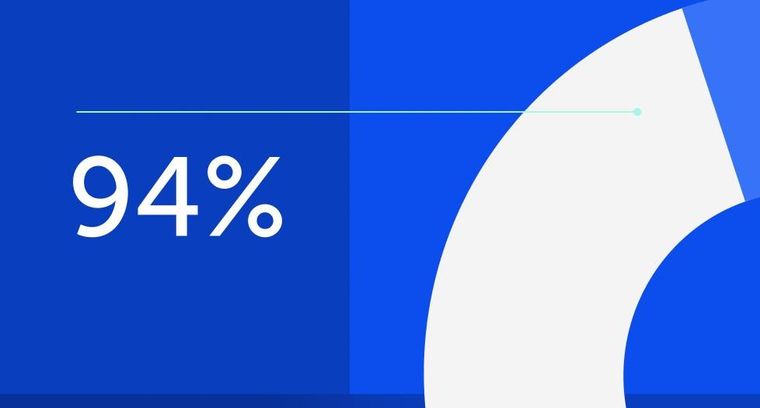
94% of researchers rate our articles as excellent or good
Learn more about the work of our research integrity team to safeguard the quality of each article we publish.
Find out more
MINI REVIEW article
Front. Food. Sci. Technol., 01 September 2023
Sec. Food Biotechnology
Volume 3 - 2023 | https://doi.org/10.3389/frfst.2023.1258087
This article is part of the Research TopicPost-Pandemic Foods: Development, Processing, Acceptance and QualityView all 5 articles
Microbial biomass is a promising supply of essential fatty acids (EFAs) for utilization in human and animal nutrition such as fish. EFAs, including polyunsaturated fatty acids (PUFAs), are needed for cell regulation and disease prevention. Oleaginous microorganisms from species like fungi (Cryptococcus, Cunninghamella, and Mortierella), microalgae (Chlorella zofingiensis and Crypthecodinium cohnii), and bacteria (Moritella sp. and Vibrio sp.) can accumulate lipids exceeding 20% of their biomass. Optimizing factors such as nitrogen and carbon sources, cultivation methods, and environmental conditions may improve their lipid production. Efficient lipid extraction methods through mechanical, non-mechanical or chemical methods are essential to obtain EFAs from microbial biomass. Challenges include substrates (carbon and nitrogen sources) cost and downstream processing and overcoming these challenges can provide a sustainable source of EFAs for human and animal nutrition. By advancing metabolic engineering, cultivation techniques, and extraction methods, microbial lipid production holds the potential to offer cost-effective and environmentally friendly EFAs. The utilization of microbial biomass as a source of EFAs can contribute to a healthier future by addressing the limitations of traditional sources and providing a sustainable solution for meeting the increasing demand for EFAs in human and animal diets.
Essential fatty acids (EFAs) are necessary for human metabolism but cannot be synthesized naturally by the body. Their consumption, especially long-chain polyunsaturated fatty acids (PUFAs), plays a vital role in regulating cell physiology and reducing the risk of various diseases (Connor, 2000). While EFAs can be obtained from sources like green leafy vegetables and vegetable oils, limitations such as caloric values, allergies, and cholesterol content restrict their consumption (Simopoulos, 1999). In the aquaculture industry, EFAs are crucial for the growth and health of fish but their availability is limited.
Microbial biomass has emerged as a promising alternative for the production of EFAs. Oleaginous microorganisms, such as fungi, yeasts, microalgae, and certain bacteria, are capable of accumulating lipids at levels exceeding 20% of their cell mass (Patel et al., 2020).
Several factors influence the lipid content and composition of microbial biomass such as the choice of carbon and nitrogen sources, the carbon-to-nitrogen ratio, and cultivation methods. Optimal growth conditions like temperature, aeration, and pH also need to be carefully controlled to enhance lipid accumulation. By optimizing these factors, microbial lipid accumulation can be enhanced, and lipids with desired characteristics can be produced for various applications.
This review aims to provide concise information about the upstream processing of EFAs production from microorganisms such as fungi, microalgae and bacteria, and the factors influencing their lipid content and composition (Figure 1). The downstream processing to extract and purify the microbial lipid will also be examined. Besides, the challenges and limitations that exist in the production of EFAs from microbial biomass will also be discussed, to aid future production of EFAs from other feedstocks using bioreactor such as fungal species of Lignosus rhinocerus and Ganoderma lucidum.
FIGURE 1. Upstream and downstream processing of essential fatty acids production from microorganisms.
EFAs are unsaturated fatty acids which the human body cannot synthesized naturally but are essential for human metabolism (Mokochinski et al., 2015). The consumption of EFAs, especially long-chain PUFA may aid in the regulation of cell physiology and reduction of blood cholesterol (Parikh et al., 2005). EFAs such as n-3 fatty acids also could prevent diseases such as coronary heart disease, Crohn disease, rheumatoid arthritis, autoimmune disorders, and certain type of cancers such as breast, colon, and prostate cancers (Connor, 2000). The importance of EFAs but inability to produce them naturally resulted in the addition of EFAs into food as daily human diet. Common sources of EFAs include leafy vegetables and oils derived from sunflower seeds, safflower seeds, corn, and soybeans (Simopoulos, 1999). EFAs can also be consumed by eating fishes and meats from animals fed with grains rich in omega-6 fatty acids (Simopoulos, 1999). High consumption of these foods rich in EFAs may be restricted due to high caloric values, allergies, and high cholesterol contents. In addition, people who are vegetarian or vegan will avoid eating animal-based food.
