- Produce Safety and Microbiology Research Unit, United States Department Agricultural, Agricultural Research Service, Albany, CA, United States
Despite efforts to control pathogenic hazards in agriculture, leafy greens grown in California were the source of several high-profile outbreaks of Shiga toxin-producing E. coli (STEC). The U.S. Food and Drug Administration (FDA) analysis of the outbreaks found three reoccurring patterns with leafy greens contaminated with STEC, specifically E. coli O 157:H7, in 2018–2020: the presence of pathogenic E. coli, common geographical regions, and issues with activities on adjacent lands, such as cattle production and migratory birds. The FDA’s response to the recurring outbreaks associated with leafy greens is the Leafy Greens STEC Action Plan (LGAP). In partnership with the U.S. Environmental Protection Agency (EPA), a regulatory pathway was created for the approval of commercial sanitizers that can be applied to agricultural irrigation water to combat STEC, specifically E. coli O 157:H7. However, the protocol has several real-world limitations and economic consequences, such as the potential to overuse sanitizing products, thus adding disinfection by-products classified as pollutants. In addition, there have been several initiatives due to systems research on the local, state, and federal levels to provide technical assistance for the further improvement of Good Agricultural Practices (GAPs). This review considers the factors involved in leafy green production, such as agricultural water, climate change, and adjacent land use, contributing to increased susceptibility to pathogens contamination and how the implementation of sanitizers impacts food safety. The review discusses potential future improvements to agricultural water safety and quality in the context of improving food safety.
1 Introduction
Leafy greens are one of the most common sources of Shiga toxin-producing E. coli (STEC) contamination in Canada and the United States (U.S.), only preceded by ground beef (Marshall et al., 2020). Ninety percent of the leafy greens grown in the U.S. are harvested from California and Arizona (California Leafy Greens Marketing Agreement, 2020). Despite surveillance technologies designed to control pathogenic hazards in agriculture, leafy greens grown in California have been subjected to several high-profile foodborne illness outbreaks (U.S. Food and Drug Administration, 2020a; U.S. FDA, 2020b; U.S. FDA, 2021). The 2020 STEC outbreak, specifically E. coli O 157:H7, in the Central Coast of California represents a repeated series of outbreaks associated with leafy greens. Following the 2020 series of outbreaks, the FDA engaged with growers about the importance of using good agricultural practices (GAPs), especially as they apply to agricultural water. However, the geographical nuances of high production leafy green fields along with climate change patterns, complicate this issue.
The U.S. Food and Drug Administration (FDA), in partnership with the Center for Disease Control (CDC), and local officials, conducted a comprehensive investigation into the causes of perennial STEC outbreaks (U.S. Food and Drug Administration, 2020a; U.S. FDA, 2021; CDC, 2019). The FDA analysis of the outbreaks determined three key reoccurring trends in the contamination of leafy greens by STEC in 2018–2020: the presence of pathogenic E. coli, common geographical regions, and issues with activities on adjacent land (U.S. Food and Drug Administration, 2020a). The traceback analysis of outbreaks that occurred in 2018–2020 confirmed a positive match of the outbreak strain to samples of cattle feces collected from adjacent lands (U.S. Food and Drug Administration, 2020a; U.S. FDA, 2020c; U.S. FDA, 2021). This finding does not provide a definitive mechanism on how E. coli contaminates leafy greens during the production and harvesting of leafy greens (U.S. FDA, 2020b). Nevertheless, it does confirm the presence of STEC, which is linked to the outbreaks in California and is a potential perennial source of contamination (U.S. FDA, 2020b). However, most mechanisms of pathogens conveyance are speculative, and confirmation requires further research into the potential avenues of transference.
The FDA has published the Leafy Greens STEC Action Plan (LGAP) in response to the recurring outbreaks associated with leafy greens (U.S. FDA, 2020c). The LGAP plan emphasizes the creation of an agricultural ecosystem based upon close collaboration with stakeholders in public and private sectors to address knowledge gaps in leafy green production safety. For example, the FDA collaborated with U.S. Environmental Protection Agency (EPA) to create a label for commercial sanitizers that can be applied to agricultural irrigation water to combat STEC (U.S. FDA, 2020c). The EPA/FDA agricultural water protocol is intended to help stakeholders develop efficacy data on commercial sanitizers. However, the protocol has several real-world limitations and economic consequences, such as the potential to overuse sanitizing products, thus negatively impacting leafy green and soil quality.
In addition to the FDA, academic and local regulators, including the California Department of Food and Agriculture (CDFA), have facilitated the leafy greens industry’s proactive stance against the perennial occurrence of STEC outbreaks (California LGMA, 2020). The Leafy Greens Marketing Agreement (LGMA) is a coalition of growers that produce 90% of the leafy greens supplied to consumers in the U.S. LGMA members are voluntarily audited multiple times throughout the season by the U.S. Department of Agriculture (USDA)-certified inspectors (California LGMA, 2020). The audits are typically pre-season assessments that specifically address water, soil amendments, environmental factors, work practices, and field sanitation (California LGMA, 2020).
This review aims to analyze the recent STEC outbreaks on leafy greens in California and the potential role of agricultural water and adjacent land use in the contamination mechanism. The review considers the factors involved in leafy green production that could contribute to an increased susceptibility to pathogen contamination. Currently, the LGMA, FDA, and other stakeholders are actively working towards a solution to sustain safe leafy green production in California. A nuanced approach to the complex leafy-STEC problem in California is urgently needed.
2 Perennial outbreaks of pathogenic E. coli on lettuce in California
Over the last 2 decades, leafy greens and pre-packed salads have been associated with numerous incidences of multistate STEC contamination (CDC, 2019) (Table 1). The temporal and geographical nature of STEC outbreaks is non-random and highly correlates with the seasonal growth of leafy greens in California (Marshall et al., 2020; U.S. FDA, 2020b; CDC, 2019). The high correlation between STEC outbreaks and California may be a result of the large quantities of leafy green produce in the state coupled with a more advanced traceability system. While leafy greens are cultivated and harvested year-round in California, the peak of the STEC outbreaks happened in the winter months between October and April (Marshall et al., 2020). This correlates with the historical use of California’s Central Valley growing region to fill leafy green production gaps when it is too hot in California’s Central Coast and desert regions of California, Arizona (also fall), and Mexico (California LGMA, 2020; Marshall et al., 2020). Therefore, a closer investigation into the relationship between the growing practices in California, agricultural water, leafy greens, and STEC is warranted. However, leafy green outbreaks are inherently difficult to solve because of their short shelf-life, contributing to lag in identifying the outbreaks’ source (Marshall et al., 2020). In addition, the short duration of most outbreaks limits all opportunities for investigators to interview ill patients in a timely fashion. This limitation can hamper hypothesis generation and limit the options to test the product for contamination directly.
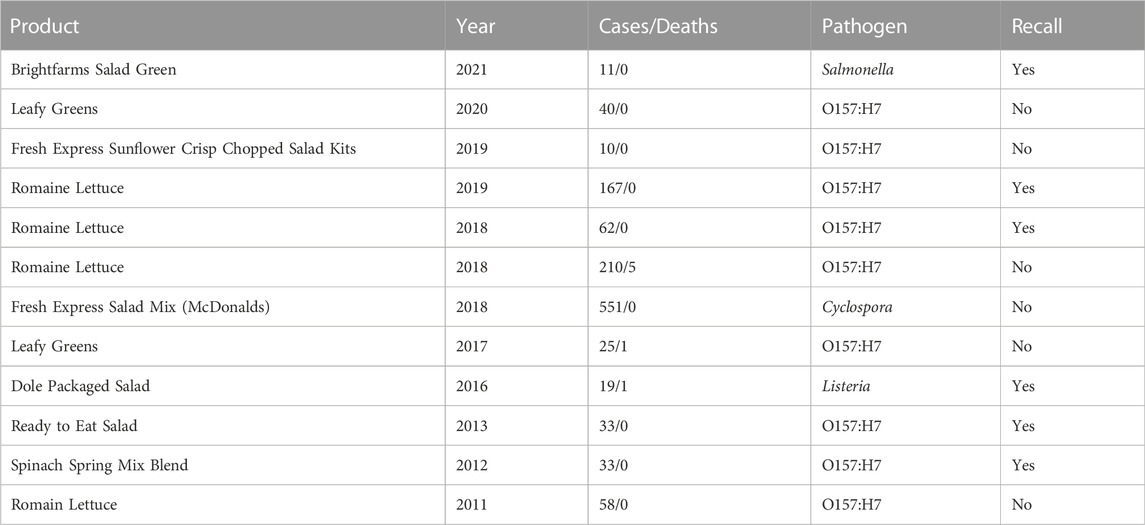
TABLE 1. CDC reported multistate outbreaks in leafy greens in the last 10 years (CDC, 2019).
