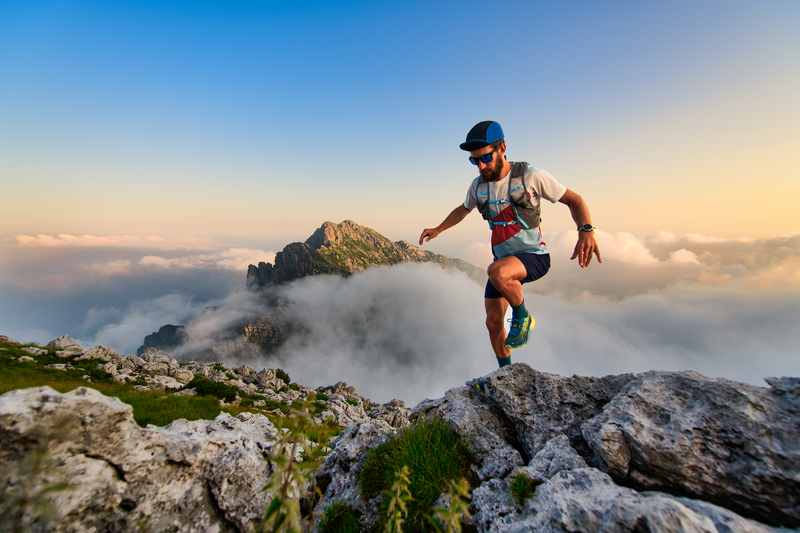
95% of researchers rate our articles as excellent or good
Learn more about the work of our research integrity team to safeguard the quality of each article we publish.
Find out more
ORIGINAL RESEARCH article
Front. For. Glob. Change , 17 October 2023
Sec. Temperate and Boreal Forests
Volume 6 - 2023 | https://doi.org/10.3389/ffgc.2023.1278409
Introduction: Insect herbivores and biotrophic pathogens are major stressors influencing natural regeneration in woodlands. Information on the effect of elevated CO2 (eCO2) on plant-insect-pathogen interactions under natural conditions is lacking.
Methods: We studied the effects of eCO2 on leaf-out phenology, as well as on levels of insect herbivory and powdery mildew (PM), i.e., reduction of leaf photosynthetic material. We then assessed the combined impacts of these biotic stressors and eCO2 on seedling photosynthesis and growth. A total of 92 naturally recruited and 114 potted seedlings of 5 temperate tree species (Quercus robur, Acer pseudoplatanus, Corylus avellana, Crataegus monogyna, and Ilex aquifolium) within a mature oak woodland were studied.
Results: We found that eCO2 advanced leaf-out phenology and was a significant explanatory variable for growth and physiological performance in potted seedlings. Potted oak seedlings experienced 11-fold higher tissue loss from insect herbivory than natural seedlings. The earliest leaf-flushing species, hawthorn, and the evergreen holly were resistant to insect attack and were not affected by PM. Oak was defoliated most but showed the highest regeneration capacity. Hazel was more resistant to PM infection than oak and sycamore. Despite being highly infected by PM, sycamore was less affected than oak. The more vigorous sycamore and oak seedlings suffered more severe PM disease.
Conclusion: No evidence emerged that eCO2 enhances natural regeneration under biotic stress for any of the species studied.
Insect herbivores and pathogenic fungi are considered the most common biotic stress agents in temperate forests (Jactel et al., 2009) and represent important barriers to seedling establishment. This combination of stress factors may also have serious consequences for forest biodiversity and ecosystem functions (Hasegawa et al., 2018; Pureswaran et al., 2018). Temperate forests are among the biggest sinks of emitted carbon (Le Quéré et al., 2018) in a world where anthropogenic activities have led to a 45% increase of atmospheric CO2 concentration, and consequently a mean 0.85°C increase in global temperature since the Industrial Revolution. Although increasing CO2 (“carbon fertilisation”) might result in an increase of the forest carbon sink (Ciais et al., 2014), the complex interplay between biotic stressors and climatic factors, combined with differing responses across tree species, makes it very difficult to predict overall consequences of climate change on forest health and forest carbon assimilation capacity (Jactel et al., 2019).
While investigating the direct impact of rising CO2 on the ecophysiology and functional response of trees can be accomplished in controlled environment chambers (e.g., Liu et al., 2004; Aranda et al., 2020), or in open-top chambers (e.g., Norby et al., 1995; Vanaja et al., 2006), investigating ecosystem-level responses requires large-scale forest Free-Air CO2 Enrichment (FACE) facilities (e.g., Karnosky et al., 2001). FACE facilities provide the most ecologically realistic environment to date for evaluating CO2 effects on forest ecosystems. The first-generation of FACE experiments were established in temperate and young monocultures (Nowak et al., 2004; DOE, 2020) and substantially improved our understanding of the capacity of forests to sequester carbon under elevated CO2 (eCO2) (Nowak et al., 2004; Ainsworth and Long, 2005; Norby et al., 2005; Norby and Zak, 2011; Medlyn et al., 2015). Second generation FACE experiments, the Eucalyptus free air carbon dioxide enrichment experiment facility (EucFACE) in Australia and the Birmingham Institute of Forest Research FACE Facility (BIFoR-FACE) in UK, have advanced this endeavour to include old-growth forests, allowing researchers to investigate whether eCO2 alters a broader range of forest processes in the canopy, subcanopy, herb-and-regeneration layer (Gherlenda et al., 2016a; Jiang et al., 2020; Roberts et al., 2022), and soils (Martins et al., 2021). EucFACE was established in a eucalyptus forest (Duursma et al., 2016), whereas BIFoR-FACE, the site of the present study, is in a more complex and mixed canopy (Hart et al., 2020) dominated by English oak (Quercus robur L.) in the top canopy, and co-dominated in the understorey by other deciduous and evergreen broadleaves such as sycamore (Acer pseudoplatanus L.), hazel (Corylus avellana L.), hawthorn (Crataegus monogyna Jacq), and holly (Ilex aquifolium L.).
In temperate deciduous forests, leaf-chewing lepidopteran larvae are a key feeding guild, such larvae can remove significant amounts of photosynthetically active material early in the season (Schowalter et al., 1986). Large outbreaks of insect herbivores are commonly followed by newly developed leaves, prone to fungal infection causing diseases such as powdery mildew (PM) (e.g., Erysiphe spp.) (Field et al., 2019). PM diseases are caused by a large family of biotrophic fungal pathogens, comprising ca. A total of 900 species of the phylum Ascomycota growing superficially on plant surfaces (Takamatsu et al., 2013). During the growing season, hyphae are produced on both upper and lower leaf surfaces, diverting plant photosynthates for the benefit of the fungus (Glawe, 2008). At early stages of PM infection, cells are not killed and photosynthesis can be stimulated, usually followed by a fast decline in activity leading to the desiccation of the leaf and subsequent death (Wang et al., 2014). Several species of PM infect oak, representing a serious threat to natural oak regeneration (Woodward et al., 1929; Lanier et al., 1976; Takamatsu et al., 2007). Other PMs infect sycamore (Talgo et al., 2011), hazel (Arzanlou et al., 2018), and hawthorn (Khairi and Preece, 1978). This disease is the most common foliar pathogen on oaks in Europe (Marçais and Desprez-Loustau, 2014) and represents a substantial biotic pressure on the species the BIFoR-FACE (Supplementary Figure 1).
It is expected that eCO2 will stimulate leaf-level photosynthesis (Norby and Zak, 2011; Ryan, 2013). However, it is not clear whether this will result in a more attractive food source to flush-feeding insects or not, since palatability could result altered if the C:N ratio increases (Du et al., 2019) or if the plant invests more carbon in defensive compounds (Robinson et al., 2012). Stimulation of photosynthesis will potentially benefit biotrophic pathogens which prefer vigorous hosts to physiologically stressed plants (Jactel et al., 2012; Che-Castaldo et al., 2019; Field et al., 2019). In contrast, increased levels of biotic stress are associated with poorer status of leaf physiological parameters such as photosynthetic rates, fluorescence of chlorophylls, or nitrogen content (Moriondo et al., 2005; Daud et al., 2012; Watanabe et al., 2014; Madriaza et al., 2019). Thus, increased insect herbivory may negatively impact biotrophic pathogens. An additional complicating factor would be if eCO2 stimulates photosynthesis and the development of the photosynthetic apparatus faster during the spring, due to more carbon availability (Linkosalo et al., 2017), thus advancing leaf-out phenology. This may entail important consequences for ecosystem structure and function in deciduous forests since leaf-out phenology defines the photosynthetically active period and the uptake of resources by deciduous trees (Polgar and Primack, 2011), and determines the duration of the growing season. While there is broad evidence that warming results in an advance of spring phenology (Chang et al., 2018; Wang et al., 2020a), little is known about the effect of eCO2 on leaf-out phenology and leaf development after leaf flushing. Faster leaf development may also influence protective functions, for instance defence against insect herbivory (Damestoy et al., 2021) or late frost tolerance (Mayoral et al., 2015).
