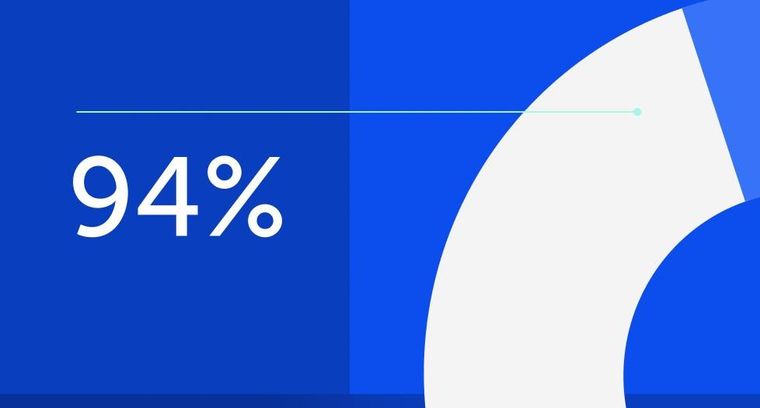
94% of researchers rate our articles as excellent or good
Learn more about the work of our research integrity team to safeguard the quality of each article we publish.
Find out more
ORIGINAL RESEARCH article
Front. Food. Sci. Technol., 13 March 2024
Sec. Food and Soft Materials
Volume 4 - 2024 | https://doi.org/10.3389/frfst.2024.1376044
Introduction: This study aimed to determine the optimal processing conditions to produce stable starch-based hydrogels by high-pressure processing (HPP) via response surface methodology.
Methods: The experiments were carried out with different starch suspensions, namely rice, corn, wheat, and tapioca starch, at a concentration in the range of 10%–40% w/w, processed at a pressure level of 600 MPa and holding times between 5 min and 15 min. Gel formation was assessed by determining the gelatinization extent and structuring level of the samples.
Results and discussion: The results demonstrated that starch/water ratio and holding time had a significant impact on gel formation in HPP treatments. Various degrees of gelatinization were observed in the treated samples due to the water absorption capacity of the starch and the molecular interactions between water and starch occurring during gelatinization. Moreover, a highly structured hydrogel formed at starch concentrations higher than 25% (w/w), whereas when starch concentration was less than 20% (w/w) lower-structured hydrogels formed, as confirmed by the values of the efficiency index measured. Completely gelatinized, highly structured, and stable HPP hydrogels were obtained from starch solutions treated at the optimized processing conditions.
Starch represents the main polysaccharide reserve material in photosynthetic plants and can be used as a cost-effective and adaptable material in polymer technology and other food and non-food applications (Halley et al., 2007; Laycock and Halley, 2014; Salimi et al., 2023). Starch can be discovered in various plants, but rice, wheat, corn, and tapioca starch are the most abundantly produced and traded worldwide. With wide applications in the food, textile, pharmaceutical, cosmetical, and, recently, polymer industries due to its abundance, cheapness, non-toxic properties, and biodegradability, starch plays a prominent role even in technological developments (Liu et al., 2022; Qamruzzaman et al., 2022). Moreover, the increasing attention to natural polymer-based products (Idrees et al., 2020; Samir et al., 2022; Pires et al., 2023) has increased the utilization of starch in various product formulations, including the production of starch-based hydrogels (Ismail et al., 2013; 2013; Edgar and Marks, 2020; Qamruzzaman et al., 2022). Hydrogels are an extensive group of polymeric materials, developed from three-dimensional crosslinked networks of hydrophilic or hydrophobic biopolymers and are widely known for their capacity to absorb and retain a significant amount of water (Singh et al., 2010; Biduski et al., 2018). Hydrogels from renewable sources such as starch, have contributed to the development of natural materials for their use in agriculture, biomedical, cosmeceutical, pharmaceutical, and food applications (Peppas et al., 2000; Hoffman, 2012; Caló and Khutoryanskiy, 2015; Parente et al., 2015; Mohammadinejad et al., 2019).
