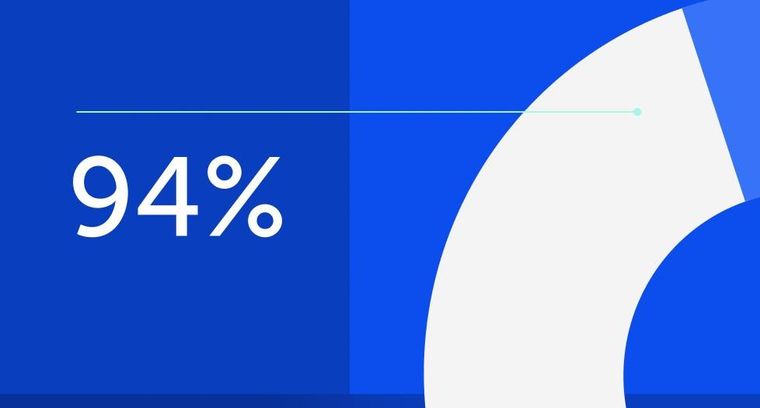
94% of researchers rate our articles as excellent or good
Learn more about the work of our research integrity team to safeguard the quality of each article we publish.
Find out more
REVIEW article
Front. Genome Ed., 18 March 2025
Sec. Genome Editing in Plants
Volume 7 - 2025 | https://doi.org/10.3389/fgeed.2025.1533197
This article is part of the Research TopicGenome Editing for Addressing the Challenges of Climate Change Adaptation in Agriculture Crops and LivestockView all articles
Climate change is a global concern for agriculture, food security, and human health. It affects several crops and causes drastic losses in yield, leading to severe disturbances in the global economy, environment, and community. The consequences on important staple crops, such as rice, maize, and wheat, will worsen and create food insecurity across the globe. Although various methods of trait improvements in crops are available and are being used, clustered regularly interspaced short palindromic repeats and CRISPR-associated protein 9 (CRISPR/Cas9) mediated genome manipulation have opened a new avenue for functional genomics and crop improvement. This review will discuss the progression in crop improvement from conventional breeding methods to advanced genome editing techniques and how the CRISPR/Cas9 technology can be applied to enhance the tolerance of the main cereal crops (wheat, rice, and maize) against any harsh climates. CRISPR/Cas endonucleases and their derived genetic engineering tools possess high accuracy, versatile, more specific, and easy to design, leading to climate-smart or resilient crops to combat food insecurity and survive harsh environments. The CRISPR/Cas9-mediated genome editing approach has been applied to various crops to make them climate resilient. This review, supported by a bibliometric analysis of recent literature, highlights the potential target genes/traits and addresses the significance of gene editing technologies in tackling the vulnerable effects of climate change on major staple crops staple such as wheat, rice, and maize.
The world is facing drastic climate change, with high global temperatures and elevated carbon dioxide (CO2) levels. This has resulted in extreme events, adversely affecting all dimensions of the world, including agriculture, biodiversity, and human community. The root cause of climate change is greenhouse gas emissions, mainly from anthropogenic activities, ascending the global surface temperature by 1.5°C since 1850 (Karavolias et al., 2021; Nunez et al., 2019). Recent reports confirmed that the year 2023 was the hottest in Earth’s history, which had several consequences such as drought, compound flooding, heavy precipitation, global sea rise, and upper sea acidification in certain regions of the planet (Li Z. et al., 2024). Rising temperatures are a warning sign for current agricultural production, although they have already started devastating effects worldwide. Climate change will mostly affect crop yield at lower latitudes more than at higher latitudes (Shukla et al., 2019). The situation of agriculture at lower latitudes might worsen with a temperature rise, whereas higher latitudes might benefit from higher temperatures, which would increase crop yield (Iizumi et al., 2018). More specifically, areas closer to the equator are more prone to desertification and eventual agricultural loss, which has already started in the Asian and African continents (Zougmoré et al., 2018). These regions are already facing high populations and unsustainable land management issues; therefore, their agricultural productivity and biodiversity are under great threat due to climate change (Viana et al., 2022). Increasing temperatures rise to over 35°C in California caused the browning of berries, reducing the yield by almost 50% (Kizildeniz et al., 2018).
The current scenario indicates that crop production in tropical areas will be most affected by high temperatures and droughts (Esquivel-Muelbert et al., 2019). It is estimated that food crop production in Africa will be reduced by 2.9% by 2050 owing to climate change. Global productivity losses disrupt other facets of the ecosystem.
The entire ecosystem relies on food supplies, occupying the highest agricultural land share. Studies have revealed that approximately 90% of food calories and 80% of the proteins and fats originate from agricultural land. Hence, agricultural land contributes to food security and various FAO sustainability goals (Avtar et al., 2020; FAO, 2017). However, most arable land has been degraded because of non-sustainable agricultural practices, including spraying chemical fertilizers, excess groundwater use, intensive farming, and deforestation. Such practices have increased greenhouse gas emissions, which are a major cause of temperature increases (Funk, 2021; Shahzad et al., 2021). Therefore, some areas of the globe might experience drought while others might be flooded owing to rising sea levels. Currently, areas suitable for crop production will soon become unsuitable (Iizumi et al., 2018). Therefore, identifying suitable areas for crop production is crucial for addressing the impacts of climate change. Several studies have focused on identifying suitable areas for agriculture in different countries (Musakwa, 2018). However, this alone is insufficient to overcome the effects of climate change.
A meta-analysis of about a hundred studies explained the impacts of climate change on biodiversity and found that a moderate rise in temperature can cause significant harm to biodiversity (Nunez et al., 2019). Owing to climate change pressure, food production needs to be enhanced, which requires more land, exacerbating biodiversity loss. For instance, the production of soybeans, palm oil, beef, and wood from 2000 to 2011 in seven countries was responsible for 40% of the deforestation of tropical forests and carbon losses (Henders et al., 2015; Ortiz et al., 2021).
Despite this, plants adapt extraordinary mechanisms to survive in the harsh climate. Such mechanisms involve root and leaf modification, stomatal regulation, osmotic adjustment, ion transport and sequestration, morphological behavior, and genetic adaptations. However, these processes require years to develop a climate-resilient plant (Krishna et al., 2023). Therefore, dealing with these issues in a short step is feasible using a genome-editing technique called clustered regularly interspaced short palindromic repeats and CRISPR-associated protein 9 (CRISPR/Cas9). Climate-smart crops in terms of increasing abiotic and biotic stress tolerance and high-yielding biofortified crops can be generated using this approach (Figure 1). Here, we summarize the progression in crop improvement from conventional breeding methods to advanced genome editing techniques and how the CRISPR/Cas9 technology can be applied to safeguard the main cereal crops (wheat, rice, and maize) from harsh climates.
Figure 1. Gene editing, a sustainable technology to reduce the Vulnerability of major Staple Crops to Climate Change (Graphical abstract).
Although climate change is progressing at an exceptional rate, it is not easy to envisage the loss it can cause to agriculture. Nevertheless, the scientific community has made tremendous efforts with conventional crop improvement techniques to combat the effects of climate change. Such approaches include breeding that produces superior varieties using donor and recipient plants with desired characteristics (Sharma et al., 2023; Van et al., 2022). This process was revolutionized in 1940–1950 when semi-dwarf wheat varieties were developed. Although hybrid varieties are superior and popular innovations, reaching the final product is time-consuming, costly, and requires intensive labor. Breeding is associated with the plant’s phenotypic trait, which is highly influenced by environmental factors, and requires several backcrosses to obtain the desired trait. Furthermore, conventional breeding methods result in selecting an inferior parent crop limiting the germplasm gene pool and causing genetic erosion (Krishna et al., 2023). Breeding can lead to the development of undesired traits because the transfer of genetic information cannot be controlled (Figure 2). Additionally, large arable land requirements with huge investments are another drawback of conventional breeding. The scientific community is making tremendous efforts using breeding to combat the effects of climate change. However, these efforts are insufficient, and more advanced strategies are required to improve agricultural techniques.
Figure 2. Conventional breeding versus Genome editing: This figure illustrates the advantages of genome editing over conventional breeding techniques. Created with BioRender.
Later, the emergence of recombinant DNA technology, where the genetic material of plants could be modified by inserting a foreign gene of interest to produce superior transgenic crops, also called genetically modified (GM) crops, changed the concept of producing superior varieties. Therefore, genetic manipulation can be performed in a controlled manner. Currently, GM crops, including BT cotton, corn, and soybean, are utilized worldwide, especially in the United States of America (USA) (Wechsler, 2018). Because this approach directly deals with transferring genetic information from one species to another, some myths addressing biodiversity and health concerns remain controversial. In addition, a long procedure that requires clinical trials and money expenditure is required to launch a GM product in the market (Van et al., 2022). Likewise, similar trials are required for genetically engineered plants produced via RNA interference (RNAi) technologies because of its several disadvantages, including, off-targeting effects that may lead to plant toxicity, development of insect resistance, incomplete or variable levels of silencing, and highly programmatic designing process (Sharma et al., 2023).
Gene-editing technologies have attracted the attention of the scientific community owing to their simplicity for designing, efficient editing, and accuracy. Genome editing technology uses molecular scissors to create double-stranded breaks in DNA, and the remaining part is undertaken by the host DNA repair machinery, which can either add or remove nucleotides randomly, leading to the formation of mutants. This modification can be achieved through site-specific insertion-deletion (indels), substitution, or epiallelic changes within the targetted DNA in a cell or organism. Genome editing is based on the principle of DNA repair, in which strand breaks are introduced using molecular scissors, such as endonucleases (Carroll, 2014), mega-nucleases (Gong and Golic, 2003), zinc finger nucleases (ZFNs) (Urnov et al., 2005), transcription activator-like effector nucleases (TALENs) (Sun and Zhao, 2013), and CRISPR/Cas9 (Jinek et al., 2012).
Among the above-mentioned methods CRISPR/Cas endonuclease based is the most popular, which has gained momentum in the last 10 years owing to its high efficiency, ease of use, and accuracy. CRISPR/Cas systems are diverse and adopted from bacteria and archaea. Currently, there are many tools such as CRISPRi, CRISPRa, base editor, gene knock-in, targeted protein tagging, and Viral mediated editing, that arose from the basic CRISPR/Cas9 system (Anzalone et al., 2020; Kampmann, 2018). Variants of Cas endonuclease, i.e., dCas9-foki, Cas9 nickase, HypaCas9, Sniper-Cas9, eSpCas9 (1.1), SpCas9-HF1, xCas9, evoCas9, SuperFi-Cas9, miCas9, evoCjCas9, SpRYc, KG, and SpdNG-QT.12j (Goldberg et al., 2021; Jeon et al., 2018; Karvelis et al., 2021; Kulcsár et al., 2022; Ma et al., 2020; Schmidheini et al., 2024; Schmidt et al., 2021; Schuler et al., 2022; Sun A. et al., 2023; Thakur et al., 2024; Wang et al., 2021a; Zhao et al., 2023). Moreover, Cas9 functional analogs such as, Cas12a-b, Cas12d-f, Cas12h-j, Cas121, Cas12n, Cas12 λ, Cas13 (C2c2), and Cas14 have been developed that extend the editing capability to the RNA and protein levels to enhance the performance of this technique (Hillary and Ceasar, 2022; Schindele et al., 2018; Yan et al., 2019). These all tools have been successfully applied to various crops for different purposes to alter the metabolic pathway (Ahmar and Gruszka, 2023; Kaur et al., 2020; Li D. et al., 2024; Ly et al., 2024; Toinga-Villafuerte et al., 2022; Wang J. D. et al., 2024; Xie Y. et al., 2024).
The detail mechanism of CRISPR/Cas9 for generating knockout events in plants is described in Figure 3. It has been applied to gene editing and transcriptional modulation of plants to improve various agronomical characteristics, such as drought tolerance, salinity tolerance, heat stress tolerance, disease resistance, nutritional enhancement, and yield improvement. Because plant phenology is affected by climate change events, this technology can also be used to control plant development-related factors for instance, flowering, male sterility, and photoperiod) (Cai et al., 2018; Liu et al., 2019; Shen et al., 2017). CRISPR/Cas9 derivatives, including prime and base editors, also provide opportunities to modify the plant genome precisely. Substantial research is currently underway to improve these techniques.
Figure 3. Mechanism of CRISPER/Cas9 in the plant: (1) The CRISPR/Cas9 system can be introduced into plant cells by Agrobacterium mediated transformation, protoplast transformation or particle bombardment. (2) The sgRNA guides the Cas9 to the target region of the genome. (3) Cas9 recognize the PAM region and creates double stranded cut. (4a) InDels are generated in the target site through the NHEJ repair system (4b) Precise corrections can be made in the DNA or directed sequences can be inserted through HDR repair system Created with BioRender.
Therefore, considering the vast potential of gene editing technologies, they may be the best solution for mitigating the effects of climate change (Singh et al., 2024a). This review describes the impact of climate change on food security and how it can affect the main cereal crops (wheat, rice, and maize) that are highly prone to climate change, and the research initiatives for their improvement via the CRISPR/Cas9 approach are also discussed in this review. The applications of this technology are extensive; however, certain limitations such as government regulations must be considered.
Wheat is an important cereal crop that serves two purposes: feed for the global community and support for the nation’s economy. Average wheat production worldwide is over 700 million tons, with China, India, the United States, the Russian Federation, and France being the top five wheat producers. The main exporters of wheat are the United States, Canada, France, Australia, and Russia, whereas the main importers are Egypt, Italy, Brazil, Japan, and Algeria (http://faostat.fao.org/). The overall production and trade of wheat reflects its significant role and demand in the global population. Approximately 95% of the total wheat produced worldwide is hexaploid bread wheat (Triticum aestivum sp. Aestivum, AABBDD, 2n = 6x = 42), whereas the remaining 5% is tetraploid durum wheat (Triticum turgidum sp. Durum, AABB, 2n = 4x = 28), also known as pasta wheat. The dough-forming ability of wheat flour increases the product range of wheat into bread, pasta, noodles, and biscuits. The key components responsible for dough formation are the grain storage proteins called gluten in wheat flour, whose interactions with water upon kneading form a proteinaceous structure (Shewry, 2019). Starch is another important component of wheat that promotes dough formation and causes gelatinization. In total, mature wheat grains contain approximately 13% water, 71% carbohydrates, 11% proteins, 2% lipids, 2% minerals, and 0.1% vitamins and phytochemicals, contributing significantly to human health (Wieser et al., 2020). Wheat has undoubtedly contributed significantly to global food security; however, wheat production is at great risk owing to climate change. As a C3 crop, wheat may benefit from high CO2 concentrations in the environment by improving water-use efficiency, photosynthesis, and transpiration. However, the grain quality can be negatively affected by higher levels of CO2. At higher temperatures, wheat productivity may decline because of a shorter crop season and an increase in transpiration (Bouras et al., 2019). A report published in Nature Climate Change estimated the impact of rising temperatures on global wheat yield using three independent models. The results indicated that the per degree rise in temperature can decline wheat productivity by 4.1%–6.4% (Liu et al., 2016). Various studies have shown that increasing temperature can have a drastic effect on wheat grain quality by shortening the grain-filling period, which will affect the gluten composition of wheat. High temperatures can change the ratio of gliadin to glutenin, weakening the dough-making properties of wheat flour. Moreover, extended heat waves and high temperatures can reduce the nitrogen level and, ultimately, the protein content of the grain (Wang and Liu, 2021b). In conclusion, climate change is going to impact wheat; therefore, the development of climate-tolerant wheat, which is difficult through conventional breeding techniques due to its hexaploidy genome, is an utmost priority. CRISPR/Cas9 technology was successfully used to obtain stable inherited mutations in wheat. Agrobacterium-mediated transformation has been used to deliver CRISPR constructs into immature wheat embryos (Howells et al., 2018; Zhang Z. et al., 2019). CRISPR/Cas9 genome editing-derived transgene-free wheat plants have also been produced using biolistic and protoplast transfection methods (Liang et al., 2017; Zhang et al., 2016). These studies have successfully implemented CRISPR/Cas9 genome editing technology in wheat, providing a platform for improving wheat concerning climate change-mediated problems. Grain weight, grain size, and grain yield per plant are the main agronomic attributes highly influenced by abiotic and biotic factors. Researchers have identified several genes that function as negative regulators of grain’s weight, size, and overall yield. For example, Receptor-like protein kinase 1 (RPK1), the Brittle rachis gene (BTR1-A), GASR7, TaSPL13, GW2, and TaARE1 (Table 1). A study reported the knockout of GASR7 and GW2 genes via the ribonucleoprotein (RNP)-derived CRISPR/Cas9 approach in bread wheat and pasta wheat resulted in transgene-free mutants with higher grain weight (Zhang Y. et al., 2021). Similarly, the TaSPL13 gene, responsible for controlling grain size and number, was edited using CRISPR/Cas9 (Gupta et al., 2023). The results revealed that mutations in this gene lead to an increase in the size and number of grains in allohexaploid wheat, demonstrating the importance of TaSPL13 in the evolution of yield-related attributes in wheat. Similarly, Ta-eIF4E alone has been targeted in wheat, resulting in viral resistance and improved plant height and grain length (Kan et al., 2023). Another important agronomic factor in wheat is early heading. Recently, the role of the thioredoxin gene (TaTRXH9) is characterized and validated in wheat using CRISPR/Cas9-mediated gene editing, and a loss-of-function mutation in this gene found in early-heading wheat (Fan et al., 2023). These studies reveal the success of CRISPR/Cas9 genome editing in improving wheat yield, which can significantly mitigate the impact of climate change on yield reduction.