Lipids like EFAs are not only required by human in their diet but also vital for animal growth such as fish in the aquaculture industry. EFAs are necessary in fish diets because they are an essential component of cellular membranes, hormone precursors, and lipid-soluble vitamin absorption (Ng and Romano, 2013). Traditionally, marine fish oil has been used as the only dietary lipid source in fish feed. However, the increase in price and demand of the fish oil coupled with less availability due to climate changes requires alternative source of EFAs for fish feed (Turchini et al., 2009). Fish feed formulation with microbial biomass high in EFAs could become a solution for these concerns.
Microorganisms play a crucial role in lipid production, with certain species capable of accumulating high amounts of lipids as reserve storage materials. These oleaginous microorganisms have become the focus of lipid production research due to their ability to store lipids at levels exceeding 20% of their biomass (Patel et al., 2020). Lipid-producing microorganisms consist of fungi, microalgae, bacteria, and heterotrophic protist like thraustochytrid. Table 1 shows the oleaginous microorganisms from previous literature and their EFAs composition.
Fungi, such as Cryptococcus, Cunninghamella, and Mortierella, are known for their high lipid accumulation capabilities (Castanha et al., 2014; Mirbagheri et al., 2015; Kikukawa et al., 2018). For example, Mortierella alpina is used in industrial processes to produce up to 70% total fatty acid (TFA) of arachidonic acid (ARA), an important fatty acid for dietary supplements (Kikukawa et al., 2018). Other fungal species, including Rhodotorula and Lipomyces, also show potential for industrial applications due to their ability to produce 29.58% TFA linoleic acid and 17.4% TFA DHA, respectively (da Silva et al., 2020; Salunke et al., 2015).
Microalgae are another important group of microorganisms used for lipid production. They have a higher growth rate compared to plants and can accumulate lipids at levels varying from 5% to 70% of their biomass, depending on the species and growth conditions (Jones et al., 2019). Notably, microalgae are capable of utilizing sunlight and carbon dioxide as their energy and carbon sources, respectively. This photoautotrophic system enables year-round production, despite being impacted by parameters such as sunshine intensity, dissolved oxygen content, pH, and nutrient availability (Jones et al., 2019). Species like Chlorella zofingiensis and Crypthecodinium cohnii are commonly employed for their lipid production capabilities, especially for the production of essential PUFAs such as docosahexaenoic acid (DHA), ARA, and eicosapentaenoic acid (EPA) (Karnaouri et al., 2020; Pratiwy and Pratiwi, 2020; Moniz et al., 2021).
Bacteria, although generally not efficient lipid producers, have certain species from the genera Moritella sp. and Vibrio sp. That are known for their ability to accumulate DHA (11.1% TFA) and EPA (10% TFA) (Abd Elrazak et al., 2013; Kautharapu et al., 2013; Moi et al., 2018). Thraustochyrid, an oleaginous heterotrophic marine protist also capable of producing high amount of DHA. For example, Thraustochytrium, Schizochytrium sp., and Aurantiochytrium sp. UMACC-T004 were demonstrated to contain around 29%–55% TFA of DHA (Ou et al., 2016; Guo et al., 2017; Guo et al., 2020; Du et al., 2021; Chi et al., 2022).
The advantages of microbial lipid synthesis include the high growth rates of microorganisms, consistent composition and quality of the produced lipids, and the capacity to utilise a diverse array of substrates, including industrial waste streams (Ochsenreither et al., 2016). Microbial oils do not contain pollutants found in plant and marine oils, which make them a cleaner source of lipids (Béligon et al., 2016).
The lipid content and lipid profile of microbial biomass are influenced by various factors that need to be carefully controlled in order to achieve optimal results. One key factor is the choice of carbon source. The most prevalent carbon source for lipid synthesis is glucose. However, other mono- or disaccharides have also been tested, with xylose, glucose, and fructose showing high cellular lipid contents in studies on Mortierella isabellina (Zeng et al., 2013; Demir and Gündes, 2020). In addition, low-cost carbon sources such as glycerol, commercial sugars, plant materials, and lignocellulosic materials demonstrated satisfactory lipid accumulation in various microorganisms (Fakas et al., 2009; Chatzifragkou et al., 2010; Gupta et al., 2013; Zeng et al., 2013).
Nitrogen source and the carbon-to-nitrogen (C/N) ratio also play important roles in lipid accumulation. Different organic and inorganic nitrogen sources have been employed individually or in combination. Yeast extract, peptone, urea, and various nitrogen compounds have been analysed for their influence on lipid accumulation in different microorganisms (Gao et al., 2013). The supply of nitrogen and its ratio to carbon sources in the growth media is critical for lipid accumulation. Limiting nitrogen while maintaining excess carbon leads to lipid accumulation as the cells continue to assimilate carbon and convert it into lipids (Calvey et al., 2016; Kolouchová et al., 2016). The capacity of oleaginous microorganisms to accumulate lipids is dependent on the constant supply of acetyl-CoA and NADPH for fatty acid synthesis when nutrients are scarce but carbon is abundant (Ochsenreither et al., 2016). The regulation of enzyme reactions initiated by nutrient limitation leads to the accumulation of citrate in the mitochondria, which is then converted to acetyl-CoA and oxaloacetate, supplying acetyl-CoA for fatty acid production (Ratledge, 2004; Tang et al., 2013).