Starch-based hydrogels can be produced using physical or chemical cross-linking and graft polymerization treatments (Ismail et al., 2013; Xiao, 2013; Edgar and Marks, 2020; Cui et al., 2022). These conventional methods have faced several limitations, the most significant being long processing times and high energy consumption. In this context, novel technologies, including high-pressure processing (HPP), have been proposed to produce starch-based hydrogels. High-pressure processing (HPP) is a non-thermal emerging technology that exposes a product to high pressures for a controlled time and temperature (Jiang et al., 2015). HPP causes the disordering of biopolymers such as starch (Barba et al., 2015; Jiang et al., 2015; Yang et al., 2017), by modifying the non-covalent intermolecular interactions until a complete gelatinization. Pressure-induced starch gelatinization is a complex process that is influenced by various variables including starch source, starch/water ratio, pressure level, processing time, and temperature (Bauer and Knorr, 2005; Pei-Ling et al., 2010; Larrea-Wachtendorff et al., 2020). Several efforts have been undertaken over the past decades to understand the impact of the physicochemical properties of starches and HPP conditions on the gelatinization process (Katopo et al., 2002; Knorr et al., 2006; Błaszczak, 2007; Kawai et al., 2007; Vittadini et al., 2008; Yamamoto and Buckow, 2016; Leite et al., 2017; Larrea-Wachtendor et al., 2019; Pulgarín et al., 2023). Despite a surge in interest and study over the past few decades for these novel structures, to date, no research has been done to optimize starch-based HPP hydrogel formulation and processing conditions to produce stable and structured polymeric materials suitable for further exploitation. In this regard, this study aimed to determine the optimal processing conditions of starch-based HPP hydrogels, investigating the starch concentration and processing time as crucial parameters in pressure-induced starch gelatinization, using response surface methodology. Additionally, the characterization of starch-based HPP hydrogels produced under optimized processing conditions was carried out by determining the gelatinization efficiency index, swelling power, zeta potential, and rheological and textural properties of the hydrogel samples obtained.
Rice (S7260) (17.7% amylose content, 96.5% purity on dry weight basis), wheat (S5127) (26.96% amylose content, 99%purity on dry weight basis), and corn (S4126) (21.17% amylose content, 97% purity on dry weight basis) starch powders were purchased from Sigma Aldrich (Steinheim, Germany). Tapioca (20.2% amylose content, 92.2% purity on dry weight basis) starch powder was obtained from Rudolf Sizing Amidos do Brazil (Ibirarema, Sao Paulo, Brazil).
Starch-water suspensions at concentrations in the range of 10%–40% were prepared by suspending the starch powders in distilled water and dissolving them under gentle mixing immediately before HPP treatments to ensure sample homogeneity and avoid particle settling.
For each sample, 3 g of the starch suspension was thoroughly mixed, and vacuum packed in flexible pouches (polymer/aluminium/polymer film OPP30-A19-LDPE70) and then treated under pressure in a laboratory-scale high-pressure unit (U111, Unipress, Warsaw, Poland). The equipment is provided with five high-pressure Cu-Be alloy vessels (inner volume 9 mL) working in parallel, submerged in a thermostatic bath containing silicon oil (M60.115.05, #85321, Novo-direct, Bagsvaerd, Denmark), and can operate at pressures up to 700 MPa and temperatures between −40°C and 100°C.
The prepared samples were treated under 600 MPa, for 5, 10, and 15 min, at room temperature (25°C). All experiments were performed in triplicate. Treated samples were stored at ambient temperature until further analysis.
The degree of gelatinization was evaluated by measuring the loss of birefringence of the starch granules using an optical inverted microscope (Nikon Eclipse, TE 2000S, Nikon Instruments Europe B.V., Amsterdam, Netherlands) with a polarisation filter and a 20× objective coupled to a DS Camera Control Unit (DS-5M-L1, Nikon Instruments Europe B. V., Amsterdam, Netherlands) for image acquisition and analysis. Before observation, a small amount of the sample was spotted on a microscope slide and covered with cover glass. The degree of gelatinization of samples was detected by measuring the loss of the optical birefringence of starch granules under polarized light (20×), calculated according to Eq. 1 (Larrea-Wachtendorff et al., 2019).
where NB is the number of granules with birefringence and N is the total number of counted starch granules.
Additionally, the structuring level of treated samples was assessed by evaluating the efficiency index (EI) according to Eq. 2, as proposed by (Larrea-Wachtendorff et al., 2020).
where Hydrogel formed refers to the drained weight of the structured material.
Response surface methodology was used to gain insights into the significance of the input factors on the response variables, as well as to determine optimal parameters to produce starch-based hydrogels by high-pressure processing (HPP). For this purpose, a two-factor face-centered central composite design (FC-CCD) was chosen to study the effect of holding time and starch concentration on gel formation under pressure. The obtained data were modeled with the quadratic model reported in Eq. 3:
being Yk the predicted response variable, β0 the intercept or regression coefficient, βi, βii and βij the linear and quadratic coefficients, and Xi and Xj the coded values of the process variables.
The swelling power of HPP starch-based hydrogels was determined by modifying the method reported by Kusumayanti et al. (2015) slightly modified according to Larrea-Wachtendorff et al. (2020). Samples HPP-treated under optimized conditions were centrifuged (PK130R, ALC, Winchester, Virginia) at 1,351× g for 10 min and the pellet was weighed before and after drying for 6 h at 105°C. The swelling power ratio, evaluated by Eq. 4, is defined as the weight of the wet pellet over the dry weight of the starch in the hydrogel samples:
A Zetasizer Nano ZS90 (Malvern Instruments, Ltd., Malvern, United Kingdom), based on phase analysis light scattering (PALS), was used to measure the ζ-potential of hydrogels, through the determination of the electrophoretic mobility with He-Ne laser emitting at 633 nm and 4.0 mW power sources at 25°C. Before analyzing the zeta potential, the HPP-treated samples were diluted in distilled water with a dilution factor of 1:30 (w sample/w water).
