- 1Julius Kühn Institute—Federal Research Centre for Cultivated Plants, Institute for Ecological Chemistry, Plant Analysis and Stored Product Protection, Berlin, Germany
- 2Institute of Pharmacy, Freie Universität Berlin, Berlin, Germany
- 3Consulting & Project Management for Medicinal and Aromatic Plants, Stahnsdorf, Germany
To understand how peppermint responds to different LED light qualities during the early vegetative phase, peppermints were illuminated with three different LED light conditions (RB = Red/Blue, RGB = Red/Green/Blue, SUN = artificial sunlight closely resembling the terrestrial sunlight spectrum between 380 and 780 nm) in an automated vertical cultivation system. RB resulted in compact growth, whereas both green-containing lighting conditions induced excessive stem and side branch elongations and significant leaf expansions. Although peppermint plants achieved marketable appearances regardless of lighting condition, essential oil (EO) compositions with highly elevated amounts of pulegone and menthofuran did not meet consumer safety requirements. Both artificial SUN and RB spectra showed lower concentrations of pulegone in the EO at 41 and 43%, respectively, than detected under RGB at more than 49%. Reasons for this undesirable EO composition are discussed as a result of the lighting conditions applied and the early harvest time, leading to an incomplete reduction of pulegone to menthone during biosynthesis. Based on these findings, aromatic peppermint cultivation under LEDs can be improved to meet regulatory requirements and highlights the need for analytical quality controls regarding consumer safety to evaluate the applicability of LED lighting for fresh herb productions.
1 Introduction
As modern agriculture is challenged by its own negative environmental impacts and climate change, indoor vertical farms are increasingly seen as an alternative food production system as they could principally enable an increased supply of high-quality foods on a regional year-round scale (Orsini et al., 2020). Once optimal lighting strategies (as well as efficient water, nutrient and climate control systems) become available and comprehensively integrated, vertical farms are expected to become a viable and sustainable food production system for a wide range of crops (Kozai 2019).
In the last decade, light-emitting diodes (LEDs) proved to be highly versatile and energetically efficient lighting systems, and their technical as well as spectral advantages over traditional light sources for plant cultivation have been well described (Eichhorn-Bilodeau et al., 2019).
As red (R) and blue (B) wavebands are maximally absorbed by the plant’s light-capturing chlorophylls (McCree 1972), most LED studies remain focused on different RB ratios to optimize plant growth, morphology and physiological responses (e.g., Pennisi et al., 2019). However, addition of other wavelengths shows potential to improve plant traits of interest even further. For example, addition of far-red wavelengths (FR, > 700 nm) can increase net photosynthesis (Park and Runkle 2017; Kalaitzoglou et al., 2019), and ultraviolet radiation (UV, < 400 nm) can promote the accumulation of certain secondary metabolites (Behn et al., 2010; Rechner et al., 2017). Green (G) light (400–500 nm) was reported to increase chlorophyll contents (Saengtharatip et al., 2020) and carbon assimilations (Terashima et al., 2009), and the latter seems to be a consequence of the G lights’ capability to penetrate deeper into the canopy than R and B wavelengths (Terashima et al., 2009). Thus, scientists hypothesized that addition of G light could potentially increase plant yields under artificial lighting systems (Sun et al., 1998; Folta 2004; Smith et al., 2017). So far however, this theoretical G light potential has only been shown for basil and tomato (Kaiser et al., 2019; Schenkels et al., 2020). In these two studies, partial replacements of R and B with G wavelengths resulted in increased biomasses, stem lengths and leaf areas.
In addition, recent LED studies using broad light spectra covering the whole range of radiation relevant for plant development (300–800 nm) suggest further improvements as greater plant yields and photosynthetic rates are found when compared to dichromatic spectra (Kozai, 2019).
Peppermint (Mentha x piperita L.), a medicinal and aromatic plant from the Lamiaceae family, does not only demonstrate a plethora of therapeutic activities, inducing antioxidant, antispasmodic, antiseptic, antibacterial, antiviral, anticarcinogenic, antitumorigenic, antiallergic, anti-inflammatory, antifungal, antimutagenic and antinauseant properties. It is also widely used as a tea infusion, culinary herb and spice, in confections, as an aromatic flavoring agent, as well as in the cosmetic, personal hygiene and perfumery industry for its fragrance properties (Malekmohammad et al., 2019).
Although peppermint represents one of the most important essential oil-bearing herbal plants worldwide (Mahendran and Rahman 2020), there is only limited knowledge about suitable supplemental lighting programs for its cultivation. Though based on experiments conducted with diverse lighting technologies, light intensities ranging from 113 to 1,200 μmol m−2 s−1 have been suggested for different mint developmental stages (Behn et al., 2010; Sabzalian et al., 2014; Alvarenga et al., 2018), and photoperiods of 14–16 h have been reported to increase the essential oil (EO) content and quality of Mentha x piperita L. (Burbott and Loomis 1967; Clark and Menary 1980). Further, UV added to white background lighting (W) as well as monochromatic R light have been shown to increase EO contents in peppermint (Behn et al., 2010; Sabzalian et al., 2014). However, monochromatic B light as well as B light added to W decreased the EO content in Mentha x piperita L. (Maffei and Scannerini 1999; Sabzalian et al., 2014). Best results in terms of plant growth and EO content were achieved under RB when compared to monochromatic R and B light supplied by LEDs (Sabzalian et al., 2014); however, the study does not include information on the composition and thus, quality of the EO, which is predominantly determined by the quantity and composition of its monoterpenoid constituents. Here, 30–55% of menthol, 14–32% of its precursor menthone and low levels of pulegone (< 4%), menthofuran (1–9%) and methyl acetate (2.8–10%) are considered peppermint EOs of high quality (Schmiedel 2008).
Related to the above-mentioned peppermint cultivation conditions and plant developmental improvements, we conducted an LED-based lighting experiment in a vertical cultivation system applying three different light qualities at equal photon flux density. The aim of this study was to investigate whether a partial replacement of R and B with G light (RGB) or a broad white light spectrum including UV and FR (SUN) results in the recently suggested improvements in terms of biomass accumulation and morphological traits in Mentha x piperita L. when compared to the commonly applied dichromatic RB spectrum. Even though it is known that cultivation conditions strongly impact the quality of peppermint EO, no data is currently available on the influence of LED light spectra on its composition (Salehi et al., 2018). Therefore, we investigated the peppermints’ EO composition with special emphasis on the harmful metabolites pulegone and menthofuran which are synthesized during early development in mint leaves grown under insufficient light intensities (Croteau et al., 2005; Rios-Estepa et al., 2008).
2 Materials and Methods
2.1 Experimental Design
To investigate morphology, biomass yields and EO composition of peppermint samples under three different spectral lighting conditions, a one-factorial experiment with a randomized block design and three spatially independent replications per light treatment (N = 270; n = 30 peppermint plants per replication) was conducted in three vertical three-leveled cultivation systems at the same time.
