- 1Department of Chemistry, Biotechnology, Lund University, Lund, Sweden
- 2Department of Food Technology, Engineering and Nutrition, Lund University, Lund, Sweden
Introducing seaweed to new food markets entails new challenges concerning efficient preservation. Hence, this study explores high-pressure processing (HPP) as an alternative technique to conventional methods by evaluating its effects on the composition, quality, and microbial safety of the Swedish grown macroalgae Saccharina latissima. The results from the physicochemical analysis showed that after high-pressure treatment the color was retained, while the algal texture was altered by up to an 87.7% reduction in hardness and a 60.0% reduction in compression. Biochemical analysis demonstrated some variations in the algal samples, but the nutritional content was overall retained after treatment. The microbial analysis showed a low microbial load of untreated fresh material, which was confirmed by a lack of amplification in polymerase chain reaction attempts and low growth during attempts on spontaneous proliferation using fresh and frozen algae. Additionally, shelf-life studies showed inconsistent growth, but overall, a low increase in unspecific bacteria, an increasing load of Enterobacteriaceae, no growth of Lactobacilli, and low fouling by mold and yeast. The results from this study can be useful in the continued attempts of introducing seaweed to new markets, with different prerequisites for post-harvest treatment.
1 Introduction
Marine macroalgae, seaweed, has received increased attention in recent years for its potential as a healthy future food in emerging markets to feed an increasing global population (McNevin et al., 2020; Cai et al., 2021). Whereas Eastern and South-East Asia dominate the global market (97% of world production in 2019), production volumes are also increasing in other parts of the world, with Europe accounting for 0.8% of global production in 2019. Although world production has increased steadily since the 1950s, it is seemingly a long process for seaweed to be introduced as a major source of food in new markets (Cai et al., 2021).
As part of the process of introducing seaweed to new markets, evaluation of its safety and shelf-life are important steps. The need to preserve seaweed arises from the high water content and water activity of seaweed (typically around 70%–90% and 0.97–0.98, respectively) which favors the growth of microorganisms (Silva et al., 2008; Albers et al., 2021). Lytou et al. (2021) found that the number of colonizing microorganisms varied greatly between harvesting years and species (ranging from close to the enumeration limit, 1.0 log CFU/g, to 6.7 log CFU/g), and that a variety of microbial species associated with different seaweed species was observed. While Scottish Alaria esculenta was colonized by Psychrobacter, Cobetia, and Pseudomonas species (all Gammaproteobacteria), Saccharina latissima was populated by Psychrobacter and Micrococcus (Actinobacteria) species. In a review by Hollants et al. (2013), the most common spoilage and pathogenic bacteria associated with seaweed were reported to include: Gammaproteobacteria (37%, percentage of published records), Bacteroidetes (20%), Alphaproteobacteria (13%), Firmicutes (10%), and Actinobacteria (9%). Prevention of the overgrowth of spoilage bacteria and potential pathogens on seaweed material is considered a substantial challenge, and preservation is, therefore, necessary for seaweed to be more widely used as a food source (Blikra et al., 2021).
Several preservation methods have been employed traditionally to increase the shelf-life of seaweed. These methods include sun-drying, oven-trying, freeze-drying, freezing, salting, and fermenting/ensiling. Different methods of preservation influence the biochemical composition and physicochemical attributes of the seaweed biomass differently, suggesting a need to investigate the impact of post-harvest processing. Although sun-drying is the least energy-consuming and least costly, its weather dependency makes it less appropriate for a Nordic climate (Badmus et al., 2019; Albers et al., 2021). Oven-drying and freeze-drying are both rather energy-consuming during the event of dehydration, whilst the freezing process consumes energy throughout the whole shelf-life period. Alternative methods to these traditional preservation techniques are therefore sought to increase profitability in the seaweed industry.
High-pressure processing (HPP) is a technique for the preservation of various fresh, refrigerated food products. The process is operated by inserting the product into the pressure vessel, which is filled with a pressure-transmitting medium (such as re-circulating water) to reach the desired pressure level for a predetermined hold time before the system is again depressurized, and the product is discharged. The pressure is transmitted in an isocratic manner so that the whole sample is subjected to a uniform force. The process is run batchwise for solid products. HPP’s antimicrobial action lies in altering cell morphology, biochemical reactions, and denaturing key enzymes. One advantage of HPP is that the process is non-thermal, and many of the desired nutritional and sensory properties of the product are maintained after treatment. This may be explained by its limited effects on covalent bonds. HPP is currently used on solid, semi-solid, and liquid products, including jams, dairy products, salads, fruit and vegetable sauces, juices, and smoothies, as well as mashes such as hummus and guacamole (Oey et al., 2008; Tao et al., 2014).
Current experience of HPP treatment on fresh seaweed has, to the knowledge of the authors, thus far been limited to the species Codium fragile, Ulva lactuca, Himanthalia elongata, Laminaria ochroleuca, Undaria pinnatifida, and Chondrus crispus collected in Spain (del Olmo et al., 2019; del Olmo et al., 2020; López-Pérez et al., 2020; Ekonomou and Boziaris, 2021; Picon et al., 2021) and Durvillaea antarctica harvested in Chile (Mateluna et al., 2020). A recent review by Løvdal et al. (2021) covering microbial food safety of seaweeds mentions HPP as a more established method among emerging trends for preservation of seaweeds. However, to date, little knowledge about seaweed shelf-life and microbial quality is available (Lytou et al., 2021).
To investigate HPP as an alternative method to conventional preservation techniques, this study evaluates, for the first time, the effects of the treatment on composition, quality, and microbial safety of S. latissima cultivated in Swedish waters.
2 Materials and methods
Saccharina latissima (L.) C.E. Lane, C. Mayes, Druehl, and G.W. Saunders, 2006, was harvested from cultivations in the Swedish National Park, Kosterhavet (N58.863, E11.071), on 12 April 2021, by the seaweed farmers at Nordic Sea Farm. The collected seaweed was transported under cooled conditions to the laboratory in Lund, Sweden, immediately after harvest. At the laboratory, the seaweed was stored in a cold room at 4°C overnight.
A flowchart of the process from harvest to treatment and analysis is depicted in Figure 1 to clarify the process steps and timelines.
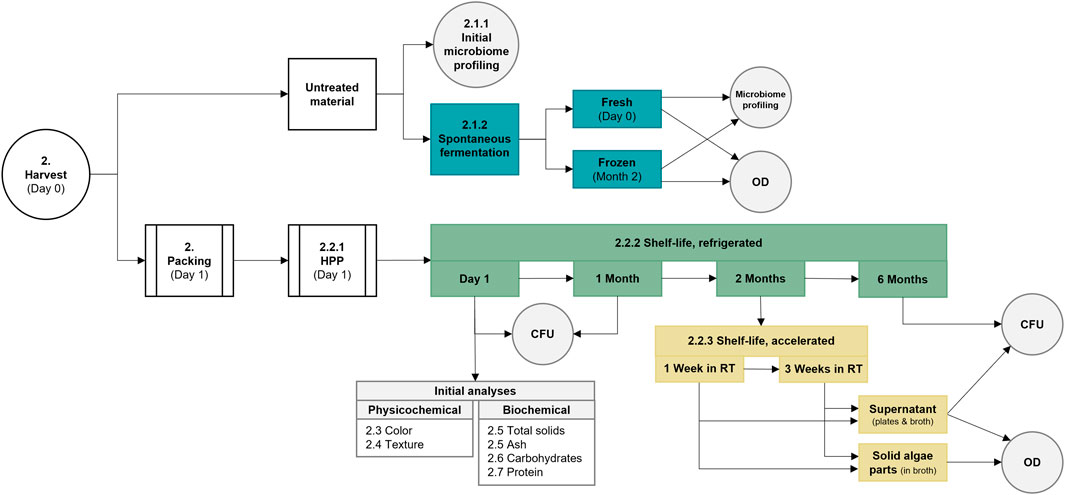
FIGURE 1. Flowchart covering processes from harvest to treatment and analyses. Various parts of the study are colored differently, with white indicating general processes, gray meaning analyses, blue representing the spontaneous fermentation, and green and yellow covering the refrigerated or accelerated shelf-life studies. CFU, colony forming units; HPP, high-pressure processing; OD, optical density; and RT, room temperature.
2.1 Microbial analyses of untreated material
2.1.1 Initial microbial profiling from Saccharina latissima biomass
Isolation of microbial DNA and PCR amplification from seaweed samples was done immediately after harvest to identify the indigenous microbial colonization of S. latissima cultivated on the Swedish west coast. Samples (n = 3) were frozen on the day of harvest and sent in dry ice to the external sequencing lab (Eurofins Genomics, Munich). Any potential microbial DNA was isolated, by overnight digestion of the seaweed biomass with proteinase K, to prepare for PCR amplification of the hypervariable regions in the bacterial 16S rRNA gene (V1-V3) and the fungal internal transcribed spacer gene (ITS1). Forward and reverse primers with sequences 5′-AGAGTTTGATCATGGCTCAG-3’ (Weisburg et al., 1991) or 5′-GTATTACCGCGGCTGCTG-3′ (Leser et al., 2002) were employed for the 16S V1-V3 regions, whereas corresponding sequences 5′-GGAAGTAAAAGTCGTAACAAGG-3′ or 5′-GCTGCGTTCTTCATCGATGC-3′ (White et al., 1990) were used for ITS1 regions.
