- 1Department of Nutrition and Dietetics, Faculty of Health Sciences, Iğdır University, Iğdır, Türkiye
- 2Research Center for Redox Applications in Foods (RCRAF), Iğdır University, Iğdır, Türkiye
- 3Innovative Food Technologies Development, Application and Research Center, Iğdır University, Iğdır, Türkiye
- 4School of Applied Sciences, University of the West of England, Bristol, United Kingdom
- 5Laboratory Center of Life Sciences, College of Life Sciences, Nanjing Agricultural University, Nanjing, China
- 6Department of Kinesiology and Outdoor Recreation, Southern Utah University, Cedar City, UT, United States
- 7Molecular Hydrogen Institute, Enoch, UT, United States
The world is confronting numerous challenges, including global warming, health epidemics, and population growth, each presenting significant threats to the stability and sustainability of our planet’s ecosystems. Such issues have collectively contributed to a reduction in agricultural productivity, corresponding with an increase in demand and costs of essential commodities. This critical situation requires more sustainable environmental, social, and technological solutions. Molecular hydrogen (H2) has been suggested as a “green” solution for our energy needs and many health, agricultural, and food applications. H2 supplementation in agriculture may represent a novel and low-carbon biotechnological strategy applicable to the abundant production of crops, vegetables, and fruits in agri-food chains. H2 is a potential green alternative to conventional chemical fertilizers. The use of a hydrogen-rich water irrigation system may also provide other health-related advantages, i.e., decreasing the heavy metal accumulation in crops. By adopting a H2 strategy, crop producers, food processors, and decision-makers can contribute to sustainable solutions in the face of global challenges such as climate change, communicable disease epidemics, and a growing population. The versatile applications of H₂ in agriculture and the wider food industry position it as a uniquely suitable approach to address today’s significant challenges, potentially fostering better crop production and positively impacting the agri-food chain. The present review is timely in combining the latest knowledge about the potential applications of H2 in the agriculture and food industry, from farm to fork.
1 Introduction
Given the perishable nature of crops, fruits, and vegetables, these natural foods experience significant quality losses during postharvest storage and distribution (Singh et al., 2022). It has been reported that more than 1.3 billion tons of food go wasted every year, with the spoilage of fruits and vegetables accounting for almost 40% of these losses (Bhatnagar et al., 2022). Therefore, storing fresh produce presents a significant challenge due to the rapidly occurring physiological changes and susceptibility to microbial contamination and spoilage. Various postharvest methods of preservation, encompassing chemical treatments, application of ozone, controlled atmospheres, and innovative packaging materials, including antimicrobial packaging, coatings, and waxes, are under consideration or in use (Ali et al., 2023). Other methods, including non-thermal technologies, e.g., cold plasma and high-pressure processing (Pasquali et al., 2016; Nasri et al., 2023), are being explored to address postharvest losses. One of the methodologies gaining recognition and importance for preserving food quality is the application of H2.
H2 is a colorless, odorless, and simple diatomic gas composed of two hydrogen atoms. It has emerged as a compelling subject of investigation across diverse scientific disciplines, including energy research, space exploration, climate change, geology, microbiology, biomedicine, agriculture, and food technology (Figure 1). Research on the biomedical and agricultural use of H2 is obscured by the copious research on its applications in the green energy sector. However, it is noteworthy that the research on the biomedical and agricultural benefits of H2 has grown exponentially over the past 16 years (LeBaron et al., 2024).
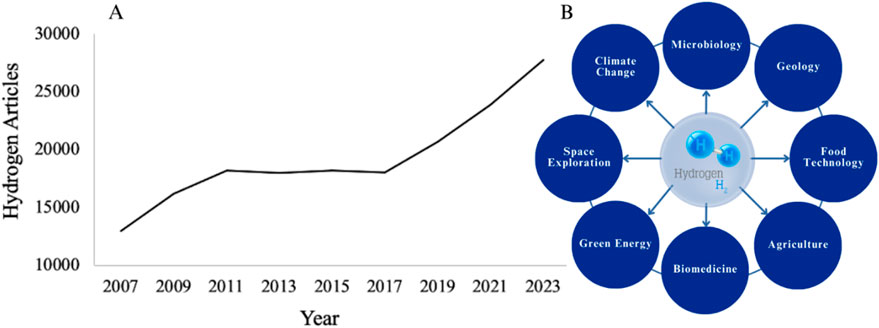
Figure 1. Scientific articles published on H2 since 2007 (A) spanning a variety of academic disciplines (B).
Food security is a major challenge of the present time. Sustainable technologies that improve crop and food production, and storage must be a priority for the future. This is not the first instance H₂ has been proposed as a green alternative to chemical-based agricultural systems (Zulfiqar et al., 2021; Alwazeer and Çiğdem, 2022). However, it is a rapidly advancing field, particularly in China and Japan, making it timely to review the literature and discuss relevant issues. Although numerous reports have explored the potential uses of H2 in agriculture, there is a lack of literature on the potential uses of H2 in food products and technologies.
The novelty of this review lies in exploring H2 as a multifaceted and sustainable solution to contemporary challenges in food and agriculture. The specific aims and objectives of this study are:
Background/Hydrogen Research: With a view to providing a comprehensive overview of hydrogen (H2) research, highlighting its emerging roles across various sectors, we review the historical development of H2 research, current advancements, and key areas where hydrogen is becoming influential.
Hydrogen in Agriculture: Here, the application of hydrogen in agricultural practices is explored and the potential of H2 for enhancing crop yield, improving soil health, and reducing the environmental footprint of agricultural practices is evaluated.
Hydrogen in Food Processing and Packaging: Assessment of the utilization of hydrogen in food processing and packaging industries allows us to analyze how H2 could improve food preservation, extend shelf life, and ensure food safety during processing and packaging.
Hydrogen in the Valorisation of Food Waste: Investigating the role of hydrogen in the valorisation of food waste and exploring the methods by which H2 can be used to convert food waste into valuable products, could help us identify a valuable resource for ensuring waste reduction and improving sustainability in the food industry.
The Bioactivity of Hydrogen: By reviewing existing empirical and theoretical studies on the effects of H2 in biological systems we examine the bioactivity of hydrogen and its potential benefits for increasing harvest yield and preserving foods.
Regulations, Toxicity, and Safety of Hydrogen: Here we provide an overview of current regulations, assessing the safety profile of H2, and identifying any potential risks in its application, highlighting toxicity, and safety considerations associated with hydrogen.
The Current Status of Research and Future Perspectives: To identify gaps in the existing literature, propose future research opportunities, and predict the potential trajectory of hydrogen applications across various sectors, we summarize the current state of hydrogen research and costs, and discuss future directions.
2 Hydrogen research
2.1 H2 and health
In 2007, it was reported that hydrogen gas exhibited selective antioxidant properties in a rat cerebral infarction model (Ohsawa et al., 2007). In this study, a 2% hydrogen gas concentration effectively suppressed brain damage induced by the ischemia/reperfusion injury. The researchers also found that dissolving the H2 gas into cell culture media could selectively reduce strong oxidants such as hydroxyl radicals (•OH) and peroxynitrite (ONOO−). However, H2 did not react with biologically important reactive oxygen species (ROS), including hydrogen peroxide (H2O2), nitric oxide (NO•), or superoxide (O2•-) (Ohsawa et al., 2007), indicating that H2 has selective antioxidant capabilities and may have important medical applications. Moreover, the high bioavailability of this gaseous molecule makes it an attractive candidate for its therapeutic actions. Hydrogen can easily diffuse through cell membranes and reach subcellular organelles due to its small and non-polar nature (Alwazeer et al., 2021).
There are now over 2,000 scientific publications, including 140 human studies, on the biomedical effects of H2, which have been reviewed elsewhere (LeBaron et al., 2024). As stated above, H2 is often administered as HRW. When HRW is ingested, the H2 molecules diffuse through the intestinal submucosa, quickly enter the bloodstream, and appear in exhaled breath within a few minutes (Mikami et al., 2019). This early work on H2 is worth considering for the discussion on agricultural systems. As will be discussed below, the effects of H2 on cellular activities will be similar in plants and animals. Much of the H2 research is on animals and biomedical uses, and much can be learned from this to translate into the plant science arenas.
The consumption of HRW has been widely reported to provide many health benefits including cardiovascular metabolic diseases (Singh et al., 2024). Moreover, the bio-accessibility and bio-availability of nutrients are fundamental properties of foods utilized in metabolic processes. Recent studies propose that drinking hydrogen-rich water after a meal, including fruits, vegetables, meats, and other foods, may enhance the bio-accessibility and bio-availability of various nutrients and vitamins (Alwazeer, 2024a).
2.2 H2 and agriculture
After the promising results of hydrogen’s impact on health, researchers have become increasingly interested in its potential applications in agriculture. Early studies in China have explored the potential uses of H2 in agriculture and its impact on crop production.
2.2.1 Effects of H2 on soil bacteria
Hydrogen is used as an energy source by diverse bacteria in soils for growth and survival (Greening et al., 2022). Soil bacteria drive hydrogen cycling in agricultural systems (Islam Z. F. et al., 2023). Such bacteria are an important sink for atmospheric H2, accounting for ∼75% of H2 uptake. Paradoxically, soil bacteria are also the main contributors to H2 emissions from soil due to the production of H2 by fermentation and nitrogen fixation. Elevated H2 exposure indeed altered soil microbial community structure and diversity (Wang et al., 2020; Xu et al., 2021). As expected, H2 promoted the enrichment of H2-oxidizing bacteria (HOB), some of these species act as plant-growth promoters demonstrated to increase root elongation in wheat seedlings and plant biomass in Arabidopsis (Maimaiti et al., 2007). These HOB isolates exert 1-aminocyclopropane-1-carboxylate (ACC) deaminase activity, phosphorus solubilization, siderophores enhancement, and antifungal activity, leading to plant nutrient absorption and growth (Maimaiti et al., 2007; Fan et al., 2022; Wu et al., 2022).
In addition, Stein et al. (2005) showed an increase in net CO2 fixation in soils was achieved with the addition of H2. Specific marker genes associated with microbial carbon fixation, denitrification, and nitrogen fixation were further observed to be more abundant in H2-treated soils (Xu et al., 2021). In contrast, Wang et al. (2020) reported that the variation in abundance of selected nitrogen-cycling genes was attributed, in part, to significant differences in soil moisture rather than H2 infusion under field conditions. Moreover, soil enzyme activities (Liu et al., 2010) and organic pollutant degradation (e.g., polychlorinated biphenyls) (Xu et al., 2020) were enhanced in response to H2 infusion. Therefore, H2 may influence soil microbial community structure and functions, and may indirectly improve soil health, promoting both plant growth and yield.
2.2.2 Effects of H2 on plant growth and yields
An increasing body of studies show that H2 may play a vital role in plant growth, developmental processes, and stress responses. This indicates that H2 may be an effective alternative or adjunctive treatment to current pesticide and fertilizer inputs, helping to promote nutritional content and yield to a certain extent. In plants, H2 can evolve under normal or various stress conditions resulting from phytohormone induction (Xie et al., 2012; Zeng et al., 2013; Jin et al., 2016). H2 was involved in the signaling pathways of phytohormones (such as auxin, abscisic acid, gibberellin, and salicylic acid (SA)), ROS, NO•, carbon monoxide (CO), hydrogen sulfide (H2S), and melatonin, resulting in improvement of plant rooting and tolerance against various biotic and abiotic stresses that limit crop yield (Xie et al., 2014; Jin et al., 2016; Wu et al., 2020a; Zhang et al., 2020; Su et al., 2021; Shao et al., 2023). For instance, rice stripe virus (RSV) is rice’s most devastating plant virus, leading to severe losses in field production. External H2 supply was observed to confer rice resistance to RSV through an SA-dependent pathway (Shao et al., 2023).
Evidence of the above H2 effects were supported by using transgenic Arabidopsis plants overexpressing a hydrogenase gene from Chlamydomonas reinhardtii (Zhang et al., 2020; Su et al., 2021; Shao et al., 2023). These results indicated that H2 might be a component of the survival strategy of plants through enhancing responses to biotic and abiotic stress. Field trials further confirmed that HRW irrigation could improve the size and weight of rice grains by regulating the transcriptional profiles of representative genes controlling quantitative traits. These include heterotrimeric G protein β-subunit (RGB1), grain size 3/5 (GS3/5), small grain 1 (SMG1), grain weight 8 (GW8), and nitrogen, phosphorus, and potassium assimilation or transport-related genes (Cheng et al., 2021). Recent research has also found that nitrate reductase (NR) might be a target of H2 sensing, positively regulating nitrate uptake and seed size in Arabidopsis thaliana (Cheng et al., 2023). Li et al. (2021b) identified that the fertilization effect of H2 resulted in the proliferation of plant-growth-promoting rhizobacteria, and the enhancement of plant nutrient uptake and use efficiency, indicating that H2 has the potential to be an alternative fertilizer to some extent and, in turn, reducing the use of chemical fertilizers (Li et al., 2021b) (Figure 2).
As can also be seen in Table 1, a range of biological effects is seen in plants when H2 is used as a treatment. The noted effects range from the modulation of seed germination (Xu et al., 2013; Huang et al., 2021), seed size (Cheng et al., 2023), stress tolerance (Zeng et al., 2013), flower senescence (Su et al., 2019), and even postharvest storage of plant materials (Hancock et al., 2022). Therefore, adding H2 to various stages of food production, from adding it to feedwater to gassing food products, may be advantageous.
