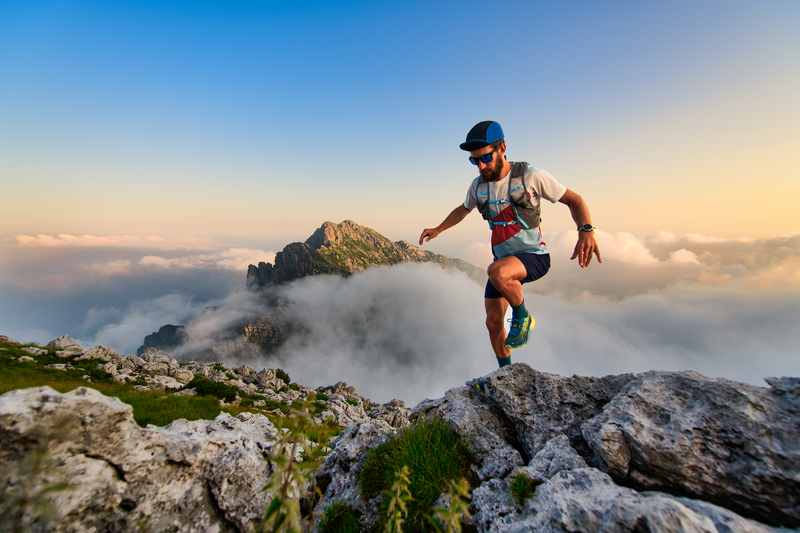
95% of researchers rate our articles as excellent or good
Learn more about the work of our research integrity team to safeguard the quality of each article we publish.
Find out more
ORIGINAL RESEARCH article
Front. Food. Sci. Technol. , 28 February 2024
Sec. Food Safety and Quality Control
Volume 4 - 2024 | https://doi.org/10.3389/frfst.2024.1309884
This article is part of the Research Topic The Future of Foods View all 9 articles
Cultivated meat, a sustainable alternative to traditional livestock farming, has gained attention for its potential environmental and health benefits. However, concerns about microplastic contamination pose challenges, especially when sourcing cells from marine organisms prone to microplastic bioaccumulation. Additionally, the pervasive presence of microplastics in laboratory settings, ingredients, and during the production, increases the risk of unintentional contamination. This study focused on Atlantic mackerel (Scomber scombrus) skeletal muscle cell lines to examine the effects of microplastic exposure, represented by fluorescent polyethylene microspheres (10–45 µm) on cell performance including cell proliferation, cell viability, gene expression, and differentiation processes critical for cultivated meat production. The results revealed significant impacts on cell attachment and proliferation at microplastic concentrations of 1 μg/mL, 10 μg/mL, and 50 μg/mL. Notably, the 10 μg/mL concentration exerted the most pronounced effects on cell viability during both attachment and proliferation phases. While the results indicated that both microplastic concentration and size influence cell viability, cell differentiation remained unaffected, and additional contributing factors require further investigation. These findings underscore the necessity of thoroughly exploring microplastic-cell interactions to ensure food safety and safeguard health within the burgeoning cultivated meat industry.
Cultivated meat, derived from the cultivation of animal cells, presents an innovative shift in food production with potential environmental and health benefits (Rischer et al., 2020; Eibl et al., 2021; Dupuis et al., 2023; Jahir et al., 2023). Produced within controlled environments, this approach not only minimizes the risks associated with conventional farming contaminants but also promises a more resource-efficient methodology (Stephens et al., 2018). Recent studies indicate that, with renewable energy integration, cultivated meat could achieve up reductions of global warming by 92%, air pollution by 93%, land use by 95%, and water consumption by 78% compared to traditional beef farming (Vergeer et al., 2021; Kim et al., 2022; Sinke et al., 2023). As the industry advances, cultivated meat is projected to command a substantial portion of the $1.7 trillion conventional meat and seafood market, addressing pressing challenges like deforestation, biodiversity loss, and antibiotic resistance (Vergeer et al., 2021; Sinke et al., 2023).
In controlled laboratory environments, cultivated meat is produced from cells, such as those from animals. These cells undergo proliferation in specialized growth mediums to form muscle tissue, representing a potentially safer, more ethical, and environmentally sustainable alternative to conventional meat production (Chriki and Hocquette, 2020; Ong et al., 2021). However, a potential safety concern in this innovation is the contamination of microplastics. One avenue of potential contamination arises from the source animals. Marine ecosystems, for instance, are known reservoirs of microplastics (Andrady, 2011; Cole et al., 2011; Ivar do Sul and Costa, 2014). This results in bioaccumulation within marine life, such as fish and oysters (Galloway et al., 2017; Sharma and Chatterjee, 2017; Bhuyan, 2022; Courtene-Jones et al., 2022). When such marine organisms serve as the source animals for cell extraction, undetected microplastics could be inadvertently introduced into the cultivation process. Existing analytical methodologies often fail to detect smaller microplastic particles, leading to potential underestimations of their abundance in source organisms (Huppertsberg and Knepper, 2018; Lv et al., 2021; Vivekanand et al., 2021; Adhikari et al., 2022). Another significant source of contamination is the laboratory environment itself. Studies have underscored the pervasive nature of microplastics in laboratory settings, emanating from the degradation of ubiquitous plastic equipment, containers, and consumables (Löder et al., 2017; Schymanski et al., 2018; Koelmans et al., 2019). The production process of cultivated meat necessitates the use of various plastic-based apparatus, including bioreactors, pipettes, cell culture flasks, and other equipment that come in direct contact with the medium and growing cells (Allan et al., 2019; Lee et al., 2022).
Microplastics, tiny fragments of plastic less than 5 mm in size, have garnered significant attention due to their ubiquity in the environment and the potential risks they pose to human health (Lim, 2021; Diamantidou et al., 2022; Leslie et al., 2022; Lwanga et al., 2022; Osman et al., 2023; Tsochatzis et al., 2023). Upon ingested, these particles can traverse the gastrointestinal tract, and some evidence suggests that smaller micro- and nanoplastic particles may even penetrate tissues, entering the circulatory and lymphatic systems (Campanale et al., 2020; Hirt and Body-Malapel, 2020; Jiang et al., 2020; Kannan & Vimalkumar, 2021; Yee et al., 2021; Diamantidou et al., 2022; Leslie et al., 2022; Fournier et al., 2023; Li et al., 2023; Ramsperger et al., 2023; Tsochatzis et al., 2023). These fragments can act as carriers for various toxicants, including heavy metals, polycyclic aromatic hydrocarbons, and endocrine-disrupting chemicals (Campanale et al., 2020; Abbasi et al., 2021; Amelia et al., 2021; Yee et al., 2021; Karla Lizzeth et al., 2023), thereby introducing these harmful agents into the human body. From a cellular perspective, the risks of microplastics become more intricate. The direct interaction between cells and microplastics can lead to physical disruptions, such as membrane damage (Fleury and Baulin, 2021; Dai et al., 2022; Wang et al., 2022), and chemicals inherent to or leached from these plastics are known to induce oxidative stress, inflammatory responses, and genotoxic effects (Hirt and Body-Malapel, 2020; Goodman et al., 2021; Alqahtani et al., 2023; Cao et al., 2023; Herrala et al., 2023; Jeyavani et al., 2023; Mattioda et al., 2023). Potential risks of such interactions encompass DNA lesions, organ dysfunctions, metabolic irregularities, immunological aberrations, neurotoxicity, and perturbations in reproductive and developmental processes (Galluzzi et al., 2018). Furthermore, previous research has indicated a potential link between microplastic exposure and the development or exacerbation of certain chronic diseases such as cardiovascular diseases including thrombosis, atherosclerosis, cancer, and diabetes (Lee et al., 2023; Wu et al., 2023). Given the documented adverse effects of microplastics upon ingestion, understanding and mitigating these risks is paramount for the cultivated meat industry (EFSA Panel on Contaminants in the Food Chain CONTAM, 2016; Rubio-Armendáriz et al., 2022; Mamun et al., 2023; Ziani et al., 2023).