2.2 Plant Material and Growth Conditions
Stock plants of Mentha x piperita var. piperita cv. Multimentha were cultivated at Julius Kühn-Institute (JKI), Institute for Breeding Research on Horticultural Crops (51.8°N, 11.1°E) under field conditions. At JKI, Institute for Ecological Chemistry, Plant Analysis and Stored Product Protection, stolon segments (5 cm) from one established stock plant (to ensure genetically identical plants) were cultivated in propagator trays filled with potting substrate (Fruhstorfer Einheitserde Type P, HAWITA, Vechta, Germany) in a greenhouse (∼22°C) under a natural average daily light integral (DLI) of 12.6 mol m2 (based on weather recordings from WetterKontor (Kontor, 2021)) starting April 10th 2019. After sprouting, uniform nodal segments with three equally developed leaf pairs were transferred to 128-cell plug trays (Ø 4 cm) filled with the same potting substrate, and covered with a plastic hood (light transmittance ≥90%, data not shown) to promote rooting on 6 May 2019 (26 days of cultivation (DOC)) in the greenhouse (∼22°C) under natural light conditions. After 10 days, on 16 May 2019 (36 DOC), 270 representative plantlets were transplanted into pots (Ø 12 cm) containing 0.69 L of substrate with an elevated nutrient composition (Fruhstorfer Einheitserde Type T, HAWITA, Vechta, Germany) and evenly placed on each of nine cultivation shelfs (lined with water-permeable drainage fleece to avoid clogging of the watering system) under the LED lighting fixtures, resulting in 30 plants per cultivation shelf. In the nine available cultivation shelfs, three light treatments were set up three times (30 peppermint plants per cultivation shelf amounted to a total of 90 peppermint plants per light treatment), resulting in three independent experimental replications per light treatment (Supplementary Figure S1). To induce branching, the youngest (not fully developed) leaf pair of each peppermint was pinched on 29 May 2019 (49 DOC). Daily, peppermints were automatically irrigated via submersion of plant pots from 9:00 to 9:15 a.m. Electrical conductivity (EC), oxygen content (O2), pH and temperature (°C) of the water were analyzed with the multi-parameter measuring device WTW Multi3430 (Weilheim, Germany) twice a day on the first four consecutive days of the experiment (n = 8): EC = 553.5 ± 28.4 μS cm−1, O2 = 8.0 ± 2.0 mg L−1, pH = 7.6 ± 0.2 and °C = 22.2 ± 4.1. After refilling the water tank on 12 June 2019, the water was measured twice with the same device (EC = 735 ± 3.55 μS cm−1, O2 = 5.9 ± 0.7 mg L−1, pH = 7.5 ± 0.1 and °C = 25.6 ± 0.5). The same day, peppermints were fertilized with NPK (15-5-15) nutrient solution (Phytogrow) supplied by GND Solutions GmbH (Berlin, Germany) by diluting 75 g of fertilizer in the tank. As the fertilized water was reused daily by the automated watering system, the peppermints were fertilized daily until the end of the experiment (18 June 2019). Salinity measurements were conducted directly in moist pot substrates with an activity measuring instrument PNT 3000 (STEP Systems GmbH, Nuremburg, Germany) once before fertilization (0.23 ± 0.02 g L−1) and four times (every other day) after fertilization (0.31 ± 0.02, 0.30 ± 0.04, 0.32 ± 0.03, 0.35 ± 0.03 g L−1; measurements took place in two pots per light treatment and replication (n = 18) to assure optimal fertilization status. To eliminate aphids detected on 27 May 2019, Chrysoperla carnea larvae (n ∼ 1,000) and Aphidius matricariae (n ∼ 1,000; recommended for 250 m2) supplied by Katzbiotech (Baruth, Germany) were applied on 29 May 2019, and resulted in aphid elimination before 11 June 2019. Climatic conditions were continuously monitored at canopy level via data loggers (EL-USB-2, Lascar, CONRAD, Hirschau, Germany). Average temperatures (C° ± standard deviation) under RB, RGB and SUN were 25.6 ± 2.9, 25.5 ± 2.8 and 25.5 ± 2.8, with a measuring accuracy of ±1°C. Average humidities (%RH ± SD) under RB, RGB and SUN were 64.0 ± 7.0, 67.1 ± 6.8 and 65.3 ± 6.9 with a measuring accuracy of 2.25 %RH. None of the climatic conditions differed between treatments. For control, genetically identical stolon segments of Mentha x piperita L. var. piperita cv. Multimentha were grown under field conditions (Table S1).
2.3 Cultivation System
Each of the three LED cultivation systems was 1.85 m high and consisted of three shelves (124 × 55 × 60 cm). For automated ebb-and-flow-watering (and nutrient supply), the interconnected vertical LED cultivation systems were connected to a water tank with a capacity of 300 L. Before water entered back into the water tank for re-use, a UV-filter sterilized the back-flowing water after submersive irrigation was completed.
2.4 Lighting Systems and Illumination Conditions
Two supplemental LED lighting systems from FUTURELED GmbH, Berlin, Germany (Apollo R1 and Lumitronics Air) were used to set up three spectral lighting conditions (RB = Red/Blue, RGB = Red/Green/Blue, SUN = artificial sunlight) at equal photon flux density (PFD). At start, all plants were illuminated with a PFD of 150 μmol m−2 s−1 (at plant pot level). Under exclusion of natural daylight, plants were subjected to LED lighting in a growth room from 6:00 a.m. to 10:00 p.m. for a daily photoperiod of 16 h during the experiment.
2.5 Irradiance Measurements
Irradiance measurements were taken using a spectral PAR meter (PG200N, UPRtek, Aachen, Germany). Spectral composition, light intensities and photon distribution were measured and recorded at plant pot level under trial conditions prior to the experiment (Figure 1; Table 1). The software package of the spectrometer (uSpectrum PC laboratory software) automatically calculated electromagnetic parameters including photon flux density (PFD in μmol m−2 s−1) between 380 and 780 nm, photosynthetic photon flux density (PPFD in μmol m−2 s−1) between 400 and 700 nm, amounts of ultraviolet (380–400 nm), blue (400–500 nm), green (500–600 nm), red (600–700 nm) and far-red (700–780 nm) radiation (in μmol m−2 s−1) and the ratios of blue-to-green and red-to-far-red light. Additionally, red-to-blue light ratio was calculated.
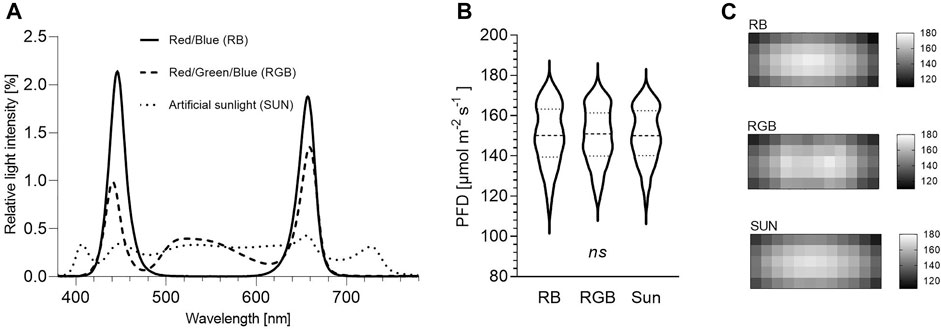
FIGURE 1. Irradiance measurements of three light spectra between 380 and 780 nm at plant pot level. RB = Red/Blue, RGB = Red/Green/Blue, SUN = artificial sunlight spectra. (A) Relative spectral composition ∗. (B) Light intensity ∗∗. (C) Photon distribution ∗∗∗. ∗ Depicted are the relative light intensities [%] per wavelength [nm] between 380 and 780 nm of the RB, RGB and SUN light spectra used during the experiment. ∗∗ Presented are photon flux densities (PFD) [µmol m−2 s−1] measured every 100 cm2 within each cultivation shelf underneath RB, RGB and SUN light spectra (n = 60 per light spectra). Violin plot represents median (dashed line) and quartiles (dotted lines). Ordinary one-way ANOVA did not detect significant differences among means of light treatments (p > 0.99). ∗∗∗ Visualized are light distribution patterns measured every 100 cm2 within each cultivation shelf underneath RB, RGB and SUN light spectra (n = 60 per light spectra). Each heat map depicts the photon flux densities (PFD) [µmol m−2 s−1].
2.6 Crop Measurements and Harvest
After the beginning of the light experiment, plant height (measured from top of soil to tip of apical bud) and number of leaf pairs (≥1 cm) were assessed weekly in 7-day intervals at 41, 48, 55, 62 and 69 days of culture (DOC). Side shoot lengths were also assessed weekly in 7-day intervals at 55, 62 and 69 DOC by continuously measuring the lengths of the first fully developed side branches. For data analysis, these two side branch measurements were averaged per peppermint plant. Before harvest on 69 DOC, four peppermint leaves from 10 randomly selected plants per replicate (N = 90, n = 30 per light treatment) were scanned (CanoScan LiDE 400) to measure the leaves’ width and length via ImageJ software (Version 1.52a). Thereby, the first, third, fifth and seventh peppermint leaf from the apex was removed. Additionally, all 270 experimental peppermint plants were harvested separately under each treatment and replication to analyze the effects of treatments on biomass yields. Therefore, fresh matter of aerial plant parts was individually recorded at harvest and total dry matter was measured after drying the samples in a circulated drying oven at 30°C for ≤ 7 days until stable mass was attained (optimal drying method was chosen and standardized as it highly influences peppermints’ EO yields (Beigi et al., 2018). After removal of shoots, leaf dry matter (LDM) was determined for all plants. Dried leaf samples were vacuum-sealed (V.300®, Landig + Lava GmbH & Co. KG, Bad Saulgau, Germany) and stored in the dark at 4°C until further processing.
