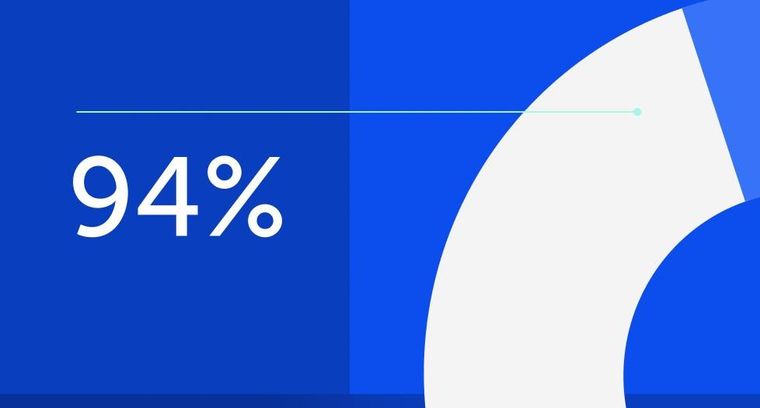
94% of researchers rate our articles as excellent or good
Learn more about the work of our research integrity team to safeguard the quality of each article we publish.
Find out more
ORIGINAL RESEARCH article
Front. Food. Sci. Technol., 12 June 2023
Sec. Food Biotechnology
Volume 3 - 2023 | https://doi.org/10.3389/frfst.2023.1208298
This article is part of the Research TopicThe Future of FoodsView all 9 articles
Growing meat in vitro using tissue engineering and bioproduction techniques (cellular agriculture) has become an increasingly promising solution to the global food security challenge. Our lab has established methods to cultivate bovine muscle tissue on decellularized plants, representing a viable low-cost, sustainable method to grow meat on edible scaffolds. Most work in this area has focused on the use of edible plant materials (i.e., spinach leaves, apple, broccoli) with inherent economic value. Harvest waste such as corn husk or jackfruit represent abundant sources of cellulose for scaffold production and may be a viable alternative. The present study aims to investigate production of cultured meat through tissue engineering and bioproduction on decellularized, edible samples of corn husk and jackfruit rind. Corn husks and jackfruit rinds were exposed to immersion decellularization. DNA quantification and histological analysis demonstrated sufficient decellularization (0.17 ± 0.06 and 0.07 ± 0.00 ug DNA/g tissue for corn husk and jackfruit rinds, respectively). Following decellularization, corn husk scaffold stiffnesses decreased from 56.67±16.71 MPa to 12.95±2.43 MPa in fiber-aligned direction, while jackfruit decreased from 7.54 ±2.42 MPa to 2.47±1.47 MPa. Seeded scaffolds with bovine satellite cells (BSCs) (11.45±2.24 ug/ul lysate/Gram) and avian (QM7s) (12.90±1.99 ug/ul lysate/Gram) demonstrated increased protein yields on jackfruit scaffolds. QM7 cultured on corn husk scaffolds yielded increased protein but PBSCs seeded on corn husks did not yield protein content higher than controls (QM7 on corn husk: 16.28±3.55, PBSCs on corn husks: 9.57±1.56 ug/ul lysate/Gram, control: 6.35±1.43 ug/ul lysate/Gram). Additionally, cell transfer from scaffold to scaffold (bead-to-bead transfer) was observed on corn husk scaffolds in a dynamic environment. These results suggest that decellularized harvest waste scaffolds may aid in realization of cultured meat products that will contribute to a more robust and environmentally sustainable food supply.
The rising world population, expected to exceed 9 billion people by 2050, is inducing unsustainable strain on current food production systems, which are already hampered by increasingly limited arable land, fresh water, and environmental concerns exacerbated by climate change (Goodland, 1997; Tuomisto, 2011). Further, animal-based protein consumption worldwide has surged in the last 50 years, rising from 61 g per person per day in 1961 to 80 g as of 2011, placing additional pressure on our agricultural systems (Sans & Combris, 2015; Newman et al., 2023). A major paradigm shift in the production of food is critical for retaining food stability.
Cellular agriculture has been proposed as a more environmentally sustainable, less resource-intensive method for expanding food production, which could help reduce greenhouse gas emission, water use, and significant global deforestation and biodiversity loss caused by conventional animal agriculture (Holmes et al., 2022; Joo et al., 2022; Knežić et al., 2022). Through these processes, meat would be generated in vitro by expanding cells acquired from skeletal muscle biopsies or other methods and expanded in bioreactors to produce meat. Despite the high capital influx and lofty predictions of marketable cultured meat products becoming available in the near future, many technological and economic barriers continue to hinder mass production (Stephens et al., 2018; Holmes et al., 2022). Radically low-cost technologies must be developed and leveraged to reduce the production cost of cultured meat.
Cellular agriculture requires a cell source and, for anchorage dependent cells, scaffold materials to offer a stiff substrate upon which cell expansion and differentiation can occur (Bhat et al., 2014; Bar-Shai et al., 2021; Andreassen et al., 2022). Bioprocesses for preparation of industrial quantities of anchorage-dependent cells employ microcarriers as scaffolds to facilitate high-volume cultivation within suspension bioreactors. Cell expansion using microcarriers is well-established for pharmaceutical applications; however, traditional carriers were not designed in consideration of the unique demands of the cellular agriculture use-case (Wang & Ouyang, 1999; Verbruggen et al., 2018; Bodiou et al., 2020; Andreassen et al., 2022). Such considerations are well reviewed and highlight unique emphases: scaffolds must be palatable and safe for consumption, nutritious, low cost, and readily-available, while still facilitating cell adhesion and growth. Additionally, exhibition of cell migration from populated carriers to unpopulated carriers within bioreactors, a phenomenon referred to as bead-to-bead transfer, may further justify use of the carrier in scaling the bioprocess.
