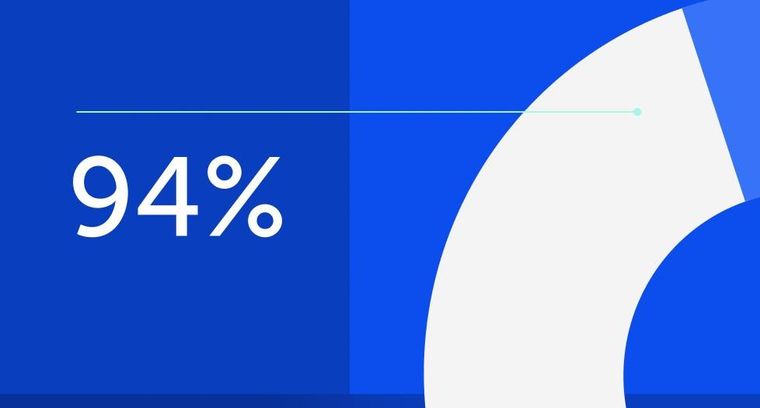
94% of researchers rate our articles as excellent or good
Learn more about the work of our research integrity team to safeguard the quality of each article we publish.
Find out more
ORIGINAL RESEARCH article
Front. Hortic., 18 March 2024
Sec. Floriculture and Landscapes
Volume 3 - 2024 | https://doi.org/10.3389/fhort.2024.1378098
This article is part of the Research TopicProduction And Breeding Of Ornamental Plants: Technical And Applied ResearchView all articles
Introduction: Gloriosa superba L. is an endangered ornamental plant of significant medicinal, commercial, and cultural value. This study establishes an efficient protocol for rapid in vitro propagation of this plant species through callus-mediated organogenesis using non-dormant corm explants.
Methods: Plant tissue culture techniques were employed to facilitate the in vitro regeneration process of Gloriosa superba L. This encompassed various stages, including the acquisition of plant material, surface sterilization, formulation of growth media, and the execution of callogenesis, shooting, rooting, and acclimatization experiments.
Results and Discussion: Investigation into the impact of plant growth regulators on callogenesis unveiled diverse callus morphologies, dependent on regulator type and concentration, with the NAA and KN combination emerging as the most effective for callus induction. Specifically, the callus induction medium supplemented with 1.5 mg L−1 NAA, 0.5 mg L−1 KN, and 10 mg L−1 casein hydrolysate (CH) achieved remarkable results, boasting an 81.25% callus induction rate and a substantial callus biomass fresh weight of 333 mg. Furthermore, the combination of BAP and NAA facilitated optimal shoot primordia induction and shooting in callus derived from non-dormant corm explants. The shoot induction medium, enriched with 2.0 mg L−1 BAP, 0.5 mg L−1 NAA, 5 mg L−1 CH, and 20% (v/v) coconut water (CW), resulted in an impressive 83.33% shoot primordia formation rate and an average of 6.86 shoot primordia per callus. For root induction, the medium supplemented with 1.0 mg L−1 IBA displayed a high root induction rate of 81.25% and robust rooting (+++). Successful acclimatization of in vitro plantlets in controlled environments and shade net houses culminated in thriving growth, and upon transplantation into a garden soil blend (garden soil : sand : vermiculite, 2:1:1, v/v), with direct sunlight exposure, the plantlets demonstrated commendable development and form, boasting a 69% survival rate at the seven-week mark. These findings offer a robust foundation for scalable and sustainable propagation strategies, ensuring the conservation and utilization of this valuable species.
● An effective in vitro mass propagation protocol was developed to meet the high demand and conservation imperative of Gloriosa superba L., an endangered plant with significant commercial potential in the ornamental and medicinal sectors.
Ornamental geophytes refer to a varied collection of flowers that are extensively used globally for purposes such as cut flowers, potted plants, landscape elements, and gardening plants. The modified organs (stem, root, leaf, or hypocotyl) produced underground and used as storage organs for plant growth and propagation are what distinguish ornamental geophytes like the lily, hyacinth, tulip, and dahlia (Zhao et al., 2022). Gloriosa superba L. is a captivating ornamental geophyte belonging to the Colchicaceae family. Its seeds and tubers are rich sources of colchicine and colchicoside, rendering it of paramount importance in modern medicine (Farooqi et al., 1993). The synthesis of secondary metabolites in plants is intricately linked to their physiological and developmental states, imposing limitations on their abundance. Most therapeutic secondary metabolites are sourced from wild or cultivated plants, with their chemical synthesis often deemed commercially impractical (Verma et al., 2012). Unfortunately, a concerning trend of rapid decline is observed in numerous therapeutic plant species (Castillo-Pérez et al., 2021). Among these, the Glory Lily stands out, facing excessive exploitation in its natural habitat due to the rich presence of colchicine and other valuable alkaloids endowed with medicinal and commercial significance (Kumar et al., 2015). Furthermore, traditional horticultural cultivation encounters limitations, exacerbated by challenges in the natural reproduction of Gloriosa superba L., characterized by low seed production and the dormancy of both seeds and tubers (Kaur and Ganjewala, 2019). Consequently, there is an imperative for alternative methodologies to propagate, enhance biomass production, and conserve this valuable species.
Plant tissue culture stands as a cornerstone discipline in plant biotechnology, delving into the intricate cellular-level intricacies of plant growth and development. At its essence, tissue culture entails the cultivation of targeted cells, tissues, or organs in a sterile synthetic medium, affording meticulous control over environmental factors such as temperature, light, and humidity (Thorpe, 2007). Plant tissue culture techniques offer a plethora of advantages, including cost-effectiveness, rapid mass in vitro propagation, and independence from unpredictable weather conditions. These techniques hold particular significance for the production of natural chemicals derived from plants that exhibit slow development or are challenging to synthesize chemically, all while considering environmental factors. Through tissue culture, a diverse array of metabolites, flavors, oils, pigments, and pharmaceutical compounds sourced from plants have been harnessed, contributing significantly to various sectors ranging from agriculture to medicine (Muranaka and Saito, 2010; Twaij et al., 2020). By enabling consistent and predictable synthesis of chemicals, plant tissue culture emerges as an appealing alternative, providing researchers and industries with a reliable method for the efficient production of valuable compounds (Farooqi et al., 1993; Mosoh et al., 2024). In addition, the application of plant tissue culture techniques has revolutionized plant conservation efforts by facilitating mass in vitro clonal propagation while simultaneously driving innovation in the commercial industry.
Plant cells possess a remarkable capacity for cell differentiation, often resulting in the formation of disorganized cell aggregates termed “callus” in response to biotic or abiotic stressors or both. Callus formation can originate from a plant part, known as an explant, or from a single specialized cell, with many callus cells exhibiting totipotency, allowing them to regenerate the entire plant organism (Nagata and Takebe, 1971). The realization that callus can be artificially induced in vitro, coupled with the understanding of the interplay between auxin and cytokinin in differentiation and dedifferentiation processes, has led to extensive utilization of callus in both fundamental scientific research and industrial applications (Gautheret, 1939; Skoog, 1957). Furthermore, plants possess intricate inherent processes to react and adjust to constantly shifting environmental conditions, demonstrating their impressive ability to adapt. Plant hormones and growth regulators play a crucial role in coordinating a wide range of physiological responses, forming the foundation of these adaptive processes. These signaling molecules delicately regulate various aspects of plant development, including germination, growth, flowering, and senescence. The intricate combination of these hormonal signals enables plants to effectively respond to obstacles such as nutrient availability, environmental pressures, and interactions with pathogens. Callus development in plant species is predominantly initiated through the application of auxin and cytokinin (Cai et al., 2015). A delicate balance between the concentrations of these plant growth regulators is crucial for the induction of callus formation, while an elevated ratio can trigger the regeneration of both roots and shoots. Auxins and cytokinins remain the focus of a lot of research on callus development and organ regeneration because they play such important roles in these processes.