2.1.2 Spontaneous fermentation of fresh and frozen seaweed
Proliferation trials of indigenous microbial species were performed to evaluate the microbial colonization level on seaweed and if a freezing procedure influences the microbial population on the seaweed. Freezing may be a necessary step in further analyses or in case fresh seaweed would not be available in complex industrial supply chains. Samples (n = 3) of 80 g fresh or frozen pieces of seaweed (100–200 cm2) were mixed with 420 mL Milli-Q water in 500 mL bottles, which were kept at a constant temperature of 37°C for 7 days, and monitored for pH change and gas production in a Gas Endeavour system (Bioprocess Control, Sweden) throughout the course of the investigation. The gas volume was normalized by the software implying that the pressure, temperature, and saturated moisture content were compensated for (1.0 standard atmospheric pressure, 0°C, and zero moisture content) based on the values of pressure and temperature registered by the Gas Endeavour measuring device. Optical density (OD620nm) was measured daily after samples were filtered with a 40 µm cell strainer to remove larger biomass particles. Fresh samples were studied immediately after harvesting, and frozen samples were stored at −20°C for 2 months prior to the investigation.
Samples of liquid supernatant from the fermentation rectors harvested at the final time point were sent to the external accredited sequencing laboratory (Eurofins Genomics, Munich) for microbiome profiling. Sample preparation, analysis, and data processing were performed by the contractor lab. Target regions from the extracted DNA included hypervariable regions in the bacterial 16S rRNA gene (V1-V3) and the fungal internal transcribed spacer gene (ITS1). The same primers as for analysis of microbial DNA from seaweed biomass were used (Section 2.2.1).
2.2 Microbial analysis after HPP treatment
2.2.1 High-pressure processing
One day after harvest (about 18 h after harvest) aliquots of 10 ± 0.1 g fresh seaweed were added to PA/PE bags, and thereafter vacuum-packed and treated by HPP. Large seaweed pieces of around 100–200 cm2 were used to minimize the handling of the raw material and therefore minimize the contamination risk.
Processing of the seaweed material was performed 24 h after harvesting in an Avure AV-10 (Avure Technologies, JBT, United States) high-pressure equipment. Pressures of 200, 400, and 600 MPa were applied, and the hold time was set to 180 s (Table 1). Untreated material, packed in PA/PE vacuum bags, was also transported to the HPP facilities, but was not subjected to processing.
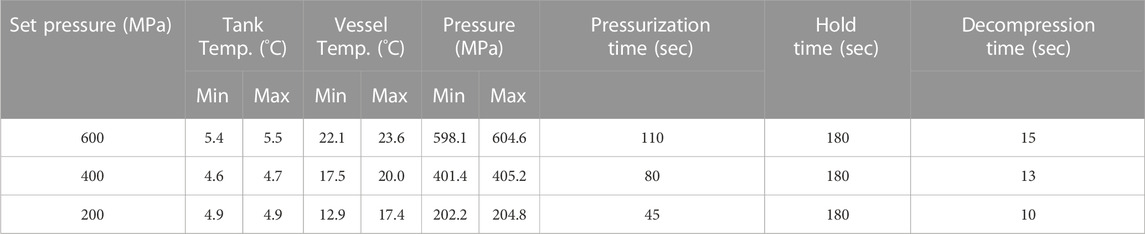
TABLE 1. Processing parameters from the high-pressure treatment of Saccharina latissima. Tank temperature refers to incoming process water whereas vessel temperature can be equated to product temperature.
After returning to the laboratory from the HPP facilities (26 h after harvest), the sealed bags with treated and untreated material were stored refrigerated at 4°C until the day of analysis.
2.2.2 Shelf-life of refrigerated samples
A shelf-life study was performed to evaluate the effectiveness of the HPP method for preservation of the S. latissima seaweed. Vacuum packed samples, subjected to HPP treatment according to Section 2.2.1, were stored under refrigerated conditions and analyzed after 0, 1, and 6 months.
Vacuum bags from HPP treatment containing aliquots of 10.0 ± 0.1 g (n = 2) randomly assembled pieces of S. latissima were opened and filled with 90 mL of sterile 0.1% peptone salt solution (Oxoid; Sigma Aldrich), and homogenized in a Seward BA6021 stomacher (Seward, United Kingdom) for 3 min at room temperature. Bags were prepared in duplicates for each processing parameter (200, 400, 600 MPa, and untreated) for the refrigerated shelf-life study. A dilution series was made by mixing the homogenate from the bags with sterile 0.1% peptone salt solution, and 0.1 mL of respective concentration was surface plated in duplicates onto the four types of agar plates. De Man, Rogosa and Sharpe (MRS; Sigma Aldrich) agar incubated at 37°C for 3 days was used for identification and quantification of gram-positive Lactobacillus species. Tryptic Soy Agar (TSA; Sigma Aldrich) incubated at 37°C for 18–48 h was used as a nonselective medium for growth of bacteria in general. Violet Red Bile Dextrose (VRBD; Sigma Aldrich) agar incubated at 30°C for 18–24 h was used for the detection and enumeration of gram-negative Enterobacteriaceae. Finally, malt agar (Sigma Aldrich) incubated at 30°C for 48–72 h was used for identification and quantification of yeast and molds.
2.2.3 Accelerated shelf-life
To evaluate the effect of elevated temperature (accelerated study) on the shelf-life of the seaweed material, samples were stored for 1 or 3 weeks at room temperature, directly after 2 months in a fridge. The preservation efficiency was evaluated by colony counting on agar plates (as in Section 2.2.2), and in liquid media. Samples were prepared as in Section 2.2.2, but in singlets instead of duplicates.
To also evaluate the distribution of bacteria in the supernatant associated with the solid seaweed parts after sample preparation, cultivation was performed in liquid media. Supernatant consisted of the peptone salt solution collected from the vacuum bags after sample preparation, and the solid fraction consisted of pieces of seaweed from the same bags. Five different liquid media were prepared for the analysis. MRS broth (Sigma Aldrich) incubated at 37°C for 64–68 h was used for identification and quantification of gram-positive Lactobacillus species. Tryptic Soy Broth (TSB; Sigma Aldrich) incubated at 37°C for 20–24 h was used as a nonselective medium for growth of bacteria in general. Enterobacteriaceae Enrichment Broth-Mossel (EE Broth Mossel; Sigma Aldrich) incubated at 37°C for 20–24 h was used for the detection and quantification of gram-negative Enterobacteriaceae. Malt broth (Sigma Aldrich) incubated at 30°C for 64–68 h was used for identification and quantification of yeast and molds. Finally, marine broth (Sigma Aldrich) incubated at 25°C for 64–68 h was used for detection and quantification of heterotrophic marine bacteria.
To evaluate the microbial content in the liquid phase after sample preparation, 0.5 mL of supernatant was added to 10 mL of liquid broth (n = 3). After incubation, the OD was measured at a wavelength of 620 nm. The microbial association to the solid part was evaluated by adding 1.00 ± 0.05 g of seaweed to 5 mL of liquid broth (n = 3). Solid material in 200 and 600 MPa samples stored 3 weeks at room temperature was completely dissolved during sample preparation, and therefore instead 0.5 mL of the unfiltered solution (n = 3) was added to 10 mL of media for these two samples. Before reading of the OD values at 620 nm, the samples were filtered through a 40 µm PluriSelect strainer to remove potential seaweed particles.
2.3 Color
Color analysis was performed using a portable spectrophotometer CM-700d (Konica Minolta, Japan) at random sites (n = 10) of the seaweed frond. One sample portion was measured directly without any means of drying from the bag (unwashed) and another fragment was rinsed with a total of 500 mL distilled water (washed). The spectrophotometer was set to measure specular component excluded (SCE) 10°/D65 and generated a spectrum between 400 and 700 nm as well as each sample’s L*, a* and b* values. The L*a*b* values compose a measure of the color space where L* indicated lightness while a* and b* specify color directions: +a* is the red direction, −a* is the green direction, +b* is the yellow direction, and −b* is the blue direction. The color data software CM-S100w SpectraMagic™ NX was used to obtain the data.
2.4 Texture
The texture of the high-pressure treated and untreated seaweed material was assessed using a TVT 300XP texture analyzer (Perten Instruments, Sweden) by measuring the hardness [force (g)] and compression [distance to peak (mm)] while penetrating a piece of seaweed. A spherical probe with 5 mm diameter was used for disrupting the seaweed which was fixed between two inserts with hole sizes of 10 mm and 18 mm, respectively. Random sites (n = 6) of the frond were selected for the analysis. The TVT software was used for obtaining and exporting the data to a spreadsheet.