The unstable weather can increase gluten levels in wheat, which makes it less suitable for consumption (Mkhabela et al., 2022). However, Chinese researchers have developed a Gluten gene Enrichment and Sequencing (GlutEnSeq) system, screened thousands of prolamin genes from different wheat varieties, and low gluten wheat was produced by modifying γ- and a-gliadin genes via CRISPR/Cas9 (Jouanin et al., 2019). A study developed improved winter and spring wheat varieties with high amylose content via CRISPR/Cas9-mediated genome editing of starch-branching enzyme (SBE) II (TaSBEIIa), and multiple transgene-free mutant lines were obtained (Li J. et al., 2021). Correspondingly, the CRISPR/Cas9 approach has been utilized to modify four genes, i.e., puroindoline b (PINb), granule-bound starch synthase gene (GBSS or WAXY), polyphenol oxidase gene (PPO), and phytoene synthase gene (PSY), in wheat via the Agrobacterium-mediated transformation method of gene delivery (Zhang Y. et al., 2021; Zhang Z. et al., 2019). The PINb gene controls grain hardness, the WAXY gene is responsible for amylose synthesis, PSY is the main gene controlling the carotenoid biosynthetic pathway, and PPO controls color via the oxidation of phenolic compounds.
Micronutrient deficiency in wheat is likely to occur because of increasing temperatures and drought, which can lead to malnutrition and other health disorders. The CRISPR/Cas9 genome editing approach is a straightforward method that can be used to enhance the nutritional composition of wheat and address this problem; for instance, Ibrahim et al. edited the Inositol Pentakisphosphate 2-Kinase 1 (TaIPK1) gene, which expresses an enzyme involved in the final step of the phytate biosynthesis pathway (Ibrahim et al., 2022). Phytic acid is considered an anti-nutrient because it reduces the bioavailability of iron and zinc in humans. The phytic in wheat tissues binds to these micronutrients as phytate and decreases their bioavailability. CRISPR/Cas9 genome engineering of TaIPK1 in wheat improved iron (1.5–2.1-fold) and zinc content (1.6–1.9-fold) and lowered phytic acid accumulation in wheat grains. Several agronomic improvements via CRISPR/Cas9-mediated genome editing in wheat are presented in Table 1.
Transcriptome profiling of wheat grains revealed several heat stress-associated gene, including heat shock transcription factor gene (TaHSFA6e), ascorbate peroxidase, β-amylase, γ-gliadin-2, and LMW-glutenin, were upregulated during the high-temperature stress (Rangan et al., 2020; Wen et al., 2023). Such studies can help select the potential target genes for CRISPR/Cas-mediated genome editing, which might lead abiotic stress resistant crops. Moreover, studies on different crops can be used to develop wheat that is tolerant to increasing temperatures caused by climate change. For example, CRISPR/Cas9-mediated knockout of the mitogen-activated protein kinase gene (SlMAPK 3) enhances heat tolerance in tomato plants, and the edited pyrabactin resistance 1 (PYR1)/PYR1-like (PYL) (pyl1/4/6) makes rice tolerant to hot weather (Miao et al., 2018; Yu et al., 2019). Since wheat is hexaploid and has a complex genome, there are fewer reports available on producing abiotic stress-tolerant wheat plants than rice. TaSAL1, TaMBF1c, and TaHAG1 genes have been edited to produce abiotic stress-tolerant wheat plants (Mohr et al., 2022; Tian et al., 2022; Zheng M. et al., 2021). The histone acetyltransferase (TaHAG1) gene contributes to salt tolerance by affecting free radical production in hexaploid wheat. MBF1c confers thermotolerance by regulating the translation of specific mRNA translation, whereas SAL1 negatively regulates drought tolerance (Table 1). These studies suggest that genome editing of wheat could be a promising approach for conferring climate change.
Rice (Oryza sativa L.) is a staple crop, with a global consumption rate of approximately 21% and 76% in Asian countries. Worldwide, 776.5 million tons of rice were produced in 2022, with approximately 90.5% of the average rice production taking place in Asia, in which China and India are the biggest producers (). This high demand for rice is due to its taste and versatility in a variety of international cuisines (Castanho et al., 2023). In addition, rice is enriched in nutrients, mainly complex carbohydrates, and moderate levels of vitamin B, phosphorous, iron, calcium, and protein. Most nutrients (minerals, vitamins, proteins, and antioxidants) are present in the rice brain, which has a brown outer layer. Rice contains all essential amino acids except lysine and is a great source of a balanced diet, as it does not contain cholesterol, fat, or sodium (Sasaki and Burr, 2000). However, the current climate change scenarios have adversely affected rice crops from farms to consumers in various ways (Zhao et al., 2016). Increasing temperature, carbon dioxide, drought, salinity, rainfall, pests, and diseases are the main stressors that can directly affect various rice attributes, such as grain size, quality, yield, nutritional constitution, and appearance. Rice cultivation can be successful if the optimum temperature and rainfall are provided; however, an increase of 1 °C can adversely affect rice yield. A recent study showed a decline in the nutritional content of rice due to rising atmospheric CO2 concentrations. Micronutrients, proteins, and several vitamins are diminished in rice, leading to malnutrition in infants and children of rice-dependent countries. A decline of approximately 17%–30% in vitamins, 5% in zinc, 8% in iron, and 10% in protein was observed in rice grown under high CO2 conditions (Smith and Myers, 2018). In contrast, Guo et al. found a 15% increase in the mineral content of rice grown under high CO2 and temperature conditions (Guo et al., 2022). Though high CO2 is normally considered a growth-stimulating agent along with high temperature, it acts antagonistically. Jing et al. reported reduced rice yield due to elevated temperatures under high CO2 conditions created using temperature-free air CO2 enrichment (T-FACE) systems (Jing L. et al., 2024). Another T-FACE experiment in China reported that increasing CO2 substandardized the sensory quality of rice by increasing chalkiness (Wang et al., 2024d). The chalkiness of rice is a major obstacle to rice marketing. Chalky rice forms owing to abnormal starch development inside the grain and appears as a scattered coarse material, thus making the surface turbid. This abnormal starch accumulation occurs due to the high temperature, mainly at the time of grain filling, which reduces the ability of α-amylase enzyme to degrade the starch and, hence, leads to malformed starch synthesis (Shimoyanagi et al., 2021). In addition to grain quality, other issues in rice that can occur due to climate change include decreased shoot biomass and carbohydrate content in the stem, decreased starch content, and reduced photosynthetic ability, transpiration, and leaf area (Cui et al., 2024; Huanhe et al., 2024; Yamori et al., 2025). Hence, concerning the future outcomes, researchers have been trying different strategies, one of which is the robust and versatile genetic editing technique called as CRISPR/Cas9 system for generating climate-smart rice crops. CRISPR/Cas9-mediated gene editing has been optimized in rice using Agrobacterium-mediated transformation, particle bombardment, and RNPs (Miao et al., 2013; Shan et al., 2013). Moreover, a multiplexing approach was successfully established for rice. Recently, a group of researchers accomplished ultra-multiplexing targeting 49 genes in rice using both Agrobacterium-mediated and biolistic approaches (Wu et al., 2024).
CRISPR/Cas9 mediated-knockout of two main quantitative trait loci associated with grain length and thousand-grain weight, i.e., GS3 and GL3.1 was reported in rice GS3 encodes a protein that restrains the cell division of the spikelet hull, leading to shorter grains, whereas GL3.1 encodes an enzyme that controls grain size by dephosphorylating the cell cycle-related protein Cyclin-T1,3 and inhibits cell proliferation in the hull. Mutations in this gene increase grain size in rice (Yuyu et al., 2020; Zhang Y. M. et al., 2021). Huang et al. used the CRISPR/Cas9 technique to create an Indica maintainer line, Mei1B, containing an edited GS3 allele to improve grain yield and quality (Huang et al., 2022). Another study mutated the OsSPL16 or GW8 gene via CRISPR/Cas9 technology to improve the cylindrical shape and grain yield of Basmati rice (Usman et al., 2021). Rice aroma is an important quality parameter that is affected by climate change. Nevertheless, improving and introducing aromas into rice is feasible to improve and introduce aromas into rice using genetic engineering techniques. Mutations in Betaine Aldehyde Dehydrogenase 2 (OsBADH2) via CRISPR/Cas9 added aroma to elite non-aromatic rice variety (Hui et al., 2022; Tang et al., 2021). Several reports are available on the use of CRISPR-mediated gene editing for improving various agronomic traits in rice (Table 2). Recently, loss-of-function mutants of OsCKX were found to affect various attributes including plant height, grain size, grain number, panicle size, seed shape, and starch accumulation. This gene encodes a cytokinin-degrading enzyme that inactivates cytokinins, which plays important roles in plant growth and cell proliferation (Zheng et al., 2023). Similarly, enhanced grain yield was observed by deleting a target site of the transcription factor An-1 in the cis-regulatory region of the Ideal Plant Architecture 1 (IPA1) gene (Song et al., 2022).
Rice quality improvement is crucial because rice passes through various downstream processes, such as dehydration, milling, removal of bran, cleaning, and cooking after harvesting, and each process directly or indirectly decreases the nutrient content of rice. For instance, cleaning alone can reduce vitamin levels by 25%–60%, potassium by 20%–40%, and proteins by 3%–7% in rice (Müller et al., 2022). Combining this with the effect of climate change, the rice produced would not be useful. Therefore, biofortification is the only method that can sustain rice nutrients. Carotenoid-enriched marker-free rice has been developed by inserting two carotenoid biosynthetic genes, SSU-CRTI and ZmPSY30, using the CRISPR/Cas9 approach (Dong et al., 2020). Achary and Reddy produced a rice variety with an enhanced accumulation of iron, zinc, potassium, calcium, and phosphorous in endosperm via CRISPR/Cas9-mediated editing of GW2 (Grain width and grain weight) locus (Achary and Reddy, 2021). Moreover, the aleurone layer gained thickness with an enhanced protein content. Another study found decreased grain chalkiness, high ammonium cation, and phosphate ion uptake, and high photosynthetic activity under high CO2 conditions in miR166-RDD1 knocked-out rice plants (Iwamoto, 2022).
Among abiotic stressors, salinity is a serious event that can potentially tarnish rice production. The development of salt-tolerant varieties is a lifesaving approach feasible with CRISPR/Cas9 genome editing technology. High salt concentrations can negatively affect crop production by disrupting the metabolic and physiological processes. These factors can significantly affect plant development, seed germination, and productivity (Zörb et al., 2019). Plants respond to salt stress by increasing the biosynthesis of antioxidants, osmoregulators, and phytohormones that support the plant by maintaining ion homeostasis; however, this ability works to a certain extent, and not all plants can protect themselves from high salinity (Raza et al., 2022). Similarly, drought stress drastically affects the physiology of plants by inhibiting nutrient uptake and other life-dependent activities, including photosynthesis, cell division and elongation, turgor pressure, and gene expression. Several rice genes have been identified and mutated using CRISPR/Cas9 to produce abiotic stress-tolerant plants (Table 2). For example, salt-tolerant T2 homozygous mutant rice was developed by cleaving the OsRR22 gene in rice using Cas9 (Zhang A. et al., 2019). Knockout of OsRR22 improved the performance of rice plants under high-salt conditions (0.75%), with no side effects on grain size, yield, or plant biomass. The same strategy was used by Han et al. to develop novel rice germplasm at the seedling stage (Han et al., 2022). In another study, the drought and salt tolerance (OsDST) gene was edited using CRISPR/Cas9 to produce the indica mega rice cultivar MTU1010 with enhanced tolerance (Santosh et al., 2020). In rice, the transcription factor OsMADS26 plays a negative role, and mutations in this gene enhance drought tolerance (Anjala and Augustine, 2022). Ogata et al. characterized the Enhanced Response to ABA1 (ERA1) gene in rice using CRISPR/Cas9-mediated editing and found frameshift mutations in mutants with increased primary root growth, high sensitivity to abscisic acid stress, and increased drought stress tolerance (Ogata et al., 2020).
The effect of climate change on disease susceptibility in rice poses a major threat to rice productivity. Climate change-induced pest and microbial emergence can annihilate agriculture, thereby substantially threatening food security. Fortunately, this can be mitigated using gene-editing applications in crops. Various studies have been published on the production of pests and microorganisms that cause disease tolerance in crops (Liu M. et al., 2024; Oliva et al., 2019; Zhou et al., 2022). Table 2 shows some examples of CRISPR/Cas9-mediated development of disease-resistant plants. However, the current work is insufficient in comparison to the upcoming consequences of climate change because this change can also increase the potential of insects by providing them with favorable conditions for their growth and development. For example, fruit flies flourished more when the temperature increased from 20°C to 35 °C in certain mango varieties. This phenomenon is observed in all species worldwide. In particular, insects in temperate regions have become more active, whereas populations of tropical insects may decline or migrate (Bhattacharjee et al., 2022). Therefore, genetically edited plants may be a powerful solution for overcoming the effects of climate change on agriculture. Several studies have been conducted to develop rice resistance to various fungal and bacterial pathogens. Xa13 is involved in pollen development and exhibits recessive resistance to bacterial blight. Studies have shown that the complete loss of function of the coding region of this gene can lead to sterility; therefore, CRISPR-assisted modification was performed in the Xa13 promoter region to produce transgene-free bacterial blight-resistant rice with retained fertility (Li C. et al., 2020). Another study targeted three salicylic acid 5-hydroxylase (OsS5H) genes (BSR-D1, PI21, and ERF922) in rice and found resistance to both rice blast and bacterial blight (Zhou et al., 2022). Recently, rice with enhanced immunity was produced by editing the NAC transcription factor gene in rice via CRISPR/Cas9 approach (Son et al., 2024). Furthermore, broad-spectrum disease resistance was achieved by editing three salicylic acid hydroxylase (OsS5H) genes in rice (Li X. et al., 2023).
Maize (Zea mays) is the most important cereal crop in the world, with the highest production after rice and wheat, and fulfills the needs of human food, animal feed, and biofuels (Chávez-Arias et al., 2021). Maize is sensitive to heat stress during seed germination and vegetative growth. It significantly affects maize plant germination and seedling emergence. Heat stress causes the formation of abscisic acid and affects the activity of enzymes responsible for breaking down starch (Chandra et al., 2023). Additionally, it inhibits the synthesis of proteins in the embryo, which reduces the germination of maize seeds at over 37°C, resulting in a decrease in plant density (Buriro et al., 2011). Increased oxidative stress, altered membrane permeability, decreased stomatal conductance, and other signs occur regularly in plants under heat stress. The rate of photosynthesis was negatively affected by a reduction in stomatal conductance. Moreover, it induces the production of reactive oxygen species and causes oxidative stress (Soengas et al., 2018; Zandalinas et al., 2017). Heat stress negatively affects the number of florets, silk number, fertilization, filling, development, and final grain yield during flowering (Lizaso et al., 2018). Recently, the continuous span of heat waves has affected maize yield and productivity. They alter the morphology, physiology, genomic expression, and biochemical metabolism of crops. In response to these alterations, plants activate tolerance mechanisms via heat shock transcription factors and proteins essential for reducing and preventing heat-related damage (Li and Zhang, 2022d). Heat stress affects the integrity of the plasma membrane and the accumulation of reactive oxygen species (Dogra and Kim, 2020). Moreover, proteins are misfolded or unfolded, which disrupts cell metabolism and physiology and eventually leads to cell death. The development of climate resilience in maize is urgently needed because heat-induced decline is high in this crop (Chandra et al., 2023).