Coconut water (CW) is a widely employed growth supplement in plant tissue culture and micropropagation due to its unique composition of carbohydrates, vitamins, minerals, amino acids, and phytohormones (Yong et al., 2009). The cytokinins present in coconut water play a pivotal role in promoting cell division and expediting growth, with potential contributions from additional phytohormones or unidentified components (Ma et al., 2008; Yong et al., 2009). Notably, coconut water induces substantial plant cell growth without instigating deleterious genetic changes (Arditti, 2009). Another significant supplement is casein hydrolysate (CH), characterized by a heterogeneous blend of organic compounds, including small-sized proteins, amino acids, vitamins, and growth-promoting substances. Its impact on plant growth lies in enhancing nitrogen availability, thereby optimizing the processing and transportation of nitrogen from organic sources (George et al., 2008). Researchers have demonstrated that casein hydrolysate serves as a valuable source of reduced nitrogen, promoting the growth of calluses and new shoots in various plant species (Salehi et al., 2017).
In vitro tissue culture techniques hold substantial promise for the clonal propagation and germplasm conservation of Gloriosa superba L. Extensive research has demonstrated the efficacy of utilizing various plant parts, including shoot tips, nodal segments, leaves, and tubers of Gloriosa superba L., to form calluses and induce organogenesis (Mahajan et al., 2016). However, there is a notable gap in the literature regarding the indirect organogenesis of Gloriosa superba L. using non-dormant corm explants in MS media. Although previous studies have investigated callus formation utilizing B5 medium or MS media supplemented with mixed supplements and varying combinations of cytokinins and auxins (Sivakumar et al., 2004; Singh et al., 2012), the overall protocol efficiency appears to be suboptimal, with the available data exhibiting limitations in clarity and comprehensiveness, thus requiring further scientific validation. This research endeavor is driven by the recognition that fine-tuning the composition of culture media by systematically varying the concentrations and combinations of these plant growth regulators (auxins and cytokinins) and supplements, such as casein hydrolysate (CH) and coconut water (CW), can significantly influence the morphogenetic processes in plant tissue culture systems, offering potential avenues for enhancing the efficiency and productivity of in vitro propagation approaches.
Therefore, this study aims to improve the efficiency of in vitro mass propagation using callus derived from non-dormant corm explants of Gloriosa superba L. The goal is to create a highly effective protocol for callogenesis and an indirect organogenesis regeneration system. This technological framework is expected to play a crucial role in preserving and advancing the germplasm resources of Gloriosa superba L. It will also facilitate research in functional genomics and progress in genetic transformation. The establishment of these protocols will contribute to the sustainable cultivation and preservation of this valuable plant species, addressing critical aspects of its propagation and genetic research.
Non-dormant corms of Gloriosa superba L. were obtained from mature plants that were in good health. These plants were found at the Pachmarhi Biosphere Reserve (PBR) in Madhya Pradesh, India. Following the harvest, the non-dormant corms were promptly placed in polyethylene chlorine bags with a blend of soil and coco peat to preserve their viability. They were then transported to a greenhouse for subsequent processing.
Murashige and Skoog (MS) medium, which Murashige and Skoog described in 1962, served as the culture medium in this investigation (Supplementary Data) (Murashige and Skoog, 1962). Several plant growth regulators and supplements were added to the medium. These were 6-benzylaminopurine (BAP), Indole-3-acetic acid (IAA), Kinetin (KN), 1-naphthaleneacetic acid (NAA), Indole-3-butyric acid (IBA), Casein hydrolysate (CH), and Coconut water (CW). The chemicals used were of analytical quality and were obtained from Sigma-Aldrich (India).
The non-dormant corm explants collected from the greenhouse were subjected to a thorough cleaning operation. Initially, the non-dormant corms were washed thoroughly under a continuous stream of tap water for 15 minutes. Afterward, the explants were subjected to an 8-minute washing treatment with 5% (v/v) Teepol solution and then rinsed five times with double-distilled water (DDW). Following a re-washing in a 2% (w/v) solution of Bavistin for 10 minutes, the explants underwent five rounds of DDW rinsing. After this, the explants were placed in an aseptic laminar airflow chamber for the continuation of the next phase of the sterilization process. This phase involved washing the explants in 70% (v/v) ethanol for 20 seconds, then rinsing them twice with sterile DDW. Following this, the explants underwent a second wash with 0.15% (w/v) HgCl2 for 8 minutes before receiving two rinses with sterile DDW. At this point, rinsing with sterile DDW was done thoroughly to eliminate any residual sterilants. Following this sterilization protocol, the surface-sterilized non-dormant corms were cut into 2 cm pieces using a sterilized blade, making them ready for culturing in the appropriate media.
The callus induction experiment utilized surface-sterilized Gloriosa superba L. non-dormant corm explants. Full-strength MS medium that had 3% (w/v) sucrose (HiMedia, India), 10 mg L-1 casein hydrolysate (CH), and 0.8% (w/v) agar (HiMedia, India) defined the callus induction medium (CIM). The pH of the medium was set to a pH of 5.8. Different treatment groups were established by varying the combination and concentrations of NAA (0.5 – 3.0 mg L-1), BAP (0.5 – 1 mg L-1) and KN (0.5 – 1 mg L-1) in the CIM (Table 1). The cultures were kept in a culture room under conditions of total darkness, with a temperature of 23 ± 2°C and a relative humidity of 60–70%. Each culture vessels contained 20 mL of solidified CIM and after inoculation of the explant, the culture vessels were sealed with parafilm to prevent contamination. To maintain the callus, it was subcultured every two weeks using the same medium composition as previously mentioned. However, plant growth regulators (PGRs) were added at concentrations that promoted cell growth and produced high-quality callus. Specifically, 1.5 mg L-1 NAA and 0.5 mg L-1 BAP were used for differentiating callus.
Table 1 Different concentrations of 6-benzylaminopurine (BAP), Kinetin (KN) and 1-naphthaleneacetic acid (NAA) used to induce callus from non-dormant corm explants of Gloriosa superba L.
Recorded experimental data included the frequency and rate of callus formation from non-dormant corm explants, the time taken for callus to form, the texture and color of the callus (labeled as C-Compact or F-Friable and G-Green, Y-Yellow, B-Brown, D-Dark, or L-Light), and the intensity of callus formation (labeled as “meager” for callus width of 0.5–1.0 cm, “moderate” for callus width of 1.0–1.5 cm, and “intense” for callus width of >1.5 cm). Additionally, the callus was weighed both while it was fresh and after it was oven-dried (Figures 1, 2).