After bud burst, the leaves of deciduous species achieve full photosynthetic capacity at different times, depending on the species and the life stage. In temperate species, the lag from bud burst to full photosynthetic capacity has been found to range from around 2 weeks for Betula pendula (silver birch) up to 70 days for Q. robur (Morecroft et al., 2008; Linkosalo et al., 2017). A differential effect of eCO2 on leaf-out phenology of coexisting tree species might, therefore, alter responses to spring abiotic and biotic stress (Pérez-Ramos et al., 2020) which in turn results in differential fitness and niche adaptation across forest tree species.
In this study, we examine the effects of eCO2 on key aspects of both natural and potted seedlings performance (leaf-out phenology, growth, leaf photosynthetic parameters) and the interaction of these parameters with defoliating insects and PM, during a year with high pressure from these biotic factors. While natural seedlings emergence occurs under different growing conditions (light, substrate, and water availability) following a random pattern, introducing potted seedlings within the FACE system allowed us for a more homogeneous growing condition to assess more accurately differences between species. Our objectives were to explore whether a higher concentration of CO2 (1) would impact on leaf-out phenology and would differentially accelerate spring leaf development of coexisting tree species; (2) would attract generalist flush-feeding insects equally to tree species with different spring leaf development; (3) would impact on the defoliation intensity and the subsequent leaf regeneration capacity of the species; (4) would stimulate photosynthesis of the studied species and PM severity; and (5) would influence the growth of the species.
Experiments were conducted in the BIFoR-FACE facilities (Hart et al., 2020), located in Mill Haft, Staffordshire, United Kingdom (52.8°N 2.30°W). The structure of this mature, temperate, oak dominated woodland is complex, comprising trees of multiple stature and age. The most representative understorey species, accompanying the canopy oaks (Q. robur L.), are sycamore (A. pseudoplatanus L.), hazel (C. avellana L.), hawthorn (C. monogyna Jacq), and holly (I. aquifolium L.) (Supplementary Table 1). The woodland is currently unmanaged although hazel was coppiced until about 30–40 years ago and has since regrown a dense, multi-stemmed canopy. The herb layer is dominated by bramble (Rubus fruticosus L.), ivy (Hedera helix L.), bluebell (Hyacinthoides non-scripta L.; see Crowley et al., 2021), and fern (Dryopteris sp.), with relatively high variability of the presence and abundance of each within the forest. Browsing and seed predation by vertebrates have been controlled since 2016, with deer excluded by fencing and squirrels numbers reduced (although not totally eliminated) by trapping (Bradwell et al., 2022).
Elevated CO2 Fumigation began in April 2017 and will last until at least 2026. Six roughly cylindrical ring structures, as high as the tree canopy (c. 25 m) and 30 m wide support pipes that can deliver CO2 in such a way that the woodland patches inside the array are immersed in eCO2 to the treatment level from ground to top canopy, while the rest of the woodland remains largely unaffected (Hart et al., 2020). Three of these arrays are used to immerse patches in an atmosphere with CO2 elevated 150 ppm above ambient (i.e., in 2019, to ∼555 ppm). The other three arrays receive unaltered forest air and so remain at ambient (i.e., ∼405 ppm, the global mean at the start of 2019)1 (Supplementary Figure 1). Three non-infrastructure, ‘ghost,’ arrays with the same area were set up in order to assess the effect of the built infrastructure on the woodland. CO2 fumigation is carried out during daylight hours of the growing season. Start-up dates for eCO2 were 27th and 29th of March in 2018 and 2019, respectively, and the switch-off was on the 31st of October in both years.
In June 2018, 121 naturally regenerated seedlings with a diameter below 1 cm at the root collar were identified across the experimental patches. We surveyed the entire accessible area at each array except areas occupied by dense bramble where the chance of finding tree regeneration was minimal due to low light levels. The least frequent species found were hazel and oak. The sample of 121 seedlings included the entire regeneration layer found in the experimental arrays for hazel and oak (12 and 65 seedlings/ha for hazel and oak, respectively, in 2018). Other species were more abundant and not all individuals were used for this study. There were not enough oak individuals in the ambient arrays and so we used seedlings in the ghost arrays to increase the sample size under ambient CO2 conditions. In 2019, 86 of these seedlings were found (24 hawthorn, 5 hazel, 18 holly, 18 oak, and 22 sycamore). Six new oak seedlings were found in 2019 and these were added to increase sample size. Hazel regeneration was again limited in 2019 therefore no new seedlings were found. The exact sample size for different measurements varied throughout the study and is summarised in Table 1.
Table 1. Number of seedlings of each species under ambient (A) and elevated (E) CO2 concentration used to measure each variable (Leaf-out phenology, LLPM, LRC, PM, D, RGR, Gas exchange and Fv/Fm) at each measurement campaign.
In July 2018, 120 (4 seedlings × 5 species × 6 arrays) 1-year-old, nursery-grown seedlings of the same 5 species (oak, sycamore, hazel, hawthorn, and holly) were introduced into the 6 infrastructure arrays (4 seedlings per species in each array). All were from the Midlands region of England (provenance area 403). Potted seedlings were used in order to more closely control the growing conditions, e.g., water nutrient and light availability. We used 7-litre (42 cm high) pots to minimise root growth constraints. All pots were filled with the same fertilised peat substrate and kept at field water capacity by means of self-irrigated trays (76 cm × 17 cm × 3.5 cm; length × width × height). The base tray provided a reservoir of 3.4 litres of water, and each tray contained 5 potted seedlings, one per species. In contrast to the naturally-regenerated seedlings, potted seedlings were grouped and placed at two different locations within each array (two trays per location with a total of 10 pots) under similar light environment on raised walkways to avoid competition with the herbaceous layer. Walkways were installed 30 cm above the ground surface (including in the “ghost” arrays) to protect the experimental site from footfall (see Hart et al., 2020). Herbaceous plants growing inside of the pots were manually removed to avoid competition. One hazel and five hawthorns died during the experimental period in winter leaving a total of 114 potted seedlings (Table 1).
Following to the temporal order of leaf events, we started monitoring the leaf phenology and leaf development of potted seedlings in late February to late April 2019 to investigate the effect of eCO2 on the leaf-out phenology. We only used seedlings growing under similar conditions (potted seedlings) to avoid the impact of heterogenous growing conditions on leaf development of natural seedlings. We used a five-point scale of development: (I) seedlings with or without buds, buds usually covered by bracts; (II) seedlings with more developed buds, leaf primordia begin to open; (III) seedlings with growing leaves, but not completely expanded or developed; (IV) seedlings with opened leaves but not completely developed; and (V) seedlings with fully opened and developed leaves.
In the UK, insect herbivory and PM damage are usually temporally and spatially separated (Field et al., 2019). The majority of insect herbivore damage in the UK occurs in late spring while PM occurs in summer. We visually monitored the loss of leaf photosynthetic material (LLPM) caused by defoliating insects from April to May (Spring 2019) and/ or severe PM infection causing necrosis of foliar tissues from June to August (Summer 2019). LLPM was recorded by trained observers as a continuous variable percentage from 0 (no loss of leaf photosynthetic material) to 100% (complete loss of leaf photosynthetic material).
Secondary bud flushes and subsequent leaf growth caused by intense insect defoliation resulted in a negative change of LLPM in early summer with respect to the previous scoring campaign. This leaf regeneration capacity, LRC (eq. 1), was calculated as the maximum of minus one times the change in LLPM, or zero:
To test for PM impact, we used a four-point ordinal scale of infection severity based on the % of total leaf surface showing symptoms of fungal mycelium: (“0”) infection ∼ 0%; (“1”) infection <50%; (“2”) 51%–75% infected; (“3”) 76%–100% infected. This scale has previously been used to score PM by other authors (Bert et al., 2016; Field et al., 2019).