The mechanical properties of starch-based HPP hydrogels were determined using a controlled stress and strain rheometer AR 2000 (TA Instruments, New Castle, Delaware, United States), equipped with a Peltier plate and a circulating water bath (DC10-Haake K10, Karlsruhe, Germany). A plate-cone geometry (40-mm diameter, 2°) with a fixed gap of 52 μm was used. The samples were loaded in the center of the Peltier plate and left undisturbed for 120 s at 25°C, allowing stress relaxation and temperature equilibration.
Flow curves of hydrogel samples were obtained by altering the shear rate from 0.1 to 100
From frequency sweep tests, recorded between 0.1 and 100 rad/s at 25°C, small deformations of samples were determined within the linear viscoelastic area of the processed samples (3% of strain) The viscoelastic parameters such as the storage or elastic modulus, G′, and the loss or viscous modulus, G″, were obtained and analysed using the abovementioned manufacturer’s software.
Stress sweep tests were carried out to assess the viscoelastic responses of hydrogel samples, namely, the elastic (G′) and viscous (G″) moduli under different stresses (10–1,000 Pa at 25°C).
The texture profile analysis (TPA), a useful tool for characterizing the texture of gummy structures, was carried out on tapioca starch hydrogels using a TA. XT2 texture analyzer (Stable Micro Systems, Surrey, United Kingdom) equipped with a load 5-kg cell connected to a microcomputer. Briefly, 3 g of the samples were loaded into a cylindrical cell (24-mm height and 25-mm ID), and compression-decompression cycles were carried out using a cylindrical probe (10-mm diameter) at room temperature and a rate of 1 mm/s up to attaining 50% of sample deformation. The compression runs were repeated using a decompression rate of 1 mm/s and a delay of 5 s between two bites, to generate force-time curves. The compression data obtained were used to calculate the hardness, adhesiveness, cohesiveness, and gumminess of hydrogels of the sample.
All the experiments on the hydrogels produced were carried out in triplicate, and the results of the analyses are presented as means ± standard deviations. One-way analysis of variance (ANOVA) was used to assess differences between mean values using the statistical software SPSS 20 (SPSS IBM, Chicago, IL, United States). Tukey test was performed to identify statistically significant differences (p < 0.05).
Figure 1 presents the micrographs of starch suspensions that were treated at different processing conditions. Various degrees of gelatinization were observed in the treated samples demonstrating that the starch/water ratio and processing time strongly influence gel formation under HPP treatments. Moreover, the determination of EI (efficiency index) values (Table 1) showed that at starch concentrations higher than 25% (w/w), a highly structured hydrogel was formed, while at concentrations lower than 20% (w/w), a lower-structured hydrogel was obtained. According to these results, it can be suggested that high starch concentrations negatively impact the occurrence of gelatinization under pressure. This effect was already discussed in a paper by (Stute et al., 1996) that at low moisture content gel formation under pressure is hindered and that the damage of starch granules increases proportionally to the pressure level applied and processing time. The predominant interactions of the molecules in the starch-water system are the hydroxyl groups of starch interactions within themselves and with water molecules, thus, with increasing the water content of starch solutions, the number of starch-to-water hydrogen bonds increases, and the number of intramolecular hydrogen bonds decreases. Being the interaction energy in starch-water systems coupled to the number of hydrogen bonds, this redistribution corresponds to a decrease of the interaction energy between the starch chains. Reduced interaction energy and increased distance between starch chains are both manifestations of the plasticizing effect of water on starch, allowing more water to interact with starch granules (Trommsdorff and Tomka, 1995). During HPP processing, water molecules penetrate the starch granules, interact with the amorphous components, and cause the starch granules to swell and lose their hilum-centred birefringence. Therefore, as observed by (Kawai et al., 2007) different hydration degrees and swelling of starch granules occur depending on starch suspension water content and HPP treatment conditions. Nonetheless, it should be pointed out that this behaviour was not observed in all hydrogels obtained in this investigation due to differences in starch structure and properties. (Debet and Gidley, 2006).
FIGURE 1. Birefringence of HPP-treated suspensions: (A)-rice starch hydrogels, (B)-corn starch hydrogels, (C)-wheat starch hydrogels, (D)-tapioca starch hydrogels.