Figure 1 Effect of different concentrations of auxin (NAA) in combination with cytokinins (BAP, KN) on callus formation from non-dormant corm explants of Gloriosa superba L. in full-strength MS medium containing 10 mg L-1 CH. Mean number of non-dormant corm explants forming callus (A), Response rate to callus induction (stratified by callus morphology) (B), Mean number of days required for callus induction (C), Response rate to callus induction (stratified by callus intensity) (D), Mean fresh weight (E) and Mean dry weight (F). Treatments are T1: 0.5 mg L-1 NAA, 0.5 mg L-1 BAP; T2: 1.0 mg L-1 NAA, 0.5 mg L-1 BAP; T3: 1.5 mg L-1 NAA, 0.5 mg L-1 BAP; T4: 2.0 mg L-1 NAA, 1.0 mg L-1 BAP; T5: 2.5 mg L-1 NAA, 1.0 mg L-1 BAP; T6: 3.0 mg L-1 NAA, 1.0 mg L-1 BAP; T7: 0.5 mg L-1 NAA, 0.5 mg L-1 KN; T8: 1.0 mg L-1 NAA, 0.5 mg L-1 KN; T9: 1.5 mg L-1 NAA, 0.5 mg L-1 KN; T10: 2.0 mg L-1 NAA, 1.0 mg L-1 KN; T11: 2.5 mg L-1 NAA, 1.0 mg L-1 KN; T12: 3.0 mg L-1 NAA, 1.0 mg L-1 KN. Bars indicate mean ± SE. Different letters indicate significant differences by Tukey’s test at P ≤ 0.05.
Figure 2 Effect of different concentrations of auxin (NAA) in combination with cytokinins (KN) on callus formation, texture, and color from non-dormant corm explants of Gloriosa superba L. in full-strength MS medium containing 10 mg L-1 CH. Treatments: (A) NAA (0.5 mg L-1) + KN (0.5 mg L-1); FYB (B) NAA (1.0 mg L-1) + KN (0.5 mg L-1); FLG (C) NAA (1.5 mg L-1) + KN (0.5 mg L-1); FG (D) NAA (2.0 mg L-1) + KN (1.0 mg L-1); CG (E) NAA (2.5 mg L-1) + KN (1.0 mg L-1); CG (F) NAA (3.0 mg L-1) + KN (1.0 mg L-1); CDB. Scale bar = 1 cm. Callus texture with color [texture (C - Compact and F - Friable) and color (G-Green, Y-Yellow, B-Brown, D-Dark, and L-Light)].
The study focused on aseptically culturing the friable green morphogenic callus, identified as the callus type with the most favorable characteristics and potential for shoot morphogenesis. This was carried out in shoot induction medium (SIM) that encompassed diverse combinations and concentrations of BAP (1.0 – 2.5 mg L-1), KN (0.5 – 1.5 mg L-1), and NAA (0.5 – 1.5 mg L-1) (Table 2 and Figure 3). Here, the SIM was defined by full-strength MS media with 5 mg L-1 of casein hydrolysate (CH), 20% (v/v) coconut water (CW), and 0.8% agar (Himedia, India). The pH of the media was adjusted to 5.8. The aseptic cultures were placed in a culture room under regulated conditions with a temperature of 23 ± 2°C and photoperiod of 16/8 h (day/night) using 40 W fluorescent tubes (Philips, India) giving off a light intensity of 20 µmol m–2 s–1. After six weeks, many parameters were evaluated, including the rate (%) and responsiveness of shoot primordia creation, the number of shoot primordia and shoots per explant, and the weight of the shoots when they were both fresh and oven-dried (Figure 3).
Table 2 Different concentrations of 6-benzylaminopurine (BAP), Kinetin (KN) and 1-naphthaleneacetic acid (NAA) used to induce shoots in callus derived from non-dormant corm explants of Gloriosa superba L.
Figure 3 Effect of various combinations of plant growth regulators (PGRs) on in vitro shoot regeneration via callus culture derived from non-dormant corm explants of Gloriosa superba L. on MS medium containing 5 mg L-1 CH + 20% CW. Mean number of calli forming shoot primordia (A), Response rate to shoot primordia formation (B) and Mean number of shoot primordia per callus (C) Treatments are T1: 1.0 mg L-1 BAP, 0.5 mg L-1 KN; T2: 1.5 mg L-1 BAP, 0.5 mg L-1 KN; T3: 2.0 mg L-1 BAP, 0.5 mg L-1 KN; T4: 2.5 mg L-1 BAP, 1.5 mg L-1 KN; T5: 1.0 mg L-1 BAP, 0.5 mg L-1 NAA; T6: 1.5 mg L-1 BAP, 0.5 mg L-1 NAA; T7: 2.0 mg L-1 BAP, 0.5 mg L-1 NAA; T8: 2.5 mg L-1 BAP, 1.5 mg L-1 NAA; T9: Control (Media without PGRs). Bars indicate mean ± SE. Different letters indicate significant differences by Tukey’s test at P ≤ 0.05.
The microshoots derived from the most optimal shoot morphogenesis treatment were precisely excised and cultured in root induction medium (RIM) which is defined by half-strength MS medium. Different treatment groups were established by varying the combination and concentrations of IBA (0.5–1.5 mg L-1), IAA (0.5–1.5 mg L-1), and NAA (0.5–1.5 mg L-1) in RIM (Table 3). Here, the control treatment consisted of RIM without any plant growth regulators. The cultures were kept in the culture room under regulated conditions with a temperature of 25 ± 2°C and 16/8-hour light/dark cycle provided by fluorescent lamps (TL 40 W/54 cool-day light) emitting a light intensity of 80 µmol m–2 s–1. The humidity level in the culture room was consistently maintained at a range of 55 to 60%. To evaluate the impact of treatment on in vitro rooting of microshoots, both the average rate and extent of rooting were assessed six weeks post-treatment. Additionally, the response to rooting treatments was categorized based on the degree of root formation detected (Figures 4, 5).
Table 3 Different concentrations of Indole-3-acetic acid (IAA), Indole-3-butyric acid (IBA) and 1-naphthaleneacetic acid (NAA) used to induce roots in in vitro derived microshoots of Gloriosa superba L. .
Figure 4 Effect of different concentrations of auxins on in vitro rooting of microshoots of Gloriosa superba L. on half-strength MS medium. Mean number of microshoots forming roots (A) and Response rate to rooting treatment (stratified by extent of rooting) (B). Treatments are T1: 0.5 mg L-1 IBA; T2: 1.0 mg L-1 IBA; T3: 1.5 mg L-1 IBA; T4: 0.5 mg L-1 IAA; T5: 1.0 mg L-1 IAA; T6: 1.5 mg L-1 IAA; T7: 0.5 mg L-1 NAA; T8: 1.0 mg L-1 NAA; T9: 1.5 mg L-1 NAA; T10: Control (Media without PGRs). Bars indicate mean ± SE. Different letters indicate significant differences by Tukey’s test at P ≤ 0.05.
Figure 5 In vitro rooting of Gloriosa superba L. microshoots in half-strength MS media supplemented with 1.0 mg L-1 IBA. (A) Initiation of rooting; (B, C) Root proliferation. Scale bar = 4 cm.