Leaf gas exchange measurements were taken on both natural and potted seedlings on 3 campaigns, representative of different phases of the 2019 spring/summer season: 24th May, intense defoliation; 10th July, early PM symptoms; and 24th July, late PM symptoms. Seedlings with leaves not fully developed, that were highly defoliated, or severely attacked by PM, were not measured. A portable open gas exchange system (LI-6400XT, LI-COR, Lincoln, NE, USA) was used to measure net photosynthetic rates (An) at a saturating light intensity of 700 μmol photons m–2 s–1 (as determined by light response curves of net photosynthetic rates) and a flow rate of 300 μmol s–1. Reference CO2 was set at either 550 ppm for eCO2 arrays or 400 ppm for ambient CO2 arrays. Data were logged once stabilised. Maximum photochemical efficiency of photosystem II (Fv/Fm) was measured using a portable Pocket PEA chlorophyll fluorimeter (Hansatech Instruments Ltd) in leaves after 30-min dark-adaptation. We conducted a 4th Fv/Fm campaign on the 29th of August because more seedlings were suitable for Fv/Fm measurements at that time, whereas they were not suitable to measure An.
Two perpendicular diameters (D) and shoot length (H) were measured in three campaigns in 2019 on seedlings previously marked near the root collar. Campaign 1: 5th of May (natural seedlings), 14th of May (potted seedlings); campaign 2: 10th of July (natural and potted seedlings); and campaign 3: 29th of August (natural and potted seedlings).
Relative growth rates (RGRd, % day–1) were calculated according to the following equation:
where, is the average of the two perpendicular diameters (mm) at times tn (days). The RGRd values were calculated as a single finite difference over the whole experimental period (RGR98 over 98 days from May to August 2019).
All analyses were performed using PROC GLIMMIX, PROC MIXED SAS version 9.4 (SAS Institute Inc, 2016) and the glmmTMB (v1.1.2.3; Magnusson, 2021) package in R 4.1.2 (R Core Team, 2022). We used generalized linear mixed models (GLMMs) and linear mixed effects models (LMM) to investigate the drivers of leaf-out phenology and leaf development, photosynthetic parameters and seedling growth. Tukey’s post-hoc tests were used to assess the significance of differences between pairs of groups.
We used a GLMM to investigate the effect of CO2 concentration and time on the leaf-out phenology of the potted seedlings. We used a multinomial distribution with cumulative logit link function to model categorical variables.
We used GLMMs to explore differences between species and CO2 concentrations in loss of leaf photosynthetic material at two representative dates (24th of May: maximum defoliation; and 29th of August: maximum PM infection). To address overdispersion and/or excess of zeros we used negative binomial response distribution for potted seedlings (in SAS) and zero inflated negative binomial response distribution for natural seedlings (in R) in both cases with log link function. We log transformed seedling height to include it as a covariate.
The leaf regeneration capacity from secondary bud flushes was investigated from the 24th of May to the 20th of June in potted seedlings, when defoliating insects affected to a greater extent. We used GLMM to test for differences between species and treatments by computing all the LRC data together, irrespective of date of data capture. To address overdispersion and excess of zeros we used a zero inflated negative binomial response distribution with log link function.
To test for differences between species and CO2 concentration in the severity of PM infection throughout the experimental period we used generalized linear mixed models with a Gaussian distribution and identity link function.
We fitted LMM in SAS to explore variations in growth (D) over time, to analyse RGR for the whole experimental period (i.e., RGR98) and to analyse leaf physiological variables An and Fv/Fm. Due to the impossibility of measuring immature leaves or intensively defoliated leaves, sample sizes were small for some measurement campaigns, and for this reason An was not analysed in late August. To avoid computational problems, An and Fv/Fm were analysed separately at each measurement campaign. Table 1 shows the number of seedlings used for the analysis of physiological variables at each campaign by treatment.
To simplify interactions between fixed effects, we separately explored differences between potted or natural seedings and between species using two-way ANOVAs by means of the models specified above for each variable.
For each of the models described above, we specified an array-specific random effect if the corrected Akaike Information Criterion (AICC) was minimized and used Tukey’s-Kramer’s adjustment for multiple comparisons between unequal sample sizes.
Model residuals were visualised to check whether assumptions were satisfied. We used the package DHARMa when models were fit by R.
Different models were used to explore the effect of eCO2 on multiple response variables. Table 2 presents information about the type of model used to fit each response variable, the goodness of fit, and the statistical significance of the explanatory variables. Table 2 is complemented by figures representing each studied variable at each corresponding subsection. The presentation of results is structure to align with the chronological order of leaf events observed during our study.
The timing of leaf emergence was significantly different between species (P < 0.0001; Figure 1). Post hoc pairwise comparison classified hawthorn in the group (A) as the earliest species to emerge, while oak leaves developed latest (C). Hazel and sycamore occupied an intermediate position (B). Due to the characteristics of this variable, we conducted subset analyses for each species (simple least squares means, Supplementary Table 2), we found a faster leaf development of oak under eCO2 compared to ambient CO2 concentration (P = 0.0209), while the rest of the species did not show differences in leaf development between treatments.
Figure 1. Leaf development of potted seedlings under ambient (a) and elevated (e) CO2 concentrations of the 4 deciduous species. Horizontal axes represent leaf developmental stages: from I -seedlings without developed buds- to V -seedlings with fully developed leaves-. Vertical axes represent number of seedlings counted in each developmental stage at each date. Capital letters represent differences between species. Symbol * represents differences between treatments. Hawthorn: Crataegus monogyna; Hazel: Corylus avellana; Holly: Ilex aquifolium; Oak: Quercus robur; Sycamore: Acer pseudoplatanus.
The two-way ANOVA showed that natural seedlings of oak, hazel and sycamore had lower total LLPM than potted seedlings (P < 0.0001; Figure 2).
Figure 2. Temporal variation in loss of leaf photosynthetic material (LLPM) throughout the experimental period under ambient (A) and elevated (E) CO2 concentrations. Vertical solid lines represent standard error of the mean. Vertical dash lines represent key dates at which statistical analyses were conducted (May 24th: maximum defoliation; and August 29th: maximum powdery mildew infection). Capital letters represent the post hoc grouping by species in each analysed date. Different letters indicate differences between species for a same scoring campaign. Hawthorn: Crataegus monogyna; Hazel: Corylus avellana; Holly: Ilex aquifolium; Oak: Quercus robur; Sycamore: Acer pseudoplatanus.
For natural seedlings, LLPM on the 24th of May 2019, corresponding with the insect herbivory peak before regrowth (Figure 2), was significantly different between species (P < 0.0085), but not between CO2 treatments for any species. Figure 2 also shows post hoc pairwise comparisons of means between species represented by capital letters, where oak and sycamore are classified (in descending order) in a different group (A) to holly (B), while hawthorn and hazel occupied an overlapping position (AB).
For potted seedlings, LLPM again differed between species (P < 0.0001), but not CO2 treatments. Post hoc pairwise comparisons between species, classified oak, hazel and sycamore in a different group (A), followed by hawthorn (B) and holly (C). LLPM increased 11-fold in potted oak compared to natural oak, reaching the maximum under ambient conditions (Table 3). On this campaign, mean LLPM in natural oak, sycamore and hazel seedlings ranged from 6.8% to 10% while in potted seedlings it ranged from 42.6% to 81.4%.
On the 29th of August, corresponding with the powdery mildew infection maximum, significant differences were found between species (P = 0.0003), as well as a significant species × treatment interaction in natural seedlings (P = 0.0136). Post hoc pairwise comparisons between species, classified natural oak and sycamore in a different group (A) to holly (B), while hazel and hawthorn remained in an overlapping position (AB). The interaction effect was due to a slightly higher LLPM in hawthorn under ambient CO2 than under eCO2 but it was not significant in the post hoc pairwise comparisons (Table 3). Although pairwise comparisons did not show differences between treatments, natural seedlings, including oak, exhibited the largest LLPM in both May and August under eCO2.
