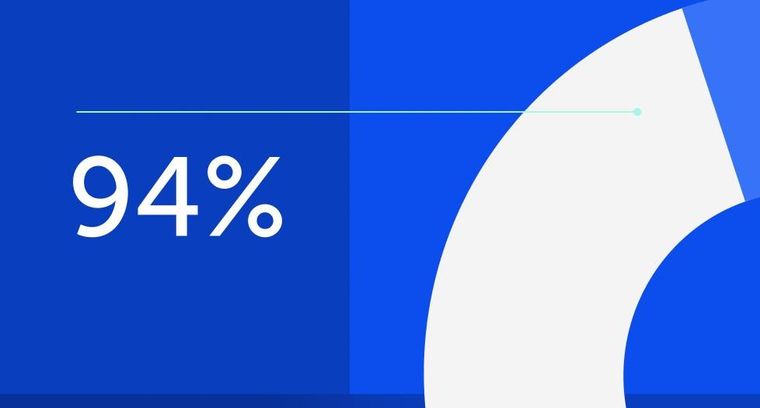
94% of researchers rate our articles as excellent or good
Learn more about the work of our research integrity team to safeguard the quality of each article we publish.
Find out more
ORIGINAL RESEARCH article
Front. Hematol., 25 August 2023
Sec. Immunobiology and Immunotherapy
Volume 2 - 2023 | https://doi.org/10.3389/frhem.2023.1144418
This article is part of the Research TopicEditors' Showcase: Immunobiology and ImmunotherapyView all 4 articles
Allogeneic hematopoietic cell transplantation (HCT) is often complicated by graft versus host disease (GvHD), an alloreactive immune response triggered by tissue damage. Interleukin (IL)-22 producing type 3 innate lymphoid cells (ILC3) protect epithelial tissues against chemo(radio)therapy-induced damage, suppress alloreactive T cells and mitigate acute GvHD symptoms after allogeneic HCT. Relatively high numbers of ILC before and after allogeneic HCT has been associated with significantly reduced tissue damage and less acute GvHD. While most transplantation conditioning and GvHD prophylaxis regimens are aimed at eliminating host and alloreactive donor lymphocytes, the effect of these regimens on ILC remain elusive. Here, we studied the effect of conditioning chemotherapy and immunosuppressive agents on the survival, proliferation, activation and function of human ILC3 in vitro. Tonsil-derived ILC3 were activated and incubated with agents commonly used to prevent and treat GvHD. While fludarabine, rapamycin, mycophenolic acid and prednisolone suppressed ILC3 to a similar degree as T cells, the effect of other agents, including cyclosporine A, methotrexate, imatinib, ibrutinib and ruxolitinib, was milder on ILC3 than on T cells. ILC3 are less sensitive to immunosuppressants potentially because of their expression of functionally active ATP Binding Cassette Subfamily B Member 1 (ABCB1) drug exporter proteins. This suggests less intracellular accumulation of immunosuppressive agents, which renders ILC3 resistant to these compounds. The present findings may help to develop strategies to simultaneously maintain the tissue protective properties of ILC3 and at the same time suppress alloreactive lymphocytes, which is important in the prevention and treatment of acute GvHD.
Allogeneic hematopoietic cell transplantation (HCT) is crucial in the treatment of hematologic malignancies, including intermediate- or high risk acute myeloid leukemia (AML) and myelodysplastic syndrome (MDS). Allogeneic HCT is life-saving when donor lymphocytes induce an anti-tumor immune response that eliminates residual malignant cells. However, alloreactive immune responses often turn against non-malignant cells and tissues of transplant recipients, leading to graft versus host disease (GvHD), which is a major contributor to the high morbidity and mortality associated with allogeneic HCT (1).
A key feature of acute GvHD pathophysiology is tissue damage, which is inflicted by pre-transplantation conditioning chemo- and radiotherapy or by opportunistic infections, such as cytomegalovirus (CMV) reactivation in the gastro-intestinal epithelium (2). Tissue damage leads to the release of pro-inflammatory signals, including damage-associated molecular patterns (DAMP), translocation of bacteria and release of pathogen-associated molecular patterns (PAMP) that ultimately activate alloreactive immune cells. The risk to develop acute GvHD is proportional to the level of tissue damage. For example, non-myeloablative or reduced-intensity allogeneic HCT is associated with a significantly lower incidence of acute GvHD than myeloablative allogeneic HCT (3).
