- 1Department of Plant Pathology, Lovely Professional University, Phagwara, India
- 2Department of Entomology and Plant Pathology, North Carolina State University, Raleigh, NC, United States
Anaerobic soil disinfestation (ASD) is a novel, eco-friendly method to improve soil health. This method creates oxygen-free conditions by adding organic amendments as a carbon source, saturating the soil to field capacity, and then covering it with plastic. These anaerobic conditions reduce soilborne pathogens, enhance microbial diversity, and increase crop productivity. This review provides an overview of the different organic amendments used in ASD, their impact on managing soilborne pathogens, the role of beneficial microbiomes, and the challenges associated with adopting ASD. It also emphasizes the potential benefits of ASD and the innovative approach to advancing it for sustainable agriculture.
1 Introduction
1.1 Anaerobic soil disinfestation concept and significance
For many years, pre-plant soil fumigation using methyl bromide 1, 3-dichloropropene, chloropicrin, and methyl iodide has been the go-to method to manage several soilborne plant pathogens in various crops (Theis and Fery, 2002; Martin, 2003; Velders et al., 2007; Hanson et al., 2011; Panth et al., 2020). However, the phase-out of ozone-depleting fumigants, including methyl bromide, in 2005 due to environmental pollution (Hanson et al., 2011; Rosskopf et al., 2015; Strauss and Kluepfel, 2015) has led to the exploration of alternative methods. Other soil fumigants such as trichloronitromethane, methyl isothiocyanate, allyl isothiocyanate (AITC), and dazomet were also used to manage soilborne pathogens (Martin, 2003). However, their use also has many drawbacks, such as reduced efficacy and regulatory concerns. The major soilborne pathogens are Verticillium spp., Fusarium spp., Pythium spp., Rhizoctonia spp., and Sclerotinia spp (Lamers et al., 2014), soil nematodes (Testen et al., 2021), and weeds (Strauss and Kluepfel, 2015; Panth et al., 2020). These pathogenic fungi can survive for long periods due to their ability to form resilient structures (Katan, 2017; Priyashantha and Attanayake, 2021). Non-chemical soil disinfestation, particularly anaerobic soil disinfestation (ASD), comes in as a potential integrated pest management (IPM) tool for managing soilborne pathogens (Rokunuzzaman et al., 2016). The potential of ASD to revolutionize pest management is a promising prospect for the future.
ASD, a unique approach that utilizes organic amendments as carbon sources, is gaining popularity in agricultural practices worldwide (Molendijk et al., 2009). This technique, known as biological soil disinfestation (BSD), was initially developed in Japan and the Netherlands (Blok et al., 2000; Momma et al., 2006). Unlike traditional organic amendments that increase organic matter, improve soil fertility, and enhance water-holding capacity, specific carbon sources can induce ASD (Poret-Peterson et al., 2019). This process stimulates the soil microbiome to efficiently break down and utilize the carbon source (e.g., sugar) as its sole energy source. By creating an oxygen-depleted environment within the soil, ASD significantly, enhanced anaerobic beneficial microbiomes compete with or suppress soilborne pathogens’ populations (Molendijk et al., 2009; Lamers et al., 2014; Strauss and Kluepfel, 2015). For instance, a strict anaerobic bacterium, Clostridia, was sharply increased in soil-amended with wheat bran and cover crops such as Brassica juncea or Avena sativa as a carbon source (Mowlick et al., 2013a, b). This review aims to describe the current status of ASD and its potential impacts on crop production systems, emphasizing future research directions regarding challenges and opportunities in using ASD (Figure 1).
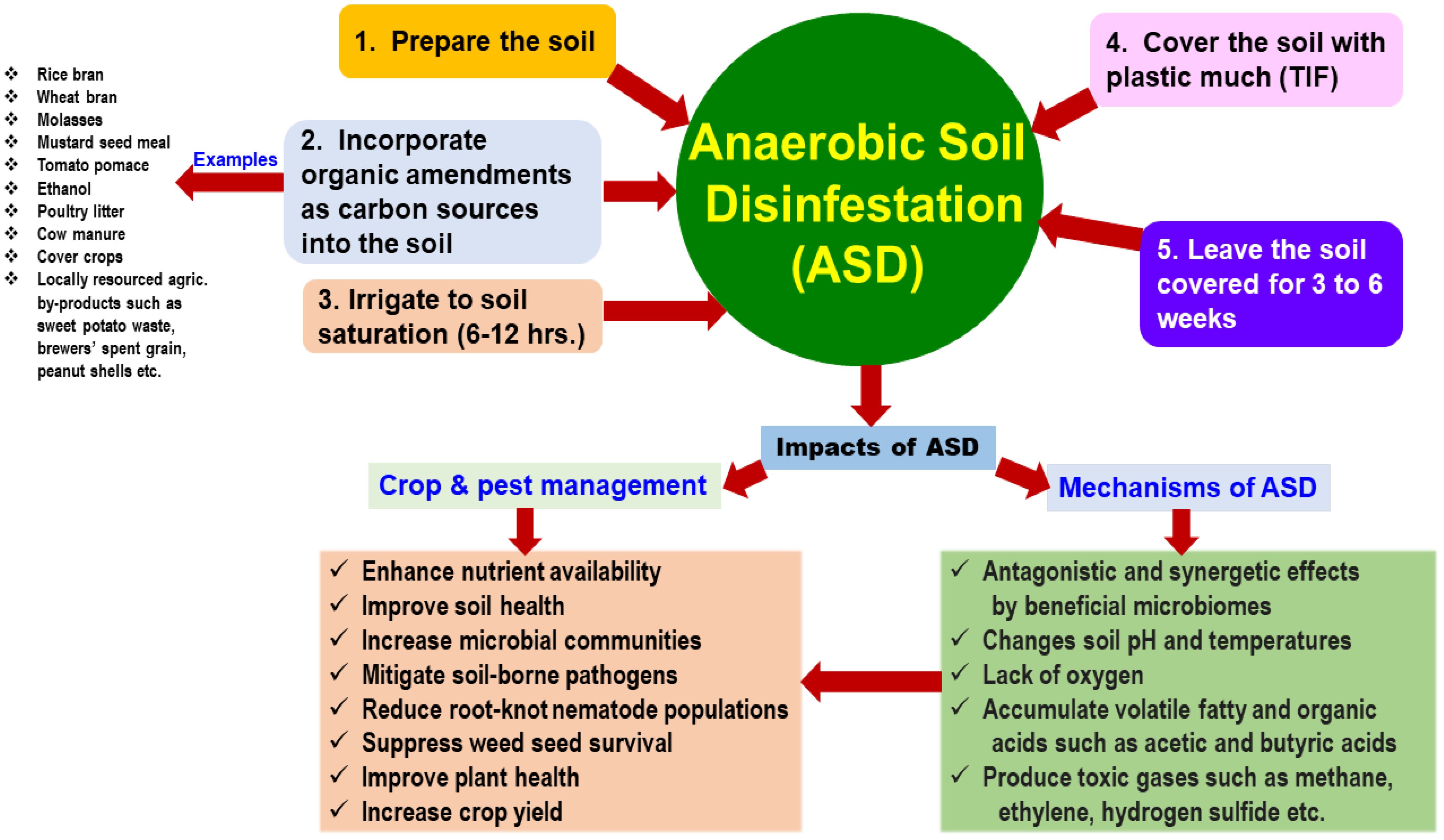
Figure 1. Overview of anaerobic soil disinfestation (ASD), which enhances soil structure, suppresses weeds, fosters microbial diversity, promotes plant health, and improves crop yield (Blok et al., 2000; Butler et al., 2012a, b; Momma et al., 2013; Hewavitharana et al., 2019; 2021; Hewavitharana and Mazzola, 2016; Lopes et al., 2022; Priyashantha and Attanayake, 2021; Shennan et al., 2014; 2018; Rosskopf et al., 2015; 2020; Strauss and Kluepfel, 2015).
1.2 The key steps and organic amendments used as a carbon source in ASD
ASD involves the addition of organic amendments to the topsoil to serve as a carbon source (Shennan et al., 2014, 2018). The first step is to till and moisten the soil to create optimal conditions. Then, a readily degradable organic material, such as fresh manure or compost, is incorporated into the soil to feed these beneficial microbes. Next, the soil surface is sealed with an impermeable tarp to create an oxygen-limited environment for 3 to 6 weeks, depending on soil temperature and target organisms. Finally, the tarp is removed, and the soil is tilled again to reintroduce oxygen and break down the organic matter (Blok et al., 2000; Momma et al., 2013; Shennan et al., 2014; Rosskopf et al., 2015; Strauss and Kluepfel, 2015; Shennan et al., 2018; Priyashantha and Attanayake, 2021; Lopes et al., 2022).
In recent years, three types of carbon sources have been utilized in ASD (Strauss and Kluepfel, 2015). The usual solid organic amendments used as carbon sources in ASD include rice bran, wheat bran, dry molasses, and mustard meal in the cultivation of fruits and vegetables (Shennan et al., 2014; Testen and Miller, 2018; Wang and Mazzola, 2019). For example, ASD with rice and wheat brans was used in strawberry fields in California (Shennan et al., 2014) and for tomato and melon cultivation in Japan (Momma et al., 2013). Wheat and rice brans were both applied at a rate of 10 t/ha in the fields, effectively suppressing Fusarium oxysporum (Momma et al., 2010) and Verticillium dahliae (Shennan et al., 2018), respectively. Furthermore, when ASD with rice bran was applied at two rates (15.7 t/ha and 202.2 t/ha) to nut-tree nurseries in California, both of these rates significantly suppressed the populations of Agrobacterium tumefaciens and Pythium spp. in the soil (Strauss and Kluepfel, 2015). Liquid organic amendments, such as ethanol, liquid molasses, and sucrose, were commonly utilized as carbon sources in ASD (Strauss and Kluepfel, 2015; Lopes et al., 2022). For instance, a blend of dry molasses on a soy hull carrier and cornstarch applied at a 4 mg C/g soil rate with a carbon-to-nitrogen ratio of 30:1 has shown promise (Swilling et al., 2021). The combination of dry and liquid organic amendments used in ASD included composted poultry litter and liquid molasses or compost with solarization, significantly increasing soil inorganic N concentrations and suppressing soilborne fungi and Meloidogyne spp. root galling (Butler et al., 2012b, 2014; Strauss and Kluepfel, 2015).
In ASD application, the incubation period (anaerobic state) has been observed to last up to 3 weeks (Momma et al., 2010; Shennan et al., 2018). Several studies have investigated using various carbon sources in ASD for different durations in multiple crops. For example, fresh broccoli as a carbon source in ASD was studied for 15 weeks (Blok et al., 2000), while perennial ryegrass and potato haulms were used in potato cultivation for six weeks (Messiha et al., 2007). Wheat and rice brans were used in ASD in tomatoes and apples (Hewavitharana et al., 2014), and mustard was studied as a substrate for spinach over three weeks (Mowlick et al., 2013b). Grape pomace was used as a substrate in pepper cultivation for four weeks (Serrano-Pérez et al., 2017), and apple substrates were examined for two weeks (Mowlick et al., 2013a; Strauss and Kluepfel, 2015).
The critical steps of ASD involve the decomposition of carbon sources and changes in the soil microbiome and metabolome over three distinct phases (Hewavitharana et al., 2019). Briefly, in phase 1 (0 to 12 hrs), there is rapid consumption of oxygen, amino acids, and polysaccharides, with a dominance of aerobic and facultative bacterial taxa such as Firmicutes (Bacillus spp.), Actinobacteria (Streptomyces spp.), Proteobacteria (Pseudomonas spp.) and fungal phylum such as Ascomycota (Trichoderma). During phase 2 (peak at 48 hrs), bacterial taxa such as Firmicutes, Actinobacteria, Proteobacteria, and fungal phylum such as Zygomycota become dominant due to strict aerobes consuming oxygen. Lactic acid levels peak during this stage and strongly correlate with the rapid multiplication of Bacillus spp (Hewavitharana et al., 2019). Moreover, acetone, acetic acid, 1-butanol, and butanoic acid accumulate as Clostridium spp. multiply. In phase 3 (up to 3 weeks), antipathogen compounds produced by strict anaerobes, especially Clostridium spp., are frequently present in soil treated with ASD. These compounds, including acetate, butyrate, volatile acids, alcohols, volatile hydrocarbons, dimethyl di- and tri-sulfide, and dimethyl sulfoxide, are released during the final stage of ASD (Hewavitharana et al., 2019).
1.3 History of ASD and geographic application
In sub-tropical developing countries, reducing arable land due to industrialization and rapid population growth poses a significant threat to crop productivity (Priyashantha and Attanayake, 2021). Other issues include small-scale farming, multiple cropping, year-round crop cultivation, high crop and plant pathogen diversity, limited access to new technology and drip irrigation, and lack of knowledge among farmers (Phalan et al., 2013; Priyashantha and Attanayake, 2021). Crop productivity in developing subtropical countries is considerably lower than in temperate countries (Thottathil et al., 2016). Therefore, it is crucial to maximize soil health for sustainable agriculture (Priyashantha and Attanayake, 2021).