Response surface methodology was used to determine the optimal processing conditions of starch gelation under pressure. Based on the experimental design (FC-CCD), Figure 2 presents a response surface plot that provides insights on how input variables, namely, starch concentration and holding time at high pressure collectively influence the level of starch gelatinization. A quadratic model was selected to fit the data obtained from the experimental design (FC-CCD).
FIGURE 2. Response surfaces of starch-based hydrogels obtained in various starch concentrations (10%–40% w/w) and holding times (5–15min): (A)-rice starch hydrogels, (B)-corn starch hydrogels, (C)-wheat starch hydrogels, (D)-tapioca starch hydrogels.
The results showed that, depending on the starch source as also discussed in section 3.1, the effects of the two variables have different significance on starch gelatinization. The linear effect of starch concentration (β1) is more significant in tapioca and wheat starch hydrogels and less significant in rice starch hydrogels. The quadratic effect of starch concentration (β11)is highly significant in all starch hydrogels obtained, whereas the linear (β2) and quadratic effect (β22) of holding time is not significant for all obtained hydrogels. However, concerning the interactions between individual factors, the alternation of both factors (β12) exerted a significant effect, specifically on wheat and rice starch-based hydrogels.
Table 2 reports the results of the ANOVA for the significant terms of the selected quadratic model and the statistics used to test its adequacy. The p-value of the model suggested that it was significant (p < 0.0001) for the selected response, thus corroborating the effectiveness of the model in describing the experimental data. In addition, the determination coefficient
TABLE 2. Analysis of variance (ANOVA) of the quadratic models for the degree of gelatinization for HPP hydrogels produced from rice, corn, wheat, and tapioca starches.
The results allow identifying the optimal processing conditions of starch-based hydrogels that led to the highest degree of starch gelatinization. The predicted optimal condition was tested experimentally to validate the results. Under optimized conditions, highly structured and stable starch-based HPP hydrogels are obtained as reported in Table 3.
The swelling power is a crucial property that indicates the water-holding capacity of starch, leading to an increase in their volume or size (Kaur et al., 2011). It is generally related to the chain-length distribution of amylopectin, their branching pattern, and molecular weight as discussed by (Jane et al., 1999). This parameter, crucial for assessing the extent of gel formation in HPP-treated samples, was evaluated for all hydrogel samples under optimized processing conditions.
In this investigation, it was observed that tapioca and wheat starch hydrogels showed higher swelling capacity compared to rice and corn, as reported in Table 4. Different factors are reported to influence the swelling of starch granules, such as botanical source, amylopectin/amylose ratio, granule size, protein content, lipid content, and ash content (Sparvoli and Cominelli, 2015). Moreover, it is well known that amylose can form a helical structure under pressure treatments together with other substances, such as iodine, lipids, alcohols, and fat-soluble bioactives. The amylose–liquid complex entangles amylopectin molecules, restricting the swelling of starch granules and enzyme hydrolysis (Katopo et al., 2002).
TABLE 4. Water-holding capacity and efficiency index of starch-based hydrogels under optimized processing conditions.
Furthermore, all samples showed a highly structured level, suggesting that not only starch source and processing time (Larrea-Wachtendorff et al., 2020) but also starch/water ratio affects the structuring level of starch-based HPP hydrogels.
Zeta potential was evaluated to get information on droplets’ electrostatic repulsion (Heydari, Razavi, and Farahnaky, 2021), and it represents an important parameter to predict the long-term stability of a colloidal dispersion including polymer hydrogels.
The results of the measurements of zeta potential of starch-based HPP hydrogels are presented in Table 5. Depending on the range of zeta potential values, hydrogels based on rice, corn, and wheat starches can be considered relatively stable, whereas tapioca starch hydrogels can be considered moderately stable. Many factors impact the static stability of a colloidal system such as pH of the solution, conductivity, particle size, and concentration of components within the formulation (Yukselen-Aksoy and Kaya, 2011). Our results suggest that static stability in starch-based HPP hydrogels is mostly affected by starch source, which includes factors such as amylose/amylopectin ratios, granule sizes, and branching patterns. Additionally, the starch concentrations exert a significant impact on the static stability of HPP hydrogels, primarily through the direct influence on molecular interactions occurring during the gelatinization process. Measuring the static stability of hydrogels is crucial because it refers to the ability of a hydrogel to maintain its structural integrity over time. However, it is worth noting that the stability of hydrogel structures depends on the sum of Van der Waals attractive forces and electrostatic repulsive forces (Kamble et al., 2022). Zeta potential provides information on the repulsive electrostatic forces, while does not consider the Van der Waals force. Thus, further characterizations to evaluate the stability of the structure are presented in the section below.
TABLE 5. Zeta potential values of starch-based hydrogels obtained under optimized processing conditions.
