) from obese mice to lean mice caused glucose intolerance and IR, while administering lean mice ATM-EVs to obese mice improved glucose tolerance and insulin sensitivity (128).
Chronic low-grade tissue inflammation is the main cause of IR, leading to islet β cell failure. Qian et al. analyzed the effects on β cells of M1-EVs and EVs isolated from islet-resident Mφ from mice fed a high-fat diet (129) and found that miR-212-5p restricted insulin secretion by targeting the SIRT2 gene and regulating the Akt/GSK-3β/β-catenin pathway (129). Moreover, miR-155, which is overexpressed in obese ATM-EVs, suppresses the expression of PPARγ and impairs the inhibitory effects of insulin on glucose production (128). Similarly, miR-29a within ATM-EVs promotes obesity-induced IR by directly targeting PPARγ (65).
Many complications can occur during the late stages of diabetes. The persistence of diabetic inflammation activates inflammatory cells, which secrete inflammation-associated cytokines (130). A common complication of diabetes is difficulty healing. Mφ-EVs show a remarkable decrease in the release of pro-inflammatory cytokines, enhancing the proliferation and migration of endothelial cells and accelerating wound healing via their anti-inflammatory effects (131). Similar research has shown that M1-EVs can regulate Mφ phenotypic reprogramming, repolarizing M1Mφ to M2Mφ, which in turn promotes wound healing (132). Bone homeostasis is also disturbed by diabetes mellitus. Zhang et al. isolated EVs from diabetic bone marrow-derived Mφ and found that they transport miR-144-5p, which inhibits osteogenesis differentiation by targeting Smad1 and suppressing facture repair in vivo (58). Diabetic nephropathy is a peripheral small artery occlusive disease caused by diabetic neuropathy and lower extremity vasculopathy. M2-EVs that transport miR-25-3p may alleviate podocyte injury induced by high glucose levels by activating autophagy of the cells through suppression of DUSP1 expression (133). Zhu et al. found that Mφ-derived EVs treated with high levels of glucose might activate glomerular mesangial cells through the TGF-β1/Smad3 pathway, promote proliferation, and induce the production of fibrotic and inflammatory factors (31). Similarly, high-glucose-treated Mφ-derived EVs induce overexpression of inflammatory cytokines and activate NF-κB p65 signaling pathways (134). ATM-EVs contain high levels of miR-210, and miR-210 in ATM-EVs has been shown to regulate glucose uptake and mitochondrial chain complex IV activity by targeting NDUFA4 gene expression, which promotes the pathogenesis of diabetes (59).
These findings highlight the importance of Mφ-EVs in adipose tissue and suggest that the contents and functions of ATM-EVs vary with the ATM phenotype.
Cancer
Cancer is the leading cause of death worldwide (135). In 2012, approximately 14.1 million new cancer cases and 8.2 million cancer deaths were recorded worldwide (135). Furthermore, with the aging population, the incidence and mortality rate of cancer have rapidly been increasing, imposing a significant burden on society. Inflammation plays a crucial role in the development of cancer (136). The “seed and soil” theory proposed in 1889 (137) compares cancer cells to seeds and the human microenvironment to soil and postulates that whether a tumor metastasizes depends on whether the soil meets the growth conditions of the seed (137). In recent years, more studies have focused on the regulatory roles of active immune cells such as Mφ, neutrophils, and mast cells in the tumor microenvironment (TME) (138, 139). Among such cells, TAMs play the most critical role in the TME (140, 141).
Mφ-EVs have diverse effects on the TME under various pathological conditions (4, 142, 143). For example, EVs transport apolipoproteins from TAMs to gastric cancer cells to promote cell migration (144), the expression of matrix metalloproteinase-2, and the pathogenesis of abdominal aortic aneurysms by activating the JNK and p38 signaling pathways (145).
EVs mediate intercellular communication in the TME via miRNA-induced epigenetic modifications in recipient cells. EVs miRNAs can regulate tumor cell migration, invasion, and drug resistance via various mechanisms, which in turn affect tumorigenesis (Table 2).
In recent years, proteomics has dramatically facilitated the study of proteomic profiles of EVs, and research on Mφ-EVs has provided new insights into how the TME is regulated (161, 162). A thorough comparative proteomic analysis of EVs revealed that TAM-derived exosomal proteins are responsible mainly for RNA processing and proteolytic functions and determined that recipient cells have an improved capacity to degrade denatured or misfolded proteins after uptake of TAM-EVs, which enhances their survival in the TME (72). Furthermore, TAM-EVs have molecular profiles associated with Th1/M1 polarization profiles; they enhance inflammation and immune responses and promote the proliferation and activation of T cells ex vivo. Thus, TAM-EVs are potent stimulators of antitumor immunity (163). They also activate the matrix metalloproteinase‐9 signaling pathway to promote hepatocellular carcinoma tumor migration by mediating the intercellular transfer of αMβ2 (CD11b/CD18) (24).
Recently, it was found that EVs contain damaged DNA from the nucleus and mitochondria, regulating tumor immunity via paracrine and activated cytoplasmic DNA sensor pathways and in specific immune cell subpopulations (164). After chemotherapy, exposing Mφ to apoptotic breast cancer cells causes higher levels of IL-6 to be released and delivered to cancer cells via increased phosphorylation of STAT3, promoting the proliferation and metastasis of the cells (8). Furthermore, Mφ-EVs may synthesize proteins such as thromboxane and thromboxane B2 (163). However, it is unclear whether they contain functional endogenous DNA. In oral squamous cell carcinoma, THP-1-derived EVs and native human Mφ-derived EVs have been shown to activate the AKT/GSK-3β signaling pathway, reducing the proliferative effects of 5-FU and cis−diamminedichloroplatinum (CDDP) and the apoptosis of OSC-4 cells (165).
Metabolic reprogramming, an essential hallmark of malignancy, is regulated by the microenvironment. TAM-derived EVs enhance aerobic metastasis and the anti-apoptotic ability of carcinoma cells by transporting a myeloid-specific long non-coding RNA and HIF-1α-stabilizing long non-coding RNA (HISLA) (166). HILSA inhibits the hydroxylation and degradation of HIF-1α, whereas carboxylic acid secreted from growing glycolytic cells upregulates HISLA in TAMs, constituting a feed-forward loop between TAMs and growing cells. Thus, HISLA inhibits metastasis and chemoresistance in carcinoma in vivo (166). This suggests that EVs-mediated metabolic reprogramming plays an important role in the intercellular communication between immune and tumor cells. Azambuja et al. proposed that glioblastoma-derived EVs reprogram M1Mφ into TAMs and promote the pro-tumor functions of M2Mφ, while these GEV-reprogrammed TAM-EVs promote glioblastoma cell migration and proliferation (27).
In summary, because Mφ are sensitive to microenvironmental stimuli, the composition of their secreted EVs in different disease models change as the Mφ themselves are altered.
Pulmonary Disease
Idiopathic pulmonary fibrosis (IPF) is an intermittent and chronic fibrotic lung disease associated with inflammatory immune damage, caused primarily by chronic alveolar epithelial injury and dysregulated wound healing due to abnormal proliferation of fibroblasts (167). The expression of miR-142-3p is markedly increased in the sputum and plasma of IPF patients, and Mφ-EVs reduce the expression of TGFβ receptor 1 by transporting miR-142-3p, suppressing the progression of pulmonary fibrosis (50). Antifibrotic miRNA delivery in the lung can effectively prevent pulmonary fibrosis and provide new therapeutic avenues for the treatment of IPF. In addition, M2-EVs may transport miR-328, facilitating the proliferation of pulmonary interstitial fibroblasts via family with sequence similarity 13, member A (61).
Another pulmonary fibrosis-related disease is silicosis, which is caused by long-term inhalation of large amounts of free silica dust and is characterized by extensive nodular fibrosis of the lungs (168). EVs have been isolated from silica-exposed Mφ and found to induce the proliferation of myofibroblasts and fibroblasts and increase their expression levels of SPP1 (36). They also induce the overproduction of proinflammatory cytokines and promote myofibroblast activation in an endoplasmic reticulum stress-dependent manner (30).
In acute lung injury, Mφ-EVs in alveolar lavage fluid release various pro-inflammatory factors mainly during the early stages of damage; this activates neutrophils to produce IL-10, which might be responsible for polarizing Mφ to M2c, leading to post-acute lung injury fibrosis (169).
Asthma, a chronic respiratory disease, is characterized by inflammation and hyperresponsiveness of the airways (170). M2-EVs deliver miR-370 to reduce cell apoptosis and relieve inflammation by suppressing the FGF1/MAPK/STAT1 axis (70). M2-EVs have also been found to upregulate AGAP2-AS1 and NOTCH2 expression and downregulate miR-296 expression to strengthen the radioresistance of lung cancer cells (44).
Gastrointestinal Disease
Chronic inflammation influences the development of spasmolytic polypeptide-expressing metaplasia (171), and deoxycholic acid (DCA) enhances the expression of enteral metaplasia markers (172). Xu et al. cocultured mouse stomachic organoids with DCA treated macrophage-derived EVs(DCA-Mφ-EVs), and the data revealed that the expression of SPEM marker proteins TFF2, GSII, SPEM-related cistron Wfdc2, Olfm4 and Cftr were considerably inflated when 72h cocultured (172). In addition, miR-30a-5p enriched in Mφ-EVs derived from DCA promotes intestinal metaplasia and inhibits the proliferation of human gastric cancer cells by targeting forkhead box D1 (54). These results suggest that Mφ-EVs may mediate intercellular communication in the DCA microenvironment and promote the progression of spasmolytic polypeptide-expressing metaplasia, providing a new target for treating gastric intestinal metaplasia.
Chronic intestinal inflammation may eventually lead to inflammatory bowel disease (173). To assess the effects of Mφ-derived EVs on inflammatory bowel disease, Yang et al. isolated EVs from the M2aMφ, M2bMφ, and M2cMφ phenotypes and established a dextran sulfate sodium-induced colitis model in mice (12). Treating the mice with M2-EVs improved colon length. Furthermore, compared with EVs derived from M2aMφ and M2cMφ, M2bMφ-derived EVs were more effective. These EVs may interact with CCR8 by releasing the CCL1 chemokine, thereby increasing the expression of IL-4 and number of regulatory T cells to promote the Th2 immune response (12).