Innate lymphoid cells (ILC) are tissue resident cells with immune regulatory and tissue reparative properties. These cells lack rearranged antigen-specific receptors, and respond rapidly to environmental cues. ILC play essential roles in the homeostatic repair and maintenance of epithelial barriers. In particular NKp44-expressing ILC3 modulate gut epithelial homeostasis via the production of interleukin (IL)-22. In murine acute GvHD models it has been demonstrated that IL-22 enhanced intestinal stem cell recovery with consequent reduced epithelium barrier damage and better restoration of the intestinal epithelium (4, 5). In addition, ecto-enzyme expressing ILC3 have shown to be able to convert pro-inflammatory extracellular ATP into adenosine, which suppresses activation of T cells (6). Furthermore, we have demonstrated that high levels of the ILC pool after remission induction chemotherapy, before allogeneic HCT, and early after allogeneic HCT was associated with reduced epithelial damage (i.e. mucositis) and a lower incidence of acute GvHD (7, 8). Notably, in patients with acute GvHD of the intestine, affected tissues were depleted of ecto-enzyme expressing ILC3, corroborating the potential significance of these cells in vivo (6).
Together, these studies suggest that ILC3 can mitigate or prevent acute GvHD and that rapid reconstitution and recovery of a functional ILC population may be prognostically beneficial for allogeneic HCT recipients. However, the key factors that determine ILC reconstitution after allogeneic HCT remain to be determined. Also, it remains elusive whether commonly used conditioning regimens deplete ILC and how immunosuppressants that are used to prevent or treat GvHD affect ILC reconstitution and function. Here we demonstrate that ILC3 are less sensitive to certain immunosuppressive agents compared to T cells, which may result from increased expression and functional activity of the drug exporter ATP Binding Cassette Subfamily B Member 1 (ABCB1) on ILC3. These findings may offer rationale to adjust GvHD prevention regimens to protocols aimed at maintaining the ILC pool while suppressing alloreactive T cells.
Study protocols were approved by the Medical Ethical Committee of the Amsterdam UMC, location AMC, Amsterdam, The Netherlands, following the Declaration of Helsinki protocols. Tonsils were obtained ‘cold’ (non-inflamed) from pediatric- and adult tonsillectomies. Donors, both male and female, ranged in age from 16-35- years. Tonsil tissue was mechanically cut in small pieces and dispersed by using the stomacher 80 Biomaster and tissue fragments were plunged through a steel strainer. Mononuclear cells were isolated by density gradient centrifugation using Ficoll-Paque Plus medium (GE Healthcare). Prior to sorting ILC3, tonsil mononuclear cell samples were magnetically depleted of T cells and B cells by labeling these cells with FITC-conjugated anti-CD3 and anti-CD19 (BioLegend) plus anti FITC microbeads (Miltenyi). ILC3 were sorted as Lineage (CD1a, CD3, CD4, CD14, CD16, CD19, CD34, CD94, CD123, BDCA2, FcER1α, TCRαβ, TCRγδ) negative, CD45+CD127+CD161+CRTH2-CD117+NKp44+ cells. T cells from the same tonsil were negatively sorted (to avoid CD3-antibody induced T cell activation) as CD45+CD19-CD14-CD16-CD56- cells on a FACSAria II (BD). Sorted cells were >95% CD3+.
Freshly sorted tonsillar ILC3 (1x104) and T cells (10x104) were rested overnight in 96-well round bottom plates, and incubated in Yssel’s medium (made ‘in house’) (9) supplemented with 1% (vol/vol) human AB serum and IL-2 (10 U/ml; Novartis). ILC3 and T cells were pre-incubated for 1 hour with IL-2 (100 U/ml; Novartis), IL-1β and IL-23 (both 50 ng/ml; R&D systems) (10), or anti-CD3/anti-CD28 Dynabeads™ (ratio 50 cells:1 bead; Thermo Fisher Scientific), respectively, and cultured for 4 days in the presence of immunosuppressive agents at dosages reported in literature (Supplemental Table 1) (11–23), or vehicle control (dimethyl sulfoxide (DMSO) or sodium chloride). DMSO and sodium chloride were diluted equal to the highest drug concentration. The final concentration of DMSO never exceeded 0.1%. Immunosuppressive agents were purchased from Selleck and dissolved in DMSO according to the manufacturer’s instructions.
To measure proliferation, cells were incubated with CellTrace Violet (Invitrogen) according to manufacturer’s instructions. To phenotype ILC3 and T cells, the following antibodies were used at recommended concentrations: fluorescein isothiocyanate (FITC)-conjugated anti-CD1a (HI149), anti-CD3 (OKT3), anti-CD4 (RPA-T4), anti-CD14 (HCD14), anti-CD16 (3G8), anti-CD19 (HIB19), anti-CD34 (581), anti-CD94 (DX22), anti-CD123 (6H6), anti-BDCA2 (CD303; 201A), anti-FcER1α (AER-37), anti-TCRαβ (IP26), anti-TCRγδ (B1); allophycocyanin (APC)-conjugated anti-CD56 (HCD56); APC-cyanine (Cy)7-conjugated anti-CD3 (UCHT1); Alexa Fluor (AF) 700-conjugated anti-CD45 (HI30); AF647–conjugated anti-NKp44 (P44-8); phycoerythrin (PE)-Cy7–conjugated anti-CD127 (A019D5); PE-CF594-conjugated anti-CRTH2 (BM16); brilliant violet (BV) 510-conjugated anti-CD161 (HP-3G10); BV605-conjugated anti-CD25 (BC96); PE-conjugated anti-CD243 (ABCB1; UIC2), AF594-conjugated anti-MRP1 (ABCC1) (QCRL-1) (all BioLegend) and PE-Cy5.5–conjugated anti-CD117 (104D2; Beckman Coulter). Appropriate isotype control antibodies (Supplemental Table 2) and unstained cells were used for the identification of cells that stained positive for each antibody used. Cell viability was measured with the LIVE/DEAD™ Fixable Green Dead Cell Stain (Invitrogen) or Fixable Viability Dye eFluor780 (eBiosciences) according to manufacturer’s instructions. For drug transporter experiments, cells were cultured in the presence or absence of the ABC-transporter antagonist verapamil (5 µg/ml) and cyclosporine A for 4 days and then incubated with pre-warmed 20 nM MitoTracker Green (MTG; Invitrogen) for 30 min. Cells were acquired on a LSRFortessa cytometer (BD Biosciences) and analyzed using FlowJo software (version 10; TreeStar). The percentage divided cells was determined by subtracting the percentage of undivided cells (generation 0) from the viable cells.