For potted seedlings, LLPM again differed between species (P < 0.0001), but not CO2 treatments. On the 29th of August, potted seedlings demonstrated a similar grouping pattern in post hoc pairwise comparisons among species to that found on the 24th of May. We found a significant effect of the covariate plant height (P = 0.002), the more vigorous seedlings showed higher impact of PM (Supplementary Figure 2).
We assessed the LRC27 in potted seedlings for two consecutive scoring periods (between 24th May and 20th June, a total of 27 days). We found differences between species (P < 0.0001) but no differences between CO2 treatments (Figure 3). Post hoc comparison between species classified holly in a separate group (B) from all other species (A), while post hoc comparisons between species and treatments classified oak under both ambient and eCO2 as the most damage tolerant species in a separated group (Table 3 and Figure 3).
Figure 3. Leaf regeneration capacity (LRC27, mean ± standard error) of the potted seedlings under ambient and elevated CO2 concentrations computed in two time intervals (24-May to 6-June and 6-June to 20-June). Tukey’s post hoc classes are shown as upper case letters above each bar; bars whose labels contain the same letter are not statistically different. Hawthorn: Crataegus monogyna; Hazel: Corylus avellana; Holly: Ilex aquifolium; Oak: Quercus robur; Sycamore: Acer pseudoplatanus.
We assessed the severity of PM infection on natural and potted seedlings. Despite the stems of 4 potted hazels and 3 potted oaks being green, we could not assess the impact of PM due to complete defoliation through herbivory damage. Thus, fully defoliated seedlings and holly (this species did not show infection) were not included in the PM analyses. For naturally regenerated hazel seedlings, the lack of PM infection, and the small sample size under ambient conditions, caused convergence problems and so was also not included into the model fitting.
Natural seedlings exhibited less severe PM infection than potted seedlings (P < 0.0001). Natural hawthorn and hazel did not exhibit any visible symptom of PM at any scoring date, while potted seedlings did (Figure 4).
Figure 4. Powdery mildew infection in (A) natural seedlings and (B) potted seedlings under ambient (a, black bars) and elevated (e, grey bars) CO2 concentrations. Horizontal axes represent infection classes: 0 (0% infection), 1 (<50% infected), 2 (51–75% infected), 3 (76–100% infected). Vertical axes represent the number of seedlings counted in each category. Capital letters represent differences between species. Species with the same letter are not statistically different. Hawthorn: Crataegus monogyna; Hazel: Corylus avellana; Holly: Ilex aquifolium; Oak: Quercus robur; Sycamore: Acer pseudoplatanus.
We found differences between species in PM levels on natural seedlings (P < 0.0001), but no differences between CO2 treatments (Figure 4). Post hoc comparisons between species for natural seedlings classified oak and sycamore as the most infected group (A) compared to hawthorn (B).
For the potted seedlings, there were no significant differences in PM between CO2 treatments. Post hoc pairwise comparisons between species classified each species in a different group (in descending order of infection) oak (A), sycamore (B), hawthorn (C), and hazel (D), while significant pair-wise overlaps occurred in the post hoc comparison between species and treatment (Table 3).
We did not find statistical differences between potted and naturally regenerated seedlings in An or Fv/Fm.
On the first campaign (24th May), An did not show differences between species or CO2 treatments for either natural or potted seedlings. For this first campaign, the interaction species × treatment in natural seedlings was significant because, contrary to the other species, sycamore showed higher An under ambient CO2 (Figure 5). A more sensitive classification by species and treatments (Post hoc pairwise comparison) is shown in Supplementary Table 3. On the second campaign (10th July), natural hawthorn and sycamore (A) showed statistically higher An than oak (B), while holly remained in an overlapping position (AB) (Figure 5). In the second campaign, potted hazel (A) showed significantly higher An than potted oak (B), the other species remained in an overlapping position (AB). There was a significant positive CO2 effect on An in potted seedlings on the second campaign, corresponding to the most favourable measurement conditions. On the third campaign (29th August) there were no differences in An between species or treatments for both natural and potted seedlings. All seedlings showed lower An compared to the other measurement campaigns. Leaf temperature was higher on this campaign (c. 0.28°C) compared to the other campaigns (c. 21°C). We found a negative correlation between An and T under both eCO2 and ambient conditions for the small range of leaf temperatures studied (data not shown).
Figure 5. Variations in An and Fv/Fm throughout the experimental period (campaign 1: 24-May; campaign 2: 10-July; campaign 3: 24-July; campaign 4: 29-August) under ambient CO2 concentration (A) and elevated CO2 concentration (E). Capital letters inside of the figure represent differences between species in the same campaign. Species whose labels contain the same letter in the same campaign are not statistically different. When the sample size of natural hazel or oak were too small they were not included in the multiple comparison tests. Hawthorn: Crataegus monogyna; Hazel: Corylus avellana; Holly: Ilex aquifolium; Oak: Quercus robur; Sycamore: Acer pseudoplatanus.
Regarding photochemical efficiency as a measure of stress in plants, Figure 5 shows variations in Fv/Fm throughout the experimental period. On the first campaign, corresponding with the maximum defoliation intensity, Fv/Fm was below 0.80 in potted hazel, sycamore and oak as well as in natural hawthorn. Such values indicate that leaves were not fully developed and/or stressed (Maxwell and Johnson, 2000; Murchie and Lawson, 2013). Post hoc pairwise comparisons by species are represented in Figure 5. Overall, holly and hawthorn showed higher Fv/Fm than sycamore, oak and hazel in both potted and natural seedlings. A more detailed analysis by species and treatments is also shown in Supplementary Table 3.
The repeated-measures analyses showed differences in the natural seedling diameters between campaigns (P = 0.0004), although there were no statistical differences between July and August (Figure 6, campaigns 2 and 3). Post hoc pairwise comparison (Supplementary Table 3) classified natural holly and sycamore into the largest diametric group A, while natural oak and hawthorn were classified into the group B. Hazel remained in an overlapping position (AB).
Figure 6. Stem diameter of natural and potted seedlings under ambient CO2 concentration (A) and elevated CO2 concentration (E). Campaign 1: 5th of May in natural seedlings and 24th of May in potted seedlings; campaign 2: 10th of July; Campaign 3: on the 29th of August. Capital letters represent differences between species in the same campaign. Species whose labels contain the same letter in the same campaign are not statistically different. Hawthorn: Crataegus monogyna; Hazel: Corylus avellana; Holly: Ilex aquifolium; Oak: Quercus robur; Sycamore: Acer pseudoplatanus.
For potted seedling diameters, we found that species, treatment, date, and the interaction between species and treatment was highly significant (P < 0.008; Figure 6). Post hoc pairwise comparisons (Supplementary Table 3) broadly followed those for the natural seedlings. Sycamore was classified as the most fast-growing species with the largest diameter (group A), followed by hazel (B), holly remained in an intermediate position between sycamore and hazel (AB), oak was classified into a different group (C), and finally hawthorn was the most slow-growing species, classified into the smallest diameter group (D).
The RGR98 was analysed from May to August (Figure 7). We did not identify any significant CO2 effect for the natural seedlings, but eCO2 significantly increased growth rate for the potted seedlings (P = 0.0223), driven to a large extent by the strong treatment effect on potted hawthorn and oak (Figure 7).
Figure 7. Relative growth rates (RGRd, % day–1) for potted and natural seedlings, calculated as a single finite difference over the whole experimental period (97 days from May to August) under ambient (a) and elevated (e) CO2 concentrations. Plotted are medians (horizontal line), means (black dots), interquartile range (box), upper and lower 5% Ilex (whiskers) and outliers (circles). Hawthorn: Crataegus monogyna; Hazel: Corylus avellana; Holly: Ilex aquifolium; Oak: Quercus robur; Sycamore: Acer pseudoplatanus.
In contrast to Norby et al. (2003), who did not find a significant CO2 effect on the leaf-out phenology of 14-year-old saplings in a deciduous FACE forest, we did find a stimulation by CO2 of the leaf development of potted oak seedlings. Oak seedlings growing under eCO2 reached more advanced developmental stages earlier than those growing under ambient conditions (Figure 1). Whilst we observed an eCO2 treatment effect on plant phenology, the lack of an observed difference in herbivory suggests that eCO2 does not affect palatability at this growth stage. Despite not analysing leaf stoichiometry in this experiment due to lack of leaf material of some seedlings, the C:N ratios of adult oaks do not show an eCO2 effect in the BIFoR-FACE forest (Gardner et al., 2022), supporting our results on invariability of the palability under eCO2.