2.5 Total solids and ash
Water content analysis of the fresh seaweed was based on the method for sample preparation from US National Renewable Energy Laboratory (Hames et al., 2008) and was determined by weighing bags or tubes containing seaweed samples before and after lyophilization. Single bags or tubes were used.
Total solids and ash were determined based on the US National Renewable Energy Laboratory method (Van Wychen and Laurens, 2015). Total solid values were used to mathematically correct for the amount of moisture present in the samples which are reported as per dry weight (DW; also commonly known as oven dry weight (ODW)). Crucibles were preconditioned in a furnace (Nabertherm Controller B180, Germany) at 575°C ± 25°C overnight to remove potential combustible contaminants. Thereafter, 100 ± 5 mg of lyophilized milled and sieved (mesh 2 mm) seaweed powders (n = 3) were added to the pre-weighted crucibles. The samples were placed in an oven (Fratelli Galli Control AG-System, Italy) at 105°C for at least 24 h. The crucible weights obtained were used to calculate the total solids of the seaweed samples. The same samples were placed in a furnace to determine the ash content of the seaweed biomass. A ramping program was used: the temperature was ramped from ambient temperature to 575°C for 30 min, held at 575°C for 3 h, and eventually allowed to cool down to ambient temperature again. Crucibles were once again weighted, and the obtained values were used to calculate the ash content of the seaweed samples.
2.6 Carbohydrates including neutral monosaccharides, mannitol, and uronic acids
Neutral monosaccharides, mannitol and uronic acids were analyzed based on the method of Van Wychen and Laurens (Van Wychen and Laurens, 2015) for determination of total carbohydrates in seaweed biomass. 25 mg freeze-dried seaweed powder (n = 2) was added to glass tubes with a screw lid and mixed with 250 µL of 72% (w/w) sulfuric acid (Alfa Aesar). The samples were incubated at 30°C at 300 rpm for 60 min, before being diluted to 4% (w/w) H2SO4 by addition of Milli-Q water. Thereafter the samples were heat treated by autoclavation at 121°C for 60 min, and centrifuged at 3,000 × g for 5 min to separate the supernatant from the solids. Sample preparation was finalized by neutralization with 0.1 M Ba(OH)2·H2O and filtration into HPLC vials using 0.2 µm syringe filters.
A High-Performance Anion Exchange Chromatography system equipped with Pulsed Amperometric Detector (HPAEC-PAD; Thermo Fisher Scientific, United States) and an analytical column and guard column (Dionex CarboPac PA-20; Thermo Fisher Scientific, Waltham, United States) was used to analyze the total carbohydrates in the seaweed samples. The analyses were run under isocratic conditions, with a flow rate of 0.5 mL/min at a temperature of 30°C. Elution of monosaccharides and mannitol was performed by using elusion mixtures A) Milli-Q water (62.5%), B) 2 mM sodium hydroxide (37.5%), and (C) 200 mM sodium hydroxide for 23 min. Uronic acids were eluted with the elution mixtures A) Milli-Q water (55%), B) 1 M sodium acetate in 200 mM sodium hydroxide (15%), and C) 200 mM sodium hydroxide (30%) for 15 min. L(−)fucose, D(+)glucose, D(+)xylose, D(+)galactose, L(+)arabinose, D(+)mannose and D-mannitol, or mannuronic acid, guluronic acid, and glucuronic acid were used as references.
2.7 Protein
Determination of protein content was based on the Dumas method by analyzing nitrogen content in seaweed samples using a N/Protein Analyzer Flash EA 1112 Series (Thermo Electron Corp., Waltham, MA, United States) coupled to the data handling software EagerSmart. About 25 mg of freeze-dried sample (n = 3) was weighted into aluminum containers for the analysis. Aspartic acid with known protein content was used as reference. Samples were combusted in a high-temperature (800°C–1,000°C) chamber in presence of oxygen to form carbon dioxide, water, and nitrogen. By-products were thereafter captured or separated in a column, and the nitrogen content was determined by a thermal conductivity detector. A nitrogen-to-protein conversion factor of 5 (Angell et al., 2016) was used to estimate the protein content.
2.8 Statistical analysis
Descriptive statistics was performed in Excel and Jamovi (The-jamovi-project, 2022), and all measurements are given as mean ± standard deviation. One-way analysis of variance (ANOVA) was performed with a significant level of 0.05 in Jamovi to investigate significant differences of the chemical and physicochemical properties of the samples. Two-way ANOVA (α = 0.05) was performed in Jamovi to distinguish potential differences between unwashed and washed samples for color analysis. Tukey’s post hoc test was used to determine which sample groups differed, if any.
3 Results
3.1 Microbial analysis of untreated seaweed
To evaluate the microbial load of freshly harvested seaweed, a series of microbial analyses was performed. Microbial profiling was first attempted by polymerase chain reaction (PCR) analysis on genetic material extracted immediately after harvest from Swedish S. latissima. Amplification by PCR did not, however, generate amplification products, neither using primers based on V1-V3 of 16S rDNA, nor from primers amplifying the ITS1 from fungal 18S region, indicative of very low colonization degree in the fresh seaweeds.
Further analysis thus focused on proliferation of indigenous microbiota by fermentation, to reach detectable levels, followed by monitoring optical density (OD620), pH, and gas production. Analysis of cell proliferation by OD620 showed in general more growth using fresh seaweed compared to frozen material, indicating that the freezing of the seaweed could reduce the microbial load. The OD values of fresh samples on average increased from 0.09 ± 0.0 to 1.29 ± 0.0 throughout the proliferation trials, whereas for frozen samples the OD increased from 0.13 ± 0.0 initially to 0.52 ± 0.3 at the end of analysis (Figures 2A, B). Fresh and frozen samples differed significantly (p < 0.05) regarding OD.
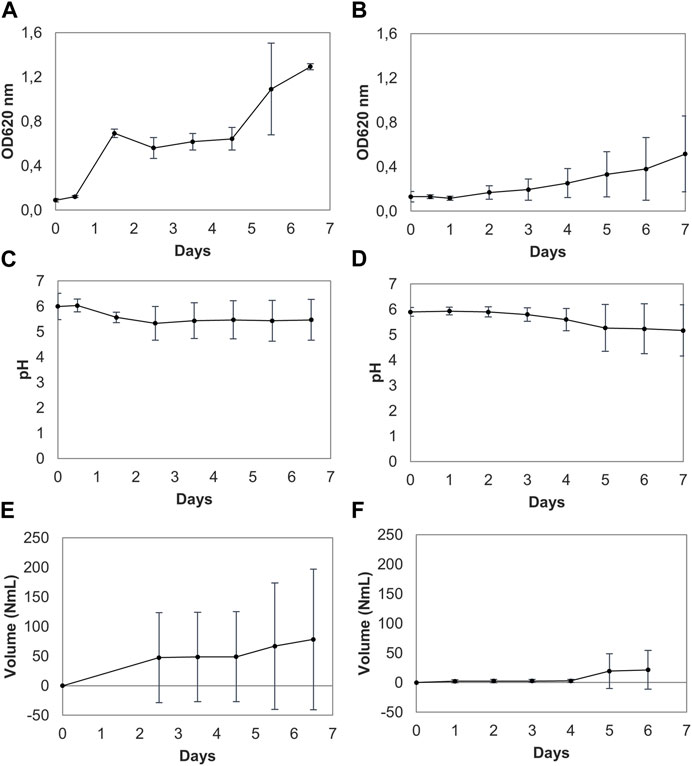
FIGURE 2. Optical density (OD), pH, and gas volume measurements of three parallel trials of spontaneously fermented fresh (A, C, E) and frozen seaweed (B, D, F). Results are presented as means with standard deviation error bars. The dotted lines in (E) form an extrapolation of the gas production since the tubing for the gas production measurements was not connected until 60 h after the start of the study; in connection with this, gas formation in one sample was observed when withdrawing an aliquot for OD measurements. Some volume of gas leaked from the system at this point.
The pH change and gas production differed slightly between the fresh and frozen samples, but not to a significant degree (p > 0.05). Moreover, the pH decrease was also shown to vary between the replicates, as seen in the trial using fresh seaweed (Figure 2C). Online pH measurements showed a low decrease of the pH throughout the study, with limited production of acids, except in one sample in the trial using frozen seaweed (Figure 2D). This makes it likely that different microbial species are proliferated in different samples (Figure 2). Large pieces of seaweed were placed in the reactor flasks to minimize contamination from handling, and might not therefore constitute a homogenous representation of the whole seaweed body. Although different pieces of seaweed were compiled randomly from the whole collected biomass, the microbial colonization may not have been homogenously distributed. On average, the pH decreased from 6.0 ± 0.5 to 5.5 ± 0.8 for fresh seaweed, and decreased from 5.9 ± 0.2 initially to 5.2 ± 1.0 for frozen seaweed.