To effectively implement ASD for managing soilborne plant pathogens, it is crucial to understand its effectiveness in different settings, such as varying times of the year, cropping systems, and soil types (Poret-Peterson et al., 2019). Identifying the optimal carbon they play a vital role in reducing production costs (Strauss and Kluepfel, 2015; Shrestha et al., 2016). The effectiveness of ASD largely depends on selecting easily applicable, locally available, and easily degradable organic amendments or agricultural by-products (Strauss and Kluepfel, 2015; Lopes et al., 2022). Most ASD studies have been carried out in temperate and sub-tropical countries. Among temperate countries, 63.3% of ASD work was done in the United States (Priyashantha and Attanayake, 2021). However, limited ASD research has been conducted in tropical developing countries.
ASD has shown promise for high soil temperatures and sandy soil. Based on a meta-analysis conducted by Shrestha et al. (2016), in both laboratory and growth chamber conditions, a 61% pathogen suppression was observed, while the field and greenhouse conditions showed slightly higher suppression at 72%. The authors suggested that at higher soil temperatures, the pathogen reduction from ASD effect was about 10% higher than at moderate and lower temperatures (Shrestha et al., 2016). Furthermore, the ASD treatment in volcanic soil from Japan demonstrated a significantly higher suppression of plant pathogens compared to sandy soil (83% vs. 64%). At the same time, no significant difference was observed between soil type and clay, gray lowland, and loam soil (Shrestha et al., 2016). This is likely due to the suppression of pathogens and weeds by lethal temperatures and adding organic matter to the sandy soils (Strauss and Kluepfel, 2015; Shrestha et al., 2016). ASD studies conducted in temperate countries like Ohio, USA, showed that average soil temperatures ranged from 16.3 to 27.8°C for high tunnel tomato production in September and October (Testen et al., 2021) or 23.6 to 30.8°C for summer tomato production in muck soils (Testen and Miller, 2018). Despite these lower soil temperatures, the loss of iron oxide paint, which indicates soil-reducing conditions, was slightly lower in most trials than in ASD trials conducted with warmer soil temperatures (Testen and Miller, 2018; Testen et al., 2021). However, high soil temperatures (20 to 25°C) are required for effectively managing the tomato soilborne disease complex (Testen et al., 2021).
ASD was first launched in Japan and effectively managed soilborne fungal pathogens. The Netherlands followed and modified the model, and ASD was later adopted in other countries, including China (Ya’nan et al., 2019), Paraguay (Sanabria-Velazquez, 2018; Quintino et al., 2023), the USA (Butler et al., 2012a, b; Garcia Gonzalez, 2021; Shennan et al., 2018), Sri Lanka (Priyashantha and Attanayake, 2021), and Nepal (Bhandari et al., 2020; Khadka and Miller, 2021; Khadka et al., 2021) to combat soilborne pathogens (Figure 2).
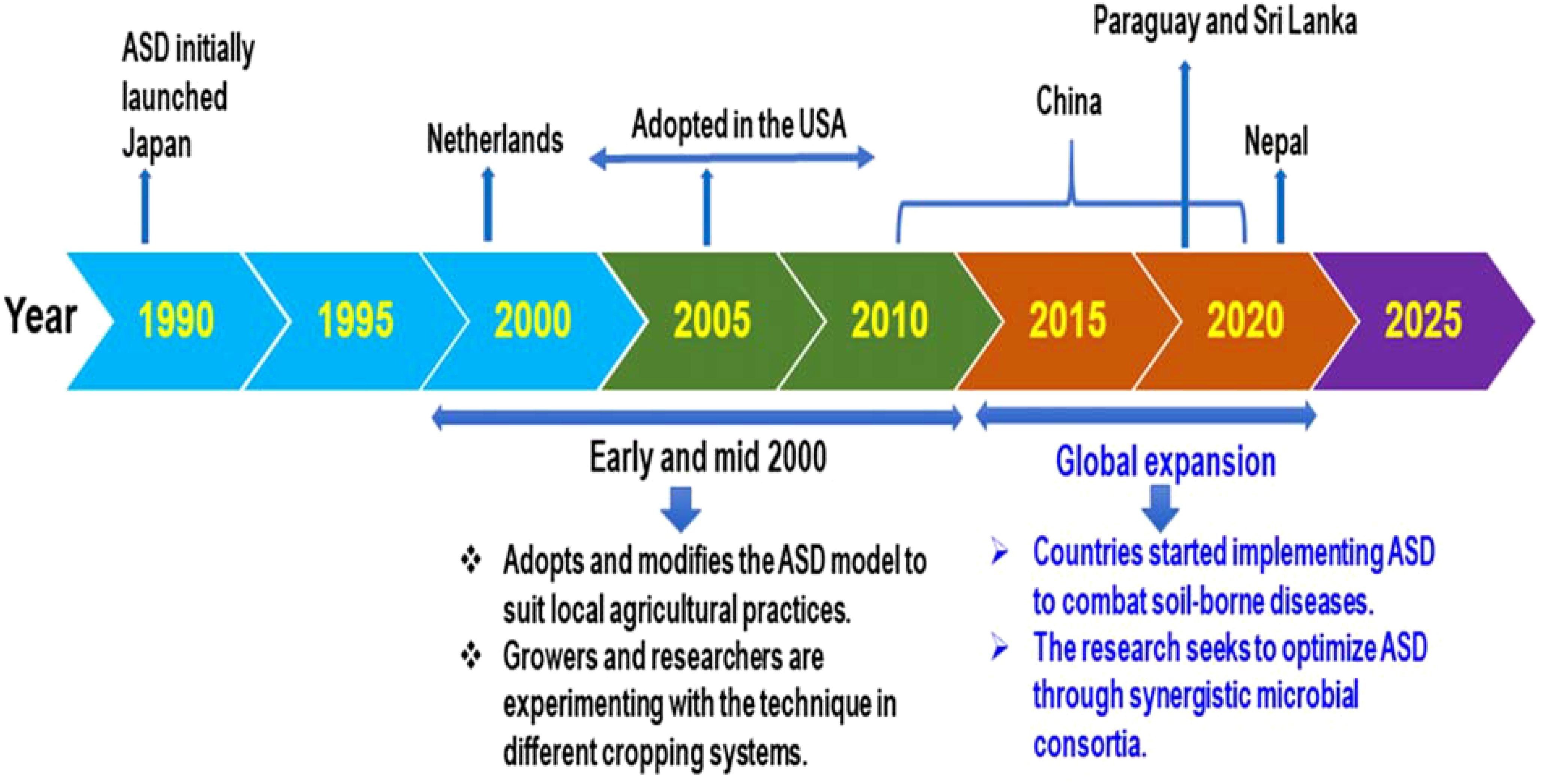
Figure 2. Mapping the global landscape: ASD innovation and adoption strategies (Momma et al., 2010; 2013; Khadka et al., 2021; Khadka and Miller, 2021; Priyashantha and Attanayake, 2021; Sanabria-Velazquez, 2018; Shennan et al., 2014; 2018; Quintino et al., 2023; Ya'nan et al., 2019).
2 Impacts of ASD
2.1 Impact of ASD on soil physiochemical properties and soil health
The success of ASD is influenced by both the physical and chemical changes and the biological shifts in soils (Strauss and Kluepfel, 2015; Shrestha et al., 2016), which may be related to the carbon sources used to induce ASD (Hewavitharana et al., 2014; Hewavitharana and Mazzola, 2016). ASD can influence individual microbial species’ composition, functionality, and interactions, making them valuable soil health indicators (Preece et al., 2019). Additionally, it can significantly alter microbial composition and diversity and improve soil health (Messiha et al., 2007; Mowlick et al., 2013a; Poret-Peterson et al., 2019, 2020). Soil temperature, water-holding capacity, soil type, and carbon sources contribute to ASD (Strauss and Kluepfel, 2015; Shrestha et al., 2016; Shennan et al., 2018).
Different organic amendments used as carbon sources are rice straw, tobacco, rice bran, rapeseed meal, and sugarcane bagasse, significantly influencing soil fertility and health (Lopes et al., 2022). ASD can alter soil pH by producing organic acids and methane through anaerobic microbiomes (Priyashantha and Attanayake, 2021). Decomposing organic matter in ASD produces acetic and n-butyric acids, lowering soil pH to around 5.5 and affecting soil-dwelling nematodes (Momma et al., 2006, 2013). This could be caused by anaerobic microbiomes consuming carbon sources, generating organic acids, and releasing iron (Fe2+) and manganese (Mn2+) ions, ultimately decreasing the soil pH (Momma et al., 2006, 2013). Adding rapeseed meal and tobacco stem in ASD significantly increased soil pH, lowered soil electrical conductivity (EC) and redox potential (Eh), and improved soil nutrient levels (Ya’nan et al., 2019). Additionally, using tobacco stems significantly increased the available potassium and organic matter levels in the soil. At the same time, rapeseed meals raised the levels of total nitrogen, organic matter, available nitrogen, and the activities of catalase and urease enzymes (Ya’nan et al., 2019). In China, soil treated with ASD using composted chicken manure showed a significant increase in ammonium nitrogen, nitrate-nitrogen, and organic matter (Song et al., 2020). Additionally, there was a substantial reduction in Fusarium spp. and Phytophthora spp. colonies in the strawberry field due to decreased oxidation-reduction potential (Song et al., 2020). The use of different carbon sources in ASD, such as alfalfa, maize, and rice straw, can result in an increase in NH4+-N content and a decrease in NO3-N accumulation in the soil (Tan et al., 2019). This, in turn, can reduce soilborne pathogens like Fusarium and Aspergillus spp. Selecting carbon sources can also influence the C: N ratio and the soil’s chemical and physical properties (Tan et al., 2019).
The C: N ratio is a crucial indicator of decomposition rate (Nicolardot et al., 2001). Shrestha et al. (2021) successfully used the ASD with organic amendments, using C: N ratios between 10:1 and 40:1, to suppress Fusarium oxysporum f. sp. lycopersici in soils above 25°C. Soil treated with ASD using wheat bran showed lower levels of water-soluble organic carbon and a higher C: N ratio compared to soil treated with other carbon sources, presumably due to the presence of recalcitrant carbon fractions such as cellulose, hemicellulose, and lignin in wheat bran (Lee et al., 2020). Dried molasses rapidly dissolved in the irrigation water during ASD due to its high water-soluble organic carbon fraction and C: N ratio (Lee et al., 2020). Previous studies have suggested that adding organic matter with a low C: N ratio to the soil could create the most anaerobic conditions and that the substrate C: N ratio is inversely related to the effectiveness of soil disinfestation (Blok et al., 2000; Shrestha et al., 2016; Testen and Miller, 2018). However, different substrates had various effects on the prokaryotic community, and the decomposition rate of the carbon sources, particularly the substrate’s C: N ratio and water-soluble organic carbon content did not show a correlation with disinfestation efficiency (Lee et al., 2020).
Soils treated with ASD contained high levels of microbial communities capable of carrying out processes such as nitrogen fixation, denitrification, and sulfur, potassium, and phosphorus cycling, indicating that ASD may improve the availability of nitrogen, potassium, and phosphorus in the soil (Di Gioia et al., 2017; Poret-Peterson et al., 2019). Zhu et al. (2023a) found that the properties of the carbon source, crop cultivation, and soil chemical properties contributed to 66.2% and 39.0% of bacterial and fungal community variation, respectively. The results also indicated that factors such as the C: N ratio, crop cultivation, soil available phosphorus, and potassium significantly influenced bacterial community composition (Zhu et al., 2023b). Similarly, the C: N ratio, oxidized carbon contents, and crop cultivation were identified as crucial factors in shaping fungal community composition. The authors suggested that the C: N ratio and oxidized carbon contents of the carbon source were critical in soil microbiome reestablishment, Fusarium reproduction, and pepper crop performance in China (Zhu et al., 2023a).
2.2 Impact of ASD on microbial community composition and diversity
ASD with different carbon sources play a crucial role in improving soil microbial functioning and communities (Van Agtmaal et al., 2015; Shennan et al., 2018; Mao et al., 2022). When the soil is saturated with water, adding an easily available carbon source is thought to stimulate the rapid growth of aerobic microbiomes (Messiha et al., 2007). For example, bacterial phyla, such as Firmicutes and Proteobacteria, increased relative abundance in anaerobic conditions (Ya’nan et al., 2019). These microbiomes consume the remaining soil oxygen and create anaerobic soil conditions. This shift to anaerobic conditions can happen quickly and is influenced by factors such as soil water content, texture, structure, quality and quantity of the carbon source, and soil temperature (Butler et al., 2012a; Shennan et al., 2014). The bacterial community structure shifted 2 to 15 days after ASD initiation using ethanol (1% v/v) as the carbon source (Momma, 2015). However, Van Agtmaal et al. (2015) found a clear long-lasting impact of previous anaerobic stress on bacterial community composition three months after the ASD treatment. During the ASD process, Firmicutes phyla, such as Bacillus, Clostridia, and Paenibacillus, become the predominant bacterial population (Mowlick et al., 2013a; Mowlick et al., 2014; Strauss and Kluepfel, 2015).