While the presence and potential hazards of microplastics are increasingly acknowledged, understanding the exact mechanisms by which they influence cellular functions remains a critical research frontier (O’Neill and Lawler, 2021; Thornton Hampton et al., 2022). To understand the cellular impacts of microplastic exposure more comprehensively, we utilized Atlantic mackerel (Scomber scombrus) skeletal muscle cell lines, previously established and characterized by Saad et al. (2023), given their relevance to cultivated meat processing. This study employed fluorescent polyethylene microspheres (10–45 µm) as representative microplastics, a size range previously documented in fish (Thiele et al., 2021; Makhdoumi et al., 2023). We aimed to elucidate the effects of microplastics on cellular performance, emphasizing cell viability during the attachment and growth phases, as well as cell differentiation, which are pivotal processes in cultivated meat production (O'Neill et al., 2021; Reiss et al., 2021). The study employed microplastic concentrations of 1 μg/mL, 10 μg/mL, and 50 μg/mL. Preliminary results indicated that all treatments significantly affected cell attachment (on day 2) and proliferation (on day 4), with no discernible effects on cell differentiation after 2 weeks. Variables such as microplastic size and concentration potentially influenced these outcomes. These findings, albeit initial, provide foundational insights for subsequent research, emphasizing the importance of understanding microplastic-cell interactions for ensuring food safety, protecting human health, and mitigating environmental impacts.
The mackerel cell lines (MACK2) used in this study were obtained from Dr. David L. Kaplan Lab at Tufts University. The cell preparation followed the protocol described in Saad et al. (2023). Briefly, frozen cells at passage 81 were thawed using 9 mL of complete growth medium, which comprised Leivovitz’s L-15 medium (Gibco™, Billings, MT, United States) and supplemented with 20% fetal bovine serum (FBS, Gibco™, Billings, MT, United States), 1 ng/mL FGF2-G3 (human) growth factor (Defined Bioscience, San Diego, CA, United States), 20 mM HEPES (Gibco™, Billings, MT, United States), and 1% Antibiotic–Antimycotic (Gibco™, Billings, MT, United States). The cell suspension was then centrifuged at 500 RCF for 6 min, and the resulting pellet was resuspended in 10 mL of growth medium. The cells were incubated in a 75 cm2 culture flask (Thermo Fisher Scientific, Waltham, MA, United States) at 27°C in a CO2 free incubator. Maintenance of the cells involved regular passaging at approximately 70% confluency and seeding at a density of approximately 5000 cells/cm2. Alternatively, cells were stored by freezing in growth medium supplemented with 10% dimethyl sulfoxide (DMSO, Sigma Aldrich, St. Louis, MO, US).
Fluorescent green polyethylene microspheres obtained from Cospheric LLC (Goleta, CA, United States) were used in this study. The microspheres exhibited a size range of 10–45 µm. Prior to experiments, a sterilization process was undertaken using 91% isopropyl alcohol (IPA), allowing excess fluid to drain as the spheres gradually underwent evaporation. Subsequently, the sterilized microspheres were integrated into the completed growth medium supplemented with 0.01% Tween 20. Microspheres were introduced into the experimental setup at concentrations of 1, 10, and 50 μg/mL based on previous studies (Schirinzi et al., 2017; Hwang et al., 2020; Palaniappan et al., 2022). An experimental control consisting of the complete growth medium with 0.01% Tween 20 but devoid of microspheres was included in the study. To achieve a consistent distribution of the microspheres within the growth medium, a pre-experimental step involved subjecting the microsphere medium to sonication prior to each experiment.
Mackerel cells were seeded in triplicate in the 6-well plates at a seeding density of approximately 5000 cells/cm2. The cells were cultured at a constant temperature of 27°C and devoid of CO2. To investigate the influence of microplastics on different stages of cell growth, two distinct experimental conditions were employed. In the first scenario, microplastics were incorporated into the cell medium prior to seeding to assess their influence on cell attachment, a crucial initial step in cell proliferation. After 48 h of incubation, cells reached the logarithmic growth phase and were detached from the plate surface using 0.25% trypsin–EDTA (Thermo Fisher Scientific, Waltham, MA, United States). The cell viability was evaluated using the Trypan Blue Assay and the Countess 3 FL Automated Cell Counter (Invitrogen™, Thermo Fisher Scientific, Waltham, MA, United States). This assay exploits differential cellular uptake of Trypan Blue dye to discern and enumerate viable versus non-viable cells. Instrument parameters, including counting thresholds and dye dilution ratios, were set in accordance with the manufacturer’s specifications to ensure accuracy and reproducibility of the viability metrics, In the second scenario, microplastics were introduced to the cell medium after the logarithmic growth phase was attained, with the old medium replaced by either microplastic-containing or control fresh medium. Subsequent to the introduction of microplastics, the cells remained undisturbed for a period of 4 days, allowing for the exploration of potential interactions between microplastics and the cells. After this interaction period, the cells were detached, and the viable cell count was determined, offering insights into the effect of microplastics on cell growth after the initial proliferation stages.
Mackerel cells at passage 82 were detached from 6-well plates using trypsin for 3–4 min and pelleted by a 7.5 min centrifugation at 500 RCF. RNA was extracted from samples using the NucleoSpin RNA kit (Mackerey-Nagel, Dueren, Germany) and quantified with a Qubit 4 Fluorometer using the Qubit RNA High Sensitivity (HS) assay kit (Thermo Fisher Scientific, Waltham, MA, United States). cDNA libraries for each sample were constructed from 100 ng of RNA using the PrimeScript RT master mix (Takara Bio, Kutatsu, Japan) under manufacturer specifications. A minus reverse transcriptase control (-RT) was made from the sample exhibiting the higher RNA yield by not adding the master mix to the sample. The PrimeScript RT master mix contains both random hexamers and oligo dT primers. RT-qPCR was performed in a CFX Opus 96 thermocycler (Bio-Rad, Hercules, CA, United States) using the TB Green Advantage qPCR premix (Takara Bio, Kutatsu, Japan) and the oligonucleotides specified in Table 1. Primers were designed using a reference genome for southern bluefin tuna (Thunnus maccoyii; NBCI RefSeq GCF_910596095.1) by Saad et al. (2023). Three technical replicates were performed per sample and gene, as well as for the—RT and no template controls (NTC). Amplification conditions were as follows: initial step of 30 s at 95°C, followed by 40 cycles of 5 s at 95°C and 30 s at 60°C, and a final dissociation analysis of 15 s at 65°C and 0.5°C/s increases until reaching 95°C. Absolute gene expression values were calculated as 2^(-ΔCt) using the hypoxanthine guanine phosphoribosyltransferase (HPRT) gene as the housekeeping gene. Relative gene expression values were calculated as 2^(-ΔΔCt) over the 0 ng/mL microplastics control.
To observe the influence of microplastics on cell differentiation, immunostaining for myosin heavy chain was conducted following the protocol outlined in Saad et al. (2023). Mackerel cells were cultured at 100% confluency in growth medium and exposed to microplastics at varying concentrations, with subsequent observation over a 14-day differentiation period. Following culture, cells were fixed with 4% paraformaldehyde at room temperature for 30 min (Thermo Fisher Scientific, Waltham, MA, United States). Subsequently, the cells were rinsed with Phosphate Buffered Saline (PBS, Sigma Aldrich, Burlington, MA, United States) and permeabilized for 10 min using 0.1% Triton-X (Sigma Aldrich, Burlington, MA, United States). Subsequent to permeabilization, the cells were blocked for 30 min using 1 × blocking buffer (Abcam, Cambridge, United Kingdom), followed by an additional PBS wash. The primary antibody solution, MF-20 (4 μg/mL), was applied to the cells and allowed to incubate overnight at 4°C. After a subsequent PBS wash, the cells underwent an additional 30-min blocking step using 1 × blocking buffer and were then incubated for 1 h with secondary antibodies—Goat Anti-Mouse IgG H&L (Alexa Fluor® 594, Abcam, Cambridge, United Kingdom), and Phalloidin-iFluor 488 Reagent (Abcam, Cambridge, United Kingdom)—each diluted at 1:1000 in 1 × blocking buffer. After a final wash with PBS, the cell nuclei were stained with 4′,6-diamidino-2-phenylindole (DAPI, 1 μg/mL, Thermo Fisher Scientific, Waltham, MA, United States) in PBS for 15 min at room temperature. Imaging was conducted using a fluorescence microscope (DP27, Olympus Life Science, Tokyo, Japan) equipped with an LED Illumination system (CoolLED, Andover, United Kingdom). Multiple objective lenses (4x, 10x, 20x) were utilized to evaluate the cell morphology and structure at various levels of detail. Images taken with 4x and 10x lenses were used for general orientation and lower-resolution overviews, while the 20x lens was employed for detailed visualization necessary for the conclusions.
Statistical analysis was conducted using One-way ANOVA, with a t-test utilized for comparisons between two parameters using Minitab Statistical Software.