In line with the variation in pH observed, gas production also varied between the sample replicates in each trial. Figures 2E, F illustrates the normalized volume of produced gas from the two trials, where the dotted lines represent extrapolations. On average, gas formation increased from 0 NmL to 78.3 ± 120 NmL for fresh seaweed throughout the trial, and from initial 0 NmL to final 21.5 ± 33 NmL using frozen seaweed for the trial. For both materials, it can be concluded that there is an increase in growth (Figures 2A, B) combined with an increase in gas production (Figures 2E, F) at around 100 h of elapsed time from the start of the trial concerned.
Microbial profiling was performed using supernatant end time samples from the spontaneous fermentation of fresh and frozen biomaterial. A library could only be generated for the V1-V3 regions of DNA extracted from one fresh and one frozen sample. Table 2 demonstrates that a variety of species in the genera Bacillus, Pseudomonas, Clostridium, Amnipila, and Lacrimispora were isolated from the fresh seaweed, whereas only a few species in the genus Bacillus were found in frozen seaweed. This indicates that the freezing procedure removed a majority of the microorganisms identified in this study.
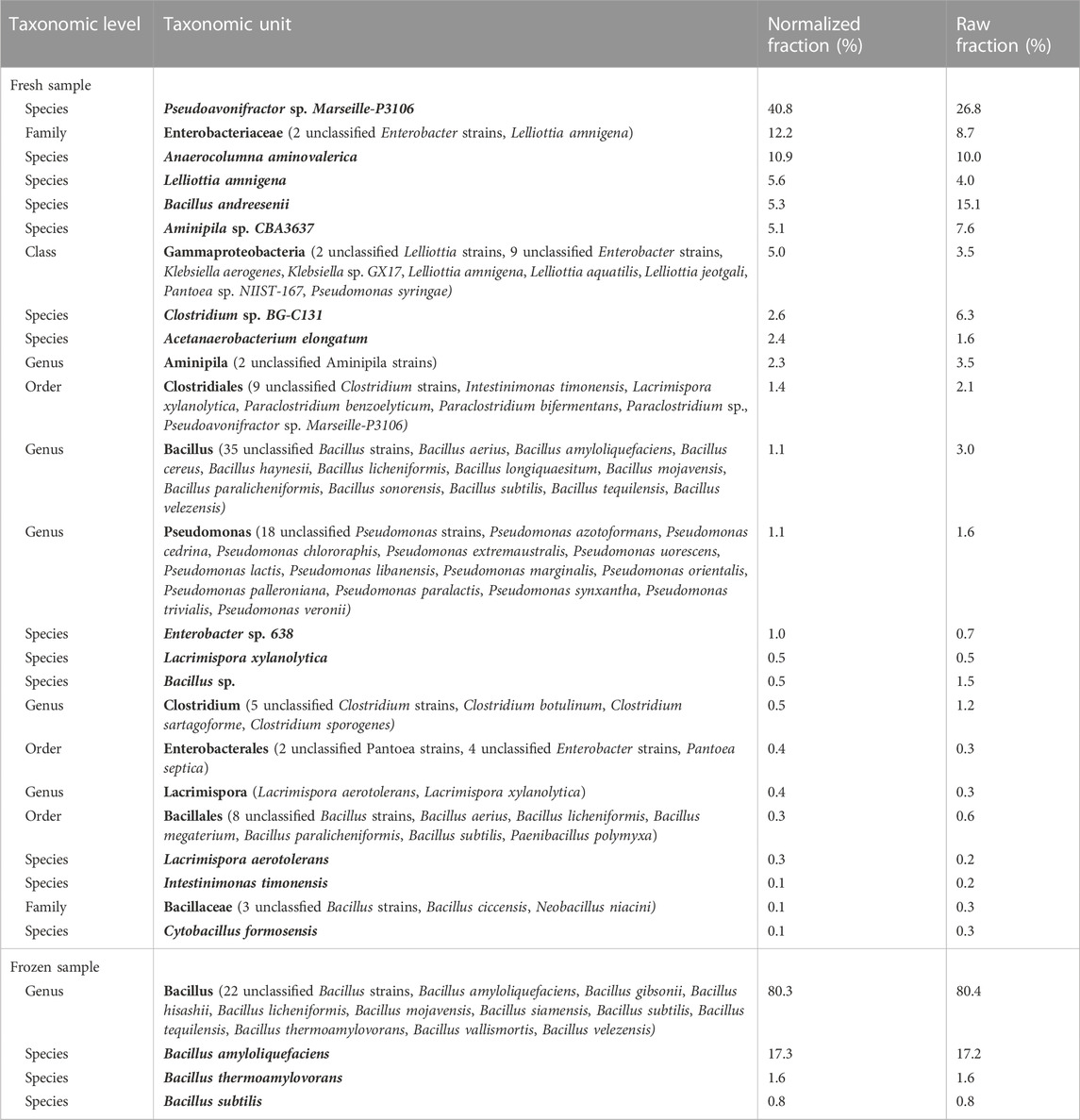
TABLE 2. Overview of the taxonomic composition of the samples. Normalization was conducted to correct for gene copy number (GCN) biases of the 16S rRNA gene according to Angly et al. (2014). It was not possible to generate a library for target region ITS1 for any of the samples, as well as for target region V1-V3 for two out of three replicates of fresh respectively frozen samples.
3.2 Microbial analysis of HPP treated material
High pressure processing was conducted on fresh seaweed samples to study the shelf-life after processing at three selected pressures (200, 400, and 600 MPa). Vacuum-packed samples, subjected to HPP treatment according to Section 2.2.1, were stored under refrigerated conditions and analyzed after 0, 1, and 6 months. An accelerated shelf-life study was also performed to investigate the microbial load after storage for up to 3 weeks at room temperature directly following 2 months in the fridge.
3.2.1 Microbial load analysis of refrigerated shelf-life samples
The vacuum-packed samples were stored refrigerated for up to 6 months and analyzed for microbial load by counting of colony forming units (CFU), using four types of media, on three occasions: initially (day 1), after 1 month and after 6 months from harvest (Table 3). Seaweed samples stored for 6 months were completely degraded after treatment by the stomacher, indicating microbial activity. Summarizing the results from the colony counting, it can be concluded that the main growth was observed in TSA and VRBD media, although no growth on VRBD plates was observed initially. No growth was observed on MRS plates throughout the whole study period, and malt plates were only occasionally covered by growth. This indicate that the samples were not colonized by gram-positive Lactobacillus species, whereas yeast or mold likely only occurred on parts of the seaweed body. Gram-negative Enterobacteriaceae occurred in countable numbers on later analysis occasions, indicating slow growth rate, which might be due to initially unavailable substrates. However, this bacterial family is recognized as being non-spore-forming (D’Agostino e al., 2016). Most of the identified microbes were, however, growing in the nonselective TSA medium, giving little idea of the bacterial family.
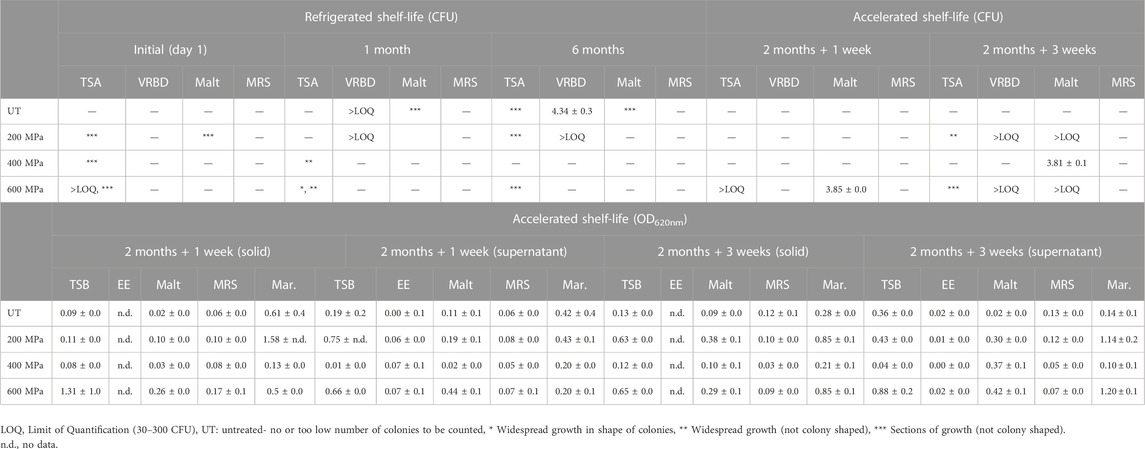
TABLE 3. Summary of microbial analysis from the refrigerated shelf-life study at time occasions, initially (day 1), 1 month and 6 months, as well as accelerated shelf-life study at time occasions, 1 week and 3 weeks in room temperature after 2 months of storage in refrigerated conditions. Microbial analyses include colony forming units (CFU) as well as optical density (OD620nm) of solid and supernatant fractions. The results from the CFU analysis (log10 CFU/mL) and the OD measurements are reported as averages values ± standard deviation. Apart from the CFU results presented in the table below (undiluted stock samples), considerable growth was observed for diluted samples on TSA initially (day 1) in the refrigerated study (replicate-pressure-dilution): 4-UT-102 (*** sections of growth), 2-UT-104 (growth above quantification limit), and 4-200-102 (*** sections of growth). In the accelerated study considerable growth was observed for diluted samples in week 3 (replicate-pressure-dilution): 1-200-103 (6.68 log CFU/mL), 2-200-103 (6.66 log CFU/mL), 1-600-103 (6.54 log CFU/mL), and 2-200-103 (6.57 log CFU/mL).
