- 1GreenTech Innovation Centre, Environmental Research and Innovation Department, Luxembourg Institute of Science and Technology, Esch-Sur-Alzette, Luxembourg
- 2PM International AG, Luxembourg, Luxembourg
- 3Louvain Institute of Biomolecular Science and Technology (LIBST), UCLouvain, Louvain-la-Neuve, Belgium
Brewer’s spent grain (BSG) is the most abundant by-product of the brewery industry. Its accessibility and chemical composition allow this material to be recycled for new applications mainly targeting the food industry, based on the health-promoting properties of phenolics in BSG extracts. This study investigates the major compounds identified in food-grade extracts from BSG by UHPLC-ESI-MS/MS analyses, with a focus on the presence of Amadori products. These fructosyl-derivatives are identified for most of the proteinogenic amino acids but for instance also for gamma-aminobutyric acid. While previously proposed to be formed, we show that Amadori products are formed by reaction of amino acses, with a focus on the presence of Amadori products. These fructosyl-derivatives are identified for most of the proteinogenic amino acids but for instance also for gamma-aminobutyric acid. While previously proposed to be formed, we show that Amadori products are formed by reaction of amino acids with oligosaccharides up to five hexoses. Moreover, the same glycation pattern was also observed for other nitrogen-containing compounds such as phosphatidylethanolamine lipids. The presence of these Maillard reaction products and glycated forms of amino-compounds, due to heat-treatment during brewing or stabilization of BSG prior to further processing, may impact the valorization of this by-product and should thus be considered in future studies.
1 Introduction
In the context of circular economy, there is currently a focus on the upcycling of by-products from industrial processes. The food industry, in general, and the brewery sector, in particular, have a long tradition of re-use of their by-products, however upcycling remains often limited. The circular use of material aims at the efficient use of resources and, through that, at environmental protection. It is coupled with the increased economic development (Chetrariu and Dabija, 2020) and is the focus of development in more and more countries. Keywords in this development are re-use, recycling and upcycling, and the term residue takes on another meaning: opportunity. As for all waste/residue streams, this also counts for residual biomass from forestry, agriculture and industrial activities.
The global production of beer reached 1.9 billion hectoliters in 2019, with 402 million hectoliters being produced in Europe. For Europe, this results yearly in the production of 3.4 million tons of brewer’s waste, that could be up/recycled into new commodities. Non-seasonality, nutritional value and low price of brewery by-products are facilitating their use as component of food, medical and biotechnological products (Karlović et al., 2020).
Barley, accounting for 19% of the European cereal production in 2020, is one of the most cultivated cereals after maize (23.7%) and wheat (41.6%). It is mainly used for animal feed and human consumption, with 21% of the cultivated barley destined for beer production (Mussatto et al., 2006). During brewing, barley is germinated and the germinated grains are subsequently dried at high temperature during the kilning process to become malt. Malt is milled and again exposed to high temperatures during mashing, a process, during which, starch is enzymatically degraded. The enzymatic degradation produces a liquid with carbohydrates, soluble proteins, polypeptides and amino acids, called wort, and a solid fraction containing residues of the grains. This suspension is filtered (lautering step), hop is added before boiling and the resulting wort is used as a fermentation base for yeast to produce beer. During the steps prior to lautering, the exposure to high temperatures induces the formation of compounds which, like the addition of different types of hop the by-products formation from yeast metabolism and the stability of flavor compounds during storage, determine the taste of the final product (Oladokun et al., 2017).
Today, the beer industry is looking for new types of products and increased productivity, which include the efficient reuse of its biomass waste (Rachwał et al., 2020). The first and most abundant biomass by-product originating from brewing is brewer’s spent grain (BSG); it is discarded after the filtration step (lautering) and is a water-rich solid phase composed of the grain residues. Afterwards, a second waste is removed: spent hop, which represents the precipitated compounds, mostly proteins, after the boiling step. The third and less significant residue eliminated before bottling is spent yeast, a by-product reused in brewing (Fărcaş et al., 2017).
BSG represents about 85% of the total by-product: around 20 kg of wet spent grains are generated for each hectoliter of beer. BSG contains the husks, pericarp, and seed coat of the grains. It is rich in cellulose and non-cellulose polysaccharides, lignin, proteins, and lipids. The exact composition is influenced by the grain variety used, the agricultural methods and brewery’s process conditions (Mussatto et al., 2006). Globally, spent grain contains 19%–30% proteins, 20%–25% hemicelluloses, 12%–25% cellulose, 12%–28% lignin and 10% lipids. It furthermore contains vitamins, phenolic compounds and minerals (Lynch et al., 2016; Rachwał et al., 2020). Due to its high moisture (approximately 80% w/w) (Chetrariu and Dabija, 2020), fermentable sugar, and protein contents, fresh BSG is susceptible to degradation by microbial activity. In this context, industrial validation of BSG generally requires drying as pre-treatment, thereby limiting microbial growth and increasing the storage time at room temperature (Mussatto et al., 2006). Most used are oven- and freeze-drying. Although studies indicate that freeze-drying is likely the best technique to maintain the chemical properties of the starting material, it is not economically sustainable on an industrial scale (Lynch et al., 2016). This makes oven-drying the most feasible technique for industrial valorization of BSG, despite its high energy consumption and influence on the chemical composition (Mussatto et al., 2006), (Lynch et al., 2016).
Because of the high amount of fibers and proteins and its low price, BSG is mainly used for animal feeding. It is known as a source of nitrogen and as a promoter of milk production in ruminants (Mussatto et al., 2006). However, environmental and economic factors render other uses more appropriate (Steiner et al., 2015). Indeed, due to the richness of its chemical composition, new potential uses of BSG have arisen in recent years, and BSG is now studied or used as an accessible and cheap base material in biorefinery, biotechnology, nutrition and medicine (Chetrariu and Dabija, 2020). Many applications are being screened: biogas production through anaerobic degradation; bricks for building; substrate for fermentation processes or to grow microorganisms and as source of enzymes, molecules or prebiotics (Chetrariu and Dabija, 2020). In nutrition, milled BSG can be used as flour or as component in the production of snacks (Lynch et al., 2016). Despite this wide range of uses, most research on BSG focusses on the health-promoting potential of BSG extracts due to the presence of antioxidants such as vitamin E and phenolic compounds (Bonifácio-Lopes et al., 2020), arabinoxylans, beta-glucans and fatty acids. Another alternative use of BSG is as source of proteins with interesting gelation properties (Hellebois et al., 2021).
In previous studies, BSG extracts have been analyzed for the identification of health-promoting compounds, with absolute quantification of ferulic acid, p-coumaric acid and catechin often used as quality markers (Moreira et al., 2013; Rachwał et al., 2020). Other parameters generally assessed are the total phenolic content and the antioxidant capacity. The total phenolic content of BSG changes according to the malt type, and values ranging from 40.97 to 114.23 mg GAE/100 g DW have been reported (Socaci et al., 2018a).
In addition to the above-mentioned well-characterized molecules, reaction products between amino acids and reducing sugars, known as Amadori products, have been identified. Amadori compounds (Figures 1A,B), 1-amino-1-deoxyketoses, represent a class of intermediates in the Maillard reaction. More precisely, their formation takes place during the initial phase of this cascade (Davidek et al., 2005a; Xue et al., 2020). A reducing sugar reacts with a free amino group forming a glycosylamine, called a Schiff base. This unstable condensation product reversibly and spontaneously rearranges itself to generate Amadori compounds. Because the formation of Amadori products is faster than the reverse reaction and is promoted in acid conditions, these keto-amine structures are predominant in equilibrium conditions compared to the Schiff base (Troise et al., 2018). In a later stage of the Maillard reaction, degradation of Amadori products gives rise to oxoaldehydes, highly reactive dicarbonyl compounds with glyoxal, methylglyoxal and 3-deoxyglucosone being the most important. Further reaction of these oxoaldehydes results in advanced glycation end products (AGEs), generated by irreversible reactions between carbonyl and amino groups. As proposed by Hodge in 1955 (Hodge and Wolfrom, 1955), a series of reactions starting from Amadori products and derivatives leads to the formation of volatile compounds and brown pigments, such as melanoidins, that give color, aroma and flavor to processed food (Hodge and Wolfrom, 1955; Hofmann and Schieberle, 2000). This mechanism occurs mainly during long-term storage and thermal processing of sugar-rich food (Del Castillo et al., 1999; Meitinger et al., 2014).
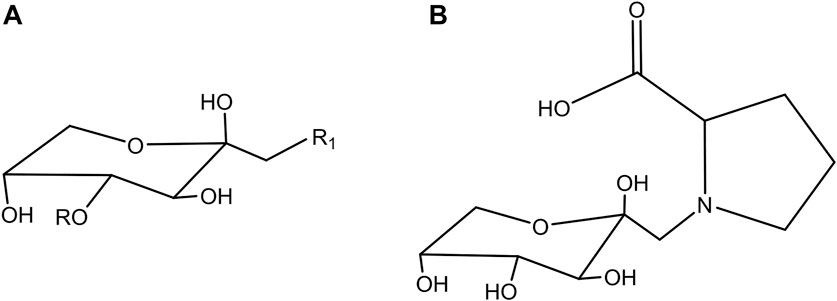
FIGURE 1. (A) Typical chemical structure of Amadori products: R = [Hex]n with n from 0 to 4 in the compounds identified in this study; R1 = - specific amino acid (Arg; Gly, Lys; Ala; GABA; Pro; Val; Met; pGlu; Tyr; Ile/Leu; Phe; Trp; His). (B) Structure of fructosyl-Pro.
The first objective of the work presented here was to identify as much as possible compounds found in BSG extracts obtained with the most frequently used solvent for food-grade extractions. With this effort, a database was built to facilitate the identification of compounds in further studies on BSG extracts. Therefore, yield optimization was not the purpose of the work presented here.
Besides all the compounds generally found in BSG extracts, we identified several early stage products of the Maillard cascade, known as Amadori products, which have not yet been described in brewer’s spent grain extracts so far. In addition, not only did we identify Amadori products for most proteinogenic amino acids, but Amadori reaction products between free amino acids and oligosaccharides of up to 5 monomers were also identified. In malt, disaccharide-derived glycated lysine (N-ε-maltulosyllysine) was previously identified and characterized and the presence of other disaccharide linked amino acids was hypothesized (Hellwig and Henle, 2020). Here, we confirm this hypothesis and extend it by identifying glycation products with oligosaccharides for most amino acids. Similar products were identified for non-proteinogenic amino acids and for phosphatidylethanolamine lipids. To our knowledge, these products have not been previously identified in BSG extracts and the formation of di-to pentasaccharide-glycated amino acids is not mentioned in the literature.