The viability of cells during both the attachment and growth phases is paramount to the cultivation of meat. In the attachment phase, cells must successfully anchor to a scaffold or matrix to prevent their loss during media changes, establishing a robust foundation for subsequent stages. Following successful attachment, it is imperative for these cells to proliferate efficiently, ensuring an adequate cellular population for muscle tissue formation. Any significant cell mortality or diminished proliferation during these phases could undermine the entire production yield and efficiency (Allan et al., 2019; Bodiou et al., 2020). In order to investigate the effects of microplastics on mackerel cell viability at attachment and growth phases, microplastics were introduced to cells at different times in this study. Figure 1A highlighted the outcomes of microplastics on initial cell attachment when introduced into the cell medium before seeding, and statistical analysis revealed a highly significant difference in cell counts between the groups (ANOVA, p < 0.001). Conversely, Figure 1B depicted the impact of microplastics on cell proliferation when added after the cells had reached the logarithmic growth phase, also demonstrated statistically significant alterations in proliferation rates (ANOVA, p < 0.001). The initial number of cells for seeding was -5000 cells/cm2, and the surface area of the 6-well is 9.6 cm2. Data collection occurred on Day 2 for Figure 1A and Day 4 for Figure 1B, corresponding with a marked increase in cell death and detachment. In Figure 1A, the experimental control group (no microplastic) showed a cell count of 410,000, whereas microplastic-treated cells evidenced a decline to approximately half this number. Similarly, Figure 1B displayed a control cell count of 1,100,000, with those treated with microplastics showing a range from 510,000 to 730,000. Notably, the presence of microplastics led to a decrease in cell counts, with the 10 μg/mL concentration consistently showing the lowest viability. The underlying causes and potential mechanisms responsible for this trend are explored in detail in the subsequent sections.
FIGURE 1. Effects of microplastics on cell viability. (A) Influence of microplastics on initial cell attachment when introduced to the cell medium prior to seeding. (B) Impact of microplastics on cell proliferation after cells reached the logarithmic growth phase. Error bars represent standard deviation. The t-test was used to evaluate the differences between each MPs treatment with experimental control; asterisks indicate a significant difference at p < 0.01 (**) and p < 0.001 (***).
This study elucidates the intricate dynamics of microplastic (MP) exposure and its impact on the viability of mackerel cells, revealing a potentially non-linear dose-response relationship. Methodologically, the cells underwent exposure to MPs at concentrations of 1, 10, and 50 μg/mL. Remarkably, the most pronounced decrease in cell viability was observed at the intermediate concentration (10 μg/mL), challenging the conventional dose-response paradigm that posits increased toxicity with higher concentrations. This was evidenced by the cell viability trends where, for Figure 1A, the order was control >1 μg/mL > 50 μg/mL > 10 μg/mL, and for Figure 1B, control >50 μg/mL > 1 μg/mL > 10 μg/mL. These findings contrast with several prior studies where a direct dose-dependent toxicity of MPs was reported. For instance, Palaniappan et al. (2022) performed an investigation involving L929 murine fibroblasts and MDCK epithelial cell lines and noted a dose-dependent decrease in cell viability when exposed to 1, 10, or 20 μg/mL of PE or PS microspheres. Furthermore, their study highlighted amplified oxidative stress at higher MP doses, as evidenced by increased SOD3 gene expression. In another study (Lee et al., 2021), human umbilical vein endothelial cells (HUVECs) were exposed to polystyrene microplastics (PS-MPs, 0–100 μg/mL), revealing that higher doses markedly reduced cell viability and disrupted angiogenic tube formation in the short term, while inducing autophagic and necrotic cell death after prolonged exposure. Besides, in a distinct investigation focusing on the human intestinal milieu (Herrala et al., 2023), researchers assessed the toxicological ramifications of ultra-high molecular-weight polyethylene particles (250–1,000 μg/mL) on human colorectal adenocarcinoma Caco-2 and HT-29 cells. A 48-h exposure to these polyethylene particles precipitated a dose-dependent decline in cell viability and a concomitant upsurge in oxidative stress. The oxidative damage was particularly pronounced in the mitochondria, illuminating the broader health concerns.
The intricate interplay between MP concentration and its potential cytotoxic effects is evident. While a substantial proportion of literature supports a dose-dependent decrease in cell viability, certain exceptions persist. In a recent in vitro study examining the impact of microplastics (PVC and PE) on gilthead seabream and European sea bass head-kidney leucocytes (HKLs) (Espinosa et al., 2018), it was observed that exposure to varying concentrations of microplastics for 1 and 24 h did not significantly affect HKL cell viability. Additionally, high doses of microplastics resulted in minimal changes to key cellular innate immune functions, including a decrease in phagocytosis and an elevation in respiratory burst activity. These divergent findings underline the significance of further investigations, accounting for microplastic type, size, and the specific cellular environment, to draw conclusive inferences on the broader impacts of MPs on cellular health. Our findings contribute to this evolving narrative, suggesting that the interaction between MPs and biological systems may be more complex than previously understood.
In addition to concentration, we observed that the size and aggregation state of MPs played a pivotal role in mediating their interaction with mackerel cells. Specifically, at a concentration of 10 μg/mL, MPs were prone to adhere to the cell surface, either individually or as small aggregates, leading to the most significant reduction in cell viability. Conversely, the 50 μg/mL concentration resulted in larger MP aggregates that remained suspended in the culture medium, limiting their contact with cells, as evidenced in Figure 2. This lack of cellular interaction, particularly in scenarios where MPs were introduced post-cell attachment, corresponds with the minimal impact on cell viability observed at this higher concentration (Figure 1B cell count trend: control >50 μg/mL >1 μg/mL >10 μg/mL). Therefore, the pronounced effects at 10 μg/mL highlight the potential for specific MP sizes that maintain close cellular contact to disrupt cell viability. Reflecting on the role of microplastic size and aggregation in cell viability, our results align with the emerging research that examines the complex effects of microplastics on cellular health. A systematic review assessed dose–response relationships regarding microplastics and cell viability by evaluating studies up to March 2021 (Danopoulos et al., 2022). Of the 17 studies reviewed, 8 were included in a meta-regression analysis. The review identified four MP-associated effects: cytotoxicity, immune response, oxidative stress, and barrier attributes, with genotoxicity showing no effect. Key predictors of cell death were irregular MP shape, exposure duration, and MP concentration. Notably, Caco-2 cells displayed heightened susceptibility to MPs. Concentrations as low as 10 μg/mL (5–200 µm) affected cell viability, while 20 μg/mL (0.4 µm) influenced cytokine release. These findings are in line with our observations, specifically the significant cell viability reduction at 10 μg/mL, suggesting that not only the concentration but also the physical form of MPs modulates their cytotoxicity.
FIGURE 2. Morphological analysis of mackerel cells with and without MP treatments: (A) Low-magnification (4X objective) images showcasing the morphology of mackerel cells after 4 days of incubation with varying concentrations of MPs. Red arrows highlight observable MP or MP aggregates. Scale bar represents 500 µm. (B) High-magnification (10X objective) images providing detailed views of cell morphology for the 10 µg/mL and 50 µg/mL MP treatments, corresponding to the samples depicted in Figure 2A. Scale bar represents 200 µm.
In a detailed investigation by Zhang et al. (2022), the impacts of polystyrene microspheres (PS-MPs) and nanospheres (PS-NPs) were explored across four distinct sizes: 0.1, 0.5, 1, and 5 μm. This research identified a marked preference for cellular uptake among the smaller nanoparticles compared to their larger counterparts. Notably, the PS-MPs presented minimal effects on cell viability and apoptosis. However, subtle indications of oxidative stress were discernible in high-concentration groups. A striking differentiation was evident in membrane damage, with PS-MPs inducing substantially greater damage than PS-NPs, emphasizing the size-dependent cellular responses to MPs. Building on the effects of microplastic size on cells, an investigation into polystyrene (PS) particle toxicity further elucidated these intricate relationships (Hwang et al., 2020). Researchers found that PS particles act as potential immune stimulants, triggering cytokine and chemokine production in a manner determined by size and concentration. Larger PS particles (10–100 µm in diameter) exhibited negligible cytotoxicity. In contrast, smaller particles, specifically those at 460 nm and 1 μm, detrimentally affected red blood cells. Their increased surface area, which facilitates stronger interactions like van der Waals forces, was pinpointed as the cause for hemolysis. Furthermore, exposure to these smaller PS particles resulted in elevated IL-6 secretion, signifying potential early-stage inflammation. However, the study also highlighted no substantial rise in histamine secretion, mitigating concerns of histamine-driven inflammation or allergic reactions. Particle uptake predominantly occurred through endocytosis and phagocytosis by phagocytic cells, leading to localized inflammation via pro-inflammatory cytokine release, rather than inducing direct cytotoxicity (Hwang et al., 2020). In addition to the examples described, other studies have consistently indicated that the size of microplastic particles significantly influences cellular interactions. Notably, smaller particles are linked to heightened cellular uptake, more pronounced inflammatory responses, increased apoptosis rates, and enhanced cellular stress responses (Wright and Kelly, 2017; Revel et al., 2018; Yong et al., 2020). These findings highlight the potential health risks associated with finer microplastic particles.