2.6 Essential Oil Isolation
Five gram of air-dried peppermint leaves (combining LDM of three to four plant samples of the same spatial replication) were ground and hydrodistilled for 60 min using a clevenger-type apparatus. Volume (ml) of the isolated EO were recorded and stored at −70°C in sealed glass vials for further processing. The EO volume was density-corrected according to the European Pharmacopoeia with factor 0.908 (Schmiedel 2008). EO content and yield, expressed on a dry weight basis, were calculated according to the following equations: EO content (%) = (distilled EO (g)/5 g) x 100; EO yield (mg g leaf dry matter−1 (LDM)) = mean of EO yield (mg g LDM−1) (n = 8 per spatial replication) x total LDM (g). To determine a possible influence of the temporary aphid infestation on the chemical composition of the monoterpenes, volatiles were extracted according to the procedure described in Tabbert et al., 2021.
2.7 GC-FID and GC-MS Analysis
1 µl of EOs was diluted in isooctane (1:1,000) [containing 1:40,000 (v/v) carvacrol as internal standard] and transferred into GC-vials. 3 µl of each sample was analyzed by GC–FID using an Agilent gas chromatograph 6890N fitted with an HP-Innowax column (30 m × 250 μm × 0.5 μm) in split mode (split ratio 10:1). Detector and injector temperatures were set to 250°C. The following oven temperature program was used: 50°C for 2 min, heating from 50 to 210°C at a rate of 3°C min−1. The final temperature was held for 6 min. Hydrogen was used as carrier gas with a constant flow rate of 1.2 ml min−1. GC-MS was performed using an Agilent 5,973 Network mass spectrometer, on an HP-Innowax column (see GC), operating at 70 eV ionization energy, using the same temperature program as above. Helium was used as carrier gas with a constant flow rate of 1.2 ml min−1. Retention indices were calculated by using retention times of C7-C40-saturated alkanes (Merck KGaA, Darmstadt, Germany) that were injected under the same chromatographic conditions.
2.8 Identification and Quantification of Essential Oil Compounds
All main compounds of the EOs were identified by comparing their mass spectra with those of the National Institute of Standards and Technology (NIST) mass spectral library and confirmed by comparing their retention indices. Additionally, identification of 1-octen-3-ol, 1,8-cineole, 3-octanol, α-pinene, α-terpineol, β-caryophyllene, β-farnesene, β-myrcene, β-pinene, γ-terpinene, caryophyllene oxide, limonene, germacrene D, iso-menthone, linalool, menthofuran, menthol, menthone, menthyl acetate, neo-menthol, ocimene, piperitenone, piperitone, pulegone, sabinene, terpinen-4-ol and viridiflorol was affirmed by authentic reference materials (RMs) with a purity of at least 95%. For compounds not verified by RMs (cis-isopulegone, trans-isopulegone, δ-terpineol and trans-verbenol), accuracy of internal reference library identification was ≥78%. EO compounds were quantified based on the known concentration of the internal standard (carvacrol).
2.9 Statistical Analysis
Statistical analyses were performed using GraphPad Prism 8.4.3.686 (San Diego, United States). Spectral light distribution data passed normality via D’Agostino & Pearsons omnibus normality test, and thus were analyzed via ordinary one-way ANOVA (p > 0.99, not significant). Collected data sets of plant height, side branch length, total plant fresh and dry weight, leaf and shoot dry weight (N = 270, n = 30 peppermint plants per replication, respectively) as well as of leaf length and leaf area (N = 90, n = 30 peppermint plants per replication, respectively) and data sets of EO compounds as well as of EO contents (N = 72, n = 8 EOs per replication, respectively) and data sets of EO yields ((N = 9, n = 3 per light treatment) of each spatial replication (n = 3) and light treatment (n = 3) were tested for normality via D’Agostino & Pearsons omnibus normality and Shapiro-Wilk test. If normality test failed, outliers were identified via ROUT method (Q ≤ 10%) and removed to establish normality of all data sets. Plant height, side branch length, length and width of fresh leaves, EO compounds and contents: Nested one-way ANOVA was used to detect differences between light treatments (nested factor: spatial replications per light treatment (n = 3). When significant (p ≤ 0.05), Tukey’s multiple comparisons test at 95% confidence interval was applied. Equal variances of data sets were visually checked by graphing homoscedasticity plots. Fresh matter, dry matter and leaf dry matter yields: Due to significant differences between spatial replications (p ≤ 0.05), means of each spatial replication and light treatment (N = 9, n = 3 per light treatment) were used to conduct an ordinary one-way ANOVA. Number of leaf pairs: Means of each spatial replication per light treatment (n = 3) were analyzed via Kruskal–Wallis nonparametric test. (Additionally, amounts of main volatiles (menthone, pulegone, menthofuran) of uninfested and temporarily aphid-infested peppermint plants (N = 58) were compared via unpaired t-test and did not differ (p ≥ 0.8), ruling out an influence of aphids on EO composition.)
3 Results
3.1 Plant Height
After 2 weeks, peppermint grown under SUN and RGB exceeded plant heights reached under RB (p = 0.03 respectively), averaging 19.4 ± 1.0, 22.2 ± 0.6 and 23.5 ± 1.6 cm under RB, RGB and SUN, respectively. With average plant heights of 33.0 ± 0.7 and 34.5 ± 1.3 cm, peppermints grown under RGB and SUN were significantly taller than peppermints grown under RB (27.2 ± 1.2 cm) after 3 weeks of cultivation (p = 0.01). Difference in plant height further increased between aforementioned light spectra after 4 weeks of the experiment (p ≤ 0.01). While peppermints grown under RGB and SUN reached average plant heights of 44.2 ± 1.0 and 47.0 ± 0.3 cm respectively, peppermints grown under RB remained significantly shorter with average heights of 35.1 ± 1.2 cm (Figure 2A).
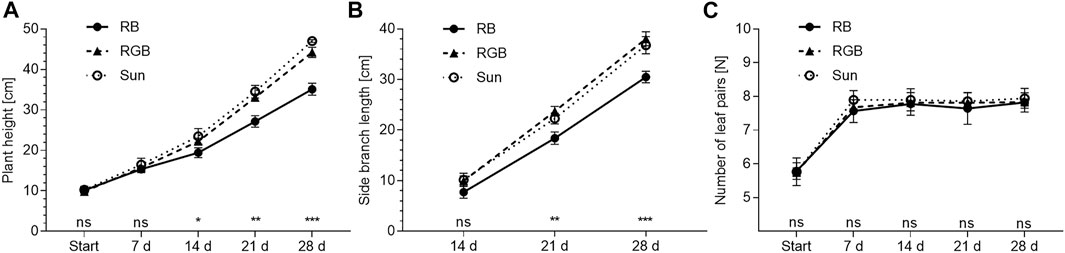
FIGURE 2. Morphological characteristics of Mentha x piperita var. piperita cv. Multimentha as effected by light spectra over time. RB = Red/Blue, RGB = Red/Green/Blue, SUN = artificial sunlight spectrum. (A) Plant height∗, (B) Side branch length∗, (C) Number of leaf pairs∗∗. ∗ Presented are mean plant heights and side branch lengths of three independent spatial replications (n = 3) ± standard deviation (SD) of 25–30 assessed peppermint plants per spatial replication and light treatment (N of plant height = 267; N of side branch length = 253). Significant differences between light treatments were determined according to nested one-way ANOVA (∗: p ≤ 0.05, ∗∗: p ≤ 0.01, ∗∗∗: p ≤ 0.001, ns: not significant) followed by Tukey’s multiple comparison test at 95% confidence level. ∗∗ Presented are mean numbers of leaf pairs of three independent spatial replications (n = 3) ± SD of 30 assessed peppermint plants per spatial replication and light treatment (N = 270). Nonparametric Kruskal–Wallis tests (p ≥ 0.9) were used to test for significant differences between light treatments (ns: not significant) at each time point.