Currently, a decrease in yield resulting from drought stress is estimated to affect over 20% of the annual maize area, and at high temperatures, an average of 7.4% is lost for every 1°C increase (Boyer et al., 2013; Malenica et al., 2021). Notably, Brazil, the third largest producer of maize worldwide, showed a decrease in yield in the 2015–16 and 2020–21 growing seasons of approximately 18 and 23 Mt, correspondingly to losses of approximately 21% compared to the 2014–15 and 2019–20 seasons (Lopes Filho et al., 2023). These crop failures occurred in years marked by extreme drought conditions, resulting in diminished yields in many of the largest producer geographies. Similarly, crop yield reductions and spiking prices were affected by the 2012 drought in the US (the world’s largest producer) (Boyer et al., 2013). Therefore, it is essential to reduce the potential losses caused by the increased frequency, severity, and duration of stresses associated with global climate change by continuously developing new maize cultivars that target better genetic adaptation and using improved agricultural practices.
Abiotic stress tolerance and yield are two complex traits strongly influenced by environmental factors and linked to small-effect genetic loci. Using genomic engineering techniques to develop superior cultivars for these traits is more difficult because of such complexity, which makes it challenging to reliably evaluate the molecular mechanisms behind gene activities and measure phenotypes. Considering the few instances developed for complex traits, transgenic maize cultivars with enhanced herbicide and insect resistance have been on the market for decades (Yassitepe et al., 2021). The difficulty in applying a transgenic approach to control complex traits that are stable in multiple environments has limited the development of biotech cultivars that could be widely used (Simmons et al., 2021).
Gene editing technology has enabled an efficient and consistent way to understand the role of key genes and develop new germplasm in maize (Doll et al., 2019; Wang Y. et al., 2022).
Potential putative functions of numerous genes involved in maize development programs and stress responses have been investigated thoroughly using CRISPR/Cas9 technology (Wang Y. et al., 2022). CRISPR has been widely used to enhance numerous agronomic traits in maize, yield, nutrition, improved pollen characteristics, drought tolerance, and disease resistance (Jiang L. et al., 2024; Kaul et al., 2024; Lv et al., 2024; Wang G. et al., 2024; Xie Z. et al., 2024). ZmGDIɑ was specifically edited using CRISPR/Cas9 to significantly increase maize resistance to the maize rough dwarf virus without negative agronomic effects (Liu Y. et al., 2023). ZmCOIɑ interacts inversely with ZmJAZ15 to alter maize immunity against Gibberella stalk rot (GSR, a teleomorph of Gibberella zeae), and further downregulation of ZmCOIɑ can increase maize resistance to GSR (Ma et al., 2021). MMS21 maintains the activity and integrity of the maize genome, resulting in improved root and vegetative growth, pollen germination, and seed development (Zhang et al., 2021b). ZmCLCg positively regulates sodium chloride stress and chloride transport in maize as a stress response (Luo et al., 2021). ZmSRL5 is essential for sustaining cuticular wax structure and drought tolerance in maize (Pan Y. et al., 2020).
Maize yield is severely affected by several environmental factors, including drought, high temperatures, floods, and unsuitable soil conditions. The breeding of stress-tolerant variants has shown great potential when applying genome editing tools compared with conventional breeding methods (Chávez-Arias et al., 2021; Chennakesavulu et al., 2021; Prasanna et al., 2021). The development of maize lines with high stalk strength has become considerably important to breeders for maintaining high and constant production, as stalk lodging caused by different environmental factors poses a significant threat to maize quality and production. STIFF1 is a negative regulator of maize stalk length, its altered allele with a 2bp deletion caused a frameshift and an early slowdown translation, conferring CRISPR-edited plants with a stronger stalk, which contributed to high-density planting and avoided stalk lodging (Armarego-Marriott, 2020). Furthermore, ZmGA20OX3 has been modified to develop semi-dwarf maize plants using CRISPR/Cas9 technology, which may be useful for developing a novel genotype that is more resilient to lodging and suitable for high-density planting (Liu Y. et al., 2023). For drought tolerance, precisely editing the promoter sequence of ARGOS8 leads to an increase in its expression and enhances maize grain yield under drought stress (Shi et al., 2017). By inserting the GOS2 promoter from maize plants at the 5ʹ untranslated regions that remain of the ARGOS8 gene’s native promoter, which acts as a negative regulator of ethylene responses (Zafar et al., 2020). Targeted alteration of the native maize promoter using CRISPR/Cas9 enhanced ARGOS8 expression and improved grain production under drought conditions. In addition, CRISPR/Cas9 has been used to target Slagamous-Like 6 (SIAGL6) to achieve heat tolerance (Doll et al., 2019). Therefore, developing new germplasm sources for breeding stress-tolerant maize has been achieved using CRISPR/Cas9 technology. Table 3 illustrates several improvements in the agronomic traits of maize using CRISPR/Cas9 gene editing.
A bibliometric analysis was conducted to examine global trends in CRISPR/Cas9 research, focusing on cereal crops and their applications for stress tolerance and yield improvement. The search used terms like “CRISPR,” “Genome editing,” “Gene editing,” and “Gene silencing,” along with crop-related terms such as “Cereal crops” and stress-related topics like “Abiotic stress,” “Biotic stress,” and “Drought tolerance.” The dataset, initially containing 1,232 articles from 2019 to 2025, was narrowed to 597 after excluding reviews, book chapters, and non-English publications. The citation analysis and other visualizations were conducted using Web of Science (WoS) and VOSviewer.
According to the database, increasing trend in publications and citations from 2019 to 2025 can be seen, demonstrating a significant increase in scholarly interest in CRISPR/Cas9 for enhancing crop stress tolerance and yield (Figure 4). After 2021, both publications and citations remained steady, suggesting the field’s maturation or a shift in research focus, with sustained interest continuing in the subsequent years (Francis et al., 2024). The tree map in Figure 5 organizes research across different subject areas, with Plant Sciences comprising the largest share, followed by biochemistry, molecular biology and agronomy. This distribution emphasizes the central focus on plant traits, particularly in cereals, while also highlighting the broader applications of CRISPR/Cas9 in fields like biochemistry and biotechnology. Smaller categories, such as Horticulture and Environmental Sciences, reflect the interdisciplinary nature of genome editing research (AlRyalat et al., 2019).
Figure 4. Annual publication trends from 2019 to 2025, showing the number of papers published each: The graph illustrates a surge in publications and citations from 2019 to 2025 in the area of CRISPR/Cas9 for enhancing crop stress tolerance and yield Created with BioRender.
Figure 5. The tree map illustrates research across different subject areas: This map focuses on research across different subject areas, with Plant Sciences comprising the largest share, followed by biochemistry, molecular biology and agronomy Created with BioRender.
The network visualization in Figure 6 further shows the relationships between key research themes. The central nodes CRISPR and genome editing are closely connected to other significant areas such as drought tolerance, yield improvement, and stress resistance, with a primary focus on rice, a key cereal crop. This network highlights the broad applications of CRISPR/Cas9 in enhancing abiotic stress tolerance and improving crop yield. The strong links between these themes suggest that improving crop resilience is a major goal in CRISPR/Cas9 research (Altaf et al., 2024).
Figure 6. Network visualization of keyword co-occurrence related to CRISPR/Cas9 research in cereals: The central nodes in the figure illustrates the relationship between CRISPR and genome editing specially in the research areas such as drought tolerance, yield improvement, and stress resistance, with a primary focus on rice. Visualization uses different colors to distinguish between research areas, with red highlighting drought tolerance, green representing stress resistance, and blue focusing on gene editing Created with BioRender.
The overlay visualization, tracks how research trends have changed over time, especially focusing on drought tolerance, salinity tolerance, and abiotic stress (Figure 7). From 2021 onward, the research has increasingly focused on these topics, particularly in cereal crops like rice, wheat, and maize. This shift reflects the growing need for developing climate-resilient crops, with more research being dedicated to improving stress resistance and water use efficiency in cereals. The overlay emphasizes the growing interest in improving cereals to make them more resilient and higher yielding under extreme environmental conditions. While Figure 6 shows the connections between topics, Figure 7 illustrates the evolution of these topics over time, especially highlighting cereals as a key focus in the search for more resilient crops to face climate change (Altaf et al., 2024).
Figure 7. The overlay visualization of research trends on CRISPR/Cas9 over time: The figure shows the research trends over time, especially focusing on drought tolerance, salinity tolerance, and abiotic stress. Since 2021, the research has increasingly focused on these topics, particularly in cereal crops like rice, wheat, and maize Created with BioRender.
Furthermore, the country distribution map, highlights the geographic spread of research on CRISPR/Cas9 applications in cereal crops (Figure 8). The heatmap reveals that countries such as China, United States, and India are at the forefront of this research, with other countries like South Korea, Brazil, and France contributing significantly (Martins et al., 2022). However, there is a noticeable gap in regions that rely heavily on cereal crops, such as the Middle East. This suggests an opportunity for further research in these areas, particularly to address local agricultural challenges related to climate stress and water scarcity.
Figure 8. Country-wise distribution of publications on CRISPR/Cas9 research in cereals from 2019 to 2025: The heatmap reveals that countries such as China, United States, and India are at the forefront of this research, with other countries like South Korea, Brazil, and France contributing significantly Created with BioRender.
Gene editing has been successfully used to generate climate-resilient crops for various climatic conditions. However, several limitations are descending its overall potential remains limited (Singh et al., 2024a; Singh et al., 2024b). The major drawback of gene-editing technologies is off-target, which can cause unwanted editing of other genes, which hinders their wide applicability for crop trait improvement.
Another disadvantage of, the lack of efficient tissue culture methods for regeneration, transformation and generation of gene edited crops. The use of a de novo meristem induction technique can be more useful and easier for recalcitrant crop species (Maher et al., 2020). Research is being conducted to increase the transformation ability of recalcitrant varieties using advanced tools to produce climate-resilient crops.
Apart from these technical drawbacks, policymakers and regulatory authorities must take the initiative to overcome the lack of clarity regarding genome-edited crops among the population. Altogether, these factors can provide ultimate success in applying these technologies to address the impact of climate change.
Intragenic, transgenic, and cisgenic (ICT) approaches have been used to improve plant characteristics using foreign genes which leverage its applications (Karavolias et al., 2021; Klümper and Qaim, 2014; Steinwand and Ronald, 2020).
Therefore, merging genome editing with ICT approaches would be the best solution for raising climate-smart crops and this can be possible using targeted gene integration using CRISPR/Cas9 technology. This strategy has been applied to maize varieties by successfully inserting a novel promoter upstream of a gene responsible for ethylene regulation to improve drought tolerance. Moreover, the entire gene can be replaced using these approaches; for example, the replacement of the japonica NRT1.1B allele with the indica allele improves nitrogen use efficiency in rice (Li et al., 2018). Although these approaches have great potential, regulations regarding these technologies have decreased their feasibility. Therefore, new advancements and technical improvements are required to overcome these limitations.
For example, base editors and prime editors are second-generation CRISPR-based genome modification tools that mediate precise editing without relying on double-stranded break formation and homology direct repair. These editors are more precise in terms of single-nucleotide modification and integration (Lin et al., 2020). Base editors involve the direct conversion of a single-nucleotide base into another (A-to-G or C-to-T, and A-to-C or C-to-G), without forming double-strand breaks, introducing specific point mutations with utmost precision. Base editing has been applied to several crops including Arabidopsis, cotton, rice, tomato, maize, tobacco, and soyabean (Li X. et al., 2024; Luo et al., 2023; Wang G. et al., 2024; Wang et al., 2024f; Wei et al., 2023; Zhong D. et al., 2024). On the other hand, prime editors integrate Cas9 nickase and a reverse transcriptase, prime editing guide RNA (pegRNA) which is a combination of Cas9 sgRNA, a reverse transcriptase template, and a primer-binding site (PBS). The pegRNA guides the nCas9 to the target site, where it makes a nick in the non-target DNA strand. Then the reverse transcriptase extends the nicked strand by utilizing the reverse transcriptase template (RTT) from the pegRNA, thereby incorporating the intended modifications. With prime editors, large deletion, replacement, and inversion of larger DNA fragments can be performed in plants with high precision. Researchers have achieved DNA inversions of up to 205.4 kb in wheat plants with 51.5% by using dual prime editors. They have also been applied to edit large DNA fragments in tobacco and tomato (Zhao et al., 2025). Similarly, a recent study utilized high-efficiency prime-editing tools to knockin a 10-bp heat-shock element (HSE) into promoters of cell-wall-invertase genes (CWINs) in rice and tomato cultivars (Lou et al., 2025). These modified CRISPR tools can leverage the gene editing efficiency of manipulating chromosomes and larger DNA segments for crop improvement.
Gene editing could be a powerful solution for the present and future anticipation of climate change consequences. With the emergence of advanced genome editing techniques, including CRISPR/Cas9, base editing, and prime editing, various agronomic traits such as disease resistance, abiotic stress tolerance, and nutritional enhancement have emerged. Despite this, most gene editing technologies are still under laboratory research and have not yet been translated into the real world. This is due to technical limitations and restrictions imposed by regulatory authorities and policymakers. However, technological innovations are rapidly expanding owing to the ongoing efforts of public and private institutions. The potential of gene editing in offering solutions for climate change in agriculture is not overlooked, even though it is not the only solution to improve agriculture. Numerous studies show that gene editing can be used to enhance agriculture and combat climate change effects greatly. Nevertheless, as indicated by bibliometric analysis, significant research gaps remain, particularly in applying CRISPR/Cas9 to underexplored crops like rice, wheat and maize for comprehensive climate resilience.
NK: Formal Analysis, Investigation, Methodology, Software, Writing–original draft. MQ: Data curation, Investigation, Methodology, Writing–original draft. DF: Writing–original draft, Formal Analysis, Software. AA: Software, Validation, Writing–review and editing. ST: Conceptualization, Supervision, Validation, Writing–review and editing. ZA: Conceptualization, Data curation, Formal Analysis, Funding acquisition, Investigation, Methodology, Project administration, Software, Supervision, Writing–review and editing.
The author(s) declare that financial support was received for the research, authorship, and/or publication of this article. The authors are grateful to United Arab Emirates University (UAEU) for providing funding through the UAEU Program for Advanced Research (UPAR) program, grant number 12F059.
The authors are thankful to the UAEU University facilities and library for supporting the research and collection of the most recent literature.
The authors declare that the research was conducted in the absence of any commercial or financial relationships that could be construed as a potential conflict of interest.
The author(s) declared that they were an editorial board member of Frontiers, at the time of submission. This had no impact on the peer review process and the final decision.
The author(s) declare that no Generative AI was used in the creation of this manuscript.
All claims expressed in this article are solely those of the authors and do not necessarily represent those of their affiliated organizations, or those of the publisher, the editors and the reviewers. Any product that may be evaluated in this article, or claim that may be made by its manufacturer, is not guaranteed or endorsed by the publisher.