To analyze the effect of immunosuppressive agents on cellular cytokine secretion, supernatants from each culture were harvested and analyzed for the presence of IL-22 and interferon-gamma (IFN-γ) using Invitrogen’s enzyme-linked immunosorbent assay (ELISA) kit according to manufacturer’s instructions.
All statistical analysis was performed using GraphPad Prism 7 for Windows. Statistical significance was determined with the nonparametric paired Wilcoxon test.
We previously demonstrated that AML remission-induction chemotherapy depletes ILC (7, 8), but the effect of pre-transplantation conditioning therapy on the ILC population is unknown. We investigated the effect of fludarabine and antithymocyte globuline (ATG), the drugs most commonly used in conditioning regimens in our institute, on human NKp44+ ILC3 sorted from tonsils and stimulated in vitro for 4 days. As a control, we used T cells that were sorted from the same tonsils. ILC3 and T cells were stimulated with cytokines (IL-2, IL-1β, IL-23) or anti-CD3/CD28 beads, respectively, and incubated with fludarabine or ATG at concentrations reported in literature (12–14). Fludarabine significantly reduced the viability of stimulated ILC3, like T cells, in a dose-dependent manner (Figures 1A–C). It should be noted that we titrated fludarabine concentrations in such a way that not all ILC and T cells were lost. ILC3 that survived incubation with fludarabine had lower CD127 expression, and a higher capacity to produce IL-22 than ILC3 that were not incubated with fludarabine (Supplemental Figure 1). This was similar to fludarabine-surviving T cells, that produced higher levels of IFN-γ (Supplemental Figure 1). While ATG induced proliferation of T cells, as published previously (12), ATG did not significantly affect ILC3 viability, proliferation or cytokine production (Figures 1B, C and Supplemental Figure 1).
Figure 1 Conditioning chemo-immunotherapy is cytotoxic to ILC3. (A) Representative experiment of tonsil-sorted, CellTrace Violet-labeled, IL-2/IL-1β/IL-23 stimulated NKp44+ ILC3 and anti-CD3/CD28 dynabead stimulated T cells cultured in the presence of vehicle or fludarabine and analyzed by flow cytometry after 4 days. Live cells were gated as indicated, numbers represent percentage of living cells. (B) Cell viability and (C) cell division for NKp44+ ILC3 (upper panels) and T cells (lower panels) treated with fludarabine (Flud; n=6) or ATG (n=5) as fold change relative to vehicle control. Each data point indicates a single donor. Error bars indicate mean percentages ± SD. Asterisks indicate p<0.05. **, p<0.01; ***, p<0.001.
We next investigated the effect of immunosuppressive agents on ILC3 proliferation, activation and cytokine production (see for representative flow cytometry examples Supplemental Figure 2). We tested cyclosporine A, rapamycin, mycophenolic acid, methotrexate, prednisolone, the tyrosine kinase inhibitor imatinib, the JAK2 inhibitor ruxolitinib and the protein kinase inhibitor ibrutinib, all immunosuppressive agents that are more or less commonly used to prevent or treat acute GvHD. Starting concentrations were based on reports in literature (see Materials and Methods) and titrated to reach concentrations that were effective on T cells, our reference population, but not toxic to T cells as we needed life cells to study the effects of drug on cell proliferation, activation and cytokine secretion. In all experiments, donor to donor variations were observed. This might be attributed to differences in age or gender, but this is outside the scope of this study. At higher concentrations, cyclosporine A induced death of T cells, but had no effect on ILC viability, while mycophenolic acid induced death of ILC3 but did not affect T cell viability. None of the other immunosuppressive agents had a significant effect on the survival of ILC3 and T cells (Figure 2A). All immunosuppressants attenuated proliferation of T cells in a dose-dependent manner, as expected, but their effect on proliferation of ILC3 was less uniform. Rapamycin, mycophenolic acid and prednisolone significantly suppressed ILC3 proliferation (Figure 2B). Under normal circumstances, ILC downregulate CD127 when activated, most likely as a self-regulatory mechanism, but incubation of ILC3 with rapamycin, mycophenolic acid and prednisolone led to an increase in CD127 expression (Supplemental Figure 3). Cyclosporine A, methotrexate, imatinib, ibrutinib and ruxolitinib did not mitigate ILC3 proliferation (Figure 2B). Activation of ILC3, as measured by CD25 expression, was significantly suppressed only by mycophenolic acid and ibrutinib (Figure 2C). In contrast, all drugs, except methotrexate and prednisolone, inhibited the activation of T cells. Only two agents significantly suppressed cytokine secretion compared to the vehicle control: prednisolone significantly inhibited IL-22 secretion by ILC3, and cyclosporine A inhibited IFN-γ secretion by T cells (Figure 2D). In addition, at higher concentrations, IL-22 production by ILC3 was significantly increased in the presence of ruxolitinib, whereas IFN-γ production by T cells tended to be reduced. Collectively, these results demonstrate that suppressive agents commonly used to prevent or treat GvHD do not suppress the proliferation, activation or cytokine production of ILC3.
