- 1Faculty of Animal Science and Food Engineering, (FZEA), University of São Paulo, São Paulo, Brazil
- 2Animal Science Department, Luiz de Queiroz College of Agriculture (ESALQ), University of São Paulo, Piracicaba, Brazil
- 3Department of Animal Science, College of Veterinary Medicine and Animal Science, Goiânia, Brazil
- 4Unit of Animal Genomics, GIGA Medical Genomics, University of Liège, Liège, Brazil
- 5Embrapa Pecuária Sudeste, São Carlos, Brazil
- 6Animal Science Department, Iowa State University, Ames, IA, United States
- 7AL Rae Centre for Genetics and Breeding, Massey University, Hamilton, New Zealand
- 8College of Veterinary Medicine and Animal Science, University of São Paulo, Pirassununga, Brazil
Pigs (Sus scrofa) are an animal model for metabolic diseases in humans. Pork is an important source of fatty acids (FAs) in the human diet, as it is one of the most consumed meats worldwide. The effects of dietary inclusion of oils such as canola, fish, and soybean oils on pig gene expression are mostly unknown. Our objective was to evaluate FA composition, identify changes in gene expression in the liver of male pigs fed diets enriched with different FA profiles, and identify impacted metabolic pathways and gene networks to enlighten the biological mechanisms’ variation. Large White male pigs were randomly allocated to one of three diets with 18 pigs in each; all diets comprised a base of corn and soybean meal to which either 3% of soybean oil (SOY), 3% canola oil (CO), or 3% fish oil (FO) was added for a 98-day trial during the growing and finishing phases. RNA sequencing was performed on the liver samples of each animal by Illumina technology for differential gene expression analyses, using the R package DESeq2. The diets modified the FA profile, mainly in relation to polyunsaturated and saturated FAs. Comparing SOY vs. FO, 143 differentially expressed genes (DEGs) were identified as being associated with metabolism, metabolic and neurodegenerative disease pathways, inflammatory processes, and immune response networks. Comparing CO vs. SOY, 148 DEGs were identified, with pathways related to FA oxidation, regulation of lipid metabolism, and metabolic and neurodegenerative diseases. Our results help explain the behavior of genes with differential expression in metabolic pathways resulting from feeding different types of oils in pig diets.
1 Introduction
Lipids have a variety of different functions within the body and are a class of molecules that are present in all types of cells (Nakamura and Nara, 2003; Cesar et al., 2016). Different types of vegetable oils affect the FA profile and differ in their cholesterol-lowering ability. For example, vegetable oils can exhibit cholesterol-lowering effects (Saedi et al., 2017). Fatty acids (FAs) are derived from both animal fats, such as tallow and poultry fat, and plant oils, such as canola, flaxseed, sunflower, and corn (Moghadasian and Shahidi, 2017; Gomes et al., 2022).
Pigs (Sus scrofa) produce pork which is one of the most consumed meats worldwide, making them an important livestock species and an important source of protein and FA in the human diet (USDA, 2022). Pork is rich in saturated fatty acids (SFAs), monounsaturated fatty acids (MUFAs), and polyunsaturated fatty acids (PUFAs). Oleic acid, omega-9 (OA, C18:1 cis 9; n-9); linoleic acid, omega-6 (LA, C18:2 cis 9, 12; n-6); and α-linolenic acid, omega-3 (ALA, C18:3 cis-9, 12, 15; n-3) are some of the unsaturated fatty acids that bring benefits to human health (Zhang et al., 2016).
The liver is a central metabolic organ and plays a key role in gene expression regulated by FAs, with PUFAs being the main FA regulators of hepatic lipogenic gene expression (Jump et al., 1993; Mater et al., 1998). In pigs, PUFA synthesis, de novo lipid and cholesterol synthesis, and FA oxidation mainly occur in the liver (Xing et al., 2019). Alterations in the metabolic balance of lipid pathways in the liver and dysregulation of energy control lead to pathological conditions such as diabetes, obesity, and metabolic syndrome (Shimizu et al., 2015; Park and Han, 2018). In addition, some nutrients and hormonal and neuronal signals are responsible for regulating the metabolism of lipids, glucose, and amino acids in the liver (Rui, 2014). However, the effects on pig gene expression of dietary inclusion of different oils such as those present in canola, fish, or soybean oils are mostly unknown. Thus, the findings of this study could help to better understand the impact of the different dietary FAs on the liver transcriptome of pigs as an animal model and their impact on biological processes such as metabolic pathways related to lipid metabolism.
PUFA, ALA, docosahexaenoic acid (DHA), and eicosapentaenoic acid (EPA) are essential FAs for human physiology, as the intake of these FAs is related to a lower risk of stroke and coronary heart disease (Jakobsen et al., 2009; Baker et al., 2016; Moghadasian and Shahidi, 2017). PUFAs are responsible for the activity of the immune system and are related to the regulation of eicosanoid synthesis (Szostak et al., 2016). MUFAs, which are present in olive and canola oils, are synthesized in the human body and help in the prevention of cardiovascular diseases (Guasch-Ferré et al., 2014; Moghadasian and Shahidi, 2017).
Understanding the transcriptome of organisms and their tissues is revolutionized by the RNA-sequencing technique (RNA-Seq), which provides levels of transcripts and their isoforms more precisely than other methods (Wang et al., 2009). Pigs are considered model animals for scientific studies on humans because some aspects of their physiology are very similar to those of humans (Groenen et al., 2012). The present study evaluated the differential expression in the liver of pigs fed diets containing different FA profiles and identified the main metabolic pathways impacted.
2 Materials and methods
2.1 Ethics approval
All experimental procedures involving animals are in accordance with the requirements of the Animal Care and Use Committee of Luiz de Queiroz College of Agriculture (University of São Paulo, Piracicaba, Brazil; protocol: 2018.5.1787.11.6 and number CEUA 2018-28) and follow ethical principles in animal research, according to the Guide for the Care and Use of Agricultural Animals in Agricultural Research and Teaching (Fass, 2010).
2.2 Animals and sample collection
In this study, we selected 54 purebred Large White male pigs. The sires and dams that originated the population were of the Large White breed. We used three sires and 32 dams to keep inbreeding below 14%. The animals were genotyped for the halothane mutation (RYR1 gene) according to Fujii et al. (1991), and all halothane homozygous negative (NN) were selected for this. The animals at 71 ± 1.8 days of age were allocated to one of three dietary treatments in a design with six replicate pens per treatment and three pigs per pen. Each pen was equipped with a three-hole dry self-feeder and a nipple drinker, which allowed ad libitum access to food and water throughout the experimental period. All the pigs were immunocastrated through the administration of two 2-mL doses of Vivax® (Pfizer Animal Health, Parkville, Australia) on days 56 and 70 of the experiment (Almeida et al., 2021), in accordance with the manufacturer’s recommendations.
The experimental diets consisted of corn–soybean meal growing–finishing diets, supplemented with either 3% soybean oil (SOY), 3% canola oil (CO), or 3% fish oil (FO) and were modified according to the growth and finishing phases. Canola oil is an important source of OA, and SO is rich in LA, while FO is an important source of DHA (C22:6; n-3) and EPA (C20:5; n-3) (Almeida et al., 2021). The diets were formulated to have similar metabolizable energy content (3.36 Mcal/kg) and were formulated to meet or exceed requirements (Rostagno, 2011). The diets were formulated to have a similar level of digestible energy. No antibiotic growth promoters were used, and all diets were provided in a mash meal form; details of the animals and diets of this study are described in Supplementary Tables S1–S4, which were adapted from our previous study (Almeida et al., 2021). The average values for the initial body weights of the animals were 28.78 kg for the SOY group, 29.56 kg for the CO group, and 28.10 kg for the FO group (p-value of 0.86). After 98 days of trial, all pigs were slaughtered, with an average final body weight of 132.13 kg (CO group), 136.63 kg (SOY group), and 133 kg (FO group) (p-value of 0.09). The right lobe of the liver samples were collected and quickly excised, snap-frozen in liquid nitrogen, and then stored at −80°C until further analyses (Almeida et al., 2021).
2.3 Fatty acid profile
The FA profile determination was performed from the total lipid isolated from 100 g of the liver samples, and a more complete description of the analyses can be found in Almeida et al. (2021). Briefly, FA were determined using the cold extraction method (Bligh and Dyer, 1959) in accordance with the procedure outlined by AOCS (2004; Method AM 5-04).
2.4 Statistical analyses
Statistical analyses to detect differences in the FA profile of the liver between the diets were performed using the MIXED procedure of the SAS statistical software (SAS Institute Inc., Cary, NC, v. 9.4), where a mixed model was fit using restricted maximum likelihood (REML) methodology. In the model, the block effects were fit as random effects and the dietary treatments as fixed effects. The UNIVARIATE procedure (v. 9.4) was used to test for departure from a normal distribution with homogeneity of residuals for each of the variables. Diagnostics of the density distribution of the studentized residual of the model were made using the Shapiro–Wilk test (SAS v. 9.4). Differences were declared significant based on the Tukey test when p-values ≤0.05.
2.5 RNA extraction, library preparation, and sequencing
Total RNA was extracted from tissue samples using the RNeasy® Mini Kit (QIAGEN, Hilden, Germany) according to the manufacturer’s instructions. Total RNA quantification, purity, and integrity were evaluated by the NanoDrop 1000 and Bioanalyzer. RNA integrity numbers (RINs) of the samples were between 7.8 and 10.0 (Supplementary Table S5). All samples presented an RIN greater than or equal to 7.8. From the total RNA of each sample, 2 µg was used for library preparation according to the protocol described in the TruSeq RNA Sample Preparation Kit v2 Guide (Illumina, San Diego, CA). The Agilent Bioanalyzer 2100 (Agilent, Santa Clara, CA, United States) was used to calculate average library size, and the libraries were quantified using quantitative PCR with the KAPA Library Quantification Kit (KAPA Biosystems, Foster City, CA, United States). Quantified samples were diluted, labeled by barcoding, and pooled to be run in different lanes (five pools of all 36 samples each) using the TruSeq DNA CD Index Plate (96 indexes, 96 samples, Illumina, San Diego, CA, United States). All samples were sequenced across five lanes of a sequencing flow cell using the TruSeq PE Cluster Kit v4-cBot-HS kit (Illumina, San Diego, CA, United States) and were clustered and sequenced using HiSeq 2500 equipment (Illumina, San Diego, CA, United States) with a TruSeq SBS Kit v4-HS (200 cycles) according to the manufacturer’s instructions. All the sequencing analyses were performed at the Genomics Center in the Animal Biotechnology Laboratory at ESALQ of the USP, Piracicaba, São Paulo, Brazil.