Before being placed in small polyethylene bags, plastic trays, plastic pots, or thermocol cups with a diameter of 7 cm, the plantlets were thoroughly washed with deionized water to eliminate any remaining medium. The pots were filled with a mixture of sterilized vermiculite and soil in equal proportions. During this initial stage of acclimatization, the young plants were exposed to a 16-hour photoperiod with 50 µmol m-2s-1 of light from white fluorescent tubes (40 W; Philips, India). To prevent dehydration and provide optimal air circulation, the plantlets were placed inside polyethylene bags with small openings, maintaining a high level of relative humidity (RH). The ambient temperature in the culture room was consistently maintained at 25 ± 2°C. Every day, the polythene casings were removed for one hour. Over two weeks, the little potted plants were watered every four days with 10 ml of a diluted solution containing half the normal concentration of MS basal salts, without including sucrose and myo-inositol.
During the second stage, which spanned from the third to the sixth week, the plantlets were relocated to medium-sized polyethylene bags, plastic cups, or thermocol cups. These containers were filled with a mixture of garden soil, sand, and vermiculite in a ratio of 2 parts soil to 1 part sand to 1 part vermiculite (volume/volume). Subsequently, these plantlets were transferred to a shade net house (SNH) and subjected to regular misting with tap water for one month. The relative humidity (RH) underwent a progressive reduction of 50% over this period. Following two weeks of being transplanted into a sterilized mixture of vermiculite and soil in the culture room (CR) and one additional week (i.e., the third week) of being transplanted into a combination of garden soil, sand, and vermiculite in a 2:1:1 ratio under shade in the net house (USNH), we measured the survival rate, plant height (in centimeters), number of shoots per plant, number of flowers per plant, and number of microtubers per plant.
The plantlets were subsequently transferred to larger earthen pots with a diameter of 15 cm. These pots contained a typical mixture of garden soil, sand, and farmyard manure in a ratio of 2 parts soil to 1 part sand to 1 part manure (volume/volume). The pots were exposed to direct sunlight for a period of up to seven weeks, specifically until the 10th week. After the transplantation, the survival rate of the plants outside of their natural environment, their height in centimeters (cm), and the number of shoots, flowers, and microtubers per plant were evaluated. This assessment took place two weeks later in a mixture of sterilized vermiculite and soil in a controlled environment, one week later in a mixture of garden soil, sand, and vermiculite under shade in a protected structure, and seven weeks later in a mixture of standard garden soil, sand, and farmyard manure under direct sunlight.
Data was gathered for 10 weeks, starting with the commencement of microshoot acclimation. Regular weekly observations were conducted after the implantation of microplantlets onto the designated potting mixtures. There were 56 microplantlets in total for each treatment, with four replicates each containing 14 microplantlets. The survival percentage of the regenerated plantlets was determined by applying the formula: survival rate (%) = (number of surviving regenerated plants/total number of transplanted regenerated plants) times 100%. The data provided provides the average values along with the standard error (SE).
This study used a completely random experimental design. Following the different experiments, non-dormant corm explants (2 cm x 1 cm), calli, microshoots, and plantlets were randomly assigned to treatment groups. Each treatment had four replicates, wherein eight non-dormant corm explants, six morphogenic calli units, and twelve microshoots were assigned to the callus formation, shooting, and rooting experiments, respectively. Each experiment was repeated twice for better reliability of the results.
Data on all variables were collected six weeks post-treatment. The callus induction rate was determined by dividing the total number of non-dormant corm explants forming callus by the total number of replicates and then multiplying the result by 100. The shoot primordia formation rate was determined by dividing the total number of calli forming shoot primordia by the total number of replicates and then multiplying the result by 100. The response rate to rooting treatment was assessed by calculating the percentage of microshoots that formed roots out of the total number of replicates.
The normality of the data was evaluated using the Shapiro-Wilk test. Data sets that were found to be normally distributed (p ≥ 0.05) after conducting the normality test were analyzed using parametric tests such as one-way ANOVA with a significance level of α = 0.05 to compare the means. If the normality test was significant (p < 0.05), a non-parametric test, namely the Kruskal-Wallis test with a significance level of α = 0.05, was used to compare the means. The data analysis was conducted using R Studio software, specifically version 4.3.1. The mean separation was determined using Tukey’s honestly significant difference (HSD) test with a significance level of α = 0.05. The findings were reported as mean values with standard error, and distinct letters in the figures indicate statistically significant differences at a significance level of p < 0.05.
This study examined the effects of various combinations of plant growth regulators (PGRs) on callogenesis (Table 1 and Figure 1). Differences were observed in key parameters like the number of days required for callus initiation, callus induction rate (%), fresh and dry weight of callus (mg), intensity and texture of callus, as well as its color. These variations were observed in response to different concentrations of plant growth regulators (PGRs). In addition to exploring the effects of PGRs on callus formation, this study also investigated their effects on the processes of in vitro shoot morphogenesis, and in vitro rooting.
Data were recorded six weeks post-treatment. The experimental results indicate substantial variation in callus induction percentage, texture, color, intensity, initiation time, and fresh/dry weight. These variances were associated with the different effects of the plant growth regulators (PGRs). Notably, there were big differences between the treatments in the percentage of callus formation and the stiffness of the new callus (Figure 2). The cytokinins most likely helped slow down the lignification of the cell wall, which then sped up the formation and growth of the callus (Kiba and Sakakibara, 2010). The growth of callus mostly began at the incision site(s) of the explants, later spreading to cover the entire surface. At first, the calli showed a range of colors from transparent to yellowish, which then changed to a pale green tone. Following an extra fortnight of incubation, the hue of some calluses underwent a transition from pale green to brown and subsequently to deep green, depending on the exact types and concentrations of plant growth regulators (PGRs) used. Notably, calli displaying hyperhydric exudates demonstrated early-stage necrosis. Certain calli exhibited pronounced lignification, leading to a hard texture, while others exhibited embryogenic traits and could easily be partitioned into smaller chunks (Figure 2).
The duration of callus initiation ranged from 9.5 to 19.8 days, while the callus formation rate, fresh weight, and dry weight varied between 37.50% and 81.25%, 160 mg and 333 mg, and 19.9 mg and 31.6 mg, respectively, depending on the concentrations and combinations of plant growth regulators (PGRs) (Figure 1C).
Significantly different (p < 0.001) callus initiation was observed in non-dormant corm explants treated with various PGR combinations. The shortest callus initiation time of 9.50 days was achieved in CIM that contained 1.5 mg L-1 NAA + 0.5 mg L-1 KN. This was followed by 2.0 mg L-1 NAA + 1.0 mg L-1 KN (10.80 days), 1.5 mg L-1 NAA + 0.5 mg L-1 BAP (11.20 days), 1.0 mg L-1 NAA + 0.5 mg L-1 BAP (12 days), and 1.0 mg L-1 NAA + 0.5 mg L-1 KN (12 days) (Figure 1C).
The callus induction rate was observed in CIM that contained 1.5 mg L-1 NAA + 0.5 mg L-1 KN (81.25%), followed by 1.5 mg L-1 NAA + 0.5 mg L-1 BAP (78.13%), and 2.0 mg L-1 NAA + 1.0 mg L-1 KN (71.88%). Even though the difference was not significant (p < 0.138), these treatments resulted in highly embryogenic friable green calli that produced above average callus intensity (> 1.5 cm width of callus) (Figures 1A, B, D). On the other hand, treatment with 3.0 mg L-1 NAA + 1.0 mg L-1 KN produced non-embryogenic calli with a dark brown hue, compact texture, and below average callus intensity (0.5 - 1.0 cm width of callus) (Figure 1D), which made them more suitable for secondary metabolite investigations (Figure 1B).