2 Material and method
2.1 Chemicals
The following chemicals were obtained commercially. Ethanol (WMR 20821.321); formic acid (LGC Standards-Promochem, SO-9679-B001); acetonitrile (Carl Roth, HN40.2); Folin-Ciocalteu reagent (Sigma Aldrich, F9252-100ML); NA2CO3 (Sigma Aldrich, 223530-500G); gallic acid (Sigma Aldrich; 27,645-250G-R); hydrochloric acid (HCl) 0.1 M (ChemLab, 31955373); Iron (III) chloride hexahydrated (FeCl3 * 6 H2O) (Merck VWR, 1.03943250); Iron (II) sulfate heptahydrate (FeSO4 * 7 H2O) (VWR; 24,244.232); Sodium acetate trihydrate (C2H3NaO2 * 3 H2O) (Sigma Aldrich, 331058-100G); acetic acid, glacial (CH3COOH) (J.T.Baker, 9524-33); Fluorescein (Sigma-Aldrich, 46955-100G-F); Trolox (Sigma-Aldrich, 238813-1G); AAPH (Sigma-Aldrich, 440914-25G); Potassium phosphate monobasic (KH2PO4) (Merck, 1048730250); Potassium phosphate dibasic (K2HPO4) (Vel VWR, PROL26931263).
2.2 Material
Fresh BSG was obtained from a local brewery (Brasserie Simon Sàrl, Wiltz, Luxembourg) and immediately frozen, vacuum-packed and stored in polypropylene bags at −20°C. Subsequently BSG was been dried in an air convection oven at 50°C for 48 h as previously described (Bonifácio-Lopes et al., 2020), vacuum-packed and stored in polypropylene bags at room temperature.
2.3 Solvent extraction procedure
Three different extraction solvents were used: 1) water 2) 20% ethanol (v/v%) and 3) 40% ethanol (v/v%). The three extractions were carried out at the ratio 33:1 (v:w; solvent:solid), based on literature and EU food-grade regulations (Meneses et al., 2013). For the extractions, dried BSG was ground with mortar and pestle and 30 mg of BSG were mixed with 1 ml of solvent (MilliQ water, ethanol 20%; ethanol 40%) and shaken at 1,400 rpm for 4 h at 21°C. Samples were centrifuged at 20,000 g at 15°C for 25 min. The supernatants were collected and dried with a vacuum concentrator (CentriVap; Labconco, Kansas City, MO, United States). All extractions were performed in quadruplicate. Dried extracts were then dissolved in 300 µl MeOH:H2O 5:95 and filtered on 0.22 µm PTFE syringe filters (Millex-LG; Merck KGaA, Darmstadt, Germany) prior to analysis.
2.4 Ultra-high pressure liquid chromatography-ESI-MS/MS identification
Five µl of each BSG extract were injected into an Acquity UPLC I-class ultra-high pressure liquid chromatography (UHPLC) system (Waters, Milford, MA, United States ) coupled to an electrospray ionization high-resolution mass spectrometer (TripleTOF 6,600 +; SCIEX, Framingham, MA, United States ) operating in both positive and negative ionisation modes. Formic acid 0.1% in water (A) and formic acid 0.1% in acetonitrile (B) were used in the following gradient: 0 min, 1% B; 4 min, 1% B; 16 min, 5% B; 35 min, 40% B; 45 min, 100% B; 50 min, 100% B; 54 min, 1% B; 60 min, 1% B. The flow was set at 0.5 ml/min and the temperature of the reverse-phase Acquity UPLC BEH C18 column (2.1 × 100 mm, 1.7 μm particle size, Waters, Milford, MA, United States ) was maintained at 50°C.
The electrospray ionisation parameters were set as follows: the source was heated at 650°C, an ion spray voltage of 4.5 and −4.5 kV was applied for the positive and negative modes, respectively, with curtain gas (nitrogen) set at 30 psi; nebulizer gas (air) at 55 psi; and turbine gas (air) at 50 psi. The declustering potential was set at 60 and −60 V in positive and negative modes, respectively. Survey scans were acquired in 175 ms for information-dependent acquisition, and only the 10 most intense monocharged precursor ions were fragmented to produce MS2 spectra in high sensitivity mode when a threshold of 100 counts per s was reached, with an accumulation time of 200 ms. The total cycle time was fixed at 2.225 s. A sweeping collision energy of 15 V below and above 15 V in positive and −15 V in negative mode was applied to all precursor ions. The dynamic exclusion was set for 2 s after three occurrences before the precursor could be fragmented again. Full high-resolution MS spectra were recorded between 100 and 2,000 mass-to-charge ratio (m/z) for MS, whereas MS2 scans were recorded between 50 and 2,000 m/z, in profile mode.
2.5 Chemical assays
2.5.1 Total phenolic content (Folin-Ciocalteu)
The total phenolic content has been measured following the method described by Singleton and Rossi (Singleton et al., 1965) with some modifications. Twenty-five μl of Folin-Ciocalteu reagent, 1 N diluted twice from a 2 N stock (Sigma Aldrich, F9252-100ML), and 50 μl of extracted samples and standard dilutions were prepared and mixed in a 96-well plate and 125 μl 7,5% w/v NA2CO3 solution were added. The plate was covered with aluminium foil and incubated for 30 min at room temperature. The absorbance was detected with a spectrophotometric microplate reader (Spark, Tecan, Männedorf, Switzerland) at 765 nm. A standard solution of gallic acid was used to build the calibration curve (0.004; 0.008; 0.0125; 0.016; 0.025; 0.03; 0.05 mg/ml) (y = 4.0529 × + 0.0407 R2 = 0.9993) and the results of total phenolic content were expressed as gallic acid equivalent (GAE). For each extraction, four biological replicates were analyzed in technical duplicates.
2.5.2 Ferric reducing/antioxidant power assay
The FRAP assay consists of the measurement of antioxidant capacity linked to the reducing power of molecules to convert Fe (III) into Fe (II) (Benzie and Strain, 1996). Ten μl of extracted samples were mixed with 190 μl of freshly prepared FRAP reagent (300 mM acetate buffer; 10 mM ferric-tripyridyltriazine; 20 mM FeCl3; pH 3.6) in a 96-well plate, covered with aluminium foil and incubated at room temperature for 20 min. A standard curve was constructed using FeSO4 at different concentrations: 0; 0.05; 0.1; 0.2; 0.4; 0.6; 0.8; 1 mM (y = 0.6787× + 0.077 R2 = 0.9981) and the results were expressed as mM of ferrous equivalent. The absorbance was detected with a spectrophotometric microplate reader (Spark, Tecan) at 595 nm. For each extraction, four biological replicates were analyzed in technical duplicates.
2.5.3 Oxygen radical absorbance capacity
The ORAC assay measures the free radical oxidation of fluorescein by the change in its fluorescence intensity. The ORAC values of the samples weremeasured using a 96-well fluorescence microplate reader (Spark, Tecan) adapting the method of Gillespie et al. (2007). Samples were diluted (100× and 25 μl diluted sample used for analysis. In each microplate well, 150 μl of fluorescein (0.08 μM of fluorescein in phosphate buffer 75 mM) and 25 μl of sample or blank (phosphate buffer 75 mM, pH7) or Trolox standards (1–20 μM) were added and mixed. The microplate was pre-incubated in the reader for 10 min at 37°C and AAPH [2,2′-Azobis (2-amidinopropane)] solution (40 mg/ml) was added. The fluorescence was recorded every 5 min for a total of 12 cycles (60 min) and raw data were exported for further calculations. Trolox was used to build the standard curve (y = 0.5745x, R2 = 0.9799) and results were expressed as μmol Trolox equivalents/g sample. For each extraction, four biological replicates were analyzed in technical duplicates.
2.6 Data analysis
For the identification of the compounds found in BSG extract, the raw positive and negative data-files were analyzed in Progenesis QI (v2.3, Nonlinear Dynamics, Newcastle upon Tyne, United Kingdom). Since the aim was to build an as broad as possible database with compounds found in BSG, no adducts were considered in the analysis. In Progenesis, runs were aligned, peaks with the same molecular weight within a specified retention time frame grouped and the peak intensity of grouped peaks normalized and used for quantification. Peaks (retention time x m/z couples) without fragmentation spectrum were filtered out as were peaks corresponding to known or suspected contaminants; the remaining RT-m/z couples were used for identification. Initially, identifications within Progenesis were done based on MS/MS similarity with the NIST and MassBank databases. Compounds not found in these databases were identified through literature search and MS/MS comparison with the following databases: LipidMaps (https://www.lipidmaps.org/); HMBD (https://hmdb.ca/spectra/ms/search) GNPS (https://gnps.ucsd.edu/ProteoSAFe/libraries.jsp); Metlin (https://metlin.scripps.edu) and PubChem (https://pubchem.ncbi.nlm.nih.gov). For other compounds, comparison between experimental and theoretical MS/MS spectra was done using MetFrag (https://ipb-halle.github.io/MetFrag/) and CFM-ID (https://cfmid.wishartlab.com/). All identifications were used to create an in-house database of compounds identified in BSG. For this an msp-type file was created and used for dereplication. An extract of this database, focused on the identified Amadori compounds, is added as supplemental file 1. Close to all identifications reported here are level 2 based on m/z of the precursor and comparison of the experimental MS/MS with experimental or predicted MS/MS spectra from the sources cited above (Salek et al., 2013).
3 Results
3.1 Compounds identified in brewer’s spent grain
BSG is mainly composed of the pericarp, coat and husks of barley grains. It is rich in sugars, lignin and proteins, although its exact composition changes based on the used barley variety, harvesting, malting and mixing time (Chetrariu and Dabija, 2020). The composition of BSG extracts likewise depends on these biological and process-related parameters (Steiner et al., 2015).
With the aim of identifying as many compounds as possible in BSG extracts and building an in-house database, 3 different extraction methods were performed using either milliQ water or milliQ water combined with 20% or 40% (v/v%) of ethanol as solvents at 21°C for 4 h. Extracts were made and analyzed with UHPLC-ESI-MS/MS, the data imported in Progenesis QI and the compounds corresponding to the most intense peaks identified. The different extractions did not result in major qualitative changes in composition, but quantitative changes were observed. The aim of the current study is however not on these relative quantitative differences, but on the profiling of BSG extracts and the identification of reaction products between amino acids and reducing sugars not commonly identified in BSG. To build a database as comprehensively as possible, multiple adducts of the same compound were considered as individual database entries.
A total of 632 compounds were identified in the BSG extracts (Figure 2), potential adducts being counted as separate compounds: 335 in negative and 297 in positive mode (Supplementary Material S2). Compounds were only considered to be identified when their m/z value matched with the predicted molecular weight and their MS/MS spectrum could be validated based on literature and/or metabolite databases (level 2 identifications). For compounds showing a series of glycosylated derivatives, similarity between the MS/MS of the parent compound and that of the glycosylated daughter needed to be attained.