The type of organic carbon source used can significantly influence the dominant bacterial species. For example, ASD using rice bran as a carbon source can increase the abundance of Acidobacteria and Burkholderia (Strauss and Kluepfel, 2015). In contrast, ASD with alfalfa as the carbon source can lead to Flavisolibacter, Rhodanobacter, and Ruminococcaceae becoming dominant bacterial genera (Huang et al., 2016). After applying ASD in vegetable production systems, the polyethylene tarp or mulch can be either removed or punctured with holes for planting (Shennan et al., 2014). This reintroduces oxygen into the soil, which helps in restructuring the diversity of the microbial community back to an aerobic state (Husson, 2013). The 16S amplicon sequencing of post-ASD samples (three weeks before transplanting) showed significant soil microbiome changes and increased Firmicutes and Proteobacteria abundance compared to non-treated controls (Hong et al., 2018). Various experiments with different carbon sources have shown that ASD enhances specific soil microbiomes (Table 1).
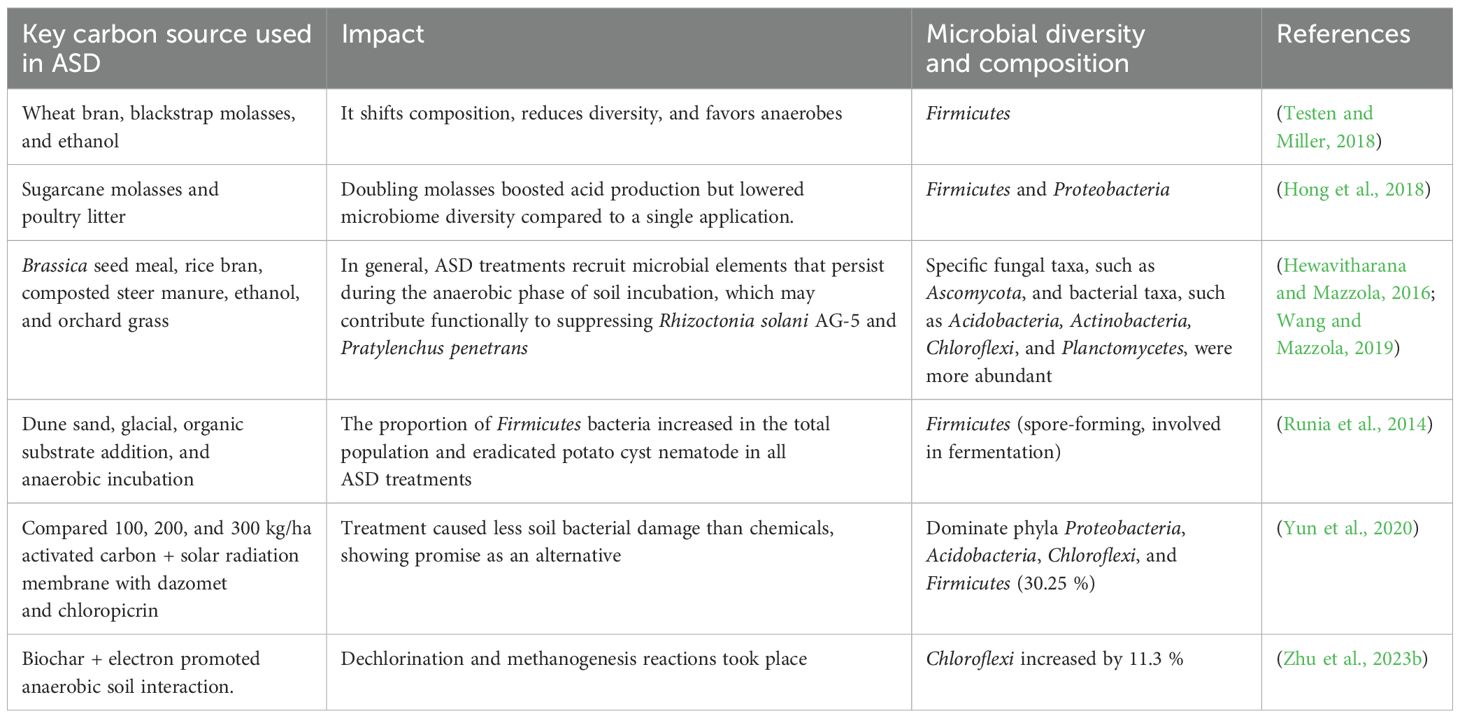
Table 1. Summary of the impact of anaerobic soil disinfestation (ASD) using common organic amendments as a carbon source on the composition of the microbial community.
Temporal changes in the microbiome composition and metabolome were observed in soil treated with ASD (Guo et al., 2018; Hewavitharana et al., 2019, 2021). Hewavitharana et al. (2019) found that Fermicutes were the most abundant bacterial communities in the soil during the anaerobic period following ASD treatment. Initially, Bacillus spp. dominated the Fermicutes community, but as the ASD treatment progressed, anaerobic Clostridia, specifically Clostridium intestinale and Clostridium roseum, displaced Bacillus spp. Poret-Peterson et al. (2019) also observed similar trends in the changes to Fermicute populations during the incubation period. Lactic acid production was noted during the aeration period between 21 and 32 days, attributed to lactic acid bacteria such as Enterobacter hormaechei and Enterobacter ludwigi (Hewavitharana et al., 2021). Carbon sources such as rice bran, orchard grass, and wheatgrass are rich in polysaccharides, monosaccharides, and amino acids, and serve as ideal substrates for soil microbiomes (Miller and Chibnall, 1932; Singh and Sogi, 2016). Several soil fungi (Christakopoulos et al., 1989; Panagiotou et al., 2005) also utilize d-glucose and sucrose as readily available and rapidly consumable substrates in ASD treatments (Hewavitharana et al., 2021). After treating the soil with ASD using a small amount of rice bran, the authors discovered a rapid sugar decrease on the first day (Hewavitharana et al., 2021). However, sugars in orchard grass and wheatgrass used for ASD were broken down during the aeration phase. Rice bran is readily digested by the soil microbiome (Hewavitharana et al., 2019), while the slower consumption of grass substrates other than rice bran may result from differences in cellulose composition (Hewavitharana et al., 2021). Consequently, increased beneficial microbial populations after applying ASD leads to pathogen suppression, competition for substrate, and an increase in the soil’s total microbial community, improving soil health (Rosskopf et al., 2020).
ASD with rapeseed meal and tobacco stem significantly altered the composition of bacterial communities and increased the number of anaerobic bacteria (Ya’nan et al., 2019). When wheat bran, sugar-contained diatoms, and dried molasses were used as substrates in ASD in a greenhouse, the microbial communities were significantly affected by variations in the substrate and disinfection period (Lee et al., 2020). Likewise, using non-rice bran carbon substrates revealed that specific types of bacteria positively respond to ASD (Poret-Peterson et al., 2019). These bacteria can perform crucial functions such as denitrification, nitrogen fixation, and fermentation (Poret-Peterson et al., 2019).
ASD influences bacterial alpha diversity in clay-textured soils (Stefan et al., 2021). Furthermore, they found that this characteristic promotes microbial proliferation through enhanced aggregate formation, increased water retention, and improved nutrient preservation. Variations in soil microbial Shannon index responses, phylogenetic diversity, and specialized microbial Shannon index to organic amendments notably depended on the initial soil pH (Shu et al., 2022). These indicators were more evident in acidic soil, possibly due to the alkalinizing effects of organic amendments (Chen et al., 2019).
Applying ASD with different carbon sources reduced microbial diversity (Hewavitharana and Mazzola, 2016; Testen and Miller, 2018; Poret-Peterson et al., 2019, 2020). The shift from aerobic to anaerobic conditions in soil reduces the available niche space for the resident microbiome (Spietz et al., 2015; Strauss et al., 2017). In addition to the fungal structure, Poret-Peterson et al. (2020) show significant changes in fungal diversity when using different organic substrates for soil disinfestation. This shift in fungal diversity was mainly driven by how soil fungi compete for resources (Hanson et al., 2008). Additionally, Poret-Peterson et al. (2020) discovered that the overall fungal Chao1 richness, Shannon diversity, and Pielou’s evenness were similar to those of the no carbon control (NCC) communities. The Shannon diversity varied significantly between the NCC and ASD treatments using rice bran, red grape, or tomato pomace, and it changed over time (Poret-Peterson et al., 2020).
The Shannon’s diversity index decreased on day 4 and remained relatively constant. However, the density of Fusarium oxysporum in tomato fields decreased after day 4 (Liu et al., 2016). Messiha et al. (2007) found that microbial diversity did not differ between carbon source-amended and non-amended soils after ASD treatment, but their community structure differed. On the other hand, ASD conducted using molasses did not alter the community structure (Shennan et al., 2014). These studies collectively suggest that microbial diversity, community structure, and soil redox potential (Eh) are not correlated with disease incidence (Lee et al., 2020).
Testen and Miller (2018) examined the effects of ASD using different carbon sources on soil bacterial composition and diversity in two farms in Ohio, USA. They found that the alpha diversity of bacterial communities in soil samples following ASD with wheat bran was significantly lower than non-amended soils (Testen and Miller, 2018). Similarly, the alpha diversity was significantly reduced in soils following ASD with ethanol in both Highland soils. However, no significant difference in alpha diversity was observed when molasses was used in ASD compared to the control soils on either farm (Testen and Miller, 2018). In another study, a reduction in total organic carbon in the soil harmed fungal community composition. This was evident in the significant decrease in fungal Shannon and Chao1 diversity indexes, further exacerbating Fusarium spp. reproduction (Saleem et al., 2019). In China, Zhan et al. (2021) investigated the effects of ASD or reductive soil disinfestation (RSD) using animal feces on soil physicochemical properties, bacterial composition, and both alpha diversity and beta diversity. The results showed that the RSD-treated soil had unique core microbiomes and diverse disease-suppressive microbiomes. Additionally, changes in bacterial structure were observed, with lower bacterial richness, diversity, and evenness compared to untreated control soil (Zhan et al., 2021).
2.3 Impact of ASD on soilborne plant pathogen suppression
ASD is an environmentally friendly and sustainable approach to managing soilborne bacterial, fungal, and nematode plant pathogens (Mao et al., 2022). Differential production of ASD antipathogen factors may explain the differing soilborne pest management. For example, wheat bran and ethanol created the most effective soil-reducing conditions (Testen and Miller, 2018). Consequently, a moderate correlation between iron oxide paint loss and disease severity data suggests that wheat bran could influence soilborne pathogen management compared to molasses (Testen and Miller, 2018).
Compared to non-treated soil, changes in the prokaryotic community in ASD-treated soil effectively reduced the Fusarium oxysporum in tomatoes within three days (Lee et al., 2020). The population of Fusarium oxysporum in tomatoes decreased during the early stages of ASD due to a significant increase in the levels of Clostridia and Bacilli (Lee et al., 2020). Additionally, Bacilli, such as Bacillus and Paenibacillus, are well-known biocontrol agents for Fusarium oxysporum in tomatoes (Ueki et al., 2018). Some Clostridia can kill Fusarium oxysporum f. sp. lycopersici directly by producing chitinase (Momma, 2008; Ueki et al., 2017). Some Bacilli may contribute to the rapid decrease in soil Eh during the initial stage of ASD treatment through oxygen consumption (Mowlick et al., 2013b). Furthermore, the increase in clostridial populations in ASD-treated soils may be associated with a higher production of VFAs and could potentially be toxic to soilborne plant pathogens (Momma et al., 2006; Mowlick et al., 2013a).