Regarding callus weight, the treatment with 1.5 mg L-1 NAA + 0.5 mg L-1 KN exhibited the highest average fresh weight (333 mg) (p < 0.001), followed by 1.5 mg L-1 NAA + 0.5 mg L-1 BAP (293 mg) (Figure 1E). Similarly, the highest average dry weight (31.6 mg) was observed in the 1.5 mg L-1 NAA + 0.5 mg L-1 KN treatment (p < 0.001), followed by 1.5 mg L-1 NAA + 0.5 mg L-1 BAP (29.9 mg) and 2.0 mg L-1 NAA + 1.0 mg L-1 BAP (28.9 mg) (Figure 1F).
Treatments such as 0.5 mg L-1 NAA + 0.5 mg L-1 BAP (19.8 days), 2.0 mg L-1 NAA + 1.0 mg L-1 BAP (14.8 days), 2.5 mg L-1 NAA + 1.0 mg L-1 BAP (17.2 days), 3.0 mg L-1 NAA + 1.0 mg L-1 BAP (17.2 days), 0.5 mg L-1 NAA + 0.5 mg L-1 KN (17.5 days), and 3.0 mg L-1 NAA + 1.0 mg L-1 KN (15 days) required more than fourteen days for callus initiation, with callus induction rates ranging from 37.5% to 68.75%, and average callus fresh weights ranging from 160 mg to 244 mg (Figures 1C, B, E).
The most optimal embryogenic calli (initiated in 1.5 mg L-1 NAA + 0.5 mg L-1 KN) were cultured in SIM containing various combinations and concentrations of BAP, KN, and NAA (Table 2). The addition of cytokinins and auxins in the culture medium influenced the rate of shoot primordia formation and the number of shoot primordia per callus (Figure 3). Shoot primordia initiation was observed within 14 to 28 days after inoculation. The results revealed the association between treatment of callus with PGRs and in vitro shoot morphogenesis.
The highest shoot primordia formation rate from callus was achieved in SIM that contained 2.0 mg L-1 BAP + 0.5 mg L-1 NAA (83.33%) (Figure 3B). This treatment showed a significant difference compared to the control treatment (PGR-free) (p < 0.0032). Other effective combinations included 2.0 mg L-1 BAP + 0.5 mg L-1 KN (75.00%), 1.5 mg L-1 BAP + 0.5 mg L-1 NAA (70.83%), 1.5 mg L-1 BAP + 0.5 mg L-1 KN (66.67%), and 2.5 mg L-1 BAP + 1.5 mg L-1 NAA (62.5%) (Figures 3A, B). Moreover, SIM that contained 2.0 mg L-1 BAP + 0.5 mg L-1 NAA resulted in the highest average number of shoot primordia per callus (6.86) (p < 0.001), which was significantly different from the control treatment (Figures 3C, 6).
Figure 6 Effect of PGR on in vitro shoot regeneration via callus culture derived from non-dormant corm explants of Gloriosa superba L. on MS medium containing 5 mg L-1 CH + 20% CW supplemented with 2.0 mg L-1 BAP + 0.5 mg L-1 NAA. (A, B) Initiation of shoot primordium (after 14 days); (C, D) Matured and large-size shoots from callus (after 6 weeks). Scale bar = 1 cm.
Root formation in microshoots excised from the callus was investigated in RIM containing different concentrations of IAA, NAA, and IBA. The results of this experiment showed that treatment with various types and concentrations of auxins in the culture medium influenced both the rate and extent of root induction.
The highest rate of root induction was observed in RIM that contained 1.0 mg L-1 IBA (81.25%), which showed a significant difference compared to the PGR-free control treatment (p < 0.001). This was followed by 1.0 mg L-1 IAA (72.92%) and 1.0 mg L-1 NAA (66.67%) (Figures 4A, B). Likewise, the highest extent of rooting was achieved in RIM that contained 1.0 mg L-1 IBA (Figures 4B, 5).
After successful in vitro rooting, the plantlets were grown on a substrate of sterilized vermiculite and soil (1:1, v/v) in a culture room for fourteen days, followed by seven days of acclimatization under shade in a net house on a substrate of garden soil, sand, and vermiculite (2:1:1, v/v) (Figures 7A, 8A–D). The acclimatization rate was 100% during this period. However, during the subsequent seven weeks of acclimatization in garden soil, sand, and vermiculite (2:1:1, v/v) under direct sunlight, the survival rate decreased to 69% (Figures 7A, 8E, F). Notably, all surviving plants had a normal phenotype, including reaching the expected plant height, displaying good shoot multiplication, flowering, and producing microtubers. These parameters were statistically significant (p < 0.001) (Figures 7B–D, 8E–F).
Figure 7 Acclimatization of in vitro regenerated microshoots of Gloriosa superba L. Plant survival rate (A) Mean plant height in cm (B) Mean number of shoots per plant (C) Mean number of flowers per plant (D) Mean number of microtubers per plant and (E) measured after two weeks of transplant in sterilized (vermiculite + soil, 1:1) grown in culture room (CR), one week (i.e. on the 3rd week) of transplant in garden soil + sand + vermiculite, 2:1:1 under shade in net house (USNH), and seven weeks (i.e. 10th week) of transplant in garden soil + sand + vermiculite, 2:1:1 under direct sunlight (DSL).
Figure 8 Acclimatization of in vitro regenerated microshoots of Gloriosa superba L. (A) Microshoots transplanted into sterilized (vermiculite + soil, 1:1) being grown in culture room (CR), (B) Plantlets were covered in polythene castings with tiny punctures. (C, D) Plantlets transplanted into medium-sized polyethylene vessels containing a mixture of garden soil + sand + vermiculite, 2:1:1 under shade in net house (USNH). (E–G) Plantlet flower development at the later stages of acclimatization, growing in earthen vessels containing a mixture of standard garden soil: sand: farmyard manure, 2:1:1 under direct sunlight (DSL). (H) Microtubers harvested at the later stage of acclimatization. Scale bar = 1 cm.
In this study, we present a comprehensive protocol for inducing callus formation from non-dormant corm explants of Gloriosa superba L., along with the processes of indirect organogenesis and ex vitro acclimatization. Distinct effects on callus formation, differentiation, and organogenesis were observed by manipulating the concentrations of plant growth regulators such as BAP, KN, and NAA in the culture media supplemented with CH, CW, or both.
Callus formation, a process driven by physical or chemical stimuli, entails the modulation of natural plant hormone levels, initiating complex regulatory cascades and cellular reprogramming (Tuskan et al., 2018). Callus displays a spectrum of characteristics and can be classified into subgroups based on observable morphological features. In this study, the texture and color of the callus varied depending on the presence and concentration of specific plant growth regulators in the media. Notably, the compact green calli favored shoot development, while the compact dark-brown calli were more suitable for secondary metabolite elicitation (Figure 2). Friable or compact callus typically lacks discernible organ renewal, while rooty, shooty, or embryonic callus exhibits varying degrees of organ regeneration, depending on the specific organs they produce (Ikeuchi et al., 2013).