2.1 The epidemiology of recent leafy green outbreaks
The FDA, along with CDC One Health, state, and local partners, investigated the multiple outbreaks of E. coli O 157:H7 in California from 2018 to 2020 (U.S. Food and Drug Administration, 2020a; U.S. FDA, 2020b; U.S. FDA, 2021)). In the fall of 2019, there were three separate outbreaks associated with romaine lettuce harvested from the Salinas Valley (U.S. Food and Drug Administration, 2020a; U.S. FDA, 2020b). In total, there were 176 reported illnesses and 27 states resulting in 85 hospitalizations and 15 cases of hemolytic uremic syndrome (HUS) (CDC, 2019). Whole-genome sequencing (WGS) determined that three outbreaks were caused by distinctly different E. coli O 157:H7 strains (CDC, 2019). Despite some genetic differences, the sampling from the suspected farms demonstrated overlaps in the supply chain of these outbreaks (U.S. FDA, 2021). This E. coli O 157:H7 strain demonstrated a close relationship to foodborne illnesses in 2018, 2019, and 2020 linked to leafy greens consumption and E. coli O 157:H7 strain isolated from clinical samples (i.e. from ill persons) in 2016 and 2017 (U.S. Food and Drug Administration, 2020a).
The epidemiological and traceback investigations confirmed a positive match of the outbreak strain to a sample of cattle feces collected uphill from where the contaminated leafy greens were grown (U.S. Food and Drug Administration, 2020a; U.S. FDA, 2020b)). The outbreak strains were genetically similar, but not identical, to strains recovered from outbreaks in 2016 and 2017 (U.S. Food and Drug Administration, 2020a; U.S. FDA, 2020b; U.S. FDA, 2021). In addition to the aforementioned strains, two additional STEC isolates were recovered from sampling areas around the Salinas Valley farms, not associated with any outbreak (U.S. Food and Drug Administration, 2020a). In the summer and fall of 2020, a STEC contamination, genetically similar to the 2019 outbreak, was associated with leafy greens (U.S. Food and Drug Administration, 2020a; U.S. FDA, 2021). To date, the traceback analysis was not able to identify the type of leafy green involved. Taken together, these outbreaks represent a troubling, reoccurring pattern of perennial contamination of leafy greens.
Investigators considered whether the application of contaminated agricultural water may have contributed to the spread of pathogenic E. coli. Although none of the farmers interviewed reported using the Salinas River water as an irrigation source, a positive test result of pathogenic E. coli was detected (U.S. Food and Drug Administration, 2020a). Each of the suspected farms had a procedure in place to treat water from the open source with sanitizer before water was used for irrigation during the growth of produce (Marshall et al., 2020; U.S. Food and Drug Administration, 2020a). However, with inherent delays in foodborne outbreak investigation, the investigators were unable to determine the effectiveness of the treatment that occurred prior to the contamination event (Marshall et al., 2020; U.S. Food and Drug Administration, 2020a).
Romaine lettuce may have some characteristics that make it more vulnerable to STEC contamination, including its shape and physiology (Marshall et al., 2020). Traceback efforts identified that 54% of the reported outbreaks were linked to romaine lettuce despite iceberg lettuce being the most harvested leafy green (Marshall et al., 2020). However, the link between an intrinsic vulnerability of romaine and pathogen contamination remains unclear. While certain cultivars of romaine lettuce have vascular systems that can potentially support pathogen internalization, the driving force for the recurring outbreaks is most likely rooted in environmental factors (Atwill et al., 2015). Most romaine lettuce shipments in the U.S. come from California’s Central Coast region from May to November and Arizona the rest of the year (Marshall et al., 2020). During 2017–2018, the three outbreaks of E. coli O 157:H7 in U.S. romaine lettuce all occurred at the tail end of California’s production season (CDC. 2018; U.S. Food and Drug Administration, 2020a). Consequently, the FDA recommended that suppliers begin labeling romaine packaging so that consumers can identify the product’s harvest region.
2.2 Historical context of STEC outbreaks in California
In 2006, a high-profile multistate outbreak of STEC was attributed to bagged spinach that was harvested in California (Gorny et al., 2006). The FDA and CDC confirmed 205 E. coli O 15:H7 illnesses associated with the outbreak, including thirty-one cases of the HUS, 104 hospitalizations, and four deaths (CDC, 2006). E. coli O 157 was isolated from 13 packages of spinach supplied by patients living in 10 states. The DNA fingerprints of all 13 of these E. coli match that of the outbreak strain (CDC, 2006). This prompted a historic nationwide recall of all pre-packed baby spinach in September of 2006 (CDC, 2006; Gorny et al., 2006). Many food safety officials consider the spinach outbreak as one of the critical events that lead-up to US Congress passing the Food Safety Modernization Act (FSMA) in 2011. This represented a huge shift in the mindset of regulators, suggesting that Good Agricultural Practices (GAPs) are not sufficient in preventing outbreaks (Smith et al., 2011). In addition, the 2006 outbreak prompted growers and retailers to form the LGMA in order the perform food safety audits in California and Arizona (Gorny et al., 2006). However, during the years since the passage of FSMA, STEC outbreaks are still prevalent in the California and Arizona leafy green production fields. The 2018 outbreak in romaine lettuce almost surpassed the 2006 outbreak in spinach (Gorny et al., 2006).
During the FDA’s investigation of the 2006 outbreak, federal and California officials found the outbreak strain in 13 bags of Dole brand baby spinach (CDC, 2006; Gorny et al., 2006). The investigation traced the product code to four fields in Monterey and San Benito counties in the Salinas Valley, CA. They traced the contamination to a Natural Selection Foods facility in San Juan Bautista, California (Ingram et al., 2011). Though E. coli O 157:H7 was found in environmental samples on all the farms, samples that matched the outbreak strain was limited to one farm in San Benito County (Gorny et al., 2006; Smith et al., 2011). The outbreak strain of E. coli was isolated from both cattle fields near the implicated spinach fields, and a wild boar was killed in one of the fields. Changes in groundwater levels during the 2006 growing season could have also contributed to contamination problems in the field. In March, the ranch’s groundwater levels were higher than the San Benito riverbed but fell to the riverbed’s level in July; the groundwater was subsequently dropped below the riverbed level later in the season (Ingram et al., 2011).
3 Adjacent land use issues in California
Numerous circumstances can undermine the safety of produce in the leafy green production environment, such as, drought, including time of year, method of irrigation, and soil type (Gorny et al., 2006). Pathogens may contaminate produce through atmospheric deposition, groundwater, poorly treated manure and compost, exposure to contaminated water by irrigation or flooding, transfer by insects, or fecal contamination generated by livestock (Growers, 2019; U.S. Food and Drug Administration, 2020a). Most of these contamination routes use water as an intermediate or direct vector (Growers, 2019; U.S. Food and Drug Administration, 2020a). Intensive efforts have been made to accurately understand the exact mechanisms of pathogens introduced into the leafy green production environment. Water contamination from adjacent land-use practices is a significant concern for the leafy greens industry (Liao et al., 2021). Problems with adjacent land have escalated because produce fields in the California growing region are often near animal production zones, thus entwining the ecological connections between wild animals, livestock, and produce (Strawn et al., 2013). Ecological biodiversity is needed to provide essential ecosystem services such as conserving natural cycles of resources (Liao et al., 2021). However, the presence of native fauna adjacent to produce fields may increase the risk of contamination of leafy greens by enteric bacteria. E. coli species are abundant in the digestive systems of mammals and birds, which survive in extra-host environments (e.g., soil) and could be dispersed across landscapes (Liao et al., 2021).
Ruminants, primarily cattle, are considered a significant reservoir for STEC, which can shed pathogens and directly or indirectly contaminate the irrigation water, runoff, or dust in the environment (Astill et al., 2020). Although the route of transmission from cattle to produce is unknown, plausible ways in which implicated leafy greens have become contaminated, including runoff from these nearby lands, direct transmission from animal operations to leafy green production fields, and application of agricultural water contaminated with fecal material (Álvarez et al., 2019; Astill et al., 2020). A comparison of pathogens of native wildlife found that the relative abundance of pathogen shedding was approximately 5% positivity rate of E. coli O 157:H7 for both cattle and wild pigs (Liao et al., 2021). One of the three growers in the 2019 outbreak investigation was adjacent to an active cattle rangeland with an 800-foot buffer zone enforced only during the growing season (Liao et al., 2021). Testing of nearby lands less than two miles upslope from a produce farm did return a positive sample that matched the genetics of the 2019 outbreak (Liao et al., 2021). This positive result was recovered from a sub-sample in which an on-farm investigator described it as visibly containing soil and manure (Whitman et al., 2006). Preliminary findings from the 2019 FDA environmental assessment suggest a correlation between STEC contamination and nearby soil and water used by cattle and pigs (U.S. FDA, 2020c).