Other environmental growth conditions, such as aeration, pH, and temperature, also influence lipid accumulation. Aeration, or oxygen concentration, can enhance or inhibit lipid accumulation depending on the microorganism species (Calvey et al., 2016; Magdouli et al., 2018). For example, a study by Calvey et al. (2016) demonstrated 50% lower lipid production when high aeration rates was used during the cultivation of Lipomyces starkeyi. On the other hand, increased in aeration rate for Schizochytrium sp. Resulted in increased lipid and DHA content (Ren et al., 2010; Yen et al., 2013). Optimal growth temperature varies among oleaginous yeasts, with higher temperatures favoring saturated fatty acid production and lower temperatures favoring unsaturated fatty acid accumulation (Amaretti et al., 2010). pH tolerance is another important factor, as strains with tolerance to a wide range of pH values can utilize agro-industrial waste materials at acidic pH to synthesize lipids (Miller and Webb, 1954; Gao et al., 2020).
The cultivation method employed, such as batch, fed-batch, or continuous culture, impacts lipid accumulation. Batch shaking flask cultivations are commonly used in studies, but the use of baffled flasks has been shown to enhance lipid accumulation, biomass production, and the synthesis of specific fatty acids (Ling et al., 2015). Stirred tank bioreactors have also demonstrated increased lipid accumulation in certain microorganisms, such as Schizochytrium spp. and Thraustochytrium sp. BM2 strain (Chen and Yang, 2018; Chi et al., 2022).
Studies have shown that optimizing fermentation conditions can significantly increase lipid yields. Wang et al. (2018) performed fermentation experiments with Schizochytrium sp. PKU#Mn4 and Thraustochytrid sp. PKU#Mn16 and obtained the highest DHA yields of 21% and 18.9%, respectively, in 5L bioreactors employing optimal conditions and a dual oxygen control technique. The production of DHA and EPA increased by 3.4 and 2.8 times (g/L) relative to non-optimized settings (Wang et al., 2018).
Several factors influence the lipid content and composition of microbial biomass, including the choice of carbon and nitrogen sources, the carbon to nitrogen ratio, cultivation method, and environmental growth conditions. Optimizing these factors can enhance lipid accumulation in microorganisms and facilitate the production of lipids with desired characteristics for various applications.
Lipid can be extracted from microbial biomass through mechanical, non-mechanical, chemical and enzymatic methods. Mechanical methods require no chemicals during the process so the issue of chemical contamination with the lipid product will not arise. However, heat generation during the mechanical process may require additional cooling system to prevent lipid damage (Ochsenreither et al., 2016). On the other hand, non-mechanical methods do not require much energy, but the techniques are difficult to scale up for industrial application. Besides, methods that used chemicals require higher cost and may also lead to chemical contamination in the lipid product.
Mechanical methods involve high stress on cells through shear, cavitation, and impingement using abrasion, high pressure, or ultrasound. Bead milling, homogenization, and ultrasound treatment are examples of mechanical disruption methods that can be used on an industrial scale. Bead milling is a simple and effective method that uses grinding beads to impact, compact, shear, and disrupt cells (Middelberg, 1995). Homogenization involves forcing biomass through an orifice under high pressure, leading to cell disruption (Middelberg, 1995; Clarke et al., 2010). Ultrasound treatment utilizes cavitation, generated by oscillating sound waves around 25 kHz in order to disrupt cells (Thompson and Doraiswamy, 1999).
Non-mechanical methods such as microwave treatment, decompression, osmotic shock, drying, and pulsed electrical fields offer gentler cell disruption but may have limitations in terms of scalability and cost. Microwave treatment utilizes microwave-induced dielectric heating to disrupt cells through localized heating and increased intracellular pressure (Orsat and Routray, 2017). Cell disruption through decompression is conducted by mixing cell with pressurized supercritical gas. The pressure is then released causing the gas which already enters the cells to expand and result in cell disruption. Osmotic shock on the other hand is conducted by exposing the cells to a high-concentration solute medium then suddenly dilute it (Middelberg, 1995; Ochsenreither et al., 2016). This action will cause an increase in intracellular pressure and disrupt the cells. Drying biomass prior to extraction is often performed, and methods such as oven and freeze drying can also contribute to cell disruption (Guldhe et al., 2014). Pulsed electrical fields create pores in the cell membrane, enabling cell disruption and increased lipid extraction (Ho and Mittal, 1996).
The extraction of lipid from microbial biomass can also be achieved through various chemical and enzymatic methods. Chemical methods involve cell disruption or permeabilization using different chemicals such as detergents, antibiotics, alkalis, acids, chelating agents, and solvents (Ochsenreither et al., 2016). However, numerous chemical treatments are not suitable for food uses due to contamination and non-food grade nature (Geciova et al., 2002; Ochsenreither et al., 2016). Solvent extraction methods, such as the Bligh and Dyer method, allow for the combination of cell disruption and extraction of lipid without additional pretreatment (Bligh and Dyer, 1959). However, the impermeability of cell walls to solvents often requires a cell conditioning or pretreatment step to enhance extraction efficiency (Ochsenreither et al., 2016).
Enzymatic methods involve the use of lytic enzymes to specifically attack cell wall components and release intracellular products. Enzymatic lysis is advantageous due to its mild reaction conditions, substrate specificity, and safety for food applications (Ochsenreither et al., 2016). Different microorganisms require different enzymatic cocktails, and the efficiency of cell disruption is dependent on the enzyme type (Zheng et al., 2011).
