- 1Ecosystem processes , Institute of Marine Research, Bergen, Norway
- 2Department of Arctic and Marine Biology, UiT The Arctic University of Norway, Tromsø, Norway
- 3Northwest Atlantic Fisheries Centre, Fisheries and Oceans Canada, St. John’s, NL, Canada
- 4College of Fisheries and Ocean Sciences, University of Alaska, Fairbanks, AK, United States
- 5Barents Sea and Arctic Ocean Program Direction, Institute of Marine Research, Tromsø, Norway
- 6Centre for Fisheries Ecosystems Research, Fisheries and Marine Institute of Memorial University of Newfoundland and Labrador, St. John’s, NL, Canada
- 7Institute of Marine Science, University of Alaska, Fairbanks, AK, United States
- 8Oceanography and Climate, Institute of Marine Research, Bergen, Norway
- 9Ecosystem Processes, Institute of Marine Research, Tromsø, Norway
- 10Département de Biologie, Université Laval, Québec City, QC, Canada
- 11Takuvik International Research Laboratory, Université Laval, Québec City, QC, Canada
- 12Royal Netherlands Institute for Sea Research, Department of Estuarine and Delta Systems, Yerseke, Netherlands
- 13Greenland Climate Research Centre, Greenland Institute of Natural Resources, Nuuk, Greenland
- 14Faculty of Biosciences, Fisheries and Economics, UiT The Arctic University of Norway, Tromsø, Norway
Climate change is rapidly modifying biodiversity across the Arctic, driving a shift from Arctic to more boreal ecosystem characteristics. This phenomenon, known as borealization, is mainly described for certain functional groups along sub-Arctic inflow shelves (Barents and Chukchi Seas). In this review, we evaluate the spatial extent of such alterations across the Arctic, as well as their effects on ecosystem-level processes and risks. Along the inflow shelves, borealization is driven by long-term strengthened inflow of increasingly warm waters from the south and punctuated by advection and low sea ice extreme events. A growing body of literature also points to an emerging borealization of the other Arctic shelf ecosystems, through a “spillover” effect, as local changes in environmental conditions enable movement or transport of new species from inflow shelves. These modifications are leading to changes across functional groups, although many uncertainties remain regarding under-sampled groups, such as microbes, and technical challenges of consistent, regular monitoring across regions. There is also clear consensus that borealization is affecting phenology, species composition, community traits, population structure and essential habitats, species interactions, and ecosystem resilience. Non-dynamic environmental factors, such as depth and photoperiod, are thought to limit the complete borealization of the system, and may lead to intermediate, “hybrid” ecosystems in the future. We expect current borders of Arctic and boreal ecosystems to progress further northward and ultimately reach an equilibrium state with seasonal borealization. Risks to the system are difficult to estimate, as adaptive capacities of species are poorly understood. However, ice-associated species are clearly most at risk, although some might find temporary refuge in areas with a slower rate of change. We discuss the likely character of future Arctic ecosystems and highlight the uncertainties. Those changes have implications for local communities and the potential to support Blue Growth in the Arctic. Addressing these issues is necessary to assess the full scale of Arctic climate impacts and support human mitigation and adaptation strategies.
1 Introduction
Global redistributions of marine species under climate change are leading to unprecedented changes in biodiversity worldwide (Pinsky et al., 2013; Poloczanska et al., 2016). These redistributions are largely driven by populations following their thermal preferences (Burrows et al., 2019), leading to long-term poleward or depth displacements of species with lower thermal affinities. At mid-latitudes, poleward shifts of fish species have led to the progressive declines of cold-affinity boreal species in favour of warm-affinity Lusitanian species (Dulvy et al., 2008; Hofstede et al., 2010; Engelhard et al., 2011). At higher latitudes, regions that border the Arctic and receive the inflows from lower latitude Atlantic- and Pacific-influenced waters are the ultimate gateways of the poleward displacements of boreal species to the Arctic. Beyond these frontiers is the Arctic, with its strong seasonal light variability, and harsh environmental and living conditions, to which resident communities are well-adapted.
Climate change and Arctic amplification are responsible for warming rates in this region nearly 4 times faster than the rest of the world (Rantanen et al., 2022), and are causing structural changes across the Arctic ecosystems. Global projections suggest increasing biomass of commercial species coming from lower latitudes and thriving in increasingly productive, warmer, and ice-free ecosystems (Lam et al., 2016; Lotze et al., 2019; Tai et al., 2019). However, these projections vary strongly both in magnitude and direction among models (Bryndum-Buchholz et al., 2019). In addition, the strong seasonality in solar radiation will remain in the north due to the Earth inclination on its axis that could limit boreal species’ northward progress and survival (Kaartvedt, 2008). A large body of literature reports evidence of such northward shifts into the Arctic by boreal species of many functional groups, driven by local habitat modifications and advection of warm water and associated species from lower latitudes (Polyakov et al., 2020a; Brandt et al., 2023), a phenomenon called “borealization” (Fossheim et al., 2015). Arctic shelves and basins vary strongly with regard to hydrological, geochemical and biological functioning (Carmack and Wassmann, 2006). However, they are also strongly connected through large scale atmospheric, sympagic and oceanic circulation patterns (Carmack and Wassmann, 2006; Wassmann et al., 2020). Understanding the possible temporal and geographical extent of this borealization initially observed at inflow shelves, the risk posed to Arctic species and ecosystem functioning, and the consequences for the dependent local human inhabitants, are essential to facilitate planning for an adaptive, equitable management of Arctic and sub-Arctic ecosystems, and for the conservation of biodiversity.
Borealization of Arctic and sub-Arctic regions can be defined as a process by which ecosystems that were historically characterised as Arctic are progressively or transitively acquiring features typical of more southern, boreal ecosystems (Polyakov et al., 2020a). Sea surface temperatures in Arctic marine ecosystems are typically near or at the freezing point year-round (in the central Arctic Ocean) or seasonally (mostly on the shelves). In the central Arctic Ocean, the stratification is strong throughout the year with a cold, fresh Arctic water mass laying on top of the warmer and saltier Atlantic water mass. The overlaying fresher Arctic water mass contributes to high local sea-ice production.
Arctic and sub-Arctic ecosystems display very distinct communities and functions tuned to local conditions. In first-year ice areas, at the edge of the central Arctic Ocean and in seasonally ice-covered shelf regions, remineralized and recycled nutrients are used by ice-algae that can lead to a strong under-ice bloom (Arrigo et al., 2012; Assmy et al., 2017), followed by a stronger pelagic bloom dominated by diatoms when the ice is melting (Ardyna and Arrigo, 2020). There is greater diatom diversity in the sea-ice compared to the water column, where diversity differs by region with intermediate levels in the Canadian Arctic Archipelago and Pacific Arctic, and lower diversity in the Russian inner shelf seas, Hudson Bay and the Central Arctic Basin (CAFF, 2017). Microbial diversity (about ∼2000 taxa for pelagic and sympagic eukaryotes, Poulin et al., 2011) includes endemic microbial populations that have evolved to thrive under the extreme ice-influenced local conditions (Lovejoy et al., 2006; Lovejoy et al., 2007; Verde et al., 2016; van Leeuwe et al., 2018; Dorrell et al., 2023). Phytoplankton especially are able to survive prolonged dark periods that limit photosynthesis (Kvernvik et al., 2018; Lacour et al., 2019; van de Poll et al., 2020), using flexible strategies such as mixotrophy or overwintering as metabolically quiescent cells or as cysts (Stoecker and Lavrentyev, 2018). Some Arctic bacteria also use normally recalcitrant sources of organic carbon, including terrestrial organic matter from the large rivers that continue to flow into the Arctic even during winter (Colatriano et al., 2018).
Zooplankton are the primary link between the primary producers and higher trophic levels in Arctic and sub-Arctic marine food-webs. Some 300–400 zooplankton species occur in the Arctic water column (Kosobokova et al., 2011). Hyperiid amphipods and large, lipid-rich mesozooplankton, such as Calanus glacialis and Calanus hyperboreus play a crucial trophic role in the Arctic ecosystem (Søreide et al., 2010; Kohlbach et al., 2016; Brown et al., 2017). Gelatinous zooplankton, including hydrozoans (e.g., Aglanta digitale), are also ubiquitous in the Arctic pelagic niche (Kosobokova et al., 2011), and in the central Arctic basins, the gelatinous zooplankton appears to be more abundant than pelagic fishes (Ingvaldsen et al., 2023). The pan-Arctic hosts over 5,000 metazoan species, including 229 fish species (Bluhm et al., 2011b; Mecklenburg et al., 2011). Benthic communities sometimes rely strongly on the sympagic-benthic coupling driven by sinking sea ice algae to the bottom after they bloom and at ice breakup (Koch et al., 2020; Cautain et al., 2022). Arctic demersal and benthic fish communities are mainly composed of species such as sculpins (Cottidae), snailfish (Liparidae), eelpouts (Zoarcidae) and eelblennies (Lumpenidae), but the benthos is typically dominated by invertebrates including many benthic polychaetes and other worms, molluscs, arthropods and echinoderms. In contrast, the pelagic compartment is less diverse. Polar cod (Boreogadus saida) sometimes represents more than 95% of the fish species abundance (Geoffroy et al., 2023), while ice cod (Arctogadus glacialis), saffron cod (Eleginus gracilis), capelin (Mallotus villosus) and Arctic sand lance (Ammodytes hexapterus) are less abundant. Mesopelagic species are scarce in the deep basins. Glacier lanternfish (Benthosema glaciale), armhook squid (Gonatus fabricii), and even single individuals of Atlantic cod (Gadus morhua) are also present in the pelagic, but in low densities (Snoeijs-Leijonmalm et al., 2022; Ingvaldsen et al., 2023).
Typically, offshore boreal ecosystems exhibit contrasting characteristics, with warmer, saltier, and less stratified waters, higher phytoplanktonic primary production, smaller mesozooplankton species and abundant krill and pelagic amphipods in the North Atlantic compared to Arctic ecosystems (e.g., Dalpadado et al., 2003; Dalpadado et al., 2014; Weydmann et al., 2014). Similar gradients exist in the Pacific-Arctic but zooplankton communities on the extensive shallow inflow shelves are dominated by neritic species (Questel et al., 2013; Hunt et al., 2014; Ershova et al., 2015). The boreal pelagic niche is more diverse than the Arctic one, including a more prominent mesopelagic compartment in deeper water hosting, among the fishes, abundant myctophidae and stomiidae (Sameoto, 1989; Byrkjedal et al., 2004). Boreal and Arctic communities of demersal fish and benthic invertebrates differ in terms of traits such as temperature tolerance windows (Aune et al., 2018; Jørgensen et al., 2022; Logerwell et al., 2022), longevity (Ravelo et al., 2017), and age-at-maturity. Boreal fish include many mobile generalists of large size, such as Atlantic cod (Gadus morhua), Pacific cod (G. macrocephalus) and walleye pollock (G. chalcogrammus). This higher generalism implies a higher trophic redundancy, but also connectivity (i.e., feeding link richness) in the trophic network (Livingston and Tjelmeland, 2000; Kortsch et al., 2019). One of the key questions posed by the occurring process of borealization is whether this process can ultimately lead to a complete transformation of Arctic shelf ecosystems into boreal ones (replacement scenario), or to a hybrid version of both ecosystems (hybridization scenario, “cocktail”), and what the consequences for overall ecosystem functioning may be.
We review the existing knowledge on the mechanisms driving borealization and compile the evidence of borealization across ecosystem compartments in the marine Arctic. Our objectives are 1) to explore the spatial and temporal extent of borealization across the Arctic shelves, 2) to explore its extent across functional groups and consequences for ecosystem functioning, 3) to discuss the degree of transformation of Arctic ecosystems into boreal ones, now and in the future, 4) to discuss consequences for societies and management.
2 The environmental context of borealization across the pan-Arctic
The Arctic climate is driven by large scale processes within, and interactions between, the atmosphere, the cryosphere and the ocean (Timmermans and Marshall, 2020). Powerful local feedback processes associated with the air-sea-ice system amplify warming and sea-ice loss (Serreze and Barry, 2011; Ivanov et al., 2016; Polyakov et al., 2020b; Previdi et al., 2021). The recent decades have shown accelerated atmospheric and oceanic warming caused by a combination of global, regional and local drivers (Polyakov et al., 2020a; Shu et al., 2021; Isaksen et al., 2022; Smedsrud et al., 2022). Numerous studies have reported the many transformations of the entire Arctic system, including the intensification of hydrological cycles (increased precipitation and river discharge), glacier and sea ice melting and thinning, and modal shifts in atmosphere altering ocean circulation patterns (Greene et al., 2008; Wood et al., 2015; Box et al., 2019; Huntington et al., 2020; Polyakov et al., 2023). Extreme seasonal ice or temperature conditions have become more frequent (Hinzman et al., 2005; Dörr et al., 2021, Pécuchet et al., 2024). The current warming appears to have peaked between 2012 and 2018 for most of the Arctic (Danielson et al., 2020; Ingvaldsen et al., 2021), accompanied by record low sea ice cover (Stabeno and Bell, 2019). Nevertheless, the years post 2018 are a temporary reprieve, and the Arctic climate is projected to continue to warm as a result of increasing levels of atmospheric carbon dioxide (Pörtner et al., 2019).