The interaction between cells and microplastics is a multifaceted process, influenced by a confluence of factors, such as the physicochemical properties of microplastics, cellular characteristics, toxicity of the monomers, additives and oligomers in particles and prevailing environmental conditions (Revel et al., 2018; Smith et al., 2018; Campanale et al., 2020; Leslie et al., 2022). This dynamic interplay gains significance when contemplating the potential ramifications of microplastics on cellular health (Lee et al., 2023; Li et al., 2023). While this study predominantly illustrated the influence of microplastic size and dose on cell viability, it is vital to embed these insights within the expansive framework of factors modulating microplastic-cell interactions. The polymer composition (e.g., polymer type, presence of additives, and potential for the microplastics to absorb other environmental contaminants) of microplastics, for instance, is often associated with discrete cytotoxic effects (Duis and Coors, 2016; Revel et al., 2018; Hwang et al., 2020). The shape of microplastics further refines this interaction spectrum. Fibrous microplastics have been reported to potentially introduce physical harm, further affect tissues or induce blockages (Wright et al., 2013; Watts et al., 2015; Diamantidou et al., 2022; Leslie et al., 2022; Tsochatzis et al., 2023), whereas spherical microbeads, prevalent in personal care products, could facilitate a smoother cellular internalization (Wright et al., 2013). Additive blooming in polymer materials such as plasticizers, lubricants, stabilizers, antoioxidants, which are added by the manufacturer to improve the properties of the polymers, may immigrate to the surface of the polymers due to the phase separation (Nouman et al., 2017). Generally, these additives are unsubstituted amides derived from long-chain fatty acids. Reports have indicated that the cytotoxicity associated with these additives can arise from the leaching of bloomed materials in a soluble form or from direct contact between the bloomed substances and the cells (Kim et al., 2003).
Nanoscale microplastics can lead to the creation of reactive oxygen species (ROS), suggesting that they may cause stress to cells by promoting oxidative reactions (Campanale et al., 2020; Paul et al., 2020; Yee et al., 2021). Concurrent inflammatory responses may destabilize cellular homeostasis, possibly marking the onset of apoptosis (Elmore, 2007; Wright et al., 2013; Lamichhane et al., 2023). There could also be direct physical effects, such as potential damage or blockages in tissues, especially in organisms with multiple cell types (Bhagat et al., 2021; Yee et al., 2021). Certain cellular stress signals, specifically p-JNK and p-p38, emphasize that microplastics can be regarded as stress-causing agents (Jeong et al., 2016; Jeong et al., 2017; Scopetani et al., 2020). It's also worth noting that different organisms and cell types can react differently to these plastics (Jeong and Choi, 2019; Bhagat et al., 2021). Comprehending these multifaceted interactions is crucial for elucidating the effects of microplastics on cellular viability and for devising strategies to mitigate their potential adverse impacts.
Cell differentiation is paramount in the cultivated meat production process, serving as an indispensable determinant of the final product’s organoleptic and nutritional characteristics. This involves guiding pluripotent or multipotent cells, predominantly stem cells, through specific differentiation pathways to yield the requisite specialized cell types constituting meat, such as myocytes, adipocytes, and fibroblasts (Allan et al., 2019; Zakrzewski et al., 2019; Reiss et al., 2021). The meticulous orchestration of myocyte differentiation is pivotal for the formation of myofibrils, conferring the unique texture and mouthfeel characteristic of meat (Listrat et al., 2016; Lee et al., 2021). In parallel, the directed differentiation of adipocytes is crucial for the deposition of intramuscular fat, a critical determinant of flavor profile and marbling (Li et al., 2020). Additionally, fibroblast differentiation and subsequent connective tissue formation offer essential structural integrity and have implications for meat tenderness (Purslow, 2020). Therefore, an in-depth understanding and precise control over these differentiation processes are indispensable for the optimization and scalability of cultivated meat, ensuring both its commercial viability and alignment with consumer expectations (Reiss et al., 2021; Bomkamp et al., 2023).
In this study, we assessed the impact of microplastics on muscle cell differentiation by measuring the expression levels of three key myogenic markers: MYOD1, MYOG, and TNNT3A. MYOD1 is characteristic of myogenic progenitors (myoblasts) during the early phases of muscle cell differentiation, MYOG is expressed at the myocyte stage, and TNNT3A acts as a late marker associated with skeletal muscle function. Figure 3A illustrates the absolute gene expression values for MYOD1, MYOG, and TNNT3A of mackerel cells cultivated in varying concentrations of microplastics (0, 1, 10, and 50 μg/mL). Remarkably, a heightened expression of MYOG compared to MYOD1 was observed, denoting that the cells were in a well-differentiated myocyte state. However, the absence of TNNT3A expression implies that full maturation into functioning muscle fibers had not occurred. Figures 3B,C depict the fold-change expression of MYOD1 and MYOG genes, represented as 2^(-ΔΔCt), over the control treatment with no microplastics. HPRT served as the housekeeping gene for expression normalization. Notably, a general lack of statistical difference was observed between the treatments in gene expression under varied microplastic concentrations, as calculated by one-way ANOVA. The exception was a single noteworthy difference in MYOD1 expression between 10 μg/mL and 50 μg/mL concentrations; however, this observation was tenuously supported with a p-value bordering on 0.05, indicating a weak statistical significance.
FIGURE 3. Expression of muscle differentiation gene markers in mackerel cell growing with/without MPs: (A) Absolute gene expression values for MYOD1, MYOG and TNNT3A of MACK cells. Gene expression is represented as 2^(-ΔCt). (B and C) Fold-change expression of MYOD1 and MYOG genes over the control treatment. Points indicate single data points for each biological replicate and bars indicate their mean value (n = 3 experimental, n = 3 technical). HPRT was used as the housekeeping gene for expression normalization. Statistical significance calculated by one-way ANOVA is indicated by asterisks, in which p < 0.05 (*).
The observed increase in MYOG expression, coupled with the non-expression of TNNT3A, indicated a state of well-differentiated cells that had not yet reached the later stages of muscle development. This observation could be attributed to the potential onset of cellular senescence or reduced cell viability, especially considering that the mackerel cell line utilized was at passage #82, a stage at which cells often exhibit altered differentiation patterns due to accumulated genetic and epigenetic changes (Di Micco et al., 2021). Despite the variations in microplastic concentrations, the overall gene expression exhibited minimal statistically significant differences, emphasizing the need for further exploration into the interactions between microplastics and cell differentiation processes.
Complementary to our qPCR findings, immunocytochemical assays provided a visual confirmation of cell differentiation processes. Cells subjected to various microplastic concentrations (0, 1, 10, 50 μg/mL) over 14 days and were subsequently probed with MF20, DAPI, and Phalloidin, targeting myosin, cellular nuclei, and actin filaments, respectively. As depicted in Figure 4, each experimental condition displayed characteristic staining patterns across the trio of molecular markers. Notably, elongated structures positive for myosin, specifically stained by MF20, were evident in all conditions. This consistent staining pattern underscores mackerel cell differentiation into muscle cells. Importantly, the similarities observed between MP-exposed groups and the control suggest that the tested MP concentrations had a negligible impact on mackerel cell differentiation.
FIGURE 4. Representative immunostaining images of mackerel cells with and without MP exposure. DAPI (blue) labels nuclei, Phalloidin (green) highlights actin filaments, and MF20 (red) detects muscle fiber differentiation. Captured using a 20X lens; scale bar = 100 µm.
The observed minimal variation in gene expression across different MP concentrations suggests that muscle cell differentiation may possess an inherent resilience to microplastic-induced stress. Such resilience has been documented in various cellular systems that maintain homeostasis and continue differentiation despite environmental perturbations (Gugliuzza and Crist, 2022). Alternatively, the consistent gene expression might imply a threshold effect, where only microplastic concentrations or particle sizes above/below a specific level are disruptive enough to perturb cellular processes (Campanale et al., 2020). For instance, literature suggests that cellular stress responses can be size-dependent when it comes to nanoparticle interaction, which will be elaborated upon in the subsequent section.