Figure 2 Immunosuppressive agents differentially affect ILC3 in comparison to T cells. Tonsil-sorted, CellTrace Violet-labeled IL-2/IL-1β/IL-23 stimulated NKp44+ ILC3 and anti-CD3/CD28 dynabeads stimulated T cells were incubated with vehicle or immunosuppressive agents as indicated. Fold change in the percentage of (A) living cells, (B) divided cells and (C) activated (CD25+) cells are depicted. (D) Signature cytokine production by activated ILC3 (IL-22) and T cells (IFN-γ) measured by ELISA and normalized to 1000 cells relative to control. CsA, cyclosporine A; Rapa, rapamycin; MPA, mycophenolic acid; MTX, methotrexate; Pred, prednisolone; Ima, imatinib; Ibr, ibrutinib; Rux, ruxolitinib; n=7 for all conditions except MTX (n=6). Each data point indicates a single donor. Error bars indicate mean ± SD. Asterisks indicate p<0.05.
In vivo ILC3 and T cells do not operate as individual subsets. In fact, they may influence each other’s behavior in response to stimulating signals and drugs. We therefore co-cultured ILC3 and T cells, in the absence and presence of cyclosporine A, an agent that suppressed T cell activation and proliferation, but not ILC3 when cultured separately (Figure 2). Stimulation of co-cultured ILC3 and T cells with IL-2/IL-1β/IL-23 and anti-CD3/CD28 beads induced proliferation and CD25 expression of both ILC3 and T cells (Figures 3A, B) comparable to when co-cultured subsets were stimulated separately (Figure 2). Cell viability of ILC3 and T cells was not affected under these conditions (Supplemental Figure 4). Adding cyclosporine A to the co-culture significantly suppressed T cell proliferation and activation, but did not affect ILC3 (Figures 3A, B). These data reinforce our finding that cyclosporine A differentially affects ILC3 and T cells, also when ILC3 and T cells are co-cultured.
Figure 3 Cyclosporine A specifically affects T cells but not ILC3 in co-culture. CellTrace labeled ILC3 and T cells were co-cultured, stimulated with IL-2/IL-1β/IL-23 and/or anti-CD3/CD28 dynabeads and incubated with vehicle control or cyclosporine A (CsA) as indicated. Fold change of the percentage of (A) divided cells and (B) activated (CD25+) cells are depicted. N=5 for conditions stimulated with IL-2/IL-1β/IL-23 and with IL-2/IL-1β/IL-23 + anti-CD3/CD28 beads; n=6 for conditions stimulated with anti-CD3/CD28 dynabeads and all CsA conditions. Each data point indicates a single donor. Error bars indicate mean ± SD; asterisks indicate p<0.05.
The observation that ILC3 appear to be unresponsive to the majority of immunosuppressive agents tested here suggests that a general mechanism underlies the drug resistance. We hypothesized that ATP-binding cassette transporters, which are membrane proteins able to efflux drugs from cells, may be involved. We focused on two well-known members of the ABC family, ATP Binding Cassette Subfamily B Member 1 (ABCB1) and ATP Binding Cassette Subfamily C Member 1 (ABCC1) (24, 25). Unstimulated, freshly isolated ILC3 and T cells showed very limited expression of ABCB1 and ABCC1 (Figures 4A, B). Following stimulation with IL-2/IL-1β/IL-23 for 4 days, ILC3 upregulated ABCB1 expression, and to a lesser extent ABCC1. Only a small percentage of stimulated T cells expressed ABCB1 and ABCC1. The proportion of ABCB1-expressing ILC3 was significantly higher compared to ABCB1-expressing T cells (Figures 4A, B).