Regional differences in climate change footprint across the Arctic can mostly be explained by the different hydrologic and geographic contexts of the sub-Arctic areas (Figure 1). These can be separated into four categories: the inflow shelves (Bering and Chukchi seas on the Pacific side, Barents Sea on the Atlantic side, west Greenland shelf), the outflow shelves (Canadian Arctic Archipelago, Western Baffin Bay, Eastern Greenland Shelf), the interior shelves (Kara, Laptev and Siberian Seas) and the Arctic basins (Carmack and Wassmann, 2006; Wassmann et al., 2020). Sea ice loss in the Barents and Bering inflow shelves are strongest during winter (Figure 2). This is a direct effect of warmer or stronger advection of sub-Arctic waters into the Arctic (Onarheim et al., 2018; Woodgate, 2018), and causes an increase in the extent of thermal habitat associated with boreal ecosystems throughout the year. Year-long suitable conditions favours the gradual borealization of the inflow shelf ecosystems, which can occur due to advection from the sub-Arctic seas and along the flow into the basins (Polyakov et al., 2020a). The Chukchi Sea and interior shelves, as well as the Canadian Arctic Archipelago, on the other hand, show strong sea ice loss during summer (Figure 2), but are strongly connected to neighbouring shelves through currents (Figure 1). Progressing borealization on to the interior shelves could occur through planktonic species advected through lateral basin-shelf or inter-shelf exchanges when sea ice loss and warming is sufficient (Nelson et al., 2014), and through mobile boreal shelf species reaching their northernmost depth habitat at the inflow shelves, thereby expanding their distribution to nearby shelves.
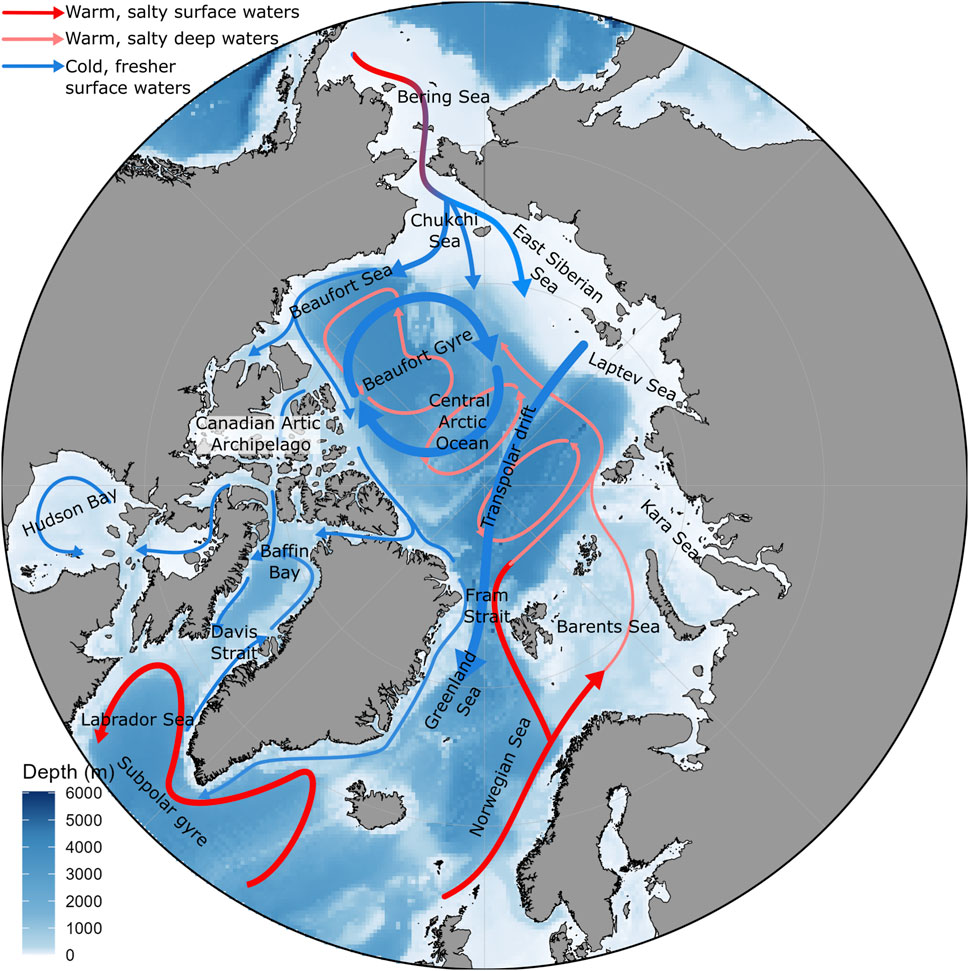
Figure 1. Main arctic and sub-arctic oceanic currents. Adapted from Anderson and Macdonald (2015), Armitage et al. (2017), and Constantin and Johnson (2023).
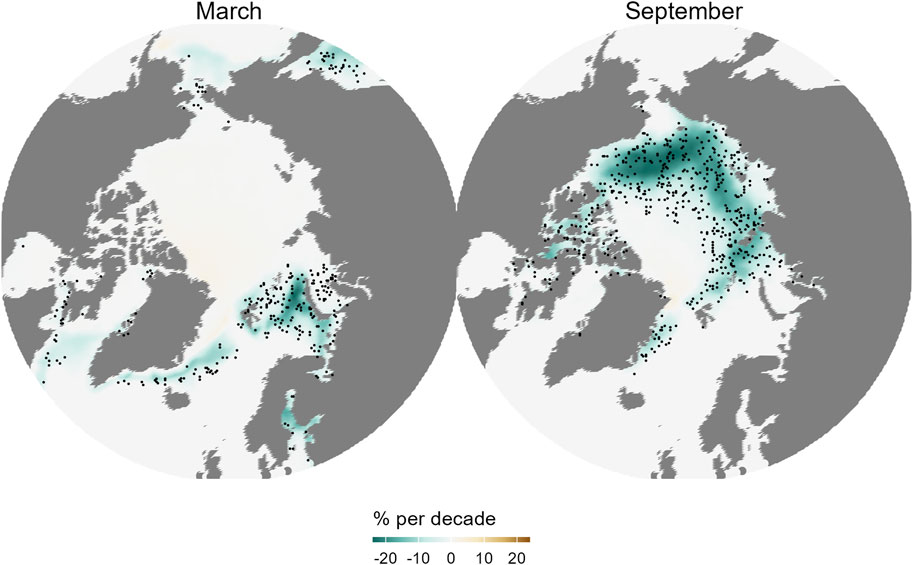
Figure 2. From copernicus climate indicators for sea-ice (https://climate.copernicus.eu/climate-indicators/sea-ice). Linear trends in sea ice concentration during 1979–2023 (% per decade) for March and September. Dots indicate areas where the trends are statistically significant. Credit: ECMWF, copernicus climate change service (C3S) C3S/ECMWF/EUMETSAT/MET Norway.
We used Sea Surface Temperature (SST) data to support our general comparison of the upper ocean warming experienced by Arctic shelves in the past century. We note that as these data are based on surface waters, they are interlinked with the loss of sea ice and may not be representative of changes at depth. The NOAA Extended Reconstructed SST V5 (ERSSTv5; Huang et al., 2017) is a global monthly SST analysis from 1854 to the present derived from the International Comprehensive Ocean-Atmosphere Data Set (ICOADS) data with missing data filled in by statistical methods. The data are available on a 2° × 2° (latitude-longitude) grid globally, but here were restricted to latitudes north of 50°N (see coverage in Figure 3). Several analyses were performed with this dataset. To illustrate the regional differences in warming rates, linear trends were calculated on the annual summer (July-September) temperatures for each pixel of the dataset for the period 1950–2023 (Figure 3) and averaged by regions (Table 1). Regions were defined following the Arctic Ocean and Adjacent Seas (AOAS) from the Conservation of Arctic Flora and Fauna (CAFF). Monthly SSTs averaged over the same regions for each year also highlight the warming trends and the increasing length of the open water season over time (Figure 4). Finally, decadal anomalies of summer SSTs (in °C) were calculated. For this analysis, the annual time series of summer mean SST for each region was built by averaging monthly values between July and September for a given year. Anomalies were calculated for each year by subtracting the long-term summer average over 1900–2000, before computing averages of the annual anomalies for each 10-year periods (1955–1964, 1965–1974, until 2015–2024). Decadal anomalies are illustrated per region (Figure 5), and values are provided for the last decade (Table 1).
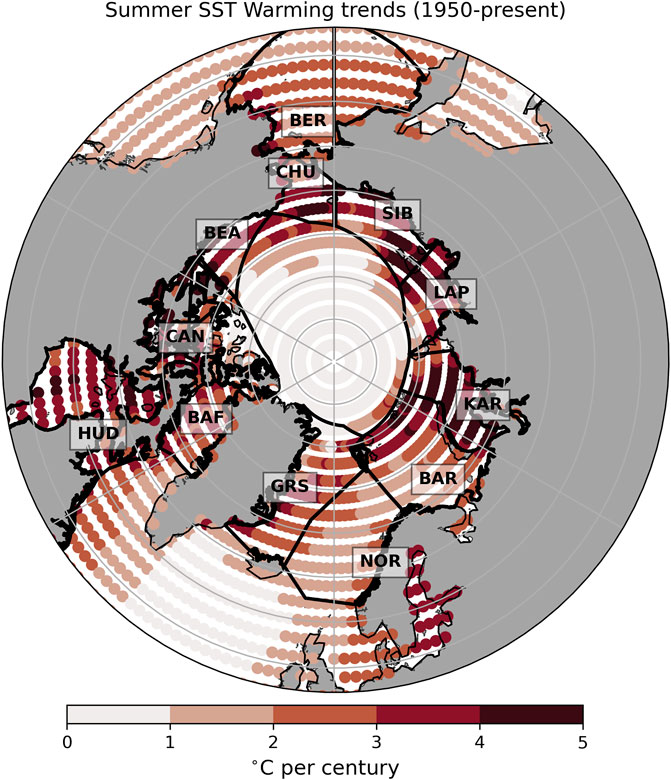
Figure 3. Map of summer (July-September average) SST warming trends (in °C per century) between 1950 and 2024 for each pixel (2° × 2° latitude-longitude) derived from ERSSTv5. The Arctic Ocean and adjacent Arctic seas (AOAS) regions, drawn here in black, were used for further regional analyses. These regions are (counterclockwise from top): Bering Sea (BER), Chukchi Sea (CHU), Beaufort Sea (BEA), Canadian Arctic Archipelago (CAN), Hudson Bay Complex (HUD), Baffin Bay (BAF), Greenland Sea (GRS), Norwegian Sea (NOR), Barents Sea (BAR), Kara Sea (KAR), Laptev Sea (LAP) and Siberian Sea (SIB).
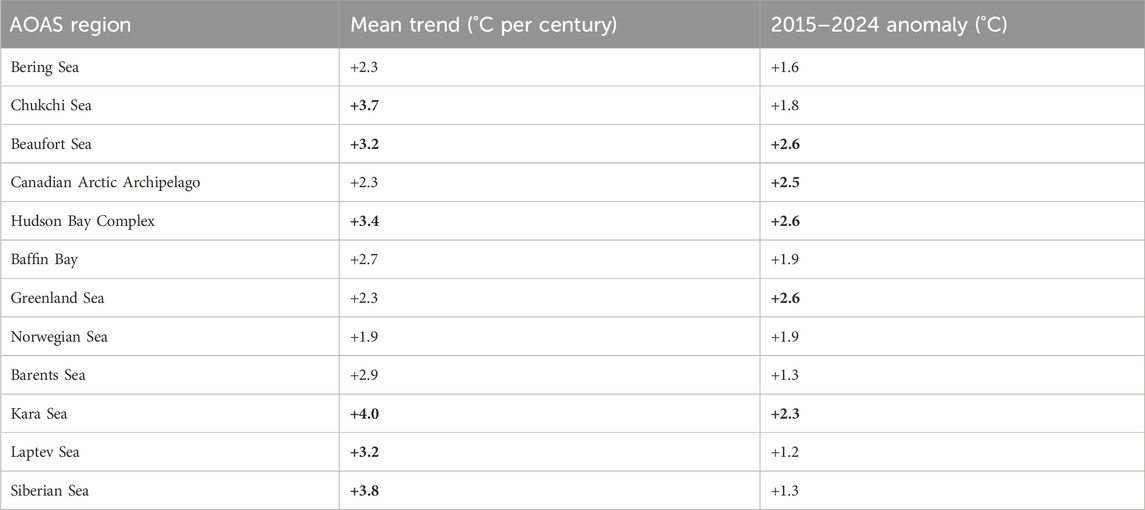
Table 1. SST mean trends (in °C per century) averaged over 1950–2023 for each of the Arctic Ocean and Adjacent Seas (AOAS) regions defined in Figure 3. The 6 regions with trends larger than 3°C per century are in bold. For SST anomalies during the last decade (2015–2024) compared to the 1900–2000 period, the 5 regions with anomalies larger than +2°C are in bold.