While our findings demonstrated cell differentiation, the effects of microplastics on this process remained indiscernible; all treatment groups and controls yielded analogous outcomes. In contrast, earlier research has highlighted distinct impacts of microplastics on cell differentiation. For instance, Najahi et al. (2022) examined the influence of polyethylene terephthalate microplastics (MPs-PET, <1 μm and <2.6 μm) on human mesenchymal stromal cells, revealing a 30% reduction in cell proliferation and alterations in the differentiation potential of adipose and bone marrow cells. Concurrently, Han et al. (2020) found that polyvinyl chloride (PVC) and acrylonitrile butadiene styrene (ABS) microplastics influenced non-adhesive peripheral blood mononuclear cells (PBMCs) to differentiate into dendritic cells, implying that such plastic exposures might instigate human immune responses. In another study, Hua et al. (2022) highlighted that PS microplastics could disrupt cortical layer differentiation in cerebral spheroids, emphasizing the potential neurotoxic implications. Similarly, Im et al. (2022) reported that polystyrene nanoparticles, especially those with decreased crosslinking density, influenced reactive oxygen species activity and notably promoted adipogenic differentiation in mesenchymal stem cells. Moreover, it was reported that the stage of cell differentiation could influence the cell’s interaction with microplastics, especially regarding the uptake of these particles (Peng et al., 2023). In that study, researchers demonstrated that 2-μm polystyrene (PS) microplastics affected human cell lines differently based on their differentiation state, with undifferentiated Caco-2 cells showing significant PS uptake, whereas differentiated cells presented a reduced capacity for PS internalization. Collectively, these findings underscore the multifaceted impacts of micro/nanoplastics on cell differentiation across different cell types, emphasizing the need for comprehensive understanding and vigilant monitoring. Additionally, variables including the specific plastic type, exposure duration, and plastic concentration remain crucial determinants that could further modulate these effects (Smith et al., 2018).
To unravel the complex dynamics between microplastics and muscle cell differentiation, it is crucial for future research to investigate the molecular interactions that support this process. Investigations should extend beyond traditional myogenic markers to include an array of molecular targets potentially influenced by microplastic exposure. A comprehensive approach that encompasses the study of cellular senescence, epigenetic modifications, and a wide spectrum of differentiation-related biomarkers will be instrumental in advancing our understanding of the implications of microplastic exposure. It is imperative that future studies incorporate these aspects to elucidate the cellular and molecular mechanisms affected by microplastics, which will ultimately refine our understanding of their impact on cell fate decisions and tissue development.
In conclusion, this study evaluated the impact of microplastics on Atlantic mackerel (S. scombrus) skeletal muscle cell lines, using fluorescent polyethylene microspheres (10–45 µm) as model microplastics. The focus was primarily on understanding the effects of microplastic exposure on essential cellular processes, namely, cell viability during attachment and growth phases and cell differentiation, which hold paramount significance in cultivated meat production. Utilizing microplastic concentrations of 1 μg/mL, 10 μg/mL, and 50 μg/mL, alongside a control devoid of microplastics, the study adopted the Trypan Blue Assay for cell viability assessment. The findings highlighted a marked difference in cell viability among microplastic-exposed treatments compared to the control. In parallel, cell differentiation was investigated using RT-qPCR for gene expression analysis and immunostaining methodologies. Notwithstanding the observable cell differentiation, the study discerned no pronounced influence of microplastics on cell differentiation.
The findings of this study elucidate the interplay between microplastics and cellular mechanisms, highlighting the potential ramifications for cellular processes. As preliminary data, this investigation lays a foundation for subsequent, more detailed studies. Given the pervasive presence of microplastics in contemporary environments, it is imperative to explore their broader effects on cellular systems. Recognizing and understanding these implications is not only vital for advancing biotechnological applications but also for discerning potential long-term impacts on broader ecological and human health contexts.
The original contributions presented in the study are included in the article/Supplementary materials, further inquiries can be directed to the corresponding author.
Ethical approval was not required for the studies on animals in accordance with the local legislation and institutional requirements because only commercially available established cell lines were used.
TS: Data curation, Investigation, Methodology, Project administration, Writing–original draft. AT: Investigation, Methodology, Writing–original draft, Formal Analysis, Software. AB: Investigation, Methodology, Writing–original draft. RO: Investigation, Methodology, Conceptualization, Data curation, Formal Analysis, Funding acquisition, Project administration, Resources, Supervision, Validation, Writing–review and editing.
The author(s) declare financial support was received for the research, authorship, and/or publication of this article. This research was financially supported by the Agriculture and Food Research Initiative (AFRI) Sustainable Agricultural Systems program, Grant No. 2021-699012-35978 from the USDA National Institute of Food and Agriculture and Texas A&M AgriLife Research.
The authors would like to gratefully acknowledge Professor David Kaplan and Michael Saad for providing the cell line for this research.
The authors declare that the research was conducted in the absence of any commercial or financial relationships that could be construed as a potential conflict of interest.
All claims expressed in this article are solely those of the authors and do not necessarily represent those of their affiliated organizations, or those of the publisher, the editors and the reviewers. Any product that may be evaluated in this article, or claim that may be made by its manufacturer, is not guaranteed or endorsed by the publisher.
Abbasi, S., Moore, F., and Keshavarzi, B. (2021). PET-microplastics as a vector for polycyclic aromatic hydrocarbons in a simulated plant rhizosphere zone. Environ. Technol. Innovation 21, 101370. doi:10.1016/j.eti.2021.101370
Adhikari, S., Kelkar, V., Kumar, R., and Halden, R. U. (2022). Methods and challenges in the detection of microplastics and nanoplastics: a mini-review. Polym. Int. 71 (5), 543–551. doi:10.1002/pi.6348
Allan, S. J., De Bank, P. A., and Ellis, M. J. (2019). Bioprocess design considerations for cultured meat production with a focus on the expansion bioreactor. Front. Sustain. Food Syst. 3. doi:10.3389/fsufs.2019.00044
Alqahtani, S., Alqahtani, S., Saquib, Q., and Mohiddin, F. (2023). Toxicological impact of microplastics and nanoplastics on humans: understanding the mechanistic aspect of the interaction. Front. Toxicol. 5, 1193386. doi:10.3389/ftox.2023.1193386
Amelia, T. S. M., Khalik, W. M. A. W. M., Ong, M. C., Shao, Y. T., Pan, H.-J., and Bhubalan, K. (2021). Marine microplastics as vectors of major ocean pollutants and its hazards to the marine ecosystem and humans. Prog. Earth Planet. Sci. 8 (1), 12. doi:10.1186/s40645-020-00405-4
Andrady, A. L. (2011). Microplastics in the marine environment. Mar. Pollut. Bull. 62 (8), 1596–1605. doi:10.1016/j.marpolbul.2011.05.030