The Supplementary Material for this article can be found online at: https://www.frontiersin.org/articles/10.3389/fgeed.2025.1533197/full#supplementary-material
Achary, V. M. M., and Reddy, M. K. (2021). CRISPR-Cas9 mediated mutation in GRAIN WIDTH and WEIGHT2 (GW2) locus improves aleurone layer and grain nutritional quality in rice. Sci. Rep. 11, 21941. doi:10.1038/s41598-021-00828-z
Ahmar, S., and Gruszka, D. (2023). CRISPR/Cas9 boosts wheat yield by reducing brassinosteroid signaling. Trends. biochem. Sci. 48, 917–919. doi:10.1016/j.tibs.2023.07.005
Alam, M. S., Kong, J., Tao, R., Ahmed, T., Alamin, M., Alotaibi, S. S., et al. (2022). CRISPR/Cas9 mediated knockout of the OsbHLH024 transcription factor improves salt stress resistance in rice (Oryza sativa L.). Plants 11, 1184. doi:10.3390/plants11091184
Alfatih, A., Wu, J., Jan, S. U., Zhang, Z. S., Xia, J. Q., and Xiang, C. B. (2020). Loss of rice PARAQUAT TOLERANCE 3 confers enhanced resistance to abiotic stresses and increases grain yield in field. Plant Cell Environ. 43, 2743–2754. doi:10.1111/pce.13856
AlRyalat, S. A. S., Malkawi, L. W., and Momani, S. M. (2019). Comparing bibliometric analysis using PubMed, scopus, and Web of science databases. JoVE 152, e58494. doi:10.3791/58494
Altaf, M. T., Liaqat, W., Jamil, A., Jan, M. F., Baloch, F. S., and Mohamed, H. I. (2024). A bibliometric analysis of genome-wide association study (GWAS) and Sorghum (Sorghum bicolor L) based on Web of Science using VOS viewer. J. Soil Sci. Plant Nutr. 24, 5012–5028. doi:10.1007/s42729-024-01888-6
An, X., Zhang, S., Jiang, Y., Liu, X., Fang, C., Wang, J., et al. (2024). CRISPR/Cas9-based genome editing of 14 lipid metabolic genes reveals a sporopollenin metabolon ZmPKSB-ZmTKPR1-1/-2 required for pollen exine formation in maize. Plant Biotechnol. J. 22, 216–232. doi:10.1111/pbi.14181
An, Y., Chen, L., Li, Y. X., Li, C., Shi, Y., Zhang, D., et al. (2022). Fine mapping qKRN5. 04 provides a functional gene negatively regulating maize kernel row number. Theor. Appl. Genet. 135, 1997–2007. doi:10.1007/s00122-022-04089-w
Anjala, K., and Augustine, R. (2022). Designing of guide RNA constructs for CRISPR/Cas9-mediated editing of rice transcription factor osmads26 for enhancing drought tolerance. J. Appl. Biol. Biotechnol. 11, 176. doi:10.7324/jabb.2023.110124
Anzalone, A. V., Koblan, L. W., and Liu, D. R. (2020). Genome editing with CRISPR-Cas nucleases, base editors, transposases and prime editors. Nat. Biotechnol. 38, 824–844. doi:10.1038/s41587-020-0561-9
Armarego-Marriott, T. (2020). Stiffening stems: identification of the stiff1 gene involved in maize stalk strength. Plant Cell. ASPB. 32, 12. doi:10.1105/tpc.19.00852
Avtar, R., Aggarwal, R., Kharrazi, A., Kumar, P., and Kurniawan, T. A. (2020). Utilizing geospatial information to implement SDGs and monitor their Progress. Environ. Monit. Assess. 192, 35. doi:10.1007/s10661-019-7996-9
Bhattacharjee, P., Warang, O., Das, S., and Das, S. (2022). Impact of climate change on fruit crops- A review. CWE 17, 319–330. doi:10.12944/CWE.17.2.4
Biswal, A. K., Hernandez, L. R. B., Castillo, A. I. R., Debernardi, J. M., and Dhugga, K. S. (2023). An efficient transformation method for genome editing of elite bread wheat cultivars. Front. Plant Sci. 14, 1135047. doi:10.3389/fpls.2023.1135047
Biswas, S., Ibarra, O., Shaphek, M., Molina-Risco, M., Faion-Molina, M., Bellinatti-Della Gracia, M., et al. (2023). Increasing the level of resistant starch in ‘Presidio’ rice through multiplex CRISPR–Cas9 gene editing of starch branching enzyme genes. TPG 16, e20225. doi:10.1002/tpg2.20225
Bouras, E., Jarlan, L., Khabba, S., Er-Raki, S., Dezetter, A., Sghir, F., et al. (2019). Assessing the impact of global climate changes on irrigated wheat yields and water requirements in a semi-arid environment of Morocco. Sci. Rep. 9, 19142. doi:10.1038/s41598-019-55251-2
Boyer, J. S., Byrne, P., Cassman, K. G., Cooper, M., Delmer, D., Greene, T., et al. (2013). The US drought of 2012 in perspective: a call to action. Glob. Food. Secur. 2, 139–143. doi:10.1016/j.gfs.2013.08.002
Buriro, M., Oad, F. C., Keerio, M. I., Tunio, S., Gandahi, A. W., Hassan, S. W. U., et al. (2011). Wheat seed germination under the influence of temperature regimes. Sarhad J. Agric. 27, 539–543.
Caddell, D., Langenfeld, N. J., Eckels, M. J. H., Zhen, S., Klaras, R., Mishra, L., et al. (2023). Photosynthesis in rice is increased by CRISPR/Cas9-mediated transformation of two truncated light-harvesting antenna. Front. Plant Sci. 14, 1050483. doi:10.3389/fpls.2023.1050483
Cai, Y., Chen, L., Liu, X., Guo, C., Sun, S., Wu, C., et al. (2018). CRISPR/Cas9-mediated targeted mutagenesis of GmFT2a delays flowering time in Soya Bean. Plant Biotechnol. J. 16, 176–185. doi:10.1111/pbi.12758
Carroll, D. (2014). Genome engineering with targetable nucleases. Annu. Rev. Biochem. 83, 409–439. doi:10.1146/annurev-biochem-060713-035418
Castanho, A., Guerra, M., Brites, C., Oliveira, J. C., and Cunha, L. M. (2023). Design thinking for food: remote association as a creative tool in the context of the ideation of new rice-based meals. Int. J. Gastron. Food Sci. 31, 100664. doi:10.1016/j.ijgfs.2023.100664
Chandra, A. K., Joshi, A., Tripathi, A., Kumar, A., Pandey, S., Singh, A., et al. (2023). Climate-resilience maize: heat stress, signaling, and molecular interventions. J. Plant Growth Regul. 42, 6349–6366. doi:10.1007/s00344-022-10844-6
Chang, Y., Tang, H., Wang, S., Li, X., Huang, P., Zhang, J., et al. (2024). Efficient induction and rapid identification of haploid grains in tetraploid wheat by editing genes TtMTL and pyramiding anthocyanin markers. Front. Plant Sci. 15, 1346364. doi:10.3389/fpls.2024.1346364
Chávez-Arias, C. C., Ligarreto-Moreno, G. A., Ramírez-Godoy, A., and Restrepo-Díaz, H. (2021). Maize responses challenged by drought, elevated daytime temperature and arthropod herbivory stresses: a physiological, biochemical and molecular view. Front. Plant Sci. 12, 702841. doi:10.3389/fpls.2021.702841
Chen, H., Su, Z., Tian, B., Hao, G., Trick, H. N., and Bai, G. (2022a). TaHRC suppresses the calcium-mediated immune response and triggers wheat Fusarium head blight susceptibility. Plant Physiol. 190 (3), 1566–1569. doi:10.1093/plphys/kiac352
Chen, H., Ye, R., Liang, Y., Zhang, S., Liu, X., Sun, C., et al. (2023). Generation of low-cadmium rice germplasms via knockout of OsLCD using CRISPR/Cas9. J. Environ. Sci. 126, 138–152. doi:10.1016/j.jes.2022.05.047
Chen, W., Chen, L., Zhang, X., Yang, N., Guo, J., Wang, M., et al. (2022b). Convergent selection of a WD40 protein that enhances grain yield in maize and rice. Science 375 (6587), eabg7985. doi:10.1126/science.abg7985
Chen, X., Guo, Q., Yang, X., Yuan, M., Song, J., Fu, H., et al. (2024). Triple gene mutations boost amylose and resistant starch content in rice: insights from sbe2b/sbe1/OE-Wxa mutants. Front. Plant Sci. 15, 1452520. doi:10.3389/fpls.2024.1452520
Chen, Z., Du, H., Tao, Y., Xu, Y., Wang, F., Li, B., et al. (2022c). Efficient breeding of low glutelin content rice germplasm by simultaneous editing multiple glutelin genes via CRISPR/Cas9. Plant Sci. 324, 111449. doi:10.1016/j.plantsci.2022.111449
Chen, Z., Ke, W., He, F., Chai, L., Cheng, X., Xu, H., et al. (2022d). A single nucleotide deletion in the third exon of FT-D1 increases the spikelet number and delays heading date in wheat (Triticum aestivum L.). Plant Biotechnol. J. 20, 920–933. doi:10.1111/pbi.13773
Chen, Z., Li, W., Gaines, C., Buck, A., Galli, M., and Gallavotti, A. (2021). Structural variation at the maize WUSCHEL1 locus alters stem cell organization in inflorescences. Nat. Commun. 12, 2378. doi:10.1038/s41467-021-22699-8
Chennakesavulu, K., Singh, H., Trivedi, P. K., Jain, M., and Yadav, S. R. (2021). State-of-the-art in CRISPR technology and engineering drought, salinity, and thermo-tolerant crop plants. Plant Cell Rep. 41, 815–831. doi:10.1007/s00299-021-02681-w
Cui, R., Zhou, T., Shu, C., Zhu, K., Ye, M., Zhang, W., et al. (2024). Effects of salt stress on grain quality and starch properties of high-quality rice cultivars. Agron. (Basel). 14, 444. doi:10.3390/agronomy14030444
Ding, Y., Zhang, F., Sun, F., Liu, J., Zhu, Z., He, X., et al. (2023). Loss of OsHRC function confers blast resistance without yield penalty in rice. Plant Biotechnol. J. 21 (8), 1516–1518. doi:10.1111/pbi.14061
Dogra, V., and Kim, C. (2020). Singlet oxygen metabolism: from genesis to signaling. Front. Plant Sci. 10, 1640. doi:10.3389/fpls.2019.01640
Doll, N. M., Gilles, L. M., Gérentes, M. F., Richard, C., Just, J., Fierlej, Y., et al. (2019). Single and multiple gene knockouts by CRISPR–Cas9 in maize. Plant Cell. Rep. 38, 487–501. doi:10.1007/s00299-019-02378-1
Dong, O. X., Yu, S., Jain, R., Zhang, N., Duong, P. Q., Butler, C., et al. (2020). Marker-free carotenoid-enriched rice generated through targeted gene insertion using CRISPR-Cas9. Nat. Commun. 11, 1178. doi:10.1038/s41467-020-14981-y
Errum, A., Rehman, N., Uzair, M., Inam, S., Ali, G. M., and Khan, M. R. (2023). CRISPR/Cas9 editing of wheat Ppd-1 gene homoeologs alters spike architecture and grain morphometric traits. Funct. Integr. Genom. 23, 66. doi:10.1007/s10142-023-00989-2
Esquivel-Muelbert, A., Baker, T. R., Dexter, K. G., Lewis, S. L., Brienen, R. J. W., Feldpausch, T. R., et al. (2019). Compositional response of Amazon forests to climate change. Glob. Change Biol. 25, 39–56. doi:10.1111/gcb.14413
Fan, Y., Li, M., Wu, Y., Wang, X., Wang, P., Zhang, L., et al. (2023). Characterization of thioredoxin gene TaTrxh9 associated with heading-time regulation in wheat. Plant Physiol. biochem. 201, 107903. doi:10.1016/j.plaphy.2023.107903
Francis, D. V., Abdalla, A. K., Mahakham, W., Sarmah, A. K., and Ahmed, Z. F. R. (2024). Interaction of plants and metal nanoparticles: exploring its molecular mechanisms for sustainable agriculture and crop improvement. Environ. Int. 190, 108859. doi:10.1016/j.envint.2024.108859
Fu, K., Song, W., Chen, C., Mou, C., Huang, Y., Zhang, F., et al. (2022). Improving pre-harvest sprouting resistance in rice by editing OsABA8ox using CRISPR/Cas9. Plant Cell Rep. 41, 2107–2110. doi:10.1007/s00299-022-02917-3
Funk, C. C. (2021). Drought, flood, fire: how climate change contributes to catastrophes. Cambridge University Press. doi:10.1017/9781108885348
Gao, L., Yang, G., Li, Y., Sun, Y., Xu, R., Chen, Y., et al. (2021). A kelch-repeat superfamily gene, ZmNL4, controls leaf width in maize (Zea mays L.). Plant J. 107, 817–830. doi:10.1111/tpj.15348
Gao, X., Li, J., Yin, J., Zhao, Y., Wu, Z., Ma, L., et al. (2024). The protein phosphatase qGL3/OsPPKL1 self-regulates its degradation to orchestrate brassinosteroid signaling in rice. Plant Commun. 5 (6), 100849. doi:10.1016/j.xplc.2024.100849
Goldberg, G. W., Spencer, J. M., Giganti, D. O., Camellato, B. R., Agmon, N., Ichikawa, D. M., et al. (2021). Engineered dual selection for directed evolution of SpCas9 PAM specificity. Nat. Commun. 12 (1), 349. doi:10.1038/s41467-020-20650-x
Gong, W. J., and Golic, K. G. (2003). Ends-out, or replacement, gene targeting in Drosophila. P. N. A. S. 100, 2556–2561. doi:10.1073/pnas.0535280100
Guan, H., Chen, X., Wang, K., Liu, X., Zhang, D., Li, Y., et al. (2022). Genetic variation in ZmPAT7 contributes to tassel branch number in maize. Int. J. Mol. Sci. 23, 2586. doi:10.3390/ijms23052586
Guo, X., Huang, B., Zhang, H., Cai, C., Li, G., Li, H., et al. (2022). T-FACE studies reveal that increased temperature exerts an effect opposite to that of elevated CO2 on nutrient concentration and bioavailability in rice and wheat grains. F. E. S. 11, e336. doi:10.1002/fes3.336
Gupta, A., Hua, L., Zhang, Z., Yang, B., and Li, W. (2023). CRISPR-induced miRNA156-recognition element mutations in TaSPL13 improve multiple agronomic traits in wheat. Plant Biotechnol. J. 21, 536–548. doi:10.1111/pbi.13969
Hahn, F., Sanjurjo Loures, L., Sparks, C. A., Kanyuka, K., and Nekrasov, V. (2021). Efficient CRISPR/Cas-Mediated targeted mutagenesis in spring and winter wheat varieties. Plants 10, 1481. doi:10.3390/plants10071481
Han, X., Chen, Z., Li, P., Xu, H., Liu, K., Zha, W., et al. (2022). Development of novel rice germplasm for salt-tolerance at seedling stage using CRISPR-cas9. Sustainability 14, 2621. doi:10.3390/su14052621
He, F., Wang, C., Sun, H., Tian, S., Zhao, G., Liu, C., et al. (2023). Simultaneous editing of three homoeologues of TaCIPK14 confers broad-spectrum resistance to stripe rust in wheat. Plant Biotechnol. J. 21, 354–368. doi:10.1111/pbi.13956
Henders, S., Persson, U. M., and Kastner, T. (2015). Trading forests: land-use change and carbon emissions embodied in production and exports of forest-risk commodities. Environ. Res. Lett. 10, 125012. doi:10.1088/1748-9326/10/12/125012
Hillary, V. E., and Ceasar, S. A. (2022). A review on the mechanism and applications of CRISPR/Cas9/Cas12/Cas13/Cas14 proteins utilized for genome engineering. Mol. Biotechnol. 1, 311–325. doi:10.1007/s12033-022-00567-0
Howells, R. M., Craze, M., Bowden, S., and Wallington, E. J. (2018). Efficient generation of stable, heritable gene edits in wheat using CRISPR/Cas9. BMC Plant Biol. 18 (1), 215. doi:10.1186/s12870-018-1433-z
Huang, J., Gao, L., Luo, S., Liu, K., Qing, D., Pan, Y., et al. (2022). The genetic editing of GS3 via CRISPR/Cas9 accelerates the breeding of three-line hybrid rice with superior yield and grain quality. Mol. Breed. 42, 22. doi:10.1007/s11032-022-01290-z
Huang, Q., Lin, B., Cao, Y., Zhang, Y., Song, H., Huang, C., et al. (2023). CRISPR/Cas9-mediated mutagenesis of the susceptibility gene OsHPP04 in rice confers enhanced resistance to rice root-knot nematode. Front. Plant Sci. 14, 1134653. doi:10.3389/fpls.2023.1134653