3.2.2 Microbial load analysis of accelerated shelf-life samples
Some vacuum-packed samples were stored refrigerated for 2 months followed by either one- or three-weeks storage at room temperature, and analyzed for microbial load both in liquid media and by CFU (Table 3). For samples stored for 1 and 3 weeks at room temperature, there were no conclusive difference between the three different treatments and the untreated samples, regarding samples cultivated both in liquid media and on agar plates. Overall, growth was denser in TSB, marine and malt broth, while not much was growing in EE Mossel broth and MRS. It can also be observed that for samples analyzed after 3 weeks, storage at room temperature the aliquots from bags subjected to 200 and 600 MPa, with completely dissolved seaweed parts, had higher OD values. Samples were filtered using a 40 µm cell strainer prior to OD measurements to ensure that larger biomass particles did not influence the readings.
The cultivation on agar plates indicated that the growth was more extensive in week 3 compared to in week 1. In week 1 growth was observed on malt and TSA plates for the 600 MPa sample, and no or little growth on the other agar types. In week 3 growth on TSA, VRBD and malt plates was predominant. MRS plates and untreated samples did not ever show any significant growth.
3.3 Analysis of the effect of HPP on physicochemical properties
Physicochemical properties of HPP-treated seaweed material were studied to evaluate to what extent the process affects the sample. Parameters of importance for seaweed for use as food are related to its sensory properties. Therefore, texture and color analysis were performed.
3.3.1 Texture
Texture analysis was performed to evaluate if HPP treatment affects the structure of the seaweed. The results (Table 4) showed that there is significant (p < 0.05) difference in both hardness (force A) and compression (distance at peak) between samples. See Table 4 for distribution of differences between samples. Force A corresponds to the force required for the instrument to penetrate the seaweed with the probe and Distance at Peak refers to the distance the probe travels from detection to disruption of the seaweed material. Untreated seaweed material showed highest resistance to puncturing by the probe (312.3 g and 5.5 mm). The resilience then decreased with increased treatment pressure; HPP 200 MPa [100.8 g (67.7% reduction) and 3.4 mm (38.2% reduction)], HPP 400 MPa [81.3 g (74.0% reduction) and 2.8 mm (49.1% reduction)], and HPP 600 MPa [38.3 g (87.7% reduction) and 2.2 mm (60.0% reduction)].
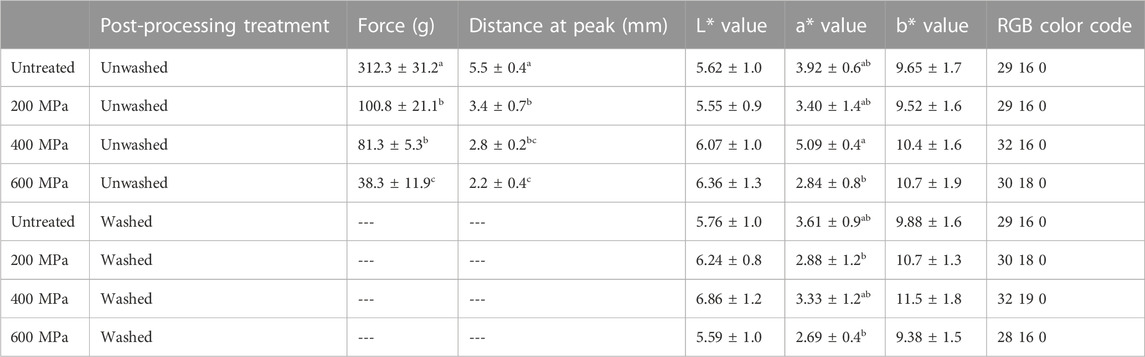
TABLE 4. Summary of results from the texture and color analysis. “Force” designates the maximum force required to puncture a piece of seaweed (hardness) and “Distance at Peak” refers to the distance the probe travel from detection to rupture of the seaweed sample (compression). Results are reported as average values ± standard deviation. Significant (p < 0.05) differences are indicated by letters (a-c).
3.3.2 Color
Color analysis was performed to evaluate if high pressure affects the perceived color of the HPP treated seaweed material. In the CIELAB color space, the L* value represents the perceptual lightness, whereas the a* axis represents a sample’s greenness/redness, and the b* axis relates to the blueness/yellowness of the sample. The color data in Table 4 indicate that L* and b* values for both washed and unwashed samples are similar at a significant level of 0.05, whereas for a* values (greenness/redness), the 400 MPa unwashed sample cannot be considered statistically similar to 600 MPa (unwashed and washed) and 200 MPa (washed). These differences are believed to arise from influence of water content from rinsing, and/or related to natural variation in the biomaterial.
3.4 Biochemical composition analysis
Biochemical analysis (Table 5) was performed to evaluate if physicochemical properties were affected by potential compositional differences, as well as to investigate if HPP treatment had an impact on the seaweed material. No liquid from the seaweed samples was expelled during the analysis, however, some inevitable separation of sap in the vacuum bags could occur during freeze drying of the seaweed.
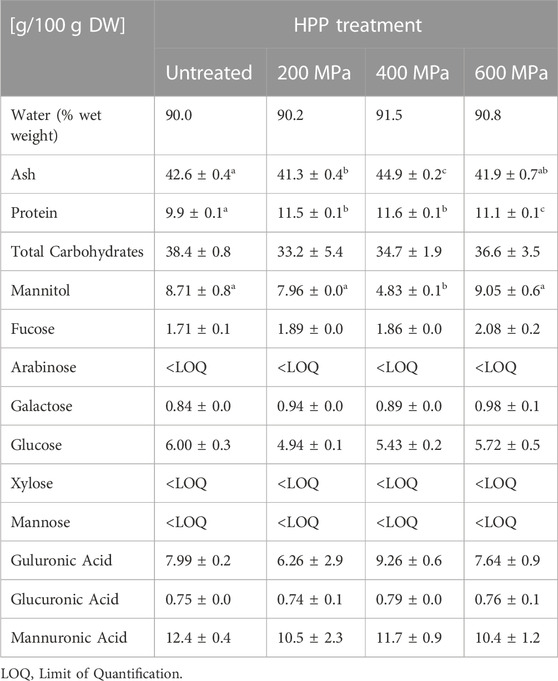
TABLE 5. Summary of composition analysis, including water, ash, protein, and carbohydrates specifying levels of neutral monosaccharides, mannitol, and uronic acids in the individual samples. Results are reported as average values ± standard deviation, and based on dry weight (DW) unless otherwise stated. Significant (p < 0.05) differences are indicated by letters (a-c).
The S. latissima seaweed samples analyzed in total were composed of around 90% water. The ash content in the different samples varied slightly from 41.3 ± 0.4 g/100 g DW in 200 MPa to 44.9 ± 0.2 g/100 g DW in the 400 MPa sample.
The levels of neutral monosaccharides, mannitol, uronic acids, and the calculated amount of total carbohydrates are summarized in Table 5. Contents of arabinose, xylose and mannose were lower than the quantification limit and thus did not give any reliable concentrations. The levels of total carbohydrates were similar in all samples. However, mannitol levels were significantly (p < 0.05) lower in the 400 MPa sample than in the other samples. The total carbohydrate composition ranged from 33.2 ± 5.4 g/100 g DW in the 200 MPa sample to 38.4 ± 0.8 g/100 g DW in the untreated sample.
Protein analysis showed that samples treated with 200 and 400 MPa pressure contained the highest levels of protein, 11.5 ± 0.1 g/100 g DW and 11.6 ± 0.1 g/100 g DW, respectively, followed by 600 MPa of 11.1 ± 0.1 g/100 g DW and untreated 9.9 ± 0.1 g/100 g DW. HPP treated material appeared to have a slightly higher protein content than untreated material.
4 Discussion
4.1 Microbial load of untreated material
The overall initial microbial load on the seaweed investigated was shown to be low. The lack of amplification of microbial DNA by PCR indicated that the seaweed contained too low levels of microorganisms for detection, which was also confirmed by subsequent analyses of microbial colonization. Marine macroalgae are reported to have a complex interchange of nutrients and substances with microbes hosting the seaweed body. This is crucial for successful metabolism by the seaweed (Martin et al., 2014; Singh and Reddy, 2014). However, the levels and species of microorganisms are dependent on the growth location (e.g., sun exposure, tides, currents, temperatures, and water properties such as salinity, acidity, nutrition), species, and time of harvest (Lytou et al., 2021). The post-harvest treatment may also influence the levels of microbes. S. latissima was harvested in mid-April when waters were still cold, and the algae were about 6 months old. The conditions in this Swedish National Park together with favorable harvesting conditions could be the reason for the little growth observed in all trials.