Bhagat, J., Nishimura, N., and Shimada, Y. (2021). Toxicological interactions of microplastics/nanoplastics and environmental contaminants: current knowledge and future perspectives. J. Hazard. Mater. 405, 123913. doi:10.1016/j.jhazmat.2020.123913
Bhuyan, M. S. (2022). Effects of microplastics on fish and in human health. Front. Environ. Sci. 10. doi:10.3389/fenvs.2022.827289
Bodiou, V., Moutsatsou, P., and Post, M. J. (2020). Microcarriers for upscaling cultured meat production. Front. Nutr. 7, 10. doi:10.3389/fnut.2020.00010
Bomkamp, C., Musgrove, L., Marques, D. M. C., Fernando, G. F., Ferreira, F. C., and Specht, E. A. (2023). Differentiation and maturation of muscle and fat cells in cultivated seafood: lessons from developmental biology. Mar. Biotechnol. 25 (1), 1–29. doi:10.1007/s10126-022-10174-4
Campanale, C., Massarelli, C., Savino, I., Locaputo, V., and Uricchio, V. F. (2020). A detailed review study on potential effects of microplastics and additives of concern on human health. Int. J. Environ. Res. Public Health 17 (4), 1212. doi:10.3390/ijerph17041212
Cao, J., Xu, R., Wang, F., Geng, Y., Xu, T., Zhu, M., et al. (2023). Polyethylene microplastics trigger cell apoptosis and inflammation via inducing oxidative stress and activation of the NLRP3 inflammasome in carp gills. Fish Shellfish Immunol. 132, 108470. doi:10.1016/j.fsi.2022.108470
Chriki, S., and Hocquette, J. F. (2020). The myth of cultured meat: a Review. Front. Nutr. 7, 7. doi:10.3389/fnut.2020.00007
Cole, M., Lindeque, P., Halsband, C., and Galloway, T. S. (2011). Microplastics as contaminants in the marine environment: a review. Mar. Pollut. Bull. 62 (12), 2588–2597. doi:10.1016/j.marpolbul.2011.09.025
Courtene-Jones, W., Clark, N. J., Fischer, A. C., Smith, N. S., and Thompson, R. C. (2022). Ingestion of microplastics by marine animals. Plastics Ocean, 349–366. doi:10.1002/9781119768432.ch12
Dai, S., Ye, R., Huang, J., Wang, B., Xie, Z., Ou, X., et al. (2022). Distinct lipid membrane interaction and uptake of differentially charged nanoplastics in bacteria. J. Nanobiotechnology 20 (1), 191. doi:10.1186/s12951-022-01321-z
Danopoulos, E., Twiddy, M., West, R., and Rotchell, J. M. (2022). A rapid review and meta-regression analyses of the toxicological impacts of microplastic exposure in human cells. J. Hazard. Mater. 427, 127861. doi:10.1016/j.jhazmat.2021.127861
Diamantidou, D., Mastrogianni, O., Tsochatzis, E., Theodoridis, G., Raikos, N., Gika, H., et al. (2022). Liquid chromatography-mass spectrometry method for the determination of polyethylene terephthalate and polybutylene terephthalate cyclic oligomers in blood samples. Anal. Bioanal. Chem. 414 (4), 1503–1512. doi:10.1007/s00216-021-03741-6
Di Micco, R., Krizhanovsky, V., Baker, D., and d'Adda di Fagagna, F. (2021). Cellular senescence in ageing: from mechanisms to therapeutic opportunities. Nat. Rev. Mol. Cell Biol. 22 (2), 75–95. doi:10.1038/s41580-020-00314-w
Duis, K., and Coors, A. (2016). Microplastics in the aquatic and terrestrial environment: sources (with a specific focus on personal care products), fate and effects. Environ. Sci. Eur. 28 (1), 2. doi:10.1186/s12302-015-0069-y
Dupuis, J. H., Cheung, L. K. Y., Newman, L., Dee, D. R., and Yada, R. Y. (2023). Precision cellular agriculture: the future role of recombinantly expressed protein as food. Compr. Rev. Food Sci. Food Saf. 22 (2), 882–912. doi:10.1111/1541-4337.13094
EFSA Panel on Contaminants in the Food Chain (CONTAM) (2016). Presence of microplastics and nanoplastics in food, with particular focus on seafood. EFSA J. 14 (6), e04501. doi:10.2903/j.efsa.2016.4501
Eibl, R., Senn, Y., Gubser, G., Jossen, V., van den Bos, C., and Eibl, D. (2021). Cellular agriculture: opportunities and challenges. Annu. Rev. Food Sci. Technol. 12, 51–73. doi:10.1146/annurev-food-063020-123940
Elmore, S. (2007). Apoptosis: a review of programmed cell death. Toxicol. Pathol. 35 (4), 495–516. doi:10.1080/01926230701320337
Espinosa, C., García Beltrán, J. M., Esteban, M. A., and Cuesta, A. (2018). In vitro effects of virgin microplastics on fish head-kidney leucocyte activities. Environ. Pollut. 235, 30–38. doi:10.1016/j.envpol.2017.12.054
Fleury, J.-B., and Baulin, V. A. (2021). Microplastics destabilize lipid membranes by mechanical stretching. Proc. Natl. Acad. Sci. 118 (31), e2104610118. doi:10.1073/pnas.2104610118
Fournier, E., Leveque, M., Ruiz, P., Ratel, J., Durif, C., Chalancon, S., et al. (2023). Microplastics: what happens in the human digestive tract? First evidences in adults using in vitro gut models. J. Hazard. Mater. 442, 130010. doi:10.1016/j.jhazmat.2022.130010
Galloway, T. S., Cole, M., and Lewis, C. (2017). Interactions of microplastic debris throughout the marine ecosystem. Nat. Ecol. Evol. 1 (5), 0116. doi:10.1038/s41559-017-0116
Galluzzi, L., Vitale, I., Aaronson, S. A., Abrams, J. M., Adam, D., Agostinis, P., et al. (2018). Molecular mechanisms of cell death: recommendations of the nomenclature committee on cell death 2018. Cell Death Differ. 25 (3), 486–541. doi:10.1038/s41418-017-0012-4
Goodman, K. E., Hare, J. T., Khamis, Z. I., Hua, T., and Sang, Q.-X. A. (2021). Exposure of human lung cells to polystyrene microplastics significantly retards cell proliferation and triggers morphological changes. Chem. Res. Toxicol. 34 (4), 1069–1081. doi:10.1021/acs.chemrestox.0c00486
Gugliuzza, M. V., and Crist, C. (2022). Muscle stem cell adaptations to cellular and environmental stress. Skelet. Muscle 12 (1), 5. doi:10.1186/s13395-022-00289-6
Han, S., Bang, J., Choi, D., Hwang, J., Kim, T., Oh, Y., et al. (2020). Surface pattern analysis of microplastics and their impact on human-derived cells. ACS Appl. Polym. Mater. 2 (11), 4541–4550. doi:10.1021/acsapm.0c00645
Herrala, M., Huovinen, M., Järvelä, E., Hellman, J., Tolonen, P., Lahtela-Kakkonen, M., et al. (2023). Micro-sized polyethylene particles affect cell viability and oxidative stress responses in human colorectal adenocarcinoma Caco-2 and HT-29 cells. Sci. Total Environ. 867, 161512. doi:10.1016/j.scitotenv.2023.161512
Hirt, N., and Body-Malapel, M. (2020). Immunotoxicity and intestinal effects of nano- and microplastics: a review of the literature. Part Fibre Toxicol. 17 (1), 57. doi:10.1186/s12989-020-00387-7
Hua, T., Kiran, S., Li, Y., and Sang, Q.-X. A. (2022). Microplastics exposure affects neural development of human pluripotent stem cell-derived cortical spheroids. J. Hazard. Mater. 435, 128884. doi:10.1016/j.jhazmat.2022.128884
Huppertsberg, S., and Knepper, T. P. (2018). Instrumental analysis of microplastics—benefits and challenges. Anal. Bioanal. Chem. 410 (25), 6343–6352. doi:10.1007/s00216-018-1210-8
Hwang, J., Choi, D., Han, S., Jung, S. Y., Choi, J., and Hong, J. (2020). Potential toxicity of polystyrene microplastic particles. Sci. Rep. 10 (1), 7391. doi:10.1038/s41598-020-64464-9
Im, G.-B., Kim, Y. G., Jo, I.-S., Yoo, T. Y., Kim, S.-W., Park, H. S., et al. (2022). Effect of polystyrene nanoplastics and their degraded forms on stem cell fate. J. Hazard. Mater. 430, 128411. doi:10.1016/j.jhazmat.2022.128411
Ivar do Sul, J. A., and Costa, M. F. (2014). The present and future of microplastic pollution in the marine environment. Environ. Pollut. 185, 352–364. doi:10.1016/j.envpol.2013.10.036
Jahir, N. R., Ramakrishna, S., Abdullah, A. A. A., and Vigneswari, S. (2023). Cultured meat in cellular agriculture: advantages, applications and challenges. Food Biosci. 53, 102614. doi:10.1016/j.fbio.2023.102614
Jeong, C.-B., Kang, H.-M., Lee, M.-C., Kim, D.-H., Han, J., Hwang, D.-S., et al. (2017). Adverse effects of microplastics and oxidative stress-induced MAPK/Nrf2 pathway-mediated defense mechanisms in the marine copepod Paracyclopina nana. Sci. Rep. 7 (1), 41323. doi:10.1038/srep41323
Jeong, C.-B., Won, E.-J., Kang, H.-M., Lee, M.-C., Hwang, D.-S., Hwang, U.-K., et al. (2016). Microplastic size-dependent toxicity, oxidative stress induction, and p-JNK and p-p38 activation in the monogonont rotifer (brachionus koreanus). Environ. Sci. Technol. 50 (16), 8849–8857. doi:10.1021/acs.est.6b01441