Various extraction methods can be employed to separate and extract the targeted class of lipids from microbial biomass. The selection of solvents is determined by the polarity of the lipids. Classical extraction methods such as Soxhlet extraction and Folch extraction are commonly used for lipid extraction (Soxhlet, 1879; Folch et al., 1957). Soxhlet extraction is a semi-continuous method that uses organic solvents under reflux, while the Folch method uses a chloroform:methanol solvent system and involves the formation of a biphasic system to separate lipids from non-lipid components (Soxhlet, 1879; Folch et al., 1957). Pressurized liquid extraction (PLE) is comparable to Soxhlet extraction but employs liquid solvents at increased temperatures and pressures, resulting in shorter extraction times and less solvent usage (Richter et al., 1996). Another method called supercritical fluid extraction (SFE) uses supercritical fluids such as supercritical CO2 to extract lipids (Fattori et al., 1988). SFE is particularly effective for extracting non-polar lipids, but the addition of co-solvents may be required for extracting polar lipids.
Lipid extracted from microorganism is attached to glycerol so it must be converted to fatty acid ethyl esters (FAEEs) form first through transesterification. However, esterified oil contains other impurities as well as target EFAs. To increase the purity of specific EFAs and remove impurities, various concentration and purification methods have been proposed. The first method is urea adduction, where the hydrolyzed free fatty acids are mixed with an ethanolic solution of urea, forming solid-phase complexes that can be filtered out (Yves et al., 2016). This method allows for the enrichment of omega-3 fatty acids in the liquid fraction and is considered eco-friendly due to the mild operating conditions and safe chemicals used (Yves et al., 2016).
Various chromatographic methods are also employed for the concentration and purification of EFAs such as preparative high-performance liquid chromatography (HPLC), centrifugal partition chromatography (CPC), supercritical fluid chromatography (SFC), and gas chromatography (GC) (Yves et al., 2016). Reversed-phase HPLC with gradient elution has been utilized for the purification of microalgal PUFAs, including DHA and EPA (Mansour, 2005). CPC is a liquid partition-based separation method that has been successfully applied to isolate and purify PUFAs from various natural sources (Murayama et al., 1988). SFC utilizing supercritical fluids as the mobile phase, has also shown promise for the concentration of EFAs (Montañés et al., 2013). Furthermore, GC analysis of fatty acid methyl esters (FAMEs) is commonly employed for identification purposes (Ou et al., 2016). These concentration and purification techniques provide valuable tools for obtaining highly pure EFAs from microbial lipid extracts, enabling their utilization in various applications.
The production of EFAs from microbial biomass faces several challenges and limitations in terms of cost and efficiency. Several costs are associated with microbial lipid production, including carbon and nutrient sources, fermentation operation, and post-processing for lipid separation. The cost of carbon and nitrogen sources directly influences the overall cost of microbial lipid production. The replacement of glucose as the carbon source while retaining high biomass and lipid yields should be one area of emphasis. Using readily available feedstocks such as sugar-rich wastewater or industrial food waste can reduce processing costs (Angerbauer et al., 2008; Leiva-Candia et al., 2014). Other waste materials such as starch wastewater, sweet sorghum bagasse, and corncob hydrolysate can also serve as valuable carbon sources for fermentations (Xue et al., 2010; Liang et al., 2012; Chang et al., 2015). While these waste substrates offer cost advantages, they often require pre-treatment processes that increase overall production costs. Therefore, recent efforts have shifted towards reducing downstream processing costs associated with cell wall disruption, oil extraction, and refining steps.
Metabolic engineering plays a crucial role in maximizing fatty acid production in microbial biomass. Metabolic engineering of lipid-producing microorganisms can be conducted by enhancing the fatty acids and triacylglycerol synthesis pathways. Overexpression of key enzyme which provide cofactors can also be done to improve production efficiency. Other strategy such as optimizing process design through the use of multifactorial research design such as response surface methodology (RSM) may help overcome some of these challenges by minimizing substrate used and make microbial lipid production more economically viable (Sohedein et al., 2020). Additionally, exploring other valuable by-products, such as bioactive compound, enzymes and cell wall polysaccharides like β-glucan alongside EFAs could help reduce costs and make production more feasible.
MS: Conceptualization, Data curation, Investigation, Software, Visualization, Writing–original draft, Writing–review and editing. ZI: Conceptualization, Data curation, Funding acquisition, Investigation, Methodology, Project administration, Resources, Supervision, Visualization, Writing–original draft, Writing–review and editing. WW-M: Project administration, Supervision, Writing–original draft, Writing–review and editing. NT: Conceptualization, Investigation, Supervision, Writing–original draft, Writing–review and editing.
The author(s) declare financial support was received for the research, authorship, and/or publication of this article. This research was funded by the Ministry of Higher Education (MOHE) under the Fundamental Research Grant Scheme (FRGS: FP062-2022) (FRGS/1/2022/STG01/UM/02/2).
The authors would like to thank Universiti Malaya and the Ministry of Higher Education (MOHE) under the Fundamental Research Grant Scheme (FRGS: FP062-2022) (FRGS/1/2022/STG01/UM/02/2).
The authors declare that the research was conducted in the absence of any commercial or financial relationships that could be construed as a potential conflict of interest.
The author ZI declared that they were an editorial board member of Frontiers at the time of submission. This had no impact on the peer review process and the final decision.
All claims expressed in this article are solely those of the authors and do not necessarily represent those of their affiliated organizations, or those of the publisher, the editors and the reviewers. Any product that may be evaluated in this article, or claim that may be made by its manufacturer, is not guaranteed or endorsed by the publisher.