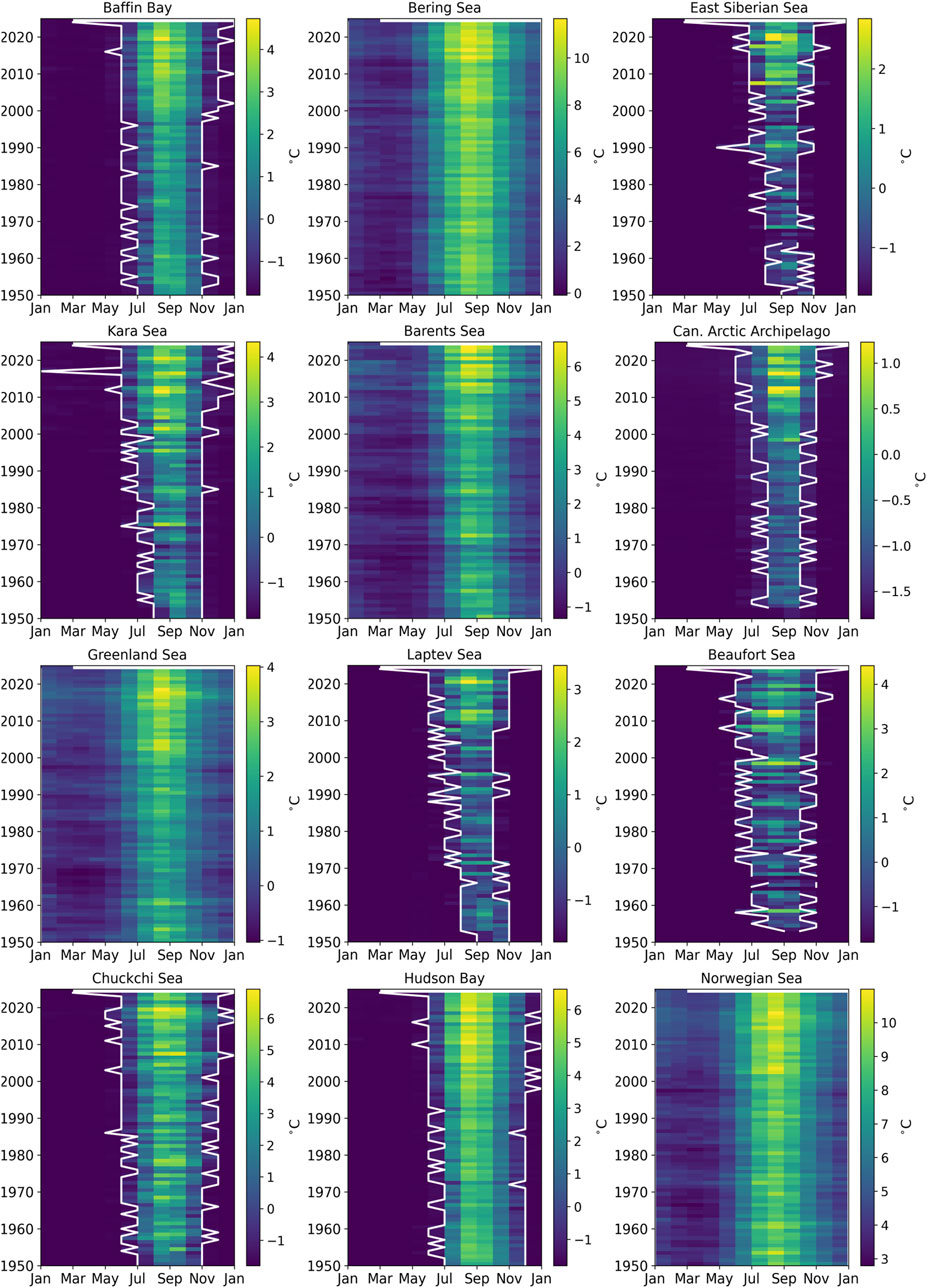
Figure 4. SST annual cycles between 1950 and present for each of the regions of the arctic identified in Figure 3. For each year (vertical axis), the averaged SST is plotted for each month on the horizontal axis. Months with a 100% ice cover are at a fixed value of −1.8°C (dark blue). This allows us to plot the opening and closure of the open water period defined here as the first and last month with average temperatures above −1.8°C (white lines). Note that the colour bar scale is different for each subplot.
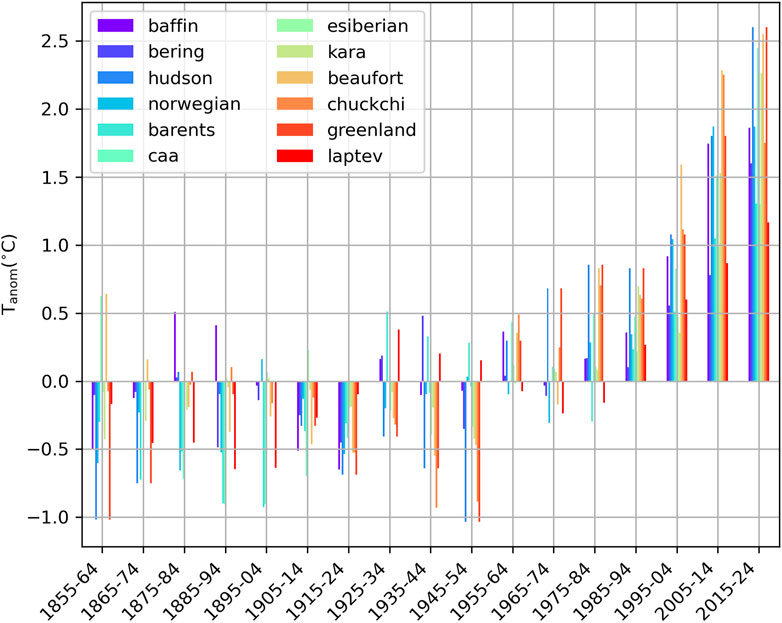
Figure 5. Decadal summer (July-September) SST anomalies referenced to the period 1900–2000 for each of the regions in Figure 3.
Summer surface waters of the Arctic Ocean have been warming unevenly over the last seven decades (Figure 3). The warming trends are in relatively close correspondence with the sea ice loss trends (Figure 2). When averaged by region, greatest warming trends are found in the northernmost regions of the Russian (Kara, Laptev and Siberian seas), Alaskan (Chukchi Sea) and Canadian Arctic coasts (Beaufort Sea and the Canadian Arctic Archipelago, Table 1). Those regions are mainly outflow shelves and interior shelves. Outflow shelves such as the East Greenland and the Canadian Arctic Archipelago also show signs of significant decrease in multi-year ice and relocation of different ice types (Sou and Flato, 2009; Michel et al., 2015). In contrast, interior shelves experience the effects of changing Arctic coasts, such as massive erosion in non-lithified coasts, and of run-off of freshwater and organic and inorganic materials contained in it (e.g., Williams and Carmack, 2015; Irrgang et al., 2022). The central Arctic basin north of Greenland and the Canadian Arctic Archipelago did not show large trends in SST due the quasi-permanent sea ice cover.
These warming trends in surface waters are, in some regions, associated with both warming and a longer ice-free summer season (deeper tones of yellow and widening of the white lines towards the top of the panels, Figure 4). The regions with the more moderate surface warming trends are generally those not exhibiting full sea ice cover for any month of the year (regions without white lines in Figure 4). Longer-term trends in summer SST anomalies (Figure 5) confirm earlier studies revealing that the warming of the Arctic started in most of the region in the second half of the 20th century, with an overall acceleration in the last three decades (Bengtsson et al., 2004; Matishov et al., 2012; Shu et al., 2021; Isaksen et al., 2022). The Canadian Arctic (Beaufort Sea, Canadian Arctic Archipelago and the Hudson Bay Complex) as well as the Greenland and Kara seas exhibited the largest SST increases in the last decade (2015–2024) compared to the long-term average (Table 1).
Compared to other regions, inflow shelves experienced slower warming trends in the summer (Figure 3; Table 1). However, this observation changes when studying other seasons. The Chukchi Sea, in autumn, and the Barents Sea, in winter, displayed the largest warming rates of air temperature in the Arctic since the 1980’s (Koenigk et al., 2020). Arctic waters cool to the freezing point prior to ice formation in autumn; warmer waters thus transfer more heat from the ocean to the atmosphere during the fall process of oceanic heat loss (Danielson et al., 2020). The Chukchi and Barents Seas are under the dual influence of the Arctic and the lower latitude systems that drive very strong inter- and intra-annual variability, with seasonal sea-ice cover, changes in temperature and important contrasts in seasonal light regime. These seas are the main gateways to borealization (Polyakov et al., 2020a). In the hydrosphere, their systems have evolved toward something less Arctic, and more similar to lower latitudes in the northern Pacific and the Atlantic, two phenomena referred as “Pacification” and “Atlantification”, respectively (Polyakov et al., 2017; Danielson et al., 2020; Ingvaldsen et al., 2021). Both are driven by anomalous advection of warmer Pacific and Atlantic water (Woodgate et al., 2010; Woodgate, 2018; Ingvaldsen et al., 2021; Smedsrud et al., 2022), leading to higher ocean heat transport into the shelf ecosystems and the Arctic (Xu et al., 2024). Consequently, average temperatures in both systems are increasing, at a rate of +0.04°C/year since 1980 in the Bering Strait and on the Chukchi Sea shelf (Danielson et al., 2020), and +0.05°C/year since 2000 in the Barents Sea (Ingvaldsen et al., 2021). The inflow shelf changes are also characterised by the loss of sea ice extent, increased open water season duration and faster transitions (Frey et al., 2015; Danielson et al., 2017; Onarheim et al., 2018; Dalpadado et al., 2020).
3 Evidence of borealization across the Arctic and impact on the functioning of ecosystems
3.1 Changes in the environment affect Arctic biogeochemistry and microbial communities
Climate driven changes in atmospheric, ice and oceanic conditions are likely resulting in shifts in the biogeochemical processes and microbial communities at pan-Arctic scales. However, a comprehensive understanding of regional trends and potential disparities among regions is hampered by the scarcity of long-term datasets of observed or modelled conditions (CAFF, 2017; Juranek, 2022). A set of studies describing the different hydrographic functioning and ecosystem production have revealed a wide variety of properties and pathways in the carbon cycle across the Arctic (Dunton et al., 2006; Grebmeier et al., 2006; Hirche et al., 2006; Hop et al., 2006; Michel et al., 2006; Rysgaard and Nielsen, 2006; Schmid et al., 2006; Tremblay et al., 2006; Wassmann et al., 2006a). These carbon cycles vary in efficiency depending on the processes transforming organic matter into nutrients (carbon mineralisation), and nutrients into organic matter (primary productivity). Productivity on the Arctic shelves is generally considered to be limited by the concentrations of available nitrogen compounds, especially in the Pacific Arctic (Mills et al., 2018; Ko et al., 2020), rather than by irradiance limitation from sea-ice and photoperiod (Tremblay and Gagnon, 2009; Tremblay et al., 2015). Most of the nutrient supply is advected through inflow shelves (Le Fouest et al., 2013b; Torres-Valdés et al., 2013; Tremblay et al., 2015). River inputs into sub-Arctic inner shelves may also be an important local source of nutrients and organic matter from land (Dittmar and Kattner, 2003; Dunton et al., 2006; Carmack et al., 2016), and estimates suggest terrestrial sources with coastal erosion, contribute to 28%–51% of net primary productivity in the Arctic Ocean via the Transpolar Drift (Le Fouest et al., 2013a; Terhaar et al., 2021), but see contrasting results in Le Fouest et al. (2013a).
At the pan-Arctic scale, rising temperatures and prolonged open water seasons have driven substantial increases (>50% over two decades) in sub-Arctic primary productivity, with earlier and extended blooms of pelagic phytoplankton (Arrigo et al., 2008; Kahru et al., 2011; Kahru et al., 2016; Hill et al., 2013; Hill et al., 2018; Ardyna et al., 2014; Arrigo and van Dijken, 2015). In addition, the decrease in multi-year ice and increase in first-year ice extent are driving a temporary increase in ice-algae habitat (Lim et al., 2022). Production increased in most regions but with varying magnitude (Arrigo and van Dijken, 2015). The Barents Sea was the area that experienced the highest increase in primary production over the last two decades (Dalpadado et al., 2020; Lewis et al., 2020). Primary production has also increased in spatial extent in the Bering Sea during warm years (Nielsen et al., 2024). The Canadian Arctic Archipelago, Baffin Bay and the eastern Greenland shelf display an altered functioning, with changes in productivity spatial-temporal patterns (Michel et al., 2015; Blais et al., 2017; Mayot et al., 2020). Data are scarce for other shelves, but short term studies also revealed interannual variation in production and particulate organic carbon export between years with varying sea ice cover (Lalande et al., 2009; Bienhold et al., 2022).