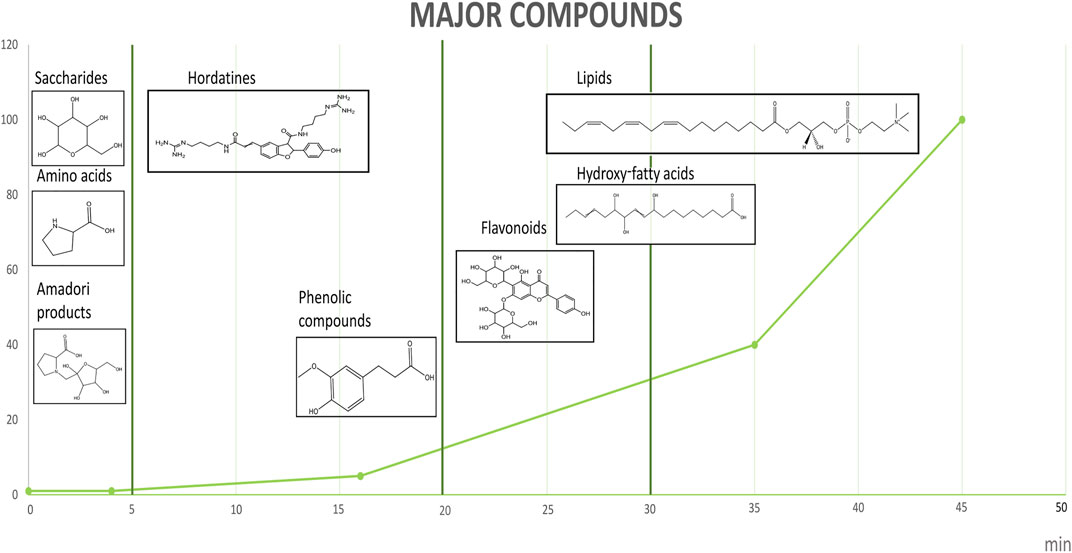
FIGURE 2. Main categories of compounds identified in brewer’s spent grain extracts with LC-MS/MS analysis. Following retention time: series of saccharides, amino acids, Amadori products, phenolics, hordatines, fatty acids, lignans and lipids.
Oligosaccharides were detected at retention times between 0 and 5 min. Oligo-hexoses containing 1 to 9 hexose residues (retention time (RT) 0.86 min m/z 1475.4741, negative mode) were identified based on m/z and MS/MS, while ions corresponding to longer oligosaccharides were detected as doubly charged but not fragmented. Free proteinogenic amino acids, as well as Amadori compounds, were also identified within the retention time frame (Table 1). Most of these reaction products between amino acids and reducing sugars have not been described in BSG extracts and represent a class of important intermediates in early stages of the Maillard reaction. These will be discussed separately. Cyclic dipeptides formed non-enzymatically under high-temperature conditions, known as diketopiperazines have been detected from 1 to 25 min. Twelve diketopiperazines containing mainly proline coupled with histidine, serine, lysine, arginine, alanine, tyrosine, leucine, phenylalanine and tryptophan have been identified (Supplementary Material S2).
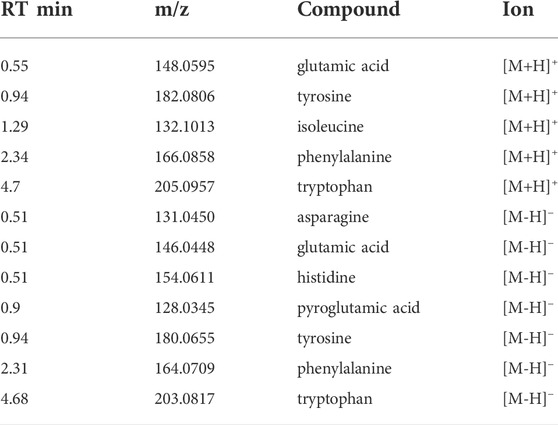
TABLE 1. Free amino acids detected in brewer’s spent grain extracts with LC-MS/MS in positive and negative ionization mode. Retention time, molecular weight-to-charge ratio and ionization mode are shown.
From 5 to 20 min, phenolics and hordatines were detected. According to Gorzolka et al. (2016), hordatines are highly abundant in BSG and present in BSG extracts (Steiner et al., 2015) made from barley based malt. Benzoxazinoids, typically found in BSG from rye or wheat based beers (Pihlava and Kurtelius, 2016; Pieczonka et al., 2021a) were not identified, in agreement with the used BSG. Hordatines are agmatine derivatives of ferulic acid and p-coumaric acid, identified in barley as homo-or heterodimers. Homodimer of p-coumaroyl-agmatine corresponds to hordatine A; homodimer of feruloyl-agmatine to hordatine C and heterodimer of feruloyl-agmatine and p-coumaroyl agmatine to hordatine B. Hordatine D, only identified during MALDI analysis of our extracts (data not shown), is a heterodimer of sinapoyl-agmatine and feruloyl-agmatine. Glycosides of hordatine A and B, known as hordatine M, and diglycosides of these two hordatines were also identified as were hydroxy- and dihydroxylated hordatine A, B, and C (Steiner et al., 2015). Furthermore, the hydroxycinnamoyl-agmatine precursors of these compounds were identified. Besides agmatine, other phenolamides, monomers and dimers of ferulic acid and p-coumaric acid conjugated to other polyamines such as putrescine and spermidine were also identified (Supplementary Material S2).
The phenolic compounds generally identified in BSG extracts were identified in the same time frame (5–20 min), namely: ferulic acid, p-coumaric acid and ± catechin. Flavones such as saponarin, luteolin-6-glucoside and dihydrokaempferol and a flavin (riboflavin, vitamin B2), were identified at higher retention times (18–25 min).
Among the BSG extracts analyzed, the 40% ethanol extract had a higher total phenolic content (TPC) (1.38 mg GAE/g DW) than extracts obtained with milliQ water and 20% ethanol (0.74 mg GAE/g DW and 0.96 mg GAE/g DW respectively) (Figure 3). The results of the analysis of the antioxidant capacity measured with the FRAP assay are in accordance with the TPC measurement since 40% ethanol extracts showed the highest antioxidant activity (6.9 mmol FeSO4/g DW 40% ethanol >3.78 mmol FeSO4/g DW 20% ethanol >2.73 mmol FeSO4/g DW milliQ water). In addition, the ORAC assay showed the same trend, confirming the extract with 40% ethanol had a higher antioxidant capacity (0.85 mmol TE/g BSG) compared to the other 2 extractions (data not shown).
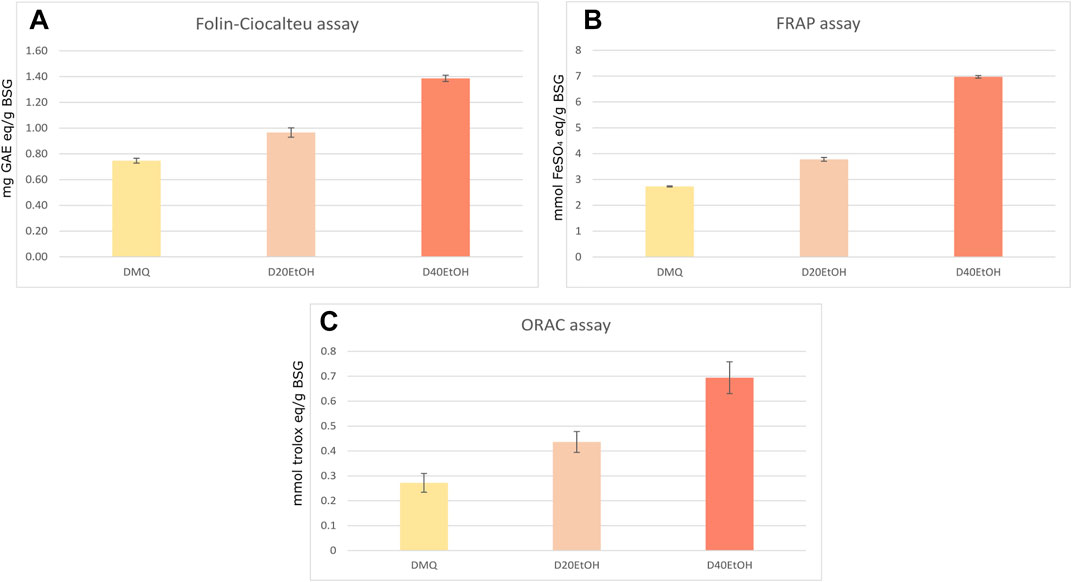
FIGURE 3. Total phenolic content (A) antioxidant capacity of brewer’s spent grain measured by FRAP (B) and by ORAC (C).
Tricin lignans and mono-, di- and trihydroxy fatty acids (18:1) to (18:3), were detected from 25 to 30 min. The highest peak in this range corresponds to hydroxylated C18:2 fatty acid, and multiple peaks correspond to di/trihydroxyoctadec-n-enoic acid (DiHOME, TriHOME) or di/trihydroxyoctadec-n,n-enoic acid (DiHODE, TriHODE) compounds. Numerous nitrogen-containing compounds are also detected. These currently unidentified compounds have a pattern of derivatisation with oligosaccharides comparable to that observed for amino acids, suggesting the presence of an amino group subject to the first stages of the Maillard reaction.
From 30 to 45 min, mono-acyl lipids were identified. Phosphatidylcholine (PC), phosphatidylethanolamine (PE) and monoacylglycerol (MG) are the main classes of lipids detected in the present study and in BSG extracts reported in literature; their acyl-composition is dominated by C18:2 and C16:0 fatty acids (Niemi et al., 2012; Fărcaş et al., 2015). Nonetheless, PC containing C14; C16; C18; C18:1; C18:2, and C18:3 fatty acids were identified in both positive and negative modes (Supplementary Table S2). Monoacyl-phosphatidylethanolamines were mainly identified as PE(18:2) and PE(16:0), both unglycated but also glycated with mono- and disaccharides (Sookwong et al., 2011; Lin et al., 2018). MG and their galactosylated forms, respectively: digalactosylmono- and diacylglycerols (DGMG and DGDG) containing C16:0 and C18:2 fatty acids were also detected.
3.2 Amadori products
As mentioned above, and known from literature, free amino acids and hexose-oligomers were identified in our extracts. Knowing the potential coupling of these compounds through the first steps of the Maillard reactions, we systematically screened our data to identify Amadori products generated by condensation between a reducing sugar and compounds containing a -NH2 group (Davidek et al., 2005b; Xue et al., 2020) (Figures 1A,B). Since glucose is the most abundant sugar monomer in malt, glucose reaction products were observed, and we identified Amadori products with most of the proteinogenic amino acids and with the non-proteinogenic amino acid gamma-aminobutyric acidgamma-aminobutyric acid (GABA). The combination of positive and negative mode analyses resulted in the identification of products of such reaction for fifteen of the twenty proteinogenic amino acids: Gly, Lys, Ala, Val, Ile/leu, Met, Tyr, Phe, Trp, Pro, Arg, His and Glu/Gln (identified as pyro-Glu as discussed below) (Tables 2, 3).