Abd Elrazak, A., Ward, A. C., and Glassey, J. (2013). Polyunsaturated fatty acid production by marine bacteria. Bioprocess Biosyst. Eng. 36, 1641–1652. doi:10.1007/s00449-013-0936-0
Amaretti, A., Raimondi, S., Sala, M., Roncaglia, L., De Lucia, M., Leonardi, A., et al. (2010). Single cell oils of the cold-adapted oleaginous yeast Rhodotorula glacialis DBVPG 4785. Microb. Cell. factories 9 (1), 73–76. doi:10.1186/1475-2859-9-73
Angerbauer, C., Siebenhofer, M., Mittelbach, M., and Guebitz, G. (2008). Conversion of sewage sludge into lipids by Lipomyces starkeyi for biodiesel production. Bioresour. Technol. 99 (8), 3051–3056. doi:10.1016/j.biortech.2007.06.045
Béligon, V., Christophe, G., Fontanille, P., and Larroche, C. (2016). Microbial lipids as potential source to food supplements. Curr. Opin. Food Sci. 7, 35–42. doi:10.1016/j.cofs.2015.10.002
Bligh, E. G., and Dyer, W. J. (1959). A rapid method of total lipid extraction and purification. Can. J. Biochem. physiology 37 (8), 911–917. doi:10.1139/o59-099
Calvey, C. H., Su, Y.-K., Willis, L. B., McGee, M., and Jeffries, T. W. (2016). Nitrogen limitation, oxygen limitation, and lipid accumulation in Lipomyces starkeyi. Bioresour. Technol. 200, 780–788. doi:10.1016/j.biortech.2015.10.104
Castanha, R. F., Mariano, A. P., Morais, L. A. S. d., Scramin, S., and Monteiro, R. T. R. (2014). Optimization of lipids production by Cryptococcus laurentii 11 using cheese whey with molasses. Braz. J. Microbiol. 45, 379–387. doi:10.1590/s1517-83822014000200003
Chang, Y.-H., Chang, K.-S., Lee, C.-F., Hsu, C.-L., Huang, C.-W., and Jang, H.-D. (2015). Microbial lipid production by oleaginous yeast Cryptococcus sp. in the batch cultures using corncob hydrolysate as carbon source. Biomass bioenergy 72, 95–103. doi:10.1016/j.biombioe.2014.11.012
Chatzifragkou, A., Fakas, S., Galiotou-Panayotou, M., Komaitis, M., Aggelis, G., and Papanikolaou, S. (2010). Commercial sugars as substrates for lipid accumulation in Cunninghamella echinulata and Mortierella isabellina fungi. Eur. J. Lipid Sci. Technol. 112 (9), 1048–1057. doi:10.1002/ejlt.201000027
Chen, C.-Y., and Yang, Y.-T. (2018). Combining engineering strategies and fermentation technology to enhance docosahexaenoic acid (DHA) production from an indigenous Thraustochytrium sp. BM2 strain. Biochem. Eng. J. 133, 179–185. doi:10.1016/j.bej.2018.02.010
Chi, G., Xu, Y., Cao, X., Li, Z., Cao, M., Chisti, Y., et al. (2022). Production of polyunsaturated fatty acids by Schizochytrium (Aurantiochytrium) spp. Biotechnol. Adv. 55, 107897. doi:10.1016/j.biotechadv.2021.107897
Clarke, A., Prescott, T., Khan, A., and Olabi, A. (2010). Causes of breakage and disruption in a homogeniser. Appl. Energy 87 (12), 3680–3690. doi:10.1016/j.apenergy.2010.05.007
Connor, W. E. (2000). Importance of n− 3 fatty acids in health and disease. Am. J. Clin. Nutr. 71 (1), 171S-5S–175S. doi:10.1093/ajcn/71.1.171S
da Silva, J., da Silva, F. L. H., Ribeiro, J. E. S., de Melo, D. J. N., Santos, F. A., and de Medeiros, L. L. (2020). Effect of supplementation, temperature and pH on carotenoids and lipids production by Rhodotorula mucilaginosa on sisal bagasse hydrolyzate. Biocatal. Agric. Biotechnol. 30, 101847. doi:10.1016/j.bcab.2020.101847
Demir, M., and Gündes, A. G. (2020). Single-cell oil production by Mortierella isabellina DSM 1414 using different sugars as carbon source. Biotechnol. Prog. 36 (6), e3050. doi:10.1002/btpr.3050
Du, F., Wang, Y.-Z., Xu, Y.-S., Shi, T.-Q., Liu, W.-Z., Sun, X.-M., et al. (2021). Biotechnological production of lipid and terpenoid from thraustochytrids. Biotechnol. Adv. 48, 107725. doi:10.1016/j.biotechadv.2021.107725
Fakas, S., Makri, A., Mavromati, M., Tselepi, M., and Aggelis, G. (2009). Fatty acid composition in lipid fractions lengthwise the mycelium of Mortierella isabellina and lipid production by solid state fermentation. Bioresour. Technol. 100 (23), 6118–6120. doi:10.1016/j.biortech.2009.06.015
Fattori, M., Bulley, N., and Meisen, A. (1988). Carbon dioxide extraction of canola seed: oil solubility and effect of seed treatment. J. Am. Oil Chemists' Soc. 65 (6), 968–974. doi:10.1007/bf02544522
Folch, J., Lees, M., and Sloane Stanley, G. H. (1957). A simple method for the isolation and purification of total lipides from animal tissues. J. Biol. Chem. 226 (1), 497–509. doi:10.1016/s0021-9258(18)64849-5