Wild bird populations are widely recognized as potential causes of significant economic losses due to crop damage, and they pose a potential public health concern due to foodborne pathogen carriage (Tellez et al., 2001). Migratory bird flocks and small flocking insectivores/granivores may enhance the dispersal of E. coli populations across environments (Navarro-Gonzalez et al., 2020). In comparison, large mammals can be kept out of produce fields by adequate fencing. However, growers have expressed concerns about the challenges of minimizing wild bird intrusions and the subsequent potential for pathogen contamination of produce, soil, and water. A longitudinal study of Canadian geese in Colorado, USA, reported that the prevalence of E. coli ranged from 2% during the coldest months to 94% during the warmest time of the year (Navarro-Gonzalez et al., 2020). European starlings, which are a nuisance pest to agriculture, were also proposed to be a potential reservoir and vector of E. coli O 157:H7 and have been reported to carry and disseminate this human pathogen to cattle (Navarro-Gonzalez et al., 2020).
Wildlife-driven dispersal of E. coli is closely entwined with watershed and high forest coverage (Whitman et al., 2006). Forests may provide a pathway for larger mammals to move around and support a high density of migratory birds (Whitman et al., 2006). This increases the chance of fecal contaminants being dispersed from the forest to adjacent produce fields. However, observing the dispersal of soil E. coli in widespread produce fields was relatively limited. The lack of connectivity in agricultural areas may impede the movement of wildlife that disperses E. coli, adding additional buffer zones (U.S. Food and Drug Administration, 2020a). One hypothesis for the outbreaks is that the pathogen persists in animal reservoirs, potentially re-introducing contamination into the environment near the growing areas of romaine lettuce (Figure 1).
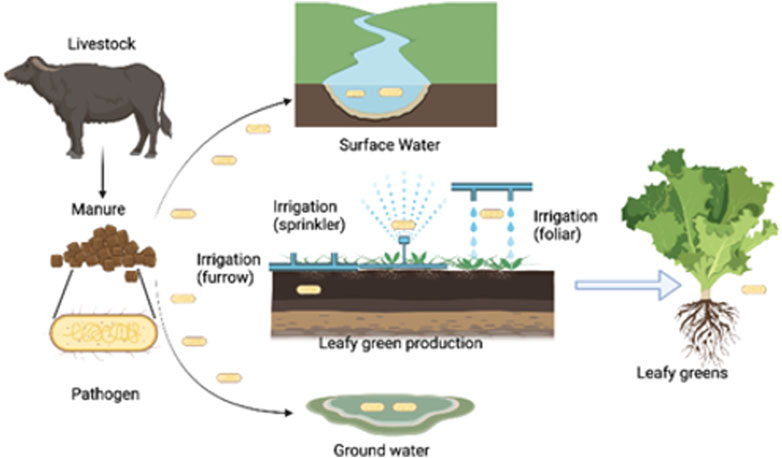
FIGURE 1. A potential route for the conveyance of pathogens in agriculture water systems via irrigation to the leafy green production environment. Plausible ways implicated leafy greens have become contaminated include runoff from these nearby lands, direct transmission from animal operations to romaine lettuce growing fields, and application of agricultural water contaminated with fecal material (California Department of Food and Agriculture. 2017).
3.1 Agricultural water as a conveyor of contamination
Agricultural water is recognized by the produce industry and food safety experts as a potential vector of enteric pathogens. The contamination mechanism varies based on the growing region because each farm has a distinct combination of environmental risk factors such as topography, land-use interactions, and climate (Gu et al., 2019; Marshall et al., 2020) (Figure 2). The watershed landscape is an important ecological driver and dispersal pattern of E. coli. Environmental stressors, such as limited availability of nutrients and water, presence of toxic molecules, and large alterations in temperature and moisture, can impact the transmission of E. coli (Gu et al., 2019; Marshall et al., 2020). Agricultural areas in such watersheds may have a higher risk of produce contamination due to fewer environmental constraints and a higher potential for dispersal of enteric bacteria between locations (Gu et al., 2019; Marshall et al., 2020).
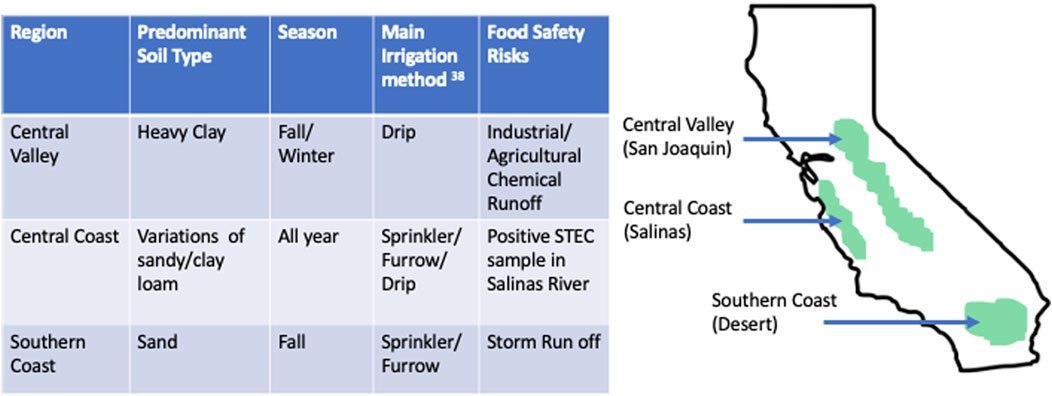
FIGURE 2. The main California leafy green cultivation regions, the most favored cultivation practice with regard to soil type and irrigation. In addition, recent examples of potential food safety risks associated with water quality are mentioned (Kalkhajeh, Y. K. et al. (2019).
In the United States, approximately 75% of the withdrawal from freshwater sources is dedicated to agricultural water, mostly utilized in irrigation infrastructure (Nurzaman, 2017; Gu et al., 2019). Irrigation and canal systems create complicated ecological environments with multiple potential sources and routes of pathogenic contamination (Nurzaman, 2017). Agricultural water transport via irrigation ditches and canals involves interaction with microbes dwelling in sediments and soils and transported through pipes containing potential biofilms (Nurzaman, 2017). Each irrigation subsystem: collection, replenishment, storage, conveyance, distribution off and on-farm, and on-farm application involves processes that have the potential to carry microbial contaminants (Marshall et al., 2020). The storage method for irrigation water can profoundly affect pathogen transmission because it involves the interaction with microbial reservoirs of bottom sediments, bank soils, and transport via pipes (Nurzaman, 2017). Other storage systems such as dams, impoundments, inter-basin transfer mechanisms, and reservoirs have been identified are additional harbinger points for pathogens (Riggio et al., 2019). Open-sourced water, such as reservoirs, ponds, and canals, is highly susceptible to contamination compared to groundwater (Growers, 2019). Industrial and municipal effluents from sewers, septic tanks, and storm drains can all potentially contaminate open water sources (Zimmer-Faust et al., 2020). Groundwater tends to be protected from microbial contamination, except if it has been exposed to surface runoff or other sources of contamination close to the aquifer. However, pathogens can survive for protracted periods in groundwater compared to surface water because groundwater is cooler and offers protection from UV light (Rothman et al., 2020). Intensive cattle production, and other farm operations, could lead to the leaching of pathogens such as E. coli in shallow groundwater, thereby contaminating it (Beckman et al., 2013). However, depending on the locality, groundwater may not be suited for leafy green production because of its mineral and salt content (Ingram et al., 2011).
How agricultural water is applied to leafy greens significantly impacts the risk of microbiological contamination (Figure 1). Overhead sprinkle irrigation poses a higher contamination risk than furrow and subsurface drip systems because drip irrigation minimizes contact between edible portions of certain plants (Growers, 2019; Álvarez et al., 2019). However, subsurface irrigation has been determined to influence the internalization of some pathogens in produce, such as spinach plants (Shen et al., 2017). According to some studies, the likelihood of internalizing pathogens increases when the organisms are introduced by water sprinkling systems as opposed to when the water is directly applied to the soil (Shen et al., 2017). In California, irrigation water is often transported via irrigation ditches and canals because of the naturally arid environment. In the southern desert, sprinklers are often used for the first five to 7 days until seedlings emerge, and then the field is furrowed and irrigated for the remainder of the season. Most fields in Central Coast are pre-irrigated to soften the soil for seeding and furrow irrigated using for is because of the strong winds. Central Valley’s major irrigation method is surface drip; this is because drip irrigation can potentially distribute water more uniformly than furrow or sprinklers and permit growers to water more frequently (Nurzaman, 2017). In most leafy green production, most water is applied in the last 30 days for harvest (California Leafy Greens Marketing Agreement, 2020).