In previous studies, our laboratory has established that decellularized plants can be leveraged as biomaterials to support mammalian cell growth (Gershlak et al., 2017). We have established that bovine satellite cells can grow and differentiate readily on decellularized spinach-derived scaffolds, and further demonstrated the use of broccoli florets as a microcarrier for suspension culture within a bioreactor (Jones et al., 2021; Thyden et al., 2022). Such plant-derived scaffolds are primarily cellulose and therefore edible. Additionally, decellularization process time and cost is minimal, requiring only readily-available detergents that are already utilized in pharmaceuticals and food production (Harris et al., 2021; Thyden et al., 2022). However, these previous studies, in addition to similar efforts by other researchers, largely employ traditional foods as starting materials for scaffolds (Modulevsky et al., 2014; 2016; Hickey & Pelling, 2019; Jones et al., 2021; Thyden et al., 2022). These foodstuffs have an implicit market value on their own, and therefore may not be ideal for cellular agriculture application.
Agricultural harvest waste materials, (plant parts such as corn husks) and food waste like fruit rinds may be alternative sources for edible cellulose scaffolds. Globally, plant waste generated by the food industry represents a major underutilized source of lignocellulosic material, with an estimated 3.8 billion metric tons of crop residue generated per year (Galiwango et al., 2019; Xu et al., 2019; Santolini et al., 2021; Araya-Chavarría et al., 2022). Repurposing these wastes as a component of a cultured meat product represents a significant opportunity to both add value back to conventional agricultural crops within the framework of a cellular agriculture economy, while simultaneously reducing pollution created from either burning or depositing the agricultural waste into landfills (Santolini et al., 2021).
In this work, we evaluate two common harvest wastes - corn husks and jackfruit rind—as scaffolds for use in cellular agriculture applications. Corn husk is a monocot leaf with parallel striations that visually resemble the anisotropy of skeletal muscle, which may be advantageous for in vitro muscle tissue growth (Pengelly et al., 2011). Jackfruit is well-established as a fibrous, nutritious meat alternative on its own (Norris et al., 2021), and the inner rind (known as jackfruit rags) is similarly fibrous and may provide a scaffold with texture that more closely mimics structured meat. We investigated decellularization efficacy, scaffold stiffness, and cell seeding and with intermittent agitation to simulate a bioreactor environment. Generally, we concluded that decellularization successfully removes cellular material and DNA, and reduces scaffold stiffness.
Bovine satellite cells (BSCs) and a quail myoblast cell line (QM7) (Antin & Ordahl, 1991) are able to adhere to both scaffolds, display bead-to-bead transfer when seeded in an environment with intermittent agitation, and contribute additional protein content to the edible scaffold. While both scaffolds present viable materials for cultured meat production, we found jackfruit to be the more promising option with respect to mechanical similarity to native bovine muscle and post-culture protein content. These results suggest that the incorporation of decellularized agricultural waste products into novel bioprocesses may successfully yield large quantities of a cell-scaffold hybrid meat product. Thus, we propose a novel closed bioreactor system for cellular agriculture products.
All primary bovine satellite cells used in each study were isolated from skeletal muscle of adult cows, sourced from a local slaughterhouse, as previously described (Jones et al., 2021). During expansion, BSCs were maintained in growth media (GM) consisting of DMEM/F-12 (Thermo Fisher Scientific, Waltham, MA, United States) that has been supplemented with 10% FBS (Thermo Fisher Scientific),1% Penicillin/Streptomycin (Thermo Fisher Scientific), and the following growth factors (Thermo Fisher Scientific): 4 ng/mL recombinant human fibroblast growth factor—2 (FGF2), 2.5 ng/mL recombinant human hepatocyte growth factor (HGF), 10 ng/mL recombinant human epidermal growth factor (EGF), and 5 ng/mL recombinant human insulin-like growth factor—1 (IGF)). QM7 satellite cells, an immortalized cell line derived from quail muscle, were purchased (ATCC, Manassas, VA, United States) and maintained under the same conditions as the bovine satellite cells. Differentiation media consisted of DMEM/F12, 2% heat-inactivated fetal bovine serum, and 1% P/S and the growth factors consistent with GM.
Fresh whole jackfruit was acquired from a local supermarket and, using a pair of iris scissors, rind fibers, exclusive of the edible fruit surrounding the large seeds, were manually separated from the fruit’s pith and rinsed with DIH20. Rind fibers were submerged in 10% SDS (Sigma-Aldrich, 124 St. Louis, MO, United States), 3% Polysorbate-20 (Sigma-Aldrich), and 10% bleach (The Clorox Co., 125 Oakland, CA, United States) in a 50 mL conical tube and placed on a laboratory roller for 48 h. Samples were then rinsed in DIH20 for 24 h and stored at 4C in DI H20. Similarly, decellularized corn husk samples were segmented using iris scissors and submerged and continuously agitated in 1% SDS in DI water for 5 days, followed by 2 days in 1% Polysorbate-20, 3% bleach, and 1 day in DI H2O. Samples were stored at 4°C in DI water until used. Solutions were exchanged daily.
The total DNA content of the fresh and decellularized samples of jackfruit and corn husk was analyzed using a CYQUANT® DNA assay kit (Thermo Fisher Scientific, Waltham, MA, United States), per the manufacturer’s instructions.
Prior to the test, fresh and decellularized samples were sequentially flash-frozen using liquid nitrogen, minced using iris scissors to ensure homogenization of the samples, and stored in 1x PBS at −80°C for up to 1 month, until analysis was performed. DNA analysis was performed using a BioTek Cytation 1 Imaging Plate Reader (Winooski, VT, United States) at 480 nm excitation and 520 nm absorption.
Fresh and decellularized samples of jackfruit and corn husk were submerged in 4% paraformaldehyde for approximately 20 min and rinsed with phosphate buffer saline. Samples were processed, embedded in paraffin, sectioned via microtome, stained using Safranin-O and fast green, and imaged via inverted microscope as previously described (Thyden et al., 2022). Additionally, paraffin sections were deparaffinized using xylene, rehydrated, and stained in 0.02% toluidine blue for 5 min and imaged brightfield.
To evaluate both the impact of decellularization on scaffold stiffness, and to compare scaffold elasticity to published values of native bovine muscle tissue, tensile stiffness of scaffolds was evaluated. To do so, fresh and decellularized jackfruit and corn husk samples were pulled to failure using an Instron 68TM-30 universal testing system (Instron, Norwood, MA) equipped with a 100 N load cell. Jackfruit samples were axially affixed into the machine and pulled at a constant 10 mm/min until failure. Young’s modulus was calculated via slope of the linear region of the resultant stress/strain graphs for both fresh and decellularized samples (n ≥ 4).