Figure 4 Activated ILC3 express the drug exporter ABCB1. (A) Representative flow cytometry experiment and (B) pooled data of ABCB1 and ABCC1 expression on unstimulated and stimulated ILC3 (IL-2/IL-1β/IL-23) and T cells (anti-CD3/CD28). N=2 for unstimulated ILC3 and T cells and n=5 for stimulated ILC3 and T cells. (C, D) Stimulated and MTG labeled ILC3 and T cells were cultured with vehicle control or verapamil (Vera; 5 µg/ml) for 4 days. (C) Representative flow cytometry experiment and (D) pooled data for expression of MTG (mean fluorescence intensity (MFI)); n=8. (E) Stimulated ILC3 and T cells cultured with vehicle control or CsA with or without verapamil. Divided cells are depicted as fold change compared to the vehicle control. Error bars indicate mean percentages ± SD. Asterisks indicate p<0.05. Each data point indicates a single donor.
We then investigated whether selective blocking of the ABCB1 transporter could reverse the relatively high resistance of ILC3 for drugs as compared to T cells. For these experiments we measured the efflux of MitoTracker Green (MTG), which is a dye that passively diffuses across the plasma membrane and binds to active mitochondria (26). Activated ILC3 were incubated with MTG in the presence of the ABCB1 inhibitor verapamil. The MTG levels in ILC3 were significantly higher in the presence of verapamil compared to ILC3 cultured without this ABCB1 inhibitor (also when the outlier was excluded from analysis (data not shown)) and comparable to the levels observed in activated T cells (Figures 4C, D). Moreover, co-incubation of stimulated ILC3 with verapamil and cyclosporine significantly reduced proliferation of these cells (Figure 4E). These results suggest that the differential effects of immunosuppressive agents on ILC3 compared to T cells may be related to upregulation and functional activity of the ABCB1 transporter on activated ILC3.
ILC are innate immune cells enriched at epithelial barrier surfaces such as the gut, lung and skin, where these cells produce inflammatory and regulatory cytokines in response to local injury. We and others have demonstrated the importance of ILC in the prevention of tissue damage and subsequent GvHD in experimental GvHD models and in allogeneic HCT recipients (5, 7, 8, 27, 28). It is unknown to what extent ILC are depleted by pre-transplantation conditioning regimens, and whether ILC are functionally suppressed by the immunosuppressive agents used to prevent acute GvHD. Therefore, we investigated the effect of commonly used pre- and post-transplantation immunomodulatory agents on the survival, proliferation, activation and cytokine production of ILC3 in vitro. We demonstrated that fludarabine depleted ILC3 and that ATG tended to lower ILC3 in a dose-dependent manner. A number of agents that suppress T cell proliferation and function, and as such are commonly used to prevent or treat acute and chronic GvHD, had differential effects on ILC3. In particular cyclosporine A, methotrexate and the more recently introduced immunomodulatory agents imatinib, ibrutinib and ruxolitinib had no effect on ILC3, contrasting their potent inhibitory effects on T cells. This drug refractoriness of ILC3 may be related to the expression of the drug transporter ABCB1 on stimulated ILC3, which allows the efflux of drugs from ILC3.
Our finding that fludarabine is toxic to ILC3 is in line with the observation that circulating numbers of ILC are low early after allogeneic HCT (8). ILC reconstitution after allogeneic HCT is often delayed, and our results suggest that this may be related to the use of immunosuppressive agents. For example, mycophenolic acid is typically used to prevent acute GVHD as it effectively dampens T cell alloreactivity, but as it suppressed ILC3 proliferation in vitro it is likely to delay ILC recovery in vivo.
Acute GvHD frequently occurs after allogeneic HCT, despite the use of lymphocyte depleting agents and of drugs that suppress surviving and recovering donor lymphocytes. In part this may be related to incomplete depletion and suppression of alloreactive cells. In recent years it has become apparent, however, that tissue damage and specifically disruption of epithelial barrier homeostasis also plays an important role in the pathophysiology of acute GvHD. Several factors may contribute to barrier loss, in addition to chemotherapy and radiotherapy. For example, damage to the microbiome deprives the gastro-intestinal epithelium from factors that are imperative for its maintenance and repair, such as aryl hydrocarbon receptor (AhR) ligands and short chain fatty acids (29). Loss and slow reconstitution of tissue reparative cells, such as IL-22 producing ILC3, further enforce the disruption of epithelial barrier homeostasis, as has been demonstrated in GvHD mouse models and in patients with GvHD (30, 31). As ‘graft versus tumor’ immunity and GvHD are intimately linked and often difficult to untangle, since both depend on alloreactive immune responses, it may be beneficial to focus on prevention of tissue damage and enhancement of tissue repair instead of further suppressing alloreactive immune responses. ILC, in particular IL-22 producing ILC3, are important candidates for such an approach. The choice of agents that do suppress alloreactive T cells, but not tissue reparative ILC3, may be of additive value in designing an optimal regimen to prevent or treat GvHD in allogeneic HCT patients. Ruxolitinib is particularly interesting in this context as it suppressed T cell proliferation, activation and cytokine production and enhanced IL-22 production by ILC3.