In recent years, ice dynamics and water temperature no longer suffice to explain increases in primary production on inflow shelves (Lewis et al., 2020). It has been hypothesised that the increased transport of deep Atlantic waters into the Arctic might have increased dissolved inorganic nitrogen (DIN) supply, favouring higher primary production in downstream regions (Henley et al., 2020; Lewis et al., 2020). However, despite increasingly northward Atlantic water flow and subsequent increased vertical mixing, multiple studies point to a long-term decrease in nutrients across most of the pan-Arctic, with the exception of the pan-Arctic slope (Oziel et al., 2022). Oxygen and nutrients seem to have decreased within the halocline of the Eastern and Western Eurasian Basin (Polyakov et al., 2020a). Similarly, there has been a decline in nitrate concentrations in the Atlantic water inflow into the Barents Sea between 1990–2010 (Oziel et al., 2017), and no long-term (35 years) trend in nutrients from Atlantic water flowing into the Arctic north of Svalbard (Duarte et al., 2021). In the Chukchi and Beaufort seas, only oxygen concentration has increased (Polyakov et al., 2020a), while nitrate and phosphate concentrations have decreased by 79% and 29% respectively (Zhuang et al., 2021). This was hypothesised to result from the joint effect of decreased vertical mixing following the strengthened stratification due to the loss of multi-year sea ice, and the increased consumption by primary producers (Zhuang et al., 2021). At interior and outflow shelves, nitrogen discharge from river outputs is usually quickly utilised, for example, through efficient denitrification in the Laptev Sea (Sanders et al., 2022; Tuerena et al., 2022), and nitrate and other dissolved inorganic nutrient fluxes from land seem to have declined (Tank et al., 2023). Lack of observations currently greatly limits our understanding of nutrient transport and budgets (Torres-Valdés et al., 2016).
Changes in primary production can also be linked to changes in phytoplankton communities. Phytoplankton communities affect carbon flux attenuation and production regimes (Ardyna et al., 2011; Wiedmann et al., 2020), and vary with environmental conditions across all Arctic shelves and basins (Ardyna et al., 2011; Min Joo et al., 2012; Lee et al., 2019; Ibarbalz et al., 2023). For example, small algae, more efficient at assimilating nutrients and thus potentially more competitive compared to local larger species, have been observed in higher quantities in the Canada Basin and coastal Canadian Arctic Ocean (Li et al., 2009; Blais et al., 2017), and in the Atlantic water masses west of Svalbard (Lalande et al., 2013). In the Beaufort Sea, microbial communities have transitioned towards taxa characteristic of oligotrophic environments (Comeau et al., 2011). In addition, strong advection at inflow shelves has driven the northward shift of temperate species of coccolithophores (Winter et al., 2014; Neukermans et al., 2018; Oziel et al., 2020). In contrast, there is no evidence of temporal changes in microbial community composition or biomass in the North Water (northern Baffin Bay) or the Laptev Sea (Freyria et al., 2021; Bienhold et al., 2022). In the rest of the Arctic, time series are scarcer, but microbial communities, including phytoplankton, have been characterised by recent studies, setting baselines for the investigation of climate impacts (Demidov et al., 2021; Polyakova et al., 2021; Sukhanova et al., 2021; Liu et al., 2023; Bezzubova et al., 2024). Shifts in microbial communities are likely to drive changes in trophic functioning, affecting the diets, phenology and development of grazing species (Søreide et al., 2010; Daase et al., 2011). Changes in planktonic communities and new environmental conditions can cause harmful algal blooms (HAB), which have direct impacts on the food-webs and human consumption (Okolodkov, 2005; Bruhn et al., 2021). Cases of HAB have been noticeably increasing northward in recent years, with many reports from the Pacific Arctic in particular (Anderson et al., 2021; Anderson et al., 2022; Einarsson et al., 2022; Fachon et al., 2024), with important impacts on top predators, including humans (Hendrix et al., 2021; Lefebvre et al., 2022; Van Hemert et al., 2022).
3.2 Altered habitats and feeding conditions affect pelagic systems
Changes in the environment and in primary productivity (species composition, spatial and temporal patterns) are propagating through the food web, driving the borealization of the pelagic compartments. Indeed, environmental changes trigger contrasting responses of the boreal and Arctic pelagic communities, due to their strong differences in biogeography, life history strategies and functions. For example, many zooplankton communities dominate successively across seasons and regions, varying in their ecological niches and functional traits (Questel et al., 2013; Balazy et al., 2018; Kimmel et al., 2018; Kimmel et al., 2023; Mańko et al., 2020; Mańko et al., 2022; Hop et al., 2021; Darnis et al., 2022; Mazanowski et al., 2023; Van Engeland et al., 2023; Wold et al., 2023). Large, lipid-rich Arctic copepod species such as C. glacialis and C. hyperboreus can utilise allochthonous production, and survive low productivity periods owing to their large lipid reserves (Hunt et al., 2014; Hirche et al., 2024). Arctic species thus tend to dominate the biomass in late summer/autumn during years with low advection and late sea ice retreat (Kimmel et al., 2018; Spear et al., 2020). However, Arctic zooplankton have a slower increase in respiration rate with rising temperatures compared to their Atlantic counterparts, which implies that, although they might be resilient to warming, Atlantic zooplankton may become more competitive under such conditions (Kaiser et al., 2022). In contrast, boreal species typically consist of smaller zooplankton with less reserves. Along the continental slope of the Atlantic Water inflow, high concentrations of C. finmarchicus prevail (Bluhm et al., 2020). Differing traits of Arctic and boreal zooplankton might be adaptative of their respective environment (Renaud et al., 2018), and a shift from lipid-rich Arctic to more boreal communities might not have strong impacts on plankton-feeders as boreal zooplankton occur in larger numbers, over a longer period of time, and have a faster life cycle. However, reduced abundances of lipid-rich C. glacialis on the Bering Sea shelf have been associated with poor energetic condition and reduced survival of young walleye pollock (Heintz et al., 2013).
Warm water advection and sea-ice retreat are major determinant of zooplankton community dominance across the Arctic (Ershova et al., 2015; Kimmel et al., 2018; Kimmel et al., 2023; Spear et al., 2019; Abe et al., 2020; Dalpadado et al., 2020; Drits et al., 2023; Ramondenc et al., 2023). Krill, gelatinous zooplankton, ichthyoplankton and young-of-the-year pelagic fish also display different life history strategies and habitat preferences and respond strongly to varying advection regimes (Eriksen and Dalpadado, 2011; Eriksen et al., 2015; Eriksen et al., 2020; Oviatt et al., 2015; Benkort et al., 2019; Deary et al., 2021; Skjoldal et al., 2022). Adult pelagic fish are often less directly affected by variations in environmental conditions. Boreal mobile pelagic fish can relocate to follow the production and expand into newly suitable habitats, thus propagating the borealization of the lower trophic levels up the food chain, although other important factors such as recruitment and availability of suitable spawning habitat also have a strong impact on population spatial-temporal dynamics (Hollowed et al., 2013b; Eriksen et al., 2017). In contrast, some Arctic species such as polar cod are very dependent on ice to complete their life cycle, and loss of sea-ice thus drives a loss of essential habitats for those species (David et al., 2016; Huserbråten et al., 2019; Gjøsæter et al., 2020; Marsh and Mueter, 2020; Deary et al., 2021; Geoffroy et al., 2023).
Long-term borealization has thus been reported on for many pelagic compartments. Shifts from lipid-rich Arctic zooplankton to communities of smaller individuals are of concern in western Greenland (Møller and Nielsen, 2020), the Bering sea (Kimmel et al., 2018; Kimmel et al., 2023), the Chukchi Sea (Abe et al., 2020; Spear et al., 2020; Ershova et al., 2021; Mueter et al., 2021a), Labrador, eastern Greenland and the Barents Sea (Trudnowska et al., 2012; Weydmann et al., 2014; Eriksen, 2016; Eriksen et al., 2017; Freer et al., 2022), and the Fram Strait, main gateway of Atlantic inflow into the Arctic (Gluchowska et al., 2017). Subtle changes in species composition may also be underway within neritic species complexes (Ershova et al., 2015). Krill is also shifting in species composition from Arctic to temperate in the Barents Sea (Eriksen and Dalpadado, 2011; Orlova et al., 2015; Eriksen, 2016), Svalbard, and Bering Sea (Hunt et al., 2016). Lack of long time series hampers our ability to detect changes in zooplankton community composition on the other shelves. Similarly, boreal gelatinous species are thought to also extend northward on the inflow shelves (Brodeur et al., 2002; Brodeur et al., 2008; Eisner et al., 2014; Eriksen et al., 2017; Geoffroy et al., 2018). But drivers of their abundance may be complex (Decker et al., 2023) and historical data and from other shelves are limited, making temporal and regional comparisons difficult.
Further up in the food web, the geographical retreat of polar cod due to the loss of sea-ice is often concomitant with the northward expansion of more southern species, such as capelin, saffron cod or walleye pollock in the Atlantic or Pacific Arctic (Hop and Gjøsæter, 2013; Stige et al., 2019; Wildes et al., 2022; Levine et al., 2023; von Biela et al., 2023). Increased abundance of more southern fish species, such as capelin and Pacific sand lance, are also observed at interior and outflow shelves: in the Canadian Arctic Archipelago (Falardeau et al., 2017), in the Hudson Bay (Gaston et al., 2003), and northern Baffin Bay (Ulrich and Tallman, 2021). While mesopelagic fish have been suggested to be limited in their northward expansion because of the light conditions (Ljungström et al., 2021; Langbehn et al., 2022) or a combination of both light and temperature (Chawarski et al., 2022), studies based on observations rather than modelling have partially challenged the light hypothesis by reporting mesopelagic sound scattering layers partly formed of boreal fish species (e.g., glacier lanternfish Benthosema glaciale and juvenile redfish) in the high Arctic (Knutsen et al., 2017; Geoffroy et al., 2019; Snoeijs-Leijonmalm et al., 2022; Ingvaldsen et al., 2023). Moreover, studies looking at both temperature and latitude (a proxy for irradiance) have concluded that temperature fronts might also contribute to hindering the northward range expansion of mesopelagic fish (Chawarski et al., 2022). Data are more limited for the inner shelves of the Kara, Laptev and East Siberian seas, but those ecosystems are often relatively poor in pelagic fish (Hirche et al., 2006; Schmid et al., 2006).
Pelagic borealization is affecting populations and ecosystem functioning. Changes in zooplankton development and community succession along the seasons have been linked to pelagic fish diet and biomass trends (Orlova et al., 2010; Dalpadado et al., 2024), propagating climate effects to upper trophic levels. They also affect first year juvenile fish survival, mainly walleye pollock and polar cod, as lipid-rich species associated with autumn blooms during cold years are a critical source of reserve for the juvenile fish before the winter (Kimmel et al., 2018; Kimmel et al., 2023; Bouchard and Fortier, 2020; Dupont et al., 2020), and as prey size impacts that of its visual predators, as shown by bioenergetic and behavioural modelling studies (Ljungström et al., 2020). In contrast, the sustained advection of krill in the Barents, Chukchi and western Beaufort seas is altering trophic controls and stabilising predator populations (Berline et al., 2008; Eriksen and Dalpadado, 2011; Orlova et al., 2013; Ashjian et al., 2021). Increasing co-occurrence of polar cod with other species such as capelin or Pacific sand lance would lead to more competition for food at inflow and interior shelves, with negative consequences for both species (Orlova et al., 2010; Falardeau et al., 2014; McNicholl et al., 2016; McNicholl et al., 2018; Pedro et al., 2020). Changes in dominant forage fish and zooplankton prey have altered marine mammals’ and seabirds’ migration patterns and diet across the pan-Arctic, sometimes leading to lower growth rate for chicks or altered demography (Provencher et al., 2012; Chambellant et al., 2013; Gaston and Elliott, 2014; Falardeau et al., 2017; Gall et al., 2017; Balazy et al., 2018; Kuletz et al., 2020; de la Vega et al., 2021; Merkel et al., 2021; Ulrich and Tallman, 2021; Descamps et al., 2022; Moore et al., 2022).
3.3 Changes in benthic demersal systems
Many studies have reported changes in benthic (mostly invertebrates) and demersal (mostly fish) ecosystems across Arctic and sub-Arctic shelves. Signals of borealization are stronger on inflow shelves, where shifts in demersal fish communities have been widely reported, such as in the Barents Sea (Fossheim et al., 2015; Bergstad et al., 2018; Spotowitz et al., 2022), in western Greenland (Post et al., 2021), as well as the northern Bering Sea and, to a lesser extent, the Chukchi Sea (Mueter et al., 2021a; Zhang et al., 2022). An emerging pattern from recent studies is that borealization of inflow regions seems to impact neighbouring areas by spillover effect. In eastern Greenland (Emblemsvåg et al., 2022a; Emblemsvåg et al., 2022b), demersal communities across depths have rapidly reorganised in response to climate change, in particular between 350 and 1000m depth. Specifically, studies have found that Atlantic cod, beaked redfish and deep-sea shrimps (Pandalus borealis) observed on the northeast Greenland shelf originated from the Barents Sea populations (Christiansen et al., 2016; Andrews et al., 2019). In addition, across the Siberian Arctic (Kara Sea, Laptev Sea, East Siberian Sea), North Atlantic species have expanded eastward while North Pacific species have expanded westward (Orlov and Volvenko, 2024).