The low microbial load on the seaweed was also corroborated by the study of spontaneous fermentation of fresh and frozen seaweed, which was observed both visually and by OD measurements. Less growth was observed in samples with frozen seaweed than fresh seaweed samples. The analyses showed, however, for pH and gas production, that there was greater variation in output within the replicates than between samples, indicating that the microbial content was unevenly spread on the seaweed surface. A relatively higher growth of mold in the samples was observed visually in the trial with frozen seaweed than for fresh material (data not shown). Indications of increased growth after around 100 h of cultivation suggested that some gas-forming microbes, perhaps sporulating bacteria, have a long lag phase but take off in growth at this time.
Microbial profiling (Angly et al., 2014) showed that the genera found in the samples included Bacillus, Pseudomonas, Clostridium, Aminipila, and Lacrimispora (Table 2). The majority of Bacillus (Bacillota) species are non-pathogenic, but can cause spoilage of food, including sterilized products as their spores survive the heating process (Liu et al., 2021), and some species have shown robust defense against freezing (Gao et al., 2007). It can thus be assumed that the spores survived freezing in the spontaneous fermentation study and germinated once incubated at 37°C. Pseudomonas spp. (proteobacteria) are common soil and water bacteria which are naturally resistant to several antibiotic types. Several species can cause spoilage of food, and certain species, including P. aeruginosa, can pose health risks to immunocompromised people. Pseudomonas spp. are known to produce volatile compounds, amino acid metabolites, proteolytic and lipolytic enzymes causing off-flavor release, discoloration and compromise quality and shelf-life of products rich in protein and lipids (Quintieri et al., 2019). Clostridium spp. (Bacillota) commonly inhabit soil, water, and animal intestines. Several species, including C. sporogenes, can cause spoilage of food, but only a few species, including C. botulinum, are known to pose a risk of food-borne illness to humans (EFSA, 2005). Aminipila (Firmicutes) and Lacrimispora (Bacillota) are less described in the literature.
The most common food-borne pathogens include B. cereus, Campylobacter jejuni, Clostridium botulinum, Clostridium perfringens, Cronobacter sakazakii, Esherichia coli, Listeria monocytogenes, Salmonella spp., Shigella spp., Staphylococccus aureus, Vibrio spp. and Yersinia enterocolitica (Bintsis, 2017). Of the species found in the fresh seaweed sample, low amounts of Bacilli in a group that may include Bacillus cereus were found (<1.1% of bacterial community), and in addition, the sequences indicated presence of low amounts of Clostridia species that may include C. botulinum (<0.5% of bacterial community) (Table 2), highlighting the importance of using preservation methodologies preventing bacterial growth.
4.2 Microbial load of HPP treated material
The low initial load, and uneven distribution of microbes in the seaweed material, made evaluation of antimicrobial effects of HPP difficult. Randomly selected large pieces of seaweed were added to the vacuum bags to minimize contamination by handling, but might not have constituted a homogeneous representation of different seaweed parts. Colony counting of the refrigerated samples in the six-month shelf-life study showed overall inconsistent results. However, it can be concluded that the microbes present prefer TSA media for growth, whereas growth was never observed on MRS plates and malt (with some exceptions), while VRBD medium was covered occasionally. This suggests that the unspecific TSA media facilitates growth of some unknown aerobic bacteria, while VRBD shows occasional growth of gram-negative Enterobacteriaceae. Species of the Lactobacillus genus were not observed, whereas yeast and mold seemingly occurred in parts of seaweed. The interaction between macroalgae and surrounding microorganisms is of high complexity (Martin et al., 2014; Singh and Reddy, 2014). The intricate interaction suggests that microorganisms might be strongly associated with the biomass and not necessarily evenly distributed in solution. The majority of microbes encountered in the natural environment are considered non-culturable and cannot be taxonomically identified, hence the performed microbial screening with PCR. Culturability is obviously dependent on the media used, but has been shown to be below 1% (Ultee et al., 2004; Cavallo et al., 2009). Lack of PCR amplification, however, showed that the initial number of microorganisms in the sample was low, which could be another explanation for the overall poor growth. According to studies by del Olmo et al. (2020), the response to HPP treatment and initial bacterial counts of colony forming units varies considerably between species.
The method for analyzing microbial load in liquid media, of samples cultivated up to 3 weeks at room temperature after 2 months in the fridge, proved to discriminate samples containing insoluble biofilm with regard to optical density. TSB, malt broth and marine broth exhibited highest levels of growth, while MRS and EE Mossel broth did not favor growth of seaweed-associated microbes. These findings agree with results obtained from cultivation on agar plates. Overall, microbial growth was rather low in the samples, which makes it difficult to draw conclusions. Consequently, it cannot be concluded from the data which pre-treatment pressure is most suitable for the purpose of inactivating microorganisms associated with seaweed. However, it is apparent from the data from week 3 that the bags with completely degraded seaweed parts contained increased levels of microbial cells favoring growth in marine broth and TSB. These cells could be any aerobic heterotrophic marine bacteria.
The microbial load on seaweed samples stored for up to 3 weeks after 2 months in the fridge was also evaluated by counting colonies on agar plates. It is evident that the microbial load overall increases with time. Week 1 agrees with the results obtained from the OD measurements using liquid media, whereas in week 3 colonies were observed on VRBD plates, although no growth was detected in the corresponding EE broth. Most growth was observed in samples subjected to 200 or 600 MPa. This agrees with findings from measurements in liquid media. This observation could be explained by these bags potentially containing more sporulating microorganisms. Additionally, at least two parameters, the nutrition availability and the mechanism for damaging the microbes, are likely to be involved in the process (Tao et al., 2014; Balasubramaniam et al., 2016). It is suggested by del Olmo et al. (2020) that while the contamination increased in control samples during the study period, the number of cells remained low in pressure-treated samples when counting cells on marine agar. Our results do not agree with their findings as untreated control samples remain low whereas pressure treated samples are, to a different extent, subjected to growth. The inconsistent growth observed in our study can be attributed to the low amounts of cells initially detected and the complex nature of microorganisms naturally inherent in aquatic environments. Also, uneven distribution of various seaweed parts in the vacuum bags could be a cause of the inconsistent growth observed. This derives from the different parts of seaweed being likely to be colonized with various types and numbers of organisms.
Overall, the observed poor growth (which is advantageous from a food perspective) may be a result of seaweed self-preservation from intrinsic antimicrobial compounds and relatively high levels of salt (Wiese et al., 2009). Also, the packaging in vacuum bags can result in unfavorable growth conditions for most microorganisms, and the packaging itself may hence have a preservative action.
4.3 Physicochemical analysis
The texture analysis proved to be an efficient method for determination of the structure of the seaweed after pressure processing. Both hardness and compression, measured by force and distance to peak, respectively, decreased with increased treatment pressure. The structural fragility was also observed visually. Different analyses of seaweed texture have been performed previously, but with varying methodologies. A study by del Olmo et al. (2020) showed a similar trend concerning the impact of pressure on the seaweed texture. The change in texture is likely explained by that the high pressure is modifying the defined special arrangements of protein and carbohydrate biopolymers in the algae. Disruption of carbohydrate structures can cause gelatinization, which has been explained to increase with increasing pressure (Balasubramaniam et al., 2016).
Analysis of color was done, firstly to compare the samples after different pressure treatments, and secondly to compare any color differences in washed and unwashed samples. Visually, there was no apparent difference between the samples, just natural color shifts in different parts of the seaweed. Only the 600 MPa washed sample had color significantly deviating from unwashed untreated and 400 MPa samples. This difference is believed to arise from natural variation in the biomaterial and/or the influence of water content after rinsing. The reflectance peak observed in this study appeared at 580 nm for all samples. To date, little comparative data is available for the reflectance spectrum, compared to the absorbance spectrum, for common pigments, because various parameters such as pigment, water content and structure variables influence the outcome. However, attempts have been made to link reflectance spectra to pigment concentration (Sims and Gamon, 2002), although this is not available for pigments in seaweed. Brown marine macroalgae comprise the pigments chlorophyll a and c (green color), as well as carotenoids (yellow, red color), dominated by fucoxanthin, which is accountable for the brown-yellow color (Kim, 2015). Both pigment groups are relatively stable with respect to pressure. Chlorophylls, studied in broccoli at pressures up to 800 MPa and 50°C, were resistant to degradation (Van Loey et al., 1998), while the pigment group, carotenoids, studied in carrots was more prone to degradation (by 26%, at a pressure of 600 MPa) (Stinco et al., 2019). This indicates that potential differences between the samples could be a result of carotenoid degradation. According to a study by del Olmo et al. (2020) the HPP treatment significantly influenced the a* and b* values of the seaweed color, in a manner contradictory to the stability of the pigments. However, such color changes, attributed to different pressure conditions, were not observed in our study.