Whatever the mechanism(s), they need to account for how H2 can lead to many effects in plants, including increased growth and stress tolerance, delayed senescence, and altered gene expression (Figure 3).
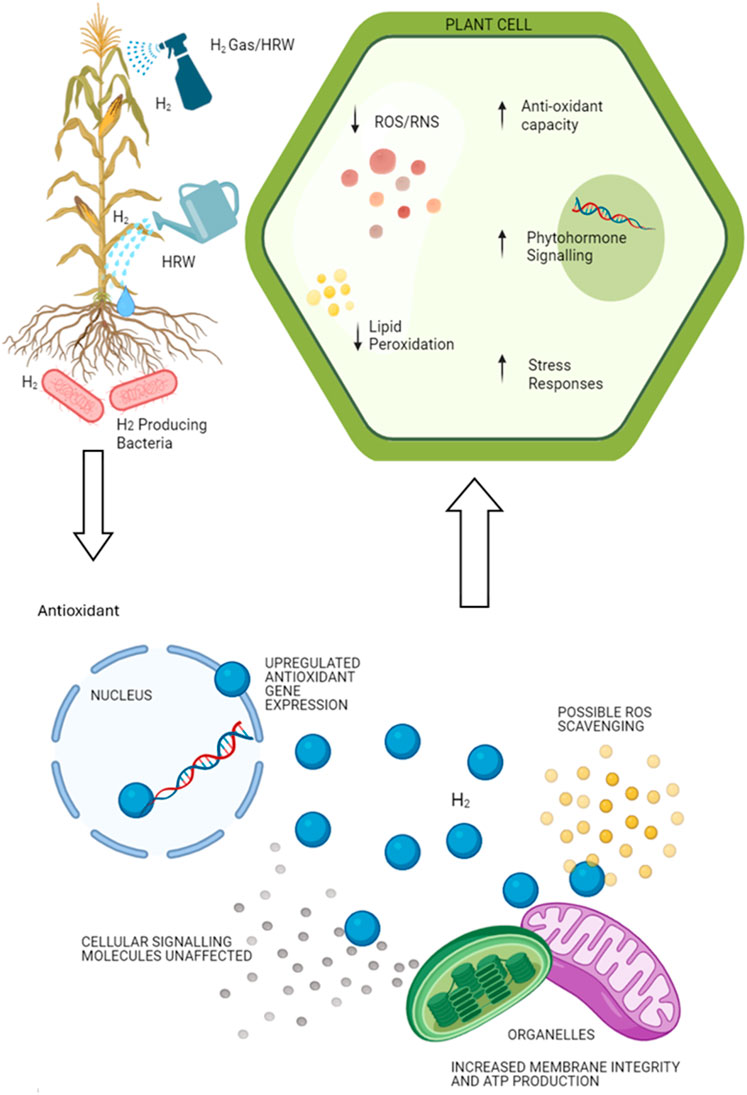
Figure 3. Hydrogen mechanisms in plants. Image created with Biorender.com.
2.2.3 Effects of H2 on heavy metal and nitrogen compound accumulation and toxicity in plants
Excessive use of chemical fertilizers and pesticides has seriously threatened environmental health and food safety (Baweja et al., 2020). As a result, heavy metals accumulate in crop plants, severely threatening human health through food chains. It has been showed that cadmium (Cd) accumulation can be inhibited by H2 in seedlings of alfalfa (Cui et al., 2013), Chinese cabbage (Wu et al., 2015), rapeseed (Zhao G. et al., 2021), and cucumber (Wang et al., 2019). H2-modulated sulfur compound metabolism, redox homeostasis, and Cd transportation were mainly responsible for alleviating Cd toxicity (Cui et al., 2013; Cui et al., 2020; Wu et al., 2021). Furthermore, HRW irrigation was observed to decrease Cd accumulation in rice grains through down-regulating Cd transport-related genes in field trials (Cheng et al., 2021). Besides this, nitrogen fertilizer overuse leads to nitrate and nitrite accumulation. H2 can regulate the expression of nitrate transporter genes (BcNRT1.5 and BcNRT1.8), simultaneously increasing activities of nitrate reductase (NR) and glutamine synthetase, resulting in a decrease in nitrate transport from roots to shoots and, in turn, the decreased accumulation of Cd in Chinese cabbage seedlings (Wei et al., 2021). During the storage of tomato fruits, nitrite accumulation was also prevented by H2, which was attributed to suppressed (NR) or increased (nitrite reductase (NiR)) activities and their corresponding coding genes (Zhang et al., 2019). In addition to the accumulation of Cd through excessive use of fertilizers, pesticide residues are another major threat to food safety. It has been found that H2 can enhance the degradation of the pesticides chlorothalonil and carbendazim in the leaves of tomato seedlings, but importantly this does not reduce their antifungal efficacy (Wang et al., 2022; Zhang et al., 2022). Further pharmacological and genetic evidence has confirmed the contribution of H2-stimulated brassinosteroids (Wang et al., 2022) and glutathione (Zhang et al., 2022) in the above responses.
2.2.4 Effects of H2 on secondary metabolism
With improving living standards, consumers are paying closer attention to the quality of agricultural products. While the use of fertilizers and pesticides boosts crop productivity, these methods can simultaneously diminish the quality of the crops. Phenylpropanoid metabolism is one of the most important secondary metabolic pathways in plants. The products of these processes include anthocyanin, flavonoids, and phenolics known to contribute to plant development and environment interplay, as well as food quality and health-promoting actions. Studies have shown that H2 increased anthocyanin and flavonoid accumulation in radish sprout hypocotyls and alfalfa seedling leaves under UV-A and UV-B, respectively, by regulating the expression of genes associated with phenylpropanoid metabolic pathway (Su et al., 2014; Xie et al., 2015). Metabolomic and transcriptomic profiles revealed that transcription factor genes related to phenylpropanoid biosynthesis and metabolism (including MYBs, bHLHs, and WRKYs) were also involved in H2-increased coumarins and flavonoids accumulation in Wuzhimaotao (Ficus hirta Vahl; a common Chinese herbal medicine) (Zeng and Yu, 2022).
Further field trials confirmed that HRW irrigation enhanced strawberries’ specific volatile compound levels, sugar-acid ratio, and sensory attributes, with/without fertilizer application (Li L. et al., 2022). Notably, the adverse effects of fertilizers on strawberry fruit aroma were alleviated after HRW irrigation.
2.2.5 Effects of H2 on nutrient levels in plants
HRW irrigation also increased the content of some nutrient elements, including phosphorus, potassium, magnesium, and iron, in white rice (Cheng et al., 2021). After 1 year of storage, the HRW-irrigated rice had higher levels of essential amino acids, especially lysine, and lower off-flavors (Cai et al., 2022). Therefore, H2 supplementation may represent a novel and low-carbon strategy applicable to enhancing the production and preservation of crops, vegetables, and fruits in the agri-food chain.
2.2.6 Further thoughts on H2 effects on plants
It is worth noting that H2 effects may vary depending on the species, genetic background, and original habitats of plants (Li L. et al., 2022). Although many unknowns exist regarding the effects of extra H2 input on plants and soils and how agricultural practices affect them, there is currently no evidence that excess H2 causes adverse effects on plant physiology and soil microbial community composition. It will be crucial to consider the optimal H2 dosage in future agricultural applications, mitigating any potential contribution to an increased proliferation of H2-oxidising plant pathogens or the inhibition of plant growth and development. Furthermore, considering the optimal dosing with H2 will also help to minimize the cost/benefit of its use and, therefore, encourage its uptake in the agricultural industries.
2.3 H2 and food
The idea that H2 can exert biological effects conoincides with an evolutionary perspective. For example, the role of hydrogen gas in microbiology has played a key role in both the genesis of life billions of years ago as well as in the evolution of prokaryotes and eukaryotes (Martin and Müller, 1998). Some microorganisms (e.g., algae, archaea, bacteria, protozoa) and most plants contain hydrogenase enzymes that can generate or consume molecular hydrogen. A mechanism which is suggested to play a role in maintaining redox homeostasis (LeBaron et al., 2023). This evolutionary insight opens up intriguing possibilities for modern applications, from enhancing plant growth in agriculture to improving food packaging technologies. Understanding the historical and evolutionary context of hydrogen’s biological effects enriches our appreciation of its potential and guides future research and application strategies.
Although the use of H2 in biology, foods, and agriculture is recent, the biological effects of hydrogen were reported in the late 18th century (Hancock and LeBaron, 2023; LeBaron et al., 2023). Hydrogen-enriched water, in the form of electrolyzed water, was first documented in an agriculture as early as 1931 (Hancock and LeBaron, 2023). This historical context underscores a longstanding interest in harnessing hydrogen for various biological applications.
In food technology, H2 was first introduced in the hydrogenation process of vegetable oils to produce vegetable shortening and margarine (Coenen, 1976). Apart from this use, no other application of hydrogen could be found in the food industry field. This may be because H2 is chemically inert under standard conditions, and safety concerns are related to forming explosive mixtures with air.
Hydrogen studies in microbiology started in the second half of the 20th century. The effect of H2 on microbial growth and heat resistance first appeared in scientific literature in the 1980s.
In 1983, in the United States, two researchers from the Department of Food Science and Nutrition, University of Minnesota, studied the effect of different gases, including H2, on the germination of Clostridium botulinum spores and found H2 reduced the oxidoreduction potential in the experimental system (Foegeding and Busta, 1983). In 1990, another United States team from the University of Wisconsin revealed that H2 could accelerate the thermal inactivation of Clostridium botulinum II3B spores (Kihm and Johnson, 1990). Further to this, in 1998, a research team from the Institute of Food Research, United Kingdom, used H2 in a study regarding the effect of oxidoreduction potential (redox) on the recovery of sub-lethally heat-damaged cells of different pathogens, including Escherichia coli O157:H7, Salmonella enteritidis, and Listeria monocytogenes (George et al., 1998), identifying that the heat resistance of all studied bacteria was higher in an H2-included atmosphere than in an O2-included one.
Early studies on the potential use of H2 in the food industry appeared in the 1990s in France when researchers in the Microbiology Laboratory of ENSBANA, Université de Bourgogne, supervised by the French microbiologist Charles Diviès, were examining the effect of redox potential on the metabolism processes of Lactic Acid Bacteria (LAB). As the LAB is used for the production of different dairy products, Diviès’s team planned to evaluate the impact of redox potential on the microbial quality, aroma, and shelf life of these products. As the region where the Diviès laboratory is located, i.e., Bourgogne, is famous for its wine, the team researched redox’s impact on the growth and the metabolism of Saccharomyces cerevisiae (Capelle, 2001). Shortly after, the research topics were expanded to include new food product groups such as ready-to-eat salads (Ouvry, 2001; Ouvry et al., 2002), fruit juice (Alwazeer, 2003; Alwazeer et al., 2003), pickles (Olsen, 2008), wine (Pham et al., 2008), dairy beverage (Giroux et al., 2008), milk gel (Martin et al., 2009), yogurt (Martin et al., 2010; 2011), and fermented milk (Ebel et al., 2011). In these different research topics of redox, hydrogen gas was used as a redox modifier (reducing agent) of the medium/product.
The aim of Diviès’s team was not to study the impact of hydrogen but instead change the redox potential of the medium by sometimes using gases, such as H2 and N2, and other times chemicals such as dithiothreitol, sodium borohydride, and ferricyanide. Hydrogen was used as a reducing agent to lower the redox value of the medium while oxidizing agents such as O2 and ferricyanide were used to raise it.
Since 2017, Alwazeer, a member of Diviès’s team, has created a new hydrogen research group in Turkey and has been exploring new food products/processes. The driving force of the new team was the promotion of the results of hydrogen’s effect in health and agriculture fields. In 2017, Alwazeer’s hydrogen team started to explore the potential applications of H2 in different food technologies, including drying, packaging, safety, and phytochemical extraction (Figure 4).
3 Hydrogen as a sustainable strategy for agriculture
There is no doubt that plants can respond to H2; indeed, it has been suggested to be suitable and advantageous for wide use in agriculture (Li et al., 2021a).
3.1 Methods of H2 application in agriculture
Exposure and treatment of plants with H2 can be carried out in a variety of methods, from H2-producing bacteria (Maimaiti et al., 2007), using it as a gas, having water or aqueous feed enriched with H2 (hydrogen-rich water (HRW)), or creation of nanobubbles (hydrogen nanobubble water (HNW)), as outlined in Table 2. Each of these has its benefits but also issues. For example, H2 gas is very light and will rapidly rise in the atmosphere, limiting the exposure time at ground level. This presents challenges for fumigation, particularly over large areas, as H₂ would dissipate too quickly in an open field. In enclosed environments, such as greenhouses, the flammability of H₂ in air poses significant safety concerns. However, as H₂ becomes more prevalent as a fuel, future protocols are expected to mitigate these flammability issues.
3.1.1 Treatment with hydrogen-rich water (HRW)
Cells need to be exposed to H2 to have action, which poses challenges for H2 use in agriculture. It is relatively easy to deliver H2 to plants in the form of HRW (or HNW), as it can be sprayed onto foliage or straight on the soil as feed water. However, H2 may not stay in solution very long (Russell et al., 2024), and therefore, using HRW (or HNW) may have a short timeframe for facilitating H2 exposure.