Potential Therapeutic Strategies
EVs are considered a promising tool for immunotherapy, drug delivery, and targeted therapy. Furthermore, some strategies were proposed to strengthen the therapeutic capabilities and broaden the applications of EVs. Recent applications are discussed below (Table 3).
Mφ-EVs as a Drug Candidate
As messengers carrying genes, proteins, and other biomolecules, EVs mediate communication in cells and have therapeutic functions. Kang et al. (25) applied M0-EVs, M1-EVs, and M2-EVs to rat calvaria defects; M2-EVs carrying miR-378a increased the expression of the mesenchymal stem cell osteoinductive genes BMP2 and BMP9 in the bone repair process to promote bone regeneration (100). Furthermore, proteomic profiling analysis of the protein composition of LPS-treated Mφ-EVs (L-Mφ-EVs) revealed that among 341 upregulated proteins in L-Mφ-EVs, 22 are involved in the NOD-like receptor signaling pathway. After L-Mφ-EVs were taken up by hepatocytes, NLRP3 was activated, which promoted acute liver injury (25). In ischemic stroke, microglia are converted into M1 phenotypes that release pro-inflammatory mediators, promoting neuronal apoptosis and brain injury (174). L-Mφ-EVs suppress inflammation, enhance microglial M2 polarization (which induces neuroprotection), and reduce the brain infarct volume in vivo after ischemic stroke (174). The miRNAs in Mφ-EVs are involved in different pathways to regulate the development of disease(Qian et al.; 7, 133). In chronic liver disease, L-Mφ-EVs have been shown to promote the proliferation and activation of hepatic stellate cells by enriching miR-103-3p and targeting Krüppel-like factor 4 (55). IL-4-treated Mφ-EVs transported anti-inflammatory miR-99a/146b/378a to inhibit inflammation by targeting NF-κB and TNF-α signaling, leading to delayed development of AS (49).
In addition, Mφ-EVs may have therapeutic functions via Mφ reprogramming. For example, M2-EVs promote wound healing by enhancing proliferation, angiogenesis, and collagen deposition (132), which suggests that Mφ phenotype reprogramming could provide valuable therapeutic options for the treatment of inflammation-related diseases. M1-EVs can also potentially be used to repolarize M2 to M1Mφ that secrete pro-inflammatory cytokines, have antitumor effects, and decrease tumor growth (175). Similarly, M1-EVs transport miR-130 and miR-33 to exert antitumor effects in breast cancer by polarizing Mφ from the M2 to M1 phenotype (69).
Mφ-EVs as Drug-Delivery Systems
Many nanocarrier delivery systems have been designed to improve the efficacy of drugs, and EVs have the following advantages compared with other nanocarrier delivery systems: they transport a variety of endogenous biomolecules, and they are biocompatible, naturally targeted, and small enough to escape the clearance effect of the mononuclear phagocyte system (191, 192). Kanchanapally et al. (176) obtained EVs from different cells, including pancreatic cancer cells, pancreatic stellate cells, and Mφ loaded with doxorubicin (DOX), and compared their antitumor effects. Mφ-EVs loaded with DOX showed the highest antitumor efficiency followed by pancreatic stellate and pancreatic cancer cells. Zhang et al. isolated EVs from mononuclear M1Mφ and M2Mφ from umbilical cord blood and loaded them with CDDP; compared with the M2-EVs, M1-EVs showed increased cytotoxicity in drug-resistant A2780/DDP cells, suggesting that M1-EVs are a potential drug carrier in drug-resistant microenvironments (177).
The inability to cross the blood brain barrier (BBB) limits the application of 98% of therapeutic agents used for the treatment of CNS-related disorders (193). Recently, Yuan et al. (40) discovered that Mφ-EVs can cross the BBB and move into brain microvessel endothelial cells via integrin white corpuscle function-associated matter 1, living thing adhesion molecule 1, and carbohydrate-binding C-type glycoprotein receptors. That study further confirmed that intravenously injected Mφ-EVs crossed the BBB and transported brain-derived neurotrophic factor to the brain (40). A novel EVs-based formulation for catalase delivery in Parkinson’s disease patients was found to have neuroprotective effects against oxidative stress by inactivating ROS, decreasing brain inflammation, and increasing neuronal survival in vivo (180). That study provided a new theoretical basis for the development of other EVs-based drug-delivery systems for the treatment of CNS diseases, as well as an experimental basis for the in-depth study of the mechanisms involved in EV passage through the BBB. Silibinin, an antioxidant with poor brain targeting, has been applied to improve behavior and cognition in Parkinson’s patients. Huo et al. loaded Mφ-EVs with silibinin to improve its targeting capacity and released the silibinin to suppress astrocyte activation and relieve neuronal damage after crossing the BBB (186). Edaravone (Edv) delays neuronal death caused by acute cerebral infarction. Li et al. (13) prepared Mφ-EVs loaded with Edv, applied them to a rat model with permanent middle cerebral artery occlusion, and found that they notably improved bioavailability and prolonged the half-life of Edv. In addition, using these Edv-loaded Mφ-EVs, it was easier to target Edv to the ischemic side, and the treatment decreased neuronal death and promoted microglia M2 polarization in vivo.
Spinal cord injury severely damages the CNS. Gao et al. (183) developed an M2-EV-loaded berberine drug-delivery system that effectively prolonged the duration of berberine and improved its targeting capacity. In addition, it had anti-inflammatory and anti-apoptotic effects by repolarizing Mφ from the M1 to M2 phenotype. Mφ-EVs loaded with baicalin have been found to ameliorate the solubility and brain-targeting ability of baicalin, leading to significant neuroprotection in patients with ischemic stroke (181).
Application of Engineered Mφ-EVs
Through genetic and chemical modifications, engineered EVs can enhance EVs targeting and therapeutic effects in cancer treatment (194). For example, Mφ loaded with paclitaxel show significant loading capacity, sustainable drug release, a profound capacity for accumulation in resistant cancer cells, and high cytotoxicity (182). Mφ loaded with paclitaxel and aminoethylbenzamide-polyethylene glycol readily accumulate in cancer cells and have a higher therapeutic effect in vivo compared with non-vectorized Mφ loaded with paclitaxel (184). M1-EVs tagged with anti-CD47 and anti-SIRPα using a pH-sensitive linker effectively target tumors, block SIRPα and CD47, and reprogram M2Mφ to M1Mφ, thereby exerting antitumor functions (185). Gong et al. stimulated THP-1 cells with phorbol 12-myristate 13-acetate to generate target-specific A15 EVs, packing Dox into them to codeliver cholesterol-modified miRNAs and chemotherapeutic drugs into triple-negative breast cancer cells; the system showed specific and robust targeting capabilities and suppressed tumor growth in vivo (187). To improve triple-negative breast cancer targetability, Li et al. (188) modified the surface of Mφ-EVs with a peptide to target mesenchymal–epithelial transition factor and developed a Mφ-EV-coated poly (lactic-co-glycolic acid) nanoplatform, which improved the efficiency of cellular uptake and the antitumor effects of DOX. Wu et al. (189) electroporated M2-EVs with FDA-approved hexyl 5-aminolevulinate hydrochloride, which produced anti-inflammatory carbon monoxide and bilirubin and further enhanced the anti-inflammatory effect by binding to surface-expressed chemokine receptors and releasing anti-inflammatory cytokines; they also relieved AS.
Modification of EVs may enhance their release of anticancer drugs and their antitumor effects by releasing pro-inflammatory Th1 cytokines. For example, M1-EV nano-formulation-loaded paclitaxel creates a pro-inflammatory environment that improves antitumor activity via the caspase-3 signaling pathway and exhibits antitumor effects in vivo (190).
In summary, Mφ-EVs have similar targeting and regulatory abilities as those of Mφ. Thus, the advantages of Mφ-EVs in terms of their nanometer size, cellular targeting, and low immunogenicity make them excellent candidates for next-generation drug-delivery systems.
Conclusion and Perspectives
There are some unresolved issues regarding Mφ-EVs, such as the efficiency of their isolation and purification (195). To address this, Jang et al. (196) created bioinspired exosome-mimetic nanovesicles by breaking down monocytes or Mφ and found that they had similar functional properties as EVs, with 100-fold better isolation and purification efficiency; they also induced TNF-α-stimulated endothelial cell death and showed antitumor activity in vivo. Choo et al. (175) prepared exosome-mimetic nanovesicles derived from M1Mφ, which effectively repolarized M2Mφ to M1Mφ and promoted the antitumor efficacy of programmed death ligand 1. EVs have been characterized based on protein content (23, 197). However, the molecular hallmarks specifically distinguishing each EV subtype remain unclear (23). This issue should be further explored and addressed.
Overall, studies have shown that Mφ-EV-based immuno-modulation strategies are effective treatments for various pathological conditions. In the future, more studies are needed to further investigate Mφ-EV-related mechanisms and develop Mφ-EVs based on therapeutic strategies.
Author Contributions
QY and YHZ initiated the project, made suggestions and revised the article. YX searched the database and wrote the first draft of the manuscript. XS, YD, MW, and YMZ revised and finalized the manuscript. All authors contributed to the article and approved the submitted version.
Funding
This work was supported by National Natural Science Foundation of China (82072435, 81871782), Tianjin Science Fund for Distinguished Young Scholars (18JCJQJC47900), and Tianjin Science and Technology Program (20JCYBJC01440).
Conflict of Interest
The authors declare that the research was conducted in the absence of any commercial or financial relationships that could be construed as a potential conflict of interest.
Publisher’s Note
All claims expressed in this article are solely those of the authors and do not necessarily represent those of their affiliated organizations, or those of the publisher, the editors and the reviewers. Any product that may be evaluated in this article, or claim that may be made by its manufacturer, is not guaranteed or endorsed by the publisher.