While steroids are the first line therapy for acute GvHD, about half of the patients with GvHD are not responsive to corticosteroid therapy. This has been attributed to differential effects of steroids on T cell subsets, with T helper (Th)1 cells being more receptive to the immunosuppressive effect of corticosteroids than Th2 or Th17 cells (32). Alternatively, steroid-refractory acute GvHD could represent a different entity, less driven by T cells and more driven by tissue damage (31). In this context it is interesting to note that prednisolone significantly reduced IL-22 production by ILC3. In patients with acute GvHD where tissue damage is dominant, prednisolone may actually aggravate disease by suppressing ILC3 mediated IL-22 production. It should be noted that patients with steroid-refractory acute GvHD often benefit from ruxolitinib (33), perhaps via enhanced IL-22 production by ILC3.
This study has several limitations. It is very difficult to verify to what extent drug concentrations used in vitro are representative for the in vivo situation. We were not able to verify findings on tissue derived ILC3 from allogeneic HCT recipients, because ILC numbers that can be derived from these patients are too low, in particular in the presence of acute GvHD. Experiments were instead performed on ILC3 isolated from tonsils, as tonsillar and gut ILC3 are phenotypically and functionally similar, and depend on the same transcription factors (6, 34–36).
In conclusion, immunosuppressive agents commonly used to prevent or treat acute GvHD do not seem to hamper ILC3 proliferation or activation. Given the importance of ILC3 for the maintenance of tissue homeostasis, prevention of tissue damage and prevention of GvHD these findings may favor the use of cyclosporine A and methotrexate rather than mycophenolic acid in GvHD prevention regimens, and offers rationale for the use of ruxolitinib and other agents in the treatment of GvHD.
The original contributions presented in the study are included in the article/Supplementary Material. Further inquiries can be directed to the corresponding author.
The studies involving humans were approved by Amsterdam UMC Medical Ethical Committee. The studies were conducted in accordance with the local legislation and institutional requirements. The participants provided their written informed consent to participate in this study.
SO designed and performed experiments, analyzed data, and drafted the manuscript. NH performed experiments, analyzed the data and revised the manuscript. VH helped optimize the experimental conditions and revised the manuscript. BB and MH conceived and supervised the study and wrote the manuscript. All authors contributed to the article and approved the submitted version.
This study was financially supported by the Dutch Research Council/Netherlands Organisation for Scientific Research (NWO ZonMW VIDI grant 016.156.362) and by the Landsteiner Foundation for Blood Transfusion Research (LSBR grant #1922).
We thank the surgeons of the department of Ear, Nose and Throat Diseases of the Onze Lieve Vrouwe Gasthuis, Amsterdam, for collecting tonsil tissues.
The authors declare that the research was conducted in the absence of any commercial or financial relationships that could be construed as a potential conflict of interest.
All claims expressed in this article are solely those of the authors and do not necessarily represent those of their affiliated organizations, or those of the publisher, the editors and the reviewers. Any product that may be evaluated in this article, or claim that may be made by its manufacturer, is not guaranteed or endorsed by the publisher.
The Supplementary Material for this article can be found online at: https://www.frontiersin.org/articles/10.3389/frhem.2023.1144418/full#supplementary-material
1. Gooley TA, Chien JW, Pergam SA, Hingorani S, Sorror ML, Boeckh M, et al. Reduced mortality after allogeneic hematopoietic-cell transplantation. N Engl. J. Med. (2010) 363(22):2091–101. doi: 10.1056/NEJMoa1004383