2.6 Quality control and alignment
The quality of the raw RNA-Seq reads after trimming was checked with FastQC software version 0.11.8 (http://www.bioinformatics.bbsrc.ac.uk/projects/fastqc/). Subsequently, the sequencing adaptors and low-complexity reads were removed in the initial data-filtering step by Trim Galore 0.6.5 software (https://www.bioinformatics.babraham.ac.uk/projects/trim_galore). Reads with a Phred score higher than 33 and a length higher than 70 nucleotides were kept after trimming. Reads were then aligned with the pig reference genome Sus Scrofa 11.1, which is available at Ensembl [http://www.ensembl.org/Sus_scrofa/Info/Index]. The abundance (read counts) of mRNAs for all annotated genes was calculated using STAR-2.7.6a (Dobin and Gingeras, 2015; Fanalli et al., 2022a; 2022b). Gene expression levels were normalized using counts scaled by the total number of reads (CPM—counts per million) using the R package DESeq2 (Love et al., 2014).
2.7 Differentially expressed genes and functional enrichment analysis
Differentially expressed genes (DEGs) between the pairwise comparisons of different diets (CO vs. SOY, SO vs. FO, and CO vs. FO) were identified using the R package DESeq2, available at Bioconductor open-source software for bioinformatics, using a multi-factor design (Love et al., 2014). Prior to statistical analysis, the read count data were filtered as follows: 1) unexpressed genes were genes with zero counts for all samples; 2) very lowly expressed genes were genes with less than one read per sample on average; and 3) rarely expressed genes were genes that were not present in at least 50% of the samples. Unexpressed, very lowly expressed, and rarely expressed genes were all removed from the analysis. The three sires were used as a fixed factor in the multi-factor model. The cut-off approach performed to identify the DEGs was the methodology of Benjamini and Hochberg (1995), which was used to maintain the false discovery rate (FDR) at 10% (Cesar et al., 2016; Fanalli et al., 2022b). The normalized read counts for the DEG were correlated to the fatty acid content of the liver samples by calculating the Pearson correlation coefficient (Pearson’s r) by using the PROC CORR procedure in SAS.
The functional enrichment analysis was performed using MetaCore software (Clarivate Analytics, 2022) to identify the pathway maps from the list of DEGs. The functional enrichment analysis of DEGs (FDR <0.10) was performed to obtain comparative networks by “analysis of a single experiment” using Homo sapiens genome annotation as the background reference and standard parameters of MetaCore software v. 21.4 build 70700. The filters used were metabolic maps: energy metabolism, lipid metabolism, and steroid metabolism; cardiovascular diseases: atherosclerosis; regulation of metabolism; nutritional and metabolic diseases; and nervous system diseases. For more detailed definitions of pathway maps, see https://portal.genego.com/legends/MetaCoreQuickReferenceGuide.pdf.
3 Results
3.1 Fatty acid profile
The composition of the FA deposited was different between treatments. EPA, DHA, and consequently total n-3 PUFA were higher in the FO group (p-value <0.01) than in the CO and SOY groups with no difference observed between the CO and SOY groups. On the other hand, a higher amount of total MUFA and myristic acid (p-value <0.05) was observed in the CO and SOY groups compared to the FO group. The n-6:n-3 ratio was lower in the FO group than in the CO and SOY groups, with no difference observed between the CO and SOY groups (p-value <0.01). The lowest atherogenic index was observed in the CO group than in the FO and SOY groups, with no difference between the FO and SOY groups, but in reference to the PUFA:SFA ratio (p-value <0.05), CO and FO showed higher percentages of tissue deposition than the SOY group. The lowest concentration of total PUFA was identified in the SOY group than in the FO and CO groups with no difference between the FO and CO groups (p-value <0.01). Concentrations of palmitic acid, stearic acid, palmitoleic acid, LA, ALA, SFA, and total n-6 PUFA were similar among all diets (see Table 1).
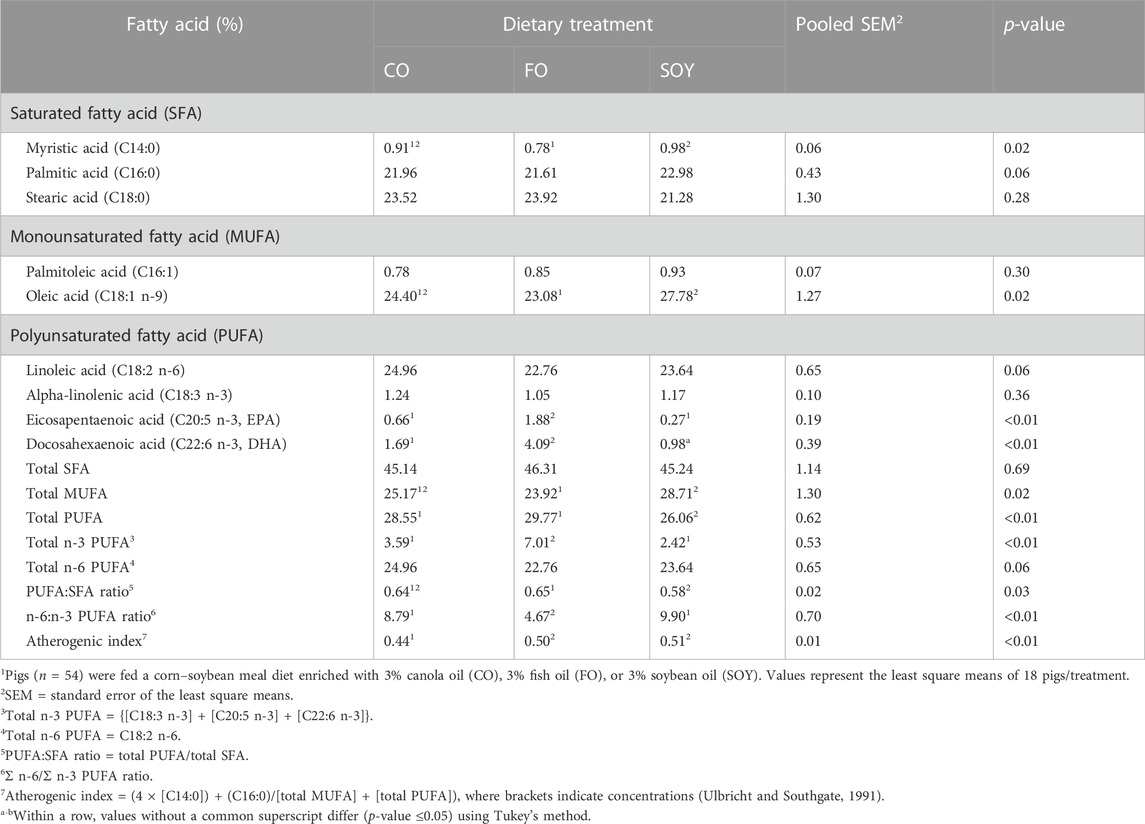
TABLE 1. Effects of dietary treatments on the fatty acid profile deposited in the liver of male pigs.
3.2 Sequencing data and differential expression analysis
The average numbers of total reads sequenced from the liver samples before and after filtering for the CO group were 35,201,462 and 34,736,732; for the soybean oil (SOY) group, 34,078,903 and 33,610,858; and for the FO group, 34,296,605 and 33,801,914, respectively. General mapping statistics are shown in Supplementary Table S5.
A total of 148 DEGs (FDR <0.10) were identified in the CO vs. SOY comparison, where 108 were downregulated (log2-fold change ranging from −4.71 to −0.29) and 40 were upregulated (log2-fold change ranging from 0.24 to 2.36) in the CO group compared to the SOY group (Table 2). For the SOY vs. FO comparison, 143 DEGs (FDR <0.10) were identified, where 87 were downregulated (log2-fold change ranging from −3.94 to −0.20) and 56 were upregulated (log2-fold change ranging from 0.28 to 1.94) in the SOY group compared to the FO group (Table 2). The CO vs. FO comparison did not show any DEG. Supplementary Table S6 presents genes and the log2-fold change found between the comparisons. Supplementary Figure S1 shows the volcano plot of log2-fold change (x-axis) vs. −log10FDR-corrected p-value (y-axis) from the DEG analysis for the liver tissue (A) CO vs. SOY and (B) SOY vs. FO.
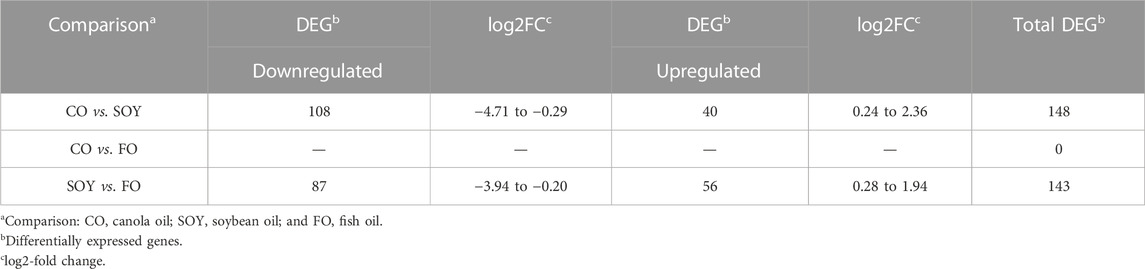
TABLE 2. Differentially expressed genes found in the liver tissue of pigs fed with different oils in the diet.
We identified 26 common genes between CO vs. SOY and SOY vs. FO (Figure 1; Table 3). Among them, cytochrome P450 family 7 subfamily A member 1 (CYP7A1; log2-fold change −2.77) was downregulated in the CO and SOY groups. On the other hand, phospholipase A2 group IID (PLA2G2D; log2-fold change +1.65) was upregulated in the CO and SOY groups.
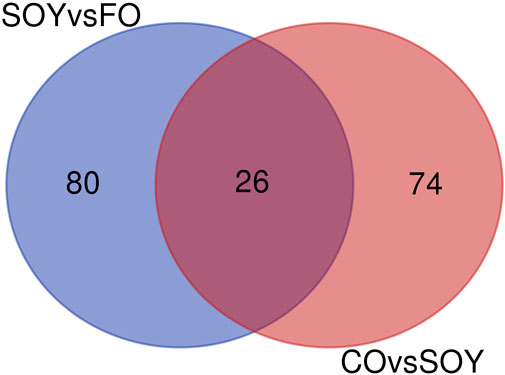
FIGURE 1. Venn diagram of the comparison of 3.0% soybean oil vs. 3.0% fish oil (SOY vs. FO) and 3.0% canola oil vs. soybean oil (CO vs. SOY).