References
1. Liu J, Wu F, Zhou H. Macrophage-Derived Exosomes in Cancers: Biogenesis, Functions and Therapeutic Applications. Immunol Lett (2020) 227:102–8. doi: 10.1016/j.imlet.2020.08.003
2. Wu P, Zhang B, Ocansey DKW, Xu W, Qian H. Extracellular Vesicles: A Bright Star of Nanomedicine. Biomaterials (2021) 269:120467. doi: 10.1016/j.biomaterials.2020.120467
3. Yánez-Mo M, Siljander PR, Andreu Z, Zavec AB, Borrà s FE, Buzas EI, et al. Biological Properties of Extracellular Vesicles and Their Physiological Functions. J Extracell Vesicles (2015) 4:27066. doi: 10.3402/jev.v4.27066
4. Kalluri R, LeBleu VS. The Biology, Function, and Biomedical Applications of Exosomes. Science (2020) 367(6478):eaau6977. doi: 10.1126/science.aau6977
5. Pan BT, Teng K, Wu C, Adam M, Johnstone RM. Electron Microscopic Evidence for Externalization of the Transferrin Receptor in Vesicular Form in Sheep Reticulocytes. J Cell Biol (1985) 101(3):942–8. doi: 10.1083/jcb.101.3.942
6. Liu Y, Wang Y, Lv Q, Li X. Exosomes: From Garbage Bins to Translational Medicine. Int J Pharm (2020) 583:119333. doi: 10.1016/j.ijpharm.2020.119333
7. Shi ZY, Yang XX, Malichewe C, Li YS, Guo XL. Exosomal microRNAs-Mediated Intercellular Communication and Exosome-Based Cancer Treatment. Int J Biol Macromol (2020) 158:530–41. doi: 10.1016/j.ijbiomac.2020.04.228
8. Yu X, Zhang Q, Zhang X, Han Q, Li H, Mao Y, et al. Exosomes From Macrophages Exposed to Apoptotic Breast Cancer Cells Promote Breast Cancer Proliferation and Metastasis. J Cancer (2019) 10(13):2892–906. doi: 10.7150/jca.31241
9. Lee KS, Lee J, Lee P, Kim CU, Kim DJ, Jeong YJ, et al. Exosomes Released From Shiga Toxin 2a-Treated Human Macrophages Modulate Inflammatory Responses and Induce Cell Death in Toxin Receptor Expressing Human Cells. Cell Microbiol (2020) 22(11):e13249. doi: 10.1111/cmi.13249
10. Zheng PM, Gao HJ, Li JM, Zhang P, Li G. [Effect of Exosome-Derived miR-223 From Macrophages on the Metastasis of Gastric Cancer Cells]. Zhonghua Yi Xue Za Zhi (2020) 100(22):1750–5. doi: 10.3760/cma.j.cn112137-20200425-01309
11. Lin J, Li J, Huang B, Liu J, Chen X, Chen XM, et al. Exosomes: Novel Biomarkers for Clinical Diagnosis. ScientificWorldJournal (2015) 2015:657086. doi: 10.1155/2015/657086
12. Yang R, Liao Y, Wang L, He P, Hu Y, Yuan D, et al. Exosomes Derived From M2b Macrophages Attenuate DSS-Induced Colitis. Front Immunol (2019) 10:2346. doi: 10.3389/fimmu.2019.02346
13. Li F, Zhao L, Shi Y, Liang J. Edaravone-Loaded Macrophage-Derived Exosomes Enhance Neuroprotection in the Rat Permanent Middle Cerebral Artery Occlusion Model of Stroke. Mol Pharm (2020) 17(9):3192–201. doi: 10.1021/acs.molpharmaceut.0c00245
14. Porcuna J, Menéndez-Gutiérrez MP, Ricote M. Molecular Control of Tissue-Resident Macrophage Identity by Nuclear Receptors. Curr Opin Pharmacol (2020) 53:27–34. doi: 10.1016/j.coph.2020.04.001
15. Orecchioni M, Ghosheh Y, Pramod AB, Ley K. Macrophage Polarization: Different Gene Signatures in M1(LPS+) vs. Classically and M2(LPS-) vs. Alternatively Activated Macrophages. Front Immunol (2019) 10:1084. doi: 10.3389/fimmu.2019.01084
16. Shapouri-Moghaddam A, Mohammadian S, Vazini H, Taghadosi M, Esmaeili SA, Mardani F, et al. Macrophage Plasticity, Polarization, and Function in Health and Disease. J Cell Physiol (2018) 233(9):6425–40. doi: 10.1002/jcp.26429
17. Du C, Bhatia M, Tang SC, Zhang M, Steiner T. Mediators of Inflammation: Inflammation in Cancer, Chronic Diseases, and Wound Healing. Mediators Inflamm (2015) 2015:570653. doi: 10.1155/2015/570653
18. Schottenfeld D, Beebe-Dimmer J. Chronic Inflammation: A Common and Important Factor in the Pathogenesis of Neoplasia. CA Cancer J Clin (2006) 56(2):69–83. doi: 10.3322/canjclin.56.2.69
19. Bauer UE, Briss PA, Goodman RA, Bowman BA. Prevention of Chronic Disease in the 21st Century: Elimination of the Leading Preventable Causes of Premature Death and Disability in the USA. Lancet (2014) 384(9937):45–52. doi: 10.1016/s0140-6736(14)60648-6
20. Hotamisligil GS. Inflammation and Metabolic Disorders. Nature (2006) 444(7121):860–7. doi: 10.1038/nature05485
21. Miyamoto T, Carrero JJ, Stenvinkel P. Inflammation as a Risk Factor and Target for Therapy in Chronic Kidney Disease. Curr Opin Nephrol Hypertens (2011) 20(6):662–8. doi: 10.1097/MNH.0b013e32834ad504
22. Scrivo R, Vasile M, Bartosiewicz I, Valesini G. Inflammation as "Common Soil" of the Multifactorial Diseases. Autoimmun Rev (2011) 10(7):369–74. doi: 10.1016/j.autrev.2010.12.006
23. Théry C, Witwer KW, Aikawa E, Alcaraz MJ, Anderson JD, Andriantsitohaina R, et al. Minimal Information for Studies of Extracellular Vesicles 2018 (MISEV2018): A Position Statement of the International Society for Extracellular Vesicles and Update of the MISEV2014 Guidelines. J Extracellular Vesicles (2018) 7(1):1535750–1535750. doi: 10.1080/20013078.2018.1535750
24. Wu J, Gao W, Tang Q, Yu Y, You W, Wu Z, et al. M2 Macrophage-Derived Exosomes Facilitate HCC Metastasis by Transferring α(M) β(2) Integrin to Tumor Cells. Hepatology (2021) 73(4):1365–80. doi: 10.1002/hep.31432
25. Wang G, Jin S, Ling X, Li Y, Hu Y, Zhang Y, et al. Proteomic Profiling of LPS-Induced Macrophage-Derived Exosomes Indicates Their Involvement in Acute Liver Injury. Proteomics (2019) 19(3):e1800274. doi: 10.1002/pmic.201800274
26. Zhang Y, Liu F, Yuan Y, Jin C, Chang C, Zhu Y, et al. Inflammasome-Derived Exosomes Activate NF-ΰB Signaling in Macrophages. J Proteome Res (2017) 16(1):170–8. doi: 10.1021/acs.jproteome.6b00599
27. Azambuja JH, Ludwig N, Yerneni SS, Braganhol E, Whiteside TL. Arginase-1+ Exosomes From Reprogrammed Macrophages Promote Glioblastoma Progression. Int J Mol Sci (2020) 21(11):3990. doi: 10.3390/ijms21113990
28. Sun NN, Zhang Y, Huang WH, Zheng BJ, Jin SY, Li X, et al. Macrophage Exosomes Transfer Angiotensin II Type 1 Receptor to Lung Fibroblasts Mediating Bleomycin-Induced Pulmonary Fibrosis. Chin Med J (Engl) (2021) 134(18):2175–85. doi: 10.1097/cm9.0000000000001605
29. Haque S, Sinha N, Ranjit S, Midde NM, Kashanchi F, Kumar S. Monocyte-Derived Exosomes Upon Exposure to Cigarette Smoke Condensate Alter Their Characteristics and Show Protective Effect Against Cytotoxicity and HIV-1 Replication. Sci Rep (2017) 7(1):16120. doi: 10.1038/s41598-017-16301-9
30. Qin X, Lin X, Liu L, Li Y, Li X, Deng Z, et al. Macrophage-Derived Exosomes Mediate Silica-Induced Pulmonary Fibrosis by Activating Fibroblast in an Endoplasmic Reticulum Stress-Dependent Manner. J Cell Mol Med (2021) 25(9):4466–77. doi: 10.1111/jcmm.16524
31. Zhu QJ, Zhu M, Xu XX, Meng XM, Wu YG. Exosomes From High Glucose-Treated Macrophages Activate Glomerular Mesangial Cells via TGF-β1/Smad3 Pathway In Vivo and In Vitro. FASEB J (2019) 33(8):9279–90. doi: 10.1096/fj.201802427RRR
32. Huang C, Huang Y, Zhou Y, Nie W, Pu X, Xu X, et al. Exosomes Derived From Oxidized LDL-Stimulated Macrophages Attenuate the Growth and Tube Formation of Endothelial Cells. Mol Med Rep (2018) 17(3):4605–10. doi: 10.3892/mmr.2018.8380
33. Lee HD, Kim YH, Kim DS. Exosomes Derived From Human Macrophages Suppress Endothelial Cell Migration by Controlling Integrin Trafficking. Eur J Immunol (2014) 44(4):1156–69. doi: 10.1002/eji.201343660
34. Lee HD, Koo BH, Kim YH, Jeon OH, Kim DS. Exosome Release of ADAM15 and the Functional Implications of Human Macrophage-Derived ADAM15 Exosomes. FASEB J (2012) 26(7):3084–95. doi: 10.1096/fj.11-201681
35. Yao Z, Jia X, Megger DA, Chen J, Liu Y, Li J, et al. Label-Free Proteomic Analysis of Exosomes Secreted From THP-1-Derived Macrophages Treated With IFN-α Identifies Antiviral Proteins Enriched in Exosomes. J Proteome Res (2019) 18(3):855–64. doi: 10.1021/acs.jproteome.8b00514