Using different carbon sources, such as peanut, rice, and wheat brans, significantly reduced tomato bacterial wilt caused by Ralstonia solanacearum (Mao et al., 2022). The disease incidence was reduced by 83 to 100%, promoting tomato growth (Messiha et al., 2007; Mao et al., 2022). This is likely due to improved soil conditions, such as lower Eh, NO3−, SO42−, and increased pH, as well as increased biological activity, such as higher dehydrogenase and urease activities (Momma et al., 2006; Mowlick et al., 2013b). Additionally, anaerobic treatments with carbon sources led to elevated production of antagonistic compounds, including Fe2+, Mn2+, citric acid, succinic acid, and ammonia (Lamers et al., 2014; Ueki et al., 2018). Applying ASD with orchard grass, wheat, and rice brans in strawberries has been found to alter the soil microbiome. This results in changes in the presence of Clostridium spp. and Bacillus spp. in the soil, reducing infections caused by Macrophomina phaseolina and Fusarium oxysporum f. sp. fragariae (Hewavitharana et al., 2021). Additionally, the mechanisms of ASD-mediated disease suppression were presumably due to the production of antifungal compounds such as volatile organic acids, hydrocarbons, and sulfur (Hewavitharana et al., 2021). These compounds and acids can suppress Verticillium dahliae in tomatoes and strawberries (Testen and Miller, 2018; Washburn, 2018; Testen et al., 2021). Similarly, Sclerotinia minor, Sclerotinia sclerotiorum, and root-knot nematodes suppressed entirely in muck soils treated with ASD (Sanabria-Velazquez, 2018). Recent studies have shown that certain microbes, like Clostridia and Zopfiella, found in soil treated with ASD, could reduce disease occurrence (Momma, 2008; Ueki et al., 2017; Liu et al., 2019). This suggests a specific microbiome may be essential for suppressing disease through ASD.
2.4 Impact of ASD on nematode suppression
There has been limited research on how ASD affects nematode suppression in soil. Meloidogyne incognita extracted from tomato root tissue and root gall ratings were generally low in all ASD using cover crop, carbon source, molasses C source, or composted poultry litter (Butler et al., 2012b). However, ASD combined with soil solarization reduced tomato root-knot nematode populations (Butler et al., 2012a). Another study examined the use of ASD with composted poultry litter and two rates of molasses in Immokalee and Citra, Florida (Di Gioia et al., 2016). ASD effectively suppressed root-knot nematode (Meloidogyne spp.) in both locations. The depth of the soil significantly influenced the effectiveness of ASD. In deep and shallow soil profiles, nematode populations decreased by 82% and 70%, respectively (Shrestha et al., 2016). However, at a moderate depth, there was a near-significant increase in nematode populations following ASD treatment. They found that an incubation period of less than two weeks significantly increased nematode survival, whereas an incubation period of 4 to 6 weeks significantly suppressed the nematode population (Shrestha et al., 2016). Although both liquid and solid carbon sources equally suppressed nematode populations, not mixing carbon sources was more effective than combining those (Shrestha et al., 2016). In Nepal, ASD with rice bran reduced root-knot severity in okra and eggplant less effectively than mustard cake and molasses (Khadka et al., 2019).
The efficacy of ASD with cover crops for their susceptibility to three common root-knot nematodes, Meloidogyne arenaria, M. incognita, and M. javanica, was tested on tomato cv. ‘Rutgers,’ a susceptible host (Kokalis-Burelle et al., 2013). They found that most cover crops tested were less susceptible to M. arenaria than tomatoes. Additionally, these cover crops did not support high populations of M. incognita in roots. Among the cover crops, arugula, cowpea, and sorghum-sudangrass were found to have low levels of all three Meloidogyne spp (Butler et al., 2012a, b). However, the susceptibility of other crops varied, depending on the specific species of Meloidogyne spp. tested (Kokalis-Burelle et al., 2013). In general, the microbial community shifts toward facultative and obligate anaerobes, the anaerobic decomposition of labile carbon sources produces short-chain organic acids (such as acetic, n-butyric, and propionic) and volatile compounds. These compounds are likely toxic to plant-parasitic nematodes (Momma et al., 2006; Momma, 2008).
The Pratylenchus penetrans density in apple roots was reduced based on the carbon source used for ASD (Hewavitharana et al., 2014). The nematicidal activity of acetic and n-butyric acids, both generated by ASD using wheat bran, was nearly equal, or additive (Katase et al., 2009). Notably, volatile fatty acids (VFAs) increased nematicidal activity as the pH decreased, suggesting their effectiveness is closely tied to their ionization (Katase et al., 2009). These findings underscore the crucial role of VFAs produced by the soil microbiome in low-oxygen soil in suppressing Meloidogyne incognita (Momma et al., 2006; Katase et al., 2009). ASD with ethanol can lead to the accumulation of acetic acids (Momma et al., 2010).
Plant-parasitic potato cyst nematodes (PCN) can be managed through ASD, with over 99.5% inactivation achieved in various soils (Runia et al., 2014). This high efficacy was attributed to the absence of organic matter. The use of ‘arugula’ could serve as a trap crop for Meloidogyne spp. (Melakeberhan et al., 2006) and may be more economically feasible than cover crops in rotations immediately preceding ASD treatments (Kokalis-Burelle et al., 2013).
2.5 Impact of ASD on weeds
Integrating ASD with cover crops can be an effective weed management strategy (Khadka et al., 2021). The effectiveness of ASD also depends on its ability to suppress weeds by accelerating the decomposition of organic matter, producing toxic acids, inhibiting seed respiration, and altering soil conditions to kill weed propagules (Fujita et al., 2020). When combined with carbon sources under anaerobic conditions, ASD has been shown to reduce weed populations by up to 95% (Singh et al., 2022). ASD treatment can eliminate weeds such as creeping yellow-field cress and common chickweed, although the overall weed population may remain unaffected (Molendijk et al., 2009; Shennan et al., 2018; Vincent et al., 2024). In. organic farms, ASD was effective against snow thistle (Molendijk et al., 2009). Although ASD is generally effective in weed control, the control of annual weeds such as Amaranthus retroflexus with ASD can vary. For instance, low success rates or outright failure have been reported, especially when using rice bran or molasses for ASD (Karimmojeni et al., 2014).
2.6 Impact of ASD on plant health and crop yield
The formation of soil aggregation and pore spaces contribute to improved root development, nutrient absorption, and plant health (Priyashantha and Attanayake, 2021; Lopes et al., 2022; Hu et al., 2023). In Ohio, ASD with wheat bran significantly increased tomato plants’ dry shoot and root biomasses compared to ASD with molasses and ethanol (Testen and Miller, 2018). In China, ASD with composted chicken manure significantly increased strawberry plants’ height, stem thickness, and yield (Song et al., 2020). ASD did not reduce the total fruit yield of crops compared to a fumigant control. However, the yield was significantly higher than a non-mended control (Shrestha et al., 2016). Furthermore, the application of manures and increased amendment rate led to an increased yield of over 50% compared to fumigated and non-amended controls (Shrestha et al., 2016).
ASD triggers several positive effects to suppress plant pathogens through specific beneficial bacterial and fungal communities, particularly those that produce antimicrobial substances and siderophores and may produce plant growth-promoting phytohormones and improve soil fertility (Lopes et al., 2022). These microbiomes compete for resources, produce antibiotics, and exhibit parasitic behavior, strengthening the soil’s defense against soilborne pathogens (Zhang et al., 2022). ASD can reduce soilborne pathogen, Thielaviopsis basicola, which can lead to increased biomass yield in tobacco varieties (Haji and Brandle, 2001). Soilborne diseases such as Verticillium wilt and charcoal rot can significantly reduce strawberry yields (Carpenter et al., 2000; Hewavitharana et al., 2021). However, ASD-amended soils showed significantly greater fresh strawberry biomass than non-treated controls in a study involving wilt and charcoal rot. Specifically, in the Fusarium wilt trial, ASD-amended plants exhibited twice the fresh biomass compared to the control (Rosskopf et al., 2015; Shennan et al., 2018; Hewavitharana et al., 2021).
When ASD with molasses was combined with solarization, the ASD plots produced equal or higher marketable bell pepper and eggplant yields than methyl bromide fumigation plots (Butler et al., 2014). The effect of ASD using a combination of composted poultry litter (22 t/ha) and two different levels of molasses (13.9 t/ha and 27.7 t/ha) on fresh-market tomato yields was assessed in two locations: Immokalee and Citra, Florida (Di Gioia et al., 2016). They found that in Immokalee, the ASD plots produced more marketable tomatoes compared to the soil fumigation plots. In contrast, the soil treatments did not affect the yield in Citra (Di Gioia et al., 2016). Additionally, soil treatments did not significantly influence the quality of the tomato fruits, although fruit mineral content was similar or higher in ASD plots than in soil fumigation plots (Di Gioia et al., 2016). The ASD treatments reduced redox potential (Eh) and lowered pH (Momma, 2008). This may significantly influence the soilborne plant pathogens and the entire soil-microbiome-plant system (Strauss and Kluepfel, 2015; Van Agtmaal et al., 2015).
Soil fumigation effectively suppressed nematodes in resistant tomato cultivars, increased tomato fruit yield (Regmi and Desaeger, 2020), and improved marketability (Theis and Fery, 2002). In contrast, ASD has less impact on managing soil nematode than resistant cultivars grown in non-fumigated soil (Gullino et al., 2022). An alternative method to chemical control involves using chitin, ASD, and marigolds. These strategies were effective in managing plant-parasitic nematodes and Verticillium dahliae, and increased yield (65%) over six years (Korthals et al., 2014).
3 Mechanisms of ASD
Although the mechanisms of soilborne plant pathogen suppression by ASD are not fully understood, available evidence suggests that ASD alters soil microbial communities through three primary mechanisms: (i) the decomposition of carbon sources that enhance beneficial microbiomes, (ii) the production of antimicrobial compounds or antagonism by anaerobic microbiomes, and (iii) the absence of oxygen (Mowlick et al., 2013a; Mowlick et al., 2014; Strauss and Kluepfel, 2015; Van Agtmaal et al., 2015; Priyashantha and Attanayake, 2021). Consequently, ASD effectively reduces the persistence of pathogen inoculums in the soil and reduces infections by soilborne pathogens and pests (Table 2).
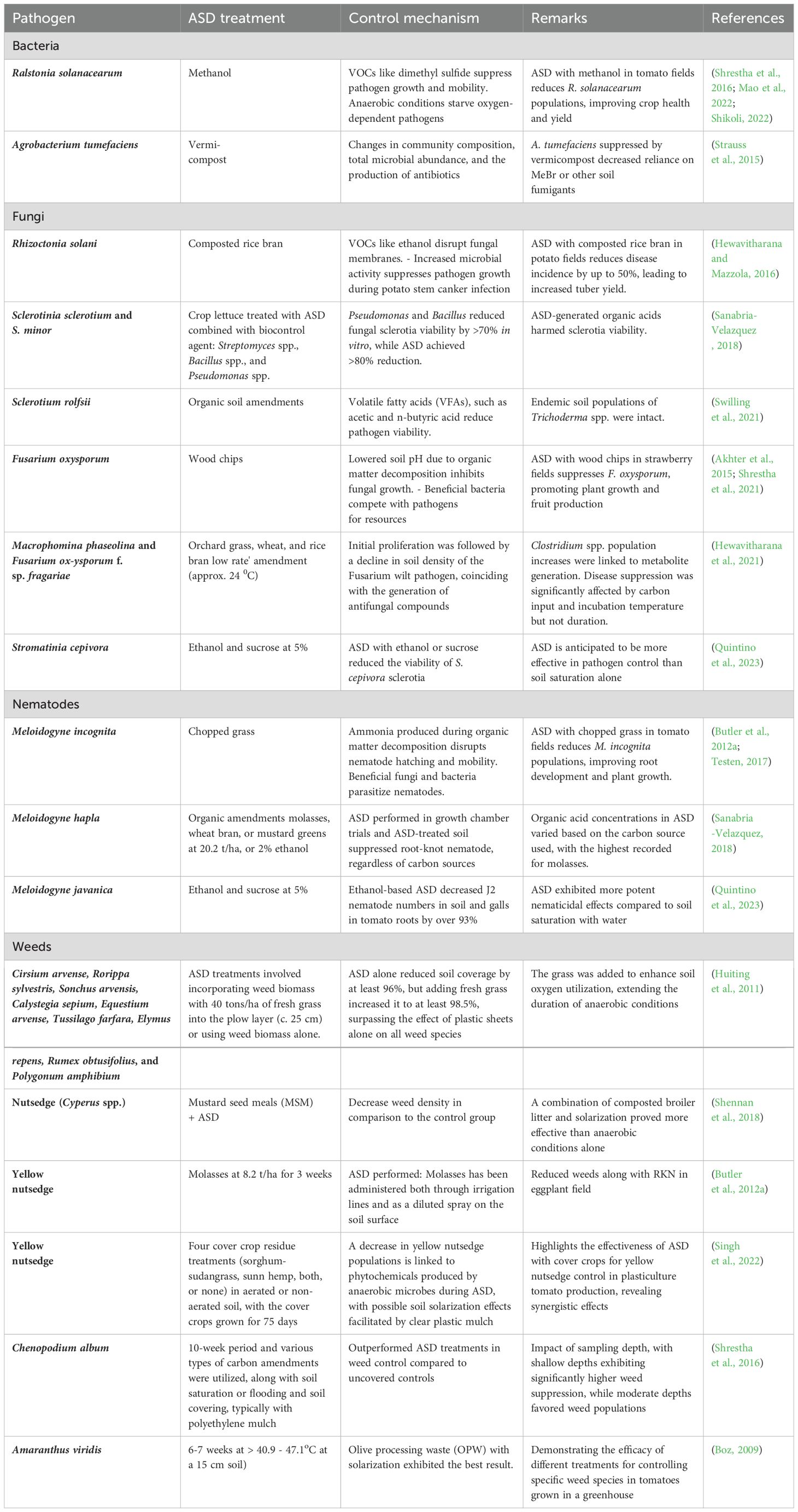
Table 2. Impact and mechanisms of anaerobic soil disinfestation (ASD) using various organic amendments as a carbon source on suppression of specific soilborne pathogens and pests.