The correlation analysis among the DEG and the fatty acid content of liver samples among the different diets is shown in Supplementary Tables S7, S8, where, in general, the magnitude of the correlation coefficient among gene expression and the fatty acid profile (phenotypes) was moderate to low.
3.3 Functional enrichment analysis for differential expression between CO and SOY
In the enriched signaling pathways (p-value <0.10; Table 4), we identified genes such as mitochondrial-encoded NADH dehydrogenase 5 (ND5 MTND5) and mitochondrial-encoded NADH dehydrogenase 6 (ND6 MTND6) that are present in the “CREB1-dependent transcriptional deregulation pathway in Huntington’s disease” (Supplementary Figure S2).
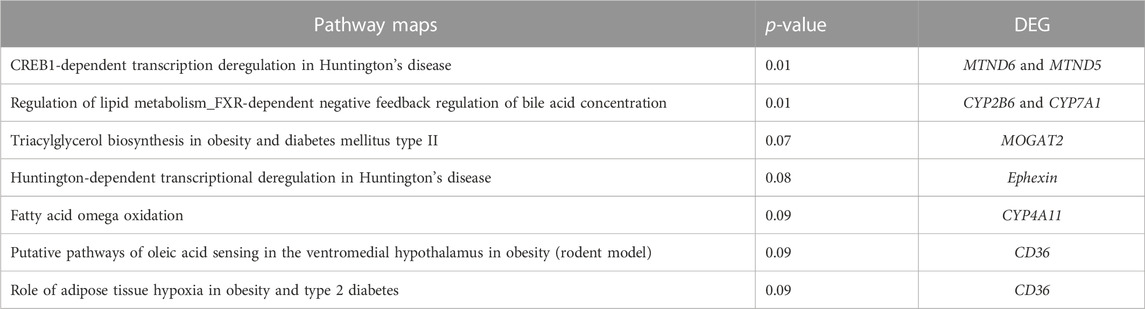
TABLE 4. Pathway maps from the list of differentially expressed genes (FDR 10%) in the liver of male pigs fed with different oil sources found using MetaCore software (p-value <0.10).
The cytochrome P450 family 7 subfamily A member 1 (CYP7A1) and cytochrome P450 family 2 subfamily B member 6 (CYP2B6) are related to the “regulation of lipid metabolism FXR-dependent negative feedback regulation of bile acid concentration” (Figure 2); monoacylglycerol O-acyltransferase 2 (MOGAT2) was found in the “triacylglycerol biosynthesis in obesity and diabetes mellitus type II” pathway (Figure 3); the neuronal guanine nucleotide exchange factor NGEF/Ephexin gene in the “Huntington-dependent transcriptional deregulation pathway in Huntington’s disease” (Supplementary Figure S3); the cytochrome P450 family 4 subfamily A member 11 (CYP4A11) in “fatty acid omega oxidation” (Supplementary Figure S4); and the cluster of differentiation 36 (CD36) that participates in “putative pathways of oleic acid sensing in the ventromedial hypothalamus in obesity (rodent model)” (Supplementary Figure S5) and the “role of adipose tissue hypoxia in obesity and type 2 diabetes” (Supplementary Figure S6).
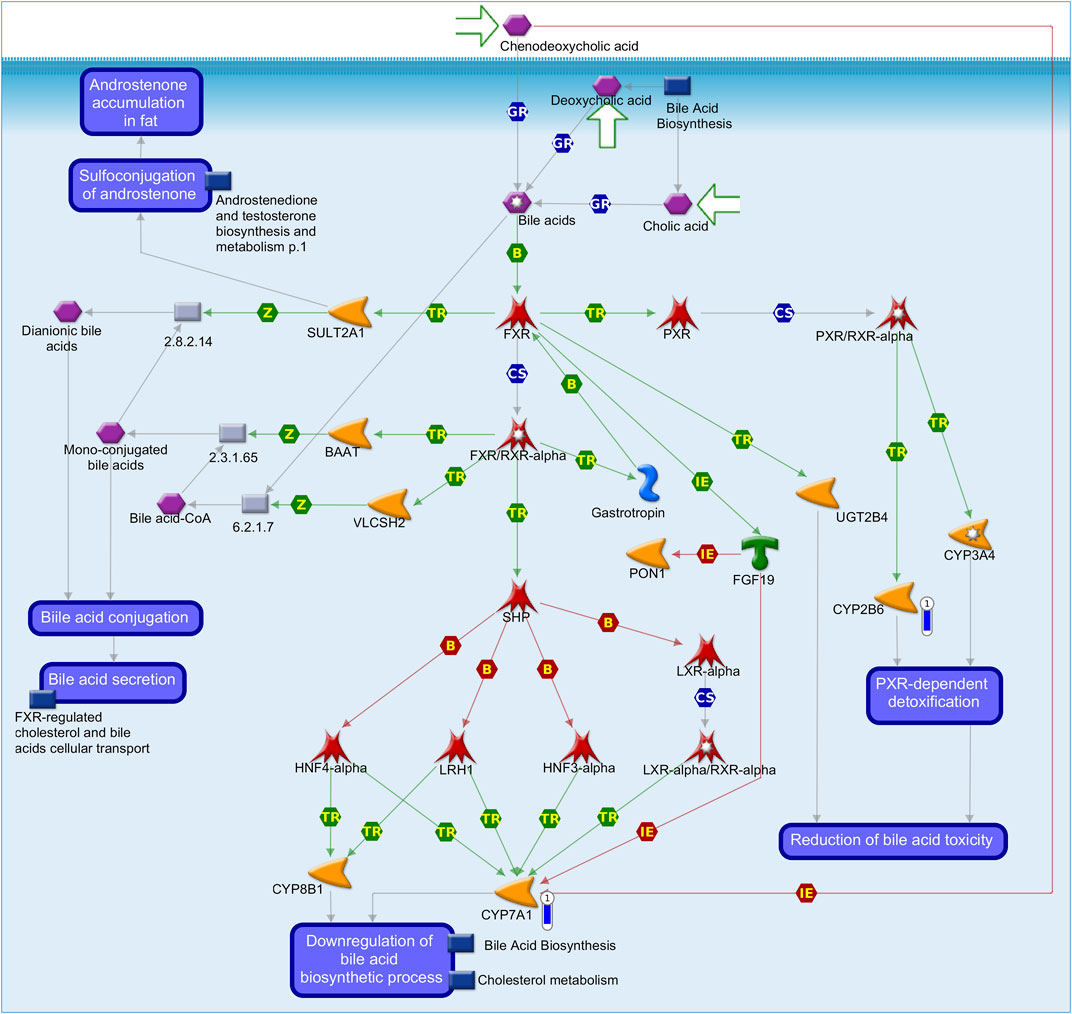
FIGURE 2. Pathway map representing the regulation of lipid metabolism_FXR-dependent negative feedback regulation of bile acid concentration using MetaCore software (p-value <0.10) based on the list of differentially expressed genes (FDR 10%) in the liver tissue of pigs fed with different oils in the diet (3.0% canola oil and 3.0% soybean oil). The blue thermometer indicates that the DEG is downregulated (log2-fold change −2.31 and −2.45) in the diet with 3.0% of canola oil (CO). Green arrows indicate positive interactions, and gray arrows indicate unspecified interactions.
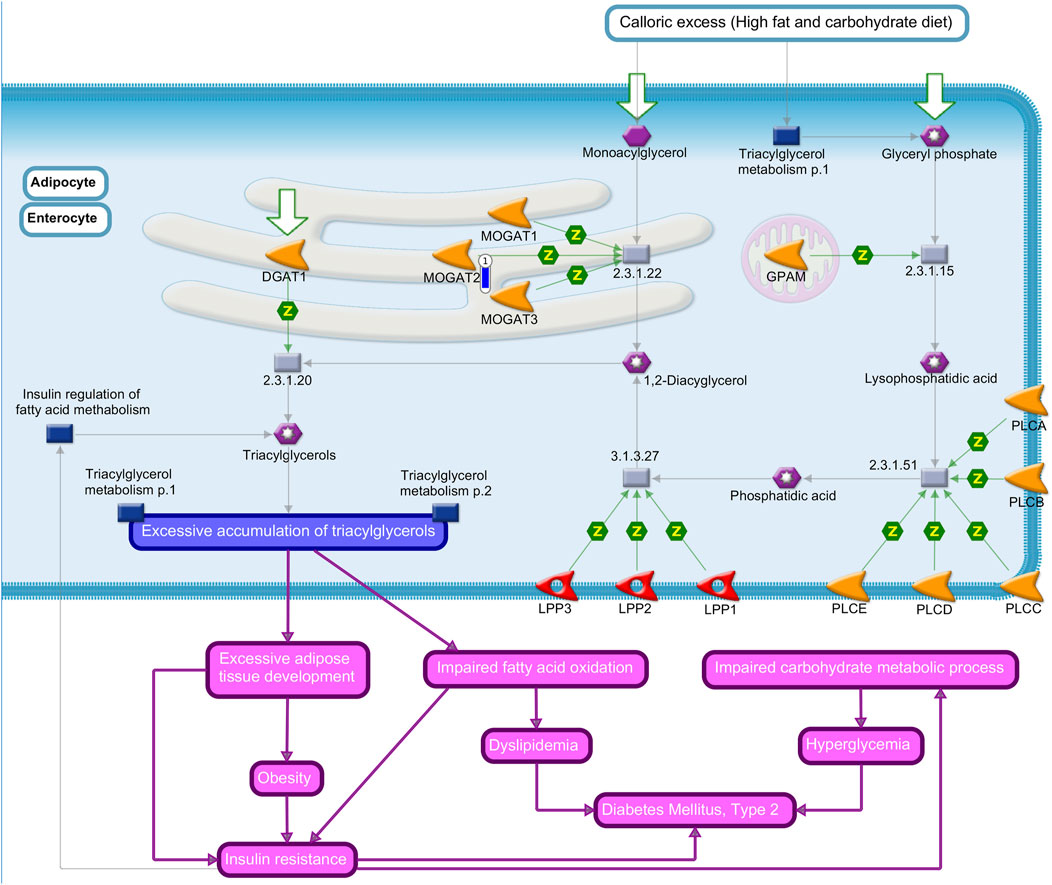
FIGURE 3. Triacylglycerol biosynthesis in obesity and the diabetes mellitus type II pathway map by MetaCore software (p-value <0.10) from the list of differentially expressed genes (FDR 10%) in the liver tissue of pigs fed with different oils in the diet (3.0% canola oil and 3.0% soybean oil). The blue thermometer indicates that the DEG is downregulated (log2-fold change −4.72) in the diet with 3.0% of CO. Purple lines indicate increases in diseases. Green arrows indicate positive interactions, and gray arrows indicate unspecified interactions.