Borealization in inflow shelves has also been documented for benthic communities, although responses are lagged with regard to climatic signals (Grebmeier, 2012; Jørgensen et al., 2019; Waga et al., 2020; Zakharov et al., 2020; Calvet et al., 2024). Interestingly, the borealization of benthic communities does not necessarily lead to an increase in productivity or biomass (Zakharov et al., 2020 for the Barents Sea; but see Krause-Jensen et al., 2020, for pan-Arctic increases in seaweeds). In contrast, unchanged community biogeography could suggest resilient communities in the Laptev Sea (Kokarev et al., 2017), and to some degree in the Bering and Chukchi Seas, over several decades (Grebmeier et al., 2015a but see Grebmeier et al., 2006; Huntington et al., 2020, for biomass declines). The lack of repeated surveys hinders our ability to evaluate the degree of borealization of benthic communities, especially on interior and outflow shelves, but bycatch data from commercial or scientific bottom trawls can serve as a baseline for future assessments (Jørgensen et al., 2022), and the Distributed Biological Observatory in the Pacific Arctic provides time series for macro- and megabenthic fauna (Grebmeier et al., 2015b; Grebmeier et al., 2018).
At smaller scales, some studies have revealed the borealization of some coastal zones across the Arctic, as suggested by an increase in boreal fish species in lagoons on the Beaufort Sea (von Biela et al., 2023), and by increased macroalgal cover (Krause-Jensen et al., 2020), abundance of boreal species and coincident shifts in benthic invertebrate communities in Svalbard fjords and near Franz Josef Land (Kortsch et al., 2012; Węsławski et al., 2018; Al-Habahbeh et al., 2020; Dvoretsky and Dvoretsky, 2024). However, the degree of borealization of benthic communities in Atlantic Arctic fjords might depend on the bathymetric features of the coastline, such as fjord sills and the interannual strength of the Atlantic Water inflow (Beuchel et al., 2006; Renaud et al., 2007; Jordà-Molina et al., 2023). A more important driver of major changes in Arctic coastal ecosystems and landscapes could instead be the melting marine terminating glaciers (Lydersen et al., 2014; Meire et al., 2017; Williams et al., 2021; Kavan and Strzelecki, 2023). Some sub-Arctic coastal regions do not display any particular response to climate change. For example, western Greenland intertidal ecosystems do not vary with latitude, which would suggest resilience to climate change, based on space-for-time substitution (Thyrring et al., 2021), and in the Baydaratskaya Bay, Kara Sea, benthic communities were unchanged until 2013, and only seemingly impacted by other local anthropogenic impacts since then (Azovsky and Kokarev, 2019).
As in the pelagic systems, demersal and benthic borealization appears to be mainly driven by changes in suitable habitats and prey distribution induced by climate change. Rates of response to environmental changes are often lagged and species-specific (Alabia et al., 2018). At the inflow shelves, the main drivers are the fluctuating extent of colder and warmer water masses and timing of ice retreat. This has been shown in the northern Bering Sea and Chukchi Sea, for both demersal and benthic communities (Grebmeier, 2012; Nishio et al., 2020; Zhang et al., 2022), and in the Barents Sea (Ingvaldsen et al., 2021; Nascimento et al., 2023). In addition to expanding suitable warmer habitats, advected waters transport demersal and benthic early life stages. Variations in water mass extent across interannual to decadal scales have been linked to the varying abundance of advected ichthyoplankton species, leading to variation in dominance regime of fish larvae in the Eastern Chukchi Sea (Randall et al., 2019; Axler et al., 2023), and in the Barents Sea (Eriksen et al., 2015; Eriksen et al., 2017). These fluctuations in water mass inflows are linked to large-scale circulation patterns, such as the sub-polar gyre in the northern Atlantic in western Greenland (Post et al., 2021), or the Arctic dipole in other inflow shelves (Polyakov et al., 2023). While warm conditions are maintained at inflow shelves and neighbouring regions are gradually warming due to local changes in the environment, mobile populations such as fish are likely able to further expand beyond inflow shelves (Andrews et al., 2019; Orlov and Volvenko, 2024), but demersal Arctic fish species living on the edge of the shelves cannot move north as they run out of shelf (Wassmann et al., 2006b; Fossheim et al., 2015). The northward expansion of less mobile boreal benthos is likely more dependent on the dispersion of the larval phase (Ershova et al., 2019; Meyer-Kaiser et al., 2022), although those will may not settle successfully (Wassmann et al., 2015; Ershova et al., 2019; Descôteaux et al., 2022).
Shifts in species distribution can drive changes in both pelagic and benthic-demersal life cycles, oceanic seascapes, population structures and interspecific interactions. In the Chukchi Sea, increasing number of boreal pre-spawning, spawning and post-spawning walleye pollock have been observed in recent years (Emelin et al., 2022). This could indicate an expansion of their spawning location or range but does not necessarily correlate with juvenile survival. Survival of the early life stages depends on species capacity to take advantage of available food and to resist harsh winter conditions. Polar cod, adapted to Arctic conditions, have a unique fat storage strategy, from diatoms and Calanus origin, that enables it to thrive in cold ice-covered region (Copeman et al., 2022). In contrast, juvenile walleye pollock have better condition in the southern than in the northern Chukchi Sea (Copeman et al., 2022), which suggests that they are not yet adapted to colder northern conditions. In the Barents Sea, climate induced changes in temperature and food availability also drive trends in 0-group fish lengths (Skjoldal et al., 2022) and distribution (Eriksen et al., 2017). Apart from their lipid storage strategy, differences in spawning timing between species is likely a key driver of their successful survival during the Polar Night. Indeed, winter surveys around Svalbard show that the spring spawning strategy of boreal fish, which contrasts with winter-spawning Arctic and arcto-boreal species, might lead to a temporal mismatch between their planktonophagous larvae and zooplanktonic blooms (Berge et al., 2015; Geoffroy and Priou, 2020). Large incoming boreal fish are often generalists which are thus able to feed on boreal as well as Arctic prey. This was shown by the diet composition of walleye pollock in the northern Chukchi Sea, where Arctic copepods, euphausids and polar cod were observed in their stomach content (Benzik et al., 2022). Walleye pollock also consume other incoming species, such as Pacific sand lance, whose abundance has been increasing with the decline of sea-ice (Baker et al., 2022). Similarly, in the Barents Sea, ctenophore species, which might benefit from warmer conditions in the region, and snow crab (Chionoectes opilio), which overlaps increasingly with Northeast Arctic cod as these expand north-eastward, increased in frequency in Northeast Arctic cod diets (Eriksen et al., 2018; Holt et al., 2021). However, there is no sign that polar cod are increasing in Northeast Arctic cod’s diets (Holt et al., 2019).
3.4 Ecosystem-level impacts of borealization
3.4.1 Biological and functional diversity across compartments
Understanding the degree of borealization of Arctic ecosystems necessitates knowledge on biological diversity of native and incoming communities. Efforts to catalogue species across the pan-Arctic are still ongoing (Renaud et al., 2006; Bluhm et al., 2011b; Bluhm et al., 2011a; Kosobokova et al., 2011; Poulin et al., 2011; Lin et al., 2014; Jørgensen et al., 2022). The Central Arctic Ocean can be divided in two main regions with distinct biodiversity: the Eurasian and American regions (Alfaro-Lucas et al., 2023). There is relatively little difference in species composition between the central Arctic Ocean basins (Bluhm et al., 2015; Alfaro-Lucas et al., 2023), yet substantial variation among benthic habitats within the basins (Ramirez-Llodra et al., 2023). However, some ecological compartments and regions are still largely under studied (CAFF, 2017; Alfaro-Lucas et al., 2023). Where long time series are available, studies highlight the strong impact of borealization on biodiversity. Species distribution ensemble models applied to 69 apex- and mesopredators revealed varying trends in species richness across the pan-Arctic over the last two decades (Alabia et al., 2023). The authors suggested that species richness has been increasing overall in the Arctic, in line with large range shifts, especially from large mobile apex predators, but more strongly in interior and outflow shelves than inflow shelves. In the Barents Sea, an 8-year study showed a steady increase in species richness and biodiversity indices, especially within the advected waters (Prokopchuk and Trofimov, 2019). A study on shorter time scales in the Chukchi Sea revealed contrasting responses of functional group diversity between warm and cooler years: during a warm year, diversity increased among zooplankton and fish communities, decreased for bacteria, protists, epibenthos and seabirds, and did not vary significantly for macrobenthos, relative to a colder year (Mueter et al., 2021a).
The documented changes in Arctic biodiversity bring about functional reconfigurations with implications for ecosystem function and vulnerability (Ingvaldsen et al., 2021). Many boreal species differ from Arctic ones in terms of functional characteristics, as exemplified by fish and copepods (e.g., Aune et al., 2018). Boreal fish species are often large, and predatory, with a generalist diet, whereas Arctic fish are rather small benthivores like sculpins (Frainer et al., 2017; Emblemsvåg et al., 2022a; Emblemsvåg et al., 2022b). In East Greenland, the incoming boreal functional traits do not compensate for the loss of Arctic traits, which leads to a decrease in functional diversity across depths (Emblemsvåg et al., 2022a). In contrast, in the Barents Sea, functional diversity increases with the northward shift of functionally-rich boreal communities (Frainer et al., 2021), but this situation might be transitory as predation and competition between newly co-occurring boreal and Arctic species might lead to the decline of Arctic species (Pecuchet et al., 2020). The alteration in functional diversity is correlated with a change in dominance of different life history strategies, including an expansion of so-called “periodic” species, which have a greater adaptive capacity owing to higher reproductive output, broader diet, and migratory behaviour (Bernardo et al., 2024). Higher functional diversity is thought to increase adaptive capacity and affect ecosystem stability and resilience to perturbations. However, these studies have so far been limited to fish communities, and a pan-Arctic overview is lacking.
3.4.2 Impacts on apex predators
Changes in environmental conditions in the Arctic also affect seabirds and marine mammals, which are often emblematic and/or Red-listed and are thus also an important component of biodiversity, despite low species richness compared to smaller groups. For example, seabirds colonies are experiencing changes in densities and species composition around the Svalbard Archipelago (Descamps and Strøm, 2021), and in the northern Bering Sea and eastern Chukchi Sea (Kuletz et al., 2020). Similarly, changes in marine mammals are observed with changes in habitat conditions: killer whales are increasingly observed in the Chukchi Sea and elsewhere as sea-ice retreats (Ferguson et al., 2010; Stafford, 2019), in East Greenland an increase in the abundance of several boreal cetaceans (humpback, fin, killer, and pilot whales and dolphins) has been seen as summer sea ice has disappeared and water temperatures have increased (Heide-Jørgensen et al., 2023), while narwhals seem to distribute preferentially where temperatures are colder, and might abandon their warmer habitats of Mideast and Southeast Greenland (Chambault et al., 2020; Heide-Jørgensen et al., 2023). Sea surface temperatures are also linked to seasonal displacements of bowhead whales in Baffin Bay (Chambault et al., 2018). With sea ice decline, polar bears are pushed to more frequent long-distance swimming (Pilfold et al., 2017). Gray whales, associated with warmer waters, occur more frequently in the Chukchi Sea to feed on abundant prey when ice conditions allow. However, recent warm events have been associated with unusual mortality events in gray whales (Stewart et al., 2023), suggesting that increased availability of prey in newly ice-free areas was insufficient to compensate for decreasing prey availability in more southern feeding areas. Concomitant displacements have been observed for Arctic ice-associated species, with poorer body condition and population status (Kovacs et al., 2011; Laidre et al., 2015). Environmental changes and prey behaviour are also altering migration patterns and timing of marine mammal species (Kuletz et al., 2024).
Changes in distribution of lower trophic level communities affect the diet of many apex predators, modifying trophic interactions and food webs (de la Vega et al., 2021). Spatial or compositional shifts in epibenthic invertebrate communities in the Bering and Chukchi Sea could affect prey species of benthivorous marine mammals like Pacific walrus and bearded seals, which are important subsistence resources for Alaska Native communities (Richman and Lovvorn, 2003; Logerwell et al., 2022), as well as benthivorous seabirds like the endangered Steller’s eiders (Richman and Lovvorn, 2003). However, current latitudinal and regional variability in ringed seals and beluga diets would suggest that those species could adapt to changes in prey (Yurkowski et al., 2016a). For polar bears, some individuals are more opportunistic than others, and those that specialise on seals are most sensitive to climate change impacts (Thiemann et al., 2008). Apart from changes in diet and habitat loss, Arctic top predators will likely face an increasing number of anthropogenic pressures, such as increased noise and pollution from shipping, plastic pollution, increasing disease and parasite occurrence, (Kovacs and Lydersen, 2008; Merkel et al., 2021). Top predators have been shown to sometimes adapt to the change in diet (e.g., Yurkowski et al., 2016b; Vihtakari et al., 2018; Sauser et al., 2023), although not always successfully, as changes in prey quality, notably fat content, would affect species fitness (e.g., Descamps et al., 2022; Anderson et al., 2023; Sauser et al., 2023). The decline of iconic marine mammals in the Arctic is a major conservation concern, with implications for biodiversity and cultural ecosystem services in the Arctic.