Huanhe, W., Xiaoyu, G., Xiang, Z., Wang, Z., Xubin, Z., Yinglong, C., et al. (2024). Grain yield, biomass accumulation, and leaf photosynthetic characteristics of rice under combined salinity-drought stress. Rice Sci. 31, 118–128. doi:10.1016/j.rsci.2023.06.006
Hui, S., Li, H., Mawia, A. M., Zhou, L., Cai, J., Ahmad, S., et al. (2022). Production of aromatic three-line hybrid rice using novel alleles of BADH2. Plant Biotechnol. J. 20, 59–74. doi:10.1111/pbi.13695
Hyde, L., Osman, K., Winfield, M., Sanchez-Moran, E., Higgins, J. D., Henderson, I. R., et al. (2023). Identification, characterization, and rescue of CRISPR/Cas9 generated wheat SPO11-1 mutants. Plant Biotechnol. J. 21, 405–418. doi:10.1111/pbi.13961
Ibrahim, S., Saleem, B., Rehman, N., Zafar, S. A., Naeem, M. K., and Khan, M. R. (2022). CRISPR/Cas9 mediated disruption of Inositol Pentakisphosphate 2-Kinase 1 (TaIPK1) reduces phytic acid and improves iron and zinc accumulation in wheat grains. J. Adv. Res. 37, 33–41. doi:10.1016/j.jare.2021.07.006
Iizumi, T., Shiogama, H., Imada, Y., Hanasaki, N., Takikawa, H., and Nishimori, M. (2018). Crop production losses associated with anthropogenic climate change for 1981–2010 compared with preindustrial levels. Int. J. Climatol. 38, 5405–5417. doi:10.1002/joc.5818
Iwamoto, M. (2022). In-frame editing of transcription factor gene RDD1 to suppress miR166 recognition influences nutrient uptake, photosynthesis, and grain quality in rice. Sci. Rep. 12, 10795. doi:10.1038/s41598-022-14768-9
Jameel, M. R., Ansari, Z., Al-Huqail, A. A., Naaz, S., and Qureshi, M. I. (2022). CRISPR/Cas9-Mediated genome editing of soluble starch synthesis enzyme in rice for low glycemic index. Agronomy 2, 2206. doi:10.3390/agronomy12092206
Jeon, Y., Choi, Y. H., Jang, Y., Yu, J., Goo, J., Lee, G., et al. (2018). Direct observation of DNA target searching and cleavage by CRISPR-Cas12a. Nat. Commun. 9, 2777. doi:10.1038/s41467-018-05245-x
Jia, H., Li, M., Li, W., Liu, L., Jian, Y., Yang, Z., et al. (2020). A serine/threonine protein kinase encoding gene KERNEL NUMBER PER ROW6 regulates maize grain yield. Nat. Commun. 11, 988. doi:10.1038/s41467-020-14746-7
Jiang, L., Guo, T., Song, X., Jiang, H., Lu, M., Luo, J., et al. (2024a). MSH7 confers quantitative variation in pollen fertility and boosts grain yield in maize. Plant Biotechnol. J. 22, 1372–1386. doi:10.1111/pbi.14272
Jiang, M., Zhang, H., Song, Y., Chen, J., Bai, J., Tang, J., et al. (2024b). Transcription factor OsbZIP10 modulates rice grain quality by regulating OsGIF1. Plant J. 119 (5), 2181–2198. doi:10.1111/tpj.16911
Jin, S. K., Xu, L. N., Leng, Y. J., Zhang, M. Q., Yang, Q. Q., Wang, S. L., et al. (2023). The OsNAC24-OsNAP protein complex activates OsGBSSI and OsSBEI expression to fine-tune starch biosynthesis in rice endosperm. Plant Biotechnol. J. 21, 2224–2240. doi:10.1111/pbi.14124
Jinek, M., Chylinski, K., Fonfara, I., Hauer, M., Doudna, J. A., and Charpentier, E. (2012). A programmable dual-RNA–guided DNA endonuclease in adaptive bacterial immunity. Science 337, 816–821. doi:10.1126/science.1225829
Jing, L., Zhou, N., Lai, S., Wang, Y., Zhu, J., Wang, Y., et al. (2024a). Interactions between elevated atmospheric CO2 and temperature on rice yield are highly dependent on growth season temperature. Field Crops Res. 307, 109270. doi:10.1016/j.fcr.2024.109270
Jing, T., Wu, Y., Yu, Y., Li, J., Mu, X., Xu, L., et al. (2024b). Copine proteins are required for brassinosteroid signaling in maize and Arabidopsis. Nat. Commun. 15, 2028. doi:10.1038/s41467-024-46289-6
Jouanin, A., Borm, T., Boyd, L. A., Cockram, J., Leigh, F., Santos, B. A. C. M., et al. (2019). Development of the GlutEnSeq capture system for sequencing gluten gene families in hexaploid bread wheat with deletions or mutations induced by γ-irradiation or CRISPR/Cas9. J. Cereal Sci. 88, 157–166. doi:10.1016/j.jcs.2019.04.008
Kampmann, M. (2018). CRISPRi and CRISPRa screens in mammalian cells for precision biology and medicine. A.C.S. Chem. Biol. 13, 406–416. doi:10.1021/acschembio.7b00657
Kan, J., Cai, Y., Cheng, C., Chen, S., Jiang, C., He, Z., et al. (2023). CRISPR/Cas9-guided knockout of eIF4E improves Wheat yellow mosaic virus resistance without yield penalty. Plant Biotechnol. J. 21, 893–895. doi:10.1111/pbi.14002
Karavolias, N. G., Horner, W., Abugu, M. N., and Evanega, S. N. (2021). Application of gene editing for climate change in agriculture. Front. Sustain. Food. Syst. 5, 685801. doi:10.3389/fsufs.2021.685801
Karvelis, T., Druteika, G., Bigelyte, G., Budre, K., Zedaveinyte, R., Silanskas, A., et al. (2021). Transposon-associated TnpB is a programmable RNA-guided DNA endonuclease. Nature 599, 692–696. doi:10.1038/s41586-021-04058-1
Kaul, T., Thangaraj, A., Jain, R., Bharti, J., Kaul, R., Verma, R., et al. (2024). CRISPR/Cas9-mediated homology donor repair base editing system to confer herbicide resistance in maize (Zea mays L.). Plant Physiol. biochem. 207, 108374. doi:10.1016/j.plaphy.2024.108374
Kaur, N., Alok, A., Kumar, P., Kaur, N., Awasthi, P., Chaturvedi, S., et al. (2020). CRISPR/Cas9 directed editing of lycopene epsilon-cyclase modulates metabolic flux for β-carotene biosynthesis in banana fruit. Metab. Eng. 59, 76–86. doi:10.1016/j.ymben.2020.01.008
Kim, J. Y., Lee, Y. J., Lee, H. J., Go, J. Y., Lee, H. M., Park, J. S., et al. (2024). Knockout of OsGAPDHC7 gene encoding cytosolic Glyceraldehyde- 3-phosphate dehydrogenase affects Energy metabolism in rice seeds. Int. J. Mol. Sci. 25, 12470. doi:10.3390/ijms252212470
Kizildeniz, T., Pascual, I., Irigoyen, J. J., and Morales, F. (2018). Using fruit-bearing cuttings of grapevine and temperature gradient greenhouses to evaluate effects of climate change (elevated CO2 and temperature, and water deficit) on the cv. red and white Tempranillo. Yield and must quality in three consecutive growing seasons (2013–2015). Agric. Water Manag. 202, 299–310. doi:10.1016/j.agwat.2017.12.001
Klümper, W., and Qaim, M. (2014). A meta-analysis of the impacts of genetically modified crops. PLOS ONE 9, e111629. doi:10.1371/journal.pone.0111629
Kong, X., Wang, F., Wang, Z., Gao, X., Geng, S., Deng, Z., et al. (2023). Grain yield improvement by genome editing of TaARF12 that decoupled peduncle and rachis development trajectories via differential regulation of gibberellin signalling in wheat. Plant Biotechnol. J. 21, 1990–2001. doi:10.1111/pbi.14107
Krishna, T. P. A., Veeramuthu, D., Maharajan, T., and Soosaimanickam, M. (2023). The Era of plant breeding: conventional breeding to genomics-assisted breeding for crop improvement. Curr. Genom. 24, 24–35. doi:10.2174/1389202924666230517115912
Kuai, P., Lin, N., Ye, M., Chen, L., Chen, S., Zu, H., et al. (2024). Identification and knockout of a herbivore susceptibility gene enhances planthopper resistance and increases rice yield. Nat. Food 5, 846–859. doi:10.1038/s43016-024-01044-4
Kulcsár, P. I., Tálas, A., Ligeti, Z., Krausz, S. L., and Welker, E. (2022). SuperFi-Cas9 exhibits remarkable fidelity but severely reduced activity yet works effectively with ABE8e. Nat. Commun. 13, 6858. doi:10.1038/s41467-022-34527-8
Li, B., Du, X., Fei, Y., Wang, F., Xu, Y., Li, X., et al. (2021a). Efficient breeding of early-maturing rice cultivar by editing PHYC via CRISPR/Cas9. Rice 14, 86. doi:10.1186/s12284-021-00527-3
Li, C., Li, W., Zhou, Z., Chen, H., Xie, C., and Lin, Y. (2020a). A new rice breeding method: CRISPR/Cas9 system editing of the Xa13 promoter to cultivate transgene-free bacterial blight-resistant rice. Plant Biotechnol. J. 18, 313–315. doi:10.1111/pbi.13217
Li, D., Zhang, S., Lin, S., Xing, W., Yang, Y., Zhu, F., et al. (2024a). Cas12e orthologs evolve variable structural elements to facilitate dsDNA cleavage. Nat. Commun. 15, 10727. doi:10.1038/s41467-024-54491-9
Li, H., Liu, H., Hao, C., Li, T., Liu, Y., Wang, X., et al. (2023a). The auxin response factor TaARF15-A1 negatively regulates senescence in common wheat (Triticum aestivum L.). Plant Physiol. 191, 1254–1271. doi:10.1093/plphys/kiac497
Li, H., Wang, L., Liu, M., Dong, Z., Li, Q., Fei, S., et al. (2020b). Maize plant architecture is regulated by the ethylene biosynthetic gene ZmACS7. Plant Physiol. 183, 1184–1199. doi:10.1104/pp.19.01421
Li, H., Zhang, Y., Wu, C., Bi, J., Chen, Y., Jiang, C., et al. (2022a). Fine-tuning OsCPK18/OsCPK4 activity via genome editing of phosphorylation motif improves rice yield and immunity. Plant Biotechnol. J. 20, 2258–2271. doi:10.1111/pbi.13905
Li, J., Jiao, G., Sun, Y., Chen, J., Zhong, Y., Yan, L., et al. (2021b). Modification of starch composition, structure and properties through editing of TaSBEIIa in both winter and spring wheat varieties by CRISPR/Cas9. Plant Biotechnol. J. 19, 937–951. doi:10.1111/pbi.13519
Li, J., Zhang, X., Sun, Y., Zhang, J., Du, W., Guo, X., et al. (2018). Efficient allelic replacement in rice by gene editing: a case study of the NRT1. 1B gene. J. Integr. Plant Biol. 60, 536–540. doi:10.1111/jipb.12650
Li, P., Li, Z., Xie, G., and Zhang, J. (2021c). Trihelix transcription factor ZmThx20 is required for kernel development in maize. Int. J. Mol. Sci. 22, 12137. doi:10.3390/ijms222212137
Li, S., Lin, D., Zhang, Y., Deng, M., Chen, Y., Lv, B., et al. (2022b). Genome-edited powdery mildew resistance in wheat without growth penalties. Nature 602, 455–460. doi:10.1038/s41586-022-04395-9
Li, W., Li, Y., Shi, H., Wang, H., Ji, K., Zhang, L., et al. (2024b). ZmMPK6, a mitogen-activated protein kinase, regulates maize kernel weight. J. Exp. Bot. erae104 75, 3287–3299. doi:10.1093/jxb/erae104
Li, X., Xie, J., Dong, C., Zheng, Z., Shen, R., Cao, X., et al. (2024c). Efficient and heritable A-to-K base editing in rice and tomato. Hortic. Res. 11, 250. doi:10.1093/hr/uhad250
Li, X., Yu, Y., Yao, W., Yin, Z., Wang, Y., Huang, Z., et al. (2023b). CRISPR/Cas9-mediated simultaneous mutation of three salicylic acid 5-hydroxylase (OsS5H) genes confers broad-spectrum disease resistance in rice. Plant Biotechnol. J. 21, 1873–1886. doi:10.1111/pbi.14099
Li, Y., Wu, S., Huang, Y., Ma, X., Tan, L., Liu, F., et al. (2023c). OsMADS17 simultaneously increases grain number and grain weight in rice. Nat. Commun. 14 (1), 3098. doi:10.1038/s41467-023-38726-9
Li, Z., Li, Q., and Chen, T. (2024d). Record-breaking high-temperature outlook for 2023: an assessment based on the China global merged temperature (CMST) dataset. Adv. Atmos. Sci. 41, 369–376. doi:10.1007/s00376-023-3200-9
Li, Z., Rao, M. J., Li, J., Wang, Y., Chen, P., Yu, H., et al. (2022c). CRISPR/Cas9 mutant rice Ospmei12 involved in growth, cell wall development, and response to phytohormone and heavy metal stress. Int. J. Mol. Sci. 23, 16082. doi:10.3390/ijms232416082
Li, Z., and Zhang, J. (2022d). Effects of raised ambient temperature on the local and systemic adaptions of maize. Plants 11, 755. doi:10.3390/plants11060755
Liang, Z., Chen, K., Li, T., Zhang, Y., Wang, Y., Zhao, Q., et al. (2017). Efficient DNA-free genome editing of bread wheat using CRISPR/Cas9 ribonucleoprotein complexes. Nat. Commun. 8, 14261. doi:10.1038/ncomms14261
Liao, S., Qin, X., Luo, L., Han, Y., Wang, X., Usman, B., et al. (2019). CRISPR/Cas9-induced mutagenesis of semi-rolled leaf1, 2 confers curled leaf phenotype and drought tolerance by influencing protein expression patterns and ROS scavenging in rice (Oryza sativa L.). Agronomy 9, 728. doi:10.3390/agronomy9110728
Lin, Q., Zong, Y., Xue, C., Wang, S., Jin, S., Zhu, Z., et al. (2020). Prime genome editing in rice and wheat. Nat. Biotechnol. 38, 582–585. doi:10.1038/s41587-020-0455-x
Liu, B., Asseng, S., Müller, C., Ewert, F., and Elliott, J. (2016). Similar estimates of temperature impacts on global wheat yield by three independent methods. Nat. Clim. Change 6, 1130. doi:10.1038/nclimate3115
Liu, B., Wang, N., Yang, R., Wang, X., Luo, P., Chen, Y., et al. (2024a). ZmADF5, a maize actin-depolymerizing factor conferring enhanced drought tolerance in maize. Plants 13, 619. doi:10.3390/plants13050619
Liu, C., Kong, M., Yang, F., Zhu, J., Qi, X., Weng, J., et al. (2022a). Targeted generation of Null Mutants in ZmGDIα confers resistance against maize rough dwarf disease without agronomic penalty. Plant Biotechnol. J. 20, 803–805. doi:10.1111/pbi.13793
Liu, K., Sakuraba, Y., Ohtsuki, N., Yang, M., Ueda, Y., and Yanagisawa, S. (2023a). CRISPR/Cas9-mediated elimination of OsHHO3, a transcriptional repressor of three AMMONIUM TRANSPORTER1 genes, improves nitrogen use efficiency in rice. Plant Biotechnol. J. 21, 2169–2172. doi:10.1111/pbi.14167
Liu, L., Gallagher, J., Arevalo, E. D., Chen, R., Skopelitis, T., Wu, Q., et al. (2021). Enhancing grain-yield-related traits by CRISPR–Cas9 promoter editing of maize CLE genes. Nat. Plants 7, 287–294. doi:10.1038/s41477-021-00858-5
Liu, M., Wang, F., He, B., Hu, J., Dai, Y., Chen, W., et al. (2024b). Targeting Magnaporthe oryzae effector MoErs1 and host papain-like protease OsRD21 interaction to combat rice blast. Nat. Plants 10, 618–632. doi:10.1038/s41477-024-01642-x
Liu, S., Zhang, F., Su, J., Fang, A., Tian, B., Yu, Y., et al. (2024c). CRISPR-targeted mutagenesis of mitogen-activated protein kinase phosphatase 1 improves both immunity and yield in wheat. Plant Biotechnol. J. 22, 1929–1941. doi:10.1111/pbi.14312
Liu, W. Y., Lin, H. H., Yu, C. P., Chang, C. K., Chen, H. J., Lin, J. J., et al. (2020). Maize ANT1 modulates vascular development, chloroplast development, photosynthesis, and plant growth. PNAS 117, 21747–21756. doi:10.1073/pnas.2012245117