36. Huang R, Hao C, Wang D, Zhao Q, Li C, Wang C, et al. SPP1 Derived From Silica-Exposed Macrophage Exosomes Triggers Fibroblast Transdifferentiation. Toxicol Appl Pharmacol (2021) 422:115559. doi: 10.1016/j.taap.2021.115559
37. Esser J, Gehrmann U, D’Alexandri FL, Hidalgo-Estévez AM, Wheelock CE, Scheynius A, et al. Exosomes From Human Macrophages and Dendritic Cells Contain Enzymes for Leukotriene Biosynthesis and Promote Granulocyte Migration. J Allergy Clin Immunol (2010) 126(5):1032–1040, 1040.e1031-1034. doi: 10.1016/j.jaci.2010.06.039
38. Hassani K, Olivier M. Immunomodulatory Impact of Leishmania-Induced Macrophage Exosomes: A Comparative Proteomic and Functional Analysis. PloS Negl Trop Dis (2013) 7(5):e2185. doi: 10.1371/journal.pntd.0002185
39. Singhto N, Kanlaya R, Nilnumkhum A, Thongboonkerd V. Roles of Macrophage Exosomes in Immune Response to Calcium Oxalate Monohydrate Crystals. Front Immunol (2018) 9:316. doi: 10.3389/fimmu.2018.00316
40. Yuan D, Zhao Y, Banks WA, Bullock KM, Haney M, Batrakova E, et al. Macrophage Exosomes as Natural Nanocarriers for Protein Delivery to Inflamed Brain. Biomaterials (2017) 142:1–12. doi: 10.1016/j.biomaterials.2017.07.011
41. Osada-Oka M, Shiota M, Izumi Y, Nishiyama M, Tanaka M, Yamaguchi T, et al. Macrophage-Derived Exosomes Induce Inflammatory Factors in Endothelial Cells Under Hypertensive Conditions. Hypertens Res (2017) 40(4):353–60. doi: 10.1038/hr.2016.163
42. Roth WW, Huang MB, Addae Konadu K, Powell MD, Bond VC. Micro RNA in Exosomes From HIV-Infected Macrophages. Int J Environ Res Public Health (2015) 13(1):ijerph13010032. doi: 10.3390/ijerph13010032
43. Yue Y, Huang S, Wu Z, Wang K, Li H, Hou J, et al. Characterization of mRNA Profiles of Exosomes From Diverse Forms of M2 Macrophages. BioMed Res Int (2020) 2020:1585306. doi: 10.1155/2020/1585306
44. Zhang F, Sang Y, Chen D, Wu X, Wang X, Yang W, et al. M2 Macrophage-Derived Exosomal Long non-Coding RNA AGAP2-AS1 Enhances Radiotherapy Immunity in Lung Cancer by Reducing microRNA-296 and Elevating NOTCH2. Cell Death Dis (2021) 12(5):467. doi: 10.1038/s41419-021-03700-0
45. Yin Z, Zhou Y, Ma T, Chen S, Shi N, Zou Y, et al. Down-Regulated lncRNA SBF2-AS1 in M2 Macrophage-Derived Exosomes Elevates miR-122-5p to Restrict XIAP, Thereby Limiting Pancreatic Cancer Development. J Cell Mol Med (2020) 24(9):5028–38. doi: 10.1111/jcmm.15125
46. Chen J, Zhou R, Liang Y, Fu X, Wang D, Wang C. Blockade of lncRNA-ASLNCS5088-Enriched Exosome Generation in M2 Macrophages by GW4869 Dampens the Effect of M2 Macrophages on Orchestrating Fibroblast Activation. FASEB J (2019) 33(11):12200–12. doi: 10.1096/fj.201901610
47. Lu J, Liu D, Tan Y, Deng F, Li R. M1 Macrophage Exosomes MiR-21a-5p Aggravates Inflammatory Bowel Disease Through Decreasing E-Cadherin and Subsequent ILC2 Activation. J Cell Mol Med (2021) 25(6):3041–50. doi: 10.1111/jcmm.16348
48. Liu S, Chen J, Shi J, Zhou W, Wang L, Fang W, et al. M1-Like Macrophage-Derived Exosomes Suppress Angiogenesis and Exacerbate Cardiac Dysfunction in a Myocardial Infarction Microenvironment. Basic Res Cardiol (2020) 115(2):22. doi: 10.1007/s00395-020-0781-7
49. Bouchareychas L, Duong P, Covarrubias S, Alsop E, Phu TA, Chung A, et al. Macrophage Exosomes Resolve Atherosclerosis by Regulating Hematopoiesis and Inflammation via MicroRNA Cargo. Cell Rep (2020) 32(2):107881. doi: 10.1016/j.celrep.2020.107881
50. Guiot J, Cambier M, Boeckx A, Henket M, Nivelles O, Gester F, et al. Macrophage-Derived Exosomes Attenuate Fibrosis in Airway Epithelial Cells Through Delivery of Antifibrotic miR-142-3p. Thorax (2020) 75(10):870–81. doi: 10.1136/thoraxjnl-2019-214077
51. McDonald MK, Tian Y, Qureshi RA, Gormley M, Ertel A, Gao R, et al. Functional Significance of Macrophage-Derived Exosomes in Inflammation and Pain. Pain (2014) 155(8):1527–39. doi: 10.1016/j.pain.2014.04.029
52. Zhu J, Liu B, Wang Z, Wang D, Ni H, Zhang L, et al. Exosomes From Nicotine-Stimulated Macrophages Accelerate Atherosclerosis Through miR-21-3p/PTEN-Mediated VSMC Migration and Proliferation. Theranostics (2019) 9(23):6901–19. doi: 10.7150/thno.37357
53. Zhang YG, Song Y, Guo XL, Miao RY, Fu YQ, Miao CF, et al. Exosomes Derived From oxLDL-Stimulated Macrophages Induce Neutrophil Extracellular Traps to Drive Atherosclerosis. Cell Cycle (2019) 18(20):2674–84. doi: 10.1080/15384101.2019.1654797
54. Xu X, Cheng J, Luo S, Huang D, Xu J, Qian Y, et al. Deoxycholic Acid-Stimulated Macrophage-Derived Exosomes Promote Intestinal Metaplasia and Suppress Proliferation in Human Gastric Epithelial Cells. Eur J Pharmacol (2020) 887:173566. doi: 10.1016/j.ejphar.2020.173566
55. Chen L, Yao X, Yao H, Ji Q, Ding G, Liu X. Exosomal miR-103-3p From LPS-Activated THP-1 Macrophage Contributes to the Activation of Hepatic Stellate Cells. FASEB J (2020) 34(4):5178–92. doi: 10.1096/fj.201902307RRR
56. Hu W, Xu B, Zhang J, Kou C, Liu J, Wang Q, et al. Exosomal miR-146a-5p From Treponema Pallidum-Stimulated Macrophages Reduces Endothelial Cells Permeability and Monocyte Transendothelial Migration by Targeting JAM-C. Exp Cell Res (2020) 388(1):111823. doi: 10.1016/j.yexcr.2020.111823
57. Xiong Y, Chen L, Yan C, Zhou W, Yu T, Sun Y, et al. M2 Macrophagy-Derived Exosomal miRNA-5106 Induces Bone Mesenchymal Stem Cells Towards Osteoblastic Fate by Targeting Salt-Inducible Kinase 2 and 3. J Nanobiotechnol (2020) 18(1):66. doi: 10.1186/s12951-020-00622-5
58. Zhang D, Wu Y, Li Z, Chen H, Huang S, Jian C, et al. MiR-144-5p, an Exosomal miRNA From Bone Marrow-Derived Macrophage in Type 2 Diabetes, Impairs Bone Fracture Healing via Targeting Smad1. J Nanobiotechnol (2021) 19(1):226. doi: 10.1186/s12951-021-00964-8
59. Tian F, Tang P, Sun Z, Zhang R, Zhu D, He J, et al. miR-210 in Exosomes Derived From Macrophages Under High Glucose Promotes Mouse Diabetic Obesity Pathogenesis by Suppressing NDUFA4 Expression. J Diabetes Res (2020) 2020:6894684. doi: 10.1155/2020/6894684
60. Ying X, Jin X, Zhu Y, Liang M, Chang X, Zheng L. Exosomes Released From Decidual Macrophages Deliver miR-153-3p, Which Inhibits Trophoblastic Biological Behavior in Unexplained Recurrent Spontaneous Abortion. Int Immunopharmacol (2020) 88:106981. doi: 10.1016/j.intimp.2020.106981
61. Yao MY, Zhang WH, Ma WT, Liu QH, Xing LH, Zhao GF. microRNA-328 in Exosomes Derived From M2 Macrophages Exerts a Promotive Effect on the Progression of Pulmonary Fibrosis via FAM13A in a Rat Model. Exp Mol Med (2019) 51(6):1–16. doi: 10.1038/s12276-019-0255-x
62. Qi Y, Zhu T, Zhang T, Wang X, Li W, Chen D, et al. M1 Macrophage-Derived Exosomes Transfer miR-222 to Induce Bone Marrow Mesenchymal Stem Cell Apoptosis. Lab Invest (2021) 101(10):1318–26. doi: 10.1038/s41374-021-00622-5
63. Dai Y, Wang S, Chang S, Ren D, Shali S, Li C, et al. M2 Macrophage-Derived Exosomes Carry microRNA-148a to Alleviate Myocardial Ischemia/Reperfusion Injury via Inhibiting TXNIP and the TLR4/NF-ΰB/NLRP3 Inflammasome Signaling Pathway. J Mol Cell Cardiol (2020) 142:65–79. doi: 10.1016/j.yjmcc.2020.02.007
64. Wang Y, Qiu Z, Yuan J, Li C, Zhao R, Liu W, et al. Hypoxia-Reoxygenation Induces Macrophage Polarization and Causes the Release of Exosomal miR-29a to Mediate Cardiomyocyte Pyroptosis. In Vitro Cell Dev Biol Anim (2021) 57(1):30–41. doi: 10.1007/s11626-020-00524-8
65. Liu T, Sun YC, Cheng P, Shao HG. Adipose Tissue Macrophage-Derived Exosomal miR-29a Regulates Obesity-Associated Insulin Resistance. Biochem Biophys Res Commun (2019) 515(2):352–8. doi: 10.1016/j.bbrc.2019.05.113