Field studies showing the exact process of produce contamination through agricultural water are laborious and relatively scarce. Non-etheless, the awareness of microbiologically compromised agricultural water has increased. Researchers have investigated the retention of pathogens in manure pats and soils using “soil box” experiments and small field trials (Nyirabahizi et al., 2020). The research indicates that grassland buffers can retain between 95 and 99% of pathogens depending on the season but can fail significantly with heavy rain events. These studies indicate that most microbial species adhere to the manure matrix and a buffer zone of at least ten feet that is efficient in filtering out most pathogens (Nyirabahizi et al., 2020; Álvarez et al., 2019; Lothrop, N. et al., 2018). However, sufficient infiltration is needed, and soil compaction in the buffer zone could significantly reduce filtration efficacy. The Southern Coastal regions in California can experience fecal contamination in municipal water systems due to storm runoff during heavy rainfall events (Gu et al., 2019). Rain events are known to increase pathogen loading by carrying animal dropping from the ground to local streams and watersheds. Additionally, stormwater runoff can cause soil erosion and high water turbidity (Gu et al., 2019). This only compounds the risk of pathogen contamination since solar radiation is an important element in naturally reducing fecal contaminates. Climate change is only expected to exacerbate the risk of fecal cross-contamination in the California water supply because of the increased frequency and intensity of these weather patterns.
4 Regulatory response to perennial outbreaks
E. coli O 157:H7 is frequently implicated in water-related outbreaks because it can survive for an extended period in low-nutrient, high-acid, and low-temperature conditions (U.S. Food and Drug Administration, 2020a; U.S. FDA, 2021). The risk of pathogen contamination of agricultural water and conveyance to farms depends on numerous factors, such as water source, method of water storage, and water application method (U.S. Food and Drug Administration, 2020a; U.S. FDA, 2021; Nyirabahizi et al., 2020; Álvarez et al., 2019; Lothrop et al., 2018). The investigation discovered one water sample from the Salinas River tested positive for a non-outbreak strain of E. coli O 157:H7 (U.S. Food and Drug Administration, 2020a; U.S. FDA, 2021). Throughout the outbreak investigation, 12 subsamples positive for non-O157 E. coli, not associated with the outbreak, were recovered in the area around the farms (U.S. Food and Drug Administration, 2020a; U.S. FDA, 2021). While these strains may not be as virulent as the outbreak strains, the presence of Shiga toxic genes is an indicator of potential fecal contamination and increased pathogen load. Although the farmers did not report the use of the river for irrigation, it does implicate agricultural water in the mechanism of contamination. Each of the investigated growers had a procedure in place to treat water from an open-source with sanitizers before water is used during the growth of produce; however, investigators were unable to determine the effectiveness of treatment that occurred prior to their onsite visits (U.S. Food and Drug Administration, 2020a; U.S. FDA, 2021). Although none of the growers interviewed indicated using Salinas River water on their crops, the positive test results of pathogenic E. coli O 157:H7 drawn from the river emphasized the need to understand better the ecology, survival, and movement of human pathogens in the region (U.S. Food and Drug Administration, 2020a; U.S. FDA, 2021).
The safety of leafy greens has been at the forefront of FDA efforts to prevent foodborne illness (U.S. Food and Drug Administration, 2020a; U.S. FDA, 2020b; U.S. FDA, 2021). The FDA Food Safety Modernization Act (FSMA) established regulations that ensure water, soil amendments (e.g., fertilizer or compost), and food contact surfaces do not contribute to producing cross-contamination (U.S. FDA, 2021). The Produce Safety Rule (PSR) sets the minimum standards for growing, harvesting, packing, and holding fruits and vegetables for human consumption (U.S. FDA, 2015). This rule also encompasses standards for agricultural water, the application of biological soil amendments of animal origin, the prevention of contamination by grazing and working animals, worker health and hygiene, and farm infrastructure (U.S. Food and Drug Administration, 2020a; U.S. FDA, 2020b; U.S.FDA, 2021). Following the implementation of the PSR in 2015, the LGMA requirements were subsequently revised in 2017 to be in alignment. The recurrent outbreaks associated with leafy greens consumption sparked the FDA to take preventive measures by publishing the 2020 Leafy Greens STEC Action Plan (LGAP) (U.S. FDA, 2021). These measures are intended to support an integrated food safety system and help foster a more urgent, collaborative, and action-oriented approach between the regulators and stakeholders (U.S. Food and Drug Administration, 2020a; U.S. FDA 2020b; U.S. FDA, 2021).
In 2020, the EPA and FDA collaborated on a protocol that sets the treatment parameters and controls for studying the efficacy of agricultural water sanitizers (U.S. FDA, 2020d) This protocol was intended to help sanitizer companies develop data sets on the effectiveness of their products in inactivating human pathogens Listeria, E. coli, and Salmonella, in preharvest agricultural water. EPA’s oversite of this protocol ensures that companies may use the data to support the registration of new treatment products for preharvest agricultural water (U.S. Food and Drug Administration, 2020a; U.S. FDA, 2020b; U.S. FDA, 2021). Adopting this protocol, with rapid and widespread testing of multiple sanitizers, individually and in combination, would benefit the participants in the lettuce and leafy green production continuum. The protocol includes standards for the test water, such as concentration for turbidity, total organic carbons, total dissolved salts, pH, and the necessary controls for comparison (U.S. FDA, 2020d). This “worst-case scenario” in water quality is intended to represent the potential range of conditions. However, the rollout of this protocol has been cumbersome because there is no one-size-fits-all solution to mitigating the nuances of irrigation practices. The protocol is laborious, with multiple control treatments, and requires a BioSafety-2 level of containment for laboratory equipment (U.S. FDA, 2020d). The nuance of regional water quality creates a significant risk of the overuse of chemical sanitizers, leading to phytotoxicity, disruption of native microflora, and exacerbation of operating costs (Lothrop et al., 2018). In addition, the test water conditions tend to represent extremes in pH, turbidity, and organic load compared to the agricultural water used during the production season in the field (U.S. FDA, 2020d).
4.1 Current state of agriculture water sanitation
Mitigating foodborne pathogens is critical to farmers’ and producers’ economic, environmental, and social security. Agriculture water for irrigation is a major cost for leafy green growers. Water costs vary considerably depending on the water district or agency, delivery, associated fees, and pumping variables. The typically estimated cost of pumped water is $228-435 per acre in California’s Central Coast growing region (Calvin et al., 2017). Most irrigation systems are maintained by the landowner and assumed to be included in the land rental cost (Calvin et al., 2017). The grower invests in and owns sprinkler pipe and drip system materials sufficient for irrigation needs, including sanitizers (Calvin et al., 2017). The total water use will vary depending upon irrigation method, soil type, weather, and the time of the year the crop is planted. For example, soil with heavy clay will retain more water than sandy soil (Nurzaman, 2017). The cost of water treatment depends on the type of system implemented, with UV systems being the most expensive and chemical treatment with calcium or sodium hypochlorite being the least expensive (Nurzaman, 2017). Many growers, especially smaller farms, are concerned about the increasing cost of water sanitization and the effectiveness of the treatments.
4.2 Opportunity for improved implementation of the FDA/EPA agricultural water protocol
There is an urgent need for a reliable supply of agricultural water that meets the current LGMA metrics in the leafy green production continuum (California Leafy Greens Marketing Agreement, 2020). The USDA National Organic Standards has formally recommended that hypochlorite application dosage not exceed 4 ppm residue limit imposed by the Safe Drinking Water Act may be outside the efficacy window placed by the FDA/EPA protocol (USDA Agricultural Marketing Service, 2020). Specifically, more information on water treatment cost per acre, impacts on soil quality, pathogen tolerance, and antimicrobial resistance is needed. Furthermore, downstream biological effects, such as bacterial antibiotic resistance and alterations in native soil ecology, have been closely associated with using these traditional chemical interventions (Lothrop et al., 2018). Therefore, new tools are needed for growers that wish to follow water sanitation metrics.
It is essential to evaluate the environmental conditions that may affect agricultural water treatment efficacy. Performance parameters, such as turbidity, total suspended solids, pH, flow rate antimicrobial, and historical microbial background, impact the effectiveness of sanitizer treatments (Growers, 2019). In the leafy green vegetable production continuum, abiotic and biotic variables present during the growing and harvest seasons modulate the growth and survival of contaminating pathogenic microorganisms. However, public health concerns have been raised about the excessive use of chemicals in water and the potential health risk of chemical by-product accumulation in produce (Growers, 2019). In addition, the sanitizer contact time varies significantly with field size, pipe diameter, distance from the injection, pipe pressure, pump, and flow rate (Growers, 2019). Therefore, a systems approach is needed so that leafy green grower can sustainably adopt measures like the EPA/FDA protocol recommendations while meeting food safety goals.