Starch-based HPP hydrogels exhibit excellent structural integrity and uniformity. Corn, rice, and wheat hydrogels, which showed a cream-like appearance, were further characterized to evaluate their mechanical properties, flow behaviour, and viscoelastic properties. The results showed that hydrogels exhibit a shear-thinning non-Newtonian behavior (Xie et al., 2009; Jiang et al., 2015). Compared to Newtonian fluids, which exhibit a constant viscosity regardless of the shear rate, gels display changes in their flow properties when subjected to increasing shear stresses (Dzuy Nguyen, Jensen, and Kristensen, 1998).
As depicted in Figure 3, in the entire range of shear rates applied, corn starch hydrogels have the highest viscosity values, confirming that they show the highest resistance to flow. The viscosity of rice and wheat starch hydrogels was much lower, typical of spreadable materials that cannot withstand flow, and have a weaker structure compared to corn starch hydrogels. Moreover, the mechanical profiles of HPP hydrogels based on rice, corn, and wheat starches obtained in this investigation (Figure 4; Figure 5) revealed that the gel structures were strong, with the elastic response being one order of magnitude higher than the viscous response (G' > G″). Both moduli are independent on frequency demonstrating that hydrogels have stable, continuous, and well-structured cross-linked networks (Fradinho et al., 2019). The mechanical profile of corn starch hydrogels was stronger than that of rice and wheat hydrogels due to the higher starch concentration used for their formulation.
FIGURE 4. Influence of frequency on the elastic response (G′ moduli) of starch-based hydrogels after HPP treatments.
FIGURE 5. Influence of frequency on the viscous response (G″ moduli) of starch-based hydrogels after HPP treatments.
The network strength of starch-based HPP hydrogels was further evaluated through stress sweep tests and the results of the measurements are illustrated in Figures 6, 7. All treated samples showed the typical behavior of gel structures, with elastic properties predominating over the viscous ones (G’ >> G″). The results highlighted that corn starch hydrogels had the highest network strength considering the linear viscoelasticity range (LVR), which indicates the range of deformation stress that a viscoelastic material withstands. Thus, the wider the extension of the LVR, the higher the network strength. Rheological instabilities were measured in rice starch hydrogels at 1,000 MPa and in wheat starch hydrogels at 500 MPa, confirming the weakness of these structures.
FIGURE 6. Influence of deformation stress on the elastic response (G′ moduli) of starch-based hydrogels after HPP treatments.
FIGURE 7. Influence of deformation stress on the viscous response (G″ moduli) of starch-based hydrogels after HPP treatments.
Furthermore, the TPA test was performed on tapioca starch HPP hydrogels that had a rubber-like structure. As reported in Table 6, tapioca starch hydrogels showed high hardness values, with the highest force needed to initially compress the sample. Additionally, the cohesiveness of samples, which is related to the magnitude of macrostructure damage after the first compression, was evaluated (Pure et al., 2021). Tapioca hydrogels showed excellent cohesiveness, confirming the strength of internal bonds (Gokhale et al., 2019). Based on the gumminess value calculated, which accounts for the force needed to reduce semisolid food to a soft and ready-to-swallow bolus, it can be again confirmed that tapioca starch hydrogels have a firm structure. Moreover, the treated samples showed high springiness values, which corresponds with the ability of materials to recover their original shape after compression.
The data obtained in this research allowed to better understand the structural and mechanical properties of starch-based hydrogels produced at optimized starch concentration and processing time conditions.
The results of this work demonstrate that the optimization of processing conditions to produce starch-based hydrogels under high pressure is a key step to obtain structured and stable structures with suitable formulation to be utilized in various sectors. Experimental design using a quadratic model was an efficient tool for the optimization of the processing condition of starch-based HPP hydrogels. The two parameters tested, namely, starch concentration in the water solution and holding time at high pressure, were revealed to affect the gelatinization degree to different extents depending on the starch source. Among others, the quadratic effect of starch concentration is highly significant in all starch hydrogels obtained in this work. Under optimized conditions, HPP hydrogels showed different physical appearances and rheological properties. HPP hydrogels produced from cereal starches, such as rice, wheat, and corn, showed a cream-like structure. Among them, corn starch hydrogels had the highest resistance to flow, stronger mechanical properties, and the highest network strength. Tapioca starch hydrogels, instead, had gummy-like structures and showed high hardness values and strong internal bonds. Moreover, tapioca and wheat starch hydrogels showed higher water-holding capacity compared to rice and corn starch hydrogels. It should be noted that the differences in structure and properties are affected by various factors, the more crucial being the HPP processing conditions and the starch botanical source.
The raw data supporting the conclusion of this article will be made available by the authors, without undue reservation.
KK: Conceptualization, Formal Analysis, Data curation, Investigation, Methodology, Validation, Writing–original draft. GF: Conceptualization, Formal Analysis, Funding acquisition, Supervision, Writing–review and editing.