66. Wang Z, Zhu H, Shi H, Zhao H, Gao R, Weng X, et al. Exosomes Derived From M1 Macrophages Aggravate Neointimal Hyperplasia Following Carotid Artery Injuries in Mice Through miR-222/CDKN1B/CDKN1C Pathway. Cell Death Dis (2019) 10(6):422. doi: 10.1038/s41419-019-1667-1
67. Long R, Gao L, Li Y, Li G, Qin P, Wei Z, et al. M2 Macrophage-Derived Exosomes Carry miR-1271-5p to Alleviate Cardiac Injury in Acute Myocardial Infarction Through Down-Regulating SOX6. Mol Immunol (2021) 136:26–35. doi: 10.1016/j.molimm.2021.05.006
68. Zhou Y, Wang X, Sun L, Zhou L, Ma TC, Song L, et al. Toll-Like Receptor 3-Activated Macrophages Confer Anti-HCV Activity to Hepatocytes Through Exosomes. FASEB J (2016) 30(12):4132–40. doi: 10.1096/fj.201600696R
69. Moradi-Chaleshtori M, Bandehpour M, Soudi S, Mohammadi-Yeganeh S, Hashemi SM. In Vitro and In Vivo Evaluation of Anti-Tumoral Effect of M1 Phenotype Induction in Macrophages by miR-130 and miR-33 Containing Exosomes. Cancer Immunol Immunother (2021) 70(5):1323–39. doi: 10.1007/s00262-020-02762-x
70. Li C, Deng C, Zhou T, Hu J, Dai B, Yi F, et al. MicroRNA-370 Carried by M2 Macrophage-Derived Exosomes Alleviates Asthma Progression Through Inhibiting the FGF1/MAPK/STAT1 Axis. Int J Biol Sci (2021) 17(7):1795–807. doi: 10.7150/ijbs.59715
71. Li M, Li S, Du C, Zhang Y, Li Y, Chu L, et al. Exosomes From Different Cells: Characteristics, Modifications, and Therapeutic Applications. Eur J Med Chem (2020) 207:112784. doi: 10.1016/j.ejmech.2020.112784
72. Zhu Y, Chen X, Pan Q, Wang Y, Su S, Jiang C, et al. A Comprehensive Proteomics Analysis Reveals a Secretory Path- and Status-Dependent Signature of Exosomes Released From Tumor-Associated Macrophages. J Proteome Res (2015) 14(10):4319–31. doi: 10.1021/acs.jproteome.5b00770
73. Li X, Tang M. Exosomes Released From M2 Macrophages Transfer miR-221-3p Contributed to EOC Progression Through Targeting CDKN1B. Cancer Med (2020) 9(16):5976–88. doi: 10.1002/cam4.3252
74. Liu G, Ouyang X, Sun Y, Xiao Y, You B, Gao Y, et al. The miR-92a-2-5p in Exosomes From Macrophages Increases Liver Cancer Cells Invasion via Altering the AR/PHLPP/p-AKT/β-Catenin Signaling. Cell Death Differ (2020) 27(12):3258–72. doi: 10.1038/s41418-020-0575-3
75. Kadiu I, Narayanasamy P, Dash PK, Zhang W, Gendelman HE. Biochemical and Biologic Characterization of Exosomes and Microvesicles as Facilitators of HIV-1 Infection in Macrophages. J Immunol (2012) 189(2):744–54. doi: 10.4049/jimmunol.1102244
76. Mayor S, Pagano RE. Pathways of Clathrin-Independent Endocytosis. Nat Rev Mol Cell Biol (2007) 8(8):603–12. doi: 10.1038/nrm2216
77. Mashouri L, Yousefi H, Aref AR, Ahadi AM, Molaei F, Alahari SK. Exosomes: Composition, Biogenesis, and Mechanisms in Cancer Metastasis and Drug Resistance. Mol Cancer (2019) 18(1):75. doi: 10.1186/s12943-019-0991-5
78. Shan X, Zhang C, Mai C, Hu X, Cheng N, Chen W, et al. The Biogenesis, Biological Functions, and Applications of Macrophage-Derived Exosomes. Front Mol Biosci (2021) 8:715461. doi: 10.3389/fmolb.2021.715461
79. Colombo M, Raposo G, Thery C. Biogenesis, Secretion, and Intercellular Interactions of Exosomes and Other Extracellular Vesicles. Annu Rev Cell Dev Biol (2014) 30:255–89. doi: 10.1146/annurev-cellbio-101512-122326
80. Henne WM, Stenmark H, Emr SD. Molecular Mechanisms of the Membrane Sculpting ESCRT Pathway. Cold Spring Harb Perspect Biol (2013) 5(9). doi: 10.1101/cshperspect.a016766
81. Raposo G, Stoorvogel W. Extracellular Vesicles: Exosomes, Microvesicles, and Friends. J Cell Biol (2013) 200(4):373–83. doi: 10.1083/jcb.201211138
82. Hessvik NP, Llorente A. Current Knowledge on Exosome Biogenesis and Release. Cell Mol Life Sci CMLS (2018) 75(2):193–208. doi: 10.1007/s00018-017-2595-9
83. Kim YB, Ahn YH, Jung JH, Lee YJ, Lee JH, Kang JL. Programming of Macrophages by UV-Irradiated Apoptotic Cancer Cells Inhibits Cancer Progression and Lung Metastasis. Cell Mol Immunol (2019) 16(11):851–67. doi: 10.1038/s41423-019-0209-1
84. Qu Y, Franchi L, Nunez G, Dubyak GR. Nonclassical IL-1 Beta Secretion Stimulated by P2X7 Receptors is Dependent on Inflammasome Activation and Correlated With Exosome Release in Murine Macrophages. J Immunol (2007) 179(3):1913–25. doi: 10.4049/jimmunol.179.3.1913
85. Valimaki E, Cypryk W, Virkanen J, Nurmi K, Turunen PM, Eklund KK, et al. Calpain Activity Is Essential for ATP-Driven Unconventional Vesicle-Mediated Protein Secretion and Inflammasome Activation in Human Macrophages. J Immunol (2016) 197(8):3315–25. doi: 10.4049/jimmunol.1501840
86. Li ZG, Scott MJ, Brzóska T, Sundd P, Li YH, Billiar TR, et al. Lung Epithelial Cell-Derived IL-25 Negatively Regulates LPS-Induced Exosome Release From Macrophages. Mil Med Res (2018) 5(1):24. doi: 10.1186/s40779-018-0173-6
87. Stein M, Keshav S, Harris N, Gordon S. Interleukin 4 Potently Enhances Murine Macrophage Mannose Receptor Activity: A Marker of Alternative Immunologic Macrophage Activation. J Exp Med (1992) 176(1):287–92. doi: 10.1084/jem.176.1.287
88. Murray PJ, Wynn TA. Obstacles and Opportunities for Understanding Macrophage Polarization. J Leukoc Biol (2011) 89(4):557–63. doi: 10.1189/jlb.0710409
89. Mantovani A, Sica A, Sozzani S, Allavena P, Vecchi A, Locati M. The Chemokine System in Diverse Forms of Macrophage Activation and Polarization. Trends Immunol (2004) 25(12):677–86. doi: 10.1016/j.it.2004.09.015
90. Sica A, Mantovani A. Macrophage Plasticity and Polarization: In Vivo Veritas. J Clin Invest (2012) 122(3):787–95. doi: 10.1172/jci59643
91. Murray PJ, Allen JE, Biswas SK, Fisher EA, Gilroy DW, Goerdt S, et al. Macrophage Activation and Polarization: Nomenclature and Experimental Guidelines. Immunity (2014) 41(1):14–20. doi: 10.1016/j.immuni.2014.06.008
92. Sica A, Erreni M, Allavena P, Porta C. Macrophage Polarization in Pathology. Cell Mol Life Sci (2015) 72(21):4111–26. doi: 10.1007/s00018-015-1995-y
93. Bashir S, Sharma Y, Elahi A, Khan F. Macrophage Polarization: The Link Between Inflammation and Related Diseases. Inflammation Res (2016) 65(1):1–11. doi: 10.1007/s00011-015-0874-1
94. Jetten N, Verbruggen S, Gijbels MJ, Post MJ, De Winther MP, Donners MM. Anti-Inflammatory M2, But Not Pro-Inflammatory M1 Macrophages Promote Angiogenesis. Vivo Angiogenesis (2014) 17(1):109–18. doi: 10.1007/s10456-013-9381-6
95. Wang N, Liang H, Zen K. Molecular Mechanisms That Influence the Macrophage M1-M2 Polarization Balance. Front Immunol (2014) 5:614. doi: 10.3389/fimmu.2014.00614
96. Porta C, Riboldi E, Ippolito A, Sica A. Molecular and Epigenetic Basis of Macrophage Polarized Activation. Semin Immunol (2015) 27(4):237–48. doi: 10.1016/j.smim.2015.10.003
97. Funes SC, Rios M, Escobar-Vera J, Kalergis AM. Implications of Macrophage Polarization in Autoimmunity. Immunology (2018) 154(2):186–95. doi: 10.1111/imm.12910
98. Wang C, Zhang C, Liu L, A X, Chen B, Li Y, et al. Macrophage-Derived Mir-155-Containing Exosomes Suppress Fibroblast Proliferation and Promote Fibroblast Inflammation During Cardiac Injury. Mol Ther (2017) 25(1):192–204. doi: 10.1016/j.ymthe.2016.09.001
99. Yang M, Chen J, Su F, Yu B, Su F, Lin L, et al. Microvesicles Secreted by Macrophages Shuttle Invasion-Potentiating microRNAs Into Breast Cancer Cells. Mol Cancer (2011) 10:117. doi: 10.1186/1476-4598-10-117
100. Kang M, Huang CC, Lu Y, Shirazi S, Gajendrareddy P, Ravindran S, et al. Bone Regeneration is Mediated by Macrophage Extracellular Vesicles. Bone (2020) 141:115627. doi: 10.1016/j.bone.2020.115627