The impact of ASD using various carbon sources in anaerobic processes to manage soilborne pests (Ludeking et al., 2010; Arriaga et al., 2011; Lamers et al., 2014; Runia et al., 2014). These specific factors are low oxygen levels, accumulation of toxic products from anaerobic decomposition such as organic acids, methyl sulfides, CO2, NH3, H2S, CH4, and N2O, and antagonism by anaerobic microbiomes (Momma et al., 2006; Runia et al., 2014; Shennan et al., 2014; Momma, 2015; Guo et al., 2017; Hewavitharana et al., 2019). The oxygen concentration in the soil decreases to below 1% within the first few hours after covering it with impermeable plastic, and this low oxygen level persists throughout the treatment period (Hewavitharana et al., 2019). Consequently, a decrease in soil redox potential (Eh) reduces the levels of oxygen, nitrate (NO3−), manganese ion (Mn4+), ferric ion (Fe3+), and sulfate (SO4) and even allows for methane production. Additionally, atmospheric conditions of O2 concentration < 4% and CO2 > 19.2% favor the production of organic acids acetate and butyrate (Runia et al., 2014).
Prokaryotes, specifically Clostridium spp., degrade the fungal cell wall, resulting in biological control of the spinach wilt pathogen (Ueki et al., 2017). Researchers examined the use of cover crops in ASD (Butler et al., 2012b; Hewavitharana et al., 2014); however, their effectiveness in muck soil systems is still unknown. In this context, cover crops could potentially be used as trap crops to stimulate the germination of Plasmodiophora brassicae (club root disease) resting spores before ASD (Friberg et al., 2006). ASD using mustard seed meal releases antifungal volatile compounds upon the hydrolysis of glucosinolates (Dassanayaka et al., 2023). Gases produced during the anaerobic phase from soils treated with ASD with ethanol, grass residues, or Brassica juncea seed meal effectively inhibited the growth of Rhizoctonia solani AG-5, Pythium ultimum, and Fusarium oxysporum (Dassanayaka et al., 2023; Hewavitharana et al., 2014). Furthermore, several volatile compounds, including dimethyl sulfide, carbon disulfide, di- and dimethyl tri-sulfide, are produced by the soil microbiome during the anaerobic phase of ASD (Hewavitharana et al., 2014).
Some of the sulfide compounds, such as dimethyl trisulfide, have been reported to have antifungal properties (Blok et al., 2000; Fernando et al., 2005) and nematicidal activities (Cabrera et al., 2009). Specific organic acetic acid and butyric acid were produced, reducing the survival of Fusarium oxysporum f. sp. lycopersici and Ralstonia solanacearum populations in soil (Momma et al., 2006). Changes in soil pH and nutrient availability also create an unfavorable environment for specific pathogens (Ebihara and Uematsu, 2014). In summary, the potential mechanisms for suppressing specific soilborne pests include producing organic acids through anaerobic decomposition of added carbon sources, releasing volatile fatty acids (VFAs), and biocontrol by the microbiome that thrives during the process (Momma et al., 2006; Shrestha et al., 2016).
4 Combination of ASD with biological control agents, fungicides, and grafting
When integrating ASD with other disease management tools, it is crucial to determine whether the effects are synergistic or antagonistic. Trichoderma spp. are beneficial fungi (Singh et al., 2014) and provide numerous benefits in crop production, such as promoting growth, suppressing diseases, improving soil quality, and enhancing nutrient availability in the soil (Harman et al., 2021; Singh et al., 2014). Soil-treated with ASD reduced the viability of Athelia rolfsii sclerotia in acidic soil conditions, with minimal impact on native Trichoderma spp (Swilling et al., 2021). Additionally, a significant increase of over 300% in Trichoderma spp. populations were reported in ASD-amended soil compared to non-amended soil. Available evidence suggests that Mucor spp. colonize sclerotia post-ASD treatment, presumably due to the potential mycoparasitic role of Mucor spp. against Sclerotinia sclerotiorum (Harvey et al., 1995).
Certain Trichoderma strains exhibit average growth at high temperatures (37 to 40°C), producing stress-protectant sugars such as trehalose, mannose, and raffinose, which help them thrive in extreme conditions (Poosapati et al., 2014). In Nepal, Khadka and Miller (2021) found that combined ASD with wheat bran, molasses, chicken manure, and mustard greens, along with Trichoderma harzianum T22 and Trichoderma asperellum NT25 achieved the lowest severity and incidence of Rhizoctonia root rot in radish plants. They observed that combining Trichoderma harzianum T22 with ASD using molasses and Trichoderma asperellum NT25 with ASD-wheat bran showed a synergistic effect in reducing disease severity (Khadka and Miller, 2021). Conversely, Trichoderma harzianum T22 with ASD-chicken manure showed an antagonistic interaction (Khadka and Miller, 2021).
ASD treatments were compared with fungicides and found to have significant impacts on managing Stevia stem rot and Septoria leaf spot (Sanabria-Velazquez et al., 2023). ASD effectively reduced the viability of Sclerotium rolfsii sclerotia in laboratory tests and micro-plot in North Carolina. In contrast, in Mexico and Paraguay, the viability of the sclerotia decreased significantly in soils treated with ASD using cornmeal plus molasses or wheat bran plus molasses in field trials (Sanabria-Velazquez et al., 2023). Combining ASD with grafting could be a practical disease management tool for specific soilborne disease complexes of tomatoes. For instance, in Ohio, ASD using wheat bran and molasses was used along with grafting tomato plants onto ‘Maxifort’-resistant rootstock to manage soilborne diseases such as corky root (caused by Pyrenochaeta lycopersici), black dot root rot (Colletotrichum coccodes), Verticillium wilt (Verticillium dahliae), and root-knot nematodes (Meloidogyne hapla and M. incognita) (Testen et al., 2021). The ASD treatment generally reduced the severity of root and taproot rot and root-knot galling. Non-grafted tomato plants grown in untreated soils showed the highest corky root rot severity, while the lowest levels were observed in plants grafted with ‘Maxifort’ rootstock (Testen, 2017; Testen et al., 2021).
5 Challenges and opportunities
ASD is a biological method that reduces the need for chemical fumigation and promotes sustainability (Van Agtmaal et al., 2015). However, the effectiveness of ASD can vary depending on several factors, such as soil types, temperatures, target soilborne pests, and carbon sources (Butler et al., 2012a; Zavatta et al., 2014; Rosskopf et al., 2015; Hewavitharana and Mazzola, 2016; Hewavitharana et al., 2021). Despite its benefits, ASD also presents challenges, especially in managing weeds and plant-parasitic nematodes at greater soil depths. Dry organic amendments used in ASD are tilled to a depth of 15 to 20 cm, which may not effectively manage soil nematodes. For instance, in Central California tree-crop nurseries, nematodes have been found to migrate from depths of over 1.5 m, exceeding the nematode management depth achieved by ASD in fields (McKenry, 2002; Zavatta et al., 2014).
When implementing ASD, selecting a suitable carbon source is crucial. Familiar carbon sources for ASD include cereal barns such as rice and wheat bran, mustard meal, animal manures, and other carbon-rich amendments (Priyashantha and Attanayake, 2021; Lopes et al., 2022). However, one significant limitation of using ASD is the high associated production costs. Thus, the cost-benefit ratio of the ASD application for soilborne pest management in fields is a critical consideration. For instance, the cost of ASD technology can be high due to the use of large quantities of carbon sources and costs of transportation, increased labor wages, application of expensive virtually impermeable plastic films (VIF), and irrigation facilities, thus making it less accessible for growers to adopt ASD (Muramoto et al., 2020; Daugovish et al., 2021, 2023). Alternatively, using locally available and renewable organic amendments or agricultural bio-products could be cheaper and reduce production costs. Recent studies have shown that carbon sources such as tomato pomace and Gramineae used for ASD were effective and altered the soil microbiome and metabolome (Poret-Peterson et al., 2019; Hewavitharana et al., 2021; Daugovish et al., 2023). Additionally, locally sourced agricultural by-products such as almond hulls, sweet potato waste, brewer’s spent grains, grape pomace, and orchard grass may offer promising carbon source alternatives for ASD (Butler et al., 2012a; Zavatta et al., 2014; Hewavitharana et al., 2021; Adhikari et al., 2023).
Agriculture in tropical developing countries includes small-scale farming systems, multiple cropping patterns, high pathogen diversity, the impact of climate changes (e.g., drought, increased temperature), and lack of agricultural technologies. As a result, crop productivity in these countries is lower than in temperate countries (Priyashantha and Attanayake, 2021). Therefore, translational research should focus on finding biodegradable farm by-products as carbon sources and utilizing durable and low-cost plastic mulch for ASD in developing countries. Importantly, ASD will be more beneficial for high-value crops such as strawberries and tomatoes than low-value crops such as eggplant, okra, and spinach. Adopting ASD technology provides a significant income from pesticide-free products for resource-poor farmers and improves soil health. Recently, the ASD strategy has been contemplated as an IPM tool to manage soilborne pathogens of Stevia in Mexico and Paraguay (Sanabria-Velazquez et al., 2023) and mitigate weed, root-knot nematode and soilborne pathogens of vegetables in Nepal (Khadka et al., 2019; Khadka, 2021; Khadka and Miller, 2021; Khadka et al., 2021).
Adding carbon sources to the soil is a common practice in all ASD treatments and provides a substrate for microbial activities (Butler et al., 2012a, b; Daugovish et al., 2015; Rosskopf et al., 2015; Hewavitharana and Mazzola, 2016; Rosskopf et al., 2020; Hewavitharana et al., 2021). Different carbon sources in ASD can affect microbial community composition and diversity (Hewavitharana and Mazzola, 2016; Testen and Miller, 2018). For instance, Clostridium spp. play a significant role in ASD by producing by-products during anaerobic respiration (Mowlick et al., 2013a; Strauss and Kluepfel, 2015; Ueki et al., 2017). ASD treatments also increased the predominant bacterial phyla Proteobacteria, Actinobacteria, Firmicutes, and Bacteroidetes, while Bacillus, Burkholderia, Enterobacter, and Pseudomonas were the most common bacterial genera (Huang et al., 2015; Rosskopf et al., 2015; Hewavitharana and Mazzola, 2016; Huang et al., 2016; Poret-Peterson et al., 2019; Rosskopf et al., 2020; Hewavitharana et al., 2021). Some bacterial endophytes are plant growth-promoting rhizobacteria (PGPR) and may enhance plant growth by producing phytohormones, antibiotics, and siderophores (Lodewyckx et al., 2002).
Specific microbiome genes contributing to plant-beneficial microbe interactions are crucial to identify them (Finkel et al., 2017). Culturable beneficial bacterial and fungal strains can be used to determine their biological functions (Armanhi et al., 2016, 2018). This approach will involve isolating single colonies of strains or multiple strains to establish community-based strains (Oberhardt et al., 2015). In addition, utilizing ‘meta-omics’ approaches such as metagenomics, metabolomics, metatranscriptomics, and metaproteomics can aid in analyzing microbial diversity, composition, and regulatory co-occurrence networks (Aguiar-Pulido et al., 2016). This will also facilitate our understanding of the interplay between plant-microbiome interactions. Additionally, engineering synthetic communities (SynComs) can improve plant health and activate the plant’s immune system to combat plant pathogens. This translation research aims to shift from using chemicals to advancing a novel, eco-friendly, biologically-based pest management (BBPM).
In conclusion, ASD offers promising opportunities for sustainable agriculture, but it also presents challenges and limitations that must be addressed for widespread adoption. Continued research and innovation are crucial for maximizing ASD’s potential in diverse agricultural production systems in developed and developing countries.
Author contributions
SM: Methodology, Writing – original draft, Writing – review & editing. ANP: Writing – review & editing. TBA: Conceptualization, Software, Writing – original draft, Writing – review & editing.
Funding
The author(s) declare financial support was received for the research, authorship, and/or publication of this article. AP was supported by the USDA-NIFA Award No. 2023-51102-40782.
Conflict of interest
The authors declare that the research was conducted in the absence of any commercial or financial relationships that could be construed as a potential conflict of interest.
The author(s) declared that they were an editorial board member of Frontiers, at the time of submission. This had no impact on the peer review process and the final decision.