HRW is relatively unstable, and H2 will rapidly enter the gas phase and limit the shelf-life of the HRW solution and, therefore, its effectiveness, as mentioned above. A variation of this is to use hydrogen-rich saline (HRS), which has been used in animal research (Zheng et al., 2009) but may be helpful for salt stress experiments in plants (e.g., Wu et al., 2020b). However, the longevity of HRS is still an issue. Nanobubble technology will partly mitigate the stability of hydrogen-rich solutions, but it is still not stable in the long term (Russell et al., 2024).
3.1.2 Hydrogen-producing nanoparticles
H2 can be supplied using donor molecules, such as ammonia borane-loaded hollow mesoporous silica nanoparticles (AB@hMSN), as used by (Wang et al., 2021) on tomatoes. Alternatively, magnesium hydride (MgH2) can be used, as reported, for the treatment of cut flowers (Nguyen and Lim, 2022). However, the use of such materials brings challenges, too. However, if donor molecules are used, by-products will be left behind, either in the plant or the environment, and such by-products may be inherently toxic. Therefore, spraying large quantities of such donor molecules onto field crops may not be desirable. It is worth noting here, too, that the use of donor molecules is most likely to cost more, and therefore, the overall cost/benefit analysis may be less financially favorable, if favorable at all.
Therefore, at least in the near future, the most likely method for H2 application in agriculture is going to be HRW or HNW. HNW can be produced quite simply. It has been reported that H2 delivered to solution through a bamboo cane (Shin et al., 2016) can create nanobubbles, which increases the persistence time of H2 in solution, making it more desirable as a delivery system. However, in the future, safe and easy-to-use H2 donors may be developed, which will increase the effectiveness of H2 treatments, ease their delivery, and potentially provide a better cost/benefit outcome, although sustainability issues with donor molecules will also need to be considered. For any method adopted in agriculture, the cost/benefit analysis must make its use attractive. However, as the manufacturing, usage, and storage of H2 becomes more common and less expensive, its use in biological systems, such as in agriculture and medicine, may increase. For example, in addition to its potential use both pre- and postharvest, H2 is being explored as a vehicle fuel source (Singla et al., 2021), which may reduce the emissions from, and the costs associated with, fuel consumption when transporting perishable goods. It is clear that H2 has many positive effects on plants and food products, and its use is safe for human health as long as the safety of H2 is considered.
3.2 H2 and the preservation of postharvest crops
As has been discussed above, H2 may be used as a gas-enriched water solution, referred to as hydrogen-rich water (HRW), and has been utilized widely (Table 3). One of the most potentially exciting uses of H2 is in the storage of postharvest plant materials, such as fruit and vegetables.
Hu et al. (2014) studied the effects of HRW on prolonging the shelf life of kiwifruit and the underlying mechanisms. Their study showed that pre-treatment with 80% HRW (0.6 mM) could effectively reduce the rot incidence and inhibit postharvest kiwifruits’ respiration intensity. Specifically, the 80% HRW treatment delayed the decrease in kiwifruit firmness by alleviating pectin solubilization and suppressing cellulase, polygalacturonase, and pectin methylesterase enzyme activities. Additionally, the HRW treatment reduced lipid peroxidation by increasing the SOD activity and free radical scavenging activity. Thus, the results demonstrate that HRW treatment could delay fruit ripening and senescence during storage by regulating the antioxidant defense. In a comprehensive study conducted by (Dong et al., 2022), the application of HRW (20%, 60%, 100%; 0.12 mM, 0.36 mM, and 0.6 mM, respectively) was found to influence the preservation of R. sterilis fruit quality. HRW treatment significantly delayed the decline of total acidity (TA), total soluble solids (TSS), and fruit firmness. Additionally, H2 application effectively postponed the onset of decay, weight loss, increased respiration rate, and malondialdehyde (MDA) accumulation. Moreover, there was a concurrent increase in the activities and expression of enzymes associated with antioxidant systems. Further analysis also indicated elevated adenosine diphosphate (ADP) and adenosine triphosphate (ATP) levels in treated fruit. These findings suggest that HRW treatment regulates the antioxidant capacity and energy status during storage at ambient temperature and holds significant promise as a preservation strategy for R. sterilis fruit. Dong B. et al. (2023) conducted further studies exploring the impact of varying concentrations of HRW at 0, 0.2, 0.4, and 0.8 mM on the quality maintenance of fresh-cut Gastrodia elata during storage at a low temperature (4°C). The findings revealed that the application of HRW effectively inhibited the decline in weight loss and the increase in respiration rate. HRW also slowed down the reduction in total acidity and soluble solids over the storage period. Furthermore, the HRW treatment minimized the production of O2•- and H2O2, increasing the activity and expression of various antioxidant-related enzymes. HRW also increased the levels of non-enzymatic antioxidant substances such as ascorbic acid and glutathione (GSH). Concomitant reduction in the activity and expression of peroxidase and polyphenol oxidase were also observed. Moreover, HRW treatment hindered the decline in ADP and ATP content and energy status, and slowed down the reduction in H+-ATPase, succinate dehydrogenase, Ca2+-ATPase, and cytochrome oxidase activities, substantiating that HRW treatment can preserve the postharvest quality of fresh-cut G. elata.
4 Hydrogen in food processing and packaging
The growing awareness of consumers towards the health risks of synthetic food additives has encouraged food processors to look for effective “green” or “natural” innovative alternatives. H2 possesses many specific physical, chemical, and biological properties. The small size, high diffusibility, and chemical neutrality, besides the biological activities of H2, have opened the door for many potential applications in the food industry. In recent years, several studies have been conducted to explore the effect of H2 on the quality, safety, and shelf-life of food products.
4.1 H2 and food drying
In food drying, the inclusion of H2 in the drying atmosphere, besides CO2 and N2 gases called Reducing Atmosphere Drying (RAD), showed a protective effect on the nutritional and sensory properties of many fruits, including apples and apricots (Alwazeer, 2018; Alwazeer and Örs, 2019). The above reports revealed that the use of an H2-incorporated atmosphere in drying fruits allowed the obtaining of dried products with the closest color values (dark–lightness (L*), green–red (a*), blue-yellow (b*) and total color change (∆E)) to the fresh sample compared with freeze, hot air, and vacuum drying methods. Additionally, The antioxidant activity (DPPH and ABTS) and total phenolic and flavonoid contents were the highest in RAD-dried fruit.
4.2 H2 and food packaging
Limited research has focused on directly utilizing pure hydrogen (H2) through the fumigation method as a postharvest treatment. However, Jiang et al. (2021) have studied the postharvest treatment of Chinese chives with H2 using fumigation and proved its potential to effectively regulate the quality of fresh produce during refrigeration storage (Jiang et al., 2021). Their findings showed a significant reduction in decay incidence, weight loss ratio, soluble protein content, ROS production, and a decelerated decrease in total phenolic, flavonoid, and vitamin C contents. These positive outcomes are attributed to the upregulated activities of superoxide dismutase, peroxidase, catalase, glutathione reductase, and ascorbate peroxidase.
In food packaging, the inclusion of H2 in the package atmosphere besides CO2 and N2 (RAP) protected the quality attributes of different foods such as strawberries (Alwazeer and Özkan, 2022), cheese (Alwazeer et al., 2020), and fish (Bulut et al., 2023).
Recognized for their high perishability and short shelf life, strawberries were the subject of examination in the study conducted by Alwazeer and Özkan (2022). The strawberries were packaged under two types of reducing atmospheres [RAP1 (5% CO2, 4% H2, 91% N2) and RAP2 (10% CO2, 4% H2, 86% N2)], two modified atmospheres [MAP1 (5% CO2, 95% N2) and MAP2 (10% CO2, 90% N2)], as well as a control group (air), before being stored for 12 weeks at 4°C. Upon concluding the storage period, RAPs exhibited superior attributes in terms of total soluble solids (TSS), firmness, lightness value (L*), and green-red chromaticity (a*), as well as phenolic and anthocyanin contents and antioxidant activity, surpassing the performance of MAP and the control. RAP2 demonstrated a more pronounced effectiveness in preserving freshness indices than RAP1, while MAP2 outperformed MAP1, with RAP2 as the most effective preservation characteristic.
H2 has also been investigated as a way to enhance the preservation of animal-derived products. The study by Bulut et al. (2023) tested different gas compositions with hydrogen to extend the shelf life of refrigerated rainbow trout. A control group and four distinct treatment samples were employed: Control (air), MAP1 (50% CO2/50% N2), MAP2 (60% CO2/40% N2), RAP1 (50% CO2/46% N2/4% H2), and RAP2 (60% CO2/36% N2/4% H2). In the control group, lipid oxidation levels surpassed acceptable levels after 5 days, contrasting with treatment samples that consistently remained within acceptable limits throughout the storage period. Similarly, acceptable limits for TVB-N were breached after 7 days in the control group, while the treatment containing H2 maintained levels below the acceptable limit. The microbial counts in the control group exhibited a gradual increase, indicative of quality deterioration, whereas the samples under the modified atmosphere experienced limited changes. Microbial levels in the treated samples remained below the acceptable limits until the 10th day, whereas the control samples surpassed the limits by the 3rd day of storage.
Alwazeer et al. (2020) conducted a study investigating the utilization of a gaseous mixture containing H2 for packaging fresh cheese samples. The cheese samples were packaged under different conditions [RAP 1 (90% CO2/6% N2/4% H2), RAP 2 (50% CO2/46% N2/4% H2)], modified atmosphere packaging (MAP) [MAP 1 (90% CO2/10% N2), MAP 2 (50% CO2/50% N2), and MAP 3 (air)], as well as an unpackaged control, all without the use of any preservatives. Subsequently, the samples were stored at + 4°C for 7 weeks. RAP one exhibited color and titratable acidity values closest to those of the fresh sample. The total mesophilic-aerobic bacteria levels varied, with the control samples having the highest and the RAP one samples showing the lowest. Over time, yeast mold counts increased in all sample groups, but the RAP groups consistently exhibited the lowest levels. These findings suggest that introducing H2 either during fumigation or into modified atmospheres holds potential for the agri-food industry, as evidenced by the positive outcomes observed.
4.3 H2 and food safety
In food safety, the H2-incorporated atmosphere provided an additional benefit to the product where H2 exhibited a restrictive effect against the formation of biogenic amines (BAs) in the product, including fish (Sezer et al., 2022), butter (Bulut et al., 2022), minced meat (Çelebi et al., 2024), and red beetroot pickle (Alwazeer et al., 2022a). Another safety concern that was examined was the presence of heavy metals. In the preparation of butter, using HRW in the washing step of cultured raw butter led to a decrease in the content of heavy metals in the product (Alwazeer et al., 2022b). On the other hand, this positive impact of H2 on reducing the heavy metal content in the product has been also shown in animal studies (Köktürk et al., 2022a; Köktürk et al., 2022b).
5 Hydrogen in the valorization of agri-food waste
Due to the negative perception of synthetic food additives among consumers, food processors have started seeking “green” or “natural” alternatives. Despite the fact that these natural options often fall short of synthetic additives in terms of technological and economic efficiency, they remain popular with consumers who prioritize health and sustainability. Furthermore, global warming and the effects of climate change have further driven the need for sustainable practices. This environmental shift has promoted the valorization of underestimated agri-food wastes, decreased crop production, and ultimately increased crop prices. Consequently, the food industry is increasingly motivated to find innovative solutions that align with both consumer preferences and environmental sustainability. To satisfy the consumer, food processors are trying to find an economical source of these “natural” ingredients for additives in the food industry. Importantly, agri-food wastes have been the most attractive sources of “natural” additives due to their low price and high availability.
An extraction process is required to separate phytochemicals from plant materials. Despite the development of many extraction methods, conventional methods like maceration are still used widely due to their low cost and infrastructure demands. Alwazeer’s team has recently developed a hydrogen extraction method by modifying the traditional maceration method. The hydrogen extraction method depends on the infusion of hydrogen gas into the solvent before (or during) immersion of the plant material. This method was examined on different agri-food wastes, showing encouraging results. The extraction of some phytochemicals such as phenolics, flavonoids, anthocyanins, and other antioxidants was enhanced when hydrogen-rich solvent was used instead of pure solvent. The extraction yield of phytochemicals improved for different solvents, including water, ethanol, methanol, and n-hexane. The safe installation of the hydrogen extraction method is possible at the laboratory or plant scale (Alwazeer, 2023).