3.2. Branch Length
Three weeks after start of the experiment, side branches were elongated under RGB and SUN as compared to branches grown under RB (p = 0.01 respectively). Branch lengths under RGB, SUN and RB averaged 23.7 ± 0.8, 22.3 ± 0.9 and 18.4 ± 1.0 cm. After 4 weeks, differences in branch elongation further increased (p ≤ 0.01) between RB- and RGB-illuminated as well as between RB- and SUN-illuminated plants, and averaged 30.5 ± 0.9, 38.1 ± 1.1 and 36.8 ± 1.4 cm under RB, RGB and SUN respectively (Figure 2B).
3.3. Number of Leaf Pairs
Number of fully developed leaf pairs along the stem did not differ between peppermints illuminated with RB, RGB or SUN light spectra (p ≥ 0.44). While all plants averaged six fully developed leaf pairs at the beginning of the experiment, the peppermints averaged eight leaf pairs along the main stem for the remaining study time (Figure 2C).
3.4. Length and Width of Leaves
Top leaves of the peppermint canopy were affected by light treatment (Figure 3). Top leaves under RB were shorter (6.8 ± 0.1 cm) and narrower (4.4 ± 0.2 cm) than those under RGB (length = 7.4 ± 0.1 cm, width = 5.1 ± 0.1 cm) (p ≤ 0.001, p ≤ 0.01 respectively). Similarly, length and width of top leaves under RB remained shorter and narrower than under SUN (length = 7.3 ± 0.2 cm, width = 4.9 ± 0.1 cm) (p ≤ 0.01, respectively). Clearly, no differences in peppermint leaves’ length and width between top leaves under RGB und SUN light spectra were detected (p ≥ 0.72). Leaves deeper within the canopy remained indifferent in size after light treatment (p ≥ 0.13).
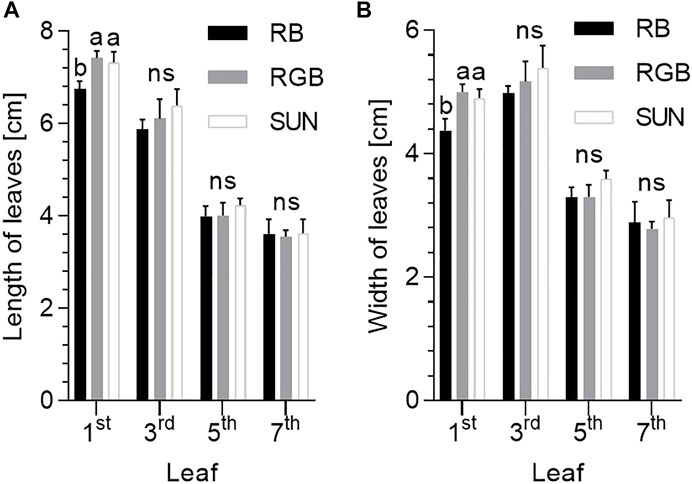
FIGURE 3. Length and width of fresh leaves of Mentha x piperita var. piperita cv. Multimentha as effected by different light spectra. RB = Red/Blue, RGB = Red/Green/Blue, SUN = artificial sunlight spectrum, first, third, fifth and seventh leaf on x-axes represent measurements of the top leaf (first) to the bottom leaf (seventh) along the shoot. (A) Length of peppermint leaves as effected by light treatment (N = 74–89, n = 5–10 leaves per spatial replication and light treatment) ∗. (B) Width of peppermint leaves as effected by light treatment (N = 74–89, n = 5–10 leaves per spatial replication and light treatment) ∗. ∗ For each leaf position (1–4), significant differences between light treatments were determined according to nested one-way ANOVA test (ns: not significant). When significant differences were determined, Tukey’s multiple comparisons test followed (different letters indicate significant differences between light treatments at 95% confidence level).
3.5. Biomass Yields
Total fresh and dry matter yields of peppermints cultivated under RB, RGB and SUN light spectra did not differ at the end of the experiment (p = 0.76 and p = 0.87, respectively). On average, peppermints under RB, RGB and SUN accumulated a fresh weight of 31.7 ± 7.0, 32.7 ± 6.2 and 30.1 ± 6.5 g per plant, respectively (Figure 4A). Dry weights per plant under the same light spectra (RB, RGB, SUN) averaged 3.5 ± 0.9, 3.5 ± 0.7 and 3.4 ± 0.9 g per plant (Figure 4B). No differences in total leaf dry matter per plant was observed (p = 0.20), averaging 2.0 ± 0.4, 1.9 ± 0.4 and 1.7 ± 0.4 g per plant for RB-, RGB- and SUN-treated peppermints (Figure 4C).
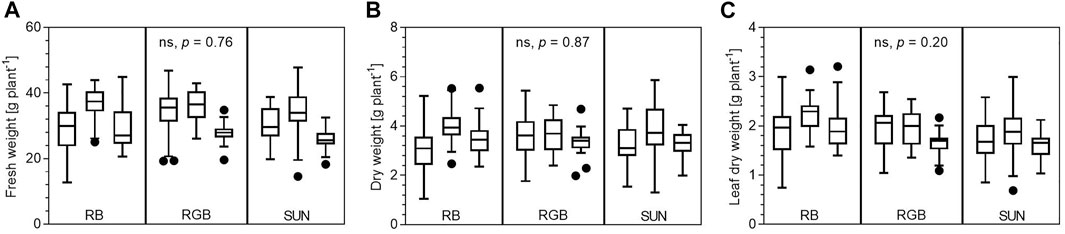
FIGURE 4. Biomass yields of Mentha x piperita var. piperita cv. Multimentha as effected by different light spectra. RB = Red/Blue, RGB = Red/Green/Blue, SUN = artificial sunlight spectrum. (A) Fresh matter yields in Gram plant−1 per light spectra and spatial replication (N = 269) ∗. (B) Dry matter yields in Gram plant−1 per light spectra and spatial replication (N = 270) ∗. (C) Leaf dry matter in Gram plant−1 per light spectra and spatial replication (N = 266) ∗. ∗ Presented are minimum, 25th percentile, median, 75th percentile and maximum yields as well as outliers (black dots) of all assessed peppermint plants per light treatment and spatial replication (n = 29–30 plants per replication). Ordinary one-way ANOVA with the means of each spatial replication was used to test for significant differences between light treatments (ns: not significant).
3.6. Composition, Content and Yield of Essential Oil
Analysis of peppermint oils enabled the identification of 24 EO compounds (Table 2). While EO yields remained unaffected, EO compositions significantly differed between the light treatments applied. While desired percentages of menthone and iso-menthone as well as piperitone and piperitenone were significantly greater under the SUN and RB light treatment, the percentages of the undesired compounds pulegone and menthofuran were significantly greater in peppermints grown under the RGB light treatment. Further, all LED light treated plants are characterized by an atypical EO composition for Mentha x piperita var. piperita cv. Multimentha when compared to its common EO composition detected under field conditions (Supplemnatry Table S1; Schulz et al., 1999; Schulz and Krüger 1999; Das Bundessortenamt 2002; Pank et al., 2013). With pulegone and menthofuran as the dominating EO components (and only small amounts of menthone and menthol) detected under all three LED light conditions, their EO compositions differ significantly from the typically menthone and menthol enriched EOs of this cultivar under field conditions.