Liu, X., Ding, Q., Wang, W., Pan, Y., Tan, C., Qiu, Y., et al. (2022b). Targeted deletion of the first intron of the wxb allele via CRISPR/Cas9 significantly increases grain amylose content in rice. Rice 15, 1. doi:10.1186/s12284-021-00548-y
Liu, X., Zhang, S., Jiang, Y., Yan, T., Fang, C., Hou, Q., et al. (2022c). Use of CRISPR/Cas9-Based gene editing to simultaneously mutate multiple homologous genes required for pollen development and male fertility in maize. Cells 11, 439. doi:10.3390/cells11030439
Liu, Y., Chen, Z., Zhang, C., Guo, J., Liu, Q., Yin, Y., et al. (2023b). Gene editing of ZmGA20ox3 improves plant architecture and drought tolerance in maize. Plant Cell Rep. 43, 18. doi:10.1007/s00299-023-03090-x
Liu, Y. C., Qiu, S. J., Jin, M., Deng, H. C., Yin, M., Chen, Z. F., et al. (2019). Study on the application of CRISPR/Cas9 technology in development of tomato (Solanum lycopersicum) Male Sterile Line. J. Agric. Biotechnol. 27, 951. doi:10.3969/j.issn.1674-7968.2019.06.001
Lizaso, J. I., Ruiz-Ramos, M., Rodríguez, L., Gabaldon-Leal, C., Oliveira, J. A., Lorite, I. J., et al. (2018). Impact of high temperatures in maize: phenology and yield components. Field Crops Res. 216, 129–140. doi:10.1016/j.fcr.2017.11.013
Lopes Filho, H., de Carvalho, T., Yassitepe, J. E., Koltun, A., Pauwels, L., Heinzen da Silva, V. C., et al. (2023). Genome editing in maize: toward improving complex traits in a global crop. Genet. Mol. Biol. 46, e20220217. doi:10.1590/1678-4685-GMB-2022-0217
Lou, H., Li, S., Shi, Z., Zou, Y., Zhang, Y., Huang, X., et al. (2025). Engineering source-sink relations by prime editing confers heat-stress resilience in tomato and rice. Cell 188, 530–549.e20. doi:10.1016/j.cell.2024.11.005
Lu, Q., Luo, X., Yang, X., Zhou, T., Zhang, Y., Lan, Y., et al. (2023). CRISPR/Cas9-mediated gene editing of vacuolar ATPase subunit d mediates phytohormone biosynthesis and virus resistance in rice. Front. Plant Sci. 14, 1122978. doi:10.3389/fpls.2023.1122978
Luo, J., Abid, M., Tu, J., Cai, X., Zhang, Y., Gao, P., et al. (2023). Cytosine base editors (CBEs) for inducing targeted DNA base editing in Nicotiana benthamiana. BMC Plant Biol. 23, 305. doi:10.1186/s12870-023-04322-8
Luo, M., Zhang, Y., Li, J., Zhang, P., Chen, K., Song, W., et al. (2021). Molecular dissection of maize seedling salt tolerance using a genome-wide association analysis method. Plant Biotechnol. J. 19, 1937–1951. doi:10.1111/pbi.13607
Lv, G., Li, Y., Wu, Z., Zhang, Y., Li, X., Wang, T., et al. (2024). Maize actin depolymerizing factor 1 (ZmADF1) negatively regulates pollen development. BBRC 703, 149637. doi:10.1016/j.bbrc.2024.149637
Ly, L. K., Ho, T. M., Bui, T. P., Nguyen, L. T., Phan, Q., Le, N. T., et al. (2024). CRISPR/Cas9 targeted mutations of OsDSG1 gene enhanced salt tolerance in rice. Funct. Integr. Genom 24, 70. doi:10.1007/s10142-024-01347-6
Ma, L., Ruan, J., Song, J., Wen, L., Yang, D., Zhao, J., et al. (2020). MiCas9 increases large size gene knock-in rates and reduces undesirable on-target and offtarget indel edits. Nat. Commun. 11, 6082. doi:10.1038/s41467-020-19842-2
Ma, L., Sun, Y., Ruan, X., Huang, P. C., Wang, S., Li, S., et al. (2021). Genome-wide characterization of jasmonates signaling components reveals the essential role of ZmCOI1a-ZmJAZ15 action module in regulating maize immunity to gibberella stalk rot. Int. J. Mol. Sci. 22, 870. doi:10.3390/ijms22020870
Maher, M. F., Nasti, R. A., Vollbrecht, M., Starker, C. G., Clark, M. D., and Voytas, D. F. (2020). Plant gene editing through de novo induction of meristems. Nat. Biotechnol. 38, 84–89. doi:10.1038/s41587-019-0337-2
Malenica, N., Dunić, J. A., Vukadinović, L., Cesar, V., and Šimić, D. (2021). Genetic approaches to enhance multiple stress tolerance in maize. Genes 12, 1760. doi:10.3390/genes12111760
Martins, J., Gonçalves, R., and Branco, F. (2022). A bibliometric analysis and visualization of e-learning adoption using VOSviewer. UAIS 1, 1177–1191. doi:10.1007/s10209-022-00953-0
Miao, C., Xiao, L., Hua, K., Zou, C., Zhao, Y., Bressan, R. A., et al. (2018). Mutations in a subfamily of abscisic acid receptor genes promote rice growth and productivity. PNAS 115, 6058–6063. doi:10.1073/pnas.1804774115
Miao, J., Guo, D., Zhang, J., Huang, Q., Qin, G., Zhang, X., et al. (2013). Targeted mutagenesis in rice using CRISPR-Cas system. Cell Res. 23, 1233–1236. doi:10.1038/cr.2013.123
Mkhabela, M., Bullock, P., Sapirstein, H., Courcelles, J., Abbasi, S., and Koksel, F. (2022). Exploring the influence of weather on gluten strength of hard red spring wheat (Triticum aestivum L.) on the Canadian Prairies. J. Cereal Sci. 104, 103410. doi:10.1016/j.jcs.2021.103410
Mohr, T., Horstman, J., Gu, Y. Q., Elarabi, N. I., Abdallah, N. A., and Thilmony, R. (2022). CRISPR-Cas9 gene editing of the Sal1 gene family in wheat. Plants 11, 2259. doi:10.3390/plants11172259
Müller, A., Nunes, M. T., Maldaner, V., Coradi, P. C., Moraes, R. S. D., Martens, S., et al. (2022). Rice drying, storage and processing: effects of post-harvest operations on grain quality. Rice Sci. 29, 16–30. doi:10.1016/j.rsci.2021.12.002
Musakwa, W. (2018). Identifying land suitable for agricultural land reform using GIS-MCDA in South Africa. Environ. Dev. Sustain. 20, 2281–2299. doi:10.1007/s10668-017-9989-6
Ning, Q., Jian, Y., Du, Y., Li, Y., Shen, X., Jia, H., et al. (2021). An ethylene biosynthesis enzyme controls quantitative variation in maize ear length and kernel yield. Nat. Commun. 12, 5832. doi:10.1038/s41467-021-26123-z
Nunez, S., Arets, E., Alkemade, R., Verwer, C., and Leemans, R. (2019). Assessing the impacts of climate change on biodiversity: is below 2° C enough? Clim. Change. 154, 351–365. doi:10.1007/s10584-019-02420-x
Ogata, T., Ishizaki, T., Fujita, M., and Fujita, Y. (2020). CRISPR/Cas9-targeted mutagenesis of OsERA1 confers enhanced responses to abscisic acid and drought stress and increased primary root growth under nonstressed conditions in rice. PLOS ONE 15, e0243376. doi:10.1371/journal.pone.0243376
Oliva, R., Ji, C., Atienza-Grande, G., Huguet-Tapia, J. C., Perez-Quintero, A., Li, T., et al. (2019). Broad-spectrum resistance to bacterial blight in rice using genome editing. Nat. Biotechnol. 37, 1344–1350. doi:10.1038/s41587-019-0267-z
Ortiz, A. M. D., Outhwaite, C. L., Dalin, C., and Newbold, T. (2021). A review of the interactions between biodiversity, agriculture, climate change, and international trade: research and policy priorities. One Earth 4, 88–101. doi:10.1016/j.oneear.2020.12.008
Pan, Y., Chen, L., Pang, L., Chen, X., Jia, X., and Li, X. (2020a). Ultrasound treatment inhibits browning and improves antioxidant capacity of fresh-cut sweet potato during cold storage. RSC Adv. 10, 9193–9202. doi:10.1039/c9ra06418d
Pan, Z., Liu, M., Zhao, H., Tan, Z., Liang, K., Sun, Q., et al. (2020b). ZmSRL5 is involved in drought tolerance by maintaining cuticular wax structure in maize. J. Integr. Plant Biol. 62, 1895–1909. doi:10.1111/jipb.12982
Pang, Y., Cao, L., Ye, F., Ma, C., Liang, X., Song, Y., et al. (2024). Identification of the maize PP2C gene family and functional studies on the role of zmPP2C15 in drought tolerance. Plants 13, 340. doi:10.3390/plants13030340
Peng, B., Liu, Y., Sun, X., Zhao, Q., Qiu, J., Tian, X., et al. (2024). The OsGAPC3 mutation significantly affects grain quality traits and improves the nutritional quality of rice. Front. Plant Sci. 15, 1470316. doi:10.3389/fpls.2024.1470316
Prasanna, B. M., Cairns, J. E., Zaidi, P. H., Beyene, Y., Makumbi, D., Gowda, M., et al. (2021). Beat the stress: breeding for climate resilience in maize for the tropical rainfed environments. Theor. Appl. Genet. 134, 1729–1752. doi:10.1007/s00122-021-03773-7
Qi, X., Guo, S., Wang, D., Zhong, Y., Chen, M., Chen, C., et al. (2022). ZmCOI2a and ZmCOI2b redundantly regulate anther dehiscence and gametophytic male fertility in maize. Plant J. 110, 849–862. doi:10.1111/tpj.15708
Qin, Q., Wang, Y., Huang, L., Du, F., Zhao, X., Li, Z., et al. (2020). A U-box E3 ubiquitin ligase OsPUB67 is positively involved in drought tolerance in rice. Plant Mol. Biol. 102, 89–107. doi:10.1007/s11103-019-00933-8
Qing, D., Chen, W., Huang, S., Li, J., Pan, Y., Zhou, W., et al. (2023). Editing of rice (Oryza sativa L.) OsMKK3 gene using CRISPR/Cas9 decreases grain length by modulating the expression of photosystem components. PROTEOMICS 23, 2200538. doi:10.1002/pmic.202200538
Rahim, A. A., Uzair, M., Rehman, N., Fiaz, S., Attia, K. A., Abushady, A. M., et al. (2024). CRISPR/Cas9 mediated TaRPK1 root architecture gene mutagenesis confers enhanced wheat yield. J. King Saud. Univ. Sci. 36, 103063. doi:10.1016/j.jksus.2023.103063
Rangan, P., Furtado, A., and Henry, R. (2020). Transcriptome profiling of wheat genotypes under heat stress during grain-filling. J. Cereal Sci. 91, 102895. doi:10.1016/j.jcs.2019.102895
Raza, A., Tabassum, J., Fakhar, A. Z., Sharif, R., Chen, H., Zhang, C., et al. (2022). Smart reprograming of plants against salinity stress using modern biotechnological tools. Crit. Rev. Biotechnol. 43, 1035–1062. doi:10.1080/07388551.2022.2093695
Ren, R. C., Kong, L. G., Zheng, G. M., Zhao, Y. J., Jiang, X., Wu, J. W., et al. (2024). Maize requires arogenate dehydratase 2 for resistance to Ustilago maydis and plant development. Plant Physiol. kiae115 195, 1642–1659. doi:10.1093/plphys/kiae115
Santosh, K. V. V., Verma, R. K., Yadav, S. K., Yadav, P., Watts, A., Rao, M. V., et al. (2020). CRISPR-Cas9 mediated genome editing of drought and salt tolerance (OsDST) gene in indica mega rice cultivar MTU1010. Physiol. Mol. Biol. Plants. 26, 1099–1110. doi:10.1007/s12298-020-00819-w
Sasaki, T., and Burr, B. (2000). International Rice Genome Sequencing Project: the effort to completely sequence the rice genome. Curr. Opin. Plant Biol. 3, 138–141. doi:10.1016/s1369-5266(99)00047-3
Schindele, P., Wolter, F., and Puchta, H. (2018). Transforming plant biology and breeding with CRISPR/Cas9, Cas12 and Cas13. FEBS Lett. 592, 1954–1967. doi:10.1002/1873-3468.13073
Schmidheini, L., Mathis, N., Marquart, K. F., Rothgangl, T., Kissling, L., Böck, D., et al. (2024). Continuous directed evolution of a compact CjCas9 variant with broad PAM compatibility. Nat. Chem. Biol. 20, 333–343. doi:10.1038/s41589-023-01427-x
Schmidt, M. J., Gupta, A., Bednarski, C., Gehrig-Giannini, S., Richter, F., Pitzler, C., et al. (2021). Improved CRISPR genome editing using small highly active and specific engineered RNA-guided nucleases. Nat. Commun. 12, 4219. doi:10.1038/s41467-021-24454-5
Schuler, G., Hu, C., and Ke, A. (2022). Structural basis for RNA-guided DNA cleavage by IscB-ωRNA and mechanistic comparison with Cas9. Science 376, 1476–1481. doi:10.1126/science.abq7220
Shah, P. R., Varanavasiappan, S., Kokiladevi, E., Ramanathan, A., and Kumar, K. K. (2019). Genome editing of rice PFT1 gene to study its role in rice sheath blight disease resistance. Int. J. Curr. Microbiol. Appl. Sci. 8, 2356–2364. doi:10.20546/ijcmas.2019.806.281
Shahzad, A., Ullah, S., Dar, A. A., Sardar, M. F., Mehmood, T., Tufail, M. A., et al. (2021). Nexus on climate change: agriculture and possible solution to cope future climate change stresses. ESPR 28, 14211–14232. doi:10.1007/s11356-021-12649-8
Shan, Q., Wang, Y., Li, J., Zhang, Y., Chen, K., Liang, Z., et al. (2013). Targeted genome modification of crop plants using a CRISPR-Cas system. Nat. Biotechnol. 31, 686–688. doi:10.1038/nbt.2650
Sharma, S., Kumar, A., Kumari, N., and Walia, A. (2023). RNAi as a tool to enhance crop yield and biotic stress management in the plants. PCTOC 152, 437–454. doi:10.1007/s11240-022-02426-x
Shen, L., Hua, Y., Fu, Y., Li, J., Liu, Q., Jiao, X., et al. (2017). Rapid generation of genetic diversity by multiplex CRISPR/Cas9 genome editing in rice. Sci. China Technol. Sci. 60, 506–515. doi:10.1007/s11427-017-9008-8
Sheng, X., Ai, Z., Tan, Y., Hu, Y., Guo, X., Liu, X., et al. (2023). Novel salinity-tolerant third-generation hybrid rice developed via CRISPR/Cas9-Mediated gene editing. Int. J. Mol. Sci. 24, 8025. doi:10.3390/ijms24098025
Shewry, P. (2019). What is gluten—why is it special? Front. Nutr. 101, 101. doi:10.3389/fnut.2019.00101
Shi, J., Gao, H., Wang, H., Lafitte, H. R., Archibald, R. L., Yang, M., et al. (2017). ARGOS 8 variants generated by CRISPR-Cas9 improve maize grain yield under field drought stress conditions. Plant Biotechnol. J. 15, 207–216. doi:10.1111/pbi.12603
Shim, K. C., Adeva, C., Kang, J. W., Luong, N. H., Lee, H. S., Cho, J. H., et al. (2022). Interaction of starch branching enzyme 3 and granule-bound starch synthase 1 alleles increases amylose content and alters physico-chemical properties in japonica rice (Oryza sativa L.). Front. Plant Sci. 13, 968795. doi:10.3389/fpls.2022.968795
Shim, Y., Seong, G., Choi, Y., Lim, C., Baek, S. A., Park, Y. J., et al. (2023). Suppression of cuticular wax biosynthesis mediated by rice LOV KELCH REPEAT PROTEIN 2 supports a negative role in drought stress tolerance. Plant Cell Environ. 46, 1504–1520. doi:10.1111/pce.14549
Shimoyanagi, R., Abo, M., and Shiotsu, F. (2021). Higher temperatures during grain filling affect grain chalkiness and rice nutrient contents. Agronomy 11, 1360. doi:10.3390/agronomy11071360
Shukla, P. R., Skeg, J., Buendia, E. C., Masson-Delmotte, V., Pörtner, H. O., Roberts, D. C., et al. (2019). Climate Change and Land: an IPCC special report on climate change, desertification, land degradation, sustainable land management, food security, and greenhouse gas fluxes in terrestrial ecosystems. Available online at: https://www.ipcc.ch/site/assets/uploads/2019/11/SRCCL-Full-Report-Compiled-191128.pdf (Accessed December 11, 2023).