Corn husk, which is classified as a monocot, possesses longitudinally striated leaf venation (Pengelly et al., 2011), causing the material to be structurally anisotropic. To best characterize its mechanics, samples were pulled to failure both parallel to leaf venation and orthogonal to it. To do so, both fresh and decellularized corn husk samples were cut into dog-bone shapes with ∼1.5 cm width in the center, and tested under the same conditions as jackfruit (n ≥ 4).
Decellularized cornhusk and jackfruit scaffolds were sterilized via submersion in 70% ethanol for a minimum of 10 min. Scaffolds were then submerged in sterile 1X PBS for 5 min three times to eliminate trace quantities of ethanol. The scaffolds were then conditioned in growth media and equilibrated for a minimum of 30 min at 37C and 5%CO2. In sterile, vented, 15 mL conical tubes BSCs or QM7s, jackfruit fibers or corn husk, and GM was inoculated at a ratio of 106 cells/100 mg scaffolds/1 mL GM, based on the weight of the scaffold. Each reactor was manually agitated every 15 min for at least 1 h. After 24 h, 5 additional mL of growth media was added to each reactor and each reactor was manually agitated every subsequent 24 h until the completion of each study. Scaffolds were then visualized using Phalloidin and Hoechst 33342 and fluorescent microscopy as previously described (Thyden et al., 2022).
For cell and scaffold visualization and protein analysis, samples were maintained in GM for 72 h, and were then maintained for 1 week in differentiation media. Media was changed every 2–3 days. Upon completion of the culture, samples were either frozen at −80C for protein analysis, or immediately fixed in 4% paraformaldehyde for staining. Additional samples were fixed for imaging after only 3 days in GM.
A BCA analysis was performed on fresh, decellularized, and recellularized samples of jackfruit and corn husk. Additional samples of decellularized jackfruit and cornhusk that had been conditioned in growth media for 1 hour and once frozen were assessed as well. Jackfruit fiber masses were recorded, and then placed into 100uL of NP-40 cell lysis buffer (Thermo Fisher Scientific) with 1ul of protease inhibitor cocktail (Thermo Fisher Scientific), for 30 min. Samples were vortexed and lysates were analyzed for total protein via Pierce BCA Assay (Thermo Fisher Scientific) against a bovine serum albumin standard curve. Absorbance at 562 nm was quantified using a BioTek Cytation 1 Imaging Plate Reader 1 plate reader. All samples were normalized to their total (final) mass in mg (3 samples were tested from 3 bioreactors for each condition).
To study the ability for cells to migrate from one scaffold to another, decellularized corn husk was shaped into circles, squares, and triangles. 100 mg of circle-shaped decellularized corn husk was sterilized and conditioned as described above, and simultaneously inoculated into 15 mL conical tubes with growth media and QM7 cells at a ratio of 2 × 106 cells/mL in 1 mL of growth media. The suspension was incubated for 24 h, and the initial cell media was replaced with fresh growth media. 100 mg of square and triangle shaped decellularized corn husk scaffolds were inoculated into the culture after 48, and 96 h, respectively (Figure 1). 48 h following the inoculation of triangle shaped scaffolds, all scaffolds were fixed with 4% paraformaldehyde for 15 min. Samples were stained for using Phalloidin and Hoechst 33342 as described above, and imaged using a BioTek Cytation 1 Imaging Plate Reader.
FIGURE 1. Bead-to-bead transfer schematic showing introduction of new scaffolds at different time points, differentiated by shape. Figure made in BioRender.
An automated tiling and stitching strategy was used to image the entirety of each scaffold at 10x to image the entirety of the scaffolds. Additionally, for each tile, z-stacks of ten images spanning the thickness of the sample were captured. The maximum intensity of each pixel from the z-stack was projected into a new image for nuclear counting. To distinguish nuclei from background autofluorescence, the Trainable Weka Segmentation plugin of FIJI was used to generate binary masks of cell nuclei, which were then counted. Nine shapes from a total of 3 bioreactors were quantified.
Statistical analysis was performed using GraphPad Prism 9. Analysis of variance (ANOVA) with multiple comparisons was used in all tests, using two-sided t-tests for post hoc analyses. Significance was considered for p-values under 0.05.
Jackfruit fibers and cornhusk segments were prepared, and decellularized as described in the protocol above. Each scaffold progressively lost its color and attained a translucent white color as the protocol was completed (Figure 2A).
FIGURE 2. (A) Images of Decellularized and Fresh jackfruit (top) and corn husk (bottom). (B) Cyquant DNA assay results Fresh and Decellularized corn husk and jackfruit. **** = p < 0.0001, NS = no significance. n = 3, all groups. (C) Safranin-O and Fast Green stained corn husk and jackfruit, both Fresh and Decellularized. Absence of cellular material (green/blue) but retention of matrix (pink) is evident in decellularized sample images. Scale bars indicated on images.
A nearly complete loss of DNA was observed when Jackfruit fibers and cornhusk segments were submitted to the decellularization process when compared to fresh samples (Figure 2B). Fresh corn husk segments and jackfruit fibers contained an average of 39.41 ± 4.46 and 4.00+/1.64 ug DNA/g tissue respectively, and decellularized corn husk segments and jackfruit fibers contained an average of 0.17 ± 0.06 and 0.07 ± 0.00 ug DNA/g tissue respectively.
Safranin-O and Fast green staining was performed on fresh and decellularized jackfruit fibers and cornhusk segments (Figure 2C). The absence of intracellular green color and dark purple nuclei in decellularized samples complement the quantitative results, thus validating the decellularization protocols for each scaffold.
Fresh and decellularized corn husk and jackfruit rind (Figure 3A) exhibited stiffnesses above native bovine muscle (∼8 kPa), in the MPa range. Corn husk stiffness was reduced post-decellularization when pulled parallel to the aligned venation (56.67±16.71 MPa vs. 12.95±2.43 MPa before/after decellularization) but not in the perpendicular direction (10.78±6.42 MPa vs. 6.18±2.42 MPa before/after decellularization). Jackfruit stiffness was also significantly reduced post-decellularization (7.54±2.42 MPa vs. 2.47±1.47 MPa, fresh vs. decellularized) (Figure 3B).