The amounts of certain flavonoids and non-flavonoids were increased several times when HRW was used as a solvent in the extraction of phytochemicals from different agri-food wastes and by-products, including tomato peels, apple peels, lemon peels, cabbage, and carrots (Alwazeer and Elnasanelkasim, 2023). Similar results could be obtained for by-products such as olive leaves when two types of HRW were tested: hydrogen bubbling (H2 water) and magnesium-water reaction (Mg water) (Alwazeer et al., 2023d). Hydrogen-rich solvent (water, ethanol, methanol) was also evaluated for extracting phytochemicals from red beetroot (Alwazeer et al., 2023b). Moreover, the incorporation of hydrogen into polar and non-polar solvents for extraction of phytochemicals from a fatty by-product, i.e., olive pomace oil, was also examined (Ceylan et al., 2023). Results showed that the incorporation of hydrogen into both methanol and n-hexane led to improved extraction of many phytochemicals, including phenolics, flavonoids, and antioxidants. Similarly, the hydrogen-rich solvent extraction method was evaluated to extract bioactive compounds from another by-product, i.e., propolis (Yurt, 2023). Incorporating hydrogen into water, methanol, and ethanol could yield higher phenolics, flavonoids, anthocyanins, and antioxidants. Hydrogen-rich solvent extraction method was also studied to compare its efficiency for extracting phytochemicals from agri-food waste, i.e., lemon peel, against the effect of temperature (Alwazeer et al., 2023c). The authors report that the hydrogen incorporation into solvents (water, ethanol, and methanol) showed the highest levels of phenolics and flavonoids in hydrogen-rich methanol, followed by hydrogen-rich ethanol at both 25°C and 35°C, with the highest phytochemical extraction found for hydrogen-rich methanol at 35°C. The study concluded that incorporating hydrogen into solvent was more efficient for extracting phytochemicals than increasing the temperature from 25°C to 35°C.
This novel extraction method of hydrogen-rich solvent was compared with the widely used emerging method, i.e., supercritical fluid extraction. Herein, the hydrogen extraction method was more potent for extracting all phytochemical groups from all studied agri-food wastes, i.e., tomato peel, green apple peel, lemon peel, orange carrot, and red cabbage. The hydrogen extraction method could also extract phenolic acids and flavonoids better than supercritical fluid extraction (Alwazeer et al., 2023a). A recently published review discussed the possible mechanisms behind this potent extractability of hydrogen-rich solvent (Alwazeer, 2023). Ryu et al. (2019) examined the superiority of the hydrogen extraction method for phenolic extraction from green tea leaves compared with other gases, including N2, CO2, and air. They found that the physical and morphological modification in the softness and flexibility of the surface of the plant material, examined by scanning electron microscopy, occurred in H2-treated green tea leaves. The hydrogen extraction method was described as being simplistic, low cost, requiring less additional equipment and low energy consumption, non-toxic, and sustainable (Alwazeer, 2024b).
6 Possible mechanisms of hydrogen action
The impact of hydrogen on various biological systems, including animals and plants, has prompted researchers to investigate its potential effects and underlying mechanisms. Despite the many effects of H2 treatment in plants, the exact molecular mechanisms by which H2 acts on plant cells have not been convincingly reported (Table 4). Several mechanisms of H2 action in cells have been mooted, although not supported by experimental data, including acting as a redox couple or directly interacting with hydrophobic pockets in proteins, which has been reviewed recently (Hancock et al., 2022).
6.1 Fe-porphyrin-mediated scavenging of hydroxyl radicals
There are several potential modes of action of H2 in cells. In their 2007 paper, Ohsawa et al. (2007) showed that H2 could remove •OH from solution, but that H2 had no effect on some other reactive signaling molecules such as H2O2 or NO• (Ohsawa et al., 2007). As •OH can act as a cellular signal (Richards et al., 2015), this could account for some of the effects seen. Indeed, many reports have been published showing that the antioxidant capacity of cells increases during H2 treatment, such as during salt stress (Xu et al., 2013; Fu et al., 2020) and nitrogen deficiency (Jiang et al., 2023).
As stated, one of the seminal papers on H2 action was by Ohsawa et al., in 2007, albeit in animal cells (Ohsawa et al., 2007). Subsequent papers have highlighted the role of heme in this process of hydroxyl radical removal. For example, Kim et al. (2022) suggested a mechanism whereby H2 reacts with heme, with the resultant products mediating the scavenging of •OH. More recently, a paper has suggested a similar mechanism (Jin et al., 2023). Here, authors identified that Fe-porphyrin is a target for H2 and that Fe-porphyrin in the free or protein-bound state can use H2 to scavenge hydroxyl radicals. This is a very significant result, as it could explain many of the downstream effects of H2. To illustrate, such a mechanism could lead to the activation of the transcription factor nuclear factor erythroid 2-related factor 2 (Nrf2), as suggested by Ohta (2023), which is known to be involved in oxidative stress responses (Yu et al., 2021) and involves a basic leucine zipper (bZIP) transcription factor. Also of significance in the Jin et al. (2023) paper, it shows that the interaction of H2 and porphyrin can lead to CO2 being converted to CO, and therefore, they suggest that H2 is upstream in CO signaling. This is also interesting as it is known that an enzyme linked to CO metabolism, i.e., heme oxygenase, is a target for H2 effects (Shen et al., 2017).
Despite findings of interactions between H2 and Fe-porphyrin molecules, it is likely that other mechanisms are also at play in order to account for all effects seen in all organisms for which H2 responses have been reported. It is also unlikely that the Fe-porphyrin-mediated scavenging role of H2 would account for all effects seen when, for example, the localization of the production of •OH and the diffusion of H2 from outside the cells - as it is often applied exogenously - are considered. Therefore, other mechanisms of H2 action, such as Fe-porphyrin-mediated CO production or other non-Fe-porphyrin mechanisms, are likely, and several mechanisms are probably being enacted simultaneously.
6.2 H2 modulates the action of heme-oxygenase
One suggested mechanism is that H2 modulates the action of heme-oxygenase, as mentioned above, particularly HO-1 (e.g., Shen et al. (2017)). This would then involve mediating effects by CO, another gas known to have cellular effects (Stucki and Stahl, 2020). However, how H2 would directly influence the activity of HO-1 is unclear. Unlike ROS and RNS, there is unlikely to be a covalent modification of proteins provoked by H2, in comparison to oxidation (by ROS) (Turell et al., 2020) and S-nitrosylation (by RNS) (Feng et al., 2019). This applies to the control of any protein, not just HO-1. The unlikelihood of direct protein modifications by H2 further underscores the attractiveness of the Fe-porphyrin molecule as a target of H2 because such interaction does lead to the production of CO (Jin et al., 2023).
6.3 H2 effects through its redox action
Some proteins rely on their function by having prosthetic groups, and such mechanisms often rely on changes in the redox state of such non-protein groups. A typical example would be the heme prosthetic of globins, cytochromes, etc. H2 can undergo redox reactions itself, and it has been reported that such action can alter the redox poise of Fe in heme groups in bacterial cytochromes, such as cytochrome c3 (Peck, 1959). Although such redox activity was ruled out as accounting for H2 action by others (Ohsawa et al., 2007), not all possible redox interactions have been studied, especially in plants. Therefore, it is too early to rule this out completely (Hancock et al., 2021).
6.4 The molecular spin effects of hydrogen electrons
A proposed, though unproven, mechanism of H2 in biological systems involves its different spin states (Hancock and Hancock, 2018) (Figure 5).
H2 can potentially alter the electron configuration of other molecules, particularly radicals. Thermal polarization favors the triplet (ortho) state, with ∼75% of H2 molecules in this state at room temperature (O’Neill et al., 2023). The ortho spin generates a weak diamagnetic force (Tarzad, 2020), making it unlikely to affect ROS formation directly via transition metal reduction.
The singlet state of H2 may not directly form radical pairs but may have active redox potential through interactions with metalloproteins. Radical pair formation occurs when forward electron translocation is inhibited (e.g., Complex 1, mitochondria), and this contributes to ROS formation (Hore and Mouritsen, 2016). For example, A flavin-superoxide pair with a singlet spin forms F + H2O2, while the triplet state disassociates into free radicals FH• and O2•- (Ramsay and Kattnig, 2022). Should H2 interact with transition metals components of proteins, as suggested by Hancock and Hancock (2018), Kim et al. (2022), Jin et al. (2023), and Ohta (2023), with electrons in the antiparallel configuration, it is possible that the metal group would more easily catalyze the reduction of radicals via reducing the disassociation energy of free H2 (∼4.64 eV–∼2.35 eV). Logically, the H2 radicals could react with radical oxidants such as O2•- and •OH, reducing the pro-oxidation status of cells, but potentially also biologically important radicals including NO•, etc. Therefore, the para (singlet) state, of H2, though less prevalent, may play a significant role, especially in cooler soil temperatures. However, as mentioned previously, this would be regulated by the spatial and temporal availability of the perceived radicals and H2.
Due to the weak diamagnetic forces at play, the triplet state of H2 may influence cellular activities such as cell signaling, gene regulation, and metabolism by stabilizing the protein structure (protein pocket theory) (Hancock et al., 2022; Alwazeer et al., 2023b). H2 might mediate signaling by affecting protein phosphorylation, depending on the protein’s temporal configuration. Hypothetically, while the singlet state molecule could influence redox balance, and the triplet state may support protein function, there is currently no evidence to support this, and the only evidence-based target of H2 remains the Fe-porphyrin target.
6.5 H2 interactions with protein pockets
Regardless of the mechanism(s) used by H2 to bring about biological effects, they have to account for several phenomena. H2 needs to be able to be moved from its administration location (treatment) to the site of action. It needs to initiate a response that is probably far greater than the H2 concentration sensed by the cell, and the response has to last longer than the possible stability of the H2 gas in the solution. Therefore, having a scavenging mechanism where the removal of •OH seems unlikely to be the sole way H2 acts. Although hydroxyl radicals do have a signaling role in plants (Richards et al., 2015), it is probably far less so than other ROS or RNS, many of which appear to have a far greater significance when it comes to signaling, such as NO• and H2O2, whilst neither are reported to be affected by H2 (Ohsawa et al., 2007).
A possible mechanism that could account for many of the phenomena seen when adding H2 to biological systems would be if H2 interacted directly with proteins. In the globin family of proteins, for example, it is known that inert gases can interact with cavities within protein structures. It has even been shown that these gaseous atoms are able to migrate into proteins through cavity structures (Turan et al., 2023). Other inert gases, such as argon, are also known to have biological effects (Ye et al., 2013). It is, therefore, not a major leap of thought that a small, relatively inert molecule such as H2 could also partake in such interactions with proteins (Hancock et al., 2022). More recently, it has also been suggested that such mechanisms may account for some of the effects seen in the presence of ROS and RNS (Hancock, 2023). Therefore, this may be a far more widespread mechanism for the control of protein function than previously thought. H2 being involved here would make much sense and would account for how H2 has such a wide range of effects, can be moved around organisms, and can have effects far after any treatment given.
The mechanism(s) of H2 action also need to account for the plethora of effects seen in plants through many developmental stages, from seed to flowers to fruits, as well as a range of stress responses, from salinity (Yu et al., 2021), heavy metal tolerance (Cui et al., 2014) to drought tolerance (Zhao G. et al., 2021). Therefore, there is almost certainly more than one pathway in which H2 is involved. This makes it more challenging to elucidate, but it could account for the range of effects seen, from being favorable for plants to being able to have therapeutic effects in humans.
7 Regulatory, toxicity, and safety of hydrogen utilization in crop and food production
7.1 Regulations of H2 use
The European Commission has approved hydrogen gas for use in foods and beverages as a food additive with an E949 code for infants and young children at the quantum satis maximum level (Alwazeer, 2024a). Although the effects of H2 are safe (European Commission, 2011; U.S. Food and Drug Administration, 2023), there could be detrimental (or beneficial) effects when H2-producing ingredients are used, whilst some by-products could be harmful. For example, metallic minerals may raise the pH of the water too high or contribute to osmotic stress in the plants. Some ingredients, such as aluminum and other metal hydrides, could be damaging. Additionally, the infusion of pure H2 gas into water sparges out dissolved oxygen gas in the water. Removal of O2 gas could be helpful in certain food preservation and packaging to reduce oxidation; however, its reduction in agricultural water may prove harmful to the plants, which may account for some of the decreased benefits of H2 at higher concentrations.
7.2 Toxicity of H2
The application of H2 as a gas (and therefore, by extension as HRW) appears to be inherently non-toxic and safe for biological materials, as exemplified by its use as a high-pressure gas for divers, which has been used for approximately 80 years (Bjurstedt and Severin, 1962). No ill effects of H2 treatment have been reported for humans, and high concentrations are tolerated as long as oxygen is present, for example, with the use of oxyhydrogen (Russell et al., 2021). However, a high concentration of H2 might have a negative effect on plant growth. Geologists documented that the lands in Brazil, Namibia, and Australia that leak hydrogen are curiously devoid of vegetation (Hand, 2023). Additionally, several studies observed that high concentrations of H2 showed reduced benefits in certain plants, which might be attributed to the different sensitivity of different plants to H2 (Li et al., 2021b). Therefore, the dosage of H2 should be considered in agricultural applications.
7.3 Safety of H2 use
Proper handling precautions and procedures are essential due to hydrogen’s flammability and potential for explosive reactions, i.e., 4%–75% and 18.3%–59% vol with air (Alwazeer, 2019). For instance, hydrogen must be stored in appropriate cylinders that are kept in well-ventilated areas, away from heat sources, ignition, and direct sunlight (Abohamzeh et al., 2021).
Given that H₂ is lighter than air, ensuring proper airflow ventilation is necessary to prevent its accumulation, which could lead to potentially explosive conditions. Equipment, fittings, and connections using H₂ should be equipped with detectors and monitors that can provide early warnings of malfunctions and potential leaks. Pressure regulators and flow meters are also essential, as they can detect sudden pressure changes and help prevent accidents related to faults in H₂-associated equipment. It is also important to ensure that individuals working with hydrogen are thoroughly trained in safe handling, storage, and emergency procedures. For example, workers should be familiar with evacuation protocols and how to handle fires safely. Regular drills and continuous education on the latest safety standards and technologies can further enhance workplace safety. Proper maintenance and adherence to safety guidelines will ensure that hydrogen can be used effectively and safely in food production and processing environments.