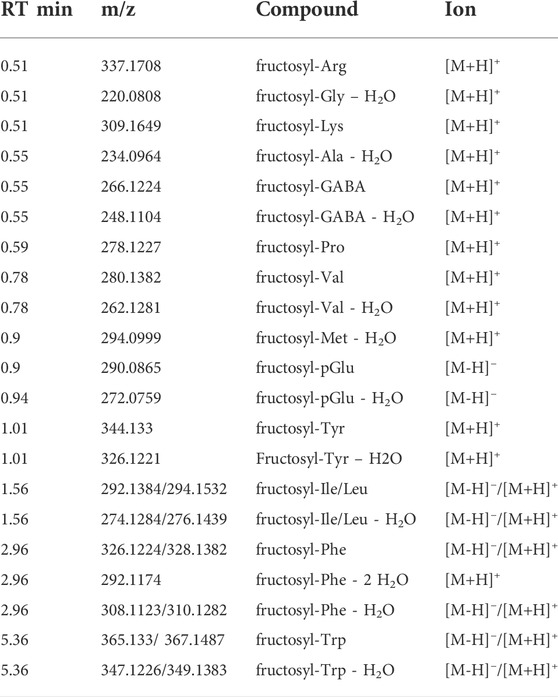
TABLE 2. Fructosyl-amino acids detected in brewer’s spent grain with LC-MS/MS in positive and negative ionization modes. Retention time, molecular weight-to-charge ratio and ionization mode are shown, amino acids are given in the three-letter code, pGlu:Pyroglutamate.
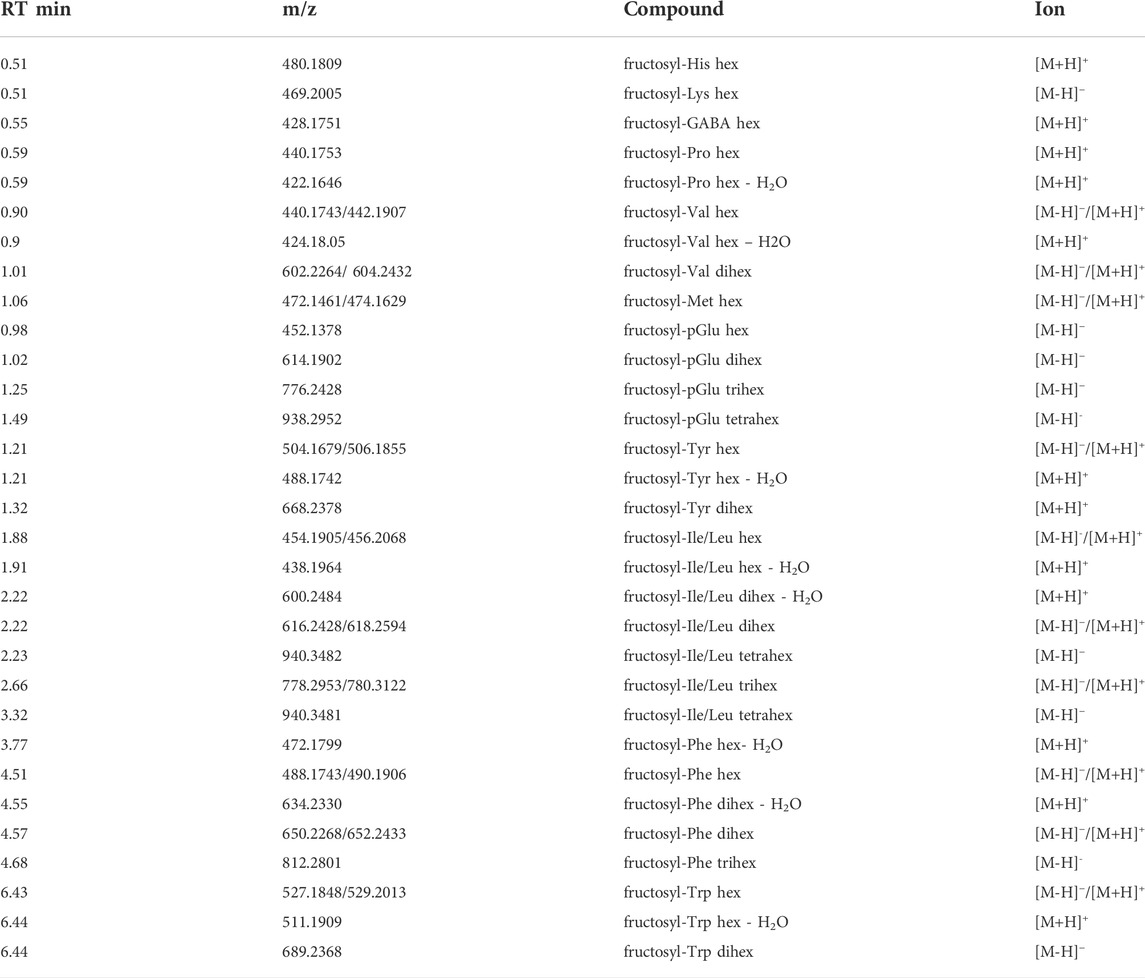
TABLE 3. [Hex]n-fructosyl-amino acids, detected in brewer’s spent grain with LC-MS/MS in positive and negative ionization mode. Retention time, molecular weight-to-charge ratio and ionization mode are shown, amino acids are given in the three-letter code, pGlu: Pyroglutamate.
In positive mode, the fragmented peaks correspond to dehydrated Amadori products for fructosyl-Gly, fructosyl-Ala, fructosyl-Met and fructosyl-Iso/Leu. In all these cases, an ion of lower intensity but identical elution profile is observed at the predicted molecular weight for the non-dehydrated compound. In MS, peaks corresponding to the neutral loss of 2 H2O and (- CO - H2O) from the non-hydrated compound are also often observed, corresponding to the typical fragmentation pattern presented in Figure 4 and resulting in spectra identical to published data (Davidek et al., 2005b). For fructosyl-Val, fructosyl-Phe, fructosyl-Trp and fructosyl-GABA, both the non-dehydrated and de-hydrated forms were fragmented and identified. Most of fructosyl-amino acids were identified in positive mode only, except for fructosyl-Ile/Leu; -Phe and -Trp, which were detected in both ionisation modes and fructosyl-pGlu that was only found in negative ionization mode.
The MS/MS spectra of detected compounds show the same fragmentation pattern: as an example of the positive mode fragmentation, the MS/MS spectrum of m/z 278.1227 RT 0.59 is given in Figure 4. This peak was identified as fructosyl-Pro based on a published fragmentation spectrum (Davidek et al., 2005b).
The pattern shows losses of 18 and 36 Da corresponding to the loss of one and two molecules of water. Afterwards, the loss of -CO combined with one, two or three water molecules results in losses of respectively 46 Da, 64 and 82 Da. Two more ions can be observed in the fragmentation profile, corresponding to the protonated amino acid and a peak at m/z of the amino acid +12 Da (Davidek et al., 2005b), corresponding to [M + H - H2O- C5H8O4]+. Lower m/z peaks corresponding to the structure of the amino acid are also observed, for instance the peak at m/z 70.0651 corresponding to the proline immonium ion in Figure 4.
In negative mode, the MS/MS fragmentation spectrum of these Amadori products with one monosaccharide has a base peak corresponding to the loss of a hexose (M-162.05). For instance, the fragmentation spectrum of fructosyl-Trp at m/z 365.13, has a base peak at m/z 203.08 [M-H -C6H10O5]-. Lower molecular weight product peaks correspond to the fragmentation pattern of the specific amino acid in negative mode. For cysteine, aspartic acid, asparagine, serine and threonine, no Maillard reaction products were identified, while the presence of fructosyl-pGlu might account for Maillard reaction products of glutamic acid and glutamine as will be discussed below.
In addition to these Amadori products resulting from the reaction between a reducing sugar monomer and the amino acid, we also identified reaction products between amino acids and longer oligosaccharides (Table 3).
Amadori products with oligosaccharides up to 5 monomers are detected, resulting in series of peaks at different retention times with an m/z shift of 162.05. Twenty-two compounds glycated with a saccharide of more than one sugar residue were detected in positive mode: 15 amino acids coupled to a disaccharide, 6 products with a trisaccharide and Ile/Leu reacted with a tetra-saccharide. In negative mode, 18 amino acids glycated with oligosaccharides were identified: 8 Amadori products with a disaccharide, 5 with a trisaccharide, 3 with tetra-saccharide and Amadori products of Ile/Leu and pGlu with a pentasaccharide. For fructosyl-Gly, fructosyl-Ala, fructosyl-Arg and fructosyl-Lys, no reaction products with more than one sugar residue were identified. An extract of the database that contains the data from the Amadori products is given in Supplementary Table S1.
In addition to Amadori products with free amino acids, fructosyl-derivatives of 7 dipeptides and 1 tripeptide were also identified. Similar to the MS/MS pattern of Amadori compounds with a single amino acid, the base peak of the fragmentation spectrum of these fructosyl-derivates corresponds to a loss of 162.05 Da ([M-H - C6H10O5]-) from the precursor ion, confirming the presence of a hexose (Figure 5B). These peptides are: pGlu-Pro; Ile/Leu-Pro; pGlu-Ile/Leu; Ile/Leu-Ile/Leu; Pro-Phe; pGlu-Glu; Leu-Pro for the dipeptides and pGlu-Pro-Gln for the tripeptide. The latter peptide sequence is abundant in grain proteins and was identified as tripeptide in wheat gluten hydrolysates (Gazme et al., 2019). The other dipeptides have previously been identified in fermented grain-based products.