Simmons, C. R., Lafitte, H. R., Reimann, K. S., Brugière, N., Roesler, K., Albertsen, M. C., et al. (2021). Successes and insights of an industry biotech program to enhance maize agronomic traits. Plant Sci. 307, 110899. doi:10.1016/j.plantsci.2021.110899
Singh, S., Chaudhary, R., Chaturvedi, S., and Tiwari, S. (2024b). “Deciphering the role of CRISPR/Cas9 in the amelioration of abiotic and biotic stress conditions,” in Gene editing in plants. Editors A. Kumar, S. Arora, S. Ogita, Y. Y. Yau, and K. Mukherjee (Singapore: Springer). doi:10.1007/978-981-99-8529-6_8
Singh, S., Chaudhary, R., Lokya, V., and Tiwari, S. (2024a). Genome editing based trait improvement in crops: current perspective, challenges and opportunities. Nucleus 67, 97–126. doi:10.1007/s13237-024-00472-8
Smith, M. R., and Myers, S. S. (2018). Impact of anthropogenic CO2 emissions on global human nutrition. Nat. Clim. Change 8, 834–839. doi:10.1038/s41558-018-0253-3
Soengas, P., Rodríguez, V. M., Velasco, P., and Cartea, M. E. (2018). Effect of temperature stress on antioxidant defenses in Brassica oleracea. ACS Omega 3, 5237–5243. doi:10.1021/acsomega.8b00242
Son, S., Song, G., Nam, S., Lee, G., Im, J., Lee, K. S., et al. (2024). CRISPR/Cas9-mediated mutagenesis of rice NAC transcription factor genes results in altered innate immunity. Plant Physiol. 195, 1138–1142. doi:10.1093/plphys/kiae084
Song, X., Chen, Z., Du, X., Li, B., Fei, Y., Tao, Y., et al. (2023). Generation of new rice germplasms with low amylose content by CRISPR/CAS9-targeted mutagenesis of the FLOURY ENDOSPERM 2 gene. Front. Plant Sci. 14, 1138523. doi:10.3389/fpls.2023.1138523
Song, X., Meng, X., Guo, H., Cheng, Q., Jing, Y., Chen, M., et al. (2022). Targeting a gene regulatory element enhances rice grain yield by decoupling panicle number and size. Nat. Biotechnol. 40, 1403–1411. doi:10.1038/s41587-022-01281-7
Sony, S. K., Kaul, T., Motelb, K. F. A., Thangaraj, A., Bharti, J., Kaul, R., et al. (2023). CRISPR/Cas9-mediated homology donor repair base editing confers glyphosate resistance to rice (Oryza sativa L.). Front. Plant Sci. 14, 1122926. doi:10.3389/fpls.2023.1122926
Steinwand, M. A., and Ronald, P. C. (2020). Crop biotechnology and the future of food. Nat. Food. 1, 273–283. doi:10.1038/s43016-020-0072-3
Sun, A., Li, C. P., Chen, Z., Zhang, S., Li, D. Y., Yang, Y., et al. (2023a). The compact Casπ (Cas12l)‘bracelet’provides a unique structural platform for DNA manipulation. Cell Res. 33, 229–244. doi:10.1038/s41422-022-00771-2
Sun, N., and Zhao, H. (2013). Transcription activator-like effector nucleases (TALENs): a highly efficient and versatile tool for genome editing. Biotechnol. Bioeng. 110, 1811–1821. doi:10.1002/bit.24890
Sun, W., Zhang, H., Yang, S., Liu, L., Xie, P., Li, J., et al. (2023b). Genetic modification of Gγ subunit AT1 enhances salt-alkali tolerance in main graminaceous crops. Natl. Sci. Rev. 10 (6), nwad075. doi:10.1093/nsr/nwad075
Tang, Y., Abdelrahman, M., Li, J., Wang, F., Ji, Z., Qi, H., et al. (2021). CRISPR/Cas9 induces exon skipping that facilitates development of fragrant rice. Plant Biotechnol. J. 19, 642–644. doi:10.1111/pbi.13514
Távora, F. T. P. K., Meunier, A. C., Vernet, A., Portefaix, M., Milazzo, J., Adreit, H., et al. (2022). CRISPR/Cas9-Targeted knockout of rice susceptibility genes OsDjA2 and OsERF104 reveals alternative sources of resistance to Pyricularia oryzae. Rice Sci. 29, 535–544. doi:10.1016/j.rsci.2022.04.001
Thakur, N., Lakhani, H., and Tiwari, S. (2024). “Detailed insight into various classes of the CRISPR/Cas system to develop future crops,” in Gene editing in plants. Editors A. Kumar, S. Arora, S. Ogita, Y. Y. Yau, and K. Mukherjee (Singapore: Springer). doi:10.1007/978-981-99-8529-6_9
Tian, X., Qin, Z., Zhao, Y., Wen, J., Lan, T., Zhang, L., et al. (2022). Stress granule-associated TaMBF1c confers thermotolerance through regulating specific mRNA translation in wheat (Triticum aestivum). New Phytol. 233, 1719–1731. doi:10.1111/nph.17865
Toinga-Villafuerte, S., Vales, M. I., Awika, J. M., and Rathore, K. S. (2022). CRISPR/Cas9-mediated mutagenesis of the granule-bound starch synthase gene in the potato variety Yukon Gold to obtain amylose-free starch in tubers. Int. J. Mol. Sci. 23, 4640. doi:10.3390/ijms23094640
Tu, B., Zhang, T., Liu, P., Yang, W., Zheng, L., Dai, Y., et al. (2024). The LCG1-OsBP5/OsEBP89-Wx module regulates the grain chalkiness and taste quality in rice. Plant Biotechnol. J. 23 (1), 36–50. doi:10.1111/pbi.14475
Urnov, F. D., Miller, J. C., Lee, Y. L., Beausejour, C. M., Rock, J. M., Augustus, S., et al. (2005). Highly efficient endogenous human gene correction using designed zinc-finger nucleases. Nature 435, 646–651. doi:10.1038/nature03556
Usman, B., Nawaz, G., Zhao, N., Liao, S., Qin, B., Liu, F., et al. (2021). Programmed editing of rice (Oryza sativa L.) OsSPL16 gene using CRISPR/Cas9 improves grain yield by modulating the expression of pyruvate enzymes and cell cycle proteins. Int. J. Mol. Sci. 22, 249. doi:10.3390/ijms22010249
Usman, B., Nawaz, G., Zhao, N., Liu, Y., and Li, R. (2020). Generation of high yielding and fragrant rice (Oryza sativa L.) lines by CRISPR/Cas9 targeted mutagenesis of three homoeologs of cytochrome P450 gene family and OsBADH2 and transcriptome and proteome profiling of revealed changes triggered by mutations. Plants 9, 788. doi:10.3390/plants9060788
Van, V. T., Das, S., Hensel, G., and Kim, J. Y. (2022). Genome editing and beyond: what does it mean for the future of plant breeding? Planta 255, 130. doi:10.1007/s00425-022-03906-2
Viana, C. M., Freire, D., Abrantes, P., Rocha, J., and Pereira, P. (2022). Agricultural land systems importance for supporting food security and sustainable development goals: a systematic review. Sci. Total Environ. 806, 150718. doi:10.1016/j.scitotenv.2021.150718
Wang, B., Zhong, Z., Wang, X., Han, X., Yu, D., Wang, C., et al. (2020a). Knockout of the OsNAC006 transcription factor causes drought and heat sensitivity in rice. Int. J. Mol. Sci. 21, 2288. doi:10.3390/ijms21072288
Wang, C., Wang, G., Gao, Y., Lu, G., Habben, J. E., Mao, G., et al. (2020b). A cytokinin-activation enzyme-like gene improves grain yield under various field conditions in rice. Plant Mol. Biol. 102, 373–388. doi:10.1007/s11103-019-00952-5
Wang, G., Wang, F., Xu, Z., Wang, Y., Zhang, C., Zhou, Y., et al. (2024a). Precise fine-turning of GhTFL1 by base editing tools defines ideal cotton plant architecture. Genome Biol. 25, 59. doi:10.1186/s13059-024-03189-8
Wang, H., Huang, Y., Li, Y., Cui, Y., Xiang, X., Zhu, Y., et al. (2024b). An ARF gene mutation creates flint kernel architecture in dent maize. Nat. Commun. 15, 2565. doi:10.1038/s41467-024-46955-9
Wang, J., Teng, Y., Zhang, R., Wu, Y., Lou, L., Zou, Y., et al. (2021a). Engineering a PAM-flexible SpdCas9 variant as a universal gene repressor. Nat. Commun. 12 (1), 6916. doi:10.1038/s41467-021-27290-9
Wang, J. D., Wang, J., Huang, L. C., Kan, L. J., Wang, C. X., Xiong, M., et al. (2024c). ABA-mediated regulation of rice grain quality and seed dormancy via the NF-YB1-SLRL2-bHLH144 Module. Nat. Commun. 15 (1), 4493. doi:10.1038/s41467-024-48760-w
Wang, W., Pan, Q., Tian, B., He, F., Chen, Y., Bai, G., et al. (2019). Gene editing of the wheat homologs of TONNEAU 1-recruiting motif encoding gene affects grain shape and weight in wheat. Plant J. 100, 251–264. doi:10.1111/tpj.14440
Wang, W., Wang, W., Pan, Y., Tan, C., Li, H., Chen, Y., et al. (2022a). A new gain-of-function OsGS2/GRF4 allele generated by CRISPR/Cas9 genome editing increases rice grain size and yield. Crop J. 10, 1207–1212. doi:10.1016/j.cj.2022.01.004
Wang, X., Cai, C., Song, L., Zhou, W., Yang, X., Gu, X., et al. (2024d). Responses of rice grain yield and quality to factorial combinations of ambient and elevated CO2 and temperature in T-FACE environments. Field Crops Res. 309, 109328. doi:10.1016/j.fcr.2024.109328
Wang, X., Guo, Y., Wang, Y., Peng, Y., Zhang, H., and Zheng, J. (2024e). ZmHDT103 negatively regulates drought stress tolerance in maize seedlings. Agronomy 14, 134. doi:10.3390/agronomy14010134
Wang, X., and Liu, F. (2021b). Effects of elevated CO2 and heat on wheat grain quality. Plants 10, 1027. doi:10.3390/plants10051027
Wang, X., Pan, W., Sun, C., Yang, H., Cheng, Z., Yan, F., et al. (2024f). Creating large-scale genetic diversity in Arabidopsis via base editing-mediated deep artificial evolution. Genome Biol. 25, 215. doi:10.1186/s13059-024-03358-9
Wang, X., Zhang, Z., Peng, W., Huang, J., Yan, X., Yao, W., et al. (2023). Inositolphosphorylceramide synthases, OsIPCSs, regulate plant height in rice. Plant Sci. 335, 111798. doi:10.1016/j.plantsci.2023.111798
Wang, Y., Tang, Q., Kang, Y., Wang, X., Zhang, H., and Li, X. (2022b). Analysis of the utilization and prospects of CRISPR-Cas technology in the annotation of gene function and creation new germplasm in maize based on patent data. Cells 11, 3471. doi:10.3390/cells11213471
Wechsler, S. J. (2018). Trends in the adoption of genetically engineered corn, cotton, and soybeans. Amber waves: the economics of food, farming, natural resources, and rural America. (1490-2020-690). United States department of agriculture. Econ. Res. Serv. 0 (11). doi:10.22004/ag.econ.302674
Wei, T., Jiang, L., You, X., Ma, P., Xi, Z., and Wang, N. N. (2023). Generation of herbicide-resistant soybean by base editing. Biology 12 (5), 741. doi:10.3390/biology12050741
Wen, J., Qin, Z., Sun, L., Zhang, Y., Wang, D., Peng, H., et al. (2023). Alternative splicing of TaHSFA6e modulates heat shock protein–mediated translational regulation in response to heat stress in wheat. New Phytol. 239, 2235–2247. doi:10.1111/nph.19100
Wieser, H., Koehler, P., and Scherf, K. A. (2020). The two faces of wheat. Front. Nutr. 7, 517313. doi:10.3389/fnut.2020.517313
Wold-McGimsey, F., Krosch, C., Alarcón-Reverte, R., Ravet, K., Katz, A., Stromberger, J., et al. (2023). Multi-target genome editing reduces Polyphenol oxidase activity in wheat (Triticum aestivum L.) grains. Front. Plant Sci. 14, 1247680. doi:10.3389/fpls.2023.1247680
Wu, Q., Liu, Y., and Huang, J. (2022). CRISPR-Cas9 mediated mutation in OsPUB43 improves grain length and weight in rice by promoting cell proliferation in spikelet hull. Int. J. Mol. Sci. 23, 2347. doi:10.3390/ijms23042347
Wu, S., Kyaw, H., Tong, Z., Yang, Y., Wang, Z., Zhang, L., et al. (2024). A simple and efficient CRISPR/Cas9 system permits ultra-multiplex genome editing in plants. Crop J. 12, 569–582. doi:10.1016/j.cj.2024.01.010
Wu, Y., Xiao, N., Cai, Y., Yang, Q., Yu, L., Chen, Z., et al. (2023). CRISPR-Cas9-mediated editing of the OsHPPD 3′ UTR confers enhanced resistance to HPPD-inhibiting herbicides in rice. Plant Commun. 4, 100605. doi:10.1016/j.xplc.2023.100605
Xie, J., Fei, X., Yan, Q., Jiang, T., Li, Z., Chen, H., et al. (2024a). The C4 photosynthesis bifunctional enzymes, PDRPs, of maize are co-opted to cytoplasmic viral replication complexes to promote infection of a prevalent potyvirus sugarcane mosaic virus. Plant Biotechnol. J. 22, 1812–1832. doi:10.1111/pbi.14304
Xie, Y., Zhao, Y., Chen, L., Wang, Y., Xue, W., Kong, D., et al. (2024b). ZmELF3.1 integrates the RA2-TSH4 module to repress maize tassel branching. New Phytol. 241, 490–503. doi:10.1111/nph.19329
Xie, Z., Sun, Y., Zhan, C., Qu, C., Jin, N., Gu, X., et al. (2024c). The E3 ligase OsPUB33 controls rice grain size and weight by regulating the OsNAC120-BG1 module. Plant Cell 37 (1), koae297. doi:10.1093/plcell/koae297
Xiong, D., Wang, R., Wang, Y., Li, Y., Sun, G., and Yao, S. (2023). SLG2 specifically regulates grain width through WOX11-mediated cell expansion control in rice. Plant Biotechnol. J. 21 (9), 1904–1918. doi:10.1111/pbi.14102
Xu, H., Yang, X., Zhang, Y., Wang, H., Wu, S., Zhang, Z., et al. (2022). CRISPR/Cas9-mediated mutation in auxin efflux carrier OsPIN9 confers chilling tolerance by modulating reactive oxygen species homeostasis in rice. Front. Plant Sci. 13, 967031. doi:10.3389/fpls.2022.967031
Xu, R., Li, Y., Sui, Z., Lan, T., Song, W., Zhang, M., et al. (2021). A C-terminal encoded peptide, ZmCEP1, is essential for kernel development in maize. J. Exp. Bot. 72, 5390–5406. doi:10.1093/jxb/erab224
Yamori, W., Nakazato, I., Qu, Y., Sanga, Y., Miyata, T., Uehara, R., et al. (2025). Chloroplast genome editing of Rubisco boosts photosynthesis and plant growth [Preprint]. Available online at: https://www.biorxiv.org/content/10.1101/2025.01.02.631008v1.full (Accessed January 29, 2025).