8 The current status, costs, challenges, and future prospects of hydrogen-based agriculture and economic prospects
8.1 Current status of H2
The observations and studies discussed so far suggest that H₂ is a promising solution to address challenges in agricultural sustainability. With the global agricultural sector facing issues such as soil degradation, water scarcity, and environmental degradation due to chemical inputs, the adoption of H2-based strategies offers a promising avenue for positive change. For example, by leveraging H2 fertilization techniques, which promote the proliferation of plant-growth-promoting rhizobacteria and enhance nutrient uptake efficiency, farmers can mitigate the reliance on chemical fertilizers while maintaining or improving crop yield and quality (Li et al., 2021b). Furthermore, the integration of HRW irrigation systems holds the potential to enhance nutritional food quality by increasing the content of beneficial phytochemicals in crops, vegetables, and fruits while simultaneously reducing heavy metal accumulation, among other benefits (Wang et al., 2019; Yao et al., 2024).
8.2 Examples of wide-scale use of H2 in agriculture
The application of hydrogen in agriculture has recently started at an economical scale. In China, the field trials of H2-based irrigation are being carried out gradually. For example, Air Liquide and Nanjing Agricultural University established Hydrogen Agricultural Bases for strawberries and tomatoes. It reported an increase in tomato yield by ∼39% and ∼28% in the absence and presence of fertilizers, respectively, by H2-based irrigation (Li et al., 2024). The flavor of strawberries (Li F. et al., 2022) and the nutrient quality of tomatoes (Li et al., 2024) were also improved. The HRW-irrigated strawberries cost up to about 42 USD/kg, while the regular ones are priced at 8–11 USD/kg. Consistently, the yield of Chinese cabbage was increased by 32.7% after HRW irrigation (Liu et al., 2024). Moreover, a good harvest of the HRW-irrigated rice was achieved in the demonstration fields in Shanghai Qingpu District (9.67 ha, an 18.8% increase in yield, in 2022) and Songjiang District (5.33 ha, a 6.9% increase in yield, in 2023), which were widely reported in the media. Based on these results, the rice planting area with HRW irrigation in Songjiang will be expanded to 26.67 ha by 2024. As for the large-scale promotion of H2-based irrigation in rice, fruits, and vegetables, the cost is the main bottleneck. At present, it may cost at least 5.5 USD to inject enough H2 into 0.067 ha mainly due to the equipment and transportation. Since the prospects of H2-based irrigation in increasing the added value of agricultural products are encouraging, governments, enterprises, and scientific research institutions may join forces to overcome difficulties and drive sustainable agriculture.
8.3 Costs of H2 use
With the rapid development of the H2 energy industry, the cost of H2 production is experiencing a significant decline. Currently, more than 95% of commercially produced H2 relies on fossil fuels, priced at 1–2 USD/kg H2 in the United States, Europe, and China, while the cost of green H2 produced through water electrolysis is 5–10 USD/kg H2 (Finke et al., 2021; Pivetta et al., 2022). Scaling up in wind power and photovoltaic technologies is expected to lead to a 30%–80% reduction in the cost of electrolytic H2 production by 2050, bringing it closer to the cost of H2 production from fossil fuels (Finke et al., 2021). Considering the limited consumption of H2, the cost of H2-based food/crop production primarily depends on equipment and labor expenses. Therefore, the technology of H2 supplementation in the agriculture and food industry emerges as both economically viable and practicable.
8.4 Challenges of H2 use
Nevertheless, despite the promising benefits of H2-based agricultural solutions, several challenges need to be addressed to ensure widespread adoption and effectiveness. These challenges include technological readiness, scalability, and regulatory frameworks governing the use of novel agricultural inputs. As discussed, H2 is a gaseous molecule that does not remain in solution indefinitely; thus, point-of-use H2 irrigation needs to be developed to ensure H2 delivery. Such devices are not readily available in the marketplace and thus need to be engineered, especially for large-scale applications. Although we are unaware of any governmental restrictions on molecular hydrogen for agricultural use, different countries may have different approval requirements.
8.5 Future prospects of H2 use in agriculture and food industry
The level of readiness by large-scale farmers to implement hydrogen-based technologies is unknown as there are still many questions that need to be addressed. It is still unclear what method is the most effective, if H2 should be applied to every part of the process, and what the exact cost-benefit analysis is on each possible application and step individually and combined. However, this hasn’t prevented some groups from implementing hydrogen technologies when convenient. The authors are aware of several smaller agricultural outlets in Asia, EU, and United States as well as individuals across the globe with personal gardens/farms that have incorporated H2 in agriculture. At this point, it is clear from the published research that using molecular hydrogen does not impair or reduce crop yields or have other negative effects. Thus, these early adopters are likely to only experience potential benefits, which, over time, should address the cost/benefit analysis that will ultimately pave the way for larger corporations to determine the viability of H2-based agriculture. This will allow stakeholders and engineers to identify key barriers to implementation and develop strategies to overcome challenges to unlock the full potential of molecular hydrogen in promoting agricultural sustainability.
9 Conclusion
In conclusion, the multifaceted potential of molecular hydrogen as a ‘green’ solution extends far beyond addressing energy needs. The critical need for sustainable solutions is paramount in the face of global challenges such as climate change, communicable disease epidemics, and a growing population. The versatile applications of H₂ in health, agriculture, and the food industry position it as a uniquely suitable approach to address today’s significant challenges, fostering abundant crop production and positively impacting the agri-food chain. Hydrogen’s role as a green alternative fertilizer is underscored by its ability to promote plant growth, enhance nutrient uptake, and reduce reliance on chemical fertilizers. Although there have been discussions about using H2 in agriculture, no reports about its potential application in the wider food industry are available in the literature.
Applying hydrogen-rich water in irrigation can offer health-related benefits by mitigating heavy metal accumulation in crops. In the food industry, adopting hydrogen-based strategies may be instrumental in preserving nutritional and sensory properties, reducing heavy metal content, limiting biogenic amine formation, increasing microbiological safety, and extending product shelf life. Embracing hydrogen within a sustainable development framework can ensure the production of safe, high-quality products, fostering a holistic approach from the field to the fork. While, it is important to consider the challenges and costs associated with implementing hydrogen-based solutions on a large scale, the potential benefits are significant. Further research and development are also necessary to optimize the hydrogen application methods according to the specifications of each crop/product and to ensure they are economically viable and widely accessible to farmers and food producers.
Author contributions
DA: Conceptualization, Data curation, Investigation, Methodology, Project administration, Resources, Software, Supervision, Visualization, Writing–original draft, Writing–review and editing. JH: Conceptualization, Data curation, Investigation, Methodology, Resources, Software, Visualization, Writing–original draft, Writing–review and editing. GR: Conceptualization, Data curation, Investigation, Resources, Software, Visualization, Writing–original draft, Writing–review and editing. AS: Conceptualization, Data curation, Investigation, Resources, Software, Visualization, Writing–original draft, Writing–review and editing. LL: Conceptualization, Data curation, Investigation, Resources, Software, Validation, Visualization, Writing–original draft, Writing–review and editing. AÇ: Investigation, Writing–original draft. TE: Data curation, Investigation, Writing–original draft. TL: Conceptualization, Data curation, Investigation, Resources, Software, Validation, Visualization, Writing–original draft, Writing–review and editing.
Funding
The author(s) declare that no financial support was received for the research, authorship, and/or publication of this article.
Acknowledgments
TE and AÇ are 100/2000 Ph.D. Scholars of the Council of Higher Education (CoHE) in the Bioengineering and Sciences program in Turkiye.
Conflict of interest
The authors declare that the research was conducted in the absence of any commercial or financial relationships that could be construed as a potential conflict of interest.
The author(s) declared that they were an editorial board member of Frontiers, at the time of submission. This had no impact on the peer review process and the final decision.
Publisher’s note
All claims expressed in this article are solely those of the authors and do not necessarily represent those of their affiliated organizations, or those of the publisher, the editors and the reviewers. Any product that may be evaluated in this article, or claim that may be made by its manufacturer, is not guaranteed or endorsed by the publisher.
References
Abohamzeh, E., Salehi, F., Sheikholeslami, M., Abbassi, R., and Khan, F. (2021). Review of hydrogen safety during storage, transmission, and applications processes. J. Loss Prev. Process Ind. 72, 104569.
Ali, M., Batool, S., Khalid, N., Ali, S., Raza, M. A., Li, X., et al. (2023). Recent trends in hydrogen-associated treatments for maintaining the postharvest quality of fresh and fresh-cut fruits and vegetables: a review. Food control. 156 (31), 110114. doi:10.1016/j.foodcont.2023.110114
Alwazeer, D. (2003). “Intérêt de la modification du Eh par des gaz pour la maîtrise des micro-organismes d’altération du jus d’orange et ses implications sur la stabilité de la couleur et de la vitamine C: développement d’un appareil adapté à l’étude des micro-organismes,”. Ph.D. Thesis (Dijon, France: University of Bourgogne).
Alwazeer, D. (2018). Reducing atmosphere drying as a new technique for the preservation of the color of dried foods. J. Inst. Sci. Technol. 8, 125–131. doi:10.21597/jist.418232
Alwazeer, D. (2019). Reducing atmosphere packaging technique for extending the shelf-life of food products. Iğdır Üniversitesi Fen Bilim. Enstitüsü Derg. 9, 2117–2123. doi:10.21597/jist.539744
Alwazeer, D. (2023). Hydrogen-rich solvent method in phytochemical extraction: potential mechanisms and perspectives. Phytochem. Anal. 35, 203–219. doi:10.1002/pca.3304
Alwazeer, D. (2024a). “Consumption of hydrogen-treated foods provides nutritional and health benefits,” in Molecular hydrogen in health and disease. Editors J. Slezak, and B. Kura (Cham: Springer Nature Switzerland), 319–337. doi:10.1007/978-3-031-47375-3_19
Alwazeer, D. (2024b). “Use of hydrogen extraction in the food industry,” in Reference module in chemistry, molecular sciences and chemical engineering. Editor J. Reedijk (Elsevier). doi:10.1016/b978-0-443-15978-7.00010-2
Alwazeer, D., Bulut, M., and Çelebi, Y. (2022a). Hydrogen-rich water can restrict the formation of biogenic amines in red beet pickles. Fermentation 8, 741. doi:10.3390/fermentation8120741
Alwazeer, D., Bulut, M., Ceylan, M. M., Çelebi, Y., Kavrut, E., Çetintaş, Y., et al. (2024). Hydrogen incorporation into butter improves its microbial and chemical stability, biogenic amine safety, quality attributes, and shelf-life. LWT 206, 116550. doi:10.1016/j.lwt.2024.116550
Alwazeer, D., Ceylan, M. M., Bulut, M., and Koyuncu, M. (2022b). Evaluation of the impact of hydrogen-rich water on the deaccumulation of heavy metals in butter. J. Food Saf. 42. doi:10.1111/jfs.13005
Alwazeer, D., and Çiğdem, A. (2022). Use of the molecular hydrogen in agriculture field. Turkish J. Agric. - Food Sci. Technol. 10, 14–20. doi:10.24925/turjaf.v10i1.14-20.4609
Alwazeer, D., Delbeau, C., Divies, C., and Cachon, R. (2003). Use of redox potential modification by gas improves microbial quality, color retention, and ascorbic acid stability of pasteurized orange juice. Int. J. Food Microbiol. 89, 21–29. doi:10.1016/S0168-1605(03)00125-9
Alwazeer, D., and Elnasanelkasim, M. A. (2023). Hydrogen-rich water as a green solvent for the extraction of phytochemicals from agri-food wastes. Sustain Chem. Pharm. 33, 101035. doi:10.1016/j.scp.2023.101035
Alwazeer, D., Elnasanelkasim, M. A., Çiçek, S., Engin, T., Çiğdem, A., and Karaoğul, E. (2023a). Comparative study of phytochemical extraction using hydrogen-rich water and supercritical fluid extraction methods. Process Biochem. 128, 218–226. doi:10.1016/j.procbio.2023.01.022
Alwazeer, D., Elnasanelkasim, M. A., Çiğdem, A., Engin, T., and LeBaron, T. W. (2023c). Incorporation of molecular hydrogen into solvents increases the extraction efficiency of phenolics, flavonoids, anthocyanins, and antioxidants: the case of lemon peels. Front. Sustain Food Syst. 7, 1223027. doi:10.3389/fsufs.2023.1223027
Alwazeer, D., Elnasanelkasim, M. A., Çiğdem, A., Engin, T., Kanmaz, H., Hayaloglu, A. A., et al. (2023b). Hydrogen incorporation into solvents can improve the extraction of phenolics, flavonoids, anthocyanins, and antioxidants: a case-study using red beetroot. Ind. Crops Prod. 202, 117005. doi:10.1016/j.indcrop.2023.117005
Alwazeer, D., Elnasanelkasim, M. A., Engin, T., and Çiğdem, A. (2023d). Use of hydrogen-rich water as a green solvent for the extraction of phytochemicals: case of olive leaves. J. Appl. Res. Med. Aromat. Plants 35, 100472. doi:10.1016/j.jarmap.2023.100472
Alwazeer, D., Liu, F. F.-C., Wu, X. Y., and LeBaron, W. T. (2021). Combating oxidative stress and inflammation in COVID-19 by molecular hydrogen therapy: mechanisms and perspectives. Oxid. Med. Cell Longev. 2021, 5513868. doi:10.1155/2021/5513868
Alwazeer, D., and Örs, B. (2019). Reducing atmosphere drying as a novel drying technique for preserving the sensorial and nutritional notes of foods. J. Food Sci. Technol. 56, 3790–3800. doi:10.1007/s13197-019-03850-2
Alwazeer, D., Örs, B., and Tan, K. (2020). Reducing atmosphere packaging as a novel alternative technique for extending shelf life of fresh cheese. J. Food Sci. Technol. 57, 3013–3023. doi:10.1007/s13197-020-04334-4
Alwazeer, D., and Özkan, N. (2022). Incorporation of hydrogen into the packaging atmosphere protects the nutritional, textural and sensorial freshness notes of strawberries and extends shelf life. J. Food Sci. Technol. 59, 3951–3964. doi:10.1007/s13197-022-05427-y
Baweja, P., Kumar, S., and Kumar, G. (2020). “Fertilizers and pesticides: their impact on soil health and environment,” in Soil health. Soil biology. Editors B. Giri, and A. Varma (Cham: Springer), 265–285. doi:10.1007/978-3-030-44364-1_15
Bhatnagar, P., Gururani, P., Bisht, B., Kumar, V., Kumar, N., Joshi, R., et al. (2022). Impact of irradiation on physico-chemical and nutritional properties of fruits and vegetables: a mini review. Heliyon 8, e10918. doi:10.1016/j.heliyon.2022.e10918
Bjurstedt, H., and Severin, G. (1962). The prevention of decompression sickness and nitrogen narcosis by the use of hydrogen as a substitute for nitrogen, the Arne Zetterstrôm method for deep-sea diving. Deep Sea Diving Sch. Stud. Med. Off. Study Mater. 1961, 249.