FIGURE 3. Uniaxial tissue mechanics on (A) Fresh corn husk and jackfruit (top) and Decellularized corn husk and jackfruit (bottom). (B) Young’s modulus calculations for corn husk in fiber parallel and fiber perpendicular directions, and for jackfruit. Fresh and Decellularized samples are compared in each group. n = 4 for all samples, * indicates p < 0.05, and **** indicates p < 0.0001, both in a two-sided Tukey post hoc t-test, NS indicates no significance.
BSCs and QM7 cells were seeded onto decellularized jackfruit fibers and decellularized corn husk segments and observed for cell nuclei and cytoskeletal filaments after having been cultured for 3 days in proliferation media. Both QM7s and BSCs were observed to have adhered over extensive scaffold regions (up to 2.5 cm^2) of the decellularized jackfruit and decellularized corn husk fibers after 3 days in proliferation media (Figures 4A–D).
FIGURE 4. Hoechst 33342 (blue) and phalloidin (pink) fluorescent imaging of PBSCs and QM7s on decellularized corn husk and jackfruit scaffolds at Day 3 of culture. (A) QM7s on jackfruit scaffold, presenting as aggregated clumps across the length of the sample. (B) Seeded deposits of QM7s on corn husk scaffold. (C) PBSCs on jackfruit scaffolds, with inserts showing cells with elongated F-actin striations. (D) PBSCs on corn husk scaffold qualitatively showing extensive scaffold colonization and cell alignment. Scale bars indicated on images.
Subsequent culture was conducted for 7 days in low-serum differentiation media and cells remain adhered after the additional 7 days (Figures 5A–D). Qualitatively, it was observed that BSCs demonstrate localized regions of cytoskeletal alignment on both scaffolds, a common metric for characterizing skeletal muscle organization, whereas it was observed that QM7 cells adhered with less organization. Additionally, when seeded onto jackfruit fiber samples, QM7 cells appeared as multicellular agglomerations.
FIGURE 5. Hoechst 33342 (blue) and phalloidin (pink) fluorescent imaging of PBSCs and QM7s on decellularized corn husk and jackfruit scaffolds at Day 7 of culture. (A, B) indicating QM7 scaffold colonization on jackfruit and corn husk scaffolds, respectively. (C) PBSCs on jackfruit scaffolds, with inserts presenting cell deposition across the fiber length of the scaffold. (D) PBSCs on corn husk scaffold indicating localized cell alignment. Scale bars indicated on images.
Protein content of cell seeded scaffolds was compared against untreated decellularized scaffolds and media-conditioned scaffolds primed for 1 h in media with 10% FBS. Untreated jackfruit scaffold protein content was negligible. Culture media-conditioned jackfruit samples had an average of 0.61 ± 0.35 ug/ul lysate protein per Gram of tissue mass while PBSC-seeded and QM7-seeded samples had 11.45±2.24 and 12.90±1.99 ug/ul lysate/Gram respectively (Figures 6A,B).
FIGURE 6. BCA protein analysis measured as protein lysate concentration in µg/µL, normalized by total tissue (cells and scaffold) mass in g, on jackfruit rind fibers (A) and corn husk (B). Groups for both scaffold types include untreated decellularized scaffolds, decellularized samples treated for 1 h in growth media (GM), scaffolds with seeded PBSCs cultured for 10 days, and scaffolds with QM7s cultured for 10 days * = p < 0.05, ** = p < 0.01, *** = p < 0.001, *** = p < 0.0001, NS = no significance between groups. n = 3 for all groups, statistics are derived from ANOVA with post hoc Tukey t-test.
There was no significant difference between the protein detected in jackfruit samples seeded with QM7 cells and PBSCs. PBSC-seeded corn husk protein content was not significantly different to corn husk conditioned in culture media. However, corn husk seeded with QM7s had a measurable increase in protein content (16.28±3.55 ug/ul lysate/Gram) to both samples seeded with PBSCs, and media-conditioned samples (9.57±1.56 and 6.35±1.43 ug/ul lysate/Gram, respectively) (Figures 6A,B). There was no significant difference between the protein detected in jackfruit samples seeded with QM7 cells and PBSCs; however, there was a significant difference between mammalian cell seeded jackfruit and jackfruit that was conditioned in growth media.
Upon completion of the study described in Figure 1, circular corn husk scaffolds maintained in media for the full culture time contained 811±618.1 nuclei/mm^2, square scaffolds seeded at Day 2 acquired 117±108 nuclei/mm^2, and triangle scaffolds introduced at Day 4 acquired 36±35 nuclei/mm^2. There was a significant difference in the nuclei density between circle and both square and triangular shapes and there was no significant difference between the nuclei density on square and triangle shapes (Figures 7A,B).
FIGURE 7. (A) Bead-to-bead transfer images showing transfer of QM7 cells at each time point. (B) QM7 cell count on scaffolds at each time point. n = 9, * indicates p < 0.05 with Tukey two-sided t-test, NS indicates no significance.
The realization of cellular agriculture may aid in satisfying protein demand while sustainably reducing dependence on unsustainable meat production strategies. Consumer transition from traditional meat products to products of cellular agriculture will depend on a number of factors, notably, the nutritional characteristics and cost parity. Several techno-economic analyses suggest that radical reductions in the bioproduction costs of mammalian cells, at scale, are necessary for cultured meat products to reach an economically competitive price for consumers (Bhat et al., 2014; Raman, 2021; Garrison et al., 2022). For reference, the USDA reports that the average cost of lean beef in 2021 was approximately $6.17/kg, versus approximately $63/kg for cultured meat (Daily Beef Reports | Agricultural Marketing Service, n.d., 2022). For this reason, scaffolds, among other reagents, and production methods for cultivation of cell-based meat must reach an exceptionally low cost. In this study, we demonstrated that plant-based scaffolds derived from agricultural waste can be used as affordable, edible scaffolds for cultured meat, and are usable as edible cell carriers for scale-up. Researchers’ efforts to yield cell masses for the many tissue constituents of meat—lean skeletal muscle (Ben-Arye et al., 2020; Stout et al., 2023), adipose tissue (Huang et al., 2023; Yuen Jr et al., 2023), or connective tissue (Pasitka et al., 2023; P; Wood et al., 2023)—consistently depend on these types of carrier scaffolds to satisfy the anchorage dependence of such cell types. While some efforts to acclimate traditionally anchorage dependent cells to suspension culture have been proposed for myoblast proliferation, adherence to stiff binding surfaces are still employed during differentiation phases. Given this current need for cell-binding scaffolds, an opportunity for the development of “hybrid-meat products”, which retain the scaffold upon which cells are expanded and differentiated is valuable and may offer an environmentally sustainable protein production method that limits the economic constraints of more complex bioproduction systems.