Yan, F., Wang, W., and Zhang, J. (2019). CRISPR-Cas12 and Cas13: the lesser known siblings of CRISPR-Cas9. Cell Biol. Toxicol. 35, 489–492. doi:10.1007/s10565-019-09489-1
Yan, Z., Li, K., Li, Y., Wang, W., Leng, B., Yao, G., et al. (2023). The ZmbHLH32-ZmIAA9-ZmARF1 module regulates salt tolerance in maize. Int. J. Biol. Macromol. 253, 126978. doi:10.1016/j.ijbiomac.2023.126978
Yang, C. h., Zhang, Y., and Huang, C. f. (2019). Reduction in cadmium accumulation in japonica rice grains by CRISPR/Cas9-mediated editing of OsNRAMP5. J. Integr. Agric. 18, 688–697. doi:10.1016/S2095-3119(18)61904-5
Yang, R. S., Xu, F., Wang, Y. M., Zhong, W. S., Dong, L., Shi, Y. N., et al. (2021). Glutaredoxins regulate maize inflorescence meristem development via redox control of TGA transcriptional activity. Nat. Plants 7, 1589–1601. doi:10.1038/s41477-021-01029-2
Yang, Y., Zhang, T., Shi, Y., Lu, Y., Li, Q., Fan, X., et al. (2024). Introgression of lac6/tl1/du13 improves the palatability of japonica rice. Crop J. 12 (4), 1259–1265. doi:10.1016/j.cj.2024.06.006
Yang, Y., Zhang, Y., Sun, Z., Shen, Z., Li, Y., Guo, Y., et al. (2023). Knocking out OsAAP11 to improve rice grain quality using CRISPR/Cas9 system. Int. J. Mol. Sci. 24, 14360. doi:10.3390/ijms241814360
Yassitepe, J. E. C. T., da Silva, V. C. H., Hernandes-Lopes, J., Dante, R. A., Gerhardt, I. R., Fernandes, F. R., et al. (2021). Maize transformation: from plant material to the release of genetically modified and edited varieties. Front. Plant Sci. 12, 766702. doi:10.3389/fpls.2021.766702
Yu, W., Wang, L., Zhao, R., Sheng, J., Zhang, S., Li, R., et al. (2019). Knockout of SlMAPK3 enhances tolerance to heat stress involving ROS homeostasis in tomato plants. BMC Plant Biol. 19, 354. doi:10.1186/s12870-019-1939-z
Yue, E., Cao, H., and Liu, B. (2020). OsmiR535, a potential genetic editing target for drought and salinity stress tolerance in Oryza sativa. Plants 9, 1337. doi:10.3390/plants9101337
Yuyu, C., Aike, Z., Pao, X., Xiaoxia, W., Yongrun, C., Beifang, W., et al. (2020). Effects of GS3 and GL3.1 for grain size editing by CRISPR/Cas9 in rice. Rice Sci. 27, 405–413. doi:10.1016/j.rsci.2019.12.010
Zafar, K., Khan, M. Z., Amin, I., Mukhtar, Z., Zafar, M., and Mansoor, S. (2023). Employing template-directed CRISPR-based editing of the OsALS gene to create herbicide tolerance in Basmati rice. AoBP 15 (2), plac059. doi:10.1093/aobpla/plac059
Zafar, S. A., Zaidi, S. S. E. A., Gaba, Y., Singla-Pareek, S. L., Dhankher, O. P., Li, X., et al. (2020). Engineering abiotic stress tolerance via CRISPR/Cas-mediated genome editing. J. Exp. Bot. 71, 470–479. doi:10.1093/jxb/erz476
Zandalinas, S. I., Balfagón, D., Arbona, V., and Gómez-Cadenas, A. (2017). Modulation of antioxidant defense system is associated with combined drought and heat stress tolerance in citrus. Front. Plant Sci. 8, 953. doi:10.3389/fpls.2017.00953
Zeng, Y., Wen, J., Zhao, W., Wang, Q., and Huang, W. (2020). Rational improvement of rice yield and cold tolerance by editing the three genes OsPIN5b, GS3, and OsMYB30 with the CRISPR–cas9 system. Front. Plant Sci. 10, 1663. doi:10.3389/fpls.2019.01663
Zhang, A., Liu, Y., Wang, F., Li, T., Chen, Z., Kong, D., et al. (2019a). Enhanced rice salinity tolerance via CRISPR/Cas9-targeted mutagenesis of the OsRR22 gene. Mol. Breed. 39, 47. doi:10.1007/s11032-019-0954-y
Zhang, C., Yun, P., Xia, J., Zhou, K., Wang, L., Zhang, J., et al. (2023a). CRISPR/Cas9-mediated editing of Wx and BADH2 genes created glutinous and aromatic two-line hybrid rice. Mol. Breed. 43, 24. doi:10.1007/s11032-023-01368-2
Zhang, J., Augustine, R. C., Suzuki, M., Feng, J., Char, S. N., Yang, B., et al. (2021a). The SUMO ligase MMS21 profoundly influences maize development through its impact on genome activity and stability. PLOS Genet. 17, e1009830. doi:10.1371/journal.pgen.1009830
Zhang, J., Zhang, H., Li, S., Li, J., Yan, L., and Xia, L. (2021b). Increasing yield potential through manipulating of an ARE1 ortholog related to nitrogen use efficiency in wheat by CRISPR/Cas9. J. Integr. Plant Biol. 63, 1649–1663. doi:10.1111/jipb.13151
Zhang, Q., Tian, S., Chen, G., Tang, Q., Zhang, Y., Fleming, A. J., et al. (2023b). A regulatory circuit involving the NADH dehydrogenase-like complex balances C4 photosynthetic carbon flow and cellular redox in maize. Available online at: https://www.biorxiv.org/content/10.1101/2023.02.23.529632v1 (Accessed October 15, 2024).
Zhang, S., Zhang, R., Gao, J., Song, G., Li, J., Li, W., et al. (2021c). CRISPR/Cas9-mediated genome editing for wheat grain quality improvement. Plant Biotechnol. J. 19, 1684–1686. doi:10.1111/pbi.13647
Zhang, W., Wang, R., Kong, D., Peng, F., Chen, M., Zeng, W., et al. (2023c). Precise and heritable gene targeting in rice using a sequential transformation strategy. Cell Rep. Methods. 3, 100389. doi:10.1016/j.crmeth.2022.100389
Zhang, Y., Iaffaldano, B., and Qi, Y. (2021d). CRISPR ribonucleoprotein-mediated genetic engineering in plants. Plant Commun. 2, 100168. doi:10.1016/j.xplc.2021.100168
Zhang, Y., Liang, Z., Zong, Y., Wang, Y., Liu, J., Chen, K., et al. (2016). Efficient and transgene-free genome editing in wheat through transient expression of CRISPR/Cas9 DNA or RNA. Nat. Commun. 7, 12617. doi:10.1038/ncomms12617
Zhang, Y., Lin, X. F., Li, L., Piao, R. H., Wu, S., Song, A., et al. (2024). CRISPR/Cas9-mediated knockout of Bsr-d1 enhances the blast resistance of rice in Northeast China. Plant Cell Rep. 43, 100. doi:10.1007/s00299-024-03192-0
Zhang, Y. M., Yu, H. X., Ye, W. W., Shan, J. X., Dong, N. Q., Guo, T., et al. (2021e). A rice QTL GS3.1 regulates grain size through metabolic-flux distribution between flavonoid and lignin metabolons without affecting stress tolerance. Commun. Biol. 4, 1171. doi:10.1038/s42003-021-02686-x
Zhang, Z., Hua, L., Gupta, A., Tricoli, D., Edwards, K. J., Yang, B., et al. (2019b). Development of an Agrobacterium-delivered CRISPR/Cas9 system for wheat genome editing. Plant Biotechnol. J. 17, 1623–1635. doi:10.1111/pbi.13088
Zhang, Z., Zhang, X., Lin, Z., Wang, J., Liu, H., Zhou, L., et al. (2020). A large transposon insertion in the stiff1 promoter increases stalk strength in maize. Plant Cell 32, 152–165. doi:10.1105/tpc.19.00486
Zhao, C., Piao, S., Wang, X., Huang, Y., Ciais, P., Elliott, J., et al. (2016). Plausible rice yield losses under future climate warming. Nat. Plants 3, 16202. doi:10.1038/nplants.2016.202
Zhao, H., Qin, Y., Xiao, Z., Li, Q., Yang, N., Pan, Z., et al. (2020). Loss of function of an RNA polymerase III subunit leads to impaired maize kernel development. Plant Physiol. 184, 359–373. doi:10.1104/pp.20.00502
Zhao, L., Koseki, S. R. T., Silverstein, R. A., Amrani, N., Peng, C., Kramme, C., et al. (2023). PAM-flexible genome editing with an engineered chimeric Cas9. Nat. Commun. 14 (1), 6175. doi:10.1038/s41467-023-41829-y
Zhao, Y., Huang, Z., Zhou, X., Teng, W., Liu, Z., Wang, W., et al. (2025). Precise deletion, replacement and inversion of large DNA fragments in plants using dual prime editing. Nat. Plants 11, 191–205. doi:10.1038/s41477-024-01898-3
Zheng, M., Lin, J., Liu, X., Chu, W., Li, J., Gao, Y., et al. (2021a). Histone acetyltransferase TaHAG1 acts as a crucial regulator to strengthen salt tolerance of hexaploid wheat. Plant Physiol. 186, 1951–1969. doi:10.1093/plphys/kiab187
Zheng, S., Ye, C., Lu, J., Liufu, J., Lin, L., Dong, Z., et al. (2021b). Improving the rice photosynthetic efficiency and yield by editing OsHXK1 via CRISPR/Cas9 system. Int. J. Mol. Sci. 22, 9554. doi:10.3390/ijms22179554
Zheng, X., Zhang, S., Liang, Y., Zhang, R., Liu, L., Qin, P., et al. (2023). Loss-function mutants of OsCKX gene family based on CRISPR-Cas systems revealed their diversified roles in rice. Plant Genom 16, e20283. doi:10.1002/tpg2.20283
Zhong, D., Pan, H., Li, K., Zhou, Y., Zhao, F., Ye, L., et al. (2024a). Targeted A-to-T and A-to-C base replacement in maize using an optimized adenine base editor. Plant Biotechnol. J. 22 (3), 541–543. doi:10.1111/pbi.14256
Zhong, T., Zhu, M., Zhang, Q., Zhang, Y., Deng, S., Guo, C., et al. (2024b). The ZmWAKL–ZmWIK–ZmBLK1–ZmRBOH4 module provides quantitative resistance to gray leaf spot in maize. Nat. Genet. 56, 315–326. doi:10.1038/s41588-023-01644-z
Zhou, J., Zhang, R., Jia, X., Tang, X., Guo, Y., Yang, H., et al. (2024a). CRISPR-Cas9 mediated OsMIR168a knockout reveals its pleiotropy in rice. Plant Biotechnol. J. 20, 310–322. doi:10.1111/pbi.13713
Zhou, S., Cai, L., Wu, H., Wang, B., Gu, B., Cui, S., et al. (2024b). Fine-tuning rice heading date through multiplex editing of the regulatory regions of key genes by CRISPR-Cas9. Plant Biotechnol. J. 22, 751–758. doi:10.1111/pbi.14221
Zhou, Y., Xu, S., Jiang, N., Zhao, X., Bai, Z., Liu, J., et al. (2022). Engineering of rice varieties with enhanced resistances to both blast and bacterial blight diseases via CRISPR/Cas9. Plant Biotechnol. J. 20, 876–885. doi:10.1111/pbi.13766
Zhu, Y., Lin, Y., Fan, Y., Wang, Y., Li, P., Xiong, J., et al. (2023). CRISPR/Cas9-mediated restoration of Tamyb10 to create pre-harvest sprouting-resistant red wheat. Plant Biotechnol. J. 21, 665–667. doi:10.1111/pbi.13981
Zörb, C., Geilfus, C. M., and Dietz, K. J. (2019). Salinity and crop yield. Plant Biol. 21, 31–38. doi:10.1111/plb.12884
Zougmoré, R. B., Partey, S. T., Ouédraogo, M., Torquebiau, E., and Campbell, B. M. (2018). Facing climate variability in sub-Saharan Africa: analysis of climate-smart agriculture opportunities to manage climate-related risks. Cah. Agric. 27, 1. doi:10.1051/cagri/2018019
BADH2 Betaine Aldehyde Dehydrogenase 2
BE Base editing
CO2 Carbon dioxide
CRISPR/Cas Clustered Regularly Interspaced Short Palindromic Repeats and CRISPR-Associated System
DST Drought and salt tolerance gene
ERA1 Enhanced Response to ABA1 gene
FAO Food and Agriculture Organization
GBSS Granule-bound starch synthase gene
GlutEnSeq Gluten gene Enrichment and Sequencing
GM Genetically Modified
GMOs Genetically Modified Organisms
gRNA Guide RNA
GSR Gibberella stalk rot
HAG1 Histone acetyltransferase gene
HSFA6e Heat shock transcription factor gene
ICT Intragenic, transgenic, and cisgenic approaches
IPA Ideal Plant Architecture 1 gene
IPK1 Inositol Pentakisphosphate 2-Kinase 1 gene
IUCN International Union for the Conservation of Nature
MLO Mildew resistance locus O
PAM Protospacer Adjacent Motif
PFT1 Phytochrome and flowering time 1 gene
PINb Puroindoline b gene
PPO Polyphenol oxidase gene
PSY Phytoene synthase gene
PYR1 Pyrabactin resistance 1 gene
RNAi RNA interference
RNP Ribonucleoprotein
S genes Susceptible genes
S5H Salicylic acid 5-hydroxylase genes
SBEIIa Starch-branching enzyme (SBE) II gene
SIAGL6 Slagamous-Like 6 gene
SlMAPK 3 Mitogen-activated protein kinase gene
TALENs Transcription activator-like effector nucleases
T-FACE Temperature-free air CO2 enrichment systems
TFs Transcription factors
TMT3B Tonoplast monosaccharide transporter 3 gene
TRM TONNEAU 1-recruiting motif
TRXH9 Thioredoxin gene
USA United States of America
WSSMV Wheat spindle streak mosaic virus
WYMV Wheat yellow mosaic virus
ZFNs Zinc finger nucleases
Keywords: climate-smart crops, food security, genome editing, maize, rice, wheat
Citation: Kaur N, Qadir M, Francis DV, Alok A, Tiwari S and Ahmed ZFR (2025) CRISPR/Cas9: a sustainable technology to enhance climate resilience in major Staple Crops. Front. Genome Ed. 7:1533197. doi: 10.3389/fgeed.2025.1533197
Received: 23 November 2024; Accepted: 27 February 2025;
Published: 18 March 2025.
Edited by:
Koppolu Raja Rajesh Kumar, Indira Gandhi National Tribal University, IndiaReviewed by:
Rushil Ramesh Mandlik, Tamil Nadu Agricultural University, IndiaCopyright © 2025 Kaur, Qadir, Francis, Alok, Tiwari and Ahmed. This is an open-access article distributed under the terms of the Creative Commons Attribution License (CC BY). The use, distribution or reproduction in other forums is permitted, provided the original author(s) and the copyright owner(s) are credited and that the original publication in this journal is cited, in accordance with accepted academic practice. No use, distribution or reproduction is permitted which does not comply with these terms.
*Correspondence: Zienab F. R. Ahmed, emllbmFiLmFobWVkQHVhZXUuYWMuYWU=
Disclaimer: All claims expressed in this article are solely those of the authors and do not necessarily represent those of their affiliated organizations, or those of the publisher, the editors and the reviewers. Any product that may be evaluated in this article or claim that may be made by its manufacturer is not guaranteed or endorsed by the publisher.
Research integrity at Frontiers
Learn more about the work of our research integrity team to safeguard the quality of each article we publish.