3.4.3 Food-web impacts
Apex- and mesopredator incoming boreal species establish new feeding links in Arctic marine ecosystems, thereby reorganising food-webs, leading to increased food-web connectance and decreased modularity, which is associated with low resilience (Kortsch et al., 2015; Blanchet et al., 2019; Pecuchet et al., 2020; Jordán et al., 2024). A core function of Arctic food webs that is affected by borealization is the sympagic-pelagic-benthic carbon coupling through the sinking of ice-algae when ice melts. This function is particularly important for benthic biomass and biodiversity (Boetius et al., 2013; Kędra et al., 2015; Hansen et al., 2020). Indeed, several studies have shown the strong reliance of megabenthic communities to this coupling in the Barents Sea, northeast Greenland shelf (Cautain et al., 2022; Cautain et al., 2024), on Siberian shelves (Schmid et al., 2006; Lalande et al., 2009), in the Northern Bering Sea and Chukchi Sea (Koch et al., 2020), in Baffin Bay (Yunda-Guarin et al., 2020) and in the Canadian high-Arctic (Yurkowski et al., 2020). The anticipated - though poorly documented (see Zhulay et al., 2023 for the Chukchi Borderland) - decline in benthic-pelagic coupling has important implications for ecosystem functioning (Niemi et al., 2024). Food web and ecosystem models can explore the consequences of declining sea ice and new trophic interactions in an integrative approach. In the Beaufort Sea, the shelf and slope ecosystem with strong riverine influence and changes in salinity has likely driven lower diversity and changes in biomass, consumption and trophic levels of marine mammals and fish, including Arctic key species (Sora et al., 2024). In the Barents Sea, multiple pressures have caused changes in the food-web over time, but an ecosystem model suggests that increased productivity fuelled the food web through bottom-up effects after the 1990’s (Pedersen et al., 2021). In Baffin Bay, an ecosystem model revealed that polar cod is a key component of the food-web structures (Pedro et al., 2023). An exploration of past dynamics could reveal the impacts of changes in the polar cod stock on the ecosystem.
4 Discussion
4.1 Spatial extent of borealization: the “spillover” effect
Local changes in environmental conditions, driven by alterations in large scale circulation patterns and fluid (water, air) masses properties, are reported across the pan-Arctic. The strongest impacts seem to affect the inflow shelves, where high connectivity to very different systems result in a high interannual variability and sensitivity to external forcings. Borealization has thus progressed more rapidly on these shelves, affecting all trophic levels (Csapó et al., 2021). Ecosystems inflow shelves are currently transitioning towards a more boreal functioning but retain some Arctic characteristics. The potential degree of borealization in the future is discussed below. A new emerging signal, as revealed by many recent studies reviewed here, is that interior and outflow shelves are also experiencing borealization through climate-induced modifications of local environmental conditions (sea-ice cover, river runoff, temperature) that render habitat suitable for species that are transported or actively relocate there (Figure 6).
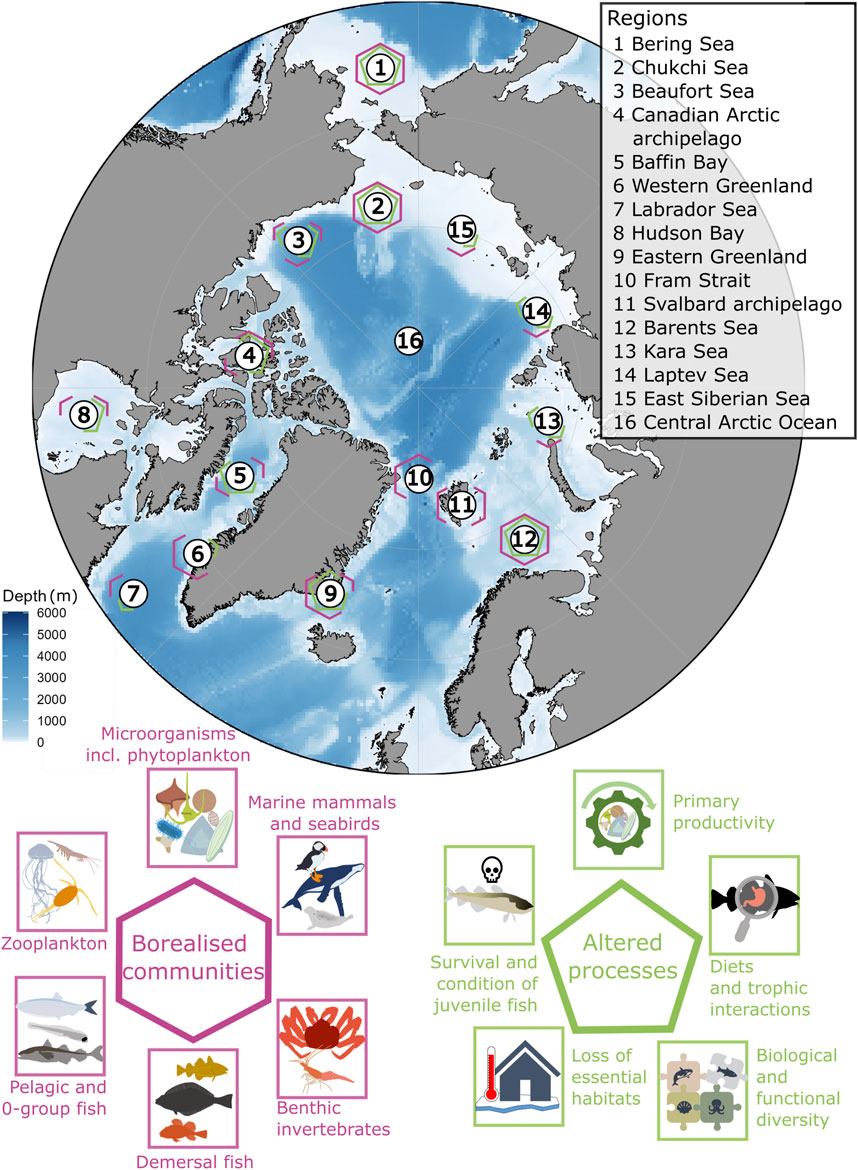
Figure 6. Map of evidence of borealization of communities and ecosystem processes across regions. Regions that are indicated are based on the naming used in the literature cited in the main text.
However, the extent of this “spillover” effect of borealization across space, time and functional groups is uncertain, due to several knowledge and data gaps. Indeed, a recent biodiversity assessment for the whole Arctic domain has highlighted the lack of data for the Siberian seas (Kara, Laptev, East Siberian seas) and the Canadian Arctic (Canadian Arctic Archipelago, Beaufort Sea, Hudson and Baffin Bay, Alfaro-Lucas et al., 2023). In addition, for all shelves and for the Arctic basins, data are largely restricted to the summer and autumn seasons, with recent but limited knowledge on winter and polar night processes (Berge et al., 2015; Berge et al., 2020; Gerland et al., 2019), and transition periods between ice-free and ice-covered seasons (Shogren et al., 2020). More seasonal and spatial coverage, including land-sea connectivity at more extensive spatial scales than currently conducted, are important for understanding physical processes such as sea ice loss, water freshening, nutrient and carbon cycles (Brown et al., 2020; Shogren et al., 2020; Timmermans and Marshall, 2020), as well as biological processes (Berge et al., 2015; Geoffroy and Priou, 2020). Deployment of dedicated autonomous technologies are often suggested to fill this data gap. In addition, approaches such as participatory modelling tools have a strong potential to fill the knowledge gaps around e.g., winter processes, using local ecological knowledge (Pedro et al., 2023). A more comprehensive overview and monitoring of Arctic ecosystem borealization would also necessitate the allocation of research resources to characterise and better understand processes of relatively understudied compartments such as microbial communities, epibenthos and infauna (CAFF, 2017).
4.2 Through the spyglass: a boreal Arctic in the future?
Predicting the extent to which Arctic borealization will continue in the future across ecosystem compartments is a priority that has received much attention from the scientific community. Climatic scenarios from the coupled model intercomparison project (CMIP) suggest various trajectories for future Arctic climatic and oceanographic conditions, but all scenarios display similar trajectories until the 2040s. The Artic will likely continue to warm at a faster rate than mid-latitude systems. Arctic amplification could continue under a low emission scenario, where ice albedo feedback would maintain the phenomenon, but it would be less prominent in a high-emission scenario (Ono et al., 2022). The increase in heat transport from the Atlantic and the Pacific will likely continue and progress poleward through the Bering Strait and the Barents Sea (Drinkwater et al., 2021; Dörr et al., 2024). All shelves will become ice free in the summer, irrespective of the climatic scenario, and the Barents Sea could become ice free in winter by the end of the century (Årthun et al., 2021). Expanding open water areas and increasing precipitation will contribute to large and rapid changes in hydrological cycles (McCrystall et al., 2021). Increased river runoffs should increase stratification and subsurface warming in the central Arctic Ocean (Nummelin et al., 2016).
It is challenging to assess the degree of borealization of biogeochemical processes and microbial communities at pan-Arctic scales. Primary production is arguably the microbial process that has received most attention in the literature, and numerous studies suggest an evolution towards a more boreal functioning in the future (Ardyna and Arrigo, 2020; Noh et al., 2024). The Pacific and Atlantic inflow shelves will likely experience the strongest increase in primary productivity, although this is more uncertain for the western Barents Sea, where oceanographic models project variable directions and magnitudes of change depending on how stratification processes are represented (Mueter et al., 2021b; Mousing et al., 2023). However, increasing cloud cover (Bélanger et al., 2013) and strong seasonality in light conditions at higher latitudes are likely to limit the transition towards a completely boreal phenology, and primary production should retain some characteristics of an Arctic system, which would lead to arctic-boreal hybrid functioning. Yool et al. (2015) projected that, by 2,100, despite an increase in productivity driven by the loss of sea ice, the Arctic will not be more productive than the Atlantic, with both systems entering a low nutrient regime. In contrast, the Pacific, richer in nutrients, might experience a longer rise in productivity (Yool et al., 2015). However, the trajectory of nutrient limitation remains a significant knowledge gap, leaving predictions of Arctic productivity in the future uncertain (Slagstad et al., 2015; Mueter et al., 2021b; Mousing et al., 2023). While observational data on other microbial processes across the pan-Arctic remain limited, experimental studies offer valuable insights into potential shifts in crucial microbial functions. For example, temperature variations might influence bacterial metabolic rates, phytoplankton community structure and chlorophyll a concentrations and, consequently, carbon cycling (Kritzberg et al., 2010).
The climate driven environmental changes will continue to trigger distributional shifts and changes in biodiversity across functional groups and Arctic regions. These shifts are quantitatively explored based on species distribution models fitted to species’ current thermal preferences. Ensemble modelling predicts an increase in phytoplankton species richness all over the globe under the highest warming scenario, by the end of the century, except in the Arctic, where no trend is expected (Benedetti et al., 2021). Other projections with similar time horizons suggest that poleward shifts in zooplankton distribution could continue at a pace of 8.7 km/decade, accompanied by a change in seasonal occurrence peak up to 13 days earlier, a high species turnover, and a northward shift of many boreal gelatinous zooplankton, sometimes only into sub-polar regions (Villarino et al., 2015; Benedetti et al., 2021; Pantiukhin et al., 2023; Pantiukhin et al., 2024; Cheung et al., 2015) also predicted a northward range expansion for Pacific pelagic fish species of 30.1 ± 2.34 (S.E.) km decade−1. However, regional predictions for Canadian waters predict a decline in biomass in both the Pacific and Atlantic sides (Bryndum-Buchholz et al., 2020). In contrast, projections under a high emission scenario reveal an increase in total forage fish biomass in Hudson Bay, but with decreasing fish size and a shift from lipid rich polar cod to smaller boreal fish (Florko et al., 2021). Overall, responses to climate change are likely region-specific, as well as species specific and are thus challenging to predict (Mueter and Litzow, 2008; Alabia et al., 2018; Husson et al., 2020; Calvet et al., 2024). The impact of climate change on benthic habitats may depend on the spatial scale of the projection. In the Bering and Chukchi seas, the habitat of many assemblages of epibenthic invertebrates is predicted to be reduced by 50% by 2050 and almost 100% by 2,100, with the exception of eurythermal species such as the basket star Gorgonocephalus cf. arcticus (Logerwell et al., 2022). At the pan-Arctic scale, however, habitat change for benthic species might be limited in the future (Renaud et al., 2019). Habitat loss should mainly affect currently cold and aragonite-oversaturated areas such as northern Barents Sea and east Greenland Sea (Renaud et al., 2019), and cold-stenothermal species such as gastropods and bivalve mussels Musculus spp. in the Bering and Chukchi Sea, leading to large losses of biodiversity locally (Logerwell et al., 2022). At the ecosystem-level, long-term projections using species distribution ensemble models suggest an increase in species richness and functional redundancy poleward with an increase in predatory taxa that will threaten Arctic species and decrease the modularity of Arctic food webs in the Bering and Chukchi seas (Alabia et al., 2020). It is predicted that the co-occurrence of boreal and arctic fish species in borealized regions will vary from 1 year to the other, with more Arctic species in cold years and more boreal species in warm years (Geoffroy et al., 2023), as seen in the Labrador and Bering seas (Marsh and Mueter, 2020).