Herein, they were identified as DEGs in the liver of pigs fed CO vs. SOY with lower expression (log2-fold change −2.31 and −2.45) in pigs of the CO group and enriched in the “lipid metabolism FXR-dependent negative feedback regulation of bile acid concentration” pathway.
MOGAT2 was identified as the lowest expressed gene (log2-fold change −4.72) in pigs of the CO group present in the “triacylglycerol biosynthesis in obesity and diabetes mellitus type II” (Figure 2).
CYP4A11 exhibited lower expression in the diet containing CO (log2-fold change −1.25) than in the SOY diet and was enriched in the “fatty acid omega oxidation” pathway.
NGEF was identified as a downregulated gene (log2-fold change −0.6) in the CO group. The NGEF appears as Ephexin in the “Huntington-dependent transcriptional deregulation in Huntington’s disease” pathway. The CD36 gene was identified as having the lowest expression (log2-fold change −0.48) in the group receiving a diet with CO and being enriched in the “putative pathways of oleic acid sensing in the ventromedial hypothalamus in obesity (rodent model)” and the “role of adipose tissue hypoxia in obesity and type 2 diabetes” pathways.
To observe the interactions of DEGs in gene networks, an analysis of process networks was performed using MetaCore software (Figure 4). The identified networks were related to inflammatory processes, metabolism, and neuropeptide signaling. Among the networks obtained, we identified (p-value <0.10) the “inflammation_kallikrein–kinin system” (p-value 7.143E-04) in which the DEG kininogen 1 (KNG1) (log2-fold change −0.65) is involved; the “signal transduction_neuropeptide signaling” pathway (p-value 2.230e-2) presented some DEGs in the Galpha(i)-specific peptide GPCR group, such as the neuropeptide Y receptor Y1 (NPY1R) (log2-fold change −1.09) and the “development/regulation of angiogenesis” pathway (p-value 5.475e-2), presenting the DEG SMAD family member 3 (SMAD3) (log2-fold change −0.32) and Galpha(i)-specific peptide GPCR such as NPY1R (log2-fold change −1.09).
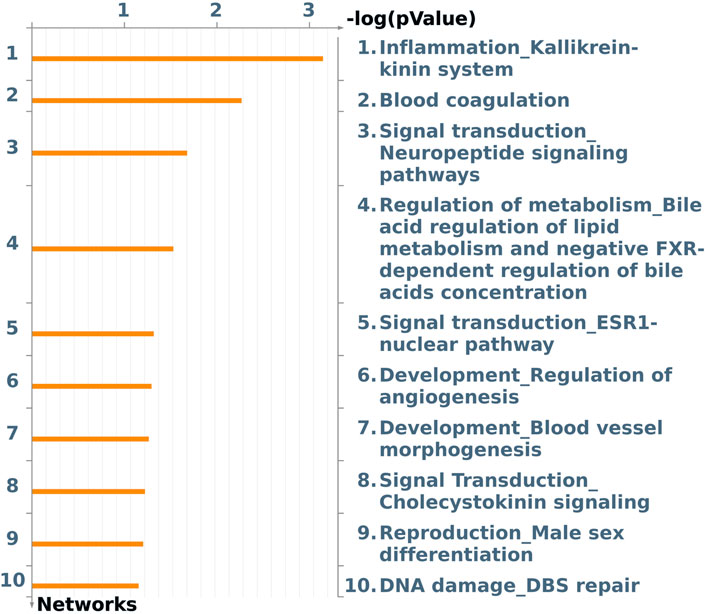
FIGURE 4. Top 10 enriched networks identified using MetaCore software applied to the list of differentially expressed genes (FDR 10%) in the liver tissue of male pigs fed with different oils (3.0% canola oil and 3.0% soybean oil).
3.4 Functional enrichment analysis for differential expression between SOY and FO
The enriched pathway maps (Figure 5) obtained (p-value <0.10) were “regulation of lipid metabolism FXR-dependent negative feedback regulation of bile acid concentration” (Supplementary Figure S7) (p-value 2.500E-03, log2-fold change −2.18 CYP2B6 DEG and CYP7A1 DEG with log2-fold change −2.78); “cholesterol metabolism” (Supplementary Figure S8) (p-value 1.851E-02, log2-fold change −0.38 ACOX2 DEG and CYP7A1); “bile acid biosynthesis” (Supplementary Figure S9) (p-value 2.140E-02, ACOX2 and CYP7A1 DEG); the “ role of inflammasome in macrophages, adipocytes, and pancreatic beta cells in type 2 diabetes” (Supplementary Figure S10) (p-value 4.253E-02, log2-fold change +0.49 IL-18 DEG); and “Huntington-dependent transcriptional deregulation in Huntington’s disease” (Supplementary Figure S11) (p-value 5.632E-02, Ephexin DEG).
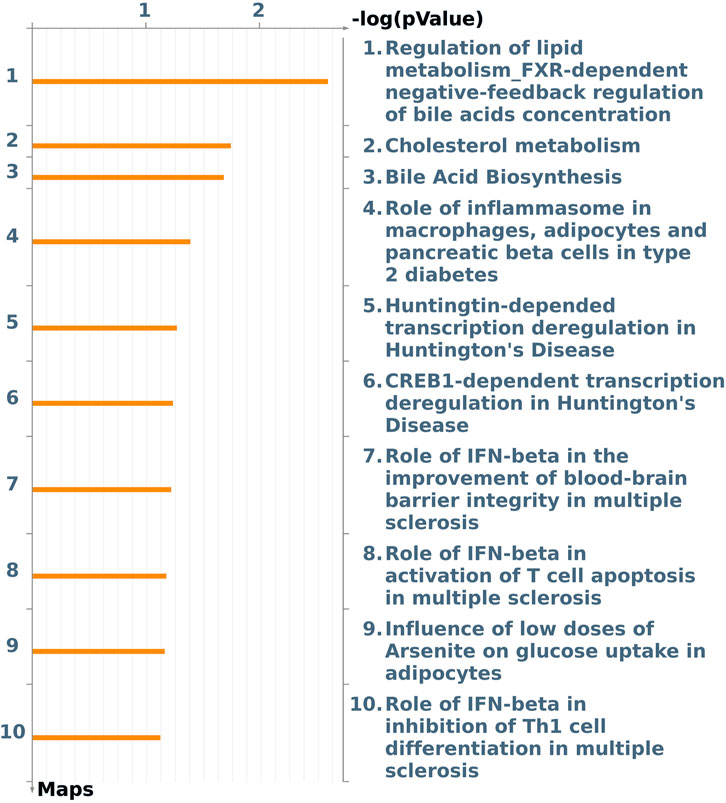
FIGURE 5. Pathway maps from the list of differentially expressed genes (FDR 10%) in the liver of male pigs fed with different oil sources (3.0% soybean oil vs. 3.0% fish oil) detected using MetaCore software (p-value <0.10).
CYP7A1 is enriched in other pathways with the gene acyl-CoA oxidase 2 (ACOX2), which was identified as a DEG and has lower expression in the group fed a diet enriched with soybean oil. The CYP7A1 and ACOX2 DEGs were enriched in “cholesterol metabolism” and “bile acid biosynthesis” pathways, highlighting the importance of their relationship to bile acids.
Interleukin-18 (IL-18) showed higher expression in the liver of pigs fed with the SOY diet and was enriched in the “role of the inflammasome pathway in macrophages, adipocytes, and pancreatic beta cells in type 2 diabetes” (Figure 6). Another enriched pathway that IL-18 is involved in is the “role of IFN-beta in inhibition of Th1 cell differentiation in multiple sclerosis” (Supplementary Figure S12).
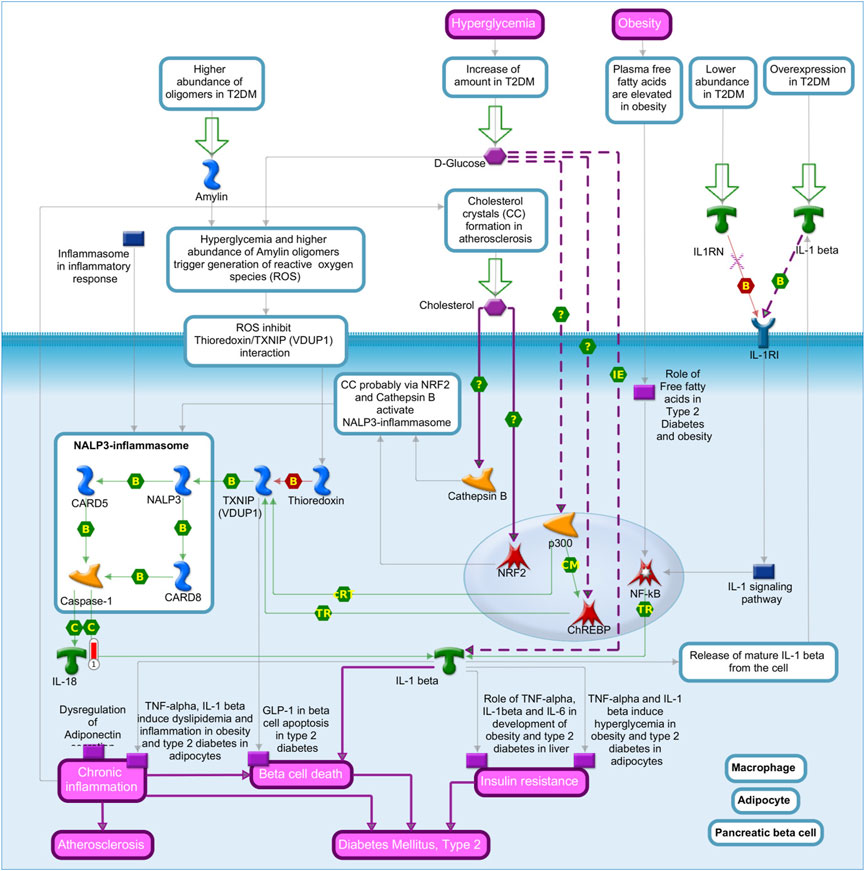
FIGURE 6. Role of the inflammasome in macrophages, adipocytes, and pancreatic beta cells in the type 2 diabetes pathway map based on MetaCore software (p-value <0.10) from the list of differentially expressed genes (FDR 10%) in the liver tissue of pigs fed with different oils in the diet (3.0% soybean oil and 3.0% fish oil). The blue thermometer indicates that the DEG is downregulated in the diet with 3.0% soybean oil (SOY). The large green arrows indicate the path to start. Green arrows indicate positive interactions, and gray arrows indicate unspecified interactions. Links in purple indicate emerging and enhanced (dotted) diseases.