Currently, LGMA standards require growers must treat their water 21 days before the scheduled harvest (California LGMA, 2020) (Table 2). Before treatment, growers must develop and maintain detailed standard operating procedures to keep up with industry standards on agricultural water (California LGMA, 2020). These procedures often require validation through a third-party laboratory that is certified to conduct a scientific study. Many of these studies focus on log reductions of target bacteria (described by EPA/FDA), total coliform, and the die-off rate of specific indicator organisms (Strawn et al., 2013). These log reductions are calculated before and after antimicrobial treatments to determine whether a sufficient reduction has occurred (Growers, 2019). However, with the mounting pathogenic outbreaks related to agricultural water, the FDA has proposed changes to the PSR. The most significant proposed change to the PSR is the removal of certain testing requirements for pre-harvest agricultural water and replacing them with agricultural water assessments (U.S. FDA, 2021). The proposed agricultural assessment would include an evaluation of the farm’s water system, agricultural water use practices, crop characteristics, environmental conditions, and other relevant factors.
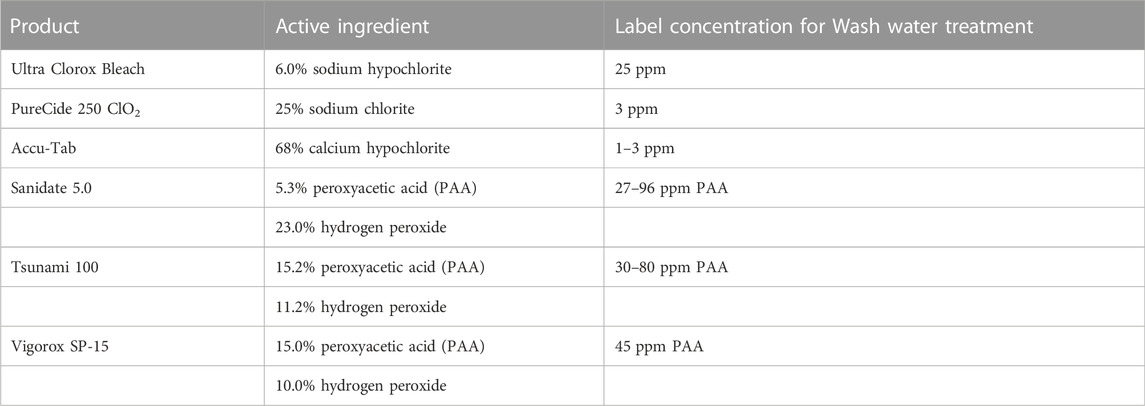
TABLE 2. Summary of sanitizing chemicals commonly used on many farms showing the differences in concentration.
4.3 System approach to agricultural water sanitation
There is a trade-off between priorities of environmental conservation and intensive agriculture, with limited ecological data supporting or refuting the role of wildlife in dispersing pathogens under normal operating conditions (Drinkwater et al., 2016). Agricultural water systems boundaries are often spatial and temporal boundaries defined by the structure of the underlying environment, socioeconomic and political structures, and land-use decisions made by farmers and farm communities (Drinkwater et al., 2016). However, agricultural watersheds are interconnected beyond human designation, and agricultural systems are composed of subsystems with unique properties. These systems are in constant flux due to a combination of local environmental events and global climate change (Drinkwater et al., 2016). Currently, the agricultural regions of California and the Southwestern United States, where 90% of leafy greens are grown, are facing extensive and increasing water constraints. Major droughts and other climate events have affected agricultural production while diminishing surface and groundwater reserves.
Farmers and ranchers across the country are uniquely affected by federal laws and the regulations based on those laws, often absorbing the cost of adherence. Studies have estimated the compliance costs for food safety standards recommended by the LGMA, like those required under the proposed PSR (Calvin et al., 2017). Growers need to ensure the safety of their produce and accommodate buyer requests by financially supporting in-house departments and/or staff especially dedicated to the supervision and management of these programs. A major cost of the food safety program is participation in third-party (independent) audits (Calvin et al., 2017). Costs associated with food safety programs vary from $50-100 per acre depending upon the farm, personnel cost, and inspection circumstances. In addition, a cost of $120 per acre per year or $60 per acre per crop is included in budget estimates for management and compliance with regulatory programs (Calvin et al., 2017). These tend to impact smaller farms more because of the resources needed for regulatory adherence.
The scarcity of water in California has contributed to the use of sub-optimal irrigation water sources. All 58 counties in California are now under a drought emergency proclamation, resulting from the driest winter in 100 years (Current drought conditions, 2021). Net water shortages for agriculture have most severely affected California’s Central Valley (CDFA, 2017). Further examination of agricultural water quality in the Central Valley is important because of its proximately to oil production and refineries (Smith et al., 2011). Drought will also pressure farmers to plant crops more appropriate for their regional water availability and climate. For example, water stress will favor lettuce production in moist coastal areas such as the Central Coast instead of inland regions where the crop’s water demand is high (California Leafy Greens Marketing Agreement, 2020). This has the potential to be a primary food safety issue because the FDA confirmed the presence of E. coli O 157:H7 in the Salinas River (U.S. FDA, 2021). These and other extreme weather events, like floods or forest fires, are also expected to be more frequent and will couple with fluctuations in precipitation and surface water supplies. The challenges that lie ahead are both extremely complex and locally diverse and will require a holistic approach. This approach will require better knowledge of the microbial risk, potential non-chemical alternatives, and consideration of the downstream biological effects.
Growers in California expressed a need for more knowledge on water quality, including microbiology and emerging contaminants as well as education on water treatment technologies (Hodson and Lewis, 2016). Communication with stakeholders about expected outcomes of food safety control measures has been essential for disseminating water treatment knowledge. However, a major barrier to implementation is that compliance costs must be estimated ahead of time, often with limited knowledge of current industry practices or likely adoption of response strategies. Therefore, researchers need to evaluate the critical input and parameters and generate statically sound assumptions.
Beneficial Management Plans (BMP) are designed based on the perceived risk and the primary need to address the potential pathogen load of adjacent lands. A major part of the risk analysis process is determining the pathogen load in areas adjacent to the watershed and potential avenues for excessive loading. The BMP is designed to address the pathogen load mostly relying on environmental factors such as time, distance, and Sun exposure that result in a natural decline in the microbial population. The EPA and FDA released a testing protocol to facilitate data development on the efficacy of sanitizers on specific foodborne pathogens in agricultural water (U.S. FDA, 2020b). This protocol is intended to standardize the procedure for sanitizer manufacturers and providers to expedite the process of obtaining EPA label approval. However, the direct cost of meeting water quality standards required for various uses (pre-and post-harvest) can be improved by providing the stakeholders with the most applicable, effective, and safe intervention technologies tailored for their handling of available water resources. More scientific research is needed for the protocol to be implemented sustainably in the leafy green production fields. As well as looking into preventive measures.
5 Potential future improvement to agricultural water safety
The current system of regulations that govern water quality relies on decades-old technology to determine if a water body is acceptable for use. Total coliforms, fecal coliforms, and generic E. coli are currently used as indicators of water quality sanitation. However, relying on coliforms as indicators of pathogen presence often leads to false-positive because generic E. coli is exponentially more abundant in watersheds than any other microbial pathogens. Various molecular techniques for improving public health have been examined for their potential to provide a scientific basis and to address emerging foodborne diseases. However, when the goal of such control measures is expressed in a numerical value (e.g., a specified reduction of a particular foodborne), the implementation of preventive measures may not be obtainable (Drinkwater et al., 2016). For example, the target of the “3 log CFU/ml” reduction is a common goal post used by the EPA/FDA protocol to determine human pathogen label claims (U.S. FDA, 2020d). This standard is often applied to post-harvest sanitation in the processing environment when the sanitizer is applied to hard surfaces or wash water. However, the preharvest field environment is more dynamic than the post-harvest processing setting. Therefore, novel methods that can operate at an immense scale are needed to control foodborne pathogen.
Currently, regulators are shifting to a systems approach to prevent pathogen contamination in the leafy green production continuum (Drinkwater et al., 2016). An annual assessment by farms of their pre-harvest agricultural water to identify any conditions likely to introduce hazards into or onto covered produce or food contact surfaces claims (U.S. FDA, 2021). Based on these assessments, farms would then determine whether corrective or mitigation measures are reasonably necessary to reduce the potential for contamination. The assessment would include an evaluation of the farm’s water system, agricultural water use practices, crop characteristics, environmental conditions, and other relevant factors, such as the results of any testing conducted to inform the assessment claims (U.S. FDA, 2021).
5.1 Improved sampling and pathogen detection
The bacterial load can change throughout the vertical water column in water stream length and width (Whitman et al., 2006; Llirós et al., 2010; Lothrop et al., 2018). A single water sample inaccurately shows water quality during the irrigation period, and microbial water acceptability should not be drawn from a limited number of water quality measurements (Won et al., 2013). These dynamics necessitate multiple water samples for analysis to adequately represent the real water quality in the irrigation channels and canals (Lothrop et al., 2018). Sampling techniques such as manual, automated, passive, and flow-proportional can be used for surface water runoff (Kalkhajeh et al., 2019).