The poleward progression of communities could lead to many changes in seascapes, with shifts in essential habitat localisation and altered connectivity, leading to declining stocks and a potential increase in population mixing and hybridization by the end of the century. Habitat forming species such as macroalgae are likely to continue their colonisation of coastal Arctic regions, with more of the Canada, Greenland and Svalbard coasts becoming suitable habitats due to less ice cover for three important macroalgae species by 2,100 (Jueterbock et al., 2013). In contrast, in the soft-sediment dominated Beaufort Sea, kelp production has been shown to be limited by wind speed that resuspends river and coastal sediments and limits light penetration to the seabed (Bonsell and Dunton, 2018). This is likely to affect nursery and feeding habitats, although their variations are only partially understood and might be affected by multiple factors. Along the Norwegian coast, Northeast Arctic cod spawning locations have been fluctuating with a northward trend, seemingly under the effect of population demography, while the effect of climatic factors is uncertain (Höffle et al., 2014; Opdal and Jørgensen, 2015). Similarly, spawning locations of Northeast Arctic haddock (Melanogrammus aeglefinus) are likely driven by density dependence, rather than temperature (Langangen et al., 2018). Finally, predation by boreal species has been shown to influence capelin’s spawning area (Olsen et al., 2024). It is thus challenging to model future locations of spawning sites. Nevertheless, modelling studies based on thermal preferences and bottlenecks of species early life stages can give a first estimate. Capelin is anticipated to shift spawning north-eastward in the Barents Sea (Huse and Ellingsen, 2008). For Northeast Arctic cod, further northward shifts in spawning sites could affect juveniles’ survival and increase their sensitivity to spawning timing (Endo et al., 2024).
Population mixing will likely increase and would probably occur via the Northeast and Northwest Passages (Vermeij and Roopnarine, 2008; Kelly et al., 2010; Wisz et al., 2015). This has likely already started, as suggested by the first observations of interoceanic dispersals (e.g., a Pacific diatom in the Atlantic waters, Reid et al., 2007). The Greenland halibut Pacific and Atlantic populations, with so far limited genetic mixing (Orlova et al., 2019), are now increasingly interacting along the Eurasian Arctic, suggesting that a potential reunification of those populations is possible in the future (Orlov and Volvenko, 2024).
To summarize, all climatic scenarios point to a profound transformation of the Arctic oceanic functioning. Under those conditions, many species will shift, but not all, and not all the way into the Arctic Basin, where conditions will remain harsh. Ecosystems’ primary productivity, biodiversity, functioning and food webs will be altered. All this suggests that Arctic marine ecosystems might not entirely transform into a boreal functioning, but rather stabilize in a hybrid state between Arctic and boreal ecosystem at a latitudinal limit north of the current one, with transient borealization during warmer periods.
4.3 Into the fog: uncertainties and key questions
Large uncertainties and bias surround projected trajectories for the Arctic and sub-Arctic ecosystems. Arctic Ocean circulation is complex, with numerous feedback and potentially tipping processes among and between the cryosphere, hydrosphere and atmosphere, and several possible future evolutions depending on climate change intensity (Lannuzel et al., 2020; Timmermans and Marshall, 2020). The most recent version of the Coupled Model Intercomparison Project (CMIP6) has persistent bias on Arctic water masses representations, inherited from the previous version, which necessitate major improvements, notably by using higher model resolutions (Khosravi et al., 2022). For example, other studies showed that heat transport from lower latitudes might be underestimated by model with too coarse spatial resolution (Xu et al., 2024).
Projections of community shifts in the future are also associated with considerable uncertainties, although hard to estimate and thus rarely mentioned in the studies. The potential for further borealization of the ecosystem depends on species habitat preferences, the location and connectivity of their essential habitats (spawning, nursery, feeding grounds), and their capacity to acclimatise and then adapt to new abiotic and biotic conditions. Projecting species future habitats using species distribution models is a first approach to anticipating species movement. However, those models are often based on strong assumptions, such as environmental niche stability, and are built around limited number of environmental covariables, ignoring the impact of population dynamics and interspecific interactions on species distributions. Among environmental variables, factors that do not vary over time are not always included. Indeed, while temperature, salinity or oxygen, which will be affected by future climate change, can be used as time-varying predictors of most fish species’ suitable habitats, poleward displacement might be limited by non-dynamic factors such as depth, light regimes, or seabed type. Considering a species’ traits and life cycle can help estimate the likelihood of that species to shift northward. Using this approach, it is assumed that cod and haddock have reached their northern limit at the shelf break of the Barents Sea and minke whale or harp seals will likely follow the ice edge, while other species, such as capelin, redfish or snow crab will likely be able to expand further into the Arctic (Hollowed et al., 2013b; Hollowed et al., 2013a; Haug et al., 2017; Mullowney et al., 2023).
When conditions in the Arctic are limiting, species that will not be able to shift their distribution will have to acclimate, then adapt, or die (Hoffmann and Parsons, 1990), and this will impact the pace and extent of borealization across regions and functional groups. Adaptation to environmental stress manifests itself mainly via two mechanisms: the species plasticity (capacity of a genotype to express different phenotypes under varying environmental stress), and the selection, on evolutionary scales, of certain phenotypes. Species phenotypic plasticity includes, among others, behavioural flexibility and physiological tolerance, both of which are challenging to observe in natural environments (Beever et al., 2017) and manifest differently across a species’ range (Donelson et al., 2019). Plasticity can be a way to cope temporarily with environmental stress, but can also hinder adaptation in some cases (Fox et al., 2019). It is thus important to assess species adaptive capacity to understand species responses to climate change and support conservation and management efforts (Hoffmann and Sgrò, 2011). This can be done by considering the species fundamental adaptive capacity, characterised via its functional traits such as longevity, mobility, and dispersal capacity (Beever et al., 2016). Such approaches, along with experiments on species thermal adaptivity, are often suggested as potential improvements to species distribution models (Drost et al., 2014; Drost et al., 2016; Logerwell et al., 2022). Evolutionary models could integrate that information and incorporate interspecific interactions to further explore possible trajectories of co-evolution for Arctic marine systems.
Finally, lasting borealization, emerging from species completing their life cycle, implies that environmental conditions are suitable for all life stages (Wassmann et al., 2015), of which early life stages and spawning adults are often the bottlenecks (Dahlke et al., 2020). Several species have been suggested to be able to complete their life cycle in areas where it was previously not observed, for example, the boreal capelin in Hudson Bay (Gaston and Elliott, 2014), the copepod C. finmarchicus and the Atlantic hyperiid amphipod Themisto compressa at the Fram Strait, with the latter two species much poorer in lipid than their Arctic counterparts (Kraft et al., 2013; Tarling et al., 2022). Connectivity between the habitats and along dispersal routes is thus a key consideration when projecting population shifts and species range shifts. Knowledge on thermal preferences of early life stages enabled modellers to estimate changes in spawning sites for cod and polar cod under various gas emission scenarios (Dahlke et al., 2020; Sanders et al., 2022). Results of these studies all suggest a deterioration of the current spawning sites for both species under high emission scenarios, and more limited impacts for low emission scenarios. In addition, some local conditions such as depth may act as barriers to dispersal of fish species across the pan-Arctic (Bouchard et al., 2018).
4.4 Risks and vulnerability of borealized socio-ecological systems
Despite some uncertainties and knowledge gaps, there is a clear consensus on the ongoing borealization of Arctic ecosystems, and its likely continuation in the future. Arctic endemic communities and ecosystems are most at risk, if they are unable to adapt to the multiple environmental stressors or to compete with new incoming species (Kędra et al., 2015). Sympagic species or species with a strong link to the ice, through their diet or life cycle, are particularly at risk (Kohlbach et al., 2016), while some may survive in the pelagic zone (Kunisch et al., 2020). The ice association is well documented for example, for polar cod. In the Chukchi Sea, its body condition appears to be lower during warm years, suggesting that the region is warming beyond the species’ thermal limits (Copeman et al., 2022). With sea ice loss, polar cod will lose its spawning habitat (Huserbråten et al., 2019), and new incoming species could outcompete it (Mueter et al., 2016). However, some studies suggest that competition for food might be limited at least for early life stages, through niche partitioning (Falardeau et al., 2014; Bouchard et al., 2022). Moreover, in the Canadian Arctic Archipelago and Central Arctic Ocean, the relaxation of the most extreme conditions with climate change could benefit polar cod, at least in the short term (Bouchard et al., 2017; Steiner et al., 2019; Geoffroy et al., 2023). There, larval survival of polar cod increases in years with earlier ice-breakup and warmer sea surface temperatures (Bouchard et al., 2017).
With sea-ice loss and increased open water area, carbon sinking and ocean acidification are likely to increase (Lannuzel et al., 2020; Terhaar et al., 2020; Qi et al., 2022), adding to the effect of warming to affect species habitats and distributions. The extent of the impacts of ocean acidification on marine populations is still not well understood. Experimental studies show that acidification affect coastal phytoplankton species growth, colony length and chain length, although the effect vary across species (Thoisen et al., 2015). In addition, increased acidification could lower phytoplankton growth rates and amplify the decrease in primary production with warming (Coello-Camba et al., 2014), and alter microbial community composition (Brussaard et al., 2013; Sugie et al., 2020). Finally, acidification can lead to the dissolution of Arctic benthic invertebrate shells (Comeau et al., 2009; Niemi et al., 2021) and, in combination with warming temperatures, increased consumption by Arctic brittle stars (Wood et al., 2011). However, some Arctic bivalves are more resilient to decreasing pH (Goethel et al., 2017).
In addition to those climate-driven pressures, Arctic ecosystems will experience increasing pressures from many other anthropogenic sources. Microplastic contamination is already affecting benthic organisms in the Chukchi Sea and other areas of the Arctic through local sources and transport (Fang et al., 2018; Bergmann et al., 2022). New ice-free conditions along the shelves and seasonally in the Central Arctic Ocean would allow Arctic shipping year-round starting in the 2070s (Min et al., 2022), leading to increased concentrations in pollutants (Svavarsson et al., 2021). This increase in shipping would also be prompted by industrial opportunities newly accessible in a future seasonally ice-free Arctic, such as mineral, oil and gas extraction, of fishing. Indeed, large global models project a high potential for Blue Economy in the Arctic, with potential for new fisheries, owing to increased local productivity and incoming new stocks (Cheung et al., 2015). However, the latest FAO report stresses the high risk associated with potential fishing in the Arctic due to very high uncertainties around the future biomass projections associated with the two main Earth system models used to force the simulations (Tittensor et al., 2021; Blanchard and Novaglio, 2024). In addition, climate is not the only factor affecting Arctic fisheries, and complex cross-scale, cross-domain (ecology, society, economy) processes can greatly affect the future of pan-Arctic fisheries (Niiranen et al., 2018). The potential growth of biomass of commercial stocks thus call for new management approaches (Christiansen et al., 2014; McBride et al., 2014) and careful consideration of potential new fishing opportunities. For example, a study investigating the potential for a fishery on walleye pollock that are expanding into the western Chukchi Sea highlight strong uncertainties around the amount of biomass entering the region, the state of the stock, and the possibility of harvesting considering the harsh local fishing conditions (Maznikova et al., 2023). Such complex systems are challenging to apprehend, and models are often a key tool to anticipate future changes. To date, few models explore regional ecosystem response to potential effects of climate change scenarios on ecosystem services and human wellbeing, with or without considering other human pressures such as fisheries. The few projections that have been completed anticipate a decrease in fish biomass or high uncertainties in biomass trajectories, thus challenging the hypothesis of a future Blue Growth in the Arctic (Bryndum-Buchholz et al., 2020; Whitehouse et al., 2021).