Another DEG identified was NGEF which exhibited lower expression (log2-fold change −0.65) in pigs fed with the SOY diet and was enriched within the “Huntington-dependent transcriptional deregulation pathway in Huntington’s disease” as Ephexin. MTND6 presented a lower expression (log2-fold change −0.92) in the SOY group, in addition to being enriched in the “CREB1-dependent transcriptional deregulation pathway in Huntington’s disease”.
Kinesin Family Member 3B (KIF3B) was identified in our study as having a lower expression (log2-fold change −0.53) in the liver of pigs fed with the SOY diet. It participates in the “influence of low doses of arsenite on glucose uptake in adipocytes” pathway.
In addition to these analyses, a process network analysis (Table 5) was performed to assist in the understanding of the enriched pathways through interactions in gene networks that are related to the change of oil in the diet. We highlight here the pathways of “regulation of bile acid metabolism,” “regulation of lipid metabolism,” “negative FXR-dependent regulation of bile acid concentration” and pathways related to signal transduction, sodium transport, and neurophysiological processes.
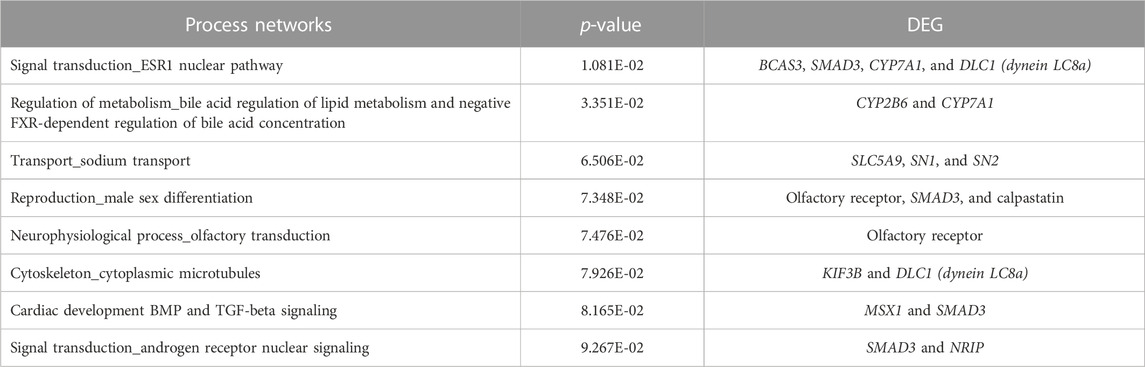
TABLE 5. Process networks from the list of differentially expressed genes (FDR 10%) in the liver tissue of male pigs fed with different oil sources found using MetaCore software (p-value <0.1).
4 Discussion
In this study, we identified the differential expression of genes in the livers of pigs fed diets containing different FA profiles. Despite the similarities in FA composition of CO and SOY diets, for example, OA, EPA, total SFA, and total MUFA compositions, transcriptome differences were found, revealing genes and pathways relevant to lipid processes and diseases. The composition of FA in tissues is not only affected by the ingestion of FA but also by the genotype of the animal, body mass, and mainly, the deposition of FA in the tissues is related to specific rates of deposition, synthesis, and desaturation (Poklukar et al., 2020; Sarri et al., 2022). More studies are needed to understand the incorporation of dietary FA in pig tissues (Sarri et al., 2022). Although the deposition of FA in the different tissues is modulated by the diet, mainly in monogastric species, this deposition is dependent on the interconversions between them, limiting the impact of dietary additions (Wood et al., 2008). The decrease in MUFA deposition has already been reported in pigs to be little affected by dietary concentrations as reviewed by Wood et al. (2008), and de novo fatty acid synthesis may dominate fatty acid profiles in some circumstances.
The correlation analysis showed moderate-to-low Pearson correlation coefficients among normalized read counts and the fatty acid content in liver tissue among the comparisons between the diets. The OA and total MUFA content correlated positively with the NCEH1 gene, and this gene has already been related to lipid metabolism in a muscle transcriptome study (Puig-Oliveras et al., 2014). Because of the fatty acid deposition complexity as phenotype, it involves several genes and biological processes related with so many interactions among genes, so to determine the linear correlation between gene expression and phenotype is still a challenge. Other alternatives to measure his correlation were proposals such as Spearman or Pearson correlation with maximal information component analysis (MICA) combined with the interaction component model or distance correlation as incorporated in weighted gene co-expression network analysis (WGCNA) (Hou et al., 2022). Our results corroborate with previous studies (Darwiche and Struhl, 2020; Hou et al., 2022), where the authors suggested that a co-expression analysis is a better approach to reveal the correlation among the DEGs and fatty acids studied herein.
When comparing the CO vs. SOY diets, we found differentially expressed genes present in a signaling pathway related to CREB1. The TF CREB1 is critical for lipid synthesis and triacylglycerol accumulation (Yao et al., 2020). This TF is involved in the regulation of adipocyte differentiation, lipogenesis, and insulin activity in adipose tissues (Klemm et al., 2001). Heat shock protein 9 of 70 kDa (GRP75) is involved in the delivery of CREB1 to mitochondria. Interruption of CREB1 activity in mitochondria leads to decreased expression of mitochondrial-encoded MTND6 and MTND5, downregulating complex I-dependent mitochondrial respiration and consequent mitochondrial dysfunction and neuronal cell death in Huntington’s disease (Lee et al., 2005). The MTND6 gene may be related to reactive oxygen species (ROS) production and transcriptional regulation of CREB1 directly, in which mitochondrial CREB1 binds to the promoter of MTND6 and promotes its expression (Lee et al., 2005). The NADH-ubiquinone oxidoreductase chain such as ND5 and ND6 has been associated with the development of important roles in liver tissue in purebred Landrace female pigs in high-feed and low-feed efficiency groups. These genes were highly expressed in the liver tissues of both groups (Wang et al., 2022). In humans, there are studies related to MT-ND genes associated with mutations in complex I subunits (Danhelovska et al., 2020). In our experimental conditions, we found the differentially expressed genes ND6 and ND5 that are downregulated in the CO group.
The CYP2B6 and CYP7A1 genes in the CO vs. SOY comparison (both downregulated in the CO group) were enriched in the “regulation of metabolism_bile acid regulation of lipid metabolism and negative FXR-dependent regulation of bile acid concentration” process network, with a lower expression in the CO group. Bile acids in high concentrations are toxic (Jung et al., 2006). The CYP2B6 enzyme is involved in the reduction of bile acid toxicity, and the downregulation of the CYP7A1 enzyme is involved in the decrease in bile acid biosynthesis and is found in the endoplasmic reticulum of hepatocytes (Jung et al., 2006; Guevara-Ramírez et al., 2022). The CYP7A1 protein is of great importance in the metabolism of steroids, cholesterol, and other lipids (Guevara-Ramírez et al., 2022). The liver is the organ that expresses all of the necessary enzymes for the bile acid synthesis. The degradation of cholesterol to form bile acids can be through CYP7A1 by the classical pathway, the main biosynthetic pathway of bile acids in humans or through mitochondrial CYP7A1 by the alternative (acidic) pathway due to the production of acid intermediates (Norlin et al., 2000; Chiang and Ferrell, 2020). In a study evaluating the liver transcriptome in pigs from extreme groups of the intramuscular composition of fatty acids, CYP7A1 was identified as a downregulated DEG in the low (L) group in contrast to the high (H) group, being considered as a candidate gene mainly related to its role in the processes of fatty acid metabolism in pigs in the liver (Ramayo-Caldas et al., 2012). The authors emphasize the importance of studies that identify how different types of FA control gene expression through a direct examination of the effect of each FA on fatty acid composition (Ramayo-Caldas et al., 2012). Szostak et al. (2016) suggested that with an increased contribution of omega-3 fatty acids in the liver, there is an association with negative regulation of lipid metabolism and increased β-oxidation. The authors identified CYP7A1 as a DEG in a study of the effect of an enriched diet based on linseed and rapeseed oil supplementation on the porcine liver transcriptome, corroborating with Ramayo-Caldas et al. (2012) and our study in which a diet with a higher amount of n-3 (CO group) showed downregulated expression of CYP7A1. In addition, PPAR-alpha showed altered expression in our study, being an important indicator of the oxidation-metabolic state and intracellular transport of FA, similar to the findings of Ramayo-Caldas et al. (2012) and Szostak et al. (2016). Other studies have related CYP7A1 to lipid metabolism (Wang et al., 2020; Lyu et al., 2022), which highlights the importance of our study when verifying that the diet with oils modified the expression in pigs. In humans, CYP2B6 is the only hepatic isoform of CYP2B that plays a role in the metabolism of numerous xenobiotic and endobiotic compounds (Heintz et al., 2022). Cholesterol and dietary fats modulate CYP7A1 activity and gene expression in humans, but the exact mechanism by which fatty acids can regulate CYP7A1 expression remains to be elucidated (Fu et al., 2011). Thus, the genes identified as belonging to the SOY dietary group may show a better relationship with detoxification, while genes from the CO dietary group showed a decrease in bile acid synthesis. Again, the importance of enriched DEGs that are involved in regulatory pathways and networks related to bile acids that regulate glucose and lipid metabolism in hepatocytes is noted.
The modification of the diets with the oils resulted in changes in CD36 gene expression, which may be involved in relevant pathways, related to inflammatory processes and diseases. CD36 binds to long-chain FAs (LCFAs) and can function in the transport and/or as a regulator of fatty acid transport (Stelzer et al., 2016) and a negative regulator of angiogenesis and inflammation. CD36 is ubiquitously expressed, being present in cells such as hepatocytes, adipocytes, cardiac and skeletal myocytes, and specialized epithelia of the breast, kidney, and intestine (Silverstein and Febbraio, 2009). According to Silverstein and Febbraio (2009), CD36 is related to the pathogenesis of diseases such as atherosclerosis and Alzheimer’s disease. The LCFAs circulate in the blood, and small portions of LCFA enter the brain, where FA metabolism within the ventromedial hypothalamic region (VHM) can function as a sensor for nutrient availability (Le Foll et al., 2009). The detection of hypothalamic OA is impaired in animal models for obesity due to an increase in proteins that reverse the esterification of OA and other effects that can decrease acyl-CoA (oleoyl-CoA), thus contributing to an increase in food intake and glucose production (Lane et al., 2008). CD36 was also enriched in the “role of adipose tissue hypoxia in obesity and type 2 diabetes” pathway, and it is a shared target of LXR, PXR, and PPAR-gamma (Yin et al., 2009). Decreased expression of FATP1 and CD36 may result in the inhibition of FA absorption and increased accumulation of free FA. The mechanisms and effects of this pathway result in a decrease in adiponectin, and an underexpression in obesity leads to insulin resistance (Chen et al., 2006; Yin et al., 2009). In addition to hypertrophy and adipocyte death that can be caused by inhibition linked to PPAR-gamma, this increase in apoptosis of adipose tissue leads to macrophage infiltration in adipose tissue and a release of stored FA (Weisberg et al., 2003; Levin et al., 2011). Furthermore, the CD36 gene in humans is currently being investigated as a potential target in some diseases, such as cardiovascular disease, metabolic syndrome, obesity, and cancer (Niculite et al., 2019). Thus, in this study, we identified CD36 modified by dietary oils, and in the CO group, a low expression of CD36 was identified when compared to SOY.