Bulut, M., Çelebi Sezer, Y., Ceylan, M. M., Alwazeer, D., and Koyuncu, M. (2022). Hydrogen-rich water can reduce the formation of biogenic amines in butter. Food Chem. 384, 132613. doi:10.1016/j.foodchem.2022.132613
Bulut, M., Okutan, G., Alwazeer, D., and Boran, G. (2023). Hydrogen inclusion in modified atmosphere extends the shelf life of chilled rainbow trout fillets. Turk J. Fish. Aquat. Sci. 23. doi:10.4194/trjfas22515
Cai, C., Zhao, Z., Zhang, Y., Li, M., Li, L., Cheng, P., et al. (2022). Molecular hydrogen improves rice storage quality via alleviating lipid deterioration and maintaining nutritional values. Plants 11, 2588. doi:10.3390/plants11192588
Capelle, N. (2001). Impact de modifications du potentiel d’oxydoréduction par des gaz sur les flux métaboliques et la physiologie de Saccharomyces cerevisiae. Dijon. Bourgogne.
Çelebi, Y., Kavrut, E., Bulut, M., Çetintaş, Y., Tekin, A., Hayaloğlu, A. A., et al. (2024). Incorporation of hydrogen-producing magnesium into minced beef meat protects the quality attributes and safety of the product during cold storage. Food Chem. 448, 139185. doi:10.1016/j.foodchem.2024.139185
Ceylan, M. M., Bulut, M., Alwazeer, D., and Koyuncu, M. (2022). Evaluation of the impact of hydrogen-rich water on the quality attribute notes of Butter. J. Dairy Res. 89, 431–439. doi:10.1017/S0022029922000681
Ceylan, M. M., Silgan, M., Elnasanelkasim, M. A., and Alwazeer, D. (2023). Impact of washing crude olive pomace oil with hydrogen-rich water and incorporating hydrogen into extraction solvents on quality attributes and phytochemical content of oil. J. Food Meas. Charact. 17, 2029–2040. doi:10.1007/s11694-022-01801-8
Chen, H., Zhang, J., Hao, H., Feng, Z., Chen, M., Wang, H., et al. (2017). Hydrogen-rich water increases postharvest quality by enhancing antioxidant capacity in Hypsizygus marmoreus. Amb. Express 7, 221. doi:10.1186/s13568-017-0496-9
Cheng, P., Wang, J., Zhao, Z., Kong, L., Lou, W., Zhang, T., et al. (2021). Molecular hydrogen increases quantitative and qualitative traits of rice grain in field trials. Plants 10, 2331. doi:10.3390/plants10112331
Cheng, P., Wang, Y., Cai, C., Li, L., Zeng, Y., Cheng, X., et al. (2023). Molecular hydrogen positively regulates nitrate uptake and seed size by targeting nitrate reductase. Plant Physiol., kiad474. doi:10.1093/plphys/kiad474
Coenen, J. W. E. (1976). Hydrogenation of edible oils. J. Am. Oil Chem. Soc. 53, 382–389. doi:10.1007/bf02605727
Cui, W., Fang, P., Zhu, K., Mao, Y., Gao, C., Xie, Y., et al. (2014). Hydrogen-rich water confers plant tolerance to mercury toxicity in alfalfa seedlings. Ecotoxicol. Environ. Saf. 105, 103–111. doi:10.1016/j.ecoenv.2014.04.009
Cui, W., Gao, C., Fang, P., Lin, G., and Shen, W. (2013). Alleviation of cadmium toxicity in Medicago sativa by hydrogen-rich water. J. Hazard Mater 260, 715–724. doi:10.1016/j.jhazmat.2013.06.032
Cui, W., Yao, P., Pan, J., Dai, C., Cao, H., Chen, Z., et al. (2020). Transcriptome analysis reveals insight into molecular hydrogen-induced cadmium tolerance in alfalfa: the prominent role of sulfur and (homo) glutathione metabolism. BMC Plant Biol. 20, 58–19. doi:10.1186/s12870-020-2272-2
Dong, B., Zhu, D., Qiuping, Y., Da, F., Chen, Y., and Ding, X. (2023a). Hydrogen-rich water treatment improves fresh-cut Gastrodia elata quality by regulating reactive oxygen species metabolism and energy metabolism during low temperature storage. Front. Sustain Food Syst. 7, 1274701. doi:10.3389/fsufs.2023.1274701
Dong, B., Zhu, D., Yao, Q., Tang, H., and Ding, X. (2022). Hydrogen-rich water treatment maintains the quality of Rosa sterilis fruit by regulating antioxidant capacity and energy metabolism. LWT 161, 113361. doi:10.1016/j.lwt.2022.113361
Dong, W., Cao, S., Zhou, Q., Jin, S., Zhou, C., Liu, Q., et al. (2023b). Hydrogen-rich water treatment increased several phytohormones and prolonged the shelf life in postharvest okras. Front. Plant Sci. 14, 1108515. doi:10.3389/fpls.2023.1108515
Dong, W., Shi, L., Li, S., Xu, F., Yang, Z., and Cao, S. (2023c). Hydrogen-rich water delays fruit softening and prolongs shelf life of postharvest okras. Food Chem. 399, 133997. doi:10.1016/j.foodchem.2022.133997
Ebel, B., Martin, F., Le, L. D. T., Gervais, P., and Cachon, R. (2011). Use of gases to improve survival of Bifidobacterium bifidum by modifying redox potential in fermented milk. J. Dairy Sci. 94, 2185–2191. doi:10.3168/jds.2010-3850
European Commission (2011). Commission regulation (EU) No 1129/2011. Official Journal of the European Union.
Fan, X., Zhang, X., Zhao, G., Zhang, X., Dong, L., and Chen, Y. (2022). Aerobic hydrogen-oxidizing bacteria in soil: from cells to ecosystems. Rev. Environ. Sci. Biotechnol. 21, 877–904. doi:10.1007/s11157-022-09633-0
Feng, J., Chen, L., and Zuo, J. (2019). Protein S-nitrosylation in plants: current progresses and challenges. J. Integr. Plant Biol. 61, 1206–1223. doi:10.1111/jipb.12780
Finke, C. E., Leandri, H. F., Karumb, E. T., Zheng, D., Hoffmann, M. R., and Fromer, N. A. (2021). Economically advantageous pathways for reducing greenhouse gas emissions from industrial hydrogen under common, current economic conditions. Energy Environ. Sci. 14, 1517–1529. doi:10.1039/d0ee03768k
Foegeding, P. M., and Busta, F. F. (1983). Effect of carbon dioxide, nitrogen and hydrogen gases on germination of Clostridium botulinum spores. J. Food Prot. 46, 987–989. doi:10.4315/0362-028X-46.11.987
Fu, X., Ma, L., Gui, R., Li, Y., Yang, X., Zhang, J., et al. (2020). Hydrogen rich water (HRW) induces plant growth and physiological attributes in fragrant rice varieties under salt stress. Research Square.
George, S. M., Richardson, L. C., Pol, I. E., and Peck, M. W. (1998). Effect of oxygen concentration and redox potential on recovery of sublethally heat-damaged cells of Escherichia coli O157:H7, Salmonella enteritidis and Listeria monocytogenes. J. Appl. Microbiol. 84, 903–909. doi:10.1046/j.1365-2672.1998.00424.x
Giroux, H. J., Acteau, G., Sabik, H., and Britten, M. (2008). Influence of dissolved gases and heat treatments on the oxidative degradation of polyunsaturated fatty acids enriched dairy beverage. J. Agric. Food Chem. 56, 5710–5716. doi:10.1021/jf800516x
Greening, C., Islam, Z. F., and Bay, S. K. (2022). Hydrogen is a major lifeline for aerobic bacteria. Trends Microbiol. 30, 330–337. doi:10.1016/j.tim.2021.08.004
Guan, Q., Ding, X. W., Jiang, R., Ouyang, P. L., Gui, J., Feng, L., et al. (2019). Effects of hydrogen-rich water on the nutrient composition and antioxidative characteristics of sprouted black barley. Food Chem. 299, 125095. doi:10.1016/j.foodchem.2019.125095
Hancock, J. T. (2023). Are protein cavities and pockets commonly used by redox active signalling molecules? Plants 12, 2594. doi:10.3390/plants12142594
Hancock, J. T., and Hancock, T. H. (2018). Hydrogen gas, ROS metabolism and cell signaling: are hydrogen spin states important? React. Oxyg. Species 6. doi:10.20455/ros.2018.869
Hancock, J. T., and LeBaron, T. W. (2023). The early history of hydrogen and other gases in respiration and biological systems: revisiting beddoes, cavallo, and davy. Oxygen 3, 102–119. doi:10.3390/oxygen3010008
Hancock, J. T., LeBaron, T. W., and Russell, G. (2021). Molecular hydrogen: redox reactions and possible biological interactions. React. Oxyg. Species (Apex) 11. doi:10.20455/ros.2021.m.803
Hancock, J. T., Russell, G., Craig, T. J., May, J., Morse, H. R., and Stamler, J. S. (2022). Understanding hydrogen: lessons to Be learned from physical interactions between the inert gases and the globin superfamily. Oxygen 2, 578–590. doi:10.3390/oxygen2040038
Hore, P. J., and Mouritsen, H. (2016). The radical-pair mechanism of magnetoreception. Annu. Rev. Biophys. 45, 299–344. doi:10.1146/annurev-biophys-032116-094545
Hu, H., Li, P., Wang, Y., and Gu, R. (2014). Hydrogen-rich water delays postharvest ripening and senescence of kiwifruit. Food Chem. 156, 100–109. doi:10.1016/j.foodchem.2014.01.067
Hu, H., Zhao, S., Li, P., and Shen, W. (2018). Hydrogen gas prolongs the shelf life of kiwifruit by decreasing ethylene biosynthesis. Postharvest Biol. Technol. 135, 123–130. doi:10.1016/j.postharvbio.2017.09.008
Huang, P., Li, C., Liu, H., Zhao, Z., and Liao, W. (2021). Hydrogen gas improves seed germination in cucumber by regulating sugar and starch metabolisms. Horticulturae 7, 456. doi:10.3390/horticulturae7110456
Islam, M. A., Shorna, M. N. A., Islam, S., Biswas, S., Biswas, J., Islam, S., et al. (2023a). Hydrogen-rich water: a key player in boosting wheat (Triticum aestivum L.) seedling growth and drought resilience. Sci. Rep. 13, 22521. doi:10.1038/s41598-023-49973-7
Islam, Z. F., Greening, C., and Hu, H. W. (2023b). Microbial hydrogen cycling in agricultural systems – plant beneficial or detrimental? Microb. Biotechnol. 16, 1623–1628. doi:10.1111/1751-7915.14300
Jiang, K., Kuang, Y., Feng, L., Liu, Y., Wang, S., Du, H., et al. (2021). Molecular hydrogen maintains the storage quality of Chinese chive through improving antioxidant capacity. Plants 10, 1095. doi:10.3390/plants10061095
Jiang, Y., Ye, Q., Ma, L., Yang, X., Zhang, J., and Mo, Z. (2023). Regulation of growth and physiological properties of fragrant rice seedlings by hydrogen-rich water (HRW) under nitrogen-deficient conditions. J. Plant Growth Regul. 42, 2221–2231. doi:10.1007/s00344-022-10696-0
Jin, Q., Cui, W., Dai, C., Zhu, K., Zhang, J., Wang, R., et al. (2016). Involvement of hydrogen peroxide and heme oxygenase-1 in hydrogen gas-induced osmotic stress tolerance in alfalfa. Plant Growth Regul. 80, 215–223. doi:10.1007/s10725-016-0159-x
Jin, Z., Zhao, P., Gong, W., Ding, W., and He, Q. (2023). Fe-porphyrin: a redox-related biosensor of hydrogen molecule. Nano Res. 16, 2020–2025. doi:10.1007/s12274-022-4860-y
Kihm, D. J., and Johnson, E. A. (1990). Hydrogen gas accelerates thermal inactivation of Clostridium botulinum 113 B spores. Appl. Microbiol. Biotechnol. 33, 705–708. doi:10.1007/bf00604942
Kim, S.-A., Jong, Y.-C., Kang, M.-S., and Yu, C.-J. (2022). Antioxidation activity of molecular hydrogen via protoheme catalysis in vivo: an insight from ab initio calculations. J. Mol. Model 28, 287. doi:10.1007/s00894-022-05264-y
Köktürk, M., Atalar, M. N., Odunkıran, A., Bulut, M., and Alwazeer, D. (2022a). Evaluation of the hydrogen-rich water alleviation potential on mercury toxicity in earthworms using ATR-FTIR and LC–ESI–MS/MS spectroscopy. Environ. Sci. Pollut. Res. 29, 19642–19656. doi:10.1007/s11356-021-17230-x
Köktürk, M., Yıldırım, S., Eser, G., Bulut, M., and Alwazeer, D. (2022b). Hydrogen-rich water alleviates the nickel-induced toxic responses (inflammatory responses, oxidative stress, DNA damage) and ameliorates cocoon production in earthworm. Biol. Trace Elem. Res. 200, 3442–3452. doi:10.1007/s12011-021-02908-7
LeBaron, T., Sharpe, R., Pyatakovich, F. A., and Artamonov, M. (2024). “Hydrogen: from stars to fuel to medicine,” in Molecular hydrogen in health and disease. Editors J. Slezak, and B. Kura (Berlin: Springe: Springer Nature Switzerland).