The PSR and LGMA require leafy green growers to monitor agricultural water quality and test for the presence of coliforms and other adulterants. Initially, a microbial water quality profile (MWQP) is assessed by sampling untreated agricultural water used during growing periods and collecting 20 samples over two to 4 years (U.S. FDA, 2015). The Equivalent Testing Methodologies for Agricultural Water list released by the FDA describes several quantitative and qualitative approaches that can be applied for testing any agricultural water. The testing frequency is based on the water source; four or more samples are required for groundwater, while 20 or more samples are for needed for surface water. Different water analysis methods are suitable for agricultural water, e.g., quantitative for pre-harvest and qualitative or quantitative tests for harvest or post-harvest handling (U.S. FDA, 2015). Sampling depth should be within 6–12 inches range below the water surface for surface water samples.
Recent studies have shown improvements in agricultural water sampling methods that can potentially assist farmers and growers. The choice of water sampling method has a significant impact on pathogen detection, and a larger volume (>100 ml) should be analyzed for an accurate evaluation of irrigation water for pathogen contamination (Sbodio et al., 2013). Culture methods have remained the gold standard for detecting pathogens from environmental samples. Advances in molecular-based methods, such as polymerase chain reaction (PCR), have emerged as reliable tools that can indicate the presence of pathogens (Summa et al., 2012). However, the complexity of environmental samples requires parallel detection methods for multiple targets to increase the breadth, sensitivity, and specificity of the detection of significant pathogens. Novel capture elements, such as bacteriophages integrated into an electrochemical-based detection system, can successfully capture STEC strains in surface water (Quintela and Wu, 2020).
The traditional Moore swab technique has been modified with a cassette which has been demonstrated to be effective in trapping and capturing STEC O157:H7 and Salmonella spp. In a large volume of irrigation water samples (Sbodio et al., 2013). However, it was observed that the prevalence of Listeria spp. Was reduced when the modified Moore swab (MMS) method was used as compared to grab samples with 0.45 µm filters (Cha et al., 2016). Binding affinity and attachment of bacteria to surfaces are shown to be significantly influenced by the structures, hydrophobicity, charge, and cell size (Bisha et al., 2014). When MMS was coupled with a colorimetric paper-based method, E. coli, Salmonella spp., and L. monocytogenes were successfully detected from large volumes (10 L) of agricultural waters (Bisha et al., 2014).
The concentration and dynamics of E. coli at the water intake during irrigation have been estimated based on 3-D distribution, which revealed hotspots at various depths across a pond. This hydrodynamic modeling can be a meaningful tool for enhancing microbial water quality monitoring efforts. In Southern Georgia, it was found that no significant differences in Salmonella spp. Or E. coli detection rates or bacterial load between sampling at the bank closest to the pump intake as compared to sampling from the bank around the pond perimeter (Lee et al., 2018). However, samples collected from the bank nearest to the intake had a higher level of agreement with the intake than those collected around the pond perimeter (Lee et al., 2018). This key information can facilitate best practices for surface water sampling. Artificial intelligence tools have also allowed screening of agricultural surface waters for specific pathogens populations, such as by training algorithms to predict the presence or absence of Salmonella spp. based on E. coli populations measured in other water environments (Polat et al., 2020).
5.2 Bacteriophages
Bacteriophages (or phages), first discovered in the 20th century, are a type of virus that can infect bacteria via lytic infection for viral replication (Twort, 1915). Phage therapy was first documented to treat plague and cholera and has been widely utilized to combat antibiotic-resistant strains in the medical field (Lewis and Hill, 2020). The FDA has approved several commercial phage products to control foodborne pathogens, such as STEC, Listeria, and Salmonella. All approved products are generally recognized as safe (GRAS) and can be applied directly to food items (Lewis and Hill, 2020). With a high tolerance to external stress, phages can be applied under various conditions (Álvarez et al., 2019). Additionally, they can be used with other antimicrobial agents as the hurdle interventions to increase antimicrobial activity while reducing the use of the antimicrobial agent, which could subsequently result in the development of antimicrobial resistance. In comparison to the post-harvest application, the information regarding the use of phages in the preharvest environment is scarce, even though the technology has been used to improve wastewater treatment and drinking water quality (Mathieu et al., 2019).
Unlike common chemical and physical treatments, phage application could resolve the frequent drawbacks deriving from using traditional intervention technologies such as antibiotic resistance. Phages can co-evolve with their bacterial host to reduce the development of phage resistance, and the quality impact on the phage-treated food products could be minimal (Oh and Park, 2017). In addition, genomic characterization of the newly isolated phages is necessary to ensure the phages are free of unwanted genes and any safety concerns (Liao et al., 2019; Zhang et al., 2021).
Considering opportunities for phage-based control in the pre-harvest environment is promising to reduce bacterial contamination levels. A previous study indicated that phage application was able to reduce the abundance of antibiotic-resistant pathogenic bacteria, such as E. coli and Pseudomonas, in the agricultural soil contaminated by livestock farming nearby (Ye et al., 2018). Another study incorporated phage application in irrigation water to effectively reduce the plant pathogen, Ralstonia solanacearum, among 300 plants (Álvarez et al., 2019). Our previous study indicated a strong in vitro antimicrobial activity of a novel phage against STEC in an aqueous environment by approximately five log within 4 hours (Liao et al., 2019). Though no direct research results were found on reducing foodborne pathogens in irrigation, these findings strongly suggest the potential of phage technology as a promising alternative technology to improve the deficiency of the current intervention technologies. The information regarding in-field phage applications with efficient delivery methods to increase the opportunity to encounter the target bacteria requires future studies.
5.3 Potential development of antimicrobial resistance due to repeated exposure to chlorine compounds
All aerobic microorganisms manage reactive oxygen species that accumulate in cells as products of the incomplete reduction of molecular oxygen. However, a protective system in a low-nutrient environment that contains variable concentrations of oxidative species, such as chlorine, can emerge as an adaptive response to repeated use. Starved cells showed significantly higher disinfection tolerance than normal cells based on the inactivation curves for both chlorine and monochloramine (Ingram et al., 2011). Consequently, the ability of E. coli to remain physiologically active and develop resistance to chlorine after sublethal stresses have potential public health implications. It may require further water treatment practices, particularly where the disinfectant residual in the water is less than 0.1 mg of chlorine/liter (Han et al., 2000). The problem of bacterial antimicrobial resistance has been closely associated with using these traditional chemical interventions, potentially increasing human health risks via the emergence of superbugs (Nyirabahizi et al., 2020). In contrast, alternative methods, such as phages, the natural predators of bacteria, are being adopted in the industry. Current interest in phage application is primarily driven by the threat of antimicrobial resistance and the growing need for natural antimicrobials. Because phages can evolve with the constant changing of their bacterial hosts, they have been considered alternative biocontrol agents to antibiotics. Phage biocontrol represents a promising intervention in the agricultural area because it is eco-friendly, host-specific, and easy to manufacture (Svircev et al., 2018).
6 Conclusion
The ever increasing demand for fresh leafy green requires a holistic approach to food safety and resource management. In the leafy green vegetable production continuum, abiotic and biotic variables influence conditions of the surface of leafy green that ultimately modulate the efficacy of water sanitation reduction in the presence of pathogen microbes. Mitigating contaminated water by foodborne pathogens, such as STEC strains, is critical to leafy green farmers’ and producers’ economic, environmental, and social security. While there is no “silver bullet” for the reoccurring outbreaks, Beneficial Management Plans (BMP) are designed based on the perceived risk, and can address the potential pathogen load of adjacent lands. The BMP is designed to address the pathogen load mostly relying on environmental factors such as time, distance, and Sun exposure that result in a natural decline in the microbial population. A major part of the risk analysis process is determining the pathogen load in areas adjacent to the watershed and potential avenues for excessive loading, which the FDA is taking under advisement when revising the PSR. Future revisions to the PSR and other food safety measures are trending towards a system-based approach that can aid in a productive and safe leafy green production continuum for all participants.
Author contributions
AL contributed to the conceptualization and design, reviewed the literature, prepared the figures and tables, and wrote the original draft of the manuscript. IQ and YT contributed to the conceptualization and design and wrote, reviewed, and edited the manuscript. VCHW contributed to the conceptualization, supervised the data, carried out the project administration, and reviewed and edited the manuscript.
Funding
This work was supported by the United States Department of Agriculture National Institute of Food and Agriculture (USDA NIFA) Specialty Crop Research Initiative (SCRI) Grant (award number 2021-51181-35905).
Conflict of interest
The authors declare that the research was conducted in the absence of any commercial or financial relationships that could be construed as a potential conflict of interest.
Publisher’s note
All claims expressed in this article are solely those of the authors and do not necessarily represent those of their affiliated organizations, or those of the publisher, the editors and the reviewers. Any product that may be evaluated in this article, or claim that may be made by its manufacturer, is not guaranteed or endorsed by the publisher.