It is important to note that cellular agriculture presents a widely different use-case for traditional bioproduction strategies than those typically used for manufacturing pharmaceutical products. For example, single use bioreactors, which are widely employed for monoclonal antibody production, are advantageous for their low start-up investment, flexibility of use, and prevalidation. Such bioreactors are disadvantageous for cellular agriculture due to their inability to sustainably and repeatedly support frequent high-throughput cell expansion (Stephenson & Grayson, 2018; Allan et al., 2019). Similarly, a cell carrier that is to be used for supporting anchorage dependent cells for cellular agriculture applications may be justified by its advantageous qualities unique for cultured meat production. The characteristics of traditional cell carriers, such as supporting cell adhesion and cell viability over time are necessary for yielding the cells and cell products desired (Nienow et al., 2014); however, for cultured meat applications, economic qualities such as the affordability and availability of the cell carrier must be considered. Pharmaceutical bioprocesses seek to yield cell products, whereas a cultured meat bioprocess seeds to yield the cells themselves. Additionally, for cultured meat products that incorporate its scaffold as hybrid-meat products, the edibility and nutritional characteristics of the scaffold are important.
We have identified corn husk and jackfruit rind as two potential sources for affordable and readily available cell carriers for hybrid-meat products. The process outlined in this study yielded decellularized samples that support satellite cells from both bovine and avian sources (Figure 2). This study exemplifies the option for farmers to further commoditize corn husks or jackfruit rinds from what may have been treated as waste, to a vital input for cellular agriculture. Decellularization is a simple process that can be completed with common affordable reagents in many regions and improves the accessibility of lab grown meat production. Thus, future studies on decellularized plant scaffolds derived from additional, site-specific, agricultural waste products are justified.
Lab grown meat must offer substantial quantities of protein to meet expectations of an equitable and environmentally sustainable approach to food production. Thus, continuous evaluation of each lab grown meat product’s total protein content is vital for judging the adequacy of the product and the efficiency of the process by which it was prepared. We demonstrated via BCA assay that cellular adhesion and culture on decellularized plant tissue increases the total protein content of the product (Figure 6). However, continuous improvements designed to optimize the amino acid profile and maximize the total protein content per unit of product is justified. There are many additional factors that may contribute to the total protein content including seeding efficiency, growth density, cell-to-scaffold mass ratio, myocyte fusion index as a measurement for differentiation efficiency, media evaluation, and electrostimulation and cell hypertrophy.
To date, many bioengineering publications describing plant decellularization have focused on fruits and vegetables like apple, asparagus, spinach, parsley, or broccoli. These are selected based upon their morphological and compositional characteristics, to meet the requirements for the biomaterial scaffold for which they are used. For example, spinach leaves, possessing an extensive vasculature with a central vessel that is easily cannulated, can be employed for studies that require scaffold perfusion (Gershlak et al., 2017). Conversely, broccoli florets are lightweight and roughly spherical, and can be used as effective microcarriers in bioreactor culture (Thyden et al., 2022). Corn husk and jackfruit similarly present unique structural characteristics we believed would be beneficial for cultured meat production. Corn husk presents uniaxial striations following its vasculature that were observed, albeit not homogenously, to encourage myocyte alignment, following during culture (Figures 4C,D, 5C,D). Microscopically, corn husk trichomes can be observed, small fibrous hairs that extend from the plant surface. We observed cell binding to trichomes, which may increase available surface area for cell proliferation, rendering the material a strong candidate for cell expansion. Jackfruit is composed of multiple loose fibers. This texture makes it a staple in vegan alternatives to meat dishes like pulled chicken or pork. The loose, fibrous structure of jackfruit may have promoted increased cell integration with the scaffold, and contributed to the higher protein content we observed compared to cell-seeded corn husk (Figure 6A). In additional support of jackfruit, the plant has previously been identified, by AI, as the plant most likely to resemble the marbling characteristics of many cuts of meat (Ong et al., 2021).
In this study, we particularly highlight the stiffness of corn husk and jackfruit scaffolds, a critical factor for both cell growth and behavior on the scaffold, and for consumer considerations like texture and mouthfeel of the final meat product. We found that decellularized jackfruit had a considerably lower modulus of elasticity versus corn husk (Figure 3), supporting its candidacy as a viable scaffold for development of structured muscle tissue. Both scaffolds were an order of magnitude higher modulus of elasticity than native bovine muscle tissue (<20 kPa) (Lapin et al., 2013; L. K; Wood et al., 2014), which may be due to properties of cellulose in these tissues, as a dense fibrous polysaccharide (Galiwango et al., 2019; Hickey & Pelling, 2019). While this study did not investigate scaffold mechanics post-cell seeding, increasing cell content on these scaffolds, in addition to inclusion of fat tissue and connective tissue into engineered tissues will likely impact scaffold mechanics (Boots et al., 2021, p. 23; Fraeye et al., 2020; K; Handral et al., 2022; Schätzlein & Blaeser, 2022). It is also likely that long-term culture of cellulose scaffolds may induce some mechanical degradation (Märtson et al., 1999; Helenius et al., 2006; Modulevsky et al., 2014). Future work should target methods to reduce scaffold stiffness, and encourage cell incorporation of matrix proteins like collagen and elastin to promote improved meat texture and increase scaffold elasticity. Similarly, it is imperative to evaluate the texture and mechanics of engineered tissues as cell seeding is optimized and tissue density increases, to determine how closely these tissues recapitulate the feel of conventional meat.