LeBaron, T. W., Kharman, J., and McCullough, M. (2021). Effects of an H2-infused, nitric oxide-producing functional beverage on exercise and cognitive performance. J. Sci. Med. 3, 1–15. doi:10.37714/josam.v3i2.79
LeBaron, T. W., Ohno, K., and Hancock, J. T. (2023). The on/off history of hydrogen in medicine: will the interest persist this time around? Oxygen 3, 143–162. doi:10.3390/oxygen3010011
Li, F., Hu, Y., Shan, Y., Liu, J., Ding, X., Duan, X., et al. (2022a). Hydrogen-rich water maintains the color quality of fresh-cut Chinese water chestnut. Postharvest Biol. Technol. 183, 111743. doi:10.1016/j.postharvbio.2021.111743
Li, L., Lou, W., Kong, L., and Shen, W. (2021a). Hydrogen commonly applicable from medicine to agriculture: from molecular mechanisms to the field. Curr. Pharm. Des. 27, 747–759. doi:10.2174/1381612826666201207220051
Li, L., Wang, J., Jiang, K., Kuang, Y., Zeng, Y., Cheng, X., et al. (2022b). Preharvest application of hydrogen nanobubble water enhances strawberry flavor and consumer preferences. Food Chem. 377, 131953. doi:10.1016/j.foodchem.2021.131953
Li, L., Zeng, Y., Cheng, X., and Shen, W. (2021b). The applications of molecular hydrogen in horticulture. Horticulturae 7, 513. doi:10.3390/horticulturae7110513
Li, M., Zhu, G., Liu, Z., Li, L., Wang, S., Liu, Y., et al. (2024). Hydrogen fertilization with hydrogen nanobubble water improves yield and quality of cherry tomatoes compared to the conventional fertilizers. Plants 13, 443. doi:10.3390/plants13030443
Liu, F., Jiang, W., Han, W., Li, J., and Liu, Y. (2017). Effects of hydrogen-rich water on fitness parameters of rice plants. Agron. J. 109, 2033–2039. doi:10.2134/agronj2017.02.0109
Liu, H., Wang, W., Cao, G., and Tang, M. (2010). Effect of hydrogen on microbial population and enzyme activity in Robinia pseudoacacia rhizosphere soil. Chin. J. Appl. Environ. Biol. doi:10.3724/SP.J.l145.2010.00515
Liu, Z., Chen, G., Yang, E., Li, L., Zeng, Y., Cheng, X., et al. (2024). Hydrogen-based irrigation increases yield and improves quality of Chinese cabbage by enhancing nutrient composition and antioxidant capabilities. Hortic. Environ. Biotechnol. 65, 593–605. doi:10.1007/s13580-023-00591-2
Lu, H., Wu, B., Wang, Y., Liu, N., Meng, F., Hu, Z., et al. (2017). Effects of hydrogen-rich water treatment on defense responses of postharvest tomato fruit to Botrytis cinerea. J. Henan Agric. Sci. 46, 64–68.
Ma, L., Kong, L., Gui, R., Yang, X., Zhang, J., Gong, Q., et al. (2021). Application of hydrogen-rich water modulates physio-biochemical functions and early growth of fragrant rice under Cd and Pb stress. Environ. Sci. Pollut. Res. 28, 58558–58569. doi:10.1007/s11356-021-14747-z
Maimaiti, J., Zhang, Y., Yang, J., Cen, Y., Layzell, D. B., Peoples, M., et al. (2007). Isolation and characterization of hydrogen-oxidizing bacteria induced following exposure of soil to hydrogen gas and their impact on plant growth. Environ. Microbiol. 9, 435–444. doi:10.1111/j.1462-2920.2006.01155.x
Martin, F., Cachon, R., Pernin, K., Coninck, J. D., Gervais, P., Guichard, E., et al. (2011). Effect of oxidoreduction potential on aroma biosynthesis by lactic acid bacteria in nonfat yogurt. J. Dairy Sci. 94, 614–622. doi:10.3168/jds.2010-3372
Martin, F., Cayot, N., Marin, A., Journaux, L., Cayot, P., Gervais, P., et al. (2009). Effect of oxidoreduction potential and of gas bubbling on rheological properties and microstructure of acid skim milk gels acidified with glucono-δ-lactone. J. Dairy Sci. 92, 5898–5906. doi:10.3168/jds.2009-2491
Martin, F., Cayot, N., Vergoignan, C., Journaux, L., Gervais, P., and Cachon, R. (2010). Impact of oxidoreduction potential and of gas bubbling on rheological properties of non-fat yoghurt. Food Res. Int. 43, 218–223. doi:10.1016/j.foodres.2009.09.032
Martin, W., and Müller, M. (1998). The hydrogen hypothesis for the first eukaryote. Nature 392, 37–41. doi:10.1038/32096
Mikami, T., Tano, K., Lee, H., Lee, H., Park, J., Ohta, F., et al. (2019). Drinking hydrogen water enhances endurance and relieves psychometric fatigue: a randomized, double-blind, placebo-controlled study. Can. J. Physiol. Pharmacol. 97, 857–862. doi:10.1139/CJPP-2019-0059
Nasri, E., Khademi, O., Saba, M. K., and Ebrahimi, R. (2023). Extension of button mushroom storability by ultrasound treatment in combination with calcium lactate. J. Food Meas. Charact. 17, 54–62. doi:10.1007/s11694-022-01560-6
Nguyen, T. K., and Lim, J. H. (2022). Is it a challenge to use molecular hydrogen for extending flower vase life? Plants 11, 1277. doi:10.3390/plants11101277
Ohsawa, I., Ishikawa, M., Takahashi, K., Watanabe, M., Nishimaki, K., Yamagata, K., et al. (2007). Hydrogen acts as a therapeutic antioxidant by selectively reducing cytotoxic oxygen radicals. Nat. Med. 13, 688–694. doi:10.1038/nm1577
Ohta, S. (2023). Molecular hydrogen may activate the transcription factor Nrf2 to alleviate oxidative stress through the hydrogen-targeted porphyrin. Aging Pathobiol. Ther. 5, 25–32. doi:10.31491/apt.2023.03.104
Olsen, M. J. (2008). Redox potential trends of cucumber fermentation as influenced by microbial growth and gas purging. Raleigh, North Carolina: North Carolina State University.
O’Neill, K. T., Al Ghafri, S., da Silva Falcão, B., Tang, L., Kozielski, K., and Johns, M. L. (2023). Hydrogen ortho-para conversion: process sensitivities and optimisation. Chem. Eng. Processing-Process Intensif. 184, 109272. doi:10.1016/j.cep.2023.109272
Ouvry, A. (2001). Effets du potentiel d’oxydoréduction sur le comportement de deux microorganismes de contamination des produits alimentaires assaisonnés: Escherichia coli et Lactobacillus plantarum. Dijon. Bourgogne.
Ouvry, A., Wache, Y., Tourdot-Marechal, R., Divies, C., and Cachon, R. (2002). Effects of oxidoreduction potential combined with acetic acid, NaCl and temperature on the growth, acidification, and membrane properties of Lactobacillus plantarum. FEMS Microbiol. Lett. 214, 257–261. doi:10.1111/j.1574-6968.2002.tb11356.x
Pasquali, F., Stratakos, A. C., Koidis, A., Berardinelli, A., Cevoli, C., Ragni, L., et al. (2016). Atmospheric cold plasma process for vegetable leaf decontamination: a feasibility study on radicchio (red chicory, Cichorium intybus L.). Food control. 60, 552–559. doi:10.1016/j.foodcont.2015.08.043
Peck, H. D. (1959). The ATP-dependent reduction of sulfate with hydrogen in extracts of Desulfovibrio desulfuricans. Proc. Natl. Acad. Sci. 45, 701–708. doi:10.1073/pnas.45.5.701
Pham, T. H., Mauvais, G., Vergoignan, C., De Coninck, J., Dumont, F., Lherminier, J., et al. (2008). Gaseous environments modify physiology in the brewing yeast Saccharomyces cerevisiae during batch alcoholic fermentation. J. Appl. Microbiol. 105, 858–874. doi:10.1111/j.1365-2672.2008.03821.x
Pivetta, D., Dall’Armi, C., and Taccani, R. (2022). Multi-objective optimization of a hydrogen hub for the decarbonization of a port industrial area. J. Mar. Sci. Eng. 10, 231. doi:10.3390/jmse10020231
Ramsay, J., and Kattnig, D. R. (2022). Radical triads, not pairs, may explain effects of hypomagnetic fields on neurogenesis. PLoS Comput. Biol. 18, e1010519. doi:10.1371/journal.pcbi.1010519
Richards, S. L., Wilkins, K. A., Swarbreck, S. M., Anderson, A. A., Habib, N., Smith, A. G., et al. (2015). The hydroxyl radical in plants: from seed to seed. J. Exp. Bot. 66, 37–46. doi:10.1093/jxb/eru398
Russell, G., May, J., and Hancock, J. T. (2024). An interplay of gases: oxygen and hydrogen in biological systems. Oxygen 4, 37–52. doi:10.3390/oxygen4010003
Russell, G., Nenov, A., Kisher, H., and Hancock, J. T. (2021). Molecular hydrogen as medicine: an assessment of administration methods. Hydrogen (Switzerland) 2, 444–460. doi:10.3390/hydrogen2040025
Ryu, J., Kim, M. J., and Lee, J. H. (2019). Extraction of green tea phenolics using water bubbled with gases. J. Food Sci. 84, 1308–1314. doi:10.1111/1750-3841.14606
Sezer, Y. Ç., Bulut, M., Boran, G., and Alwazeer, D. (2022). The effects of hydrogen incorporation in modified atmosphere packaging on the formation of biogenic amines in cold stored rainbow trout and horse mackerel. J. Food Compos. Analysis 112, 104688. doi:10.1016/j.jfca.2022.104688
Shao, Y., Lin, F., Wang, Y., Cheng, P., Lou, W., Wang, Z., et al. (2023). Molecular hydrogen confers resistance to rice stripe virus. Microbiol. Spectr. 11, e0441722. doi:10.1128/spectrum.04417-22
Shen, N.-Y., Bi, J.-B., Zhang, J.-Y., Zhang, S.-M., Gu, J.-X., Qu, K., et al. (2017). Hydrogen-rich water protects against inflammatory bowel disease in mice by inhibiting endoplasmic reticulum stress and promoting heme oxygenase-1 expression. World J. Gastroenterol. 23, 1375–1386. doi:10.3748/wjg.v23.i8.1375
Shin, D., Cho, E. S. R., Bang, H.-T., and Shim, K. S. (2016). Effects of oxygenated or hydrogenated water on growth performance, blood parameters, and antioxidant enzyme activity of broiler chickens. Poult. Sci. 95, 2679–2684. doi:10.3382/ps/pew237
Singh, A., Vaidya, G., Jagota, V., Darko, D. A., Agarwal, R. K., Debnath, S., et al. (2022). Recent advancement in postharvest loss mitigation and quality management of fruits and vegetables using machine learning frameworks. J. Food Qual. 2022, 1–9. doi:10.1155/2022/6447282
Singh, R. B., Sumbalova, Z., Fatima, G., Mojto, V., Fedacko, J., Tarnava, A., et al. (2024). Effects of molecular hydrogen in the pathophysiology and management of cardiovascular and metabolic diseases. Rev. Cardiovasc Med. 25, 33. doi:10.31083/j.rcm2501033
Singla, M. K., Nijhawan, P., and Oberoi, A. S. (2021). Hydrogen fuel and fuel cell technology for cleaner future: a review. Environ. Sci. Pollut. Res. 28, 15607–15626. doi:10.1007/s11356-020-12231-8
Stein, S., Selesi, D., Schilling, R., Pattis, I., Schmid, M., and Hartmann, A. (2005). Microbial activity and bacterial composition of H2-treated soils with net CO2 fixation. Soil Biol. Biochem. 37, 1938–1945. doi:10.1016/j.soilbio.2005.02.035
Stucki, D., and Stahl, W. (2020). Carbon monoxide–beyond toxicity? Toxicol. Lett. 333, 251–260. doi:10.1016/j.toxlet.2020.08.010
Su, J., Nie, Y., Zhao, G., Cheng, D., Wang, R., Chen, J., et al. (2019). Endogenous hydrogen gas delays petal senescence and extends the vase life of lisianthus cut flowers. Postharvest Biol. Technol. 147, 148–155. doi:10.1016/j.postharvbio.2018.09.018
Su, J., Yang, X., Shao, Y., Chen, Z., and Shen, W. (2021). Molecular hydrogen–induced salinity tolerance requires melatonin signalling in Arabidopsis thaliana. Plant Cell Environ. 44, 476–490. doi:10.1111/pce.13926
Su, N., Wu, Q., Liu, Y., Cai, J., Shen, W., Xia, K., et al. (2014). Hydrogen-rich water reestablishes ROS homeostasis but exerts differential effects on anthocyanin synthesis in two varieties of radish sprouts under UV-A irradiation. J. Agric. Food Chem. 62, 6454–6462. doi:10.1021/jf5019593
Tarzad, U. (2020). Supreme theory of everything: whole universe in a simple formula. Lond. J. Res. Sci. Nat. Formal.