There was no differential gene expression observed between the CO and FO diets, which was expected based on the fatty acid profile of the liver of animals fed with both diets (Table 1). We observed a significant difference in the amounts of EPA and DHA and consequently in the n-3, n-6:n-3 ratio, and atherogenic index. However, the increased EPA and DHA deposition was not sufficient to differentially modify the liver transcriptome as found in other studies (Zhang et al., 2019). Adequate intake of both n-3 and n-6 contributes to the long-term reduction of LDL cholesterol (Lima et al., 2022). The CO and FO oils are rich in PUFA, with a high content of OA, which are used as an alternative to improve the lipid profile of meat products (Lima et al., 2022). Canola and fish oils showed a beneficial immunoregulatory effect and controlled atherosclerosis in a study with cells (macrophages derived from human blood monocytes) treated with fatty oils of different omega-6/omega-3 ratios (Subash-Babu and Alshatwi, 2018).
In the comparison between SOY and FO in the cholesterol metabolism pathway, ACOX2 participates through the activating effect on the catalysis mechanism of a bile acid intermediate (Van Veldhoven et al., 1994). ACOX2 encodes an enzyme related to the metabolism of branched chain FA and bile acid intermediates (Zhang et al., 2021). Thus, cholesterol metabolism can be decreased with the expression of CYP7A1 and ACOX2 in the SOY group in relation to FO. In a muscle transcriptome study using pigs with extreme values of FA composition performed by Puig-Oliveras et al. (2014), ACOX2 was enriched related to lipid metabolism with functions related to oxidation, synthesis, and insulin resistance. ACOX2 deficiency has been reported in human liver-related diseases (Zhang et al., 2021).
Concerning the SOY vs. FO comparison, DEGs were enriched in relevant signaling pathways, such as IL-18. The IL-18 DEG is a classical member of the IL-1 superfamily of cytokines and is a pro-inflammatory, pro-atherogenic cytokine (Aso et al., 2003). IL-18 binds to its specific receptor, triggering intercellular responses that result in the activation of NF-κB and inflammatory processes (Somm and Jornayvaz, 2022). Pro-IL-18 is produced by various cells, but initially, the main source of IL-18 described was the Kupffer cell (liver-resident macrophage), which plays an important pathophysiological role in health and disease (Yasuda et al., 2019; Somm and Jornayvaz, 2022).
In a study with an obese mini-pig model fed a diet with high sugar content (33% sugar) and fat (10% lard), IL-18, together with TNF-α, IL-6, leptin, and IL -1β, increased significantly (Zhu et al., 2019). High levels of this cytokine were identified in a study with humans presenting with type 2 diabetes (Aso et al., 2003), showing possible participation in disease development (Thorand et al., 2005). Also, in humans, elevated circulating levels of IL-18, a product of the activation of the nucleotide binding and oligomerization domain-like receptor family pyrin domain-containing 3 (NLRP3) inflammasome, have been identified in patients with metabolic syndrome (Yasuda et al., 2019). However, IL-18 alone does not exhibit a pro-inflammatory effect when other cytokines are low in expression (Yasuda et al., 2019).
IL-18 was enriched in the “role of the inflammasome in macrophages, adipocytes, and pancreatic beta cells in type 2 diabetes” pathway by MetaCore. IL-18 may be related to metabolic syndrome and its consequences, that is, it may be involved in the set of risk factors that identify a population at increased risk for developing metabolic syndrome, which encompasses type 2 diabetes and cardiovascular diseases (Trøseid et al., 2010). Furthermore, IL-18 has atherogenic properties through effects on IL-6, TNF-α, and interferon-γ; thus, dietary interventions have been proposed to decrease IL-18 levels in obese women resulting in weight loss as an important intervention to reduce IL-18 levels (Esposito et al., 2002). In our study, IL-18 is also enriched in the “role of IFN-beta in the inhibition of T helper 1 (Th1) cell differentiation in multiple sclerosis” pathway, and in this pathway, IL-18 plays a fundamental role in the differentiation of Th1 cells, which initiate signaling pathways that lead to Th1 cell differentiation and IFN-gamma production (Codarri et al., 2010). Multiple sclerosis is an autoimmune disease mediated by autoreactive Th1 that causes damage to the myelin sheath upon entering the central nervous system (Hemmer et al., 2002). IL-18 was upregulated in the SOY group with possible relation to a pro-inflammatory condition, and on the other hand, the atherogenic index showed no difference between the diets.
With the differential expression between the two comparisons, we also identified common genes such as CYP7A1 DEG and PLA2G2D, which have a function related to the conversion of cholesterol into bile acids and related to the production of lysophospholipids and free FA, respectively. PLA2G2D was negatively associated with serum triglyceride and cholesterol concentrations and fractions in the study of Ossabaw pigs. In the study, the pigs were fed a diet high in saturated fat, cholesterol, and refined carbohydrates and low in fiber, compared to pigs fed a diet high in unsaturated fat (oils canola, soy, and corn), unrefined carbohydrates, fruit/vegetables and fiber, and low cholesterol (Ye et al., 2021), demonstrating that the oil sources in pigs’ diets have altered relevant genes. Studies relating the FA profile and differential gene expression are still limited in pigs, and new approaches are needed. Other studies have already emphasized the importance of studying the porcine transcriptome in the study of genes regulating lipid metabolism due to the similarities between pigs and humans (Corominas et al., 2013). The lipid and FA compositions of meat have an important impact on human health in the modulation of genes involved in diseases, such as obesity, diabetes, and neurodegenerative diseases. In this study, we highlight the main genes involved in metabolic and disease pathways regulated by FA in the liver transcriptome. Thus, when we use the human species as a background list for pathway enrichment, we observe mechanisms that can have effects with modifications arising from the diet with different oils. More studies are needed to analyze the FA that specifically modulates each of the genes.
5 Conclusion
In this study, we identified relevant changes in the transcriptome profiles and FA deposits in the liver, resulting from feeding different diets containing SOY, CO, or FO, which contain different FA profiles. Between the CO and SOY comparison, despite similar FAs, relevant differences were identified in gene expression. Between SOY and FO, FO presented a greater amount of total n-3 and a consequent reduction in the n-6:n-3 ratio. Pathways were enriched in signaling pathways related to metabolism and metabolic and neurodegenerative diseases. Our results help understand the effects of different oil sources on the behavior of genes with differential expression in metabolic pathways and gene networks in the liver. The identified genes and signaling pathways play an essential role in affecting the liver, and further studies should be performed to elucidate the mechanisms.
Data availability statement
The datasets presented in this study can be found in online repositories. The names of the repository/repositories and accession number(s) can be found in the article/Supplementary Material.
Ethics statement
All experimental procedures involving animals are in accordance with the requirements of the Animal Care and Use Committee of Luiz de Queiroz College of Agriculture (University of São Paulo, Piracicaba, Brazil; protocol: 2018.5.1787.11.6 and number CEUA 2018-28) and followed ethical principles in animal research according to the Guide for the Care and Use of Agricultural Animals in Agricultural Research and Teaching (Fass, 2010).
Author contributions
Conceptualization: SF, GCM, JR, JK, DK, DG, HF, LCA, JB, LLC, GBM, AL, and AC; writing—original draft preparation: SF, BS, JG, MD, and AC; data analyses: SF, VA, FF, and AC; collaboration for interpretation and discussion of the results: VA, GCM, BS-V, JA, JR, JK, DK, DG, HF, LCA, GBM, SA, LLC, AL, and AC; supervision: AC; and funding acquisition: AC. All authors have read and agreed to the published version of the manuscript.
Funding
This study was supported by the São Paulo Research Foundation (FAPESP, grant numbers: 2020/10042-6, 2017/25180-2, 2018/15653-3, 2018/26797-6, 2018/26816-0, 2021/06553-8, and 2014/02493-7), the Brazilian National Council for Scientific and Technological Development (CNPq), which provided a researcher fellowship to AM and LC. This study was financed in part by the Coordenação de Aperfeiçoamento de Pessoal de Nível Superior—Brasil (CAPES)—Finance Code 001.
Acknowledgments
The authors would like to thank the collaborative efforts between the University of São Paulo and Iowa State University. We also thank DB Genética Suína for providing the animals, housing, feeds, and employees who helped carry out this research.
Conflict of interest
The authors declare that the research was conducted in the absence of any commercial or financial relationships that could be construed as a potential conflict of interest.
Publisher’s note
All claims expressed in this article are solely those of the authors and do not necessarily represent those of their affiliated organizations, or those of the publisher, the editors, and the reviewers. Any product that may be evaluated in this article, or claim that may be made by its manufacturer, is not guaranteed or endorsed by the publisher.