Of the four deciduous species, hawthorn exhibited the highest resistance to herbivory, showing low defoliation intensity in both natural (3.5%) and potted seedlings (9%) (Figure 2). One explanation is that the early leaf-out phenology of hawthorn was advantageous. Hawthorn leaf-out began significantly earlier, in February, compared to the other species, and leaves were fully expanded by April 2019 (Figure 1, top row), before the insect defoliation peak (Figure 2, left-most column). Oak flushing began significantly later, and even by late April oak leaves were not completely expanded in any of the experimental plots (Figure 1, second row and Figure 2, fourth column from left). As leaves age, they become physically tougher and contain greater concentrations of unpalatable defence compounds (Feeny, 1970; Polgar and Primack, 2011). Consequently, many defoliating herbivores feed primarily on young leaves, which supports the idea that leaf-out phenology influences the extent of herbivory experienced by these tree species. Another explanation is that even though the key defoliating herbivore species across the experimental site are generalists (a survey of insects took place between 2017 and 2019, see Crowley et al., 2023) they do not exert a consistent herbivory pressure on all the tree species (Hittenbeck et al., 2019). We are not able to discriminate between these possibilities.
We found no significant impact of eCO2 on LLPM through insect herbivory for each tree species studied (Figure 2 and Table 2). These results agree with previous findings in Euc-FACE (Gherlenda et al., 2016b) and are consistent with other herbivory studies at BIFoR FACE that found no defining pattern of herbivory change under eCO2 (data not shown). One possible explanation for this, is that all these studies were conducted during the first 3–4 years of fumigation of the BIFoR-FACE, and so impacts on herbivory may yet emerge. Our results show that oak potted seedlings experienced 11-fold higher LLPM due to insect herbivory than natural seedlings (Figure 2, May 24th data point). Indeed, within this experiment, the majority of the species growing in natural conditions were much better able to avoid or to defend themselves against herbivory. In this context, recent research has highlighted the role of interactions with root symbionts and other soil microbes in plants induced resistance against herbivore insects (e.g., Castaño et al., 2020). This complex soil ecosystem was lacking for potted seedlings and suggests delving deeper into these intricate relationships may offer valuable insights into the mechanisms driving the divergent herbivory responses. In addition, our results highlight clear differences in herbivory damage across tree species under all conditions tested, and identifying what factors contribute to these differences is key to predicting future winners and losers given that pest damage is expected to increase with global climate change (Guyot et al., 2016).
In this study, the only evergreen species, holly, did not show symptoms of herbivory or pathogen attack. Low palatability of holly to feeding adult insects (Southwood, 1986) is consistent with the finding of the present study and we did not find literature supporting PM infection on holly. Leaf-mining larvae, known to be present in the BIFoR FACE site, are the main cause of LLPM in holly, however, damage by this feeding guild has been recorded mainly in more mature plants than those seedlings growing at the site, which may explain why no damage was recorded in this study.
Species-specific responses such as compensatory growth to insect herbivory have been found within the BIFoR-FACE forest. Compensatory growth has often been reported as a tolerance strategy after defoliation (Massey et al., 2005; Fuenzalida et al., 2019; Wang et al., 2020b). Defoliation intensity and recovery time play a crucial role in compensatory growth capacity. Oak exhibited the most favourable response to defoliating insects, demonstrating an increase in leaf production through “lammas” shoots and a robust recovery of photosynthetic material (Figures 2, 3). We find no eCO2 effect on compensatory growth (leaf production) for any species, although longer term assessments are required. Successive years of intense defoliation may strongly reduce compensation because of induced morphological changes such as vessel size or defects in secondary xylem (Anttonen et al., 2002). Previous studies have found lower survival rate and performance of younger seedlings subjected to experimental defoliation higher than 50% (Barton, 2016; Lorca et al., 2019).
Within the BIFoR-FACE site, oak showed the most severe PM infection, followed by sycamore. This result is in agreement with the classical theory of the host density effect where higher abundance of a particular tree species is related to higher infection levels (Burdon and Chilvers, 1982). Our results are also consistent with Hantsch et al. (2013), who found a strong species identity effect in Quercus spp. in relation to specialized foliar biotrophic fungi in temperate forests, specifically that the presence of particular disease-prone species explained higher pathogen loads. Unlike other species, mature oak trees show PM infection every year, thus producing a dense pool of spores in the deciduous leaves at ground level to infect seedlings as they emerge the following spring.
The existing studies conducted to investigate the effect of elevated CO2 on the severity of PM are contrasting. For instance, Sanchez-Lucas et al. (2023) found a significant CO2 effect on the severity of PM infection in oak seedlings grown at very high CO2 concentration (1000 ppm) in controlled environment conditions. Whereas Watanabe et al. (2014), who studied photosynthetic traits in a FACE coppice forest of Japanese oak infected by PM, did find lower infection level under eCO2. We did not find a CO2 effect on the severity of PM, however, we found that the more vigorous sycamore and oak seedlings suffered more severe disease (see slopes of these 2 species; Supplementary Figure 2), suggesting that the species in BIFoR FACE traded-off photosynthate between growth and defence differently.
The lowest net photosynthetic rates (An) measured during our study were found in late July (Figure 5), at the same time as the maximum mildew infection. This pattern was observed in all species; therefore, we cannot differentiate whether the decreased An was due to PM or some environmental factor. The combination of decreased photosynthetic activity and loss of photosynthetic material may have critical consequences, especially for oak regeneration. Oak trees produce a part of their annual ring before budburst; therefore, a limited photosynthetic ability can compromise the reserve of carbohydrates required in the next spring (Marçais and Desprez-Loustau, 2014).
Photochemical efficiency (Fv/Fm) is an indicator of the state of the photosynthetic machinery, and a reliable diagnostic indicator of responses to stress (e.g., Maxwell and Johnson, 2000; Molina-Montenegro et al., 2013). Some authors have found a correlation between compensatory photosynthesis in response to leaf damage, for instance Madriaza et al. (2019) found an interesting compensation of Fv/Fm and relative growth rates after defoliation and propose the use of Fv/Fm as an indicator of tolerance to herbivory. In contrast, Amagai and Kudo (2019) found compensation of Fv/Fm after defoliation, but this effect did not influence shoot growth. Our results of Fv/Fm highlighted damage caused by biotic stress in oak, sycamore and hazel, which exhibited low Fv/Fm values throughout the experiment (Figure 5), while the most resistant species, holly and hawthorn, showed higher values of Fv/Fm. Therefore, our experiment did not reveal any indications of compensatory changes in Fv/Fm values. Nevertheless, it is important to note that this effect might have been mitigated by the influence of powdery mildew infection, a factor previously associated with decreased Fv/Fm values (Wang et al., 2014).
In contrast to sycamore, which exhibited the largest diameter of the 5 species, oak and hawthorn showed the smallest diameters at the beginning of the experiment and were characterised as the slowest-growing species. However, hawthorn, one of the species less affected by PM, grew in diameter throughout the experiment, while oak practically did not grow during the PM infection (from July to August) under both natural and potted conditions, likely a direct consequence of nutrient uptake by the biotrophic fungus (Desprez-Loustau et al., 2014; Figure 5). In summary, when considering all the species examined in our study, our results for potted seedlings are consistent with those reported by Watanabe et al. (2014), confirming an increase in An on the second measurement campaign (10th of July), enhance diameter growth and higher RGR98 under elevated eCO2 conditions, regardless of the presence of PM infection (Table 2).
The impacts of the interaction of pathogens and pests on complex forest communities have been previously investigated, e.g., under drought-stress situations (Jactel et al., 2012) or different combinations of tree species and increasing diversity (Field et al., 2019). Very few studies have assessed how CO2 enrichment might alter this interaction under natural conditions in a natural forest (Gherlenda et al., 2016a,b), and no studies to date have investigated how it might impact regeneration of a temperate deciduous mixed woodland. Our results showed that the relationship between biotic stressors and seedlings was mainly driven by tree species and whether they were naturally established or potted. We did not find a CO2 treatment effect on the defoliation intensity nor PM severity even when there was a positive eCO2 effect on foliar growth, as in potted oak seedlings.