Gao, D., Zeng, J., Zheng, Y., Yu, X., and Chen, S. (2013). Microbial lipid production from xylose by Mortierella isabellina. Bioresour. Technol. 133, 315–321. doi:10.1016/j.biortech.2013.01.132
Gao, R., Li, Z., Zhou, X., Bao, W., Cheng, S., and Zheng, L. (2020). Enhanced lipid production by Yarrowia lipolytica cultured with synthetic and waste-derived high-content volatile fatty acids under alkaline conditions. Biotechnol. Biofuels 13 (1), 3–16. doi:10.1186/s13068-019-1645-y
Geciova, J., Bury, D., and Jelen, P. (2002). Methods for disruption of microbial cells for potential use in the dairy industry—A review. Int. Dairy J. 12 (6), 541–553. doi:10.1016/s0958-6946(02)00038-9
Guldhe, A., Singh, B., Rawat, I., Ramluckan, K., and Bux, F. (2014). Efficacy of drying and cell disruption techniques on lipid recovery from microalgae for biodiesel production. Fuel 128, 46–52. doi:10.1016/j.fuel.2014.02.059
Guo, D.-S., Tong, L.-L., Ji, X.-J., Ren, L.-J., and Ding, Q.-Q. (2020). Development of a strategy to improve the stability of culture environment for docosahexaenoic acid fermentation by Schizochytrium sp. Appl. Biochem. Biotechnol. 192, 881–894. doi:10.1007/s12010-020-03298-7
Guo, D. S., Ji, X. J., Ren, L. J., Li, G. L., and Huang, H. (2017). Improving docosahexaenoic acid production by Schizochytrium sp. using a newly designed high-oxygen-supply bioreactor. AIChE J. 63 (10), 4278–4286. doi:10.1002/aic.15783
Gupta, A., Singh, D., Barrow, C. J., and Puri, M. (2013). Exploring potential use of Australian thraustochytrids for the bioconversion of glycerol to omega-3 and carotenoids production. Biochem. Eng. J. 78, 11–17. doi:10.1016/j.bej.2013.04.028
Hashem, A. H., Suleiman, W. B., Abu-elreesh, G., Shehabeldine, A. M., and Khalil, A. M. A. (2020). Sustainable lipid production from oleaginous fungus Syncephalastrum racemosum using synthetic and watermelon peel waste media. Bioresour. Technol. Rep. 12, 100569. doi:10.1016/j.biteb.2020.100569
Ho, S., and Mittal, G. S. (1996). Electroporation of cell membranes: A review. Crit. Rev. Biotechnol. 16 (4), 349–362. doi:10.3109/07388559609147426
Jones, A. D., Boundy-Mills, K. L., Barla, G. F., Kumar, S., Ubanwa, B., and Balan, V. (2019). Microbial lipid alternatives to plant lipids. Microb. lipid Prod. methods Protoc. 1995, 1–32. doi:10.1007/978-1-4939-9484-7_1
Karnaouri, A., Chalima, A., Kalogiannis, K. G., Varamogianni-Mamatsi, D., Lappas, A., and Topakas, E. (2020). Utilization of lignocellulosic biomass towards the production of omega-3 fatty acids by the heterotrophic marine microalga Crypthecodinium cohnii. Bioresour. Technol. 303, 122899. doi:10.1016/j.biortech.2020.122899
Kautharapu, K. B., Rathmacher, J., and Jarboe, L. R. (2013). Growth condition optimization for docosahexaenoic acid (DHA) production by Moritella marina MP-1. Appl. Microbiol. Biotechnol. 97, 2859–2866. doi:10.1007/s00253-012-4529-7
Kikukawa, H., Sakuradani, E., Ando, A., Shimizu, S., and Ogawa, J. (2018). Arachidonic acid production by the oleaginous fungus Mortierella alpina 1S-4: A review. J. Adv. Res. 11, 15–22. doi:10.1016/j.jare.2018.02.003
Kolouchová, I., Maťátková, O., Sigler, K., Masák, J., and Řezanka, T. (2016). Lipid accumulation by oleaginous and non-oleaginous yeast strains in nitrogen and phosphate limitation. Folia Microbiol. 61, 431–438. doi:10.1007/s12223-016-0454-y
Leiva-Candia, D., Pinzi, S., Redel-Macías, M., Koutinas, A., Webb, C., and Dorado, M. (2014). The potential for agro-industrial waste utilization using oleaginous yeast for the production of biodiesel. Fuel 123, 33–42. doi:10.1016/j.fuel.2014.01.054
Liang, Y., Tang, T., Siddaramu, T., Choudhary, R., and Umagiliyage, A. L. (2012). Lipid production from sweet sorghum bagasse through yeast fermentation. Renew. Energy 40 (1), 130–136. doi:10.1016/j.renene.2011.09.035
Ling, X., Guo, J., Liu, X., Zhang, X., Wang, N., Lu, Y., et al. (2015). Impact of carbon and nitrogen feeding strategy on high production of biomass and docosahexaenoic acid (DHA) by Schizochytrium sp. LU310. Bioresour. Technol. 184, 139–147. doi:10.1016/j.biortech.2014.09.130