References
Álvarez, B., López, M. M., and Biosca, E. G. (2019). Biocontrol of the major plant pathogen Ralstonia solanacearum in irrigation water and host plants by novel waterborne lytic bacteriophages’, Frontiers in microbiology. Frontiers 0, 2813. doi:10.3389/FMICB.2019.02813
Astill, G. M., Kuchler, F., Todd, J. E., and Page, E. T. (2020). ‘Shiga Toxin-Producing Escherichia coli (STEC) O157:H7 and romaine lettuce: Source labeling, prevention, and business’, American Journal of Public Health. Am. J. Public Health 110 (3), 322–328. doi:10.2105/AJPH.2019.305476
Atwill, E. R., Chase, J. A., Oryang, D., Bond, R. F., Koike, S. T., Cahn, M. D., et al. (2015). Transfer of Escherichia coli O157:H7 from simulated wildlife scat onto romaine lettuce during foliar irrigation. J. Food Prot. 78 (2), 240–247. doi:10.4315/0362-028X.JFP-14-277
Beckman, S., Materna, B., Goldmacher, S., Zipprich, J., D'Alessandro, M., Novak, D., et al. (2013). Evaluation of respiratory protection programs and practices in California hospitals during the 2009-2010 H1N1 influenza pandemic. Am. J. Infect. Control 41 (11), 1024–1031. doi:10.1016/j.ajic.2013.05.006
Bisha, B., Adkins, J. A., Jokerst, J. C., Chandler, J. C., Perez-Mendez, A., Coleman, S. M., et al. (2014). Colorimetric paper-based detection of Escherichia coli, Salmonella spp., and Listeria monocytogenes from large volumes of agricultural water. J. Vis. Exp. JoVE. MyJoVE Corp. 88 (88), 51414. doi:10.3791/51414
California Department of Food and Agriculture, (2017). Cdfa - statistics. California Department of Food and Agriculture. Available at: https://www.cdfa.ca.gov/Statistics/ (Accessed: July 27, 2021).
California Drought Action (2022). Current drought conditions. Available at: https://drought.ca.gov/current-drought-conditions/ (Accessed: July 7, 2022).
California Leafy Greens Marketing Agreement. Food safety program (2020). Available at: https://lgma.ca.gov/food-safety-program?tab=3#downloads (Accessed: 3 March 2022).
Calvin, L., Jensen, H., Klonsky, K., and Cook, R. (2017). United States department of agriculture food safety practices and costs under the California leafy greens marketing agreement. Available at: www.ers.usda.gov/publications (Accessed May 18, 2021).
Centers for Disease Control, (2006). Multistate outbreak of E. coli O157:H7 InfectionsLinked to fresh spinach (FINAL UPDATE). Available at: https://www.cdc.gov/ecoli/2006/spinach-10-2006.html (Accessed: July 27, 2021).
Centers for Disease Control (2019). National outbreak reporting system (NORS). Atlanta, GA: Centers For Diasease Control and Prevention, 1–6. Available at: https://wwwn.cdc.gov/norsdashboard/ (Accessed July 27, 2021).
Centers for Disease Control (2019). Outbreak of E. coli infections linked to RomaineLettuce. Available at: https://www.cdc.gov/ecoli/2018/o157h7-11-18/index.html (Accessed: November 22, 2022).
Cha, J. Y., Han, S., and Hong, H. J. (2016)., 10. Berlin Germany: Nature Publishing Group, 119–129. doi:10.1038/ismej.2015.95Microbial and biochemical basis of a Fusarium wilt-suppressive soilISME J.1
Drinkwater, L. E., Friedman, D., and Buck, L. (2016). Innovative solutions to complex challenges. Available at: http://www.sare.org/Learning-Center/Books/Systems-Research-for-Agriculture.
Gorny, J. R., Hank, G., Gombas, D., and Means, K. (2006). Commodity specific food safety guidelines for the lettuce and leafy greens supply chain, 45. Available at: http://www.fda.gov/downloads/Food/GuidanceRegulation/UCM169008.pdf.
Gu, G., Yin, H. B., Ottesen, A., Bolten, S., Patel, J., Rideout, S., et al. (2019). Microbiomes in ground water and alternative irrigation water, and spinach microbiomes impacted by irrigation with different types of water. Phytobiomes J. 3 (2), 137–147. doi:10.1094/PBIOMES-09-18-0037-R
Han, Y., Linton, R. H., Nielsen, S. S., and Nelson, P. E. (2000). Inactivation of Escherichia coli O157:H7 on surface-uninjured and -injured green pepper (Capsicum annuum L.) by chlorine dioxide gas as demonstrated by confocal laser scanning microscopy. Acad. Press, 17, 643–655. doi:10.1006/fmic.2000.0357
Hodson, A., and Lewis, E. (2016). Managing for soil health can suppress pests. Calif. Agric. 70 (3), 137–141. doi:10.3733/ca.2016a0005
Ingram, D. T., Pate, J., and Sharma, M. (2011). Effect of repeated irrigation with water containing varying levels of total organic carbon on the persistence of Escherichia coli O157:H7 on baby spinach. J. Food Prot. 74 (5), 709–717. doi:10.4315/0362-028X.JFP-10-426
Kalkhajeh, Y. K., Jabbarian Amiri, B., Huang, B., Henareh Khalyani, A., Hu, W., Gao, H., et al. (201920191889). Methods for sample collection, storage, and analysis of freshwater phosphorus. Multidiscip. Digit. Publ. Inst. Vol. 1111 (9), 1889. doi:10.3390/W11091889
Lee, D., Tertuliano, M., Vellidis, G., Harris, C., Grossman, M. K., Rajeev, S., et al. (2018). Evaluation of grower-friendly, science-based sampling approaches for the detection of Salmonella in ponds used for irrigation of fresh produce’, Foodborne Pathogens and Disease. Foodborne Pathog. Dis. 15 (10), 627–636. doi:10.1089/fpd.2018.2441
Lewis, R., and Hill, C. (2020)., 61. Elsevier Current Trends, 38–44. doi:10.1016/J.COPBIO.2019.09.018Overcoming barriers to phage application in food and feedCurr. Opin. Biotechnol.
LGMA (2020). The California leafy greens marketing agreement. Available at: https://lgma.ca.gov/news/audit_data_water (Accessed: May 18, 2021).
Liao, J., Bergholz, P., and Wiedmann, M. (2021). ‘Adjacent terrestrial landscapes impact the biogeographical pattern of soil Escherichia coli strains in produce fields by modifying the importance of environmental selection and dispersal’, Applied and Environmental Microbiology. Am. Soc. Microbiol. (ASM) 87 (6), e02516-20–e02517. doi:10.1128/AEM.02516-20
Liao, Y. Te, Salvador, A., Harden, L. A., Liu, F., Lavenburg, V. M., Li, R. W., et al. (2019). ‘Characterization of a lytic bacteriophage as an antimicrobial agent for biocontrol of shiga toxin-producing Escherichia coli o145 strains’, Antibiotics. MDPI Ag. 8 (2), 74. doi:10.3390/antibiotics8020074
Llirós, M., Gich, F., Plasencia, A., Auguet, J-C., and Darchambeau, F. (2010). Vertical distribution of ammonia-oxidizing crenarchaeota and methanogens in the epipelagic waters of Lake Kivu (Rwanda-Democratic Republic of the Congo). Appl. Environ. Microbiol 76 (20), 6853. doi:10.1128/AEM.02864-09
Lothrop, N., Bright, K. R., Sexton, J., Walker, J. P., Reynolds, K. A., and Verhougstraete, M. P. (2018)., 199. Elsevier, 86–92. doi:10.1016/j.agwat.2017.12.018Optimal strategies for monitoring irrigation water qualityAgric. Water Manag.