Having demonstrated that agricultural waste can serve as an edible cell carrier for satellite cells, that cells exhibit bead-to-bead transfer upon them (Figure 7), and understanding that for theoretical hybrid products, the scaffolds are included in the final product, the context for unique bioprocess design can be established. For example, a traditional, non-continuous bioprocess (Figure 8A) could be improved upon by developing a closed, two bioreactor, continuous bioprocess which consists of a proliferation bioreactor for cell expansion on decellularized plant scaffolds, a sensor for detecting confluent scaffolds, a differentiation reactor to which confluent scaffolds are continuously passed, and a final sensor for detecting fully differentiated units of the final hybrid product (Figure 8B).
FIGURE 8. Schematics of batch processes for cultured meat scale up. (A) Conventional process requiring multiple systems for proliferation and differentiation steps. (B) Proposed continuous process using bead-to-bead transfer to reduce labor steps in cultured meat cultivation. Figure made in BioRender.
Bioprocesses for manufacturing cultured meat products must be designed for affordability. Decellularized plant scaffolds maintain many unique characteristics that are advantageous to cellular agriculture applications, such as their availability, simple preparation, and edibility. We have demonstrated the preparation of decellularized plant scaffolds derived from waste products, thus exemplifying affordable acquisition of a critical bioprocess component. We have demonstrated the variability in decellularized plants in regard to their stiffness, a key characteristic of traditional tissue engineering scaffolds. Additionally, we have noted the ability of cells to both contribute protein to decellularized plant scaffolds, as well as migrate between them. These results justify further investigation into methods for improving the affordability of the decellularization process, preparation of scaffolds from other agricultural waste products, establishment of methods for improving the cellular contribution of total protein, and designing novel bioreactors in consideration of access to decellularized plant-based scaffolds derived from agricultural waste. Ultimately, such efforts may improve the affordability of cultured meat bioprocesses and enable the realization of cellular agriculture.
The raw data supporting the conclusion of this article will be made available by the authors, without undue reservation.
Conceptualization GG, TD, LP, RT, and JJ; planning GG, TD, LP, RT, and JJ; data curation LP and RT; formal analysis LP and RT; funding acquisition GG, TD, and RT; project supervision GG, TD, LP, and RT; imaging LP and RT; histology RT; DNA quantification RT, LP, AP, and DR, decellularization and scaffold preparation LP, RT, AP, DR, JN, and JK; protein quantification LP and RT; writing LP and RT; editing LP, RT, AP, DR, JK, JJ, GG, and TD; Review LP, RT, AP, DR, JN, JK, JJ, TD, and GG. All authors contributed to the article and approved the submitted version.
Funding from Boston College research startup funds were used to support this research. This project was also supported in part by a New Harvest Fellowship awarded to RT.
We thank Bret Judson and the Boston College Imaging Core for their infrastructure and support. We would like to thank Sarah Barbrow and the Boston College Library for their support and advice. Lastly, we would like to thank Jyotsna Patel and the WPI histology core for their advice and support. Figures 1, 8 were created with BioRender.
The authors declare that the research was conducted in the absence of any commercial or financial relationships that could be construed as a potential conflict of interest.
All claims expressed in this article are solely those of the authors and do not necessarily represent those of their affiliated organizations, or those of the publisher, the editors and the reviewers. Any product that may be evaluated in this article, or claim that may be made by its manufacturer, is not guaranteed or endorsed by the publisher.
Allan, S. J., De Bank, P. A., and Ellis, M. J. (2019). Bioprocess design considerations for cultured meat production with a focus on the expansion bioreactor. Front. Sustain. Food Syst. 3, 44. doi:10.3389/fsufs.2019.00044
Andreassen, R. C., Rønning, S. B., Solberg, N. T., Grønlien, K. G., Kristoffersen, K. A., Høst, V., et al. (2022). Production of food-grade microcarriers based on by-products from the food industry to facilitate the expansion of bovine skeletal muscle satellite cells for cultured meat production. Biomaterials 286, 121602. doi:10.1016/j.biomaterials.2022.121602
Antin, P. B., and Ordahl, C. P. (1991). Isolation and characterization of an avian myogenic cell line. Dev. Biol. 143 (1), 111–121. doi:10.1016/0012-1606(91)90058-B
Araya-Chavarría, K., Rojas, R., Ramírez-Amador, K., Sulbarán-Rangel, B., Rojas, O., and Esquivel-Alfaro, M. (2022). Cellulose nanofibers as functional biomaterial from pineapple stubbles via TEMPO oxidation and mechanical process. Waste Biomass Valorization 13 (3), 1749–1758. doi:10.1007/s12649-021-01619-3
Bar-Shai, N., Sharabani-Yosef, O., Zollmann, M., Lesman, A., and Golberg, A. (2021). Seaweed cellulose scaffolds derived from green macroalgae for tissue engineering. Sci. Rep. 11 (1), 11843. doi:10.1038/s41598-021-90903-2