4.4 Biochemical composition analysis
Ash content differed significantly (p < 0.05) between some samples, which may be associated to natural variations in the seaweed. The results of water and ash are assumed not to affect the seaweed’s physicochemical attributes. Seaweed samples were not rinsed after processing, and potentially extracted metals may therefore be accounted for.
Levels of monosaccharides and uronic acids were similar between the different samples, only mannitol levels in the 400 MPa sample were significantly (p < 0.05) lower than in the other samples. Levels of mannitol in seaweed have shown to alter when the seaweed are subjected to stress derived for instance from osmotic pressure or salinity (Groisillier et al., 2014). The lower levels of mannitol in one sample can thus arise from release of mannitol in sap liquid, which may have been unevenly included in samples for analysis. However, the results show overall that HPP does not cause carbohydrate degradation, in contrast to other treatment methods such chemical processing (Allahgholi et al., 2020).
The composition results obtained for S. latissima in this study (ash 41%–45% DW, carbohydrates 33%–38% DW and protein 10%–12% DW) was compared to analysis performed on the same species harvested on the Swedish west coast in 2012 (Vilg et al., 2015). Ash, total carbohydrate, and protein content in sugar kelp harvested in 2021 were generally higher than in material from 2012. Slightly different methods were employed in the two studies. However, the levels obtained in this study are considered normal values for brown seaweed in general (ash 17%–44% DW, carbohydrates 12.2%–56.4% DW, and protein 4.3%–24.0% DW) (Salehi et al., 2019). No study has, to the knowledge of the authors, previously analyzed the compositional effects of seaweed after HPP treatment.
Only a minor variation in protein content, but to a significant degree (p < 0.05), was observed between the different samples. The variation in protein content, as well as inconsiderable variation in total carbohydrate concentrations, may be attributed to the natural variances in biomaterials. But, the analysis of total protein content does not account for composition of amino acids, and knowledge of the protein structure in the samples after HPP is hence lacking. Slightly higher protein content was observed in HPP-treated samples than untreated, which is likely due to natural variances in biomass. Another potential explanation could be that the protein structures are more subjected to degradation after pressure treatment than untreated material. The seaweed matrix in untreated material might not be completely oxidized, and the Dumas method is selective for total nitrogen but lacks some selectivity for undegraded protein (Moore et al., 2010).
Considering that the chemical analysis demonstrated that samples had similar composition, the overall effect on physicochemical attributes and microbial fouling cannot be ascribed to composition but rather to the structural level.
5 Conclusion
The overarching aim of this study was to evaluate the effects of HPP treatment on seaweed material considering its chemical, physicochemical, and microbial characteristics. It was concluded from the different microbial analyses that the initial load was very low. This is promising when considering seaweed for food. Accordingly, spontaneous fermentation demonstrated overall low growth over time, but exhibited a rather wide variance between replicates, suggesting that microorganisms are not uniformly distributed on the fronds. It was also concluded that freezing has an impact on cell viability, although measured growth was relatively low in both fresh and frozen samples. This could be considered in future studies if frozen seaweed is to be used.
The study further concluded that pressurization of seaweed material has a high impact on the texture and structure of the material, which was observed visually and by texture analysis. This alteration could be interesting in cases where further mechanical processing or softening of the algae texture is desired. Ultimately, the efficiency of HPP treatment as an alternative preservation method for seaweed material was difficult to assess due to the low initial microbial load. However, the method proved to be effective in altering the texture while retaining the color and nutritional content of the seaweed.
Data availability statement
The original contributions presented in the study are publicly available. This data can be found here: Sequence Read Archive (SRA) accession numbers: SRR24210685, SRR2421068.
Author contributions
MJ, ENK, and MR contributed to conception and design of the study. MJ and LA conducted the investigation. MJ performed the data- and statistical analysis and visualization. MJ wrote the first draft of the manuscript. All authors contributed to manuscript revision, read, and approved the submitted version. ENK and MR supervised. ENK was responsible for funding acquisition.
Funding
The authors wish to express their thanks for the financial support for the projects: Marine food resources for new markets granted by Formas (Grant No. 2018-01863), the Era-net project SeaPro supported by Vinnova (Grant No. 2021-03368), the MariKat project enabled by Formas (Grant No. 2019-02359), and SeaMark funded by Horizon Europe (Project 101060379).
Acknowledgments
The authors wish to direct special thanks to Nordic Sea Farm for kindly providing the project with freshly harvested seaweed.
Conflict of interest
The authors declare that the research was conducted in the absence of any commercial or financial relationships that could be construed as a potential conflict of interest.
Publisher’s note
All claims expressed in this article are solely those of the authors and do not necessarily represent those of their affiliated organizations, or those of the publisher, the editors and the reviewers. Any product that may be evaluated in this article, or claim that may be made by its manufacturer, is not guaranteed or endorsed by the publisher.
References
Albers, E., Malmhäll-Bah, E., Olsson, J., Sterner, M., Mayers, J. J., Nylund, G. M., et al. (2021). Influence of preservation methods on biochemical composition and downstream processing of cultivated Saccharina latissima biomass. Algal Res. 55, 102261. doi:10.1016/j.algal.2021.102261
Allahgholi, L., Sardari, R. R., Hakvåg, S., Ara, K. Z., Kristjansdottir, T., Aasen, I. M., et al. (2020). Composition analysis and minimal treatments to solubilize polysaccharides from the Brown seaweed Laminaria digitata for microbial growth of thermophiles. J. Appl. Phycol. 32 (3), 1933–1947. doi:10.1007/s10811-020-02103-6
Angell, A. R., Mata, L., de Nys, R., and Paul, N. A. (2016). The protein content of seaweeds: A universal nitrogen-to-protein conversion factor of five. J. Appl. Phycol. 28 (1), 511–524. doi:10.1007/s10811-015-0650-1
Angly, F. E., Dennis, P. G., Skarshewski, A., Vanwonterghem, I., Hugenholtz, P., and Tyson, G. W. (2014). CopyRighter: A rapid tool for improving the accuracy of microbial community profiles through lineage-specific gene copy number correction. Microbiome 2 (1), 11–13. doi:10.1186/2049-2618-2-11
Badmus, U. O., Taggart, M. A., and Boyd, K. G. (2019). The effect of different drying methods on certain nutritionally important chemical constituents in edible Brown seaweeds. J. Appl. Phycol. 31 (6), 3883–3897. doi:10.1007/s10811-019-01846-1
Balasubramaniam, V., Barbosa-Cánovas, G. V., and Lelieveld, H. (2016). High pressure processing of food: Principles, technology and applications. Springer.
Bintsis, T. (2017). Foodborne pathogens. AIMS Microbiol. 3(3), 529. 563. Available at: https://www.doi.org/10.3934/microbiol.2017.3.529.
Blikra, M. J., Altintzoglou, T., Løvdal, T., Rognså, G., Skipnes, D., Skåra, T., et al. (2021). Seaweed products for the future: Using current tools to develop a sustainable food industry. Trends Food Sci. Technol. 118, 765–776. doi:10.1016/j.tifs.2021.11.002
Cai, J., Lovatelli, A., Stankus, A., and Zhou, X. (2021). “Seaweed revolution: Where is the next milestone?,” in FOA aquaculture news (Rome, Italy: FAO).
Cavallo, R., Acquaviva, M., and Stabili, L. (2009). Culturable heterotrophic bacteria in seawater and Mytilus galloprovincialis from a mediterranean area (northern ionian Sea–Italy). Environ. Monit. Assess. 149 (1), 465–475. doi:10.1007/s10661-008-0223-8
D’Agostino, M., Cook, N., Caballero, B., Finglas, P. M., and Toldrá, F. (2016). Encyclopedia of Food and Health. Oxford: Academic Press, 83–86.Foodborne pathogens
del Olmo, A., Picon, A., and Nuñez, M. (2019). High pressure processing for the extension of Laminaria ochroleuca (kombu) shelf-life: A comparative study with seaweed salting and freezing. Innovative Food Sci. Emerg. Technol. 52, 420–428. doi:10.1016/j.ifset.2019.02.007
del Olmo, A., Picon, A., and Nuñez, M. (2020). Preservation of five edible seaweeds by high pressure processing: Effect on microbiota, shelf life, colour, texture and antioxidant capacity. Algal Res. 49, 101938. doi:10.1016/j.algal.2020.101938
EFSA (2005). Opinion of the Scientific Panel on biological hazards (BIOHAZ) related to Clostridium spp in foodstuffs. EFSA J. 3 (4), 199.
Ekonomou, S. I., and Boziaris, I. S. (2021). Non-thermal methods for ensuring the microbiological quality and safety of seafood. Appl. Sci. 11 (2), 833. doi:10.3390/app11020833
Gao, W., Smith, D., and Li, Y. (2007). Effects of freezing on the survival of Escherichia coli and Bacillus and response to UV and chlorine after freezing. Water Environ. Res. 79 (5), 507–513. doi:10.2175/106143006X115426
Groisillier, A., Shao, Z., Michel, G., Goulitquer, S., Bonin, P., Krahulec, S., et al. (2014). Mannitol metabolism in Brown algae involves a new phosphatase family. J. Exp. Bot. 65 (2), 559–570. doi:10.1093/jxb/ert405
Hames, B., Ruiz, R., Scarlata, C., Sluiter, A., Sluiter, J., and Templeton, D. (2008). "Preparation of Samples for Compositional Analysis". (Golden, CO: Midwest Research Institute). Available at: https://www.nrel.gov/docs/gen/fy08/42620.pdf.