101. Reuter S, Gupta SC, Chaturvedi MM, Aggarwal BB. Oxidative Stress, Inflammation, and Cancer: How are They Linked? Free Radic Biol Med (2010) 49(11):1603–16. doi: 10.1016/j.freeradbiomed.2010.09.006
102. Kunnumakkara AB, Sailo BL, Banik K, Harsha C, Prasad S, Gupta SC, et al. Chronic Diseases, Inflammation, and Spices: How are They Linked? J Transl Med (2018) 16(1):14. doi: 10.1186/s12967-018-1381-2
103. Tabas I, Glass CK. Anti-Inflammatory Therapy in Chronic Disease: Challenges and Opportunities. Science (2013) 339(6116):166–72. doi: 10.1126/science.1230720
104. Hwang I. Cell-Cell Communication via Extracellular Membrane Vesicles and its Role in the Immune Response. Mol Cells (2013) 36(2):105–11. doi: 10.1007/s10059-013-0154-2
105. Baig MS, Roy A, Rajpoot S, Liu D, Savai R, Banerjee S, et al. Tumor-Derived Exosomes in the Regulation of Macrophage Polarization. Inflammation Res (2020) 69(5):435–51. doi: 10.1007/s00011-020-01318-0
106. Duan S, Yu S, Yuan T, Yao S, Zhang L. Exogenous Let-7a-5p Induces A549 Lung Cancer Cell Death Through BCL2L1-Mediated PI3Kγ Signaling Pathway. Front Oncol (2019) 9:808. doi: 10.3389/fonc.2019.00808
107. Lan J, Sun L, Xu F, Liu L, Hu F, Song D, et al. M2 Macrophage-Derived Exosomes Promote Cell Migration and Invasion in Colon Cancer. Cancer Res (2019) 79(1):146–58. doi: 10.1158/0008-5472.can-18-0014
108. Moore KJ, Sheedy FJ, Fisher EA. Macrophages in Atherosclerosis: A Dynamic Balance. Nat Rev Immunol (2013) 13(10):709–21. doi: 10.1038/nri3520
109. Koelwyn GJ, Corr EM, Erbay E, Moore KJ. Regulation of Macrophage Immunometabolism in Atherosclerosis. Nat Immunol (2018) 19(6):526–37. doi: 10.1038/s41590-018-0113-3
110. Tabas I, Bornfeldt KE. Macrophage Phenotype and Function in Different Stages of Atherosclerosis. Circ Res (2016) 118(4):653–67. doi: 10.1161/circresaha.115.306256
111. Moore KJ, Tabas I. Macrophages in the Pathogenesis of Atherosclerosis. Cell (2011) 145(3):341–55. doi: 10.1016/j.cell.2011.04.005
112. Llodrá J, Angeli V, Liu J, Trogan E, Fisher EA, Randolph GJ. Emigration of Monocyte-Derived Cells From Atherosclerotic Lesions Characterizes Regressive, But Not Progressive, Plaques. Proc Natl Acad Sci U.S.A. (2004) 101(32):11779–84. doi: 10.1073/pnas.0403259101
113. Chistiakov DA, Orekhov AN, Bobryshev YV. Extracellular Vesicles and Atherosclerotic Disease. Cell Mol Life Sci (2015) 72(14):2697–708. doi: 10.1007/s00018-015-1906-2
114. Hoyer FF, Giesen MK, Nunes França C, Lütjohann D, Nickenig G, Werner N. Monocytic Microparticles Promote Atherogenesis by Modulating Inflammatory Cells in Mice. J Cell Mol Med (2012) 16(11):2777–88. doi: 10.1111/j.1582-4934.2012.01595.x
115. Ismail N, Wang Y, Dakhlallah D, Moldovan L, Agarwal K, Batte K, et al. Macrophage Microvesicles Induce Macrophage Differentiation and miR-223 Transfer. Blood (2013) 121(6):984–95. doi: 10.1182/blood-2011-08-374793
116. Nguyen MA, Karunakaran D, Geoffrion M, Cheng HS, Tandoc K, Perisic Matic L, et al. Extracellular Vesicles Secreted by Atherogenic Macrophages Transfer MicroRNA to Inhibit Cell Migration. Arterioscler Thromb Vasc Biol (2018) 38(1):49–63. doi: 10.1161/atvbaha.117.309795
117. Van den Bossche J, Baardman J, Otto NA, van der Velden S, Neele AE, van den Berg SM, et al. Mitochondrial Dysfunction Prevents Repolarization of Inflammatory Macrophages. Cell Rep (2016) 17(3):684–96. doi: 10.1016/j.celrep.2016.09.008
118. van der Vorst EPC, Weber C. Novel Features of Monocytes and Macrophages in Cardiovascular Biology and Disease. Arterioscler Thromb Vasc Biol (2019) 39(2):e30–7. doi: 10.1161/atvbaha.118.312002
119. Jablonski KA, Gaudet AD, Amici SA, Popovich PG, Guerau-de-Arellano M. Control of the Inflammatory Macrophage Transcriptional Signature by miR-155. PloS One (2016) 11(7):e0159724. doi: 10.1371/journal.pone.0159724
120. Richards J, Gabunia K, Kelemen SE, Kako F, Choi ET, Autieri MV. Interleukin-19 Increases Angiogenesis in Ischemic Hind Limbs by Direct Effects on Both Endothelial Cells and Macrophage Polarization. J Mol Cell Cardiol (2015) 79:21–31. doi: 10.1016/j.yjmcc.2014.11.002
121. Zhao T, Wu W, Sui L, Huang Q, Nan Y, Liu J, et al. Reactive Oxygen Species-Based Nanomaterials for the Treatment of Myocardial Ischemia Reperfusion Injuries. Bioact Mater (2022) 7:47–72. doi: 10.1016/j.bioactmat.2021.06.006
122. Johnson AM, Olefsky JM. The Origins and Drivers of Insulin Resistance. Cell (2013) 152(4):673–84. doi: 10.1016/j.cell.2013.01.041
123. Ehses JA, BÃni-Schnetzler M, Faulenbach M, Donath MY. Macrophages, Cytokines and Beta-Cell Death in Type 2 Diabetes. Biochem Soc Trans (2008) 36(Pt 3):340–2. doi: 10.1042/bst0360340
124. Eguchi K, Manabe I. Macrophages and Islet Inflammation in Type 2 Diabetes. Diabetes Obes Metab (2013) 15 Suppl;3:152–8. doi: 10.1111/dom.12168
125. Gregor MF, Hotamisligil GS. Inflammatory Mechanisms in Obesity. Annu Rev Immunol (2011) 29:415–45. doi: 10.1146/annurev-immunol-031210-101322
126. Lumeng CN, Deyoung SM, Bodzin JL, Saltiel AR. Increased Inflammatory Properties of Adipose Tissue Macrophages Recruited During Diet-Induced Obesity. Diabetes (2007) 56(1):16–23. doi: 10.2337/db06-1076
127. Zeyda M, Stulnig TM. Adipose Tissue Macrophages. Immunol Lett (2007) 112(2):61–7. doi: 10.1016/j.imlet.2007.07.003
128. Ying W, Riopel M, Bandyopadhyay G, Dong Y, Birmingham A, Seo JB, et al. Adipose Tissue Macrophage-Derived Exosomal miRNAs Can Modulate In Vivo and In Vitro Insulin Sensitivity. Cell (2017) 171(2):372–384.e312. doi: 10.1016/j.cell.2017.08.035
129. Qian B, Yang Y, Tang N, Wang J, Sun P, Yang N, et al. M1 Macrophage-Derived Exosomes Impair Beta Cell Insulin Secretion via miR-212-5p by Targeting SIRT2 and Inhibiting Akt/GSK-3î²/β-Catenin Pathway in Mice. Diabetologia (2021) 64(9):2037–51. doi: 10.1007/s00125-021-05489-1
130. Wang W, Yan X, Lin Y, Ge H, Tan Q. Wnt7a Promotes Wound Healing by Regulation of Angiogenesis and Inflammation: Issues on Diabetes and Obesity. J Dermatol Sci (2018) S0923-1811(18)30103-30108. doi: 10.1016/j.jdermsci.2018.02.007
131. Li M, Wang T, Tian H, Wei G, Zhao L, Shi Y. Macrophage-Derived Exosomes Accelerate Wound Healing Through Their Anti-Inflammation Effects in a Diabetic Rat Model. Artif Cells Nanomed Biotechnol (2019) 47(1):3793–803. doi: 10.1080/21691401.2019.1669617
132. Kim H, Wang SY, Kwak G, Yang Y, Kwon IC, Kim SH. Exosome-Guided Phenotypic Switch of M1 to M2 Macrophages for Cutaneous Wound Healing. Advanced Sci (Weinheim Baden-Wurttemberg Germany) (2019) 6(20):1900513–1900513. doi: 10.1002/advs.201900513
133. Huang H, Liu H, Tang J, Xu W, Gan H, Fan Q, et al. M2 Macrophage-Derived Exosomal miR-25-3p Improves High Glucose-Induced Podocytes Injury Through Activation Autophagy via Inhibiting DUSP1 Expression. IUBMB Life (2020) 72(12):2651–62. doi: 10.1002/iub.2393
134. Zhu M, Sun X, Qi X, Xia L, Wu Y. Exosomes From High Glucose-Treated Macrophages Activate Macrophages Andinduce Inflammatory Responses via NF-ΰB Signaling Pathway In Vitro and In Vivo. Int Immunopharmacol (2020) 84:106551. doi: 10.1016/j.intimp.2020.106551
135. Torre LA, Siegel RL, Ward EM, Jemal A. Global Cancer Incidence and Mortality Rates and Trends–An Update. Cancer Epidemiol Biomarkers Prev (2016) 25(1):16–27. doi: 10.1158/1055-9965.epi-15-0578
136. Colotta F, Allavena P, Sica A, Garlanda C, Mantovani A. Cancer-Related Inflammation, the Seventh Hallmark of Cancer: Links to Genetic Instability. Carcinogenesis (2009) 30(7):1073–81. doi: 10.1093/carcin/bgp127
137. Paget S. The Distribution of Secondary Growths in Cancer of the Breast. 1889. Cancer Metastasis Rev (1989) 8(2):98–101.