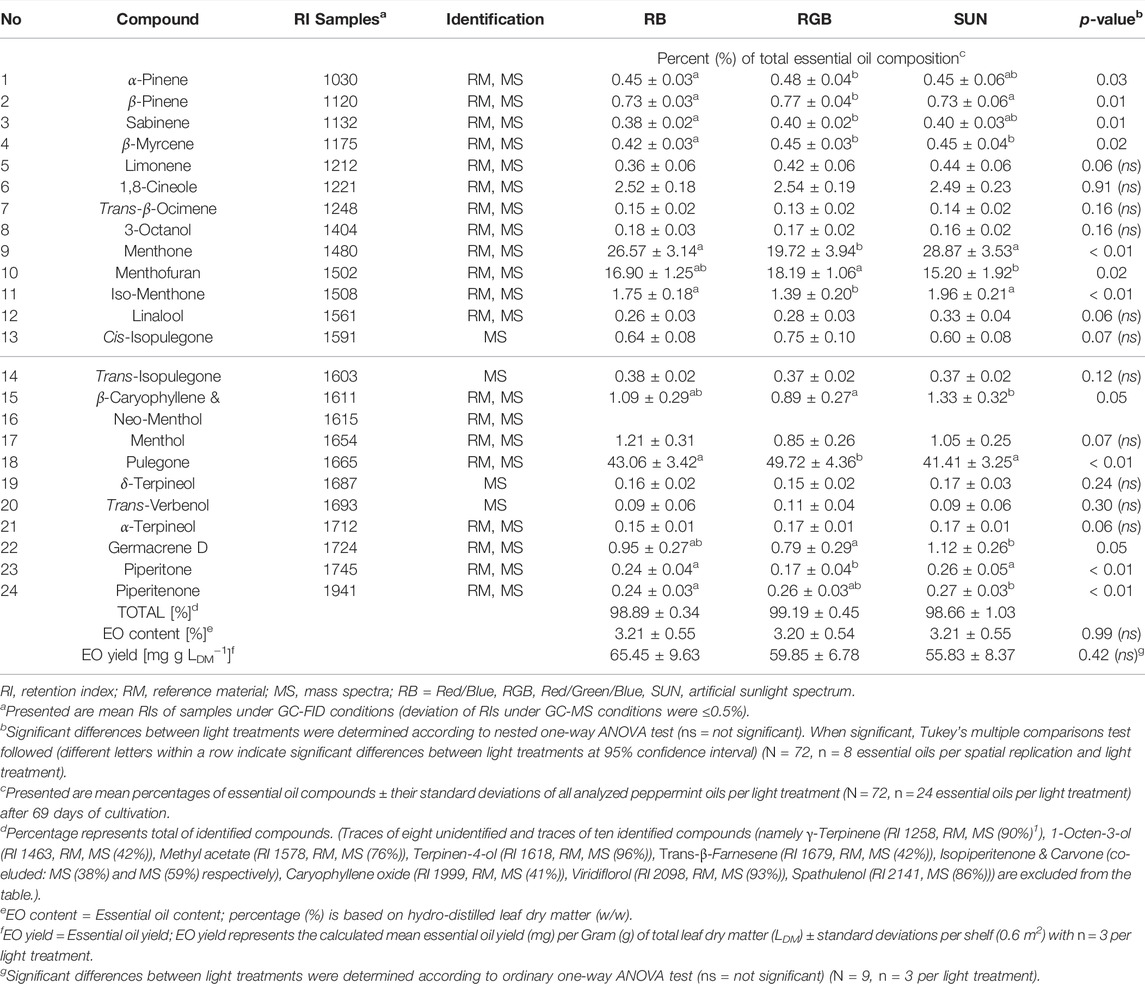
TABLE 2. Effect of three light qualities on the chemical composition of 24 identified essential oil compounds, essential oil content and yield of Mentha x piperita L. var. piperita cv. Multimentha after 69 days of cultivation.
4 Discussion
As known from recent studies on the impact of G wavelengths inducing stem elongations and leaf expansions in basil and tomato (Kaiser et al., 2019; Schenkels et al., 2020), RB resulted in a compact peppermint growth, whereas both G-containing light conditions induced stem and side branch elongations as well as significant expansion of top leaves in Mentha x piperita. Contrary to our expectations, these profound morphological differences where not accompanied with biomass and EO yield increases at the time of harvest. However, the broad SUN spectrum as well as the RB spectrum significantly affected the composition of the EOs by accelerating the reduction of pulegone to menthone (and iso-menthone), representing an important quality-determining transformation step during menthol biosynthesis.
Plant Height
It is well documented that B light inhibits hypocotyl growth and stem elongation (Sellaro et al., 2010; Pedmale et al., 2016), independent of different R light proportions (Hernandez and Kubota 2016; Spalholz et al., 2020). These observations coincide with our findings as the peppermints developed a more compact phenotype under RB in comparison to RGB and SUN. To the contrary, low B/G ratios as well as low R/FR ratios are both independently known to elongate stems in a variety of plants, representing a typical plant adaptation known as shade avoidance symptom in order to increase light capture (Franklin 2008; Zhang et al., 2011; Smith et al., 2017). For example, G induced extreme stem and branch elongations under low B/G ratios in Arabidopsis, basil, tomato and different lettuce cultivars (Sellaro et al., 2010; Zhang et al., 2011; Kaiser et al., 2019; Schenkels et al., 2020; Spalholz et al., 2020). Also, low R/FR ratios have been reported to induce stem elongations e.g. in Arabidopsis, basil and squash (Yang et al., 2012; Carvalho et al., 2016; Pedmale et al., 2016). In our study however, the excessive stem and side branch elongations detected under RGB and SUN compared to RB are likely a result of the high G fluence rates the peppermints were exposed to (likely by strongly stimulating the expression of hypocotyl growth-promoting genes as proposed by Pedmale et al., 2016). That the supplied FR light under SUN had no additional stem- or branch-elongating effect on the peppermints when compared to RGB lacking FR can be attributed to the specific R/FR ratio of 2.2 used in our study. Based on the phytochrome-mediated model, the SUN-treated peppermints absorbed more R than FR photons. Thus, the involved phytochrome-photoreceptor (PhyB) remained in its active R-absorbing form, which restricts elongation (by inhibiting phytochrome-interacting factor (PIF) activity required for the biosynthesis of the elongation-promoting phytohormone auxin (Frankhauser and Batschauer 2016)).
Leaf Growth and Expansion
Peppermint leaves of the upper canopy level (first to third leaf pairs from the top) reached lengths between 5.7 and 7.5 cm under all light treatments, which represents the upper size range described for peppermint. As reviewed by Mahendran and Rahman (2020), leaves of Mentha x piperita L. are usually between 2 and 7 cm long. This indicates leaf expansion responses due to the light treatments applied, and represents another typical shade avoidance response of the young peppermints (Franklin 2008; Zhang et al., 2011; Smith et al., 2017).
Furthermore, significant treatment differences were observed on the fully expanded top leaves, as the peppermints’ top leaves under RGB and SUN were significantly longer and wider than the top leaves exposed to RB. This outcome is in agreement with multiple studies that reported increased leaf areas when RB spectra were either partially replaced with G light or compared to broad white lighting conditions for a variety of plant species (Zhang et al., 2011; Lin et al., 2013; Carvalho et al., 2016; Kaiser et al., 2019; Schenkels et al., 2020; Spalholz et al., 2020). Thus, the greater leaf expansions detected under RGB and SUN compared to expansions detected under RB can be attributed to the low B/G ratios supplied by RGB and SUN.
To investigate, if the leaf expansions also take place deeper within the canopy of the peppermints, we additionally measured the lengths and widths from the third, fifth and seventh leaf pair underneath. Contrary to our expectation, an increase in leaf size under the G-containing light treatments deeper within the canopy (as G can penetrate deeper into leaves and canopies than R and B (Smith et al., 2017; Saengtharatip et al., 2020; Schenkels et al., 2020)) was not observed.
Biomass Accumulation
G has the potential to drive photosynthesis more effectively than R and B by increasing carbon fixation under PPFDs greater than 200 μmol m−2 s−1 in spinach leaves (Terashima et al., 2009). Thus, scientists increasingly recommend the investigation and implementation of G light in order to increase biomass accumulations and yields under LED-based lighting systems (Folta 2004; Smith et al., 2017). However, studies describing yield increases due to incorporation of G light are scarce and were only focused on a few plant species (Novičkovas et al., 2012; Lin et al., 2013; Schenkels et al., 2020). For other plants, biomass increases were not observed (Spalholz et al., 2020), supporting our findings. Neither the peppermints’ excessive stem elongations nor the partial leaf expansions in the upper canopy observed in our study resulted in significant increases of fresh or dry mass accumulations during the peppermints’ early vegetative phase in the automated vertical cultivation system.
Most likely, the received light intensity as well as the early time of harvest constrained the detection of possible differences in biomass yields. However, it is also possible that the high variabilities in the light distribution patterns within each cultivation shelf (as depicted in Figures 1B,C) increased the variance of biomasses per plant and thus, hindered the detection of statistical effects.
Essential Oil
Peppermints’ EO content increases rapidly with leaf development and reaches its maximum when the leaf is fully expanded. Thus, EO formation of peppermint is under pronounced endogenous control (Gershenzon et al., 2000; McConkey et al., 2000). As the peppermints’ leaf development as well as biomasses remained indifferent between the light qualities, it was not surprising that total EO yields remained unaffected under the applied light treatments.