Ben-Arye, T., Shandalov, Y., Ben-Shaul, S., Landau, S., Zagury, Y., Ianovici, I., et al. (2020). Textured soy protein scaffolds enable the generation of three-dimensional bovine skeletal muscle tissue for cell-based meat. Nat. Food 1 (4), 210–220. doi:10.1038/s43016-020-0046-5
Bhat, Z. F., Bhat, H., and Pathak, V. (2014). “Chapter 79—prospects for in vitro cultured meat – a future harvest,” in Principles of tissue engineering. Editors R. Lanza, R. Langer, and J. Vacanti (Academic Press), 1663–1683. doi:10.1016/B978-0-12-398358-9.00079-3
Bodiou, V., Moutsatsou, P., and Post, M. J. (2020). Microcarriers for upscaling cultured meat production. Front. Nutr. 7, 10. doi:10.3389/fnut.2020.00010
Boots, J. N. M., Humblet-Hua, N. P. K., Tonneijck, L., Fokkink, R., van der Gucht, J., and Kodger, T. E. (2021). Characterization of the local mechanical texture of animal meat and meat replacements using multi-point indentation. J. Food Eng. 300, 110505. doi:10.1016/j.jfoodeng.2021.110505
Fraeye, I., Kratka, M., Vandenburgh, H., and Thorrez, L. (2020). Sensorial and nutritional aspects of cultured meat in comparison to traditional meat: Much to Be inferred. Front. Nutr. 7, 35. doi:10.3389/fnut.2020.00035
Galiwango, E., Abdel Rahman, N. S., Al-Marzouqi, A. H., Abu-Omar, M. M., and Khaleel, A. A. (2019). Isolation and characterization of cellulose and α-cellulose from date palm biomass waste. Heliyon 5 (12), e02937. doi:10.1016/j.heliyon.2019.e02937
Garrison, G. L., Biermacher, J. T., and Brorsen, B. W. (2022). How much will large-scale production of cell-cultured meat cost? J. Agric. Food Res. 10, 100358. doi:10.1016/j.jafr.2022.100358
Gershlak, J. R., Hernandez, S., Fontana, G., Perreault, L. R., Hansen, K. J., Larson, S. A., et al. (2017). Crossing kingdoms: Using decellularized plants as perfusable tissue engineering scaffolds. Biomaterials 125, 13–22. doi:10.1016/j.biomaterials.2017.02.011
Goodland, R. (1997). Environmental sustainability in agriculture: Diet matters. Ecol. Econ. 23 (3), 189–200. doi:10.1016/S0921-8009(97)00579-X
Handral, K. H., Hua Tay, S., Wan Chan, W., and Choudhury, D. (2022). 3D Printing of cultured meat products. Crit. Rev. Food Sci. Nutr. 62 (1), 272–281. doi:10.1080/10408398.2020.1815172
Harris, A. F., Lacombe, J., and Zenhausern, F. (2021). The emerging role of decellularized plant-based scaffolds as a new biomaterial. Int. J. Mol. Sci. 22 (22), 12347. doi:10.3390/ijms222212347
Helenius, G., Bäckdahl, H., Bodin, A., Nannmark, U., Gatenholm, P., and Risberg, B. (2006). In vivo biocompatibility of bacterial cellulose. J. Biomed. Mater. Res. Part A Official J. Soc. Biomaterials 76 (2), 431–438.
Hickey, R. J., and Pelling, A. E. (2019). Cellulose biomaterials for tissue engineering. Front. Bioeng. Biotechnol. 7, 45. doi:10.3389/fbioe.2019.00045
Holmes, D., Humbird, D., Dutkiewicz, J., Tejeda-Saldana, Y., Duffy, B., and Datar, I. (2022). Cultured meat needs a race to mission not a race to market. Nat. Food 3 (10), 785–787. doi:10.1038/s43016-022-00586-9
Huang, Z., Cheng, Y.-M., Hong, P.-C., Zhu, H.-N., Qin, J., Song, M.-M., et al. (2023). An immortal porcine preadipose cell strain for efficient production of cell-cultured fat. https://europepmc.org/article/ppr/ppr650380.
Jones, J. D., Rebello, A. S., and Gaudette, G. R. (2021). Decellularized spinach: An edible scaffold for laboratory-grown meat. Food Biosci. 41, 100986. doi:10.1016/j.fbio.2021.100986
Joo, S.-T., Choi, J.-S., Hur, S.-J., Kim, G.-D., Kim, C.-J., Lee, E.-Y., et al. (2022). A comparative study on the taste characteristics of satellite cell cultured meat derived from chicken and cattle muscles. Food Sci. Animal Resour. 42 (1), 175–185. doi:10.5851/kosfa.2021.e72
Knežić, T., Janjušević, L., Djisalov, M., Yodmuang, S., and Gadjanski, I. (2022). Using vertebrate stem and progenitor cells for cellular agriculture, state-of-the-art, challenges, and future perspectives. Biomolecules 12 (5), 699. doi:10.3390/biom12050699
Lapin, M. R., Gonzalez, J. M., and Johnson, S. E. (2013). Substrate elasticity affects bovine satellite cell activation kinetics in vitro. J. Animal Sci. 91 (5), 2083–2090. doi:10.2527/jas.2012-5732
Märtson, M., Viljanto, J., Hurme, T., Laippala, P., and Saukko, P. (1999). Is cellulose sponge degradable or stable as implantation material? An in vivo subcutaneous study in the rat. Biomaterials 20 (21), 1989–1995. doi:10.1016/s0142-9612(99)00094-0
Modulevsky, D. J., Cuerrier, C. M., and Pelling, A. E. (2016). Biocompatibility of subcutaneously implanted plant-derived cellulose biomaterials. PloS One 11 (6), e0157894. doi:10.1371/journal.pone.0157894
Modulevsky, D. J., Lefebvre, C., Haase, K., Al-Rekabi, Z., and Pelling, A. E. (2014). Apple derived cellulose scaffolds for 3D mammalian cell culture. PloS One 9 (5), e97835. doi:10.1371/journal.pone.0097835
Newman, L., Fraser, E., Newell, R., Bowness, E., Newman, K., and Glaros, A. (2023). “Chapter 1—cellular agriculture and the sustainable development goals,” in Genomics and the global bioeconomy. Editors C. Lopez-Correa, and A. Suarez-Gonzalez (Cambridge, MA, USA: Academic Press), 3–23. doi:10.1016/B978-0-323-91601-1.00010-9
Nienow, A. W., Rafiq, Q. A., Coopman, K., and Hewitt, C. J. (2014). A potentially scalable method for the harvesting of hMSCs from microcarriers. Biochem. Eng. J. 85, 79–88. doi:10.1016/j.bej.2014.02.005
Norris, A., van’t Hag, L., Su, C., Attenborough, E., Buckingham, E., Perry, O., et al. (2021). Processing jackfruit into ready-to-eat products and ingredients. NSW, Australia: AgriFutures Australia Wagga Wagga.