The author(s) declare financial support was received for the research, authorship, and/or publication of this article. This work was financially supported by ProdAl Scarl (own funds) and the University of Salerno (Fund FARB 2020 n. 300395FRB20 FERRA), Italy.
The authors declare that the research was conducted in the absence of any commercial or financial relationships that could be construed as a potential conflict of interest.
The author(s) declared that they were an editorial board member of Frontiers, at the time of submission. This had no impact on the peer review process and the final decision.
All claims expressed in this article are solely those of the authors and do not necessarily represent those of their affiliated organizations, or those of the publisher, the editors and the reviewers. Any product that may be evaluated in this article, or claim that may be made by its manufacturer, is not guaranteed or endorsed by the publisher.
The Supplementary Material for this article can be found online at: https://www.frontiersin.org/articles/10.3389/frfst.2024.1376044/full#supplementary-material
Barba, F. J., Shiferaw, N., Buckow, R., Knorr, D., and Orlien, V. (2015). New opportunities and perspectives of high pressure treatment to improve health and safety attributes of foods. A review. FRIN 77, 725–742. doi:10.1016/j.foodres.2015.05.015
Bauer, B. A., and Knorr, D. (2005). The impact of pressure, temperature and treatment time on starches: pressure-induced starch gelatinisation as pressure time temperature indicator for high hydrostatic pressure processing. J. Food Eng. 68, 329–334. doi:10.1016/j.jfoodeng.2004.06.007
Biduski, B., Max, W., Colussi, R., Lisie, S., El, D. M., Lim, L., et al. (2018). Starch hydrogels: the influence of the amylose content and gelatinization method. Int. J. Biol. Macromol. 113, 443–449. doi:10.1016/j.ijbiomac.2018.02.144
Błaszczak, W., Fornal, J., Kiseleva, V., Yuryev, V., Sergeev, A., and Sadowska, J. (2007). Effect of high pressure on thermal, structural and osmotic properties of waxy maize and Hylon VII starch blends. Carbohydr. Polym. 68, 387–396. doi:10.1016/j.carbpol.2006.12.023
Caló, E., and Khutoryanskiy, V. V. (2015). Biomedical applications of hydrogels: a review of patents and commercial products. Eur. Polym. J. 65, 252–267. doi:10.1016/j.eurpolymj.2014.11.024
Cui, C., Jia, Y., Sun, Q., Yu, M., Ji, N., Dai, L., et al. (2022). Recent advances in the preparation, characterization, and food application of starch-based hydrogels. Carbohydr. Polym. 291, 119624. doi:10.1016/j.carbpol.2022.119624
Debet, M. R., and Gidley, M. J. (2006). Three classes of starch granule swelling: influence of surface proteins and lipids. Carbohydr. Polym. 64, 452–465. doi:10.1016/j.carbpol.2005.12.011
Dzuy Nguyen, Q., Jensen, T. B., and Kristensen, P. G. (1998). Chemical Engineering Journal ELSEVIER Experimental and modelling studies of the flow properties of maize and waxy maize starch pastes.
Edgar, K. J., and Marks, J. A. (2020). “Green hydrogels based on starch: preparation methods for biomedical applications,” in ACS symposium series (American Chemical Society), 173–196. doi:10.1021/bk-2020-1372.ch010
Fradinho, P., Sousa, I., and Raymundo, A. (2019). Functional and thermorheological properties of rice flour gels for gluten-free pasta applications. Int. J. Food Sci. Technol. 54, 1109–1120. doi:10.1111/ijfs.14001
Gokhale, J. P., Mahajan, H. S., and Surana, S. S. (2019). Quercetin loaded nanoemulsion-based gel for rheumatoid arthritis: in vivo and in vitro studies. Biomed. Pharmacother. 112, 108622. doi:10.1016/j.biopha.2019.108622
Halley, P. J., Truss, R. W., Markotsis, M. G., Chaleat, C., Russo, M., Sargent, A. L., et al. (2007). “A review of biodegradable thermoplastic starch polymers,” in Polymer durability and radiation effects, 287–300. doi:10.1021/bk-2007-0978.ch024
Heydari, A., Razavi, S. M. A., and Farahnaky, A. (2021). Effect of high pressure-treated wheat starch as a fat replacer on the physical and rheological properties of reduced-fat O/W emulsions. Innovative Food Sci. Emerg. Technol. 70, 102702. doi:10.1016/j.ifset.2021.102702
Hoffman, A. S. (2012). Hydrogels for biomedical applications. Adv. Drug Deliv. Rev. 64, 18–23. doi:10.1016/j.addr.2012.09.010