Marshall, K. E., Hexemer, A., Seelman, S. L., Fatica, M. K., Blessington, T., Hajmeer, M., et al. (2020). Emerging infectious diseases • www lessons learned from a decade of investigations of shiga toxin-producing Escherichia coli outbreaks linked to leafy greens. U. S. Canada’ 26 (10), 2319–2328. doi:10.3201/eid2610.191418
Mathieu, J., Yu, P., Zuo, P., Da Silva, M. L. B., and Alvarez, P. J. J. (2019). Going viral: Emerging opportunities for phage-based bacterial control in water treatment and reuse’, Accounts of Chemical Research. Am. Chem. Soc. 52 (4), 849–857. doi:10.1021/acs.accounts.8b00576
Navarro-gonzalez, N., Wright, S., Aminabadi, P., Gwinn, A., Suslow, T. V., and Jay-Russell, M. T. (2020). Carriage and subtypes of foodborne pathogens identified in wild birds residing near agricultural lands in California: A repeated cross-sectional study. Appl. Environ. Microbiol. 86 (3), 016788-e1719. doi:10.1128/AEM.01678-19
Nyirabahizi, E., Tyson, G. H., Dessai, U., Zhao, S., Kabera, C., Crarey, E., et al. (2020). Evaluation of Escherichia coli as an indicator for antimicrobial resistance in Salmonella recovered from the same food or animal ceca samples. Food control., 115, 107280. doi:10.1016/j.foodcont.2020.107280
Oh, J. H., and Park, M. K. (2017). ‘Recent trends in Salmonella outbreaks and emerging technology for biocontrol of Salmonella using phages in foods: A review’, Journal of Microbiology and biotechnology. J. Microbiol. Biotechnol. 27 (12), 2075–2088. doi:10.4014/jmb.1710.10049
Pathak, T. B., Maskey, M., Dahlberg, J., Kearns, F., Bali, K., and Zaccaria, D. (2018). Climate change trends and impacts on California agriculture: A detailed review’, agronomy. MDPI Ag. 8 (3), 25. doi:10.3390/AGRONOMY8030025
Polat, H., Topalcengiz, Z., and Danyluk, M. D. (2020). Prediction of Salmonella presence and absence in agricultural surface waters by artificial intelligence approaches Journal of Food Safety. John Wiley Sons, Ltd 40 (1), e12733. doi:10.1111/JFS.12733
Quintela, I. A., and Wu, V. C. H. (2020). A sandwich-type bacteriophage-based amperometric biosensor for the detection of Shiga toxin-producing Escherichia coli serogroups in complex matrices. RSC Adv. R. Soc. Chem. 10 (59), 35765–35775. doi:10.1039/D0RA06223E
Riggio, G. M., Jones, S. L., and Gibson, K. E. (2019). Risk of human pathogen internalization in leafy vegetables during lab-scale hydroponic cultivation. Horticulturae 5 (1), 25–22. doi:10.3390/horticulturae5010025
Rothman, J. A., Loveless, T. B., Griffith, M. L., Steele, J. A., Griffith, J. F., and Whiteson, K. L. (2020). Metagenomics of wastewater influent from southern California wastewater treatment facilities in the era of COVID-19’, microbiology resource announcements. Am. Soc. Microbiol. 9 (41). doi:10.1128/mra.00907-20
Sbodio, A., Maeda, S., Lopez-Velasco, G., and Suslow, T. V. (2013). Modified Moore swab optimization and validation in capturing E. coli O157:H7 and Salmonella enterica in large volume field samples of irrigation water. FRIN 51, 654–662. doi:10.1016/j.foodres.2013.01.011
Shen, Z., Mustapha, A., Lin, M., and Zheng, G. (2017). Biocontrol of the internalization of Salmonella enterica and Enterohaemorrhagic Escherichia coli in mung bean sprouts with an endophytic Bacillus subtilis. Int. J. Food Microbiol. 250, 37–44. doi:10.1016/j.ijfoodmicro.2017.03.016
Smith, R., et al. (2011). Leaf lettuce production in California’, leaf lettuce Production in California. Berkeley: University of California. doi:10.3733/UCANR.7216
Strawn, L. K., Fortes, E. D., Bihn, E. A., Nightingale, K. K., Grohn, Y. T., Worobo, R. W., et al. (2013). Landscape and meteorological factors affecting prevalence of three food-borne pathogens in fruit and vegetable farms. Appl. Environ. Microbiol. 79 (2), 588–600. doi:10.1128/AEM.02491-12
Summa, M., von Bonsdorff, C. H., and Maunula, L. (2012). Evaluation of four virus recovery methods for detecting noroviruses on fresh lettuce, sliced ham, and frozen raspberries. J. Virological Methods 183 (2), 154–160. doi:10.1016/j.jviromet.2012.04.006
Svircev, A., Roach, D., and Castle, A. (2018). Framing the future with bacteriophages in agriculture’, Viruses. MDPI Ag. 10 (5), 218. doi:10.3390/v10050218
Tellez, G., Petrone, V. M., EscorciaM., , Morishita, T. Y., Cobb, C. W., ViLLasenor, L., et al. (2001). Evaluation of avian-specific probiotic and Salmonella enteritidis-Salmonella typhimurium-and Salmonella Heidelberg-specific antibodies on cecal colonization and organ invasion of Salmonella enteritidis in broilers’, Journal of Food Protection. IAMFES 64 (3), 287–291. doi:10.4315/0362-028X-64.3.287
Twort, F. W. (1915)., 186. Elsevier, 1241–1243. doi:10.1016/S0140-6736(01)20383-3An investigation on the nature of ultra-microscopic virusesLancet4814
United States Department of Agriculture Agricultural Marketing Service (2020) 5026: The use of chlorine materials in organic production and handling | Washington, DC: Agricultural Marketing Service. Available at: https://www.ams.usda.gov/rules-regulations/organic/handbook/5026 (Accessed: 18 May 2021).
United States Food & Drug Administration (2020a). Factors potentially contributing to the contamination of leafy greens implicated in the fall 2020 outbreak of E. coli O157:H7. Available at: https://www.fda.gov/food/outbreaks-foodborne-illness/outbreak-investigation-e-coli-romaine-salinas-california-november-2019 (Accessed: June 25, 2021).
United States Food & Drug Administration (2015). Fda at a glance key requirements: Final rule on produce safety, 1–5. Available at: http://www.fda.gov/downloads/Food/GuidanceRegulation/FSMA/UCM472887.pdf (Accessed June 25, 2021).
United States Food & Drug Administration (2020b) FDA proposes changes to food safety modernization Act rule to enhance safety of agricultural water used on produce. Silver Springs, MD: FDA. Available at: https://www.fda.gov/news-events/press-announcements/fda-proposes-changes-food-safety-modernization-act-rule-enhance-safety-agricultural-water-used (Accessed: 7 July 2022).
United States Food & Drug Administration (2021) FDA releases investigation report following fall 2020 outbreak of E. coli O157:H7 illnesses linked to leafy greens | Silver Springs, MD: FDA. Available at: https://www.fda.gov/news-events/press-announcements/fda-releases-investigation-report-following-fall-2020-outbreak-e-coli-o157h7-illnesses-linked-leafy (Accessed: 18 May 2021).
United States Food & Drug Administration (2020c). Outbreak investigation of E. coli: Romaine from Salinas, California. Silver Springs, MD: U.S. Food & Drug Administration. Available at: https://www.fda.gov/food/outbreaks-foodborne-illness/outbreak-investigation-e-coli-romaine-salinas-california-november-2019 (Accessed: June 25, 2021).
United States Food and Drug Administration (2020d). United States Environmental Protection Agency Washington, dc 20460 office of chemical safety and pollution prevention Formulation from the Label. N/A.
Whitman, R. L., Nevers, M. B., and Byappanahalli, M. N. (2006). Examination of the watershed-wide distribution of Escherichia coli along southern lake Michigan: An integrated approach’, Applied and environmental microbiology. Am. Soc. Microbiol. 72 (11), 7301–7310. doi:10.1128/AEM.00454-06
Won, G., Kline, T. R., and LeJeune, J. T. (2013). Spatial-temporal variations of microbial water quality in surface reservoirs and canals used for irrigation. Agric. Water Manag. 116, 73–78. doi:10.1016/J.AGWAT.2012.10.007
Ye, M., Sun, M., Zhao, Y., Jiao, W., Xia, B., Liu, M., et al. (2018). ‘Targeted inactivation of antibiotic-resistant Escherichia coli and Pseudomonas aeruginosa in a soil-lettuce system by combined polyvalent bacteriophage and biochar treatment’, Environmental Pollution. Environ. Pollut. 241, 978–987. doi:10.1016/j.envpol.2018.04.070
Zhang, Y., Liao, Y-T., Salvador, A., Lavenburg, V. M., and Wu, V. C. H. (2021). Characterization of two new shiga toxin-producing Escherichia coli O103-infecting phages isolated from an organic farm. Microorganisms Zurich, Switzerland 99 (7), 15271527. doi:10.3390/MICROORGANISMS9071527
Keywords: lettuce, leafy greens, STEC, E. coli, California
Citation: Lacombe A, Quintela IA, Liao Y-T and Wu VCH (2022) Shiga toxin-producing Escherichia coli outbreaks in California’s leafy greens production continuum. Front. Food. Sci. Technol. 2:1068690. doi: 10.3389/frfst.2022.1068690
Received: 13 October 2022; Accepted: 13 December 2022;
Published: 22 December 2022.
Edited by:
Manoj Kumar Solanki, University of Silesia in Katowice, PolandReviewed by:
Kim Stanford, University of Lethbridge, CanadaAnna Szczerba-Turek, University of Warmia and Mazury in Olsztyn, Poland
Copyright © 2022 Lacombe, Quintela, Liao and Wu. This is an open-access article distributed under the terms of the Creative Commons Attribution License (CC BY). The use, distribution or reproduction in other forums is permitted, provided the original author(s) and the copyright owner(s) are credited and that the original publication in this journal is cited, in accordance with accepted academic practice. No use, distribution or reproduction is permitted which does not comply with these terms.
*Correspondence: Vivian C.H. Wu, dml2aWFuLnd1QHVzZGEuZ292