Publisher’s note
All claims expressed in this article are solely those of the authors and do not necessarily represent those of their affiliated organizations, or those of the publisher, the editors and the reviewers. Any product that may be evaluated in this article, or claim that may be made by its manufacturer, is not guaranteed or endorsed by the publisher.
References
Adhikari T. B., Philbrick A., Louws F. J. (2023). Deciphering the active strawberry microbiome associated with roots and rhizosphere soil. 12th International Society of Plant Pathology at the satellite event Phytobiomes Research for Plant Health, Lyon, France, August 19–25. 2023.
Aguiar-Pulido V., Huang W., Suarez-Ulloa V., Cickovski T., Mathee K., Narasimhan G. (2016). Metagenomics, metatranscriptomics, and metabolomics approaches for microbiome analysis. Evol. Bioinf. 12, 5–16. doi: 10.4137/EBO.S36436
Akhter A., Hage-Ahmed K., Soja G., Steinkellner S. (2015). Compost and biochar alter mycorrhization, tomato root exudation, and development of Fusarium oxysporum f. sp. lycopersici. Front. Plant Sci. 6, 146969. doi: 10.3389/fpls.2015.00529
Armanhi J. S., de Souza R. S., de Araújo L. M., Okura V. K., Mieczkowski P., Imperial J., et al. (2016). Multiplex amplicon sequencing for microbe identification in community-based culture collections. Sci. Rep. 6, 295. doi: 10.1038/srep29543
Armanhi J. S. L., de Souza R. S. C., Damasceno N. B., de Araújo L. M., Imperial J., Arruda P. (2018). A community-based culture collection for targeting novel plant growth-promoting bacteria from the sugarcane microbiome. Front. Plant Sci. 8, 2191. doi: 10.3389/fpls.2017.02191
Arriaga H., Núñez-Zofio M., Larregla S., Merino P. (2011). Gaseous emissions from soil biodisinfestation by animal manure on a greenhouse pepper crop. Crop Prot. 30, 412–419. doi: 10.1016/j.cropro.2010.12.012
Bhandari A., Sah L. P., Devkota M., Rajbhandari B. P. (2020). Evaluation of anaerobic soil disinfestations in the management of club root disease. J. Nep. Agric. Res. 19, 5–11.
Blok W. J., Lamers J. G., Termorshuizen A. J., Bollen G. J. (2000). Control of soilborne plant pathogens by incorporating fresh organic amendments followed by tarping. Phytopathology 90, 253–259. doi: 10.1094/PHYTO.2000.90.3.253
Boz Ö. (2009). Effects of olive processing waste, chicken manure and Dazomet on weeds with or without soil solarization. Afr. J. Biotechnol. 8, 4946–4952.
Butler D. M., Kokalis-Burelle N., Muramoto J., Shennan C., McCollum T. G., Rosskopf E. N. (2012a). Impact of anaerobic soil disinfestation combined with soil solarization on plant–parasitic nematodes and introduced inoculum of soilborne plant pathogens in raised-bed vegetable production. Crop Prot. 39, 33–40. doi: 10.1016/j.cropro.2012.03.019
Butler D. M., Ownley B. H., Dee M. E., Eichler Inwood S. E., McCarty D. G., Shrestha U., et al. (2014). Low carbon amendment rates during anaerobic soil disinfestation (ASD) at moderate soil temperatures do not decrease viability of Sclerotinia sclerotiorum sclerotia or Fusarium root rot of common bean. Acta Hortic. 1044, 203–208. doi: 10.17660/ActaHortic.2014.1044.23
Butler D. M., Rosskopf E. N., Kokalis-Burelle N., Albano J. P., Muramoto J., Shennan C. (2012b). Exploring warm-season cover crops as carbon sources for anaerobic soil disinfestation (ASD). Plant Soil 355, 149–165. doi: 10.1007/s11104-011-1088-0
Cabrera J. A., Kiewnick S., Grimm C., Dababat A. A., Sikora R. A. (2009). Efficacy of abamectin seed treatment on Pratylenchus zeae, Meloidogyne incognita and Heterodera schachtii. J. Plant Dis. Prot. 116, 124–128. doi: 10.1007/BF03356298
Carpenter J. E., Gianessi L. P., Lynch L. (2000). The economic impact of the scheduled US phaseout of methyl bromide (Washington DC: National Center for Food and Agricultural Policy), 70–137.
Chen L., Jiang Y., Liang C., Luo Y., Xu Q., Cheng H., et al. (2019). Competitive interaction with keystone taxa induced negative priming under biochar amendments. Microbiome 7, 77. doi: 10.1186/s40168-019-0693-7
Christakopoulos P., Macris B. J., Kekos D. (1989). Direct fermentation of cellulose to ethanol bynFusarium oxysporum. Enzyme Microb. Technol. 11, 236–239. doi: 10.1016/0141-0229(89)90098-7
Dassanayaka M. P., Casonato S. G., Jones E. E. (2023). In vitro inhibition of Sclerotinia sclerotiorum mycelial growth and reduction of sclerotial viability by the volatile bioactive compounds of Brassicaceae crops. J. Appl. Microbiol. 134 (12), ad289. doi: 10.1093/jambio/lxad289
Daugovish O., Muramoto J., Shennan C., Zavatta M. (2021). Plant-derived carbon sources for anaerobic soil disinfestation in Southern California. Glob. J. Agric. Innov. Res. Dev. 8, 169–175. doi: 10.15377/2409-9813.2021.08.13
Daugovish O., Shennan C., Muramoto J., Mazzola M. (2015). “Carbon source and irrigation affect anaerobic soil disinfestation in strawberry,” in Proceedings of Annual International Research Conference on Methyl Bromide Alternatives, San Diego, CA, 12.1–12.3.
Daugovish O., Valdes-Berriz M., Muramoto J., Shennan C., Zavatta M., Henry P. (2023). Carbon sources for anaerobic soil disinfestation in Southern California strawberry. Agronomy 13, 1635. doi: 10.3390/agronomy13061635
Di Gioia F., Ozores-Hampton M., Hong J., Kokalis-Burelle N., Albano J., Zhao X., et al. (2016). The effects of anaerobic soil disinfestation on weed and nematode control, fruit yield, and quality of Florida fresh-market tomato. HortSci 51, 703–711. doi: 10.21273/HORTSCI.51.6.703
Di Gioia F., Ozores-Hampton M., Zhao X., Thomas J., Wilson P., Li Z., et al. (2017). Anaerobic soil disinfestation impact on soil nutrients dynamics and nitrous oxide emissions in fresh-market tomato. Agric. Ecosyst. Environ. 240, 194–205. doi: 10.1016/j.agee.2017.02.025
Ebihara Y., Uematsu S. (2014). Survival of strawberry-pathogenic fungi Fusarium oxysporum f. sp. fragariae, Phytophthora cactorum and Verticillium dahliae under anaerobic conditions. J. Gen. Plant Pathol. 80, 50–58. doi: 10.1007/s10327-013-0476-0
Fernando W. D., Ramarathnam R., Krishnamoorthy A. S., Savchuk S. C. (2005). Identification and use of potential bacterial organic antifungal volatiles in biocontrol. Soil Biol Biochem. 37 (5), 955–964.
Finkel O. M., Castrillo G., Herrera Paredes S., González I. S., Dangl J. L. (2017). Understanding and exploiting plant-beneficial microbes. Curr. Opin. Plant Biol. 38, 155–163. doi: 10.1016/j.pbi.2017.04.018
Friberg H., Lagerlöf J., Rämert B. (2006). Usefulness of nonhost plants in managing Plasmodiophora brassicae. Plant Pathol. 55, 690–695. doi: 10.1111/j.1365-3059.2006.01408.x
Fujita K., Kunito T., Otsuka S., Nagaoka K. (2020). Anaerobic soil disinfestation using diluted ethanol increases phosphorus availability in arable Andosols. Biol. Fertil. Soils 56, 927–941. doi: 10.1007/s00374-020-01472-x
Garcia Gonzalez J. F. (2021). Investigating management alternatives for Southern blight on vegetables in the mid-Atlantic United States. (Blacksburg, VA: Virginia Tech Universality), 122. Available at: http://hdl.handle.net/10919/112654.
Gullino M. L., Garibaldi A., Gamliel A., Katan J. (2022). Soil disinfestation: From soil treatment to soil and plant health. Plant Dis. 106, 1541–1554. doi: 10.1094/PDIS-09-21-2023-FE
Guo H., Di Gioia F., Zhao X., Ozores-Hampton M., Swisher M. E., Hong J., et al. (2017). Optimizing anaerobic soil disinfestation for fresh market tomato production: nematode and weed control, yield, and fruit quality. Sci. Hortic. 218, 105–116. doi: 10.1016/j.scienta.2017.01.054
Guo H., Zhao X., Rosskopf E. N., Di Gioia F., Hong J. C., McNear D. H. Jr. (2018). Impacts of anaerobic soil disinfestation and chemical fumigation on soil microbial communities in field tomato production system. Appl. Soil Ecol. 126, 165–173. doi: 10.1016/j.apsoil.2017.12.018
Haji H. M., Brandle J. E. (2001). Evaluation of host resistance and soil fumigation for the management of black root rot of tobacco in Ontario. Plant Dis. 85, 1145–1148. doi: 10.1094/PDIS.2001.85.11.1145
Hanson B. D., Gao S., Gerik J. S., Shrestha A., Qin R., McDonald J. A. (2011). Effects of emission reduction surface seal treatments on pest control with shank-injected 1, 3-dichloropropene and chloropicrin. Crop Prot. 30, 203–207. doi: 10.1016/j.cropro.2010.10.011
Hanson C. A., Allison S. D., Bradford M. A., Wallenstein. M. D., Treseder K. K. (2008). Fungal taxa target different carbon sources in forest soil. Ecosystems 11, 1157–1167. doi: 10.1007/s10021-008-9186-4
Harman G. E., Doni F., Khadka R. B., Uphoff N. (2021). Endophytic strains of Trichoderma increase plants’ photosynthetic capability. J. Appl. Microbiol. 130, 529–546. doi: 10.1111/jam.14368
Harvey I. C., Foley L. M., Saville D. J. (1995). Survival and germination of shallow-buried sclerotia of Sclerotinia sclerotiorum in pastures in Canterbury. New Z. J. Agric. Res. 38, 279–284.
Hewavitharana S. S., Mazzola M. (2016). Carbon source-dependent effects of anaerobic soil disinfestation on soil microbiome and suppression of Rhizoctonia solani AG-5 and. Pratylenchus penetrans. Phytopathol. 106, 1015–1028. doi: 10.1094/PHYTO-12-15-0329-R
Hewavitharana S. S., Klarer E., Muramoto J., Shennan C., Mazzola M. (2021). Analysis of environmental variables and carbon input on soil microbiome, metabolome and disease control efficacy in strawberry attributable to anaerobic soil disinfestation. Microorganisms 9, 1638. doi: 10.3390/microorganisms9081638
Hewavitharana S. S., Klarer E., Reed A. J., Leisson R., Poirier B., Honaas L., et al. (2019). Temporal dynamics of the soil metabolome and microbiome during simulated anaerobic soil disinfestation. Front. Microbiol. 10, 2365. doi: 10.3389/fmicb.2019.02365
Hewavitharana S. S., Ruddell D., Mazzola M. (2014). Carbon source-dependent antifungal and nematicidal volatiles derived during anaerobic soil disinfestation. Eur. J. Plant Path. 140, 39–52. doi: 10.1007/s10658-014-0442-5
Hong J. C., Di Gioia F., Jones J. B., Turechek W., Kokalis-Burelle N., Johns C. W., et al. (2018). “Defining anaerobic soil disinfestation through changes in the microbiome,” in IX International Symposium on Soil and Substrate Disinfestation, Vol. 1270. 97–110.
Hu J., Wan L., Qasim W., Lv H., Zhao Y., Li G., et al. (2023). Anaerobic soil disinfestation promotes soil microbial stability and antagonistic bacteria abundance in greenhouse vegetable production systems. Agronomy 13, 939. doi: 10.3390/agronomy13030939
Huang X., Liu L., Wen T., Zhang J., Wang F., Cai Z. (2016). Changes in the soil microbial community after reductive soil disinfestation and cucumber seedling cultivation. Appl. Microbiol. Biotechnol. 100, 5581–5593. doi: 10.1007/s00253-016-7362-6
Huang X., Liu L., Wen T., Zhu R., Zhang J., Cai Z. (2015). Illumina MiSeq investigations on the changes of microbial community in the Fusarium oxysporum f. sp. cubense infected soil during and after reductive soil disinfestation. Microbiol. Res. 181, 33–42. doi: 10.1016/j.micres.2015.08.004
Huiting H. F., Bleeker P. O., Riemens M. M. (2011). “Control of perennial weeds by mechanical methods and anaerobic soil disinfection,” in 9th Workshop of the EWRS Working Group: Physical and Cultural Weed Control, Samsun, Turkey. pp, 15.