Supplementary material
The Supplementary Material for this article can be found online at: https://www.frontiersin.org/articles/10.3389/fgene.2023.1053021/full#supplementary-material
References
Almeida, V. V., Silva, J. P. M., Schinckel, A. P., Meira, A. N., Moreira, G. C. M., Gomes, J. D., et al. (2021). Effects of increasing dietary oil inclusion from different sources on growth performance, carcass and meat quality traits, and fatty acid profile in genetically lean immunocastrated male pigs. Livest. Sci. 248, 104515. doi:10.1016/j.livsci.2021.104515
Aso, Y., Okumura, K. I., Takebayashi, K., Wakabayashi, S., and Inukai, T. (2003). Relationships of plasma interleukin-18 concentrations to hyperhomocysteinemia and carotid intimal-media wall thickness in patients with type 2 diabetes. Diabetes Care 26, 2622–2627. doi:10.2337/diacare.26.9.2622
Baker, E. J., Miles, E. A., Burdge, G. C., Yaqoob, P., and Calder, P. C. (2016). Metabolism and functional effects of plant-derived omega-3 fatty acids in humans. Prog. Lipid Res. 64, 30–56. doi:10.1016/j.plipres.2016.07.002
Benjamini, Y., and Hochberg, Y. (1995). Controlling the false discovery rate: A practical and powerful approach to multiple testing. J. R. Stat. Soc. Ser. B 57, 289–300. doi:10.1111/j.2517-6161.1995.tb02031.x
Cesar, A. S. M., Regitano, L. C. A., Poleti, M. D., Andrade, S. C. S., Tizioto, P. C., Oliveira, P. S. N., et al. (2016). Differences in the skeletal muscle transcriptome profile associated with extreme values of fatty acids content. BMC Genomics 17, 961. doi:10.1186/s12864-016-3306-x
Chen, B., Lam, K. S. L., Wang, Y., Wu, D., Lam, M. C., Shen, J., et al. (2006). Hypoxia dysregulates the production of adiponectin and plasminogen activator inhibitor-1 independent of reactive oxygen species in adipocytes. Biochem. Biophys. Res. Commun. 341, 549–556. doi:10.1016/j.bbrc.2006.01.004
Chiang, J. Y. L., and Ferrell, J. M. (2020). Up to date on cholesterol 7 alpha-hydroxylase (CYP7A1) in bile acid synthesis. Liver Res. 4, 47–63. doi:10.1016/j.livres.2020.05.001
Codarri, L., Fontana, A., and Becher, B. (2010). Cytokine networks in multiple sclerosis: Lost in translation. Curr. Opin. Neurol. 23, 205–211. doi:10.1097/WCO.0b013e3283391feb
Corominas, J., Ramayo-Caldas, Y., Puig-Oliveras, A., Estellé, J., Castelló, A., Alves, E., et al. (2013). Analysis of porcine adipose tissue transcriptome reveals differences in de novo fatty acid synthesis in pigs with divergent muscle fatty acid composition. BMC Genomics 14, 843. doi:10.1186/1471-2164-14-843
Danhelovska, T., Kolarova, H., Zeman, J., Hansikova, H., Vaneckova, M., Lambert, L., et al. (2020). Multisystem mitochondrial diseases due to mutations in mtDNA-encoded subunits of complex i. BMC Pediatr. 20, 41. doi:10.1186/s12887-020-1912-x
Darwiche, R., and Struhl, K. (2020). Pheno-RNA, a method to associate genes with a specific phenotype, identifies genes linked to cellular transformation. Proc. Natl. Acad. Sci. U. S. A. 117, 28925–28929. doi:10.1073/pnas.2014165117
Dobin, A., and Gingeras, T. R. (2015). Mapping RNA-seq reads with STAR. Curr. Protoc. Bioinforma. 51, 1–11. doi:10.1002/0471250953.bi1114s51
Esposito, K., Pontillo, A., Ciotola, M., Di Palo, C., Grella, E., Nicoletti, G., et al. (2002). Weight loss reduces interleukin-18 levels in obese women. J. Clin. Endocrinol. Metab. 87, 3864–3866. doi:10.1210/jcem.87.8.8781
Fanalli, S. L., Silva, B. P. M. D., Gomes, J. D., Almeida, V. V., Freitas, F. A. O., Moreira, G. C. M., et al. (2022b). Differential gene expression associated with soybean oil level in the diet of pigs. Anim 202212, 1632. doi:10.3390/ANI12131632
Fanalli, S. L., Silva, B. P. M. da, Gomes, J. D., Almeida, V. V., Afonso, J., Reecy, J. M., et al. (2022a). Effect of dietary soybean oil inclusion on liver-related transcription factors in a pig model for metabolic diseases. Sci. Rep. 12, 10318. doi:10.1038/s41598-022-14069-1
Fass (2010). Guide for the care and use of agricultural animals in research and teaching. 3rd ed. Champaign, IL: Federation of Animal Science Societies.
Fu, L., Zhao, Y., Wu, X., Liu, H., Shi, J., Lu, J., et al. (2011). CYP7A1 genotypes and haplotypes associated with hypertension in an obese Han Chinese population. Hypertens. Res. 34, 722–727. doi:10.1038/hr.2011.18
Fujii, J., Otsu, K., Zorzato, F., De Leon, S., Khanna, V. K., Weiler, J. E., et al. (1991). Identification of a mutation in porcine ryanodine receptor associated with malignant hyperthermia. Science 80, 448–451. doi:10.1126/science.1862346
Gomes, J. D., Fanalli, S. L., Silva, B. P. M., Silva, J. P. M., Luchiari Filho, A., and Cesar, A. S. M. (2022). “Ácidos graxos componentes funcionais da carne: Revisão,” in Pesquisas E atualizações em ciência dos alimentos. doi:10.53934/9786599539657-2
Groenen, M. A. M., Archibald, A. L., Uenishi, H., Tuggle, C. K., Takeuchi, Y., Rothschild, M. F., et al. (2012). Analyses of pig genomes provide insight into porcine demography and evolution. Nature 491, 393–398. doi:10.1038/nature11622
Guasch-Ferré, M., Hu, F. B., Martínez-González, M. A., Fitó, M., Bulló, M., Estruch, R., et al. (2014). Olive oil intake and risk of cardiovascular disease and mortality in the PREDIMED Study. BMC Med. 12, 78. doi:10.1186/1741-7015-12-78
Guevara-Ramírez, P., Cadena-Ullauri, S., Ruiz-Pozo, V. A., Tamayo-Trujillo, R., Paz-Cruz, E., Simancas-Racines, D., et al. (2022). Genetics, genomics, and diet interactions in obesity in the Latin American environment. Front. Nutr. 9, 1063286. doi:10.3389/fnut.2022.1063286
Heintz, M. M., Eccles, J. A., Olack, E. M., Maner-Smith, K. M., Ortlund, E. A., and Baldwin, W. S. (2022). Human CYP2B6 produces oxylipins from polyunsaturated fatty acids and reduces diet-induced obesity. PLoS One 17, 02770533–e277128. doi:10.1371/journal.pone.0277053
Hemmer, B., Cepok, S., Nessler, S., and Sommer, N. (2002). Pathogenesis of multiple sclerosis: An update on immunology. Curr. Opin. Neurol. 15, 227–231. doi:10.1097/00019052-200206000-00001
Hou, J., Ye, X., Feng, W., Zhang, Q., Han, Y., Liu, Y., et al. (2022). Distance correlation application to gene co-expression network analysis. BMC Bioinforma. 23, 81. doi:10.1186/s12859-022-04609-x
Jakobsen, M. U., O’Reilly, E. J., Heitmann, B. L., Pereira, M. A., Bälter, K., Fraser, G. E., et al. (2009). Major types of dietary fat and risk of coronary heart disease: A pooled analysis of 11 cohort studies. Am. J. Clin. Nutr. 89, 1425–1432. doi:10.3945/ajcn.2008.27124
Jump, D. B., Clarke, S. D., Macdougald, O., and Thelen, A. (1993). Polyunsaturated fatty acids inhibit S14 gene transcription in rat liver and cultured hepatocytes. Proc. Natl. Acad. Sci. U. S. A. 90, 8454–8458. doi:10.1073/pnas.90.18.8454
Jung, D., Mangelsdorf, D. J., and Meyer, U. A. (2006). Pregnane X receptor is a target of farnesoid X receptor. J. Biol. Chem. 281, 19081–19091. doi:10.1074/jbc.M600116200
Klemm, D. J., Leitner, J. W., Watson, P., Nesterova, A., Reusch, J. E. B., Goalstone, M. L., et al. (2001). Insulin-induced adipocyte differentiation: Activation of CREB rescues adipogenesis from the arrest caused by inhibition of prenylation. J. Biol. Chem. 276, 28430–28435. doi:10.1074/jbc.M103382200
Lane, M. D., Wolfgang, M., Cha, S. H., and Dai, Y. (2008). Regulation of food intake and energy expenditure by hypothalamic malonyl-CoA. Int. J. Obes. 32, S49–S54. doi:10.1038/ijo.2008.123
Le Foll, C., Irani, B. G., Magnan, C., Dunn-Meynell, A. A., and Levin, B. E. (2009). Characteristics and mechanisms of hypothalamic neuronal fatty acid sensing. Am. J. Physiol. - Regul. Integr. Comp. Physiol. 297, R655–R664. doi:10.1152/ajpregu.00223.2009
Lee, J., Kim, C. H., Simon, D. K., Aminova, L. R., Andreyev, A. Y., Kushnareva, Y. E., et al. (2005). Mitochondrial cyclic AMP response element-binding protein (CREB) mediates mitochondrial gene expression and neuronal survival. J. Biol. Chem. 280, 40398–40401. doi:10.1074/jbc.C500140200
Levin, B. E., Magnan, C., Dunn-Meynell, A., and Le Foll, C. (2011). Metabolic sensing and the brain: Who, what, where, and how? Endocrinology 152, 2552–2557. doi:10.1210/en.2011-0194
Lima, T. L. S., da Costa, G. F., Alves, R. D. N., de Araújo, C. D. L., da Silva, G. F. G., Ribeiro, N. L., et al. (2022). Vegetable oils in emulsified meat products: A new strategy to replace animal fat. Food Sci. Technol. 42. doi:10.1590/fst.103621
Love, M. I., Huber, W., and Anders, S. (2014). Moderated estimation of fold change and dispersion for RNA-seq data with DESeq2. Genome Biol. 15, 550. doi:10.1186/s13059-014-0550-8
Lyu, W., Xiang, Y., Wang, X., Li, J., Yang, C., Yang, H., et al. (2022). Differentially expressed hepatic genes revealed by transcriptomics in pigs with different liver lipid contents. Oxid. Med. Cell. Longev. 2022, 2315575. doi:10.1155/2022/2315575
Mater, M. K., Pan, D., Bergen, W. G., and Jump, D. B. (1998). Arachidonic acid inhibits lipogenic gene expression in 3T3-L1 adipocytes through a prostanoid pathway. J. Lipid Res. 39, 1327–1334. doi:10.1016/s0022-2275(20)32513-x
Moghadasian, M. H., and Shahidi, F. (2017). Fatty acids. Int. Encycl. Public Heal., 114–122. doi:10.1016/B978-0-12-803678-5.00157-0
Nakamura, M. T., and Nara, T. Y. (2003). Essential fatty acid synthesis and its regulation in mammals. Prostagl. Leukot. Essent. Fat. Acids 68, 145–150. doi:10.1016/S0952-3278(02)00264-8
Niculite, C. M., Enciu, A. M., and Hinescu, M. E. (2019). CD 36: Focus on epigenetic and post-transcriptional regulation. Front. Genet. 10, 680. doi:10.3389/fgene.2019.00680
Norlin, M., Andersson, U., Björkhem, I., and Wikvall, K. (2000). Oxysterol 7α-hydroxylase activity by cholesterol 7α-hydroxylase (CYP7a). J. Biol. Chem. 275, 34046–34053. doi:10.1074/jbc.M002663200
Park, C. Y., and Han, S. N. (2018). “Lipid pathway in liver cells and its modulation by dietary extracts,” in The molecular nutrition of fats. doi:10.1016/B978-0-12-811297-7.00008-1
Poklukar, K., Čandek-Potokar, M., Lukač, N. B., Tomažin, U., and Škrlep, M. (2020). Lipid deposition and metabolism in local and modern pig breeds: A review. Animals 10. doi:10.3390/ani10030424
Puig-Oliveras, A., Ramayo-Caldas, Y., Corominas, J., Estellé, J., Pérez-Montarelo, D., Hudson, N. J., et al. (2014). Differences in muscle transcriptome among pigs phenotypically extreme for fatty acid composition. PLoS One 9, e99720. doi:10.1371/journal.pone.0099720
Ramayo-Caldas, Y., Mach, N., Esteve-Codina, A., Corominas, J., Castelló, A., Ballester, M., et al. (2012). Liver transcriptome profile in pigs with extreme phenotypes of intramuscular fatty acid composition. BMC Genomics 13, 547. doi:10.1186/1471-2164-13-547
Rostagno, H. S. (2011). Tabelas brasileiras para aves e suínos: Composição de alimentos e exigências nutricionais. Tabelas Bras. aves suínos Compos. Aliment. Exig. Nutr. 2, 186.