Nevertheless, the light treatments significantly influenced the composition of the obtained EOs. The biosynthetic pathway of the quality-determining EO constituent menthol is characterized by a series of well-described transformation reactions (Croteau et al., 2005). With percentages of 41–50% and 20–29%, pulegone and menthone are the two main EO constituents detected during the early vegetative phase of Mentha x piperita L. in our study, and represent the last two central intermediates during menthol biosynthesis. The metabolite pulegone becomes reduced to menthone by pulegone reductase. To a smaller extent, the same enzyme reduces pulegone to iso-menthone. Then, menthone and iso-menthone are finally reduced to menthol and iso-menthol by menthone reductase (Croteau et al., 2005). As percentages of pulegone decreased and percentages of menthone and iso-menthone significantly increased under RB and SUN, our results indicate an accelerated conversion of pulegone to menthone and iso-menthone in comparison to RGB.
As R light has been shown to promote EO content in Mentha x piperita (Sabzalian et al., 2014), the accelerated conversion detected under RB may be explained by the elevated R fluence rate in comparison to the rate of R supplied by the RGB treatment during the trial period. However, as the amounts of R, B and G light between RGB and SUN were almost identical, the flanking regions including UV-A and/or FR light must have contributed to the enhanced conversion from pulegone to menthone (and iso-menthone) under SUN as well. As FR light actually suppresses terpene production (Tanaka et al., 1989; Yamaura et al., 1991), and partially reduces volatile emissions under low R/FR ratios (Kegge et al., 2013), it appears that the FR light fraction under the SUN treatment (with a high R/FR ratio) was not involved in the observed maturation process. Thus, the SUNs’ proportion of UV-A must have expedited the conversion under our experimental conditions, which is further supported by findings of Behn et al. (Behn et al., 2010), who reported an accelerated monoterpene transformation during flowering of Mentha x piperita exposed to low solar radiation including UV-B (as compared to low solar radiation excluding UV-B).
With a percentage of 15–18%, menthofuran is the third most abundant EO component detected under all supplied light qualities. As menthofuran synthesis represents a diversion from the desired menthol biosynthetic pathway at the branch point metabolite pulegone (Croteau et al., 2005), all three light treatments severely reduced menthol production potential.
According to the European Pharmacopoeia (Schmiedel 2008), the detected percentages of pulegone (41–50%) and menthofuran (15–18%) highly exceeded the legal limits of 4 and 9%, as both compounds have shown to be hepatotoxic (Malekmohammad et al., 2019). As menthofuran and pulegone productions have been shown to be favored under continuous low light intensities and short day lengths (Burbott and Loomis 1967; Clark and Menary 1980; Voirin et al., 1990; Croteau et al., 2005), the low light intensities at the bottom of the shelves received by the peppermints (∼200 μmol−2 s−1) were far too low for obtaining an EO of desired compositional quality. As shown in Figure 5, LED light intensities decrease tremendously with increasing distance to the LED light source (e.g. light intensity 5 cm from the light source: ∼ 1,150 μmol m−2 s−1; light intensity 10 cm from the light source: ∼ 650 μmol m−2 s−1). Thus, most of the plant was cultivated under low light intensities of ≥150 μmol m−2 s−1. As our results indicate, these light intensities lead to the divergence from and incompletion of menthol biosynthesis and thus—from a consumer safety perspective—to non-marketable peppermint plants. The drastically increasing light intensity (up to ∼1,200 μmol m−2 s−1) with decreasing plant distance to the LED light source in the vertical cultivation system could not change the direction of the biosynthetic pathway during the trial period. The impact of these steep light intensity gradients of LED systems need to receive more attention in future plant-dependent light studies, including a stronger focus on EO composition. So far, most published studies addressing the effects of LED lighting on plant performance have focused on morphological traits and EO contents, however, as shown in this study, the complex interaction of LED light spectrum and intensity also affect the composition of EOs—a research question that has received too little attention yet.
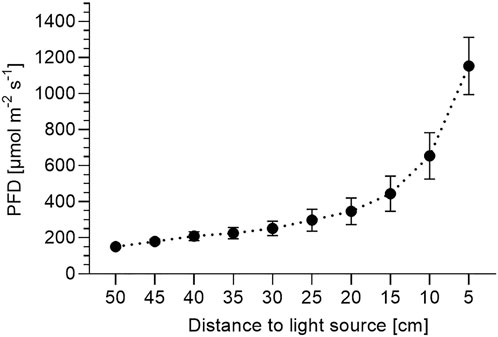
FIGURE 5. Photon flux density depending on distance to light source. Presented are average photon flux densities (PFD) [µmol m−2 s−1] ± SD between 380 and 780 nm recorded in 5 cm intervals starting at plant pot level 950 cm from the light source) from nine representative positions across the cultivation shelf per light treatment (N = 27) at each distance.
Conclusion
Despite substantial light intensity increases with decreasing distance to LED-light sources in the vertical cultivation systems (up to ∼1,200 μmol m−2 s−1), the G-containing treatments RGB and SUN with their low B/G ratios induced such excessive stem and branch elongations, that peppermint cultivation remained constrained to the vegetative phase. In contrast, RB with its high B fluence rate induced compact growth (appropriate for prolonged space-limited cultivation of peppermint). The impact of G light on peppermints’ stem and branch elongation in this study has been shown for the first time and thus, supports the G-induced elongations revealed recently in other plant species. Further, our study supports the potential of G light to induce leaf expansions as recently shown by other authors. However, the expected leaf expansion deeper within the canopy was not observed in the young peppermint plants at the time of harvest. In the future, further trials with more mature peppermint plants and thus with more mature leaves deeper within the canopy should be conducted, in order to assess the G light potential for leaf expansions deeper within the canopy. The extreme elongations and partial leaf expansions did not result in the expected biomass and EO yield increases, and may again be attributed to the short trial period due to the peppermints’ excessive stem elongations in the vertical cultivation system.
Further, the pronounced vertical loss of light intensity in the vertical cultivation system resulted in insufficient photosynthetic conditions and crucially determined the direction of monoterpene transformation reactions in peppermint. The low light intensities led to unwanted high pulegone contents and triggered the undesired synthesis of menthofuran under all light qualities. Thus, the light intensities perceived by the peppermint plants are not suitable for obtaining peppermint oil of quality as specified by the European Pharmacopoeia. Nevertheless, R as well as UV-A represent promising spectral light regions for accelerating the peppermints’ EO maturation process as reductions of pulegone to menthone appeared significantly enhanced.
Subsequent studies with light intensities near the light saturation point of peppermint should be conducted, with special emphasis on high proportions of R, B and UV-A. Further fundamental studies, including the impact of specific G narrow wavebands, of G light at different PPFDs and of different B/G and R/G ratios are necessary to improve horticultural applicability of G light. In addition, uniformity of LED light distribution patterns remains a focal point for technical improvements to ensure uniform plant growth in vertical cultivation systems.
Data Availability Statement
The original contributions presented in the study are included in the article/Supplementary Material, further inquiries can be directed to the corresponding authors.
Author Contributions
JT Conceptualization, project administration, formal analysis, validation, investigation, data curation, writing—original draft preparation. AK: Conceptualization, Writing—Review & Editing. HS: Conceptualization, project administration, methodology, funding acquisition, resources, supervision.
Funding
This work was supported and funded by the European Innovation Partnership for Improvement of Agricultural Productivity and Sustainability (grant number 204016000016/80168353) via the European Agricultural Fund for Rural Development.
Conflict of Interest
Dr. HS is the owner of the company Consulting & Project Management for Medicinal and Aromatic Plants.
The remaining authors declare that the research was conducted in the absence of any commercial or financial relationships that could be construed as a potential conflict of interest.
Publisher’s Note
All claims expressed in this article are solely those of the authors and do not necessarily represent those of their affiliated organizations, or those of the publisher, the editors and the reviewers. Any product that may be evaluated in this article, or claim that may be made by its manufacturer, is not guaranteed or endorsed by the publisher.
Acknowledgments
We thank Roland Buchhorn and Claudia Könecke for cultivation assistance, Konradin Feierabend for assisting in data curation and René Grünwald for analytical assistance. We thank Torsten Meiners and Christoph Böttcher for data validation. We further thank Matthias Melzig from Free University Berlin for his support.