Husson O. (2013). Redox potential (Eh) and pH as drivers of soil/plant/microorganism systems: a transdisciplinary overview pointing to integrative opportunities for agronomy. Plant Soil 362, 389–417. doi: 10.1007/s11104-012-1429-7
Karimmojeni H., Bazrafshan A. H., Majidi M. M., Torabian S., Rashidi B. (2014). Effect of maternal nitrogen and drought stress on seed dormancy and germinability of Amaranthus retroflexus. Plant Species Biol. 29, E1–E8. doi: 10.1111/1442-1984.12022
Katan J. (2017). Diseases caused by soilborne pathogens: biology, management and challenges. J. Plant Pathol. 99, 305–315.
Katase M., Kubo C., Ushio S., Ootsuka E., Takeuchi T., Mizukubo T. (2009). Nematicidal activity of volatile fatty acids generated from wheat bran in reductive soil disinfestation. Nematol. Res. 39, 53–62. doi: 10.3725/jjn.39.53
Khadka R. B. (2021). Application of Nepalese Trichoderma spp. with anaerobic soil disinfestation (ASD) to control soilborne diseases and effect of ASD on weeds. A dissertation was presented in partial fulfillment of the requirements for the Doctor of Philosophy degree at the Graduate School of Ohio State University. (Columbus, OH: Ohio State University), 365.
Khadka R. B., Miller S. A. (2021). Synergy of anaerobic soil disinfestation and Trichoderma spp. in Rhizoctonia root rot suppression. Front. Sustain. Food Syst. 5, 645736. doi: 10.3389/fsufs.2021.645736
Khadka R. B., Cardina J., Miller S. A. (2021). Perspectives on anaerobic soil disinfestation for weed management. J. Inte. Pest Manage. 12, 1–11. doi: 10.1093/jipm/pmab027
Khadka R. B., Marasini M., Rawal R., Testen A. L., Miller S. A. (2019). Effects of anaerobic soil disinfestation carbon sources on soilborne diseases and weeds of okra and eggplant in Nepal. Crop Prot. 135, 104846. doi: 10.1016/j.cropro.2019.104846
Kokalis-Burelle N., Butler D. M., Rosskopf E. N. (2013). Evaluation of cover crops with potential for use in anaerobic soil disinfestation (ASD) for susceptibility to three species of Meloidogyne. J. Nematol. 45, 272.
Korthals G. W., Thoden T. C., Van den Berg W., Visser J. H. M. (2014). Long-term effects of eight soil health treatments to control plant-parasitic nematodes and Verticillium dahliae in agro-ecosystems. Appl. Soil Ecol. 76, 112–123. doi: 10.1016/j.apsoil.2013.12.016
Lamers J., Mazzola M., Rosskopf E. N., Kokalis-Burelle N., Momma N., Butler D. M., et al. (2014). “Anaerobic soil disinfestation for soil borne disease control in strawberry and vegetable systems: current knowledge and future directions,” in VIII International Symposium on Chemical and Non-Chemical Soil and Substrate Disinfestation, Vol. 1044. 165–175.
Lee C. G., Kunitomo E., Iida T., Nakaho K., Ohkuma M. (2020). Soil prokaryotes are associated with decreasing Fusarium oxysporum density during anaerobic soil disinfestation in the tomato field. Appl. Soil Ecol. 155, 103632. doi: 10.1016/j.apsoil.2020.103632
Liu J., Kong H., Cui J., Zhang F., Wang Z., Cai Z., et al. (2016). Relationships of decomposability and C/N ratio in different types of organic matter with suppression of Fusarium oxysporum and microbial communities during reductive soil disinfestation. Biol. Control 101, 103–113. doi: 10.1016/j.biocontrol.2016.06.011
Liu L., Huang X., Zhao J., Zhang J., Cai Z. (2019). Characterizing the key agents in a disease-suppressed soil managed by reductive soil disinfestation. Appl. Environ. Microbiol. 85 (7), e02992–18. doi: 10.1128/AEM.02992-18
Lodewyckx C., Vangronsveld J., Porteous F., Moore E. R., Taghavi S., Mezgeay M., et al. (2002). Endophytic bacteria and their potential applications. Crit. Rev. Plant Sci. 21, 583–606. doi: 10.1080/0735-260291044377
Lopes E. A., Canedo E. J., Gomes V. A., Vieira B. S., Parreira D. F., Neves W. S. (2022). Anaerobic soil disinfestation for the management of soilborne pathogens: A review. Appl. Soil Ecol. 174, 104408. doi: 10.1016/j.apsoil.2022.104408
Ludeking D. J. W., Paternotte S. J., Runia W. T., Molendijk L. P. G. (2010). Biological soil disinfestation with organic fermentation products. Acta Hortic. 915, 133–139.
Mao Y., Hafeez A., Pan T., Wu C., Wang L., Muramoto J., et al. (2022). Suppression of tomato bacterial wilt by anaerobic soil disinfestation and associations with production of antagonistic compounds. Plant Soil. 477, 539–552. doi: 10.1007/s11104-022-05452-y
Martin F. N. (2003). Development of alternatives strategies for management of soilborne pathogens currently controlled with methyl bromide. Annu. Rev. Phytopathol. 41, 325–350. doi: 10.1146/annurev.phyto.41.052002.095514
McKenry M. (2002). Field Evaluations/Input for grower replant settings and new lines of nematode resistance. Walnut Res. Rep., 509–516.
Melakeberhan H., Xu A., Kravchenko A., Mennan S., Riga E. (2006). Potential use of arugula (Eruca sativa L.) as a trap crop for Meloidogyne hapla. Nematology 8 (5), 793–799.
Messiha N. A., van Diepeningen A. D., Wenneker M., van Beuningen A. R., Janse J. D., Coenen T. G., et al. (2007). Biological soil disinfestation (BSD), a new control method for potato brown rot, caused by Ralstonia solanacearum race 3 biovar 2. Eur. J. Plant Path. 117, 403–415. doi: 10.1007/s10658-007-9109-9
Miller E. J., Chibnall A. C. (1932). The proteins of grasses: Preliminary communication. Biochem. J. 26, 392–402. doi: 10.1042/bj0260392
Molendijk L. P. G., Bleeker P. O., Lamers J. G., Runia W. T. (2009). Perspectives of anaerobic soil disinfestation. Acta Hortic. 883, 277–283.
Momma N. (2008). Biological soil disinfestation (BSD) of soilborne pathogens and its possible mechanisms. Jpn. Agric. Res.Q. 42, 7–12. doi: 10.6090/jarq.42.7
Momma N. (2015). Studies on mechanisms of anaerobicity-mediated biological soil disinfestation and its practical application. J. Gen. Plant Pathol. 81, 480–482. doi: 10.1007/s10327-015-0612-0
Momma N., Kobara Y., Uematsu S., Kita N., Shinmura A. (2013). Development of biological soil disinfestations in Japan. Appl. Microbiol. Biotechnol. 97, 3801–3809. doi: 10.1007/s00253-013-4826-9
Momma N., Momma M., Kobara Y. (2010). Biological soil disinfestation using ethanol: effect on Fusarium oxysporum f. sp. lycopersici and soil microorganisms. J. Gen. Plant Pathol. 76, 336–344. doi: 10.1007/s10327-010-0252-3
Momma N., Yamamoto K., Simandi P., Shishido M. (2006). Role of organic acids in the mechanisms of biological soil disinfestation (BSD). J. Gen. Plant Pathol. 72, 247–252. doi: 10.1007/s10327-006-0274-z
Mowlick S., Inoue T., Takehara T., Kaku N., Ueki K., Ueki A. (2013a). Changes and recovery of soil bacterial communities influenced by biological soil disinfestation as compared with chloropicrin-treatment. AMB Express 3, 1–12. doi: 10.1186/2191-0855-3-46
Mowlick S., Inoue T., Takehara T., Tonouchi A., Kaku N., Ueki K., et al. (2014). Usefulness of Japanese-radish residue in biological soil disinfestation to suppress spinach wilt disease accompanying with proliferation of soil bacteria in the Firmicutes. Crop Prot. 61, 64–73. doi: 10.1016/j.cropro.2014.03.010
Mowlick S., Yasukawa H., Inoue T., Takehara T., Kaku N., Ueki K., et al. (2013b). Suppression of spinach wilt disease by biological soil disinfestation incorporated with Brassica juncea plants in association with changes in soil bacterial communities. Crop Prot. 54, 185–193. doi: 10.1016/j.cropro.2013.08.012
Muramoto J., Shennan C., Mazzola M., Wood T., Miethke E., Resultay E., et al. (2020). Use of a summer cover crop as a partial carbon source for anaerobic soil disinfestation in coastal California. Acta Hortic. 1270, 37–44. doi: 10.17660/ActaHortic.2020.1270.4
Nicolardot B., Recous S., Mary B. (2001). Simulation of C and N mineralisation during crop residue decomposition: a simple dynamic model based on the C: N ratio of the residues. Plant Soil 228, 83–103. doi: 10.1023/A:1004813801728
Oberhardt M. A., Zarecki R., Gronow S., Lang E., Klenk H. P., Gophna U., et al. (2015). Harnessing the landscape of microbial culture media to predict new organism–media pairings. Nat. Commun. 6, 8493. doi: 10.1038/ncomms9493
Panagiotou G., Christakopoulos P., Villas-Bôas S. G., Olsson L. (2005). Fermentation performance and intracellular metabolite profiling of Fusarium oxysporum cultivated on a glucose-xylose mixture. Enzyme Microb. Technol. 3, 100–106. doi: 10.1016/j.enzmictec.2004.07.009
Panth M., Hassler S. C., Baysal-Gurel F. (2020). Methods for management of soilborne diseases in crop production. Agriculture 10, 16. doi: 10.3390/agriculture10010016
Phalan B., Bertzky M., Butchart S. H. M., Donald P. F., Scharlemann J. P. W., Stattersfield A. J., et al. (2013). Crop expansion and conservation priorities in tropical countries. PloS One 8, 1–13. doi: 10.1371/journal.pone.0051759
Poosapati S., Ravulapalli P. D., Tippirishetty N., Vishwanathaswamy D. K., Chunduri S. (2014). Selection of high temperature and salinity tolerant Trichoderma isolates with antagonistic activity against Sclerotium rolfsii. Springerplus 3, 641. doi: 10.1186/2193-1801-3-641
Poret-Peterson A. T., Albu S., McClean A. E., Kluepfel D. A. (2019). Shifts in soil bacterial communities as a function of carbon source used during anaerobic soil disinfestation. Front. Environ. Sci. 6, 160. doi: 10.3389/fenvs.2018.00160
Poret-Peterson A. T., Sayed N., Glyzewski N., Forbes H., González-Orta E. T., Kluepfel D. A., et al. (2020). Temporal responses of microbial communities to anaerobic soil disinfestation. Microbial. Ecol. 80, 191–201. doi: 10.1007/s00248-019-01477-6
Preece C., Verbruggen E., Liu L., Weedon J. T., Peñuelas J. (2019). Effects of past and current drought on the composition and diversity of soil microbial communities. Soil Biol. Biochem. 131, 28–39. doi: 10.1016/j.soilbio.2018.12.022
Priyashantha A. H., Attanayake R. N. (2021). Can anaerobic soil disinfestation (ASD) be a game changer in tropical agriculture? Pathogens 10, 133. doi: 10.3390/pathogens10020133
Quintino A. N. A., Canedo E. J., Lopes E. A., Vieira B. S., Júnior V. L., Parreira D. F., et al. (2023). Anaerobic soil disinfestation with ethanol or sucrose reduces the viability of Meloidogyne javanica and Stromatinia cepivora. Acta Scientiarum Biol. Sci. 45, e64832. doi: 10.4025/actascibiolsci.v45i1.64832
Regmi H., Desaeger J. (2020). Integrated management of root-knot nematode (Meloidogyne spp.) in Florida tomatoes combining host resistance and nematicides. Crop Prot. 134, 105170.
Rokunuzzaman M., Hayakawa A., Yamane S., Tanaka S., Ohnishi K. (2016). Effect of soil disinfection with chemical and biological methods on bacterial communities. Egypt. J. Basic Appl. Sci. 3, 141–148. doi: 10.1016/j.ejbas.2016.01.003
Rosskopf E., Di Gioia F., Hong J. C., Pisani C., Kokalis-Burelle N. (2020). Organic amendments for pathogen and nematode control. Annu. Rev. Phytopathol. 58, 277–311. doi: 10.1146/annurev-phyto-080516-035608
Rosskopf E. N., Serrano-Pérez P., Hong J., Shrestha U., Rodríguez-Molina M. D. C., Martin K., et al. (2015). “Anaerobic soil disinfestation and soilborne pest management,” in Organic Amendments and Soil Suppressiveness in Plant Disease Management. Eds. Meghvansi M., Varma A. (Cham, Switzerland, Springer), 277–305.