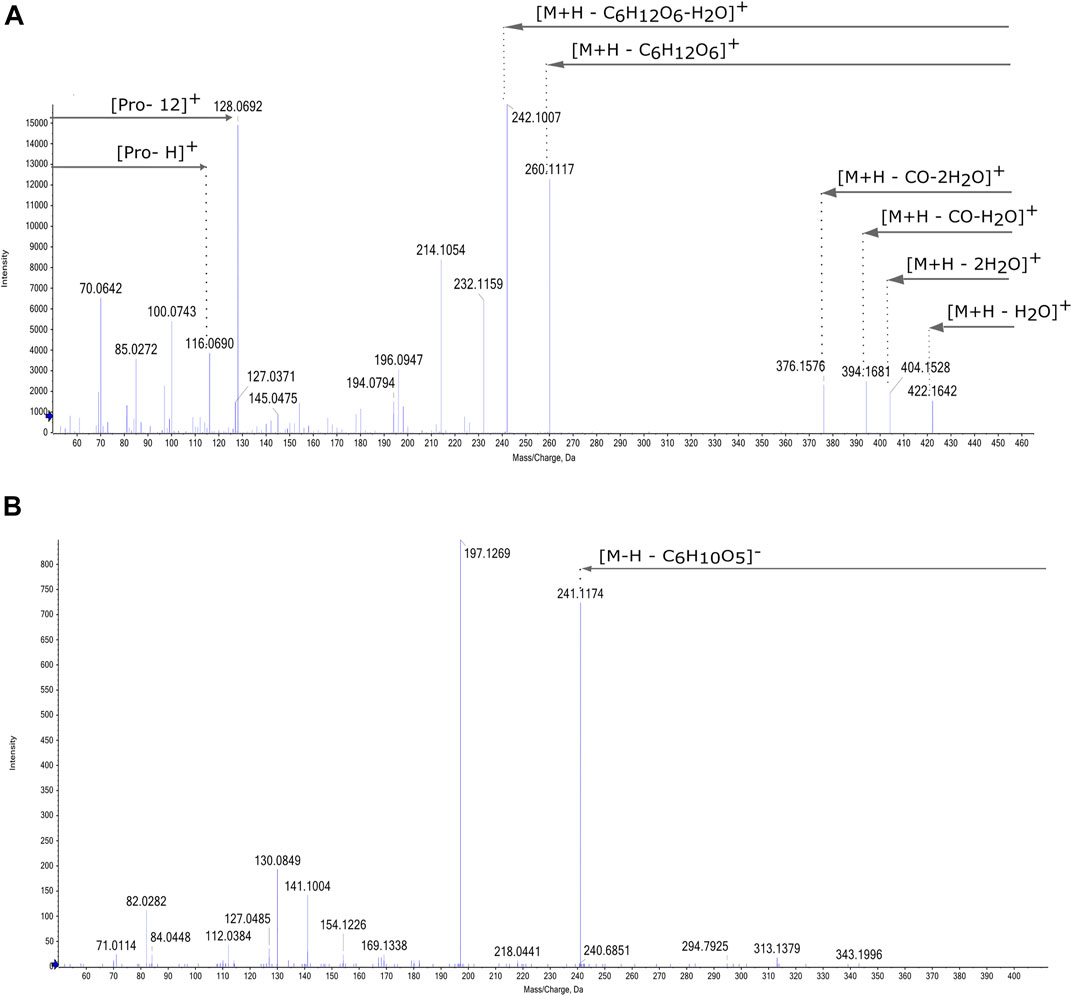
FIGURE 5. (A) MS/MS spectrum of fructosyl-proline hexoside in positive ionization mode. (B) MS/MS spectrum of fructosyl-pGlu-Ile/Leu in negative ionization mode.
Concerning products of the second stage of the Maillard reactions, pyrraline (RT 5.11 m/z 253.1179) and perlolyrine (RT 22.37 m/z 265.0963, positive mode) were identified (Chieri et al., 2019).
4 Discussion
The worldwide increase in beer production results in high amount of biomass by-products that are currently poorly valorised as low-cost cattle feed or end up in landfills. The bulk of this residual biomass is BSG, the solid residue after filtration of mashed grains (Rachwał et al., 2020). In recent years, this by-product is intensively studied as source of bioactive molecules and a protein fraction with interesting properties (Hellebois et al., 2021).
In this study, BSG has been oven-dried, because it is the most frequently used stabilisation method in research and, based on previous study (Mussatto et al., 2006), the preferred technique for industrial applications. Three solvents were used to make extracts from dried BSG: milliQ water and milliQ-ethanol mixtures (20% (v/v) and 40% (v/v)). The emphasis of the current study was on the identification of compounds and the generation of a database for use in subsequent optimisations. The global composition of the extracts is similar, and the quantitative differences observed in our dataset are those expected based on the polarity of the solvents used.
The chemical composition of the extracts is in line with previously reported analyses (Fărcaş et al., 2015; Gorzolka et al., 2016; Ikram et al., 2017). Phenolics are one of the most studied categories of compounds present in BSG extracts because of their antioxidant capacity and their benefit for human health. Ferulic acid and p-coumaric acid are the most frequently identified phenolics in BSG extracts (Meneses et al., 2013; Connolly et al., 2021). These were likewise found in our dataset both as free compound and conjugated to polyamines (putrescine, spermidine and agmatine in the identified hordatines) to form phenolamides.
The total phenolic content (TPC) and the antioxidant capacity measured in milliQ water, 20% ethanol and 40% ethanol extracts are in line with those reported in literature (Meneses et al., 2013; Socaci et al., 2018b; Bonifácio-Lopes et al., 2020). Here, the same trend is observed in the 3 chemical assays (FRAP, ORAC, TPC), the highest values are obtained for extractions with 40% EtOH and these decrease further from 20% EtOH to water. The antioxidant capacity of the 40% EtOH extract, measured with the FRAP assay, showed higher values than those reported by Meneses et al. (2013). The highest value of TPC corresponds to 1.38 mg GAE/g DW of 40% EtOH extracts, which is higher than that of a 40% EtOH extract presented by Socaci et al., 2018b (1.01 mg GAE/ g DW). Most studies agree that extractions with solvents mixtures (water:ethanol) result in extracts with higher TPC values than extractions with pure water (Meneses et al., 2013; Bonifácio-Lopes et al., 2020), as we obtained (Figure 3). Contrary to this, Almeida et al. (2017) presented data showing a decrease in TPC with increasing percentage of organic solvent in the extraction solvent. Beside ethanol, other solvent mixtures have been used to extract phenolics from BSG, some of them: 60:40 v/v acetone:water (Meneses et al., 2013); 1:80:10 v/v/v HCl:methanol:water; 0.75% NaOH:water (Moreira et al., 2013), resulted in higher TPC values [respectively, 9.90 mg GAE/g DW (Meneses et al., 2013); 4.53 mg GAE/g DW (Guido and Moreira, 2017) and 19.5 mg GAE/g DW (Moreira et al., 2013)] than those presented in this work. However, these solvents are considered not environmental friendly and limited by food-grade regulations (Chetrariu and Dabija, 2020). An alternative, greener method for BSG extraction is the application of super critical CO2 (Spinelli et al., 2016), however the TPC values reported are lower than those from solvent-based extractions (Macias-Garbett et al., 2021). In this study, we focused on the identification of Amadori products extracted from BSG. Fructosyl-Lys, free and protein bound, is the most abundant Amadori product in malt and further processing of the malt results in flavour compounds originating from fructosyl-Lys in beer (Hellwig et al., 2016). However, the fructosyl-derivatives of the other amino acids are rarely identified. We show that these products are present in BSG extracts obtained according to current practice. Moreover, we confirm that Amadori products are formed between different amino acids and oligo-saccharides, as was recently shown for N-ε-maltulosyllysine (Hellwig et al., 2016).
Amadori products were identified in processed food subjected to drying, roasting and fermentation. In dried vegetables (tomato, asparagus, carrot and celery), fructosyl-derivatives of Ile/Leu, Phe, Val, Gln, Asn, Thr, GABA, pyroglutamate, Ser, Arg, Glu, and Asp were detected (Eichner et al., 1993). In dried pepper and tomato, fructosyl-Met, fructosyl-Tyr and fructosyl-His were identified (Meitinger et al., 2014) and Amadori products also were detected in dried fruit like jujubes, raisins (Yuan et al., 2017), apricots and plums (Davidek et al., 2005b). In roasted coffee and cocoa, fructosyl-Ile/Leu, fructosyl-Phe, fructosyl-Met, fructosyl-Val, fructosyl-Tyr and fructosyl-His were found (Meitinger et al., 2014); compounds also detected in barley, wheat malt and wheat beer (Meitinger et al., 2014). In a recent study, direct injection of extracts from brewery products and analysis with a Fourier transform mass spectrometer resulted in the generation of a Maillard reaction metabolome comprising 2,800 small molecules (Pieczonka et al., 2021b). However, the resulting compound list was comprised of exact molecular weights and their associated molecular formulae and not of identified compounds. In a follow-up study, the same group compared the metabolite signatures of beers with different carbohydrate sources (wheat, barley, rice or corn) and grain-species specific compounds, but no Maillard reaction products, were identified (Pieczonka et al., 2021a).
As presented in Figure 4, the fragmentation pattern in positive mode is similar for all fructosyl-derivates. The base peaks are generated by de-hydration and the consecutive loss of -CO from the sugar molecule. The amino acid can be identified by the presence of an ion corresponding to the protonated amino acid, a peak at + 12 Da and lower molecular weight peaks due to the fragmentation of the amino acid (Davidek et al., 2005b; Meitinger et al., 2014).
In addition to these well-known compounds, Amadori products with longer oligosaccharides of up to 5 hexoses were identified: 11 AA coupled to a disaccharide; 5 to a trisaccharide, 3 to a tetrasaccharide and 2 to a pentasaccharide. In this count Ile and Leu are considered as one AA. In Figure 5A, the MS/MS spectrum of fructosyl-Pro hexoside, representative of the observed MS/MS pattern in positive mode, is shown. It is characterized by two losses of 180 and 324 from the precursor ion at m/z 440, respectively resulting in a product ions at 260.11 m/z [M + H – C6H12O6]+ and at 116.06 m/z [M + H – 2 C6H10O5]+, corresponding to proline. Lower molecular weight peaks correspond to those of the amino acid, observed as protonated ion (m/z 116.06 [AA + H]+ in Figure 4) and with the addition of 12 Da (m/z 128.07 [AA+12]+ in Figure 4) based on the annotation of Davidek et al. (2005b). In negative mode, MS/MS spectra of fructosyl-derivatives show fragment ions corresponding to the losses of hexoses from the precursor and the specific fragmentation of the amino acid. Amadori products of several dipeptides and one tripeptide were also detected, the fragmentation pattern of these compounds shows a base peak resulting from the loss of 162.05 Da from the precursor ion and further products characterizing the peptide, as shown in Figure 5B.