The main cause of reduction of leaf photosynthetic material in natural seedlings was PM, representing a leaf loss c. 34% in natural oak and sycamore (Figure 2), and up to 80% in potted oak (Figure 2). Several factors we cannot distinguish between might explain the lower defoliation in naturally regenerated seedlings: epigenetic factors; or the greater distances between seedlings, i.e., “dilution” in the wider environment cf. the potted seedlings (Stastny and Agrawal, 2014).
The earliest leaf flushing species, hawthorn, and the evergreen holly showed fewer symptoms of PM infection and defoliation by insects, therefore their regeneration capacity remains mostly unknown because they were not defoliated. Hazel (potted and natural) showed both good regeneration capacity after intense defoliation close to that of oak, and more resistance to PM infection than oak and sycamore. In the context and with respect to the pressures we have studied, these traits place the regeneration of hazel in a better position compared to oak. Sycamore was more resistant to defoliation and PM than oak. The same high regeneration capacity that confers oak the highest tolerance against insects (“lammas” shoots), also makes this species most vulnerable to PM, since the pathogen benefits from younger tissues (Field et al., 2019). We find no evidence that the regeneration of oak, the dominating species, will be improved under eCO2.
The raw data supporting the conclusions of this article will be made available by the authors, without undue reservation.
CM: Methodology, Software, Validation, Writing – original draft, Writing – review and editing, Conceptualization, Data curation, Formal analysis, Investigation. SI: Writing – review and editing. EL: Writing – review and editing. LC: Writing – review and editing. SH: Writing – review and editing. JS: Writing – review and editing. AM: Investigation, Funding acquisition, Resources, Writing – review and editing.
The author(s) declare financial support was received for the research, authorship, and/or publication of this article. Authors acknowledge support from the UK Natural Environment Research Council [CM and AM: NE/S015833/1 (QUINTUS); EL, SH, and AM: NE/V021346/1]. The BIFoR-FACE facility acknowledges generous underpinning support from the JABBS Trust, Norbury Park Estate, The John Horseman Trust, Ecological Continuity Trust, and the University of Birmingham.
We gratefully acknowledge assistance from Prof. Michael Tausz, the BIFoR-FACE operations team, and Silvia Bugallo, a student on the Erasmus+ program.
The authors declare that the research was conducted in the absence of any commercial or financial relationships that could be construed as a potential conflict of interest.
All claims expressed in this article are solely those of the authors and do not necessarily represent those of their affiliated organizations, or those of the publisher, the editors and the reviewers. Any product that may be evaluated in this article, or claim that may be made by its manufacturer, is not guaranteed or endorsed by the publisher.
The Supplementary Material for this article can be found online at: https://www.frontiersin.org/articles/10.3389/ffgc.2023.1278409/full#supplementary-material
Ainsworth, E. A., and Long, S. P. (2005). What have we learned from 15 years of free-air CO2 enrichment (FACE)? A meta-analytic review of the responses of photosynthesis, canopy properties and plant production to rising CO2. N. Phytol. 165, 351–372. doi: 10.1111/j.1469-8137.2004.01224.x
Amagai, Y., and Kudo, G. (2019). Responses of photochemical efficiency and shoot growth of alpine dwarf-pine Pinus pumila to experimental warming, shading, and defoliation in Japan. Alpine Bot. 129, 43–52.
Anttonen, S., Piispanen, R., Ovaska, J., Mutikainen, P., Saranpää, P., and Vapaavuori, E. (2002). Effects of defoliation on growth, biomass allocation, and wood properties of Betula pendula clones grown at different nutrient levels. Can. J. For. Res. 32, 498–508.
Aranda, I., Cadahía, E., and de Simón, B. F. (2020). Leaf ecophysiological and metabolic response in Quercus pyrenaica Willd seedlings to moderate drought under enriched CO2 atmosphere. J. Plant Physiol. 244:153083. doi: 10.1016/j.jplph.2019.153083
Arzanlou, M., Torbati, M., and Golmohammadi, H. (2018). Powdery mildew on hazelnut (Corylus avellana) caused by Erysiphe corylacearum in Iran. For. Pathol. 48, e12450. doi: 10.1094/PDIS-10-20-2281-PDN
Barton, K. E. (2016). Low tolerance to simulated herbivory in Hawaiian seedlings despite induced changes in photosynthesis and biomass allocation [Article]. Ann. Bot. 117, 1053–1062. doi: 10.1093/aob/mcw021
Bert, D., Lasnier, J.-B., Capdevielle, X., Dugravot, A., and Desprez-Loustau, M.-L. (2016). Powdery mildew decreases the radial growth of oak trees with cumulative and delayed effects over years. PLoS One 11:e0155344. doi: 10.1371/journal.pone.0155344
Bradwell, J., Foyer, C., and MacKenzie, R. (2022). Forest innovation to tackle the climate and biodiversity emergencies. Birmingham: University of Birmingham.
Burdon, J. J., and Chilvers, G. A. (1982). Host density as a factor in plant disease ecology. Annu. Rev. Phytopathol. 20, 143–166.
Castaño, C., Camarero, J. J., Zas, R., Sampedro, L., Bonet, J. A., Alday, J. G., et al. (2020). Insect defoliation is linked to a decrease in soil ectomycorrhizal biomass and shifts in needle endophytic communities. Tree Physiol. 40, 1712–1725. doi: 10.1093/treephys/tpaa104
Chang, H., Han, S. H., An, J., Park, M. J., and Son, Y. (2018). Warming results in advanced spring phenology, delayed leaf fall, and developed abnormal shoots in Pinus densiflora seedlings. Trees-Struct. Funct. 32, 1473–1479.
Che-Castaldo, C., Crisafulli, C. M., Bishop, J. G., Zipkin, E. F., and Fagan, W. F. (2019). Disentangling herbivore impacts in primary succession by refocusing the plant stress and vigor hypotheses on phenology. Ecol. Monogr. 89, e01389.
Ciais, P., Sabine, C., Bala, G., Bopp, L., Brovkin, V., Canadell, J., et al. (2014). “Carbon and other biogeochemical cycles,” in Climate change 2013: the physical science basis. Contribution of Working Group I to the Fifth Assessment Report of the Intergovernmental Panel on Climate Change, (Cambridge: Cambridge University Press), 465–570.
Crowley, L. M., Ivison, K., Enston, A., Garrett, D., Sadler, J. P., Pritchard, J., et al. (2023). A comparison of sampling methods and temporal patterns of arthropod abundance and diversity in a mature, temperate, Oak woodland. Acta Oecol. 118:103873.
Crowley, L. M., Sadler, J. P., Pritchard, J., and Hayward, S. A. (2021). Elevated CO2 Impacts on Plant–Pollinator Interactions: A Systematic Review and Free Air Carbon Enrichment Field Study. Insects 12:512. doi: 10.3390/insects12060512
Damestoy, T., Moreira, X. Q., Jactel, H., Valdes-Correcher, E., Plomion, C., and Castagneyrol, B. (2021). Growth and mortality of the oak processionary moth, Thaumetopoea processionea, on two oak species: direct and trait-mediated effects of host and neighbour species. Entomol. Gener. 41, 13–25.
Daud, R. D., de Cássia Conforto, E., and Feres, R. J. F. (2012). Changes in leaf physiology caused by Calacarus heveae (Acari, Eriophyidae) on rubber tree. Exp. Appl. Acarol. 57, 127–137. doi: 10.1007/s10493-012-9552-y
Desprez-Loustau, M.-L., Saint-Jean, G., Barrès, B., Dantec, C. F., and Dutech, C. (2014). Oak powdery mildew changes growth patterns in its host tree: host tolerance response and potential manipulation of host physiology by the parasite. Ann. For. Sci. 71, 563–573.
DOE (2020). US Department of energy free-Air CO2 enrichment experiments: FACE results, lessons, and legacy. London: DOE.
Du, C. J., Wang, X. D., Zhang, M. Y., Jing, J., and Gao, Y. H. (2019). Effects of elevated CO2 on plant C-N-P stoichiometry in terrestrial ecosystems: A meta-analysis. Sci. Total Environ. 650, 697–708. doi: 10.1016/j.scitotenv.2018.09.051
Duursma, R. A., Gimeno, T. E., Boer, M. M., Crous, K. Y., Tjoelker, M. G., and Ellsworth, D. S. (2016). Canopy leaf area of a mature evergreen Eucalyptus woodland does not respond to elevated atmospheric [CO 2] but tracks water availability. Glob. Change Biol. 22, 1666–1676.