In previous studies, callus initiation timelines and responses varied significantly. Singh et al. (2012) observed callus formation after three weeks, yielding globular and white calli in a medium comprising MS with 2.5 mg L−1 BAP, 1 mg L−1 IAA, 0.5 mg L−1 KN, and 15% CW, under a 14-hour photoperiod. Conversely, Arumugam and Gopinath (2012) noted yellowish callus initiation (98.30%) after 25 to 30 days on MS media supplemented with 1.0 mg L−1 2,4-D and 0.5 mg L−1 IAA. In contrast, our study demonstrated a remarkable callus initiation response of 81.25% within a mere 9.50 days when surface sterilized non-dormant corm explants were treated with 1.5 mg L−1 NAA, 0.5 mg L−1 KN, and 10 mg L−1 CH. Auxins, particularly NAA, have been widely recognized as effective plant growth regulators (PGRs) for promoting callus formation (Rathore et al., 2020). This expedited response underscores a substantial improvement in efficiency, achieving callus initiation three times faster than previously reported methods. In contrast to our findings, a previous investigation focusing on Nigella damascena L. revealed that a combination of NAA and BAP proved more effective in promoting callus formation compared to using NAA with KN. This discrepancy underscores the inherent variation in callus formation responses among different plant species, stressing the crucial influence of the specific type, concentration, and combination of plant growth regulators (PGRs) used in the process (Klimek-Chodacka et al., 2020).
Under specific conditions, callus cells can undergo organogenesis, generating organs from cultured explants like roots, leaves, or flowers, leading to the development of shoots or roots. According to Ashokhan et al. (2020), the pivotal roles played by auxins and cytokinins in controlling cell division and growth highlight their significance in this procedure, influencing the activities of meristematic cells. This study revealed that treatment with 2.0 mg L-1 BAP, 0.5 mg L-1 NAA, 5 mg L-1 CH, and 20% CW yielded a shooting response rate of 83.33% and produced an impressive average of 6.86 shoot primordia per callus. This outcome surpasses that reported by Arumugam and Gopinath (2012), where treatment with half-strength MS medium containing 1.0 mg L-1 KN, 1.5 mg L-1 BAP, and 20% CW achieved a higher shooting response rate (94%) but yielded a lower overall shoot per callus count. The delicate balance between these plant growth regulators profoundly influences the outcome, with the auxin-to-cytokinin ratio serving as a crucial determinant. Elevated auxin concentrations prompt callus formation, while heightened cytokinin levels redirect cell fate, promoting meristem development (Motte et al., 2014).
The findings of this study indicate that BAP is the most effective cytokinin for shoot induction, whereas KN performed better for callus formation in Gloriosa superba L. The inclusion of cytokinin in the culture media, along with auxin, is essential for the induction of indirect adventitious shoot formation (Sen et al., 2014). Moreover, the incorporation of casein hydrolysate (CH) and coconut water (CW) in the basal media has demonstrated positive effects on callus formation (Kamaruzzaman et al., 2018), shoot morphogenesis (Samiei et al., 2021), and overall plant development (Mohammed and Ali, 2010). Furthermore, these findings are consistent with prior studies that demonstrate the advantage of BAP compared to other cytokinins, such as KN, in promoting shoot formation (Hesami et al., 2019). Hassan and Roy (2005) showed that when apical and axillary buds from young sprouts of naturally grown Gloriosa superba L. plants were cultured in MS basal media supplemented with 1.5 mg L-1 BAP and 0.5 mg L-1 NAA, they were able to regenerate numerous shoots per culture. The consistent results seen in multiple investigations emphasize the effectiveness of BAP in stimulating shoot growth, indicating its potential as a crucial factor in improving micropropagation techniques for Gloriosa superba L.
The rooting response of Gloriosa superba L. non-dormant corm callus-derived microshoots proved to be significantly influenced by the type and concentration of exogenous auxins (NAA, IBA, and IAA). Notably, RIM that was supplemented with 1.0 mg L-1 IBA demonstrated the highest rate of root regeneration and root intensity, suggesting the superiority of IBA in facilitating root initiation in Gloriosa superba L microshoots. This finding resonates with a prior investigation on Gloriosa superba L., which documented the emergence of long and robust roots in MS medium supplemented with 2.0 mg L-1 IBA (Mahajan et al., 2016). Consistent observations have also been reported across various plant species, including Passiflora foetida L (Shekhawat et al., 2015), Cotinus coggygria Scop (Ilczuk and Jacygrad, 2016), Hemidesmus indicus (L.) (Shekhawat and Manokari, 2016), and Capparis spinosa L (Gianguzzi et al., 2020), wherein the incorporation of IBA into MS medium at different concentrations led to the development of vigorous root systems. These findings underscore the efficacy of IBA as a potent inducer of root formation and highlight its broad applicability in optimizing micropropagation protocols across diverse plant species.
Plantlets exhibited a 100% survival rate after two weeks of acclimatization in a culture room, where they were grown on a substrate of sterilized vermiculite soil (1:1, v/v). Subsequently, they were transferred to a net house and kept under shade for an additional week, using a substrate of garden soil-sand-vermiculite (2:1:1, v/v). However, when the plantlets were subsequently exposed to direct sunlight outside the net house, the survival rate decreased to 69% after seven weeks. This decrease in survival can be attributed to the reduction in vermiculite ratio and the increased photosynthetically active radiation (PAR) in the garden soil-sand-vermiculite mixture (Mosoh et al., 2023). A previous study by Hoang et al. (2020) supported the notion that changes in substrate composition and increased PAR can affect plant growth and survival. Despite the difference in survival rates, no noticeable variations were observed in the morphological or growth characteristics of the acclimatized plants.
This study presents an effective micropropagation method for Gloriosa superba L. utilizing a combination of casein hydrolysate (CH) and coconut water (CW) along with plant growth regulators. Adding CH and/or CW to CIM and SIM had a positive impact on the formation of calli and shoot regeneration in this species, respectively. By adding 1.5 mg L-1 NAA, 0.5 mg L-1 KN, and 10 mg L-1 CH to the CIM, there was a significant improvement in callus induction and development. This resulted in the speed of callus formation being tripled when compared to previous reports. Moreover, SIM that was supplemented with 2.0 mg L-1 BAP, 0.5 mg L-1 NAA, 5 mg L-1 CH, and 20% CW yielded multiple shoots per callus. Additionally, 1.0 mg L-1 IBA was found to be the most effective in promoting in vitro rooting of Gloriosa superba L. microshoots. The plantlets were successfully acclimatized, with a survival rate of 69% after seven weeks.
This study presents an efficient protocol for the rapid, large-scale in vitro propagation of Gloriosa superba L. through indirect organogenesis, marking a significant advancement in the field. The developed protocol is promising, not only for facilitating the commercial cultivation of this valuable species, but also for aiding in the restoration of its dwindling populations in the wild. By providing a reliable method for mass propagation, this protocol offers a tangible solution to the conservation challenges faced by Gloriosa superba L. This contributes to its preservation and sustainable utilization.
The raw data supporting the conclusions of this article will be made available by the authors, without undue reservation.
DM: Data curation, Formal analysis, Funding acquisition, Investigation, Methodology, Software, Validation, Visualization, Writing – original draft, Writing – review & editing. AK: Conceptualization, Investigation, Methodology, Project administration, Resources, Supervision, Validation, Writing – review & editing. SV: Investigation, Methodology, Supervision, Writing – review & editing. WV: Funding acquisition, Investigation, Supervision, Validation, Writing – review & editing.
The author(s) declare financial support was received for the research, authorship, and/or publication of this article. This study has received support from the USDA National Institute of Food and Agriculture, specifically through the Hatch project 7001563.