In our extracts, Amadori products of most proteinogenic amino acids were identified, except for glutamic acid, glutamine, aspartic acid, asparagine, threonine, serine and cysteine. In the following, the absence of these compounds will be rationalized. Given that glutamic acid and glutamine are abundant in BSG extracts (Kumari et al., 2019) and that the fructosyl-Glu and fructosyl-Gln were absent in our analysis, we hypothesize that compounds identified as fructosyl-pGlu represent the Maillard reaction products of these two amino acids (Schlichtherle-Cerny and Amadò, 2002). The data presented by Eichner et al. (1993) are in line with this and it is proposed to use the abundance of fructosyl-pGlu as representing the sum of the content of fructosyl-Glu and fructosyl-Gln (Eichner et al., 1993). The low-level detection of fructosyl-Glu in other heat-exposed biomass containing relatively high amounts of free glutamic acid, such as sweet rice wine (Inoue et al., 2016), could likewise be due to the formation of pyroglutamic acid from glutamate. Similarly, while fructosyl-Glu and nine other fructosyl-amino acids were identified in malt, fructosyl-Glu is the only one not found in the resulting beer (Wittmann and Eichner, 1989) after completion of the brewing process. Glutamine is known to be unstable: it degrades rapidly when exposed to high temperatures. At ambient temperature, Gln degrades slowly to pyroglutamate and ammonia but after 30 min of incubation at 120°C of Gln-solutions a close to complete conversion of Gln to pyroglutamate is observed (Niquet and Tessier, 2007). Prolonged exposure to high temperatures, as during mashing, may thus explain the disappearance of fructosyl-Glu observed by Wittmann and Eichner, 1989 and the fact that we do not observe fructosyl-Gln while we observe fructosyl-pGlu. Therefore, the observed pyroglutamate RT 0.90 (m/z 128.0345), fructosyl-pGlu and Amadori compounds with longer oligosaccharides are likely the sum of the contributions from Maillard reactions of both amino acids. However, specific studies on the thermal stability of fructosyl-Glu/Gln, that could confirm this hypothesis, are missing.
Why no Amadori product from asparagine is identified, although this amino acid is an important nitrogen source for yeast during the fermentation of wort (Hill and Stewart, 2019) and is present as free amino acid in BSG extracts (Table 1), is not clear. Because of its negative health effects, acrylamide, mainly formed through Maillard reactions with asparagine, is an important Maillard reaction product. However, in a recent study it was shown that no acrylamide was found in BSG extracts (Patrignani and González-Forte, 2021). Based on the latter study, the rapid formation of acrylamide from fructosyl-Asn cannot explain why we did not identify it.
No Amadori products of the two hydroxyl-containing amino acids, threonine and serine, were identified although molecular weights of known Maillard products derived from both (Noda et al., 2016) were systematically screened. In malt, fructosyl-Ser and fructosyl-Thr together with fructosyl-Gly showed the lowest concentrations compared to the other detected Amadori products (Wittmann and Eichner, 1989). Nevertheless, hydroxyl amino acids have been found to be the most reactive in the formation of fructosyl-derivatives in several studies (Piloty and Baltes, 1979; Fry and Stegink, 1982). The early elution of these compounds together with their low abundance in malt (Wittmann and Eichner, 1989) may explain why ions corresponding to these products were not observed/fragmented in this study.
Neither cysteine as free amino acid nor fructosyl-Cys were detected in our extracts. This is likely due to the high reactivity of the thiol-group with carbonyl or unsaturated carbonyl groups, inhibiting pigment formation through the Maillard cascade (Noda et al., 2016). Nonetheless, 2,4-dihydroxy-2,5- dimethyl-3(2H)-thiophenone (DHDMT), a product of the reaction between glucose and cysteine, was detected in processed food such as soy sauce, miso, beer, burnt onion, crust of bread and roasted wheat grains (Noda et al., 2016). DHDMT was not identified in the present study.
Other known Maillard reaction products, used to assess quality for instance in honey (Sanz et al., 2003) and prepared food (Yu et al., 2018), were also looked for and none of these were identified.
In our extracts, oligo-hexoses containing 1 to 9 monomers were confirmed by MS/MS (Supplementary Table S2) and oligosaccharides of up to 20 monomers were observed based on m/z alone. With this mixture of oligo-hexoses in BSG and a lack of knowledge on the impact of oligosaccharide length on the reactivity of the reducing sugar towards amino acids, we postulate that Amadori products with even longer oligosaccharides are formed. The same reasoning was recently used to explain the higher abundance of N-ε-fructosyllysine over N-ε-maltulosyllysine by the predominance of glucose over maltose in malt (Hellwig and Henle, 2020). Detailed quantitative data on the content of oligosaccharides in BSG is limited, but more information is available for malt. Although the range of data reported for each compound is broad, most likely due to biological and process-related factors, glucose is, in general, more abundant than maltose. One study reported 19.5 g/kg for glucose and 11.8 g/kg for maltose in malt (Allosio-Ouarnier et al., 2000). Since these values are given in g/kg and maltose has a higher molecular weight than glucose but also only one reducing sugar equivalent, the contribution of glucose to the Maillard reaction is expected to be several times higher than that of maltose. Furthermore, the quantity of longer oligo-hexoses was found to decrease significantly both in the previously cited study (Allosio-Ouarnier et al., 2000) and in other comparable analyses (Duke and Henson, 2008). The dominance of small saccharides over larger ones corresponds to the observed intensity of the fructosyl-AA-[Hex]n products, with Amadori products with more than 3 hexoses falling below the detection limit for most amino acids.
The identification of PE lipids with oligo-hexoses attached to the ethanolamine group furthermore indicates that a similar reaction takes place between the PE head group and reducing sugars. The formation of these products is known to occur in heat-treated food and was recently studied in detail (Lin et al., 2018). High-temperature incubation of PE with glucose resulted in the fast formation and transient accumulation of Amadori-PE, an intermediate in the slower formation of carboxymethyl-PE (CM-PE) and carboxyethyl-PE (CE-PE). These products have also been reported to be formed in vivo in red blood cells (Fountain et al., 1999) and their monitoring is indicated as important in the assessment of ageing and age-related diseases (Sookwong et al., 2011). Furthermore, the quantification of these compounds was proposed as a quality criterion for processed food (Utzmann and Lederer, 2000).
Cyclic dipeptides, known as diketopiperazines, were identified in our extracts. Diketopiperazine formation is considered a side reaction of the Maillard cascade and, as other Maillard reaction products, contributes to the taste and colour of food. Furthermore, they display a broad spectrum of bioactivities including activities sought for in BSG extracts (Borthwick and Da Costa, 2017).
Amadori products are the precursors of AGEs. The latter can alter protein structure or conformation, leading to modification in tissues by changing intermolecular links, increasing the number of free radicals and inducing inflammatory responses. These biological activities are prone to result in the development of cardiovascular diseases and diabetes (Delgado-Andrade, 2014), have a detrimental effect on cartilage and bones and result in a reduction of skin elasticity thereby promoting the ageing-process. All these physiological effects are the opposite of what food supplements are targeted at. On the other side, detailed studies of some of the compounds identified here, notably fructosyl-Arg and fructosyl-Tyr, show that they have a metal-chelating and antioxidant capacity (Ide et al., 1999; Mossine and Mawhinney, 2007). Other compounds such as fructosyl-Trp, -Tyr, -Gly, -Lys, and -Leu have a measured antitumor and anti-inflammatory activity (Mossine and Mawhinney, 2007; Lee et al., 2016). Other beneficial biological activities (anti-bacterial and anti-fungal) are likewise attributed to some of these compounds or AGE products generated from these compounds. Considering these contradicting activities for the different compounds, more studies are needed on their metabolism during digestion and their bioavailability, as well as on the effects of their long-term ingestion.
The identifications reported here indicate that in the valorisation of BSG extracts as food supplement other compounds than those generally quantified should be considered. Although some of the compounds reported here only give a low-intensity signal during MS and thus are probably of relatively low abundance, their known biological activities may make their detection and quantification important.
The data presented here show that BSG extracts, and most likely other processed food products, contain a larger diversity of Maillard reaction products than generally studied. Not only were monosaccharide Amadori products found for most proteinogenic amino acids, for dipeptides and for amino group-containing lipids, but reaction products with longer oligosaccharides were likewise identified. By these identifications in BSG, our data support the recent hypothesis that disaccharide-derived glycated amino acids other than lysine are present in malt (Hellwig and Henle, 2020).
During malting, the kilning step, high temperature drying, is the first step that may result in Amadori products (Wittmann and Eichner, 1989) followed by the mashing phase. After mashing BSG is separated from the liquid phase, which continues the brewing process. Different kilning conditions and high temperatures during mashing are essential for taste development and, given the high temperatures, contribute to the formation of Amadori products found in beer (Inns et al., 2007), and thus BSG. Furthermore, the drying step prior to extraction, considered necessary to avoid microbial degradation in most studies on BSG valorization, can affect the Amadori content and composition (Eichner et al., 1993). During mashing, free amino acids and reducing sugars are released enzymatically from malt proteins and oligosaccharides and solubilized in the wort. These free amino acids and reducing sugars are not only found in the wort but, as a comparative study on malt before mashing and on freeze-dried BSG showed (Robertson et al., 2010; Jin et al., 2022), also in BSG. While no exhaustive data on Amadori products is available on extracts from fresh, undried BSG it can thus be assumed that Amadori products identified in malt and after kilning are also present in BSG prior to drying. This however does not exclude that drying of BSG prior to the extractions done in this study contributes to the observation/abundance of these compounds. This is even highly likely knowing the presence of numerous Amadori products in dried food (Meitinger et al., 2014). Nonetheless, stabilization of BSG is considered essential due to its richness and its capacity to support microbial growth. Several methods have been used to stabilize BSG; besides oven drying, these include freeze drying or direct freezing. Although freeze-drying is considered a better technique, as the cold temperatures applied during the process maintain the BSG composition unaltered, it is not economically sustainable considering the amount of BSG biomass produced (Mussatto et al., 2006; Lynch et al., 2016). For this reason, oven drying is still the most suitable method for BSG stabilization due to its ease and lower execution costs (Mussatto et al., 2006; Krakowska-Sieprawska et al., 2022).
The biological activities of some of the Amadori products with sugar monomers identified here have been studied and often gave conflicting results from health-promoting to detrimental for health. These may thus contribute or counteract the targeted effects of food supplements based on BSG extracts. Furthermore, the biological activities of Amadori products formed with sugar oligomers is not at all known. For this reason, the quantification of some of the compounds identified here, and in general detailed studies on the composition offood supplements, may be needed to optimize the use of BSG as source of food supplements.
Data availability statement
The original contributions presented in the study are included in the article/Supplementary Material, further inquiries can be directed to the corresponding author.
Author contributions
All authors listed, have made substantial, direct and intellectual contribution to the work, and approved it for publication. MM, SC, JR, and KS performed the experiments and analysed the data. MI, YL, JR, and J-FH provided input on the experimental setup and contributed to the interpretation of the data. MM and KS acquired the funding. MM wrote the first draft of this manuscript which was read and edited by all co-authors, who agreed for submission.
Funding
The present study is part of the project BEO-FIT supported by the National Research Fund, Luxembourg (project reference 14640575).
Acknowledgments
The authors thank Valentin Ambroise, Joana Corte-Real and Laurent Solinhac for their help and technical support.
Conflict of interest
MI was employed by PM International AG.
The remaining authors declare that the research was conducted in the absence of any commercial or financial relationships that could be construed as a potential conflict of interest.
Publisher’s note
All claims expressed in this article are solely those of the authors and do not necessarily represent those of their affiliated organizations, or those of the publisher, the editors and the reviewers. Any product that may be evaluated in this article, or claim that may be made by its manufacturer, is not guaranteed or endorsed by the publisher.