Ong, S., Loo, L., Pang, M., Tan, R., Teng, Y., Lou, X., et al. (2021). Decompartmentalisation as a simple color manipulation of plant-based marbling meat alternatives. Biomaterials 277, 121107. doi:10.1016/j.biomaterials.2021.121107
Pasitka, L., Cohen, M., Ehrlich, A., Gildor, B., Reuveni, E., Ayyash, M., et al. (2023). Spontaneous immortalization of chicken fibroblasts generates stable, high-yield cell lines for serum-free production of cultured meat. Nat. Food 4 (1), 35–50. doi:10.1038/s43016-022-00658-w
Pengelly, J. J. L., Kwasny, S., Bala, S., Evans, J. R., Voznesenskaya, E. V., Koteyeva, N. K., et al. (2011). Functional analysis of corn husk photosynthesis. Plant Physiol. 156 (2), 503–513. doi:10.1104/pp.111.176495
Raman, R. (2021). “Lab-grown meat and leather,” in Biofabrication (MIT Press), 83–102. https://ieeexplore.ieee.org/document/9592237.
Sans, P., and Combris, P. (2015). World meat consumption patterns: An overview of the last fifty years (1961–2011). Meat Sci. 109, 106–111. doi:10.1016/j.meatsci.2015.05.012
Santolini, E., Bovo, M., Barbaresi, A., Torreggiani, D., and Tassinari, P. (2021). Turning agricultural wastes into biomaterials: Assessing the sustainability of scenarios of circular valorization of corn cob in a life-cycle perspective. Appl. Sci. 11 (14), 6281. doi:10.3390/app11146281
Schätzlein, E., and Blaeser, A. (2022). Recent trends in bioartificial muscle engineering and their applications in cultured meat, biorobotic systems and biohybrid implants. Commun. Biol. 5 (1), 737. doi:10.1038/s42003-022-03593-5
Stephens, N., Di Silvio, L., Dunsford, I., Ellis, M., Glencross, A., and Sexton, A. (2018). Bringing cultured meat to market: Technical, socio-political, and regulatory challenges in cellular agriculture. Trends Food Sci. Technol. 78, 155–166. doi:10.1016/j.tifs.2018.04.010
Stephenson, M., and Grayson, W. (2018). Recent advances in bioreactors for cell-based therapies. F1000Res. 7. doi:10.12688/f1000research.12533.1
Stout, A. J., Zhang, X., Letcher, S. M., Rittenberg, M. L., Shub, M., Chai, K. M., et al. (2023). Engineered autocrine signaling eliminates muscle cell FGF2 requirements for cultured meat production. BioRxiv 23. doi:10.1101/2023.04.17.537163
Thyden, R., Perreault, L. R., Jones, J. D., Notman, H., Varieur, B. M., Patmanidis, A. A., et al. (2022). An edible, decellularized plant derived cell carrier for lab grown meat. Appl. Sci. 12 (10), 5155. doi:10.3390/app12105155
Tuomisto, H. T. de M., and Joost, M. (2011). Environmental impacts of cultured meat production. Environ. Sci. Technol. 45. doi:10.1021/es200130u
U.S. Department Of Agriculture, (2022). Daily beef reports agricultural marketing Service. https://www.ams.usda.gov/market-news/daily-beef-reports.
Verbruggen, S., Luining, D., van Essen, A., and Post, M. J. (2018). Bovine myoblast cell production in a microcarriers-based system. Cytotechnology 70 (2), 503–512. doi:10.1007/s10616-017-0101-8
Wang, Y., and Ouyang, F. (1999). Bead-to-bead transfer of Vero cells in microcarrier culture. Bioprocess Eng. 21 (3), 221–224. doi:10.1023/A:1008079013375
Wood, L. K., Kayupov, E., Gumucio, J. P., Mendias, C. L., Claflin, D. R., and Brooks, S. V. (2014). Intrinsic stiffness of extracellular matrix increases with age in skeletal muscles of mice. J. Appl. Physiology Bethesda, Md 117 (4), 363–369. doi:10.1152/japplphysiol.00256.2014
Wood, P., Thorrez, L., Hocquette, J.-F., Troy, D., and Gagaoua, M. (2023). Cellular agriculture: Current gaps between facts and claims regarding cell-based meat. Anim. Front. 13 (2), 68–74. doi:10.1093/af/vfac092
Xu, C., Nasrollahzadeh, M., Selva, M., Issaabadi, Z., and Luque, R. (2019). Waste-to-wealth: Biowaste valorization into valuable bio (nano) materials. Chem. Soc. Rev. 48 (18), 4791–4822. doi:10.1039/c8cs00543e
Keywords: cellular-agriculture, edible scaffolds, decellularization, agriculture waste, cultured meat, corn-husk, jackfruit, bead-to-bead-transfer
Citation: Perreault LR, Thyden R, Kloster J, Jones JD, Nunes J, Patmanidis AA, Reddig D, Dominko T and Gaudette GR (2023) Repurposing agricultural waste as low-cost cultured meat scaffolds. Front. Food. Sci. Technol. 3:1208298. doi: 10.3389/frfst.2023.1208298
Received: 18 April 2023; Accepted: 01 June 2023;
Published: 12 June 2023.
Edited by:
Maria G. Corradini, University of Guelph, CanadaReviewed by:
Shiv Dutt Purohit, Yeungnam University, Republic of KoreaCopyright © 2023 Perreault, Thyden, Kloster, Jones, Nunes, Patmanidis, Reddig, Dominko and Gaudette. This is an open-access article distributed under the terms of the Creative Commons Attribution License (CC BY). The use, distribution or reproduction in other forums is permitted, provided the original author(s) and the copyright owner(s) are credited and that the original publication in this journal is cited, in accordance with accepted academic practice. No use, distribution or reproduction is permitted which does not comply with these terms.
*Correspondence: Glenn R. Gaudette, Z2F1ZGV0dGdAYmMuZWR1
†These authors have contributed equally to this work and share first authorship
Disclaimer: All claims expressed in this article are solely those of the authors and do not necessarily represent those of their affiliated organizations, or those of the publisher, the editors and the reviewers. Any product that may be evaluated in this article or claim that may be made by its manufacturer is not guaranteed or endorsed by the publisher.
Research integrity at Frontiers
Learn more about the work of our research integrity team to safeguard the quality of each article we publish.