Idrees, H., Zaidi, S. Z. J., Sabir, A., Khan, R. U., Zhang, X., and Hassan, S. U. (2020). A review of biodegradable natural polymer-based nanoparticles for drug delivery applications. Nanomaterials 10, 1–22. doi:10.3390/nano10101970
Ismail, H., Irani, M., and Ahmad, Z. (2013). Starch-based hydrogels: present status and applications. Int. J. Polym. Mater. Polym. Biomaterials 62, 411–420. doi:10.1080/00914037.2012.719141
Jiang, B., Li, W., Shen, Q., Hu, X., Wu, J., Jiang, B., et al. (2015). Effects of high hydrostatic pressure on rheological properties of rice starch. Int. J. Food Prop. 18, 1334–1344. doi:10.1080/10942912.2012.709209
Kamble, S., Agrawal, S., Cherumukkil, S., Sharma, V., Jasra, R. V., and Munshi, P. (2022). Revisiting zeta potential, the key feature of interfacial phenomena, with applications and recent advancements. ChemistrySelect 7. doi:10.1002/slct.202103084
Katopo, H., Song, Y., and Jane, J. L. (2002). Effect and mechanism of ultrahigh hydrostatic pressure on the structure and properties of starches. Carbohydr. Polym. 47, 233–244. doi:10.1016/S0144-8617(01)00168-0
Kaur, M., Oberoi, D. P. S., Sogi, D. S., and Gill, B. S. (2011). Physicochemical, morphological and pasting properties of acid treated starches from different botanical sources. J. Food Sci. Technol. 48, 460–465. doi:10.1007/s13197-010-0126-x
Kawai, K., Fukami, K., and Yamamoto, K. (2007). Effects of treatment pressure, holding time, and starch content on gelatinization and retrogradation properties of potato starch-water mixtures treated with high hydrostatic pressure. Carbohydr. Polym. 69, 590–596. doi:10.1016/j.carbpol.2007.01.015
Knorr, D., Heinz, V., and Buckow, R. (2006). High pressure application for food biopolymers. Biochim. Biophys. Acta Proteins Proteom 1764, 619–631. doi:10.1016/j.bbapap.2006.01.017
Kusumayanti, H., Handayani, N. A., and Santosa, H. (2015). Swelling power and water solubility of cassava and sweet potatoes flour. Procedia Environ. Sci. 23, 164–167. doi:10.1016/j.proenv.2015.01.025
Larrea-Wachtendor, D., Tabilo-munizaga, G., and Ferrari, G. (2019). Potato starch hydrogels produced by high hydrostatic pressure (HHP): a first approach. Polym. (Basel) 11, 1673. doi:10.3390/polym11101673
Larrea-Wachtendorff, D., Sousa, I., and Ferrari, G. (2020). Starch-based hydrogels produced by high-pressure processing (HPP): effect of the starch source and processing time. Food Eng. Rev. 13, 622. doi:10.1007/s12393-020-09264-7/Published
Laycock, B. G., and Halley, P. J. (2014). “Starch applications: state of market and New trends,” in Starch polymers: from genetic engineering to green applications (Elsevier BV), 381–419. doi:10.1016/B978-0-444-53730-0.00026-9
Leite, T. S., de Jesus, A. L. T., Schmiele, M., Tribst, A. A. L., and Cristianini, M. (2017). High pressure processing (HPP) of pea starch: effect on the gelatinization properties. LWT 76, 361–369. doi:10.1016/j.lwt.2016.07.036
Liu, D., Zhao, P., Chen, J., Yan, Y., and Wu, Z. (2022). Recent advances and applications in starch for intelligent active food packaging: a review. Foods 11, 2879. doi:10.3390/foods11182879
Mohammadinejad, R., Maleki, H., Larrañeta, E., Fajardo, A. R., Nik, A. B., Shavandi, A., et al. (2019). Status and future scope of plant-based green hydrogels in biomedical engineering. Appl. Mater Today 16, 213–246. doi:10.1016/j.apmt.2019.04.010
Parente, M. E., Ochoa Andrade, A., Ares, G., Russo, F., and Jiménez-Kairuz, A. (2015). Bioadhesive hydrogels for cosmetic applications. Int. J. Cosmet. Sci. 37, 511–518. doi:10.1111/ics.12227
Pei-Ling, L., Xiao-Song, H., and Qun, S. (2010). Effect of high hydrostatic pressure on starches: a review. Starch/Staerke 62, 615–628. doi:10.1002/star.201000001
Peppas, N. A., Bures, P., Leobandung, W., and Ichikawa, H. (2000). Hydrogels in pharmaceutical formulations. Eur. J. Pharm. Biopharm. 50, 27–46. doi:10.1016/S0939-6411(00)00090-4
Pires, P. C., Mascarenhas-Melo, F., Pedrosa, K., Lopes, D., Lopes, J., Macário-Soares, A., et al. (2023). Polymer-based biomaterials for pharmaceutical and biomedical applications: a focus on topical drug administration. Eur. Polym. J. 187, 111868. doi:10.1016/j.eurpolymj.2023.111868