Feeny, P. (1970). Seasonal changes in oak leaf tannins and nutrients as a cause of spring feeding by winter moth caterpillars. Ecology 51, 565–581.
Field, E., Schönrogge, K., Barsoum, N., Hector, A., and Gibbs, M. (2019). Individual tree traits shape insect and disease damage on oak in a climate-matching tree diversity experiment. Ecol. Evol. 9, 8524–8540. doi: 10.1002/ece3.5357
Fuenzalida, T. I., Hernández-Moreno, Á, and Piper, F. I. (2019). Secondary leaves of an outbreak-adapted tree species are both more resource acquisitive and more herbivore resistant than primary leaves. Tree Physiol. 39, 1499–1511. doi: 10.1093/treephys/tpz083
Gardner, A., Ellsworth, D. S., Crous, K. Y., Pritchard, J., and MacKenzie, A. R. (2022). Is photosynthetic enhancement sustained through three years of elevated CO2 exposure in 175-year-old Quercus robur? Tree Physiol. 42, 130–144. doi: 10.1093/treephys/tpab090
Gherlenda, A. N., Esveld, J. L., Hall, A. A., Duursma, R. A., and Riegler, M. (2016a). Boom and bust: rapid feedback responses between insect outbreak dynamics and canopy leaf area impacted by rainfall and CO2. Glob. Change Biol. 22, 3632–3641. doi: 10.1111/gcb.13334
Gherlenda, A. N., Moore, B. D., Haigh, A. M., Johnson, S. N., and Riegler, M. (2016b). Insect herbivory in a mature Eucalyptus woodland canopy depends on leaf phenology but not CO2 enrichment. BMC Ecol. 16:47. doi: 10.1186/s12898-016-0102-z
Glawe, D. A. (2008). The powdery mildews: a review of the world’s most familiar (yet poorly known) plant pathogens. Annu. Rev. Phytopathol. 46, 27–51. doi: 10.1146/annurev.phyto.46.081407.104740
Guyot, V., Castagneyrol, B., Vialatte, A., Deconchat, M., and Jactel, H. (2016). Tree diversity reduces pest damage in mature forests across Europe [Article]. Biol. Lett. 12:5. doi: 10.1098/rsbl.2015.1037
Hantsch, L., Braun, U., Scherer-Lorenzen, M., and Bruelheide, H. (2013). Species richness and species identity effects on occurrence of foliar fungal pathogens in a tree diversity experiment [Article]. Ecosphere 4:12. doi: 10.1890/es13-00103.1
Hart, K. M., Curioni, G., Blaen, P., Harper, N. J., Miles, P., Lewin, K. F., et al. (2020). Characteristics of free air carbon dioxide enrichment of a northern temperate mature forest. Glob. Change Biol. 26, 1023–1037. doi: 10.1111/gcb.14786
Hasegawa, S., Piñeiro, J., Ochoa-Hueso, R., Haigh, A. M., Rymer, P. D., Barnett, K. L., et al. (2018). Elevated CO 2 concentrations reduce C4 cover and decrease diversity of understorey plant community in a Eucalyptus woodland. J. Ecol. 106, 1483–1494.
Hittenbeck, A., Bialozyt, R., and Schmidt, M. (2019). Modelling the population fluctuation of winter moth and mottled umber moth in central and northern Germany. Forest Ecosystems 6, 1–18.
SAS Institute Inc (2016). SAS® 9.4 Language Reference: Concepts, Sixth Edn. Cary, NC: SAS Institute Inc.
Jactel, H., Koricheva, J., and Castagneyrol, B. (2019). Responses of forest insect pests to climate change: not so simple. Curr. Opin. Insect sci. 35, 103–108.
Jactel, H., Nicoll, B. C., Branco, M., Gonzalez-Olabarria, J. R., Grodzki, W., Långström, B., et al. (2009). The influences of forest stand management on biotic and abiotic risks of damage. Ann. For. Sci. 66, 701–701.
Jactel, H., Petit, J., Desprez-Loustau, M. L., Delzon, S., Piou, D., Battisti, A., et al. (2012). Drought effects on damage by forest insects and pathogens: A meta-analysis. Glob. Change Biol. 18, 267–276.
Jiang, M., Medlyn, B. E., Drake, J. E., Duursma, R. A., Anderson, I. C., Barton, C. V., et al. (2020). The fate of carbon in a mature forest under carbon dioxide enrichment. Nature 580, 227–231. doi: 10.1038/s41586-020-2128-9
Karnosky, D., Gielen, B., Ceulemans, R., Schlesinger, W., Norby, R., Oksanen, E., et al. (2001). “FACE systems for studying the impacts of greenhouse gases on forest ecosystems,” in The impact of carbon dioxide and other greenhouse gases on forest ecosystems, eds D. F. Karnosky, R. Ceulemans, G. E. Scarascia-Mugnozza, and J. L. Innes (Wallingford: CABI Publishing).
Khairi, S., and Preece, T. (1978). Hawthorn powdery mildew: Overwintering mycelium in buds and the effect of clipping hedges on disease epidemiology. Trans. Br. Mycol. Soc. 71, 399–404.
Lanier, L., Joly, P., Bondoux, P., and Bellemère, A. (1976). Mycologie et pathologie forestieres-v. 1: Mycologie forestiere.-v. 2. Paris: Masson.
Le Quéré, C., Andrew, R. M., Friedlingstein, P., Sitch, S., Hauck, J., Pongratz, J., et al. (2018). Global Carbon Budget 2018. Earth Syst. Sci. Data 10, 2141–2194.
Linkosalo, T., El-Khouri, H., Mäkipää, R., Pulkkinen, P., and Juurola, E. (2017). Increased atmospheric CO2 concentration enhances the development of photosynthetic capacity beyond the temperature effect for silver birch in simulated future climate. Scand. J For. Res. 32, 651–657.
Liu, X., Kozovits, A. R., Grams, T. E., Blaschke, H., Rennenberg, H., and Matyssek, R. (2004). Competition modifies effects of enhanced ozone/carbon dioxide concentrations on carbohydrate and biomass accumulation in juvenile Norway spruce and European beech. Tree Physiol. 24, 1045–1055.
Lorca, E. A., Ferreras, A. E., and Funes, G. (2019). Seed size and seedling ontogenetic stage as modulators of damage tolerance after simulated herbivory in a woody exotic species. Austral. J. Bot. 67, 159–164.
Madriaza, K., Saldaña, A., Salgado-Luarte, C., Escobedo, V. M., and Gianoli, E. (2019). Chlorophyll Fluorescence May Predict Tolerance to Herbivory. Int. J. Plant Sci. 180, 81–85.
Marçais, B., and Desprez-Loustau, M.-L. (2014). European oak powdery mildew: impact on trees, effects of environmental factors, and potential effects of climate change. Ann. For. Sci. 71, 633–642.
Martins, C. S., Nazaries, L., Delgado-Baquerizo, M., Macdonald, C. A., Anderson, I. C., and Singh, B. K. (2021). Rainfall frequency and soil water availability regulate soil methane and nitrous oxide fluxes from a native forest exposed to elevated carbon dioxide. Funct. Ecol. 35, 1833–1847.
Massey, F. P., Press, M. C., and Hartley, S. E. (2005). Long- and short-term induction of defences in seedlings of Shorea leprosula (Dipterocarpaceae): Support for the carbon: Nutrient balance hypothesis [Article]. J. Trop. Ecol. 21, 195–201. doi: 10.1017/s0266467404002111
Maxwell, K., and Johnson, G. N. (2000). Chlorophyll fluorescence—a practical guide. J. Exp. Bot. 51, 659–668. doi: 10.1093/jxb/51.345.659
Mayoral, C., Strimbeck, R., Sánchez-González, M., Calama, R., and Pardos, M. (2015). Dynamics of frost tolerance during regeneration in a mixed (pine–oak–juniper) Mediterranean forest. Trees 29, 1893–1906.
Medlyn, B. E., Zaehle, S., De Kauwe, M. G., Walker, A. P., Dietze, M. C., Hanson, P. J., et al. (2015). Using ecosystem experiments to improve vegetation models. Nat. Clim. Change 5, 528–534.