Jeong, J., and Choi, J. (2019). Adverse outcome pathways potentially related to hazard identification of microplastics based on toxicity mechanisms. Chemosphere 231, 249–255. doi:10.1016/j.chemosphere.2019.05.003
Jeyavani, J., Sibiya, A., Stalin, T., Vigneshkumar, G., Al-Ghanim, K. A., Riaz, M. N., et al. (2023). Biochemical, genotoxic and histological implications of polypropylene microplastics on freshwater fish oreochromis mossambicus: an aquatic eco-toxicological assessment. Toxics 11 (3), 282. doi:10.3390/toxics11030282
Jiang, B., Kauffman, A. E., Li, L., McFee, W., Cai, B., Weinstein, J., et al. (2020). Health impacts of environmental contamination of micro- and nanoplastics: a review. Environ. Health Prev. Med. 25 (1), 29. doi:10.1186/s12199-020-00870-9
Kannan, K., and Vimalkumar, K. (2021). A review of human exposure to microplastics and insights into microplastics as obesogens. Front. Endocrinol. 12, 724989. doi:10.3389/fendo.2021.724989
Karla Lizzeth, M.-C., Leandro, H.-C., Carlos, M. A.-A., Luis Alberto Peralta, P., and Carolina, P.-M. (2023). “Micro(Nano) Plastics as carriers of toxic agents and their impact on human health,” in Advances and challenges in microplastics. Editor S. El-Sayed (London, UK: IntechOpen). Ch. 10). doi:10.5772/intechopen.111889
Kim, S., Beier, A., Schreyer, H. B., and Bakshi, B. R. (2022). Environmental life cycle assessment of a novel cultivated meat burger patty in the United States. Sustainability 14 (23), 16133. doi:10.3390/su142316133
Kim, Y. H., Han, D. K., Park, K. D., and Kim, S. H. (2003). Enhanced blood compatibility of polymers grafted by sulfonated PEO via a negative cilia concept. Focus Biomaterials Sci. Asia 24, 2213–2223. doi:10.1016/S0142-9612(03)00023-1
Koelmans, A. A., Mohamed Nor, N. H., Hermsen, E., Kooi, M., Mintenig, S. M., and De France, J. (2019). Microplastics in freshwaters and drinking water: critical review and assessment of data quality. Water Res. 155, 410–422. doi:10.1016/j.watres.2019.02.054
Lamichhane, G., Acharya, A., Marahatha, R., Modi, B., Paudel, R., Adhikari, A., et al. (2023). Microplastics in environment: global concern, challenges, and controlling measures. Int. J. Environ. Sci. Technol. 20 (4), 4673–4694. doi:10.1007/s13762-022-04261-1
Lee, D. Y., Lee, S. Y., Jung, J. W., Kim, J. H., Oh, D. H., Kim, H. W., et al. (2022). Review of technology and materials for the development of cultured meat. Crit. Rev. Food Sci. Nutr. 63, 8591–8615. doi:10.1080/10408398.2022.2063249
Lee, H.-S., Amarakoon, D., Wei, C.-i., Choi, K. Y., Smolensky, D., and Lee, S.-H. (2021). Adverse effect of polystyrene microplastics (PS-MPs) on tube formation and viability of human umbilical vein endothelial cells. Food Chem. Toxicol. 154, 112356. doi:10.1016/j.fct.2021.112356
Lee, K. Y., Loh, H. X., and Wan, A. C. A. (2021). Systems for muscle cell differentiation: from bioengineering to future food. Micromachines (Basel) 13 (1), 71. doi:10.3390/mi13010071
Lee, Y., Cho, J., Sohn, J., and Kim, C. (2023). Health effects of microplastic exposures: current issues and perspectives in South Korea. Yonsei Med. J. 64 (5), 301–308. doi:10.3349/ymj.2023.0048
Leslie, H. A., Van Velzen, M. J., Brandsma, S. H., Vethaak, A. D., Garcia-Vallejo, J. J., and Lamoree, M. H. (2022). Discovery and quantification of plastic particle pollution in human blood. Environ. Int. 163, 107199. doi:10.1016/j.envint.2022.107199
Li, X., Fu, X., Yang, G., and Du, M. (2020). Review: enhancing intramuscular fat development via targeting fibro-adipogenic progenitor cells in meat animals. Animal 14 (2), 312–321. doi:10.1017/S175173111900209X
Li, Y., Tao, L., Wang, Q., Wang, F., Li, G., and Song, M. (2023). Potential health impact of microplastics: a review of environmental distribution, human exposure, and toxic effects. Environ. Health 1, 249–257. doi:10.1021/envhealth.3c00052
Lim, X. (2021). Microplastics are everywhere - but are they harmful? Nature 593 (7857), 22–25. doi:10.1038/d41586-021-01143-3
Listrat, A., Lebret, B., Louveau, I., Astruc, T., Bonnet, M., Lefaucheur, L., et al. (2016). How muscle structure and composition influence meat and flesh quality. ScientificWorldJournal 2016, 3182746. doi:10.1155/2016/3182746
Löder, M. G. J., Imhof, H. K., Ladehoff, M., Löschel, L. A., Lorenz, C., Mintenig, S., et al. (2017). Enzymatic purification of microplastics in environmental samples. Environ. Sci. Technol. 51 (24), 14283–14292. doi:10.1021/acs.est.7b03055
Lv, L., Yan, X., Feng, L., Jiang, S., Lu, Z., Xie, H., et al. (2021). Challenge for the detection of microplastics in the environment. Water Environ. Res. 93 (1), 5–15. doi:10.1002/wer.1281
Lwanga, E. H., Beriot, N., Corradini, F., Silva, V., Yang, X., Baartman, J., et al. (2022). Review of microplastic sources, transport pathways and correlations with other soil stressors: a journey from agricultural sites into the environment. Chem. Biol. Technol. Agric. 9 (1), 20. doi:10.1186/s40538-021-00278-9
Makhdoumi, P., Hossini, H., and Pirsaheb, M. (2023). A review of microplastic pollution in commercial fish for human consumption. Rev. Environ. Health 38 (1), 97–109. doi:10.1515/reveh-2021-0103
Mamun, A. A., Prasetya, T. A. E., Dewi, I. R., and Ahmad, M. (2023). Microplastics in human food chains: food becoming a threat to health safety. Sci. Total Environ. 858, 159834. doi:10.1016/j.scitotenv.2022.159834
Mattioda, V., Benedetti, V., Tessarolo, C., Oberto, F., Favole, A., Gallo, M., et al. (2023). Pro-Inflammatory and cytotoxic effects of polystyrene microplastics on human and murine intestinal cell lines. Biomolecules 13 (1), 140. doi:10.3390/biom13010140
Najahi, H., Alessio, N., Squillaro, T., Conti, G. O., Ferrante, M., Di Bernardo, G., et al. (2022). Environmental microplastics (EMPs) exposure alter the differentiation potential of mesenchymal stromal cells. Environ. Res. 214 (4), 114088. doi:10.1016/j.envres.2022.114088
Nouman, M., Saunier, J., Jubeli, E., and Yagoubi, N. (2017). Additive blooming in polymer materials: consequences in the pharmaceutical and medical field. Polym. Degrad. Stab. 143, 239–252. doi:10.1016/j.polymdegradstab.2017.07.021
O'Neill, E. N., Cosenza, Z. A., Baar, K., and Block, D. E. (2021). Considerations for the development of cost-effective cell culture media for cultivated meat production. Compr. Rev. Food Sci. Food Saf. 20 (1), 686–709. doi:10.1111/1541-4337.12678
O’Neill, S. M., and Lawler, J. (2021). Knowledge gaps on micro and nanoplastics and human health: a critical review. Case Stud. Chem. Environ. Eng. 3, 100091. doi:10.1016/j.cscee.2021.100091
Ong, K. J., Johnston, J., Datar, I., Sewalt, V., Holmes, D., and Shatkin, J. A. (2021). Food safety considerations and research priorities for the cultured meat and seafood industry. Compr. Rev. Food Sci. Food Saf. 20 (6), 5421–5448. doi:10.1111/1541-4337.12853
Osman, A. I., Hosny, M., Eltaweil, A. S., Omar, S., Elgarahy, A. M., Farghali, M., et al. (2023). Microplastic sources, formation, toxicity and remediation: a review. Environ. Chem. Lett. 21, 2129–2169. doi:10.1007/s10311-023-01593-3
Palaniappan, S., Sadacharan, C. M., and Rostama, B. (2022). Polystyrene and polyethylene microplastics decrease cell viability and dysregulate inflammatory and oxidative stress markers of MDCK and L929 cells in vitro. Expo. Health 14 (1), 75–85. doi:10.1007/s12403-021-00419-3
Paul, M. B., Stock, V., Cara-Carmona, J., Lisicki, E., Shopova, S., Fessard, V., et al. (2020). Micro- and nanoplastics - current state of knowledge with the focus on oral uptake and toxicity. Nanoscale Adv. 2 (10), 4350–4367. doi:10.1039/D0NA00539H
Peng, M., Vercauteren, M., Grootaert, C., Rajkovic, A., Boon, N., Janssen, C., et al. (2023). Cellular and bioenergetic effects of polystyrene microplastic in function of cell type, differentiation status and post-exposure time. Environ. Pollut. 337, 122550. doi:10.1016/j.envpol.2023.122550