Turan, H. T., Boittier, E., and Meuwly, M. (2023). Interaction at a distance: xenon migration in mb. J. Chem. Phys. 158, 125103. doi:10.1063/5.0124502
Turell, L., Zeida, A., and Trujillo, M. (2020). Mechanisms and consequences of protein cysteine oxidation: the role of the initial short-lived intermediates. Essays Biochem. 64, 55–66. doi:10.1042/EBC20190053
U.S. Food and Drug Administration (2023). Hydrogen gas. Available at: https://www.cfsanappsexternal.fda.gov/scripts/fdcc/?set=GRASNotices&id=520&sort=GRN_No&order=DESC&startrow=1&type=basic&search=hydrogen.
Wang, B., Bian, B., Wang, C., Li, C., Fang, H., Zhang, J., et al. (2019). Hydrogen gas promotes the adventitious rooting in cucumber under cadmium stress. PLoS One 14, e0212639. doi:10.1371/journal.pone.0212639
Wang, X. B., Schmidt, R., Yergeau, É., and Constant, P. (2020). Field H2 infusion alters bacterial and archaeal communities but not fungal communities nor nitrogen cycle gene abundance. Soil Biol. Biochem. 151, 108018. doi:10.1016/j.soilbio.2020.108018
Wang, Y., Lv, P., Kong, L., Shen, W., and He, Q. (2021). Nanomaterial-mediated sustainable hydrogen supply induces lateral root formation via nitrate reductase-dependent nitric oxide. Chem. Eng. J. 405, 126905. doi:10.1016/j.cej.2020.126905
Wang, Y., Zhang, T., Wang, J., Xu, S., and Shen, W. (2022). Regulation of chlorothalonil degradation by molecular hydrogen. J. Hazard Mater 424, 127291. doi:10.1016/j.jhazmat.2021.127291
Wei, X., Chen, J., Chen, H., Wu, X., Tian, J., Su, N., et al. (2021). Hydrogen-rich water ameliorates the toxicity induced by Ca(NO3)2 excess through enhancing antioxidant capacities and re-establishing nitrate homeostasis in Brassica campestris spp. chinensis L. seedlings. Acta Physiol. Plant. 43, 50. doi:10.1007/s11738-021-03220-6
Wu, Q., Su, N., Cai, J., Shen, Z., and Cui, J. (2015). Hydrogen-rich water enhances cadmium tolerance in Chinese cabbage by reducing cadmium uptake and increasing antioxidant capacities. J. Plant Physiol. 175, 174–182. doi:10.1016/j.jplph.2014.09.017
Wu, Q., Su, N., Huang, X., Ling, X., Yu, M., Cui, J., et al. (2020a). Hydrogen-rich water promotes elongation of hypocotyls and roots in plants through mediating the level of endogenous gibberellin and auxin. Funct. Plant Biol. 47, 771–778. doi:10.1071/FP19107
Wu, Q., Su, N., Shabala, L., Huang, L., Yu, M., and Shabala, S. (2020b). Understanding the mechanistic basis of ameliorating effects of hydrogen rich water on salinity tolerance in barley. Environ. Exp. Bot. 177, 104136. doi:10.1016/j.envexpbot.2020.104136
Wu, X., Rensing, C., Han, D., Xiao, K.-Q., Dai, Y., Tang, Z., et al. (2022). Genome-Resolved metagenomics reveals distinct phosphorus acquisition strategies between soil microbiomes. mSystems 7, e0110721. doi:10.1128/msystems.01107-21
Wu, X., Su, N., Yue, X., Fang, B., Zou, J., Chen, Y., et al. (2021). IRT1 and ZIP2 were involved in exogenous hydrogen-rich water-reduced cadmium accumulation in Brassica chinensis and Arabidopsis thaliana. J. Hazard Mater 407, 124599. doi:10.1016/j.jhazmat.2020.124599
Xie, Y., Mao, Y., Lai, D., Zhang, W., and Shen, W. (2012). H2 enhances Arabidopsis salt tolerance by manipulating ZAT10/12-mediated antioxidant defence and controlling sodium exclusion. PLoS One 7, e49800. doi:10.1371/journal.pone.0049800
Xie, Y., Mao, Y., Zhang, W., Lai, D., Wang, Q., and Shen, W. (2014). Reactive oxygen species-dependent nitric oxide production contributes to hydrogen-promoted stomatal closure in Arabidopsis. Plant Physiol. 165, 759–773. doi:10.1104/pp.114.237925
Xie, Y., Zhang, W., Duan, X., Dai, C., Zhang, Y., Cui, W., et al. (2015). Hydrogen-rich water-alleviated ultraviolet-B-triggered oxidative damage is partially associated with the manipulation of the metabolism of (iso)flavonoids and antioxidant defence in Medicago sativa. Funct. Plant Biol. 42, 1141–1157. doi:10.1071/FP15204
Xu, S., Zhu, S., Jiang, Y., Wang, N., Wang, R., Shen, W., et al. (2013). Hydrogen-rich water alleviates salt stress in rice during seed germination. Plant Soil 370, 47–57. doi:10.1007/s11104-013-1614-3
Xu, Y., Teng, Y., Dong, X., Wang, X., Zhang, C., Ren, W., et al. (2021). Genome-resolved metagenomics reveals how soil bacterial communities respond to elevated H2 availability. Soil Biol. Biochem. 163, 108464. doi:10.1016/j.soilbio.2021.108464
Xu, Y., Teng, Y., Wang, X., Li, R., and Christie, P. (2020). Exploring bacterial community structure and function associated with polychlorinated biphenyl biodegradation in two hydrogen-amended soils. Sci. Total Environ. 745, 140839. doi:10.1016/j.scitotenv.2020.140839
Yao, Y., Zhao, Z., Ding, Z., Yao, K., Yang, Y., Hou, X., et al. (2024). Hydrogen-rich water irrigation promotes fruit ripening and nutritional composition in tomato. Postharvest Biol. Technol. 213, 112920. doi:10.1016/j.postharvbio.2024.112920
Ye, Z., Zhang, R., and Sun, X. (2013). Bustling argon: biological effect. Med. Gas. Res. 3, 22–24. doi:10.1186/2045-9912-3-22
Yu, Y., Zhang, H., Xing, H., Cui, N., Liu, X., Meng, X., et al. (2021). Regulation of growth and salt resistance in cucumber seedlings by hydrogen-rich water. J. Plant Growth Regul. 42, 134–153. doi:10.1007/s00344-021-10536-7
Yun, Z., Gao, H., Chen, X., Chen, Z., Zhang, Z., Li, T., et al. (2021). Effects of hydrogen water treatment on antioxidant system of litchi fruit during the pericarp browning. Food Chem. 336, 127618. doi:10.1016/j.foodchem.2020.127618
Yun, Z., Gao, H., Chen, X., Duan, X., and Jiang, Y. (2022). The role of hydrogen water in delaying ripening of banana fruit during postharvest storage. Food Chem. 373, 131590. doi:10.1016/j.foodchem.2021.131590
Yurt, B. (2023). Effect of hydrogen-enriched solvents on the extraction of phytochemicals in propolis. ACS Omega 8, 14264–14270. doi:10.1021/acsomega.3c01673
Zeng, J., and Yu, H. (2022). Integrated metabolomic and transcriptomic analyses to understand the effects of hydrogen water on the roots of Ficus hirta Vahl. Plants 11, 602. doi:10.3390/plants11050602
Zeng, J., Zhang, M., and Sun, X. (2013). Molecular hydrogen is involved in phytohormone signaling and stress responses in plants. PLoS One 8, e71038. doi:10.1371/journal.pone.0071038
Zhang, T., Wang, Y., Zhao, Z., Xu, S., and Shen, W. (2022). Degradation of carbendazim by molecular hydrogen on leaf models. Plants 11, 621. doi:10.3390/plants11050621
Zhang, Y., Cheng, P., Wang, Y., Li, Y., Su, J., Chen, Z., et al. (2020). Genetic elucidation of hydrogen signaling in plant osmotic tolerance and stomatal closure via hydrogen sulfide. Free Radic. Biol. Med. 161, 1–14. doi:10.1016/j.freeradbiomed.2020.09.021
Zhang, Y., Zhao, G., Cheng, P., Yan, X., Li, Y., Cheng, D., et al. (2019). Nitrite accumulation during storage of tomato fruit as prevented by hydrogen gas. Int. J. Food Prop. 22, 1425–1438. doi:10.1080/10942912.2019.1651737
Zhao, G., Cheng, P., Zhang, T., Abdalmegeed, D., Xu, S., and Shen, W. (2021a). Hydrogen-rich water prepared by ammonia borane can enhance rapeseed (Brassica napus L.) seedlings tolerance against salinity, drought or cadmium. Ecotoxicol. Environ. Saf. 224, 112640. doi:10.1016/j.ecoenv.2021.112640
Zhao, X., Meng, X., Li, W., Cheng, R., Wu, H., Liu, P., et al. (2021b). Effect of hydrogen - rich water and slightly acidic electrolyzed water treatments on storage and preservation of fresh - cut kiwifruit. J. Food Meas. Charact. 15, 5203–5210. doi:10.1007/s11694-021-01000-x
Zheng, X., Zheng, X., Mao, Y., Cai, J., Li, Y., Liu, W., et al. (2009). Hydrogen-rich saline protects against intestinal ischemia/reperfusion injury in rats. Free Radic. Res. 43, 478–484. doi:10.1080/10715760902870603
Zhu, Y., Liao, W., Wang, M., Niu, L., Xu, Q., and Jin, X. (2016). Nitric oxide is required for hydrogen gas-induced adventitious root formation in cucumber. J. Plant Physiol. 195, 50–58. doi:10.1016/j.jplph.2016.02.018
Zor, M., Bulut, M., Göksu Karagöz, S., Çetintaş, Y., and Alwazeer, D. (2023). Use of Hydrogen-Rich water in rice milk preparation improves the nutritional and sensory properties of product. Food Chem. 437, 137821. doi:10.1016/j.foodchem.2023.137821
Keywords: crops, foods, food security, green strategy, hydrogen-rich water, molecular hydrogen, sustainable technology
Citation: Alwazeer D, Hancock JT, Russell G, Stratakos AC, Li L, Çiğdem A, Engin T and LeBaron TW (2024) Molecular hydrogen: a sustainable strategy for agricultural and food production challenges. Front. Food. Sci. Technol. 4:1448148. doi: 10.3389/frfst.2024.1448148
Received: 12 June 2024; Accepted: 03 September 2024;
Published: 18 September 2024.
Edited by:
Hayriye Sebnem Harsa, Izmir Institute of Technology, TürkiyeReviewed by:
Oluwafemi James Caleb, Stellenbosch University, South AfricaMohini Prabha Singh, Punjab Agricultural University, India
Copyright © 2024 Alwazeer, Hancock, Russell, Stratakos, Li, Çiğdem, Engin and LeBaron. This is an open-access article distributed under the terms of the Creative Commons Attribution License (CC BY). The use, distribution or reproduction in other forums is permitted, provided the original author(s) and the copyright owner(s) are credited and that the original publication in this journal is cited, in accordance with accepted academic practice. No use, distribution or reproduction is permitted which does not comply with these terms.
*Correspondence: Duried Alwazeer, YWx3YXplZXJkQGdtYWlsLmNvbQ==
†ORCID: Duried Alwazeer, orcid.org/0000-0002-2291-1628; Tyler W. LeBaron, orcid.org/0000-0001-9164-6728