Molina-Montenegro, M. A., Torres-Díaz, C., Gallardo-Cerda, J., Leppe, M., and Gianoli, E. (2013). Seabirds modify El Niño effects on tree growth in a southern Pacific island. Ecology 94, 2415–2425.
Morecroft, M. D., Stokes, V. J., Taylor, M. E., and Morison, J. I. (2008). Effects of climate and management history on the distribution and growth of sycamore (Acer pseudoplatanus L.) in southern British woodland in comparison to native competitors. Forestry 81, 59–74.
Moriondo, M., Orlandini, S., Giuntoli, A., and Bindi, M. (2005). The effect of downy and powdery mildew on grapevine (Vitis vinifera L.) leaf gas exchange. J. Phytopathol. 153, 350–357.
Murchie, E. H., and Lawson, T. (2013). Chlorophyll fluorescence analysis: a guide to good practice and understanding some new applications. J. Exp. Bot. 64, 3983–3998. doi: 10.1093/jxb/ert208
Norby, R. J., and Zak, D. R. (2011). Ecological lessons from free-air CO2 enrichment (FACE) experiments. Annu. Rev. Ecol. Evol. Syst. 42, 181–203.
Norby, R. J., Jastrow, J. D., Miller, M. R., Matamala, R., Boutton, T. W., Rice, C. W., et al. (2005). Elevated atmospheric carbon dioxide increases soil carbon. Glob. Change Biol. 11, 2057–2064.
Norby, R. J., Sholtis, J. D., Gunderson, C. A., and Jawdy, S. S. (2003). Leaf dynamics of a deciduous forest canopy: no response to elevated CO 2. Oecologia 136, 574–584. doi: 10.1007/s00442-003-1296-2
Norby, R. J., Wullschleger, S. D., Gunderson, C. A., and Nietch, C. T. (1995). Increased growth efficiency of Quercus alba trees in a CO2-enriched atmosphere. N. Phytol. 131, 91–97. doi: 10.1111/j.1469-8137.1995.tb03058.x
Nowak, R. S., Ellsworth, D. S., and Smith, S. D. (2004). Functional responses of plants to elevated atmospheric CO2–do photosynthetic and productivity data from FACE experiments support early predictions? N. Phytol. 162, 253–280.
Pérez-Ramos, I. M., Cambrollé, J., Hidalgo-Galvez, M., Matías, L., Montero-Ramírez, A., Santolaya, S., et al. (2020). Phenological responses to climate change in communities of plants species with contrasting functional strategies. Environ. Exp. Bot. 170:103852.
Polgar, C. A., and Primack, R. B. (2011). Leaf-out phenology of temperate woody plants: From trees to ecosystems. N. Phytol. 191, 926–941.
Pureswaran, D. S., Roques, A., and Battisti, A. (2018). Forest insects and climate change. Curr. For. Rep. 4, 35–50.
R Core Team (2022). R: A language and environment for statistical computing. Vienna: R Foundation for Statistical Computing.
Roberts, A. J., Crowley, L. M., Sadler, J. P., Nguyen, T. T., Gardner, A. M., Hayward, S. A., et al. (2022). Effects of elevated atmospheric CO2 concentration on insect herbivory and nutrient fluxes in a mature temperate Forest. Forests 13:998.
Robinson, E. A., Ryan, G. D., and Newman, J. A. (2012). A meta-analytical review of the effects of elevated CO2 on plant–arthropod interactions highlights the importance of interacting environmental and biological variables. N. Phytol. 194, 321–336. doi: 10.1111/j.1469-8137.2012.04074.x
Ryan, M. G. (2013). Three decades of research at Flakaliden advancing whole-tree physiology, forest ecosystem and global change research. Tree Physiol. 33, 1123–1131. doi: 10.1093/treephys/tpt100
Sanchez-Lucas, R., Mayoral, C., Raw, M., Mousouraki, M., and Luna, E. (2023). Elevated CO2 alters photosynthesis, growth and susceptibility to powdery mildew of oak seedlings. Biochem. J. 480, 1429–1443. doi: 10.1042/BCJ20230002
Schowalter, T., Hargrove, W., and Crossley, D. (1986). Herbivory in forested ecosystems. Annu. Rev. Entomol. 31, 177–196.
Stastny, M., and Agrawal, A. A. (2014). Love thy neighbor? Reciprocal impacts between plant community structure and insect herbivory in co-occurring Asteraceae [Article]. Ecology 95, 2904–2914. doi: 10.1890/13-1115.1
Takamatsu, S., Braun, U., Limkaisang, S., Kom-Un, S., Sato, Y., and Cunnington, J. H. (2007). Phylogeny and taxonomy of the oak powdery mildew Erysiphe alphitoides sensu lato. Mycol. Res. 111, 809–826. doi: 10.1016/j.mycres.2007.05.013
Takamatsu, S., Matsuda, S., and Grigaliunaite, B. (2013). Comprehensive phylogenetic analysis of the genus Golovinomyces (Ascomycota: Erysiphales) reveals close evolutionary relationships with its host plants. Mycologia 105, 1135–1152.
Talgo, V., Sundheim, L., Gjarum, H., Herrero, M., Suthaparan, A., Toppe, B., et al. (2011). Powdery mildew on ornamental trees and shrubs in Norway. Eur. J. Plant Sci. Biotechnol. 5, 86–92.
Vanaja, M., Maheswari, M., Ratnakumar, P., and Ramakrishna, Y. (2006). Monitoring and controlling of CO2 concentrations in open top chambers for better understanding of plants response to elevated CO 2 levels. Indian J. Radio Space Phys. 35, 193–197.
Wang, H. J., Wang, H., Ge, Q. S., and Dai, J. H. (2020a). The interactive effects of chilling, photoperiod, and forcing temperature on flowering phenology of temperate woody plants [Article]. Front. Plant Sci. 11:443. doi: 10.3389/fpls.2020.00443
Wang, L.-F., Wang, M., and Zhang, Y. (2014). Effects of powdery mildew infection on chloroplast and mitochondrial functions in rubber tree. Trop. Plant Pathol. 39, 242–250.
Wang, N., Zhao, M., Li, Q., Liu, X., Song, H., Peng, X., et al. (2020b). Effects of defoliation modalities on plant growth, leaf traits, and carbohydrate allocation in Amorpha fruticosa L. and Robinia pseudoacacia L. seedlings. Ann. For. Sci. 77:53.
Watanabe, M., Kitaoka, S., Eguchi, N., Watanabe, Y., Satomura, T., Takagi, K., et al. (2014). Photosynthetic traits and growth of Quercus mongolica var. crispula sprouts attacked by powdery mildew under free-air CO 2 enrichment. Eur. J. For. Res. 133, 725–733.
Keywords: FACE forest, defoliation, trophic, phenology, regeneration, leaf physiology
Citation: Mayoral C, Ioni S, Luna E, Crowley LM, Hayward SAL, Sadler JP and MacKenzie AR (2023) Elevated CO2 does not improve seedling performance in a naturally regenerated oak woodland exposed to biotic stressors. Front. For. Glob. Change 6:1278409. doi: 10.3389/ffgc.2023.1278409
Received: 16 August 2023; Accepted: 25 September 2023;
Published: 17 October 2023.
Edited by:
Pedro Álvarez-Álvarez, University of Oviedo, SpainReviewed by:
Christer Bjorkman, Swedish University of Agricultural Sciences, SwedenCopyright © 2023 Mayoral, Ioni, Luna, Crowley, Hayward, Sadler and MacKenzie. This is an open-access article distributed under the terms of the Creative Commons Attribution License (CC BY). The use, distribution or reproduction in other forums is permitted, provided the original author(s) and the copyright owner(s) are credited and that the original publication in this journal is cited, in accordance with accepted academic practice. No use, distribution or reproduction is permitted which does not comply with these terms.
*Correspondence: Carolina Mayoral, Yy5tYXlvcmFsQGJoYW0uYWMudWs=; A. Robert MacKenzie, YS5yLm1hY2tlbnppZUBiaGFtLmFjLnVr
Disclaimer: All claims expressed in this article are solely those of the authors and do not necessarily represent those of their affiliated organizations, or those of the publisher, the editors and the reviewers. Any product that may be evaluated in this article or claim that may be made by its manufacturer is not guaranteed or endorsed by the publisher.
Research integrity at Frontiers
Learn more about the work of our research integrity team to safeguard the quality of each article we publish.