Supplementary material
The Supplementary Material for this article can be found online at: https://www.frontiersin.org/articles/10.3389/frfst.2022.968865/full#supplementary-material
Abbreviations
Arg, arginine; Ala, alanine; BSG, brewer’s spent grains; Gly, glycine; Lys, lysine; GABA, gamma-aminobutyric acid; Pro, proline; Val, valine; Met, methionine; pGlu, pyroglutamic acid; Tyr, tyrosine; Ile/Leu, iso/leucine; Phe, phenylalanine; Trp, tryptophan; His, histidine; DiHOME, dihydroxyoctadec-n-enoic acid trihydroxyoctadec-n-enoic acid; TriHOME, trihydroxyoctadec-n-enoic acid; DiHODE, dihydroxyoctadec-n,n-enoic acid; TriHODE, trihydroxyoctadec-n,n-enoic acid; PC, phosphatidylcholine; PE, phosphatidylethanolamine; MG, monoacylglycerol; DGMG, digalactosylmonoacylglycerols; DGDG, digalactosyldiacylglycerols.
References
Allosio-Ouarnier, N., Quemener, B., Bertrand, D., and Boivin, P. (2000). Application of high performance anion exchange chromatography to the study of carbohydrate changes in barley during malting. J. Inst. Brew. 106, 45–52. doi:10.1002/j.2050-0416.2000.tb00039.x
Almeida, A. R., Geraldo, M. R. F., Ribeiro, L. F., Silva, M. V., Maciel, M. V. de O. B., and Haminiuk, C. W. I. (2017). Compostos bioativos do bagaço de malte: Compostos fenólicos, ácidos graxos e capacidade antioxidante in vitro. Acta Sci. - Technol. 39, 269–277.
Benzie, I. F. F., and Strain, J. J. (1996). The ferric reducing ability of plasma (FRAP) as a measure of "antioxidant power": The FRAP assay. Anal. Biochem. 239, 70–76. doi:10.1006/abio.1996.0292
Bonifácio-Lopes, T., Vilas Boas, A. A., Coscueta, E. R., Costa, E. M., Silva, S., Campos, D., et al. (2020). Bioactive extracts from brewer’s spent grain. Food Funct. 11, 8963–8977. doi:10.1039/d0fo01426e
Borthwick, A. D., and Da Costa, N. C. (2017). 2, 5-diketopiperazines in food and beverages: Taste and bioactivity. Crit. Rev. Food Sci. Nutr. 57, 718–742. doi:10.1080/10408398.2014.911142
Chetrariu, A., and Dabija, A. (2020). Brewer’s spent grains: Possibilities of valorization, a review. Appl. Sci. (Basel). 10, 5619. doi:10.3390/app10165619
Chieri, N., Kyoko, N., Akari, K., Yuko, T., and Masatsune, M. (2019). Original paper A Low-Molecular weight maillard pigment from beer was identified as perlolyrine , a maillard reaction product from tryptophan. Food Sci. Technol. Res. 25, 81–88. doi:10.3136/fstr.25.81
Connolly, A., Cermeño, M., Alashi, A. M., Aluko, R. E., and FitzGerald, R. J. (2021). Generation of phenolic-rich extracts from brewers’ spent grain and characterisation of their in vitro and in vivo activities. Innov. Food Sci. Emerg. Technol. 68, 102617. doi:10.1016/j.ifset.2021.102617
Davidek, T., Kraehenbuehl, K., Devaud, S., Robert, F., and Blank, I. (2005). Analysis of Amadori compounds by high-performance cation exchange chromatography coupled to tandem mass spectrometry. Anal. Chem. 77, 140–147. doi:10.1021/ac048925a
Davidek, T., Kraehenbuehl, K., Devaud, S., Robert, F., and Blank, I. (2005). Analysis of Amadori compounds by high-performance cation exchange chromatography coupled to tandem mass spectrometry. Anal. Chem. 77, 140–147. doi:10.1021/ac048925a
Del Castillo, M. D., Corzo, N., and Olano, A. (1999). Early stages of Maillard reaction in dehydrated orange juice. J. Agric. Food Chem. 47, 4388–4390. doi:10.1021/jf990150x
Delgado-Andrade, C. (2014). Maillard reaction products: Some considerations on their health effects. Clin. Chem. Lab. Med. 52, 53–60. doi:10.1515/cclm-2012-0823
Duke, S. H., and Henson, C. A. (2008). A comparison of barley malt quality measurements and malt sugar concentrations. J. Am. Soc. Brew. Chem. 66, 151–161. doi:10.1094/ASBCJ-2008-0612-01
Eichner, K., Reutter, M., and Wittmann, R. (1993). “Detection of Amadori compounds in heated foods,” in Thermally generated flavors. Editors T. H. Parliment, M. J. Morello, and R. J. McGorrin (Washington: FAO), 42–54. doi:10.1021/bk-1994-0543.ch005
Fărcaş, A. C., Socaci, S. A., Dulf, F. V., Tofană, M., Mudura, E., and Diaconeasa, Z. (2015). Volatile profile, fatty acids composition and total phenolics content of brewers’ spent grain by-product with potential use in the development of new functional foods. J. Cereal Sci. 64, 34–42. doi:10.1016/j.jcs.2015.04.003
Fărcaş, A. C., Socaci, S. A., Mudura, E., Dulf, F. V., Vodnar, D. C., Tofană, M., et al. (2017). “Exploitation of brewing industry wastes to produce functional ingredients,” in Brewing Technology. Editor K. Makoto (London: IntechOpen). doi:10.5772/intechopen.69231
Fountain, W. C., Requena, J. R., Jenkins, A. J., Lyons, T. J., Smyth, B., Baynes, J. W., et al. (1999). Quantification of N-(glucitol)ethanolamine and N-(carboxymethyl)serine: Two products of nonenzymatic modification of aminophospholipids formed in vivo. Anal. Biochem. 272, 48–55. doi:10.1006/abio.1999.4147
Fry, L. K., and Stegink, L. D. (1982). Formation of Maillard reaction products in parenteral alimentation solutions. J. Nutr. 112, 1631–1637. doi:10.1093/jn/112.8.1631
Gazme, B., Boachie, R. T., Tsopmo, A., and Udenigwe, C. C. (2019). Occurrence, properties and biological significance of pyroglutamyl peptides derived from different food sources. Food Sci. Hum. Wellness 8, 268–274. doi:10.1016/j.fshw.2019.05.002
Gillespie, K. M., Chae, J. M., and Ainsworth, E. A. (2007). Rapid measurement of total antioxidant capacity in plants. Nat. Protoc. 2, 867–870. doi:10.1038/nprot.2007.100
Gorzolka, K., Kölling, J., Nattkemper, T. W., and Niehaus, K. (2016). Spatio-Temporal metabolite profiling of the barley germination process by MALDI MS imaging. PLoS One 11, e0150208–25. doi:10.1371/journal.pone.0150208
Guido, L. F., and Moreira, M. M. (2017). Techniques for extraction of brewer’s spent grain polyphenols: A review. Food bioproc. Tech. 10, 1192–1209. doi:10.1007/s11947-017-1913-4
Hellebois, T., Gaiani, C., Planchon, S., Renaut, J., and Soukoulis, C. (2021). Impact of heat treatment on the acid induced gelation of brewers’ spent grain protein isolate. Food Hydrocoll. 113, 106531. doi:10.1016/j.foodhyd.2020.106531
Hellwig, M., and Henle, T. (2020). Maillard reaction products in different types of brewing malt. J. Agric. Food Chem. 68, 14274–14285. doi:10.1021/acs.jafc.0c06193
Hellwig, M., Witte, S., and Henle, T. (2016). Free and protein-bound maillard reaction products in beer: Method development and a survey of different beer types. J. Agric. Food Chem. 64, 7234–7243. doi:10.1021/acs.jafc.6b02649
Hill, A. E., and Stewart, G. G. (2019). Free amino nitrogen in brewing. Fermentation 5, 22. doi:10.3390/fermentation5010022
Hodge, E. (1955). “The Amadori rearrangement by john,” in Advances in carbohydrate chemistry and biochemistry. Editor M. L. Wolfrom (Academic Press), 169–205. doi:10.1016/S0096-533260392-6
Hofmann, T., and Schieberle, P. (2000). Formation of aroma-active Strecker-aldehydes by a direct oxidative degradation of Amadori compounds. J. Agric. Food Chem. 48, 4301–4305. doi:10.1021/jf000076e
Ide, N., Lau, B. H. S., Ryu, K., Matsuura, H., and Itakura, Y. (1999). Antioxidant effects of fructosyl arginine, a Maillard reaction product in aged garlic extract. J. Nutr. Biochem. 10 (6), 372–376. doi:10.1016/s0955-2863(99)00021-2
Ikram, S., Huang, L. Y., Zhang, H., Wang, J., and Yin, M. (2017). Composition and nutrient value proposition of brewers spent grain. J. Food Sci. 82, 2232–2242. doi:10.1111/1750-3841.13794
Inns, E. L., Buggey, L. A., Booer, C., Nursten, H. E., and Ames, J. M. (2007). Effect of heat treatment on the antioxidant activity, color, and free phenolic acid profile of malt. J. Agric. Food Chem. 55, 6539–6546. doi:10.1021/jf0710231
Inoue, Y., Katsumata, T., Watanabe, H., and Hayase, F. (2016). Mechanisms of D-amino acid formation during maturation of sweet rice wine (mirin). Food Sci. Technol. Res. 22, 679–686. doi:10.3136/fstr.22.679
Jin, Z., Lan, Y., Ohm, J. B., Gillespie, J., Schwarz, P., and Chen, B. (2022). Physicochemical composition, fermentable sugars, free amino acids, phenolics, and minerals in brewers’ spent grains obtained from craft brewing operations. J. Cereal Sci. 104, 103413. doi:10.1016/j.jcs.2022.103413
Karlović, A., Jurić, A., Ćorić, N., Habschied, K., Krstanović, V., and Mastanjević, K. (2020). By-products in the malting and brewing industries-re-usage possibilities. Fermentation 6, 82–17. doi:10.3390/FERMENTATION6030082
Krakowska-Sieprawska, A., Kiełbasa, A., Rafińska, K., Ligor, M., and Buszewski, B. (2022). Modern methods of pre-treatment of plant material for the extraction of bioactive compounds. Molecules 27, 730. doi:10.3390/molecules27030730
Kumari, B., Tiwari, B. K., Walsh, D., Griffin, T. P., Islam, N., Lyng, J. G., et al. (2019). Impact of pulsed electric field pre-treatment on nutritional and polyphenolic contents and bioactivities of light and dark brewer’s spent grains. Innov. Food Sci. Emerg. Technol. 54, 200–210. doi:10.1016/j.ifset.2019.04.012
Lee, S. H., Jeong, S. J., Jang, G. Y., Kim, M. Y., Hwang, I. G., Kim, H. Y., et al. (2016). Isolation and identification of an antiproliferative compound from fructose-tryptophan maillard reaction products. J. Agric. Food Chem. 64, 3041–3047. doi:10.1021/acs.jafc.6b00157