Liu, J., Huang, J., Jiang, Y., and Chen, F. (2012). Molasses-based growth and production of oil and astaxanthin by Chlorella zofingiensis. Bioresour. Technol. 107, 393–398. doi:10.1016/j.biortech.2011.12.047
Mansour, M. P. (2005). Reversed-phase high-performance liquid chromatography purification of methyl esters of C16–C28 polyunsaturated fatty acids in microalgae, including octacosaoctaenoic acid [28: 8 (n-3)]. J. Chromatogr. A 1097 (1-2), 54–58. doi:10.1016/j.chroma.2005.08.011
Magdouli, S., Brar, S. K., and Blais, J. F. (2018). Morphology and rheological behaviour of yarrowia lipolytica: impact of dissolved oxygen level on cell growth and lipid composition. Process Biochem. 65, 1–10. doi:10.1016/j.procbio.2017.10.021
Middelberg, A. P. (1995). Process-scale disruption of microorganisms. Biotechnol. Adv. 13 (3), 491–551. doi:10.1016/0734-9750(95)02007-p
Miller, J., and Webb, N. (1954). Isolation of yeasts from soil with the aid of acid, rose bengal, and oxgall. Soil Sci. 77 (3), 197–204. doi:10.1097/00010694-195403000-00003
Mirbagheri, M., Nahvi, I., and Emamzade, R. (2015). Reduction of chemical and biological oxygen demands from oil wastes via oleaginous fungi: an attempt to convert food by products to essential fatty acids. Iran. J. Biotechnol. 13 (2), 25–30. doi:10.15171/ijb.1026
Mohamed, H., El-Shanawany, A.-R., Shah, A. M., Nazir, Y., Naz, T., Ullah, S., et al. (2020). Comparative analysis of different isolated oleaginous Mucoromycota fungi for their γ-linolenic acid and carotenoid production. BioMed Res. Int., 2020, 3621543, doi:10.1155/2020/3621543
Moi, I. M., Leow, A. T. C., Ali, M. S. M., Rahman, R. N. Z. R. A., Salleh, A. B., and Sabri, S. (2018). Polyunsaturated fatty acids in marine bacteria and strategies to enhance their production. Appl. Microbiol. Biotechnol. 102, 5811–5826. doi:10.1007/s00253-018-9063-9
Mokochinski, J. B., López, B. G., Sovrani, V., Dalla Santa, H. S., González-Borrero, P. P., Sawaya, A. C. H. F., et al. (2015). Production of Agaricus brasiliensis mycelium from food industry residues as a source of antioxidants and essential fatty acids. Int. J. Food Sci. Technol. 50 (9), 2052–2058. doi:10.1111/ijfs.12861
Moniz, P., Silva, C., Oliveira, A. C., Reis, A., and Lopes da Silva, T. (2021). Raw glycerol based medium for DHA and lipids production, using the marine heterotrophic microalga Crypthecodinium cohnii. Processes 9 (11), 2005. doi:10.3390/pr9112005
Montañés, F., Catchpole, O. J., Tallon, S., Mitchell, K., and Lagutin, K. (2013). Semi-preparative supercritical chromatography scale plant for polyunsaturated fatty acids purification. J. Supercrit. Fluids 79, 46–54. doi:10.1016/j.supflu.2012.11.018
Murayama, W., Kosuge, Y., Nakaya, N., Nunogaki, Y., Nunogaki, K. I., Cazes, J., et al. (1988). Preparative separation of unsaturated fatty acids esters by centrifugal partition chromatography (CPC). J. Liq. Chromatogr. 11 (1), 283–300. doi:10.1080/01483919808068329
Ng, W. K., and Romano, N. (2013). A review of the nutrition and feeding management of farmed tilapia throughout the culture cycle. Rev. Aquac. 5 (4), 220–254. doi:10.1111/raq.12014
Ochsenreither, K., Glück, C., Stressler, T., Fischer, L., and Syldatk, C. (2016). Production strategies and applications of microbial single cell oils. Front. Microbiol. 7, 1539. doi:10.3389/fmicb.2016.01539
Orsat, V., and Routray, W. (2017). “Microwave-assisted extraction of flavonoids,” in Water extraction of bioactive compounds (Germany: Elsevier), 221–244.
Ou, M.-C., Yeong, H.-Y., Pang, K.-L., and Phang, S.-M. (2016). Fatty acid production of tropical thraustochytrids from Malaysian mangroves. Bot. Mar. 59 (5), 321–338. doi:10.1515/bot-2016-0031
Parikh, P., McDaniel, M. C., Ashen, M. D., Miller, J. I., Sorrentino, M., Chan, V., et al. (2005). Diets and cardiovascular disease: an evidence-based assessment. J. Am. Coll. Cardiol. 45 (9), 1379–1387. doi:10.1016/j.jacc.2004.11.068
Patel, A., Karageorgou, D., Rova, E., Katapodis, P., Rova, U., Christakopoulos, P., et al. (2020). An overview of potential oleaginous microorganisms and their role in biodiesel and omega-3 fatty acid-based industries. Microorganisms 8 (3), 434. doi:10.3390/microorganisms8030434
Pratiwy, F. M., and Pratiwi, D. Y. (2020). The potentiality of microalgae as a source of DHA and EPA for aquaculture feed: A review. Int. J. Fish. Aquat. Stud. 8, 39–41.