Hollants, J., Leliaert, F., De Clerck, O., and Willems, A. (2013). What we can learn from sushi: A review on seaweed–bacterial associations. FEMS Microbiol. Ecol. 83 (1), 1–16. doi:10.1111/j.1574-6941.2012.01446.x
Leser, T. D., Amenuvor, J. Z., Jensen, T. K., Lindecrona, R. H., Boye, M., and Moller, K. (2002). Culture-independent analysis of gut bacteria: The pig gastrointestinal tract microbiota revisited. Appl. Environ. Microbiol. 68 (2), 673–690. doi:10.1128/AEM.68.2.673-690.2002
Liu, J., Luo, Y., Xu, Z., and Kjellerup, B. V. (2021). “Food pathogens,” in Innovative food analysis (Elsevier), 295–321.
López-Pérez, O., del Olmo, A., Picon, A., and Nuñez, M. (2020). Volatile compounds and odour characteristics during long-term storage of kombu seaweed (Laminaria ochroleuca) preserved by high pressure processing, freezing and salting. LWT 118, 108710. doi:10.1016/j.lwt.2019.108710
Løvdal, T., Lunestad, B. T., Myrmel, M., Rosnes, J. T., and Skipnes, D. (2021). Microbiological food safety of seaweeds. Foods 10 (11), 2719. doi:10.3390/foods10112719
Lytou, A. E., Schoina, E., Liu, Y., Michalek, K., Stanley, M. S., Panagou, E. Z., et al. (2021). Quality and safety assessment of edible seaweeds Alaria esculenta and Saccharina latissima cultivated in Scotland. Foods 10 (9), 2210. doi:10.3390/foods10092210
Martin, M., Portetelle, D., Michel, G., and Vandenbol, M. (2014). Microorganisms living on macroalgae: Diversity, interactions, and biotechnological applications. Appl. Microbiol. Biotechnol. 98 (7), 2917–2935. doi:10.1007/s00253-014-5557-2
Mateluna, C., Figueroa, V., Ortiz, J., and Aguilera, J. M. (2020). Effect of processing on texture and microstructure of the seaweed Durvillaea Antarctica. J. Appl. Phycol. 32 (6), 4211–4219. doi:10.1007/s10811-020-02259-1
McNevin, A., Cousteau, A., Yap, A. Y., Cai, J., Cottier-Cook, E., Giercksky, E., et al. (2020). in Seaweed revolution: A manifesto for a sustainable future. Editors V. Doumeizel, and K. Aass
Moore, J. C., DeVries, J. W., Lipp, M., Griffiths, J. C., and Abernethy, D. R. (2010). Total protein methods and their potential utility to reduce the risk of food protein adulteration. Compr. Rev. food Sci. food Saf. 9 (4), 330–357. doi:10.1111/j.1541-4337.2010.00114.x
Oey, I., Lille, M., Van Loey, A., and Hendrickx, M. (2008). Effect of high-pressure processing on colour, texture and flavour of fruit-and vegetable-based food products: A review. Trends Food Sci. Technol. 19 (6), 320–328. doi:10.1016/j.tifs.2008.04.001
Picon, A., Del Olmo, A., and Nuñez, M. (2021). Bacterial diversity in six species of fresh edible seaweeds submitted to high pressure processing and long-term refrigerated storage. Food Microbiol. 94, 103646. doi:10.1016/j.fm.2020.103646
Quintieri, L., Fanelli, F., and Caputo, L. (2019). Antibiotic resistant Pseudomonas spp. spoilers in fresh dairy products: An underestimated risk and the control strategies. Foods 8 (9), 372. doi:10.3390/foods8090372
Salehi, B., Sharifi-Rad, J., Seca, A. M., Pinto, D. C., Michalak, I., Trincone, A., et al. (2019). Current trends on seaweeds: Looking at chemical composition, phytopharmacology, and cosmetic applications. Molecules 24 (22), 4182. doi:10.3390/molecules24224182
Silva, V. M. d., Silva, L. A., Andrade, J. B. d., Veloso, M. C., and Santos, G. V. (2008). Determination of moisture content and water activity in algae and fish by thermoanalytical techniques. Quimica Nova 31 (4), 901–905. doi:10.1590/S0100-40422008000400030
Sims, D. A., and Gamon, J. A. (2002). Relationships between leaf pigment content and spectral reflectance across a wide range of species, leaf structures and developmental stages. Remote Sens. Environ. 81 (2-3), 337–354. doi:10.1016/S0034-4257(02)00010-X
Singh, R. P., and Reddy, C. (2014). Seaweed–microbial interactions: Key functions of seaweed-associated bacteria. FEMS Microbiol. Ecol. 88 (2), 213–230. doi:10.1111/1574-6941.12297
Stinco, C. M., Szczepanska, J., Marszalek, K., Pinto, C. A., Inacio, R. S., Mapelli-Brahm, P., et al. (2019). Effect of high-pressure processing on carotenoids profile, colour, microbial and enzymatic stability of cloudy carrot juice. Food Chem. 299, 125112. doi:10.1016/j.foodchem.2019.125112
Tao, Y., Sun, D.-W., Hogan, E., and Kelly, A. L. (2014). High-pressure processing of foods: An overview. Emerg. Technol. food Process., 3–24. doi:10.1016/B978-0-12-411479-1.00001-2
Ultee, A., Souvatzi, N., Maniadi, K., and König, H. (2004). Identification of the culturable and nonculturable bacterial population in ground water of a municipal water supply in Germany. J. Appl. Microbiol. 96 (3), 560–568. doi:10.1111/j.1365-2672.2004.02174.x
Van Loey, A., Ooms, V., Weemaes, C., Van den Broeck, I., Ludikhuyze, L., Indrawati, O., et al. (1998). Thermal and pressure-temperature degradation of chlorophyll inBroccoli (Brassica oleraceaL.italica) juice: A kinetic study. J. Agric. Food Chem. 46 (12), 5289–5294. doi:10.1021/jf980505x
Van Wychen, S., and Laurens, L. M. L. (2015). "Determination of total solids and ash in algal biomass". (Golden, CO: National Renewable Energy Laboratory). Available at: https://www.nrel.gov/docs/fy16osti/60956.pdf.
Vilg, J. V., Nylund, G. M., Werner, T., Qvirist, L., Mayers, J. J., Pavia, H., et al. (2015). Seasonal and spatial variation in biochemical composition of Saccharina latissima during a potential harvesting season for Western Sweden. Bot. Mar. 58 (6), 435–447. doi:10.1515/bot-2015-0034
Weisburg, W. G., Barns, S. M., Pelletier, D. A., and Lane, D. J. (1991). 16S ribosomal DNA amplification for phylogenetic study. J. Bacteriol. 173 (2), 697–703. doi:10.1128/jb.173.2.697-703.1991
White, T. J., Bruns, T., Lee, S., and Taylor, J. (1990). “Amplification and direct sequencing of fungal ribosomal RNA genes for phylogenetics,” in PCR protocols. A guide to methods and applications. Editors M. A. Innis, D. H. Gelfand, J. J. Sninsky, and T. J. White (San Diego: Academic Press), 315–322.
Keywords: post-harvest treatment, physicochemical effects, microbial effects, stability, seaweed, composition
Citation: Jönsson M, Allahgholi L, Rayner M and Nordberg Karlsson E (2023) Exploration of high-pressure processing (HPP) for preservation of the Swedish grown brown macroalgae Saccharina latissima. Front. Food. Sci. Technol. 3:1150482. doi: 10.3389/frfst.2023.1150482
Received: 24 January 2023; Accepted: 24 April 2023;
Published: 05 June 2023.
Edited by:
Christos Soukoulis, Luxembourg Institute of Science and Technology (LIST), LuxembourgReviewed by:
Dagbjørn Skipnes, Norwegian Institute of Food, Fisheries and Aquaculture Research (Nofima), NorwayDaniela Borda, Dunarea de Jos University, Romania
Copyright © 2023 Jönsson, Allahgholi, Rayner and Nordberg Karlsson. This is an open-access article distributed under the terms of the Creative Commons Attribution License (CC BY). The use, distribution or reproduction in other forums is permitted, provided the original author(s) and the copyright owner(s) are credited and that the original publication in this journal is cited, in accordance with accepted academic practice. No use, distribution or reproduction is permitted which does not comply with these terms.
*Correspondence: Madeleine Jönsson, bWFkZWxlaW5lLmpvbnNzb25AYmlvdGVrLmx1LnNl
†ORCID: Madeleine Jönsson, orcid.org/0009-0001-4186-2876; Eva Nordberg Karlsson, orcid.org/000-0002-8597-7050