The corresponding author extends heartfelt gratitude to Chief MOSOH Paul Tandong and Chieftess Ateyim Espe MOSOH Ostensia Nkeng of PINYIN (Santa, North-West Region, Cameroon) for their tremendous support. The corresponding author extends heartfelt gratitude to Mr. Tetu Acha Samuel (MD, USA) for his prompt and invaluable assistance with procurement appropriation. We equally acknowledge the invaluable assistance provided by Dr. Rohit Sharma from the Centre for Biodiversity Exploration and Conservation (CBEC) during the initial stages of this project. His contributions have been instrumental in shaping the direction of our research. Additionally, we express our sincere appreciation to the reviewers for their diligent review of our work, and for their insightful comments and suggestions, which have significantly enriched the quality of our manuscript.
AK is employed by the Centre of Excellence in Research & Entrepreneurship Development, Bambooram Agro PVT. LTD, Sehore, MP, India.
The remaining authors declare that the research was conducted in the absence of any commercial or financial relationships that could be constructed as a potential conflict of interest.
All claims expressed in this article are solely those of the authors and do not necessarily represent those of their affiliated organizations, or those of the publisher, the editors and the reviewers. Any product that may be evaluated in this article, or claim that may be made by its manufacturer, is not guaranteed or endorsed by the publisher.
The Supplementary Material for this article can be found online at: https://www.frontiersin.org/articles/10.3389/fhort.2024.1378098/full#supplementary-material
BAP, 6-Benzylaminopurine; CH, Casein hydrolysate; CW, Coconut water; IAA, Indole 3-acetic acid; KN, Kinetin; NAA, 1-Naphthaleneacetic acid; PGR, Plant growth regulator; CRD, Completely randomized design; IBA, Indole-3-butyric acid; MS, Murashige and Skoog, 1962 medium; CIM, Callus induction medium; SIM, Shoot induction medium; RIM, Root induction medium.
Arumugam A., Gopinath K. (2012). In vitro Micropropagation using Corm Bud Explants: An Endangered Medicinal Plant of Gloriosa superba L. Asian J. Biotechnol. 4, 120–128. doi: 10.3923/ajbkr.2012.120.128
Ashokhan S., Othman R., Abd Rahim M. H., Karsani S. A., Yaacob J. S. (2020). Effect of plant growth regulators on coloured callus formation and accumulation of azadirachtin, an essential biopesticide in azadirachta indica. Plants 9(3):352. doi: 10.3390/plants9030352
Cai Z., Jing X., Tian X., Jiang J., Liu F., Wang X. (2015). Direct and indirect in vitro plant regeneration and the effect of brassinolide on callus differentiation of Populus euphratica Oliv. South Afr. J. Bot. 97, 143–148. doi: 10.1016/j.sajb.2015.01.006
Castillo-Pérez L. J., Alonso-Castro A. J., Fortanelli-Martínez J., Carranza-Álvarez C. (2021). “Chapter 2—Biotechnological approaches for conservation of medicinal plants,” in Phytomedicine. Eds. Bhat R. A., Hakeem K. R., Dervash M. A. (Cambridge, MA, USA: Academic Press), 35–58. doi: 10.1016/B978-0-12-824109-7.00002-9
Farooqi A., Kumaraswamy B., Bojappa K., Pusalkar V., Gupta R. (1993). Plantations of the clinically important Gloriosa superba. Indian Horticult 37 (4), 26–29.
Gautheret R. (1939). Sur la possibilité de réaliser la culture indéfinie des tissus de tubercules de carotte. CR. Hebd. Seances. Acad. Sc. 208, 118–120.
Gautheret R. (2008). The components of plant tissue culture media ii: organic additions, osmotic and ph effects, and support systems. In; George E.F., Hall M.A., Klerk GJ.D. (eds) Plant Propagation by Tissue Culture (Dordrecht: Springer). doi: 10.1007/978-1-4020-5005-3_4
Gianguzzi V., Barone E., Sottile F. (2020). In vitro Rooting of Capparis spinosa L. as Affected by Genotype and by the Proliferation Method Adopted During the Multiplication Phase. Plants 9. doi: 10.3390/plants9030398
Hassan A., Roy S. K. (2005). Micropropagation of Gloriosa superba L. through high frequency shoot proliferation. Plant Tissue Cult. 15, 67–74.
Hesami M., Naderi R., Tohidfar M. (2019). Modeling and optimizing medium composition for shoot regeneration of chrysanthemum via radial basis function-non-dominated sorting genetic algorithm-II (RBF-NSGAII). Sci. Rep. 9, 18237. doi: 10.1038/s41598-019-54257-0
Hoang N. N., Kitaya Y., Shibuya T., Endo R. (2020). Effects of supporting materials in in vitro acclimatization stage on ex vitro growth of wasabi plants. Sci. Hortic. 261, 109042. doi: 10.1016/j.scienta.2019.109042
Ikeuchi M., Sugimoto K., Iwase A. (2013). Plant callus: mechanisms of induction and repression. Plant Cell 25, 3159–3173. doi: 10.1105/tpc.113.116053
Ilczuk A., Jacygrad E. (2016). The effect of IBA on anatomical changes and antioxidant enzyme activity during the in vitro rooting of smoke tree (Cotinus coggygria Scop.). Sci. Hortic. 210, 268–276. doi: 10.1016/j.scienta.2016.07.036
Kamaruzzaman N. D. A., Jaafar Sidik N., Saleh A. (2018). Pharmacological activities and benefits of coconut water in plant tissue culture: A review/Nur Dayana Athirah Kamaruzzaman, Norrizah Jaafar Sidik and Azani Saleh. Sci. Lett. (ScL). 12 (2), 1-10.
Kiba T., Sakakibara H. (2010). “Role of Cytokinin in the Regulation of Plant Development,” in Plant Developmental Biology—Biotechnological Perspectives, vol. 2 . Eds. Pua E. C., Davey M. R. (New York: Springer), 237–254. doi: 10.1007/978-3-642-04670-4_13
Klimek-Chodacka M., Kadluczka D., Lukasiewicz A., Malec-Pala A., Baranski R., Grzebelus E. (2020). Effective callus induction and plant regeneration in callus and protoplast cultures of Nigella damascena L. Plant Cell. Tissue Organ Culture. (PCTOC) 143, 693–707. doi: 10.1007/s11240-020-01953-9
Kumar C. N., Jadhav S. K., Tiwari K. L., Afaque Q. (2015). In vitro tuberization and colchicine content analysis of Gloriosa superba L. Biotechnology 14, 142–147. doi: 10.3923/biotech.2015.142.147
Kaur G., Ganjewala D. (2019). “Stress protectant secondary metabolites and their metabolic engineering to enhance abiotic stress tolerance in plants,” In Vitro Plant Breeding Towards Novel Agronomic Traits eds Kumar M., Muthusamy A., Kumar V., Bhalla-Sarin N. (Singapore: Springer). doi: 10.1007/978-981-32-9824-8
Ma Z., Ge L., Lee A. S., Yong J. W. H., Tan S. N., Ong E. S. (2008). Simultaneous analysis of different classes of phytohormones in coconut (Cocos nucifera L.) water using high-performance liquid chromatography and liquid chromatography–tandem mass spectrometry after solid-phase extraction. Analytica. Chim. Acta 610, 274–281. doi: 10.1016/j.aca.2008.01.045
Mahajan R., Kapoor N., Billowria P. (2016). Callus proliferation and in vitro organogenesis of Gloriosa superba: An endangered medicinal plant. Ann. Plant Sci. 5, 1466. doi: 10.21746/aps.2016.12.003
Mohammed S., Ali M. (2010). Effect of coconut water on callus growth on cyamopsis tetragonolobust. Pharmacia 1, 25–27.