Supplementary Material
The Supplementary Material for this article can be found online at: https://www.frontiersin.org/articles/10.3389/frfst.2022.852155/full#supplementary-material
References
Alvarenga, J. P., Pacheco, F. V., Bertolucci, S. K. V., Silva, S. T., de Oliveira, T., and Pinto, J. E. B. P. (2018). In Vitro culture of Mentha Viridis: Quality and Intensity of Light on Growth and Production of Volatiles. Acta Hortic. 1224, 175–182. doi:10.17660/ActaHortic.2018.1224.23
Behn, H., Albert, A., Marx, F., Noga, G., and Ulbrich, A. (2010). Ultraviolet-B and Photosynthetically Active Radiation Interactively Affect Yield and Pattern of Monoterpenes in Leaves of Peppermint (Mentha × Piperita L.). J. Agric. Food Chem. 58 (12), 7361–7367. doi:10.1021/jf9046072
Beigi, M., Torki-Harchegani, M., and Ghasemi Pirbalouti, A. (2018). Quantity and Chemical Composition of Essential Oil of Peppermint (Mentha × Piperita L.) Leaves under Different Drying Methods. Int. J. Food Prop. 21 (1), 267–276. doi:10.1080/10942912.2018.1453839
Burbott, A. J., and Loomis, W. D. (1967). Effects of Light and Temperature on the Monoterpenes of Peppermint. Plant Physiol. 42 (1), 20–28. doi:10.1104/pp.42.1.20
Carvalho, S. D., Schwieterman, M. L., Abrahan, C. E., Colquhoun, T. A., and Folta, K. M. (2016). Light Quality Dependent Changes in Morphology, Antioxidant Capacity, and Volatile Production in Sweet Basil (Ocimum Basilicum). Front. Plant Sci. 7, 1328. doi:10.3389/fpls.2016.01328
Clark, R., and Menary, R. (1980). Environmental Effects on Peppermint (Mentha Piperita L.). I. Effect of Daylength, Photon Flux Density, Night Temperature and Day Temperature on the Yield and Composition of Peppermint Oil. Funct. Plant Biol. 7 (6), 685–692. doi:10.1071/PP9800685
Croteau, R. B., Davis, E. M., Ringer, K. L., and Wildung, M. R. (2005). (−)-Menthol Biosynthesis and Molecular Genetics. Naturwissenschaften 92 (12), 562–577. doi:10.1007/s00114-005-0055-0
Das Bundessortenamt (BSA) (2002). “Multimentha,” in Beschreibende Sortenliste Arznei- und Gewürzpflanzen. 1st ed (Hannover, Germany: Deutscher Landwirtschaftsverlag GmbH), 121.
Eichhorn Bilodeau, S., Wu, B.-S., Rufyikiri, A.-S., MacPherson, S., and Lefsrud, M. (2019). An Update on Plant Photobiology and Implications for Cannabis Production. Front. Plant Sci. 10, 296. doi:10.3389/fpls.2019.00296
Fankhauser, C., and Batschauer, A. (2016). Shadow on the Plant: a Strategy to Exit. Cell. 164 (1-2), 15–17. doi:10.1016/j.cell.2015.12.043
Folta, K. M. (2004). Green Light Stimulates Early Stem Elongation, Antagonizing Light-Mediated Growth Inhibition. Plant Physiol. 135 (3), 1407–1416. doi:10.1104/pp.104.038893
Franklin, K. A. (2008). Shade Avoidance. New Phytol. 179, 930–944. doi:10.1111/j.1469-8137.2008.02507.x
Gershenzon, J., McConkey, M. E., and Croteau, R. B. (2000). Regulation of Monoterpene Accumulation in Leaves of Peppermint. Plant Physiol. 122 (1), 205–214. doi:10.1104/pp.122.1.205
Hernández, R., and Kubota, C. (2016). Physiological Responses of Cucumber Seedlings under Different Blue and Red Photon Flux Ratios Using LEDs. Environ. Exp. Bot. 121, 66–74. doi:10.1016/j.envexpbot.2015.04.001
Kaiser, E., Weerheim, K., Schipper, R., and Dieleman, J. A. (2019). Partial Replacement of Red and Blue by Green Light Increases Biomass and Yield in Tomato. Sci. Hortic. 249, 271–279. doi:10.1016/j.scienta.2019.02.005
Kalaitzoglou, P., van Ieperen, W., Harbinson, J., van der Meer, M., Martinakos, S., Weerheim, K., et al. (2019). Effects of Continuous or End-Of-Day Far-Red Light on Tomato Plant Growth, Morphology, Light Absorption, and Fruit Production. Front. Plant Sci. 10, 322. doi:10.3389/fpls.2019.00322
Kegge, W., Weldegergis, B. T., Soler, R., Eijk, M. V. V., Dicke, M., Voesenek, L. A. C. J., et al. (2013). Canopy Light Cues Affect Emission of Constitutive and Methyl Jasmonate‐induced Volatile Organic Compounds in A Rabidopsis Thaliana. New Phytol. 200, 861–874. doi:10.1111/nph.12407
Kontor, W. (2021). Wetterrückblick Berlin-Dahlem. Retrieved from: https://www.wetterkontor.de/de/wetter/deutschland/rueckblick.asp?id=20&datum0=10.04.2019&datum1=06.05.2019&jr=2021&mo=3&datum=08.03.2021&t=8&part=0 (Accessed October 25, 2021).
Kozai, T. (2019). Towards Sustainable Plant Factories with Artificial Lighting (PFALs) for Achieving SDGs. Int. Agric. Biol. Eng. 12 (5), 28–37. doi:10.25165/j.ijabe.20191205.5177
Lin, K. H., Huang, M. Y., Huang, W. D., Hsu, M. H., Yang, Z. W., and Yang, C. M. (2013). The Effects of Red, Blue, and White Light-Emitting Diodes on the Growth, Development, and Edible Quality of Hydroponically Grown Lettuce (Lactuca sativa L. Var. Capitata). Sci. Hortic. 150, 86–91. doi:10.1016/j.scienta.2012.10.002
Maffei, M., and Scannerini, S. (1999). Photomorphogenic and Chemical Responses to Blue Light inMentha Piperita. J. Essent. Oil Res. 11 (6), 730–738. doi:10.1080/10412905.1999.9712007
Mahendran, G., and Rahman, L. U. (2020). Ethnomedicinal, Phytochemical and Pharmacological Updates on Peppermint ( Mentha × Piperita L.)-A Review. Phytotherapy Res. 34 (9), 2088–2139. doi:10.1002/ptr.6664
Malekmohammad, K., Rafieian-Kopaei, M., Sardari, S., and Sewell, R. D. E. (2019). Toxicological Effects of Mentha X Piperita (Peppermint): a Review. Toxin Rev. 40, 445–459. doi:10.1080/15569543.2019.1647545
McConkey, M. E., Gershenzon, J., and Croteau, R. B. (2000). Developmental Regulation of Monoterpene Biosynthesis in the Glandular Trichomes of Peppermint. Plant Physiol. 122 (1), 215–224. doi:10.1104/pp.122.1.215
McCree, K. J. (1972). The Action Spectrum Absorptance and Quantum Yield of Photosynthesis in Crop Plants. Agr. Meteorol. 9, 191–216. doi:10.1016/0002-1571(71)90022-7
National Renewable Energy Laboratory (NREL). (2020). Reference Air Mass 1.5 Spectra. Retrieved from: https://www.nrel.gov/grid/solar-resource/spectra-am1.5.html (Accessed March 24, 2020).
Novičkovas, A., Brazaitytė, A., Duchovskis, P., Jankauskienė, J., Samuolienė, G., Virsilė, A., et al. (2012). Solid-state Lamps (LEDs) for the Short-Wavelength Supplementary Lighting in Greenhouses: Experimental Results with Cucumber. Acta Hortic. 927, 723–730. doi:10.17660/ActaHortic.2012.927.90
Orsini, F., Pennisi, G., Pennisi, G., Zulfiqar, F., and Gianquinto, G. (2020). Sustainable Use of Resources in Plant Factories with Artificial Lighting (PFALs). Eur. J. Hortic. Sci. 85 (5), 297–309. doi:10.17660/eJHS.2020/85.5.1
Pank, F., Blaschek, W., Bomme, U., Hammer, K., Schliephake, E., and Schmatz, R. (2013). “Pfefferminze (Mentha X Piperita L.),” in Handbuch des Arznei- und Gewürzpflanzenbaus. Band 5. Arznei- und Gewürzpflanzen L-Z. Editor B. Hoppe. 1st ed (Bernburg, Germany: Gartenbauwissenschaf), 316.