Ratledge, C. (2004). Fatty acid biosynthesis in microorganisms being used for single cell oil production. Biochimie 86 (11), 807–815. doi:10.1016/j.biochi.2004.09.017
Ren, L.-J., Ji, X.-J., Huang, H., Qu, L., Feng, Y., Tong, Q.-Q., et al. (2010). Development of a stepwise aeration control strategy for efficient docosahexaenoic acid production by Schizochytrium sp. Appl. Microbiol. Biotechnol. 87, 1649–1656. doi:10.1007/s00253-010-2639-7
Richter, B. E., Jones, B. A., Ezzell, J. L., Porter, N. L., Avdalovic, N., and Pohl, C. (1996). Accelerated solvent extraction: A technique for sample preparation. Anal. Chem. 68 (6), 1033–1039. doi:10.1021/ac9508199
Salunke, D., Manglekar, R., Gadre, R., Nene, S., and Harsulkar, A. M. (2015). Production of polyunsaturated fatty acids in recombinant Lipomyces starkeyi through submerged fermentation. Bioprocess Biosyst. Eng. 38, 1407–1414. doi:10.1007/s00449-015-1382-y
Simopoulos, A. P. (1999). Essential fatty acids in health and chronic disease. Am. J. Clin. Nutr. 70 (3), 560S-569S–569s. doi:10.1093/ajcn/70.3.560s
Sohedein, M. N. A., Wan, W. A. A. Q. I., Hui-Yin, Y., Ilham, Z., Chang, J.-S., Supramani, S., et al. (2020). Optimisation of biomass and lipid production of a tropical thraustochytrid Aurantiochytrium sp. UMACC-T023 in submerged-liquid fermentation for large-scale biodiesel production. Biocatal. Agric. Biotechnol. 23, 101496. doi:10.1016/j.bcab.2020.101496
Soxhlet, F. v. (1879). Die gewichtsanalytische bestimmung des milchfettes. Polytech. J. 232 (5), 461–465.
Tang, X., Feng, H., and Chen, W. N. (2013). Metabolic engineering for enhanced fatty acids synthesis in Saccharomyces cerevisiae. Metab. Eng. 16, 95–102. doi:10.1016/j.ymben.2013.01.003
Thompson, L. H., and Doraiswamy, L. (1999). Sonochemistry: science and engineering. Industrial Eng. Chem. Res. 38 (4), 1215–1249. doi:10.1021/ie9804172
Turchini, G. M., Torstensen, B. E., and Ng, W. K. (2009). Fish oil replacement in finfish nutrition. Rev. Aquac. 1 (1), 10–57. doi:10.1111/j.1753-5131.2008.01001.x
Wang, Q., Ye, H., Sen, B., Xie, Y., He, Y., Park, S., et al. (2018). Improved production of docosahexaenoic acid in batch fermentation by newly-isolated strains of Schizochytrium sp. and Thraustochytriidae sp. through bioprocess optimization. Synthetic Syst. Biotechnol. 3 (2), 121–129. doi:10.1016/j.synbio.2018.04.001
Xue, F., Gao, B., Zhu, Y., Zhang, X., Feng, W., and Tan, T. (2010). Pilot-scale production of microbial lipid using starch wastewater as raw material. Bioresour. Technol. 101 (15), 6092–6095. doi:10.1016/j.biortech.2010.01.124
Yen, H.-W., Hu, I.-C., Chen, C.-Y., Ho, S.-H., Lee, D.-J., and Chang, J.-S. (2013). Microalgae-based biorefinery–from biofuels to natural products. Bioresour. Technol. 135, 166–174. doi:10.1016/j.biortech.2012.10.099
Yves, H., Korma, S. A., Ali, A. H., Tuyishime, M. A., Habinshuti, I., and Abed, S. M. (2016). Extraction, refining and purification of ω-3 PUFA through different techniques—A review. Am. J. Food Sci. Nutr. Res. 4, 18–26.
Zeng, J., Zheng, Y., Yu, X., Yu, L., Gao, D., and Chen, S. (2013). Lignocellulosic biomass as a carbohydrate source for lipid production by Mortierella isabellina. Bioresour. Technol. 128, 385–391. doi:10.1016/j.biortech.2012.10.079
Zheng, H., Yin, J., Gao, Z., Huang, H., Ji, X., and Dou, C. (2011). Disruption of Chlorella vulgaris cells for the release of biodiesel-producing lipids: A comparison of grinding, ultrasonication, bead milling, enzymatic lysis, and microwaves. Appl. Biochem. Biotechnol. 164, 1215–1224. doi:10.1007/s12010-011-9207-1
Keywords: essential fatty acids, microbial biomass, microbial oil, PUFA, single cell oil
Citation: Sohedein MNA, Ilham Z, Wan-Mohtar WAAQI and Taufek NM (2023) Upstream and downstream processing of essential fatty acids from microbial biomass. Front. Food. Sci. Technol. 3:1258087. doi: 10.3389/frfst.2023.1258087
Received: 13 July 2023; Accepted: 22 August 2023;
Published: 01 September 2023.
Edited by:
Octavio Paredes-Lopez, Centro de Investigación y de Estudios Avanzados del Instituto Politécnico Nacional (CINVESTAV), MexicoReviewed by:
Jianguo Zhang, University of Shanghai for Science and Technology, ChinaCopyright © 2023 Sohedein, Ilham, Wan-Mohtar and Taufek. This is an open-access article distributed under the terms of the Creative Commons Attribution License (CC BY). The use, distribution or reproduction in other forums is permitted, provided the original author(s) and the copyright owner(s) are credited and that the original publication in this journal is cited, in accordance with accepted academic practice. No use, distribution or reproduction is permitted which does not comply with these terms.
*Correspondence: Zul Ilham, aWxoYW1AdW0uZWR1Lm15
Disclaimer: All claims expressed in this article are solely those of the authors and do not necessarily represent those of their affiliated organizations, or those of the publisher, the editors and the reviewers. Any product that may be evaluated in this article or claim that may be made by its manufacturer is not guaranteed or endorsed by the publisher.
Research integrity at Frontiers
Learn more about the work of our research integrity team to safeguard the quality of each article we publish.