Pulgarín, O., Larrea-Wachtendorff, D., and Ferrari, G. (2023). Effects of the amylose/amylopectin content and storage conditions on corn starch hydrogels produced by high-pressure processing (HPP). Gels 9, 87. doi:10.3390/gels9020087
Pure, A. E., Yarmand, M. S., Farhoodi, M., and Adedeji, A. (2021). Microwave treatment to modify textural properties of high protein gel applicable as dysphagia food. J. Texture Stud. 52, 638–646. doi:10.1111/jtxs.12611
Qamruzzaman, M., Ahmed, F., and Mondal, M. I. H. (2022). An overview on starch-based sustainable hydrogels: potential applications and aspects. J. Polym. Environ. 30, 19–50. doi:10.1007/s10924-021-02180-9
Salimi, M., Channab, B. E., El Idrissi, A., Zahouily, M., and Motamedi, E. (2023). A comprehensive review on starch: structure, modification, and applications in slow/controlled-release fertilizers in agriculture. Carbohydr. Polym. 322, 121326. doi:10.1016/j.carbpol.2023.121326
Samir, A., Ashour, F. H., Hakim, A. A. A., and Bassyouni, M. (2022). Recent advances in biodegradable polymers for sustainable applications. Npj Mater Degrad. 6, 68. doi:10.1038/s41529-022-00277-7
Sparvoli, F., and Cominelli, E. (2015). Nutritional value (of legumes) special issue “phytic acid and mineral biofortification strategies: from plant science to breeding and biotechnological approaches” view project bio belief: BIOfortification of common bean to promote heaLthy dIEt and food security in a context of climatic variation view project. doi:10.1007/9781493927975
Stute, R., Klingler, R. W., Boguslawski, S., Eshtiaghi, M. N., Knorr, D., and Germany, B. (1996). Effects of high pressures treatment on starches. 42, 200–206.
Trommsdorff, U., and Tomka, I. (1995). Structure of amorphous starch. 2. Molecular interactions with water. Macromolecules 28, 6138–6150. doi:10.1021/ma00122a022
Vittadini, E., Carini, E., Chiavaro, E., Rovere, P., and Barbanti, D. (2008). High pressure-induced tapioca starch gels: physico-chemical characterization and stability. Eur. Food Res. Technol. 226, 889–896. doi:10.1007/s00217-007-0611-2
Xiao, C. (2013). Current advances of chemical and physical starch-based hydrogels. Starch/Staerke 65, 82–88. doi:10.1002/star.201200113
Xie, F., Yu, L., Su, B., Liu, P., Wang, J., Liu, H., et al. (2009). Rheological properties of starches with different amylose/amylopectin ratios. J. Cereal Sci. 49, 371–377. doi:10.1016/j.jcs.2009.01.002
Yamamoto, K., and Buckow, R. (2016). Pressure gelatinization of starch. High pressure processing of food. 433–459. doi:10.1007/978-1-4939-3234-4
Yang, Z., Chaib, S., Gu, Q., and Hemar, Y. (2017). Impact of pressure on physicochemical properties of starch dispersions. Food Hydrocoll. 68, 164–177. doi:10.1016/j.foodhyd.2016.08.032
Keywords: starch-based hydrogels, high-pressure processing, optimal processing conditions, response surface methodology, stable structures
Citation: Koshenaj K and Ferrari G (2024) Optimization of processing conditions of starch-based hydrogels produced by high-pressure processing (HPP) using response surface methodology. Front. Food. Sci. Technol. 4:1376044. doi: 10.3389/frfst.2024.1376044
Received: 24 January 2024; Accepted: 01 March 2024;
Published: 13 March 2024.
Edited by:
Xianyang Bao, Harvard University, United StatesReviewed by:
Zhili Ji, Wuhan Polytechnic University, ChinaCopyright © 2024 Koshenaj and Ferrari. This is an open-access article distributed under the terms of the Creative Commons Attribution License (CC BY). The use, distribution or reproduction in other forums is permitted, provided the original author(s) and the copyright owner(s) are credited and that the original publication in this journal is cited, in accordance with accepted academic practice. No use, distribution or reproduction is permitted which does not comply with these terms.
*Correspondence: Giovanna Ferrari, Z2ZlcnJhcmlAdW5pc2EuaXQ=
Disclaimer: All claims expressed in this article are solely those of the authors and do not necessarily represent those of their affiliated organizations, or those of the publisher, the editors and the reviewers. Any product that may be evaluated in this article or claim that may be made by its manufacturer is not guaranteed or endorsed by the publisher.
Research integrity at Frontiers
Learn more about the work of our research integrity team to safeguard the quality of each article we publish.