Runia W. T., Thoden T. C., Molendijk L. P. G., Van Den Berg W., Termorshuizen A. J., Streminska M. A., et al. (2014). “Unravelling the mechanism of pathogen inactivation during anaerobic soil disinfestation,” in VIII International Symposium on Chemical and Non-Chemical Soil and Substrate Disinfestation, Vol. 1044. 177–193.
Saleem M., Hu J., Jousset A. (2019). More than the sum of its parts: microbiome biodiversity as a driver of plant growth and soil health. Annu. Rev. Ecol. Evol. Syst. 50, 145–168. doi: 10.1146/annurev-ecolsys-110617-062605
Sanabria-Velazquez A. D. (2018). Effects of anaerobic soil disinfestation combined with biological control on root-knot nematode and lettuce drop. A Thesis submitted to the Graduate School in partial fulfillment of the requirements for the Master of Science degree in Plant Pathology, the Ohio State University. (Columbus, OH: Ohio State University), 277.
Sanabria-Velazquez A. D., Enciso-Maldonado G. A., Maidana-Ojeda M., Diaz-Najera J. F., Ayvar-Serna S., Thiessen L. D., et al. (2023). Integrated pathogen management in Stevia using anaerobic soil disinfestation combined with different fungicide programs in the USA, Mexico, and Paraguay. Agronomy 13, 1358. doi: 10.3390/agronomy13051358
Serrano-Pérez P., Rosskopf E., De Santiago A., del Carmen Rodríguez-Molina M. (2017). Anaerobic soil disinfestation reduces survival and infectivity of Phytophthora nicotianae chlamydospores in pepper. Scientia Horticulturae 215, 38–48. doi: 10.1016/j.scienta.2016.12.003
Shennan C., Muramoto J., Koike S., Baird G., Fennimore S., Samtani J., et al. (2018). Anaerobic soil disinfestation is an alternative to soil fumigation for control of some soilborne pathogens in strawberry production. Plant Pathol. 67, 51–66. doi: 10.1111/ppa.12721
Shennan C., Muramoto J., Lamers J., Mazzola M., Rosskopf E. N., Kokalis-Burelle N., et al. (2014). Anaerobic soil disinfestation for soilborne disease control in strawberry and vegetable systems: current knowledge and future directions. Acta Hortic. 1044, 165–175. doi: 10.17660/ActaHortic.2014.1044.20
Shikoli E. M. (2022). Influence of bunching onion (Allium fistulosum) crude extract and irrigation levels on bacterial wilt incidence and tomato (Solanum lycopersicum l.) growth, yield, and quality. A thesis was submitted to the graduate school in partial fulfillment of the requirements for the Master of Science degree in Horticulture. (Kenya: Egerton University) 87.
Shrestha U., Augé R. M., Butler D. M. (2016). A meta-analysis of the impact of anaerobic soil disinfestation on pest suppression and yield of horticultural crops. Front. Plant Sci. 7, 203107. doi: 10.3389/fpls.2016.01254
Shrestha U., Ownley B. H., Bruce A., Rosskopf E. N., Butler D. M. (2021). Anaerobic soil disinfestation efficacy against Fusarium oxysporum is affected by soil temperature, amendment type, rate, and C: N ratio. Phytopathology 111, 1380–1392. doi: 10.1094/PHYTO-07-20-0276-R
Shu X., He J., Zhou Z., Xia L., Hu Y., Zhang Y., et al. (2022). Organic amendments enhance soil microbial diversity, microbial functionality and crop yields: A meta-analysis. Sci. Total Environ. 829, 154627. doi: 10.1016/j.scitotenv.2022.154627
Singh A., Sarma B. K., Singh H. B., Upadhyay R. S. (2014). “Trichoderma: a silent worker of plant rhizosphere,” in Biotechnology and Biology of Trichoderma. Eds. Gupta V. K., Schmoll M., Herrera-Estrella A., Upadhyay R. S., Druzhinina I., Tuohy M. G. (Elsevier, Amsterdam), 533–542.
Singh G., Ward B., Levi A., Cutulle M. (2022). Weed management by in situ cover crops and anaerobic soil disinfestation in plasticulture. Agronomy 12, 2754. doi: 10.3390/agronomy12112754
Singh T. P., Sogi D. S. (2016). Inhibition of lipase activity in commercial rice bran of coarse, fine, and superfine cultivars. Cogent Food Agric. 2, 1146055. doi: 10.1080/23311932.2016.1146055
Song Z., Massart S., Yan D., Cheng H., Eck M., Berhal C., et al. (2020). Composted chicken manure for anaerobic soil disinfestation increased the strawberry yield and shifted the soil microbial communities. Sustainability 12, 1–15. doi: 10.3390/su12166313
Spietz R. L., Williams C. M., Rocap G., Horner-Devine M. C. (2015). A dissolved oxygen threshold for shifts in bacterial community structure in a seasonally hypoxic estuary. PloS One 10, e0135731. doi: 10.1371/journal.pone.0135731
Stefan L., Hartmann M., Engbersen N., Six J., Schöb C. (2021). Positive effects of crop diversity on productivity driven by changes in soil microbial composition. Front. Microbiol. 12, 660749. doi: 10.3389/fmicb.2021.660749
Strauss S. L., Kluepfel D. A. (2015). Anaerobic soil disinfestation: A chemical-independent approach to pre-plant control of plant pathogens. J. Int. Agric. 14, 2309–2318. doi: 10.1016/S2095-3119(15)61118-2
Strauss S. L., Greenhut R. F., McClean A. E., Kluepfel D. A. (2017). Effect of anaerobic soil disinfestation on the bacterial community and key soilborne phytopathogenic agents under walnut tree-crop nursery conditions. Plant Soil 415, 493–506. doi: 10.1007/s11104-016-3126-4
Strauss S. L., Stover J. K., Kluepfel D. A. (2015). Impact of biological amendments on Agrobacterium tumefaciens survival in soil. Appl. Soil Ecol. 87, 39–48. doi: 10.1016/j.apsoil.2014.10.016
Swilling K. J., Shrestha U., Ownley B. H., Gwinn K. D., Butler D. M. (2021). Mechanisms of anaerobic soil disinfestation: volatile fatty acids reduce the viability of Athelia (Sclerotium) rolfsii sclerotia in acidic soil conditions and have limited effects on endemic Trichoderma spp. Front. Sustain. Food Syst. 5, 747176. doi: 10.3389/fsufs.2021.747176
Tan X., Liao H., Shu L., Yao H. (2019). Effect of different substrates on soil microbial community structure and the mechanisms of reductive soil disinfestation. Front. Microbiol. 10, 2851. doi: 10.3389/fmicb.2019.02851
Testen A. L. (2017). Participatory research to improve soil and plant health on vegetable farms in Tanzania and Ohio. A dissertation presented in partial fulfillment of the requirements for the degree Doctor of Philosophy in the Graduate School of the Ohio State University. (Columbus, OH: Ohio State University), 300.
Testen A. L., Miller S. A. (2018). Carbon source and soil origin shape soil microbiomes and tomato soilborne pathogen populations during anaerobic soil disinfestation. Phytobiomes 2, 138–150. doi: 10.1094/PBIOMES-02-18-0007-R
Testen A. L., Martínez M. B., Madrid A. J., Deblais L., Taylor C. G., Pierce P. A., et al. (2021). On-farm evaluations of anaerobic soil disinfestation and grafting for management of a widespread soilborne disease complex in protected culture tomato production. Phytopathology 111, 954–965. doi: 10.1094/PHYTO-07-20-0288-R
Theis J. A., Fery R. L. (2002). Host plant resistance as an alternative to methyl bromide for managing Meloidogyne incognita in pepper. J. Nemato. 34, 374.
Thottathil G. P., Jayasekaran K., Othman A. S. (2016). Sequencing crop genomes: A gateway to improve tropical agriculture. Trop. Life Sci. Res. 27, 93–114.
Ueki A., Kaku N., Ueki K. (2018). Role of anaerobic bacteria in biological soil disinfestation for elimination of soilborne plant pathogens in agriculture. Appl. Microbiol. Biotechnol. 102, 6309–6318. doi: 10.1007/s00253-018-9119-x
Ueki A., Takehara T., Ishioka G., Kaku N., Ueki K. (2017). Degradation of the fungal cell wall by clostridial strains isolated from soil subjected to biological soil disinfestation and biocontrol of Fusarium wilt disease of spinach. Appl. Microbiol. Biotechnol. 101, 8267–8277. doi: 10.1007/s00253-017-8543-7
Van Agtmaal M., Van Os G. J., Hol W. H. G., Hundscheid M. P. J., Runia W. T., Hordijk C. A., et al. (2015). Legacy effects of anaerobic soil disinfestation on soil bacterial community composition and production of pathogen-suppressing volatiles. Front. Microbiol. 6, 701. doi: 10.3389/fmicb.2015.00701
Velders G. J. M., Andersen S. O., Daniel J. S., Fahey D. W., McFarland M. (2007). The importance of the Montreal protocol in protecting climate. Proc. Natl. Acad. Sci. U.S.A. 104, 4814–4819. doi: 10.1073/pnas.0610328104
Vincent I. R., Rosskopf E. N., Brecht J. K., Dufault N. S., Sandoya-Miranda G., Zhao X. (2024). Effects of anaerobic soil disinfestation for soilborne disease and weed management on baby leaf lettuce performance in a high tunnel organic production system. Agronomy 14 (4), 764. doi: 10.3390.14040764
Wang L., Mazzola M. (2019). Field evaluation of reduced rate Brassicaceae seed meal amendment and rootstock genotype on the microbiome and control of apple replant disease. Phytopathology 109, 378–1391. doi: 10.1094/PHYTO-02-19-0045-R
Washburn M. (2018). Alternative control methods for Verticillium wilt: A literature review. Available online at: http://hdl.handle.net/10919/86197. (accessed April 8, 2024).
Ya’nan L. I. U., Guangsheng H. E., Jianyu W. E. I., Haijiang J. I. A., Chongjun H. U. A. N. G., Yixia C. A. I., et al. (2019). Effects of anaerobic soil disinfection on the quality and bacterial community of tobacco-growing soils. Chin. Tob. Sci. 40, 39–46.
Yun C., Liu E., Rippa M., Mormile P., Sun D., Yan C., et al. (2020). Effects of chemical and solar soil-disinfection methods on soil bacterial communities. Sustainability 12 (23), 9833. doi: 10.3390/su12239833
Zavatta M., Shennan C., Muramoto J., Mazzola M. (2014). “Evaluating C-sources for anaerobic soil disinfestation,” in 2014 Annual International Research Conference on Methyl Bromide Alternatives and Emissions Reductions, 12-1- 12-4. Nov. 4-6, 2014. Orlando, FL.
Zhan Y., Yan N., Miao X. Y., Li Q., Chen C. B. (2021). Different responses of soil environmental factors, soil bacterial community, and root performance to reductive soil disinfestation and soil fumigant chloropicrin. Front. Microbiol. 12, 796191. doi: 10.3389/fmicb.2021.796191
Zhang D., Qiang R., Zhao J., Zhang J., Cheng J., Zhao D., et al. (2022). Mechanism of a volatile organic compound (6-methyl-2-heptanone) emitted from Bacillus subtilis ZD01 against Alternaria solani in potato. Front. Microbiol. 12, 808337. doi: 10.3389/fmicb.2021.808337
Zhu M., Zhang L., Xu J., He Y. (2023). Improved understanding on biochar effect in electron supplied anaerobic soil as evidenced by dechlorination and methanogenesis processes. Sci. Total Environ. 857, 15934. doi: 10.1016/j.scitotenv.2022.159346
Keywords: anaerobic soil disinfestation, organic amendments, soilborne pathogens, soil microbiome, sustainable agriculture
Citation: Meshram S, Philbrick AN and Adhikari TB (2024) Anaerobic soil disinfestation: a biologically-based solution for sustainable crop production. Front. Hortic. 3:1436782. doi: 10.3389/fhort.2024.1436782
Received: 22 May 2024; Accepted: 02 August 2024;
Published: 26 August 2024.
Edited by:
Juan Fernando Hirzel, Agricultural Research Institute, ChileReviewed by:
Aditi Satpute, Texas A&M University Kingsville, United StatesKgabo Martha Pofu, University of Limpopo, South Africa
Copyright © 2024 Meshram, Philbrick and Adhikari. This is an open-access article distributed under the terms of the Creative Commons Attribution License (CC BY). The use, distribution or reproduction in other forums is permitted, provided the original author(s) and the copyright owner(s) are credited and that the original publication in this journal is cited, in accordance with accepted academic practice. No use, distribution or reproduction is permitted which does not comply with these terms.
*Correspondence: Tika B. Adhikari, dGJhZGhpa2FAbmNzdS5lZHU=