Lin, Q., Han, L., Liu, G., Cheng, W., and Wang, L. (2018). A preliminary study on the formation pathways of glycated phosphatidylethanolamine of food rich in phospholipid during the heat-processing. RSC Adv. 8, 11280–11288. doi:10.1039/c8ra01072b
Lynch, K. M., Steffen, E. J., and Arendt, E. K. (2016). Brewers’ spent grain: A review with an emphasis on food and health. J. Inst. Brew. 122, 553–568. doi:10.1002/jib.363
Macias-Garbett, R., Serna-Hernández, S. O., Sosa-Hernández, J. E., and Parra-Saldívar, R. (2021). Phenolic compounds from brewer’s spent grains: Toward green recovery methods and applications in the cosmetic industry. Front. Sustain. Food Syst. 5, 1–10. doi:10.3389/fsufs.2021.681684
Meitinger, M., Hartmann, S., and Schieberle, P. (2014). Development of stable isotope dilution assays for the quantitation of Amadori compounds in foods. J. Agric. Food Chem. 62, 5020–5027. doi:10.1021/jf501464g
Meneses, N. G. T., Martins, S., Teixeira, J. A., and Mussatto, S. I. (2013). Influence of extraction solvents on the recovery of antioxidant phenolic compounds from brewer’s spent grains. Sep. Purif. Technol. 108, 152–158. doi:10.1016/j.seppur.2013.02.015
Moreira, M. M., Morais, S., Carvalho, D. O., Barros, A. A., Delerue-Matos, C., and Guido, L. F. (2013). Brewer’s spent grain from different types of malt: Evaluation of the antioxidant activity and identification of the major phenolic compounds. Food Res. Int. 54, 382–388. doi:10.1016/j.foodres.2013.07.023
Mossine, V. V., and Mawhinney, T. P. (2007). Nalpha-(1-deoxy-D-fructos-1-yl)-L-histidine ("D-Fructose-L-histidine"): A potent copper chelator from tomato powder. J. Agric. Food Chem. 55, 10373–10381. doi:10.1021/jf072092i
Mussatto, S. I., Dragone, G., and Roberto, I. C. (2006). Brewers’ spent grain: Generation, characteristics and potential applications. J. Cereal Sci. 43, 1–14. doi:10.1016/j.jcs.2005.06.001
Niemi, P., Tamminen, T., Smeds, A., Viljanen, K., Ohra-Aho, T., Holopainen-Mantila, U., et al. (2012). Characterization of lipids and lignans in brewer’s spent grain and its enzymatically extracted fraction. J. Agric. Food Chem. 60, 9910–9917. doi:10.1021/jf302684x
Niquet, C., and Tessier, F. J. (2007). Free glutamine as a major precursor of Brown products and fluorophores in Maillard reaction systems. Amino Acids 33, 165–171. doi:10.1007/s00726-006-0388-9
Noda, K., Terasawa, N., and Murata, M. (2016). Formation scheme and antioxidant activity of a novel Maillard pigment, pyrrolothiazolate, formed from cysteine and glucose. Food Funct. 7, 2551–2556. doi:10.1039/c5fo01625h
Oladokun, O., James, S., Cowley, T., Dehrmann, F., Smart, K., Hort, J., et al. (2017). Perceived bitterness character of beer in relation to hop variety and the impact of hop aroma. Food Chem. 230, 215–224. doi:10.1016/j.foodchem.2017.03.031
Patrignani, M., and González-Forte, L. del S. (2021). Characterisation of melanoidins derived from brewers’ spent grain: New insights into their structure and antioxidant activity. Int. J. Food Sci. Technol. 56, 384–391. doi:10.1111/ijfs.14653
Pieczonka, S. A., Hemmler, D., Moritz, F., Lucio, M., Zarnkow, M., Jacob, F., et al. (2021). Hidden in its color: A molecular-level analysis of the beer’s maillard reaction network. Food Chem. 361, 130112. doi:10.1016/j.foodchem.2021.130112
Pieczonka, S. A., Paravicini, S., Rychlik, M., and Schmitt-Kopplin, P. (2021). On the trail of the German purity law: Distinguishing the metabolic signatures of wheat, corn and rice in beer. Front. Chem. 9, 715372. doi:10.3389/fchem.2021.715372
Pihlava, J., and Kurtelius, T. (2016). Determination of benzoxazinoids in wheat and rye beers by HPLC-DAD and UPLC-QTOF MS. Food Chem. 204, 400–408. doi:10.1016/j.foodchem.2016.02.148
Piloty, M., and Baltes, W. (1979). Untersuchungen zur Reaktion von Aminos iuren mit -Dicarbonylverbindungen. Z. Leb. Unters. Forch. 168, 368–373. doi:10.1007/BF01186494
Rachwał, K., Waśko, A., Gustaw, K., and Polak-Berecka, M. (2020). Utilization of brewery wastes in food industry. PeerJ 8, e9427–28. doi:10.7717/peerj.9427
Robertson, J. A., Anson, K. J. A. I., Treimo, J., Faulds, C. B., Brocklehurst, T. F., Eijsink, V. G. H., et al. (2010). Profiling brewers ’ spent grain for composition and microbial ecology at the site of production. LWT - Food Sci. Technol. 43, 890–896. doi:10.1016/j.lwt.2010.01.019
Salek, R. M., Steinbeck, C., Viant, M. R., Goodacre, R., and Dunn, W. B. (2013). The role of reporting standards for metabolite annotation and identification in metabolomic studies. Gigascience 2, 13. doi:10.1186/2047-217X-2-13
Sanz, M. L., Del Castillo, M. D., Corzo, N., and Olano, A. (2003). 2-Furoylmethyl amino acids and hydroxymethylfurfural as indicators of honey quality. J. Agric. Food Chem. 51, 4278–4283. doi:10.1021/jf021235s
Schlichtherle-Cerny, H., and Amadò, R. (2002). Analysis of taste-active compounds in an enzymatic hydrolysate of deamidated wheat gluten. J. Agric. Food Chem. 50, 1515–1522. doi:10.1021/jf010989o
Singleton, V. L., Rossi, J. A., and Rossi, J. A. (1965). Colorimetry of total phenolics with phosphomolybdic-phosphotungstic acid reagents. Am. J. Enol. Vitic. 16, 144–158. http://www.ajevonline.org/cgi/content/abstract/16/3/144.
Socaci, S. A., Fărcaş, A. C., Diaconeasa, Z. M., Vodnar, D. C., Rusu, B., and Tofană, M. (2018). Influence of the extraction solvent on phenolic content, antioxidant, antimicrobial and antimutagenic activities of brewers’ spent grain. J. Cereal Sci. 80, 180–187. doi:10.1016/j.jcs.2018.03.006
Socaci, S. A., Fărcaş, A. C., Diaconeasa, Z. M., Vodnar, D. C., Rusu, B., and Tofană, M. (2018). Influence of the extraction solvent on phenolic content, antioxidant, antimicrobial and antimutagenic activities of brewers’ spent grain. J. Cereal Sci. 80, 180–187. doi:10.1016/j.jcs.2018.03.006
Sookwong, P., Nakagawa, K., Fujita, I., Shoji, N., and Miyazawa, T. (2011). Amadori-glycated phosphatidylethanolamine, a potential marker for hyperglycemia, in streptozotocin-induced diabetic rats. Lipids 46, 943–952. doi:10.1007/s11745-011-3588-3
Spinelli, S., Conte, A., Lecce, L., Padalino, L., and Del Nobile, M. A. (2016). Supercritical carbon dioxide extraction of brewer’s spent grain. J. Supercrit. Fluids 107, 69–74. doi:10.1016/j.supflu.2015.08.017
Steiner, J., Procopio, S., and Becker, T. (2015). Brewer’s spent grain: Source of value-added polysaccharides for the food industry in reference to the health claims. Eur. Food Res. Technol. 241, 303–315. doi:10.1007/s00217-015-2461-7
Troise, A. D., Wiltafsky, M., Fogliano, V., and Vitaglione, P. (2018). The quantification of free Amadori compounds and amino acids allows to model the bound Maillard reaction products formation in soybean products. Food Chem. 247, 29–38. doi:10.1016/j.foodchem.2017.12.019
Utzmann, C. M., and Lederer, M. O. (2000). Identification and quantification of aminophospholipid-linked Maillard compounds in model systems and egg yolk products. J. Agric. Food Chem. 48, 1000–1008. doi:10.1021/jf9911489
Wittmann, R., and Eichner, K. (1989). Nachweis von Maillard-Produkten in Malzen, Bieren und Braucouleuren. Z. Leb. Unters. Forch. 188, 212–220. doi:10.1007/BF02112877
Xue, C., Shi, Z., He, Z., Wang, Z., Qin, F., Chen, J., et al. (2020). formation of three selected AGEs and their corresponding intermediates in aldose- and ketose-lysine systems. eFood 1, 270–278. doi:10.2991/efood.k.200508.001
Yu, J., Zhang, S., and Zhang, L. (2018). Evaluation of the extent of initial Maillard reaction during cooking some vegetables by direct measurement of the Amadori compounds. J. Sci. Food Agric. 98, 190–197. doi:10.1002/jsfa.8455
Keywords: BSG, amadori products, glycation, maillard reaction, by-product, bioactive compounds
Citation: Minestrini M, Charton S, Iken M, Larondelle Y, Renaut J, Hausman J-F and Sergeant K (2022) Identification of amadori products and oligosaccharide-glycated amino acids in brewer’s spent grain extract. Front. Food. Sci. Technol. 2:968865. doi: 10.3389/frfst.2022.968865
Received: 14 June 2022; Accepted: 14 July 2022;
Published: 30 August 2022.
Edited by:
Majid Nooshkam, Ferdowsi University of Mashhad, IranReviewed by:
Moein Bashash, Ferdowsi University of Mashhad, IranBehrooz Alizadeh Behbahani, Ramin Agriculture and Natural Resources University of Khouzestan, Iran
Copyright © 2022 Minestrini, Charton, Iken, Larondelle, Renaut, Hausman and Sergeant. This is an open-access article distributed under the terms of the Creative Commons Attribution License (CC BY). The use, distribution or reproduction in other forums is permitted, provided the original author(s) and the copyright owner(s) are credited and that the original publication in this journal is cited, in accordance with accepted academic practice. No use, distribution or reproduction is permitted which does not comply with these terms.
*Correspondence: Kjell Sergeant, a2plbGwuc2VyZ2VhbnRAbGlzdC5sdQ==