138. Williams CB, Yeh ES, Soloff AC. Tumor-Associated Macrophages: Unwitting Accomplices in Breast Cancer Malignancy. NPJ Breast Cancer (2016) 2:15025–. doi: 10.1038/npjbcancer.2015.25
139. Salmaninejad A, Valilou SF, Soltani A, Ahmadi S, Abarghan YJ, Rosengren RJ, et al. Tumor-Associated Macrophages: Role in Cancer Development and Therapeutic Implications. Cell Oncol (Dordr) (2019) 42(5):591–608. doi: 10.1007/s13402-019-00453-z
140. Guerriero JL. Macrophages: The Road Less Traveled, Changing Anticancer Therapy. Trends Mol Med (2018) 24(5):472–89. doi: 10.1016/j.molmed.2018.03.006
141. Vitale I, Manic G, Coussens LM, Kroemer G, Galluzzi L. Macrophages and Metabolism in the Tumor Microenvironment. Cell Metab (2019) 30(1):36–50. doi: 10.1016/j.cmet.2019.06.001
142. van der Pol E, BÃing AN, Harrison P, Sturk A, Nieuwland R. Classification, Functions, and Clinical Relevance of Extracellular Vesicles. Pharmacol Rev (2012) 64(3):676–705. doi: 10.1124/pr.112.005983
143. Steinbichler TB, Dudás J, Riechelmann H, Skvortsova II. The Role of Exosomes in Cancer Metastasis. Semin Cancer Biol (2017) 44:170–81. doi: 10.1016/j.semcancer.2017.02.006
144. Zheng P, Luo Q, Wang W, Li J, Wang T, Wang P, et al. Tumor-Associated Macrophages-Derived Exosomes Promote the Migration of Gastric Cancer Cells by Transfer of Functional Apolipoprotein E. Cell Death Dis (2018) 9(4):434. doi: 10.1038/s41419-018-0465-5
145. Wang Y, Jia L, Xie Y, Cai Z, Liu Z, Shen J, et al. Involvement of Macrophage-Derived Exosomes in Abdominal Aortic Aneurysms Development. Atherosclerosis (2019) 289:64–72. doi: 10.1016/j.atherosclerosis.2019.08.016
146. Zheng P, Chen L, Yuan X, Luo Q, Liu Y, Xie G, et al. Exosomal Transfer of Tumor-Associated Macrophage-Derived miR-21 Confers Cisplatin Resistance in Gastric Cancer Cells. J Exp Clin Cancer Res (2017) 36(1):53. doi: 10.1186/s13046-017-0528-y
147. Yin Z, Ma T, Huang B, Lin L, Zhou Y, Yan J, et al. Macrophage-Derived Exosomal microRNA-501-3p Promotes Progression of Pancreatic Ductal Adenocarcinoma Through the TGFBR3-Mediated TGF-β Signaling Pathway. J Exp Clin Cancer Res (2019) 38(1):310. doi: 10.1186/s13046-019-1313-x
148. Guan H, Peng R, Fang F, Mao L, Chen Z, Yang S, et al. Tumor-Associated Macrophages Promote Prostate Cancer Progression via Exosome-Mediated miR-95 Transfer. J Cell Physiol (2020) 235(12):9729–42. doi: 10.1002/jcp.29784
149. Zhu X, Shen H, Yin X, Yang M, Wei H, Chen Q, et al. Macrophages Derived Exosomes Deliver miR-223 to Epithelial Ovarian Cancer Cells to Elicit a Chemoresistant Phenotype. J Exp Clin Cancer Res (2019) 38(1):81. doi: 10.1186/s13046-019-1095-1
150. Hu Y, Li D, Wu A, Qiu X, Di W, Huang L, et al. TWEAK-Stimulated Macrophages Inhibit Metastasis of Epithelial Ovarian Cancer via Exosomal Shuttling of microRNA. Cancer Lett (2017) 393:60–7. doi: 10.1016/j.canlet.2017.02.009
151. Wu Q, Wu X, Ying X, Zhu Q, Wang X, Jiang L, et al. Suppression of Endothelial Cell Migration by Tumor Associated Macrophage-Derived Exosomes is Reversed by Epithelial Ovarian Cancer Exosomal lncRNA. Cancer Cell Int (2017) 17:62. doi: 10.1186/s12935-017-0430-x
152. Zhou J, Li X, Wu X, Zhang T, Zhu Q, Wang X, et al. Exosomes Released From Tumor-Associated Macrophages Transfer miRNAs That Induce a Treg/Th17 Cell Imbalance in Epithelial Ovarian Cancer. Cancer Immunol Res (2018) 6(12):1578–92. doi: 10.1158/2326-6066.cir-17-0479
153. Aucher A, Rudnicka D, Davis DM. MicroRNAs Transfer From Human Macrophages to Hepato-Carcinoma Cells and Inhibit Proliferation. J Immunol (2013) 191(12):6250–60. doi: 10.4049/jimmunol.1301728
154. Wang Y, Wang B, Xiao S, Li Y, Chen Q. miR-125a/B Inhibits Tumor-Associated Macrophages Mediated in Cancer Stem Cells of Hepatocellular Carcinoma by Targeting CD90. J Cell Biochem (2019) 120(3):3046–55. doi: 10.1002/jcb.27436
155. Liu G, Yin L, Ouyang X, Zeng K, Xiao Y, Li Y. M2 Macrophages Promote HCC Cells Invasion and Migration via miR-149-5p/MMP9 Signaling. J Cancer (2020) 11(5):1277–87. doi: 10.7150/jca.35444
156. Binenbaum Y, Fridman E, Yaari Z, Milman N, Schroeder A, Ben David G, et al. Transfer of miRNA in Macrophage-Derived Exosomes Induces Drug Resistance in Pancreatic Adenocarcinoma. Cancer Res (2018) 78(18):5287–99. doi: 10.1158/0008-5472.can-18-0124
157. Challagundla KB, Wise PM, Neviani P, Chava H, Murtadha M, Xu T, et al. Exosome-Mediated Transfer of microRNAs Within the Tumor Microenvironment and Neuroblastoma Resistance to Chemotherapy. J Natl Cancer Inst (2015) 107(7):djv135. doi: 10.1093/jnci/djv135
158. Yang F, Wang T, Du P, Fan H, Dong X, Guo H. M2 Bone Marrow-Derived Macrophage-Derived Exosomes Shuffle microRNA-21 to Accelerate Immune Escape of Glioma by Modulating PEG3. Cancer Cell Int (2020) 20:93. doi: 10.1186/s12935-020-1163-9
159. Wu ATH, Srivastava P, Yadav VK, Tzeng DTW, Iamsaard S, Su EC, et al. Ovatodiolide, Isolated From Anisomeles Indica, Suppresses Bladder Carcinogenesis Through Suppression of mTOR/β-Catenin/CDK6 and Exosomal miR-21 Derived From M2 Tumor-Associated Macrophages. Toxicol Appl Pharmacol (2020) 401:115109. doi: 10.1016/j.taap.2020.115109
160. Mi X, Xu R, Hong S, Xu T, Zhang W, Liu M. M2 Macrophage-Derived Exosomal lncRNA AFAP1-AS1 and MicroRNA-26a Affect Cell Migration and Metastasis in Esophageal Cancer. Mol Ther Nucleic Acids (2020) 22:779–90. doi: 10.1016/j.omtn.2020.09.035
161. Simpson RJ, Lim JW, Moritz RL, Mathivanan S. Exosomes: Proteomic Insights and Diagnostic Potential. Expert Rev Proteomics (2009) 6(3):267–83. doi: 10.1586/epr.09.17
162. Fontana S, Saieva L, Taverna S, Alessandro R. Contribution of Proteomics to Understanding the Role of Tumor-Derived Exosomes in Cancer Progression: State of the Art and New Perspectives. Proteomics (2013) 13(10-11):1581–94. doi: 10.1002/pmic.201200398
163. Cianciaruso C, Beltraminelli T, Duval F, Nassiri S, Hamelin R, Mozes A, et al. Molecular Profiling and Functional Analysis of Macrophage-Derived Tumor Extracellular Vesicles. Cell Rep (2019) 27(10):3062–3080.e3011. doi: 10.1016/j.celrep.2019.05.008
164. Sharma A, Johnson A. Exosome DNA: Critical Regulator of Tumor Immunity and a Diagnostic Biomarker. J Cell Physiol (2020) 235(3):1921–32. doi: 10.1002/jcp.29153
165. Tomita R, Sasabe E, Tomomura A, Yamamoto T. Macrophage‒derived Exosomes Attenuate the Susceptibility of Oral Squamous Cell Carcinoma Cells to Chemotherapeutic Drugs Through the AKT/Gsk‒3β Pathway. Oncol Rep (2020) 44(5):1905–16. doi: 10.3892/or.2020.7748
166. Chen F, Chen J, Yang L, Liu J, Zhang X, Zhang Y, et al. Extracellular Vesicle-Packaged HIF-1α-Stabilizing lncRNA From Tumour-Associated Macrophages Regulates Aerobic Glycolysis of Breast Cancer Cells. Nat Cell Biol (2019) 21(4):498–510. doi: 10.1038/s41556-019-0299-0
167. Wilson MS, Wynn TA. Pulmonary Fibrosis: Pathogenesis, Etiology and Regulation. Mucosal Immunol (2009) 2(2):103–21. doi: 10.1038/mi.2008.85
168. Tan S, Chen S. Macrophage Autophagy and Silicosis: Current Perspective and Latest Insights. Int J Mol Sci (2021) 22(1):453. doi: 10.3390/ijms22010453
169. Ye C, Li H, Bao M, Zhuo R, Jiang G, Wang W. Alveolar Macrophage - Derived Exosomes Modulate Severity and Outcome of Acute Lung Injury. Aging (Albany NY) (2020) 12(7):6120–8. doi: 10.18632/aging.103010
170. Alwarith J, Kahleova H, Crosby L, Brooks A, Brandon L, Levin SM, et al. The Role of Nutrition in Asthma Prevention and Treatment. Nutr Rev (2020) 78(11):928–38. doi: 10.1093/nutrit/nuaa005
171. Busada JT, Ramamoorthy S, Cain DW, Xu X, Cook DN, Cidlowski JA. Endogenous Glucocorticoids Prevent Gastric Metaplasia by Suppressing Spontaneous Inflammation. J Clin Invest (2019) 129(3):1345–58. doi: 10.1172/jci123233