2. Jenq RR, van den Brink MR. Allogeneic haematopoietic stem cell transplantation: Individualized stem cell and immune therapy of cancer. Nat. Rev. Cancer (2010) 10(3):213–21. doi: 10.1038/nrc2804
3. Zeiser R, Blazar BR. Acute graft-versus-host disease - biologic process, prevention, and therapy. N Engl. J. Med. (2017) 377(22):2167–79. doi: 10.1056/NEJMra1609337
4. Hanash AM, Dudakov JA, Hua G, O'Connor MH, Young LF, Singer NV, et al. Interleukin-22 protects intestinal stem cells from immune-mediated tissue damage and regulates sensitivity to graft versus host disease. Immunity (2012) 37(2):339–50. doi: 10.1016/j.immuni.2012.05.028
5. Lindemans CA, Calafiore M, Mertelsmann AM, O'Connor MH, Dudakov JA, Jenq RR, et al. Interleukin-22 promotes intestinal-stem-cell-mediated epithelial regeneration. Nature (2015) 528(7583):560–4. doi: 10.1038/nature16460
6. Hazenberg MD, Haverkate NJE, van Lier YF, Spits H, Krabbendam L, Bemelman WA, et al. Human ectoenzyme-expressing ilc3: Immunosuppressive innate cells that are depleted in graft-versus-host disease. Blood Adv. (2019) 3(22):3650–60. doi: 10.1182/bloodadvances.2019000176
7. Kroeze A, van Hoeven V, Verheij MW, Turksma AW, Weterings N, van Gassen S, et al. Presence of innate lymphoid cells in allogeneic hematopoietic grafts correlates with reduced graft-versus-host disease. Cytotherapy (2022) 24(3):302–10. doi: 10.1016/j.jcyt.2021.10.011
8. Munneke JM, Bjorklund AT, Mjosberg JM, Garming-Legert K, Bernink JH, Blom B, et al. Activated innate lymphoid cells are associated with a reduced susceptibility to graft-versus-host disease. Blood (2014) 124(5):812–21. doi: 10.1182/blood-2013-11-536888
9. Yssel H, De Vries JE, Koken M, Van Blitterswijk W, Spits H. Serum-free medium for generation and propagation of functional human cytotoxic and helper T cell clones. J. Immunol. Methods (1984) 72(1):219–27. doi: 10.1016/0022-1759(84)90450-2
10. van Hoeven V, Munneke JM, Cornelissen AS, Omar SZ, Spruit MJ, Kleijer M, et al. Mesenchymal stromal cells stimulate the proliferation and il-22 production of group 3 innate lymphoid cells. J. Immunol. (2018) 201(4):1165–73. doi: 10.4049/jimmunol.1700901
11. Allison AC, Eugui EM. Mycophenolate mofetil and its mechanisms of action. Immunopharmacology (2000) 47(2-3):85–118. doi: 10.1016/s0162-3109(00)00188-0
12. Broady R, Yu J, Levings MK. Atg-induced expression of foxp3 in human cd4(+) T cells in vitro is associated with T-cell activation and not the induction of foxp3(+) T regulatory cells. Blood (2009) 114(24):5003–6. doi: 10.1182/blood-2009-04-214437
13. Consoli U, El-Tounsi I, Sandoval A, Snell V, Kleine HD, Brown W, et al. Differential induction of apoptosis by fludarabine monophosphate in leukemic B and normal T cells in chronic lymphocytic leukemia. Blood (1998) 91(5):1742–8. doi: 10.1182/blood.V91.5.1742
14. Gassner FJ, Weiss L, Geisberger R, Hofbauer JP, Egle A, Hartmann TN, et al. Fludarabine modulates composition and function of the T cell pool in patients with chronic lymphocytic leukaemia. Cancer Immunol. Immunother. (2011) 60(1):75–85. doi: 10.1007/s00262-010-0920-3
15. He X, Smeets RL, Koenen HJ, Vink PM, Wagenaars J, Boots AM, et al. Mycophenolic acid-mediated suppression of human cd4+ T cells: More than mere guanine nucleotide deprivation. Am. J. Transplant. (2011) 11(3):439–49. doi: 10.1111/j.1600-6143.2010.03413.x
16. Humar R, Kiefer FN, Berns H, Resink TJ, Battegay EJ. Hypoxia enhances vascular cell proliferation and angiogenesis in vitro via rapamycin (Mtor)-dependent signaling. FASEB J. (2002) 16(8):771–80. doi: 10.1096/fj.01-0658com
17. Kay JE, Kromwel L, Doe SE, Denyer M. Inhibition of T and B lymphocyte proliferation by rapamycin. Immunology (1991) 72(4):544–9.
18. Koehl GE, Wagner F, Stoeltzing O, Lang SA, Steinbauer M, Schlitt HJ, et al. Mycophenolate mofetil inhibits tumor growth and angiogenesis in vitro but has variable antitumor effects in vivo, possibly related to bioavailability. Transplantation (2007) 83(5):607–14. doi: 10.1097/01.tp.0000253756.69243.65
19. Lillehoj HS, Malek TR, Shevach EM. Differential effect of cyclosporin a on the expression of T and B lymphocyte activation antigens. J. Immunol. (1984) 133(1):244–50.