Mosoh D. A., Khandel A. K., Verma S. K., Vendrame W. A. (2023). Effects of sterilization methods and plant growth regulators on in vitro regeneration and tuberization in Gloriosa superba (L.). In Vitro Cell Dev. Biol. Plant 59, 792–807. doi: 10.1007/s11627-023-10387-9
Mosoh D. A., Khandel A. K., Verma S. K., Vendrame W. A. (2024). Phytochemical analysis and enhanced production of alkaloids in non-dormant corm-derived callus of Gloriosa superba (L.) using plant growth regulators and abiotic elicitors. Plant Cell, Tissue and Organ Culture (PCTOC) 156, 89. doi: 10.1007/s11240-023-02674-5
Motte H., Vereecke D., Geelen D., Werbrouck S. (2014). The molecular path to in vitro shoot regeneration. Biotechnol. Adv. 32, 107–121. doi: 10.1016/j.biotechadv.2013.12.002
Muranaka T., Saito K. (2010). “3.17—Production of pharmaceuticals by Plant Tissue Cultures,” in Comprehensive Natural Products II. Eds. (Ben) Liu H.-W., Mander L. (Oxford: Elsevier), 615–628. doi: 10.1016/B978-008045382-8.00065-4
Murashige T., Skoog F. (1962). A revised medium for rapid growth and bio assays with tobacco tissue cultures. Physiol. Plantarum. 15, 473–497. doi: 10.1111/j.1399-3054.1962.tb08052.x
Nagata T., Takebe I. (1971). Plating of isolated tobacco mesophyll protoplasts on agar medium. Planta 99, 12–20. doi: 10.1007/BF00392116
Rathore M. S., Patel P. R., Siddiqui S. A. (2020). Callus culture and plantlet regeneration in date palm (Phoneix dactylifera L.): An important horticultural cash crop for arid and semi-arid horticulture. Physiol. Mol. Biol. Plants 26, 391–398. doi: 10.1007/s12298-019-00733-w
Salehi M., Moieni A., Safaie N. (2017). A novel medium for enhancing callus growth of hazel (Corylus avellana L.). Sci. Rep. 7, 15598. doi: 10.1038/s41598-017-15703-z
Samiei L., Davoudi Pahnehkolayi M., Tehranifar A., Karimian Z. (2021). Organic and inorganic elicitors enhance in vitro regeneration of Rosa canina. J. Genet. Eng. Biotechnol. 19, 60. doi: 10.1186/s43141-021-00166-7
Sen M. K., Nasrin S., Rahman S., Jamal A. H. M. (2014). In vitro callus induction and plantlet regeneration of Achyranthes aspera L., a high value medicinal plant. Asian Pac. J. Trop. Biomed. 4, 40–46. doi: 10.1016/S2221-1691(14)60206-9
Shekhawat M. S., Kannan N., Manokari M., Ravindran C. P. (2015). In vitro regeneration of shoots and ex vitro rooting of an important medicinal plant Passiflora foetida L. through nodal segment cultures. J. Genet. Eng. Biotechnol. 13, 209–214. doi: 10.1016/j.jgeb.2015.08.002
Shekhawat M. S., Manokari M. (2016). In vitro regeneration frequency, micro-morphological studies and ex vitro rooting of Hemidesmus indicus (L.) R. Br.: A multi-potent endangered climber. Indian J. Plant Physiol. 21, 151–160. doi: 10.1007/s40502-016-0216-5
Singh D., Mishra M., Yadav A. S. (2012). Callus induction from corm of Gloriosa superba Linn: An endangered medicinal plant. Biotechnol. an Indian J. Rev. 6, 53–55.
Sivakumar G., Krishnamurthy K. V., Hao J., Paek K. Y. (2004). Colchicine production in Gloriosa superba calluses by feeding precursors. Chem. Natural Compounds. 40, 499–502. doi: 10.1007/s10600-005-0020-3
Skoog F. (1957). Chemical regulation of growth and organ formation in plant tissue cultured in vitro. Symp. Soc. Exp. Biol. 11, 118–131.
Thorpe T. A. (2007). History of plant tissue culture. Mol. Biotechnol. 37, 169–180. doi: 10.1007/s12033-007-0031-3
Tuskan G. A., Mewalal R., Gunter L. E., Palla K. J., Carter K., Jacobson D. A., et al. (2018). Defining the genetic components of callus formation: A GWAS approach. PloS One 13, e0202519. doi: 10.1371/journal.pone.0202519
Twaij B. M., Jazar Z. H., Hasan M. N. (2020). Trends in the use of tissue culture, applications and future aspects. Int. J. Plant Biol. 11, 8385. doi: 10.4081/pb.2020.8385
Verma A. K., Singh R. R., Singh S. (2012). Improved alkaloid content in callus culture of Catharanthus roseus. Botanica Serbica. 36, 123–130.
Yong J. W. H., Ge L., Ng Y. F., Tan S. N. (2009). The Chemical composition and biological properties of coconut (Cocos nucifera L.) water. Molecules 14 (12), 5144-5164. doi: 10.3390/molecules14125144
Keywords: acclimatization, callogenesis, germplasm conservation, floriculture, in vitro mass propagation, ornamental plants, shoot morphogenesis, plant growth regulators
Citation: Mosoh DA, Khandel AK, Verma SK and Vendrame WA (2024) Optimizing callus induction and indirect organogenesis in non-dormant corm explants of Gloriosa superba (L.) via media priming. Front. Hortic. 3:1378098. doi: 10.3389/fhort.2024.1378098
Received: 29 January 2024; Accepted: 01 March 2024;
Published: 18 March 2024.
Edited by:
Enrico Doria, University of Pavia, ItalyReviewed by:
Ozkan Kaya, Erzincan Horticultural Research Institute, TürkiyeCopyright © 2024 Mosoh, Khandel, Verma and Vendrame. This is an open-access article distributed under the terms of the Creative Commons Attribution License (CC BY). The use, distribution or reproduction in other forums is permitted, provided the original author(s) and the copyright owner(s) are credited and that the original publication in this journal is cited, in accordance with accepted academic practice. No use, distribution or reproduction is permitted which does not comply with these terms.
*Correspondence: Dexter Achu Mosoh, bW9zb2hkZXh0ZXJAaG90bWFpbC5jb20=
†ORCID: Dexter Achu Mosoh, orcid.org/0000-0001-9721-0263
Ashok Kumar Khandel, orcid.org/0000-0002-0725-8165
Sandeep Kumar Verma, orcid.org/0000-0002-9324-747X
Wagner A. Vendrame, orcid.org/0000-0001-6391-7623
Disclaimer: All claims expressed in this article are solely those of the authors and do not necessarily represent those of their affiliated organizations, or those of the publisher, the editors and the reviewers. Any product that may be evaluated in this article or claim that may be made by its manufacturer is not guaranteed or endorsed by the publisher.
Research integrity at Frontiers
Learn more about the work of our research integrity team to safeguard the quality of each article we publish.