172. Xu X, Cheng J, Luo S, Gong X, Huang D, Xu J, et al. Deoxycholic Acid-Stimulated Macrophage-Derived Exosomes Promote Spasmolytic Polypeptide-Expressing Metaplasia in the Stomach. Biochem Biophys Res Commun (2020) 524(3):649–55. doi: 10.1016/j.bbrc.2020.01.159
173. Di Sabatino A, Lenti MV, Giuffrida P, Vanoli A, Corazza GR. New Insights Into Immune Mechanisms Underlying Autoimmune Diseases of the Gastrointestinal Tract. Autoimmun Rev (2015) 14(12):1161–9. doi: 10.1016/j.autrev.2015.08.004
174. Zheng Y, He R, Wang P, Shi Y, Zhao L, Liang J. Exosomes From LPS-Stimulated Macrophages Induce Neuroprotection and Functional Improvement After Ischemic Stroke by Modulating Microglial Polarization. Biomater Sci (2019) 7(5):2037–49. doi: 10.1039/c8bm01449c
175. Choo YW, Kang M, Kim HY, Han J, Kang S, Lee JR, et al. M1 Macrophage-Derived Nanovesicles Potentiate the Anticancer Efficacy of Immune Checkpoint Inhibitors. ACS Nano (2018) 12(9):8977–93. doi: 10.1021/acsnano.8b02446
176. Kanchanapally R, Deshmukh SK, Chavva SR, Tyagi N, Srivastava SK, Patel GK, et al. Drug-Loaded Exosomal Preparations From Different Cell Types Exhibit Distinctive Loading Capability, Yield, and Antitumor Efficacies: A Comparative Analysis. Int J Nanomed (2019) 14:531–41. doi: 10.2147/ijn.s191313
177. Zhang X, Liu L, Tang M, Li H, Guo X, Yang X. The Effects of Umbilical Cord-Derived Macrophage Exosomes Loaded With Cisplatin on the Growth and Drug Resistance of Ovarian Cancer Cells. Drug Dev Ind Pharm (2020) 46(7):1150–62. doi: 10.1080/03639045.2020.1776320
178. Giri PK, Schorey JS. Exosomes Derived From M. Bovis BCG Infected Macrophages Activate Antigen-Specific CD4+ and CD8+ T Cells In Vitro and In Vivo. PloS One (2008) 3(6):e2461. doi: 10.1371/journal.pone.0002461
179. Cheng L, Wang Y, Huang L. Exosomes From M1-Polarized Macrophages Potentiate the Cancer Vaccine by Creating a Pro-Inflammatory Microenvironment in the Lymph Node. Mol Ther (2017) 25(7):1665–75. doi: 10.1016/j.ymthe.2017.02.007
180. Haney MJ, Klyachko NL, Zhao Y, Gupta R, Plotnikova EG, He Z, et al. Exosomes as Drug Delivery Vehicles for Parkinson’s Disease Therapy. J Control Release (2015) 207:18–30. doi: 10.1016/j.jconrel.2015.03.033
181. Huang Z, Guo L, Huang L, Shi Y, Liang J, Zhao L. Baicalin-Loaded Macrophage-Derived Exosomes Ameliorate Ischemic Brain Injury via the Antioxidative Pathway. Mater Sci Eng C Mater Biol Appl (2021) 126:112123. doi: 10.1016/j.msec.2021.112123
182. Kim MS, Haney MJ, Zhao Y, Mahajan V, Deygen I, Klyachko NL, et al. Development of Exosome-Encapsulated Paclitaxel to Overcome MDR in Cancer Cells. Nanomedicine (2016) 12(3):655–64. doi: 10.1016/j.nano.2015.10.012
183. Gao ZS, Zhang CJ, Xia N, Tian H, Li DY, Lin JQ, et al. Berberine-Loaded M2 Macrophage-Derived Exosomes for Spinal Cord Injury Therapy. Acta Biomater (2021) 126:211–23. doi: 10.1016/j.actbio.2021.03.018
184. Kim MS, Haney MJ, Zhao Y, Yuan D, Deygen I, Klyachko NL, et al. Engineering Macrophage-Derived Exosomes for Targeted Paclitaxel Delivery to Pulmonary Metastases: In Vitro and In Vivo Evaluations. Nanomed: Nanotechnol Biol Med (2018) 14(1):195–204. doi: 10.1016/j.nano.2017.09.011
185. Nie W, Wu G, Zhang J, Huang LL, Ding J, Jiang A, et al. Responsive Exosome Nano-Bioconjugates for Synergistic Cancer Therapy. Angew Chem Int Ed Engl (2020) 59(5):2018–22. doi: 10.1002/anie.201912524
186. Huo Q, Shi Y, Qi Y, Huang L, Sui H, Zhao L. Biomimetic Silibinin-Loaded Macrophage-Derived Exosomes Induce Dual Inhibition of Aβ Aggregation and Astrocyte Activation to Alleviate Cognitive Impairment in a Model of Alzheimer’s Disease. Mater Sci Eng C Mater Biol Appl (2021) 129:112365. doi: 10.1016/j.msec.2021.112365
187. Gong C, Tian J, Wang Z, Gao Y, Wu X, Ding X, et al. Functional Exosome-Mediated Co-Delivery of Doxorubicin and Hydrophobically Modified microRNA 159 for Triple-Negative Breast Cancer Therapy. J Nanobiotechnol (2019) 17(1):93. doi: 10.1186/s12951-019-0526-7
188. Li S, Wu Y, Ding F, Yang J, Li J, Gao X, et al. Engineering Macrophage-Derived Exosomes for Targeted Chemotherapy of Triple-Negative Breast Cancer. Nanoscale (2020) 12(19):10854–62. doi: 10.1039/d0nr00523a
189. Wu G, Zhang J, Zhao Q, Zhuang W, Ding J, Zhang C, et al. Molecularly Engineered Macrophage-Derived Exosomes With Inflammation Tropism and Intrinsic Heme Biosynthesis for Atherosclerosis Treatment. Angew Chem Int Ed Engl (2020) 59(10):4068–74. doi: 10.1002/anie.201913700
190. Wang P, Wang H, Huang Q, Peng C, Yao L, Chen H, et al. Exosomes From M1-Polarized Macrophages Enhance Paclitaxel Antitumor Activity by Activating Macrophages-Mediated Inflammation. Theranostics (2019) 9(6):1714–27. doi: 10.7150/thno.30716
191. Lai RC, Yeo RW, Tan KH, Lim SK. Exosomes for Drug Delivery - a Novel Application for the Mesenchymal Stem Cell. Biotechnol Adv (2013) 31(5):543–51. doi: 10.1016/j.biotechadv.2012.08.008
192. Tran TH, Mattheolabakis G, Aldawsari H, Amiji M. Exosomes as Nanocarriers for Immunotherapy of Cancer and Inflammatory Diseases. Clin Immunol (2015) 160(1):46–58. doi: 10.1016/j.clim.2015.03.021
193. Pardridge WM. Drug Transport Across the Blood-Brain Barrier. J Cereb Blood Flow Metab (2012) 32(11):1959–72. doi: 10.1038/jcbfm.2012.126
194. Luan X, Sansanaphongpricha K, Myers I, Chen H, Yuan H, Sun D. Engineering Exosomes as Refined Biological Nanoplatforms for Drug Delivery. Acta Pharmacol Sin (2017) 38(6):754–63. doi: 10.1038/aps.2017.12
195. Zhao X, Zhao Y, Sun X, Xing Y, Wang X, Yang Q. Immunomodulation of MSCs and MSC-Derived Extracellular Vesicles in Osteoarthritis. Front Bioeng Biotechnol (2020) 8:575057. doi: 10.3389/fbioe.2020.575057
196. Jang SC, Kim OY, Yoon CM, Choi DS, Roh TY, Park J, et al. Bioinspired Exosome-Mimetic Nanovesicles for Targeted Delivery of Chemotherapeutics to Malignant Tumors. ACS Nano (2013) 7(9):7698–710. doi: 10.1021/nn402232g
197. Latvall J, Hill AF, Hochberg F, Buzás EI, Di Vizio D, Gardiner C, et al. Minimal Experimental Requirements for Definition of Extracellular Vesicles and Their Functions: A Position Statement From the International Society for Extracellular Vesicles. J Extracell Vesicles (2014) 3:26913. doi: 10.3402/jev.v3.26913
Keywords: macrophage-derived extracellular vesicles, immunomodulation, chronic diseases, therapeutic strategy, inflammation
Citation: Xing Y, Sun X, Dou Y, Wang M, Zhao Y, Yang Q and Zhao Y (2021) The Immuno-Modulation Effect of Macrophage-Derived Extracellular Vesicles in Chronic Inflammatory Diseases. Front. Immunol. 12:785728. doi: 10.3389/fimmu.2021.785728
Received: 04 October 2021; Accepted: 22 November 2021;
Published: 16 December 2021.
Edited by:
Chaofeng Han, Second Military Medical University, ChinaReviewed by:
Stefania Raimondo, University of Palermo, ItalyLei Shi, Georgia State University, United States
Zhanli Wang, Baotou Medical College, China
Copyright © 2021 Xing, Sun, Dou, Wang, Zhao, Yang and Zhao. This is an open-access article distributed under the terms of the Creative Commons Attribution License (CC BY). The use, distribution or reproduction in other forums is permitted, provided the original author(s) and the copyright owner(s) are credited and that the original publication in this journal is cited, in accordance with accepted academic practice. No use, distribution or reproduction is permitted which does not comply with these terms.
*Correspondence: Yanmei Zhao, emhhb3lhbm1laUAxMjYuY29t; Qiang Yang, eWFuZ3FpYW5nMTk4MEAxMjYuY29t; Yanhong Zhao, bGVhZnpoQDEyNi5jb20=