Park, Y., and Runkle, E. S. (2017). Far-red Radiation Promotes Growth of Seedlings by Increasing Leaf Expansion and Whole-Plant Net Assimilation. Environ. Exp. Bot. 136, 41–49. doi:10.1016/j.envexpbot.2016.12.013
Pedmale, U. V., Huang, S. S. C., Zander, M., Cole, B. J., Hetzel, J., Ljung, K., et al. (2016). Cryptochromes Interact Directly with PIFs to Control Plant Growth in Limiting Blue Light. Cell. 164 (1-2), 233–245. doi:10.1016/j.cell.2015.12.018
Pennisi, G., Blasioli, S., Cellini, A., Maia, L., Crepaldi, A., Braschi, I., et al. (2019). Unraveling the Role of Red:blue LED Lights on Resource Use Efficiency and Nutritional Properties of Indoor Grown Sweet Basil. Front. Plant Sci. 10, 305. doi:10.3389/fpls.2019.00305
Rechner, O., Neugart, S., Schreiner, M., Wu, S., and Poehling, H.-M. (2017). Can Narrow-Bandwidth Light from UV-A to Green Alter Secondary Plant Metabolism and Increase Brassica Plant Defenses against Aphids? PLoS ONE 12 (11), e0188522. doi:10.1371/journal.pone.0188522
Rios-Estepa, R., Turner, G. W., Lee, J. M., Croteau, R. B., and Lange, B. M. (2008). A Systems Biology Approach Identifies the Biochemical Mechanisms Regulating Monoterpenoid Essential Oil Composition in Peppermint. Proc. Natl. Acad. Sci. U.S.A. 105 (8), 2818–2823. doi:10.1073/pnas.0712314105
Sabzalian, M. R., Heydarizadeh, P., Zahedi, M., Boroomand, A., Agharokh, M., Sahba, M. R., et al. (2014). High Performance of Vegetables, Flowers, and Medicinal Plants in a Red-Blue LED Incubator for Indoor Plant Production. Agron. Sustain. Dev. 34 (4), 879–886. doi:10.1007/s13593-014-0209-6
Saengtharatip, S., Goto, N., Kozai, T., and Yamori, W. (2020). Green Light Penetrates inside Crisp Head Lettuce Leading to Chlorophyll and Ascorbic Acid Content Enhancement. Acta Hortic. 1273, 261–270. doi:10.17660/ActaHortic.2020.1273.35
Salehi, B., Stojanović-Radić, Z., Matejić, J., Sharopov, F., Antolak, H., Kręgiel, D., et al. (2018). Plants of Genus Mentha: From Farm to Food Factory. Plants 7 (3), 70. doi:10.3390/plants7030070
Schenkels, L., Saeys, W., Lauwers, A., and De Proft, M. P. (2020). Green Light Induces Shade Avoidance to Alter Plant Morphology and Increases Biomass Production in Ocimum Basilicum L. Sci. Hortic. 261, 109002. doi:10.1016/j.scienta.2019.109002
Schmiedel, R. (2008). Europäisches Arzneibuch (Pharmacopoeia Europaea). 6th ed. Stuttgart, Germany: Deutscher Apotheker Verlag, 3633–3634.
Schulz, H., Drews, H.-H., and Krüger, H. (1999). Rapid NIRS Determination of Quality Parameters in Leaves and Isolated Essential Oils ofMenthaSpecies. J. Essent. Oil Res. 11, 185–190. doi:10.1080/10412905.1999.9701106
Schulz, H., and Krüger, H. (1999). Zur Verbreitung, Züchtung und Verarbeitung von Pfefferminze und Krauseminze. AvaliableAt: https://www.researchgate.net/publication/287643299_Zur_Verbreitung_Zuchtung_und_Verarbeitung_von_Pfefferminze_und_Krauseminze (Accessed January 4, 2022).
Sellaro, R., Crepy, M., Trupkin, S. A., Karayekov, E., Buchovsky, A. S., Rossi, C., et al. (2010). Cryptochrome as a Sensor of the Blue/green Ratio of Natural Radiation in Arabidopsis. Plant Physiol. 154 (1), 401–409. doi:10.1104/pp.11.16082010.1104/pp.110.160820
Smith, H. L., McAusland, L., and Murchie, E. H. (2017). Don't Ignore the Green Light: Exploring Diverse Roles in Plant Processes. J. Exp. Bot. 68 (9), 2099–2110. doi:10.1093/jxb/erx098
Spalholz, H., Perkins-Veazie, P., and Hernández, R. (2020). Impact of Sun-Simulated White Light and Varied Blue:red Spectrums on the Growth, Morphology, Development, and Phytochemical Content of Green- and Red-Leaf Lettuce at Different Growth Stages. Sci. Hortic. 264, 109195. doi:10.1016/j.scienta.2020.109195
Sun, J., Nishio, J. N., and Vogelmann, T. C. (1998). Green Light Drives CO2 Fixation Deep within Leaves. Plant Cell. Physiology 39 (10), 1020–1026. doi:10.1093/oxfordjournals.pcp.a029298
Tabbert, J. M., Schulz, H., and Krähmer, A. (2021). Increased Plant Quality, Greenhouse Productivity and Energy Efficiency with Broad-Spectrum LED Systems: A Case Study for Thyme (Thymus Vulgaris L.). Plants 10, 960. doi:10.3390/plants10050960
Tanaka, S., Yamaura, T., Shigemoto, R., and Tabata, M. (1989). Phytochrome-mediated Production of Monoterpenes in Thyme Seedlings. Phytochemistry 28 (11), 2955–2957. doi:10.1016/0031-9422(89)80260-2
Terashima, I., Fujita, T., Inoue, T., Chow, W. S., and Oguchi, R. (2009). Green Light Drives Leaf Photosynthesis More Efficiently Than Red Light in Strong White Light: Revisiting the Enigmatic Question of Why Leaves Are Green. Plant Cell. Physiol. 50 (4), 684–697. doi:10.1093/pcp/pcp034
Voirin, B., Brun, N., and Bayet, C. (1990). Effects of Daylength on the Monoterpene Composition of Leaves of Mentha X Piperita. Phytochemistry 29 (3), 749–755. doi:10.1016/0031-9422(90)80012-6
Yamaura, T., Tanaka, S., and Tabata, M. (1991). Participation of Phytochrome in the Photoregulation of Terpenoid Synthesis in Thyme Seedlings. Plant Cell. Physiol. 32 (5), 603–607. doi:10.1093/oxfordjournals.pcp.a078122
Yang, Z.-C., Kubota, C., Chia, P.-L., and Kacira, M. (2012). Effect of End-Of-Day Far-Red Light from a Movable LED Fixture on Squash Rootstock Hypocotyl Elongation. Sci. Hortic. 136, 81–86. doi:10.1016/j.scienta.2011.12.023
Keywords: light-emitting diode, peppermint (Mentha piperita L.), essential oil, plant morphological characteristics, biomass
Citation: Tabbert JM, Schulz H and Krähmer A (2022) Investigation of LED Light Qualities for Peppermint (Mentha x Piperita L.) Cultivation Focusing on Plant Quality and Consumer Safety Aspects. Front. Food. Sci. Technol. 2:852155. doi: 10.3389/frfst.2022.852155
Received: 10 January 2022; Accepted: 17 June 2022;
Published: 19 July 2022.
Edited by:
Fernando Perez Rodriguez, University of Cordoba, SpainCopyright © 2022 Tabbert, Schulz and Krähmer. This is an open-access article distributed under the terms of the Creative Commons Attribution License (CC BY). The use, distribution or reproduction in other forums is permitted, provided the original author(s) and the copyright owner(s) are credited and that the original publication in this journal is cited, in accordance with accepted academic practice. No use, distribution or reproduction is permitted which does not comply with these terms.
*Correspondence: Jenny Manuela Tabbert, amVubnkudGFiYmVydEBqdWxpdXMta3VlaG4uZGU=; Andrea Krähmer, YW5kcmVhLmtyYWVobWVyQGp1bGl1cy1rdWVobi5kZQ==