20. McCormack G, O'Donoghue D, Baird A. In-vitro cyclosporin sensitivity of proliferating lymphocytes is predictive of in-vivo therapeutic response in ulcerative colitis. Aliment Pharmacol. Ther. (2001) 15(5):665–8. doi: 10.1046/j.1365-2036.2001.00977.x
21. Szamel M, Martin M, Resch K. Inhibition of lymphocyte activation by cyclosporin A: Interference with the early activation of the membrane phospholipid metabolism in rabbit lymphocytes stimulated with concanavalin a, anti-rabbit immunoglobulin or the ca2+ Ionophore a 23187. Cell Immunol. (1985) 93(2):239–49. doi: 10.1016/0008-8749(85)90131-5
22. Traitanon O, Mathew JM, La Monica G, Xu L, Mas V, Gallon L. Differential effects of tacrolimus versus sirolimus on the proliferation, activation and differentiation of human B cells. PLoS One (2015) 10(6):e0129658. doi: 10.1371/journal.pone.0129658
23. Seggewiss R, Lore K, Greiner E, Magnusson MK, Price DA, Douek DC, et al. Imatinib inhibits T-cell receptor-mediated T-cell proliferation and activation in a dose-dependent manner. Blood (2005) 105(6):2473–9. doi: 10.1182/blood-2004-07-2527
24. Rebecchi IM, Rodrigues AC, Arazi SS, Genvigir FD, Willrich MA, Hirata MH, et al. Abcb1 and abcc1 expression in peripheral mononuclear cells is influenced by gene polymorphisms and atorvastatin treatment. Biochem. Pharmacol. (2009) 77(1):66–75. doi: 10.1016/j.bcp.2008.09.019
25. Silverton L, Dean M, Moitra K. Variation and evolution of the abc transporter genes abcb1, abcc1, abcg2, abcg5 and abcg8: Implication for pharmacogenetics and disease. Drug Metabol Drug Interact. (2011) 26(4):169–79. doi: 10.1515/DMDI.2011.027
26. Dimeloe S, Frick C, Fischer M, Gubser PM, Razik L, Bantug GR, et al. Human regulatory T cells lack the cyclophosphamide-extruding transporter abcb1 and are more susceptible to cyclophosphamide-induced apoptosis. Eur. J. Immunol. (2014) 44(12):3614–20. doi: 10.1002/eji.201444879
27. Bruce DW, Stefanski HE, Vincent BG, Dant TA, Reisdorf S, Bommiasamy H, et al. Type 2 innate lymphoid cells treat and prevent acute gastrointestinal graft-versus-host disease. J. Clin. Invest. (2017) 127(5):1813–25. doi: 10.1172/JCI91816
28. Ponce DM, Alousi AM, Nakamura R, Slingerland J, Calafiore M, Sandhu KS, et al. A phase 2 study of interleukin-22 and systemic corticosteroids as initial treatment for acute gvhd of the lower gi tract. Blood (2023) 141(12):1389–401. doi: 10.1182/blood.2021015111
29. Shono Y, van den Brink MRM. Gut microbiota injury in allogeneic haematopoietic stem cell transplantation. Nat. Rev. Cancer (2018) 18(5):283–95. doi: 10.1038/nrc.2018.10
30. Omar SZ, Blom B, Hazenberg MD. Innate lymphoid cells in treatment-induced gastrointestinal pathogenesis. Curr. Opin. Support Palliat Care (2020) 14(2):135–41. doi: 10.1097/SPC.0000000000000499
31. Voermans C, Hazenberg MD. Cellular therapies for graft-versus-host disease: A tale of tissue repair and tolerance. Blood (2020) 136(4):410–7. doi: 10.1182/blood.2019000951
32. Toubai T, Magenau J. Immunopathology and biology-based treatment of steroid-refractory graft-versus-host disease. Blood (2020) 136(4):429–40. doi: 10.1182/blood.2019000953
33. Luo C, Huang X, Wei L, Wu G, Huang Y, Ding Y, et al. Second-line therapy for patients with steroid-refractory agvhd: Systematic review and meta-analysis of randomized controlled trials. Front. Immunol. (2023) 14:1211171. doi: 10.3389/fimmu.2023.1211171
34. Artis D, Spits H. The biology of innate lymphoid cells. Nature (2015) 517(7534):293–301. doi: 10.1038/nature14189
35. Hazenberg MD, Spits H. Human innate lymphoid cells. Blood (2014) 124(5):700–9. doi: 10.1182/blood-2013-11-427781
Keywords: immunosuppressive therapy, ABCB drug exporter, ILC, GvHD, mucositis
Citation: Omar SZ, Haverkate NJE, van Hoeven V, Blom B and Hazenberg MD (2023) Drug exporter expression correlates with group 3 innate lymphoid cell resistance to immunosuppressive agents. Front. Hematol. 2:1144418. doi: 10.3389/frhem.2023.1144418
Received: 14 January 2023; Accepted: 07 August 2023;
Published: 25 August 2023.
Edited by:
Jaebok Choi, Washington University in St. Louis, United StatesReviewed by:
Diana Passaro, INSERM U1016 Institut Cochin, FranceCopyright © 2023 Omar, Haverkate, van Hoeven, Blom and Hazenberg. This is an open-access article distributed under the terms of the Creative Commons Attribution License (CC BY). The use, distribution or reproduction in other forums is permitted, provided the original author(s) and the copyright owner(s) are credited and that the original publication in this journal is cited, in accordance with accepted academic practice. No use, distribution or reproduction is permitted which does not comply with these terms.
*Correspondence: Mette D. Hazenberg, bS5kLmhhemVuYmVyZ0BhbXN0ZXJkYW11bWMubmw=
Disclaimer: All claims expressed in this article are solely those of the authors and do not necessarily represent those of their affiliated organizations, or those of the publisher, the editors and the reviewers. Any product that may be evaluated in this article or claim that may be made by its manufacturer is not guaranteed or endorsed by the publisher.
Research integrity at Frontiers
Learn more about the work of our research integrity team to safeguard the quality of each article we publish.