Purslow, P. P. (2020). The structure and role of intramuscular connective tissue in muscle function. Front. Physiology 11, 495. doi:10.3389/fphys.2020.00495
Ramsperger, A., Bergamaschi, E., Panizzolo, M., Fenoglio, I., Barbero, F., Peters, R., et al. (2023). Nano- and microplastics: a comprehensive review on their exposure routes, translocation, and fate in humans. NanoImpact 29, 100441. doi:10.1016/j.impact.2022.100441
Reiss, J., Robertson, S., and Suzuki, M. (2021). Cell sources for cultivated meat: applications and considerations throughout the production workflow. Int. J. Mol. Sci. 22 (14), 7513. doi:10.3390/ijms22147513
Revel, M., Châtel, A., and Mouneyrac, C. (2018). Micro(nano)plastics: a threat to human health? Curr. Opin. Environ. Sci. Health 1, 17–23. doi:10.1016/j.coesh.2017.10.003
Rischer, H., Szilvay, G. R., and Oksman-Caldentey, K.-M. (2020). Cellular agriculture — industrial biotechnology for food and materials. Curr. Opin. Biotechnol. 61, 128–134. doi:10.1016/j.copbio.2019.12.003
Rubio-Armendáriz, C., Alejandro-Vega, S., Paz-Montelongo, S., Gutiérrez-Fernández Á, J., Carrascosa-Iruzubieta, C. J., and Hardisson-de la Torre, A. (2022). Microplastics as emerging food contaminants: a challenge for food safety. Int. J. Environ. Res. Public Health 19 (3), 1174. doi:10.3390/ijerph19031174
Saad, M. K., Yuen, J. S. K., Joyce, C. M., Li, X., Lim, T., Wolfson, T. L., et al. (2023). Continuous fish muscle cell line with capacity for myogenic and adipogenic-like phenotypes. Sci. Rep. 13 (1), 5098. doi:10.1038/s41598-023-31822-2
Schirinzi, G. F., Pérez-Pomeda, I., Sanchís, J., Rossini, C., Farré, M., and Barceló, D. (2017). Cytotoxic effects of commonly used nanomaterials and microplastics on cerebral and epithelial human cells. Environ. Res. 159, 579–587. doi:10.1016/j.envres.2017.08.043
Schymanski, D., Goldbeck, C., Humpf, H.-U., and Fürst, P. (2018). Analysis of microplastics in water by micro-Raman spectroscopy: release of plastic particles from different packaging into mineral water. Water Res. 129, 154–162. doi:10.1016/j.watres.2017.11.011
Scopetani, C., Esterhuizen, M., Cincinelli, A., and Pflugmacher, S. (2020). Microplastics exposure causes negligible effects on the oxidative response enzymes glutathione reductase and peroxidase in the oligochaete Tubifex tubifex. Toxics 8 (1), 14. doi:10.3390/toxics8010014
Sharma, S., and Chatterjee, S. (2017). Microplastic pollution, a threat to marine ecosystem and human health: a short review. Environ. Sci. Pollut. Res. 24 (27), 21530–21547. doi:10.1007/s11356-017-9910-8
Sinke, P., Swartz, E., Sanctorum, H., van der Giesen, C., and Odegard, I. (2023). Ex-ante life cycle assessment of commercial-scale cultivated meat production in 2030 (vol 28, pg 234, 2023). Int. J. Life Cycle Assess. 28 (9), 1225–1228. doi:10.1007/s11367-023-02183-9
Smith, M., Love, D. C., Rochman, C. M., and Neff, R. A. (2018). Microplastics in seafood and the implications for human health. Curr. Environ. Health Rep. 5 (3), 375–386. doi:10.1007/s40572-018-0206-z
Stephens, N., Di Silvio, L., Dunsford, I., Ellis, M., Glencross, A., and Sexton, A. (2018). Bringing cultured meat to market: technical, socio-political, and regulatory challenges in cellular agriculture. Trends Food Sci. Technol. 78, 155–166. doi:10.1016/j.tifs.2018.04.010
Thiele, C. J., Hudson, M. D., Russell, A. E., Saluveer, M., and Sidaoui-Haddad, G. (2021). Microplastics in fish and fishmeal: an emerging environmental challenge? Sci. Rep. 11 (1), 2045. doi:10.1038/s41598-021-81499-8
Thornton Hampton, L. M., Bouwmeester, H., Brander, S. M., Coffin, S., Cole, M., Hermabessiere, L., et al. (2022). Research recommendations to better understand the potential health impacts of microplastics to humans and aquatic ecosystems. Microplastics Nanoplastics 2 (1), 18. doi:10.1186/s43591-022-00038-y
Tsochatzis, E. D., Theodoridis, G., and Corredig, M. (2023). Analysis of oligomers to assess exposure to microplastics from foods. A perspective. Front. Nutr. 10, 1186951. doi:10.3389/fnut.2023.1186951
Vergeer, R., Sinke, P., and Odegard, I. (2021). TEA of cultivated meat, Future projections of different scenarios - corrigendum. C. Delft. 21.
Vivekanand, A. C., Mohapatra, S., and Tyagi, V. K. (2021). Microplastics in aquatic environment: challenges and perspectives. Chemosphere 282, 131151. doi:10.1016/j.chemosphere.2021.131151
Wang, W., Zhang, J., Qiu, Z., Cui, Z., Li, N., Li, X., et al. (2022). Effects of polyethylene microplastics on cell membranes: a combined study of experiments and molecular dynamics simulations. J. Hazard. Mater. 429, 128323. doi:10.1016/j.jhazmat.2022.128323
Watts, A. J. R., Urbina, M. A., Corr, S., Lewis, C., and Galloway, T. S. (2015). Ingestion of plastic microfibers by the crab carcinus maenas and its effect on food consumption and energy balance. Environ. Sci. Technol. 49 (24), 14597–14604. doi:10.1021/acs.est.5b04026
Wright, S. L., and Kelly, F. J. (2017). Plastic and human health: a micro issue? Environ. Sci. Technol. 51 (12), 6634–6647. doi:10.1021/acs.est.7b00423
Wright, S. L., Rowe, D., Thompson, R. C., and Galloway, T. S. (2013). Microplastic ingestion decreases energy reserves in marine worms. Curr. Biol. 23 (23), R1031–R1033. doi:10.1016/j.cub.2013.10.068
Wu, D., Feng, Y., Wang, R., Jiang, J., Guan, Q., Yang, X., et al. (2023). Pigment microparticles and microplastics found in human thrombi based on Raman spectral evidence. J. Adv. Res. 49, 141–150. doi:10.1016/j.jare.2022.09.004
Yee, M. S., Hii, L. W., Looi, C. K., Lim, W. M., Wong, S. F., Kok, Y. Y., et al. (2021). Impact of microplastics and nanoplastics on human health. Nanomater. (Basel) 11 (2), 496. doi:10.3390/nano11020496
Yong, C. Q. Y., Valiyaveettil, S., and Tang, B. L. (2020). Toxicity of microplastics and nanoplastics in mammalian systems. Int. J. Environ. Res. Public Health 17 (5), 1509. doi:10.3390/ijerph17051509
Zakrzewski, W., Dobrzyński, M., Szymonowicz, M., and Rybak, Z. (2019). Stem cells: past, present, and future. Stem Cell Res. Ther. 10 (1), 68. doi:10.1186/s13287-019-1165-5
Zhang, Y., Wang, S., Olga, V., Xue, Y., Lv, S., Diao, X., et al. (2022). The potential effects of microplastic pollution on human digestive tract cells. Chemosphere 291, 132714. doi:10.1016/j.chemosphere.2021.132714
Keywords: cultivated meat, cell-based seafood, microplastics, cell growth, gene expression
Citation: Sun T, Timoneda A, Banavar A and Ovissipour R (2024) Enhancing food safety and cultivated meat production: exploring the impact of microplastics on fish muscle cell proliferation and differentiation. Front. Food. Sci. Technol. 4:1309884. doi: 10.3389/frfst.2024.1309884
Received: 08 October 2023; Accepted: 19 February 2024;
Published: 28 February 2024.
Edited by:
Noemi Elisabet Zaritzky, National University of La Plata, ArgentinaReviewed by:
Emmanouil Tsochatzis, Aarhus University, DenmarkCopyright © 2024 Sun, Timoneda, Banavar and Ovissipour. This is an open-access article distributed under the terms of the Creative Commons Attribution License (CC BY). The use, distribution or reproduction in other forums is permitted, provided the original author(s) and the copyright owner(s) are credited and that the original publication in this journal is cited, in accordance with accepted academic practice. No use, distribution or reproduction is permitted which does not comply with these terms.
*Correspondence: Reza Ovissipour, b3Zpc3NpQHRhbXUuZWR1
Disclaimer: All claims expressed in this article are solely those of the authors and do not necessarily represent those of their affiliated organizations, or those of the publisher, the editors and the reviewers. Any product that may be evaluated in this article or claim that may be made by its manufacturer is not guaranteed or endorsed by the publisher.
Research integrity at Frontiers
Learn more about the work of our research integrity team to safeguard the quality of each article we publish.