Rui, L. (2014). Energy metabolism in the liver. Compr. Physiol. 4, 177–197. doi:10.1002/cphy.c130024
Saedi, S., Noroozi, M., Khosrotabar, N., Mazandarani, S., and Ghadrdoost, B. (2017). How canola and sunflower oils affect lipid profile and anthropometric parameters of participants with dyslipidemia. Med. J. Islam. Repub. Iran. 31, 5. doi:10.18869/mjiri.31.5
Sarri, L., Balcells, J., Seradj, A. R., Pena, R. N., Ramírez, G. A., Tor, M., and de la Fuente, G. (2022). Age evolution of lipid accretion rate in boars selected for lean meat and duroc barrows. Anim. (Basel) 12 (14), 1868. doi:10.3390/ani12141868
Shimizu, N., Maruyama, T., Yoshikawa, N., Matsumiya, R., Ma, Y., Ito, N., et al. (2015). A muscle-liver-fat signalling axis is essential for central control of adaptive adipose remodelling. Nat. Commun. 6, 6693. doi:10.1038/ncomms7693
Silverstein, R. L., and Febbraio, M. (2009). CD36, a scavenger receptor involved in immunity, metabolism, angiogenesis, and behavior. Sci. Signal. 2, re3. doi:10.1126/scisignal.272re3
Somm, E., and Jornayvaz, F. R. (2022). Interleukin-18 in metabolism: From mice physiology to human diseases. Front. Endocrinol. (Lausanne). 13, 971745–971814. doi:10.3389/fendo.2022.971745
Stelzer, G., Rosen, N., Plaschkes, I., Zimmerman, S., Twik, M., Fishilevich, S., et al. (2016). The GeneCards suite: From gene data mining to disease genome sequence analyses. Curr. Protoc. Bioinforma. 54, 1. doi:10.1002/cpbi.5
Subash-Babu, P., and Alshatwi, A. A. (2018). Effects of increasing ratios of dietary omega-6/omega-3 fatty acids on human monocyte immunomodulation linked with atherosclerosis. J. Funct. Foods 41, 258–267. doi:10.1016/j.jff.2017.12.020
Szostak, A., Ogłuszka, M., Te Pas, M. F. W., Poławska, E., Urbański, P., Juszczuk-Kubiak, E., et al. (2016). Effect of a diet enriched with omega-6 and omega-3 fatty acids on the pig liver transcriptome. Genes Nutr. 11, 9–17. doi:10.1186/s12263-016-0517-4
Thorand, B., Kolb, H., Baumert, J., Koenig, W., Chambless, L., Meisinger, C., et al. (2005). Elevated levels of interleukin-18 predict the development of type 2 diabetes: Results from the MONICA/KORA Augsburg study. Diabetes 54. doi:10.2337/diabetes.54.10.2932
Trøseid, M., Seljeflot, I., and Arnesen, H. (2010). The role of interleukin-18 in the metabolic syndrome. Cardiovasc. Diabetol. 9, 11. doi:10.1186/1475-2840-9-11
USDA (2022). Livestock and poultry. UNITED STATES Dep. Agric. PDS ONLINE. Available at: https://apps.fas.usda.gov/psdonline/circulars/livestock_poultry.pdf.
Van Veldhoven, P. P., Van Rompuy, P., Vanhooren, C. T., and Mannaerts, G. P. (1994). Purification and further characterization of peroxisomal trihydroxycoprostanoyl-CoA oxidase from rat liver. Biochem. J. 304, 195–200. doi:10.1042/bj3040195
Wang, Y., Zhang, W., Wu, X., Wu, C., Qian, L., Wang, L., et al. (2020). Transcriptomic comparison of liver tissue between anqing six-end-white pigs and yorkshire pigs based on rna sequencing. Genome 63, 203–214. doi:10.1139/gen-2019-0105
Wang, Z., Gerstein, M., and Snyder, M. (2009). RNA-seq: A revolutionary tool for transcriptomics. Nat. Rev. Genet. 10, 57–63. doi:10.1038/nrg2484
Wang, Z., He, Y., and Tan, Z. (2022). Transcription analysis of liver and muscle tissues from Landrace finishing pigs with different feed conversion ratios. Genes (Basel). 13, 2067. doi:10.3390/genes13112067
Weisberg, S. P., McCann, D., Desai, M., Rosenbaum, M., Leibel, R. L., and Ferrante, A. W. (2003). Obesity is associated with macrophage accumulation in adipose tissue. J. Clin. Invest 112, 1796–1808. doi:10.1172/jci19246
Wood, J. D., Enser, M., Fisher, A. V., Nute, G. R., Sheard, P. R., Richardson, R. I., et al. (2008). Fat deposition, fatty acid composition and meat quality: A review. Meat Sci. 78, 343–358. doi:10.1016/j.meatsci.2007.07.019
Xing, K., Zhao, X., Ao, H., Chen, S., Yang, T., Tan, Z., et al. (2019). Transcriptome analysis of miRNA and mRNA in the livers of pigs with highly diverged backfat thickness. Sci. Rep. 9, 16740. doi:10.1038/s41598-019-53377-x
Yao, D., Yang, C., Ma, J., Chen, L., Luo, J., Ma, Y., et al. (2020). cAMP response element binding protein 1 (CREB1) promotes monounsaturated fatty acid synthesis and triacylglycerol accumulation in goat mammary epithelial cells. Animals 10, 1871. doi:10.3390/ani10101871
Yasuda, K., Nakanishi, K., and Tsutsui, H. (2019). Interleukin-18 in health and disease. Int. J. Mol. Sci. 20, 649. doi:10.3390/ijms20030649
Ye, S., Matthan, N. R., Lamon-Fava, S., Aguilar, G. S., Turner, J. R., Walker, M. E., et al. (2021). Western and heart healthy dietary patterns differentially affect the expression of genes associated with lipid metabolism, interferon signaling and inflammation in the jejunum of Ossabaw pigs. J. Nutr. Biochem. 90, 108577. doi:10.1016/j.jnutbio.2020.108577
Yin, J., Gao, Z., He, Q., Zhou, D., Guo, Z., and Ye, J. (2009). Role of hypoxia in obesity-induced disorders of glucose and lipid metabolism in adipose tissue. Am. J. Physiol. - Endocrinol. Metab. 296, E333–E342. doi:10.1152/ajpendo.90760.2008
Zhang, J., Xu, X., Zhu, H., Wang, Y., Hou, Y., and Liu, Y. (2019). Dietary fish oil supplementation alters liver gene expressions to protect against LPS-induced liver injury in weanling piglets. Innate Immun. 25, 60–72. doi:10.1177/1753425918821420
Zhang, Q., Zhang, Y., Sun, S., Wang, K., Qian, J., Cui, Z., et al. (2021). ACOX2 is a prognostic marker and impedes the progression of hepatocellular carcinoma via PPARα pathway. Cell Death Dis. 12, 15. doi:10.1038/s41419-020-03291-2
Zhang, W., Yang, B., Zhang, J., Cui, L., Ma, J., Chen, C., et al. (2016). Genome-wide association studies for fatty acid metabolic traits in five divergent pig populations. Sci. Rep. 6, 24718. doi:10.1038/srep24718
Keywords: RNA-Seq, metabolic diseases, transcriptome, metabolic disease, neurodegenerative diseases, liver, pig
Citation: Fanalli SL, Silva BPMd, Gomes JD, Durval MC, Almeida VVd, Moreira GCM, Silva-Vignato B, Afonso J, Freitas FAO, Reecy JM, Koltes JE, Koltes D, Garrick D, Correia de Almeida Regitano L, Balieiro JCdC, Mourão GB, Coutinho LL, Fukumasu H, Alencar SMd, Luchiari Filho A and Cesar ASM (2023) RNA-seq transcriptome profiling of pigs’ liver in response to diet with different sources of fatty acids. Front. Genet. 14:1053021. doi: 10.3389/fgene.2023.1053021
Received: 24 September 2022; Accepted: 09 January 2023;
Published: 25 January 2023.
Edited by:
Chao-Qiang Lai, Tufts University, United StatesCopyright © 2023 Fanalli, Silva, Gomes, Durval, Almeida, Moreira, Silva-Vignato, Afonso, Freitas, Reecy, Koltes, Koltes, Garrick, Correia de Almeida Regitano, Balieiro, Mourão, Coutinho, Fukumasu, Alencar, Luchiari Filho and Cesar. This is an open-access article distributed under the terms of the Creative Commons Attribution License (CC BY). The use, distribution or reproduction in other forums is permitted, provided the original author(s) and the copyright owner(s) are credited and that the original publication in this journal is cited, in accordance with accepted academic practice. No use, distribution or reproduction is permitted which does not comply with these terms.
*Correspondence: Aline Silva Mello Cesar, YWxpbmVjZXNhckB1c3AuYnI=