In the meantime, some traditional local fisheries are threatened by ecosystem changes. For example, the winter ice fishery on Greenland Halibut (Reinhardtius hippoglossoides) is now being threatened by the progressive loss of sea ice (Hussey et al., 2017). Fishermen in Greenland are shifting their traditional fishing practices in response to changes in ice cover, and are observing new species (Schiøtt et al., 2022). Local ecological knowledge, including knowledge from Indigenous communities whose livelihoods and cultures are tightly linked to Arctic ecosystems, is increasingly highlighted as a most valuable tool to face future challenges and apply an adaptive strategy to the fast changing Arctic (Riedlinger and Berkes, 2001; Schwoerer et al., 2021). Local fishermen and hunters have detailed knowledge on seasonal and spatial scales that are rarely covered by any scientific survey, and are highly useful to combine with scientific knowledge and data do detect changes in Arctic ecosystems and threats to traditional livelihoods (Chila et al., 2022; Falardeau et al., 2022; Bouchard et al., 2023; Dunmall et al., 2024). Better inclusion of this rich source of information could be done through community-based monitoring and co-construction of scenarios to directly inform management (Nilsson et al., 2021; Falardeau et al., 2022). Indeed, balancing ecosystem and livelihood conservation and the increasing economic interests in the Arctic necessitate consideration of the whole socio-ecological system, but a holistic, interdisciplinary and systematic approach is currently lacking to properly picture the complexity of the Arctic socio-ecological system and the pressures that affect it (Falardeau and Bennett, 2020; Eerkes-Medrano and Huntington, 2021). Identified gaps include for example: the heterogenous knowledge base across the Arctic, limited research on cumulative impacts of climate change with other anthropogenic pressures, a lack of knowledge on some feedbacks between ecosystem services and human well-being, and a lack of projection into the future for human systems (Falardeau and Bennett, 2020). Considering the local and global importance of Arctic climatic and biological processes, urgent mitigation and adaptive actions are needed (Overland et al., 2019).
Author contributions
BH: Conceptualization, Writing–original draft, Writing–review and editing. BB: Writing–review and editing. FC: Conceptualization, Formal Analysis, Writing–review and editing. SD: Conceptualization, Writing–review and editing. EE: Writing–review and editing. MF: Writing–review and editing. MG: Writing–review and editing. RH: Writing–review and editing. RBI: Conceptualization, Writing–review and editing. LJ: Writing–review and editing. CL: Writing–review and editing. LM: Writing–review and editing. FM: Writing–review and editing. RP: Writing–review and editing. MW: Writing–review and editing.
Funding
The author(s) declare that financial support was received for the research, authorship, and/or publication of this article. BH, EE, MF, RBI and LLJ work was supported by internal funding from the Institute of Marine Research, Norway. BB contributed as a member of the Circumpolar Biodiversity Monitoring Program under the Conservation of Arctic Fauna and Flora Working group of the Arctic Council and acknowledges their support. MG was financially supported by Crown-Indigenous Relations and Northern Affairs Canada (CIRNAC) and Natural Sciences and Engineering Research Council of Canada (NSERC). SD was financially supported by NSF OPP grant #2053084.
Acknowledgments
We thank the ECMWF and the Copernicus Climate Change Service for allowing us to use their figure on sea ice indicator. We are grateful to AMAP/CBMP-CAFF for sharing publicly the shapefiles of the Arctic Ocean and Adjacent Seas.
Conflict of interest
The authors declare that the research was conducted in the absence of any commercial or financial relationships that could be construed as a potential conflict of interest.
Publisher’s note
All claims expressed in this article are solely those of the authors and do not necessarily represent those of their affiliated organizations, or those of the publisher, the editors and the reviewers. Any product that may be evaluated in this article, or claim that may be made by its manufacturer, is not guaranteed or endorsed by the publisher.
References
Abe, Y., Matsuno, K., Fujiwara, A., and Yamaguchi, A. (2020). Review of spatial and inter-annual changes in the zooplankton community structure in the western Arctic Ocean during summers of 2008–2017. Prog. Oceanogr. 186, 102391. doi:10.1016/j.pocean.2020.102391
Alabia, I. D., García Molinos, J., Hirata, T., Mueter, F. J., and David, C. L. (2023). Pan-Arctic marine biodiversity and species co-occurrence patterns under recent climate. Sci. Rep. 13, 4076. doi:10.1038/s41598-023-30943-y
Alabia, I. D., García Molinos, J., Saitoh, S.-I., Hirawake, T., Hirata, T., and Mueter, F. J. (2018). Distribution shifts of marine taxa in the Pacific Arctic under contemporary climate changes. Divers. Distributions 24, 1583–1597. doi:10.1111/ddi.12788
Alabia, I. D., Molinos, J. G., Saitoh, S.-I., Hirata, T., Hirawake, T., and Mueter, F. J. (2020). Multiple facets of marine biodiversity in the Pacific Arctic under future climate. Sci. Total Environ. 744, 140913. doi:10.1016/j.scitotenv.2020.140913
Alfaro-Lucas, J. M., Chaudhary, C., Brandt, A., and Saeedi, H. (2023). Species composition comparisons and relationships of Arctic marine ecoregions. Deep Sea Res. Part I Oceanogr. Res. Pap. 198, 104077. doi:10.1016/j.dsr.2023.104077
Al-Habahbeh, A. K., Kortsch, S., Bluhm, B. A., Beuchel, F., Gulliksen, B., Ballantine, C., et al. (2020). Arctic coastal benthos long-term responses to perturbations under climate warming. Philosophical Trans. R. Soc. A Math. Phys. Eng. Sci. 378, 20190355. doi:10.1098/rsta.2019.0355
Anderson, D. M., Fachon, E., Hubbard, K., Lefebvre, K. A., Lin, P., Pickart, R., et al. (2022). Harmful algal blooms in the alaskan arctic: an emerging threat as the ocean warms. Oceanography 35, 130–139. doi:10.5670/oceanog.2022.121
Anderson, D. M., Fachon, E., Pickart, R. S., Lin, P., Fischer, A. D., Richlen, M. L., et al. (2021). Evidence for massive and recurrent toxic blooms of Alexandrium catenella in the Alaskan Arctic. Proc. Natl. Acad. Sci. 118, e2107387118. doi:10.1073/pnas.2107387118
Anderson, L. G., and Macdonald, R. W. (2015). Observing the Arctic Ocean carbon cycle in a changing environment. Polar Res. 34, 26891. doi:10.3402/polar.v34.26891
Anderson, M. A., Fisk, A. T., Laing, R., Noël, M., Angnatok, J., Kirk, J., et al. (2023). Changing environmental conditions have altered the feeding ecology of two keystone Arctic marine predators. Sci. Rep. 13, 14056. doi:10.1038/s41598-023-39091-9
Andrews, A. J., Christiansen, J. S., Bhat, S., Lynghammar, A., Westgaard, J.-I., Pampoulie, C., et al. (2019). Boreal marine fauna from the Barents Sea disperse to arctic northeast Greenland. Sci. Rep. 9, 5799. doi:10.1038/s41598-019-42097-x
Ardyna, M., and Arrigo, K. R. (2020). Phytoplankton dynamics in a changing Arctic Ocean. Nat. Clim. Change 10, 892–903. doi:10.1038/s41558-020-0905-y
Ardyna, M., Babin, M., Gosselin, M., Devred, E., Rainville, L., and Tremblay, J.-É. (2014). Recent Arctic Ocean sea ice loss triggers novel fall phytoplankton blooms. Geophys. Res. Lett. 41, 6207–6212. doi:10.1002/2014GL061047
Ardyna, M., Gosselin, M., Michel, C., Poulin, M., and Tremblay, J.-É. (2011). Environmental forcing of phytoplankton community structure and function in the Canadian High Arctic: contrasting oligotrophic and eutrophic regions. Mar. Ecol. Prog. Ser. 442, 37–57. doi:10.3354/meps09378
Armitage, T. W. K., Bacon, S., Ridout, A. L., Petty, A. A., Wolbach, S., and Tsamados, M. (2017). Arctic Ocean surface geostrophic circulation 2003–2014. Cryosphere 11, 1767–1780. doi:10.5194/tc-11-1767-2017
Arrigo, K. R., Dijken, G. van, and Pabi, S. (2008). Impact of a shrinking Arctic ice cover on marine primary production. Geophys. Res. Lett. 35. doi:10.1029/2008GL035028
Arrigo, K. R., Perovich, D. K., Pickart, R. S., Brown, Z. W., Dijken, G. L. V., Lowry, K. E., et al. (2012). Massive phytoplankton blooms under arctic sea ice. Science 336, 1408. doi:10.1126/science.1215065
Arrigo, K. R., and van Dijken, G. L. (2015). Continued increases in Arctic Ocean primary production. Prog. Oceanogr. 136, 60–70. doi:10.1016/j.pocean.2015.05.002
Årthun, M., Onarheim, I. H., Dörr, J., and Eldevik, T. (2021). The seasonal and regional transition to an ice-free arctic. Geophys. Res. Lett. 48, e2020GL090825. doi:10.1029/2020GL090825
Ashjian, C. J., Okkonen, S. R., Campbell, R. G., and Alatalo, P. (2021). Lingering Chukchi Sea sea ice and Chukchi Sea mean winds influence population age structure of euphausiids (krill) found in the bowhead whale feeding hotspot near Pt. Barrow, Alaska. PLOS ONE 16, e0254418. doi:10.1371/journal.pone.0254418
Assmy, P., Fernández-Méndez, M., Duarte, P., Meyer, A., Randelhoff, A., Mundy, C. J., et al. (2017). Leads in Arctic pack ice enable early phytoplankton blooms below snow-covered sea ice. Sci. Rep. 7, 40850. doi:10.1038/srep40850
Aune, M., Aschan, M. M., Greenacre, M., Dolgov, A. V., Fossheim, M., and Primicerio, R. (2018). Functional roles and redundancy of demersal Barents Sea fish: ecological implications of environmental change. PLOS ONE 13, e0207451. doi:10.1371/journal.pone.0207451
Axler, K. E., Goldstein, E. D., Nielsen, J. M., Deary, A. L., and Duffy-Anderson, J. T. (2023). Shifts in the composition and distribution of Pacific Arctic larval fish assemblages in response to rapid ecosystem change. Glob. Change Biol. 29, 4212–4233. doi:10.1111/gcb.16721
Azovsky, A. I., and Kokarev, V. N. (2019). Stable but fragile: long-term dynamics of arctic benthic macrofauna in Baydaratskaya Bay (the Kara Sea). Polar Biol. 42, 1307–1322. doi:10.1007/s00300-019-02519-y
Baker, M. R., De Robertis, A., Levine, R. M., Cooper, D. W., and Farley, E. V. (2022). Spatial distribution of arctic sand lance in the Chukchi Sea related to the physical environment. Deep Sea Res. Part II Top. Stud. Oceanogr. 206, 105213. doi:10.1016/j.dsr2.2022.105213
Balazy, K., Trudnowska, E., Wichorowski, M., and Błachowiak-Samołyk, K. (2018). Large versus small zooplankton in relation to temperature in the Arctic shelf region. Polar Res. 37, 1427409. doi:10.1080/17518369.2018.1427409
Beever, E. A., Hall, L. E., Varner, J., Loosen, A. E., Dunham, J. B., Gahl, M. K., et al. (2017). Behavioral flexibility as a mechanism for coping with climate change. Front. Ecol. Environ. 15, 299–308. doi:10.1002/fee.1502
Beever, E. A., O’Leary, J., Mengelt, C., West, J. M., Julius, S., Green, N., et al. (2016). Improving conservation outcomes with a new paradigm for understanding species’ fundamental and realized adaptive capacity. Conserv. Lett. 9, 131–137. doi:10.1111/conl.12190
Bélanger, S., Babin, M., and Tremblay, J.-É. (2013). Increasing cloudiness in Arctic damps the increase in phytoplankton primary production due to sea ice receding. Biogeosciences 10, 4087–4101. doi:10.5194/bg-10-4087-2013
Benedetti, F., Vogt, M., Elizondo, U. H., Righetti, D., Zimmermann, N. E., and Gruber, N. (2021). Major restructuring of marine plankton assemblages under global warming. Nat. Commun. 12, 5226. doi:10.1038/s41467-021-25385-x
Bengtsson, L., Semenov, V. A., and Johannessen, O. M. (2004). The early twentieth-century warming in the arctic—a possible mechanism. J. Clim. 17, 4045–4057. doi:10.1175/1520-0442(2004)017<4045:tetwit>2.0.co;2
Benkort, D., Plourde, S., Winkler, G., Cabrol, J., Ollier, A., Cope, L.-E., et al. (2019). Individual-based modeling explains the contrasted seasonality in size, growth, and reproduction of the sympatric Arctic (Thysanoessa raschii) and Nordic krill (Meganyctiphanes norvegica) in the St. Lawrence Estuary, eastern Canada. Limnol. Oceanogr. 64, 217–237. doi:10.1002/lno.11032
Benzik, A. N., Budanova, L. K., and Orlov, A. M. (2022). Hard life in cold waters: size distribution and gonads show that Greenland halibut temporarily inhabit the Siberian Arctic. Water Biol. Secur. 1, 100037. doi:10.1016/j.watbs.2022.100037
Berge, J., Johnsen, G., and Cohen, J. H. (2020). POLAR NIGHT marine ecology: life and light in the dead of night (Cham: Springer International Publishing). doi:10.1007/978-3-030-33208-2
Berge, J., Renaud, P. E., Darnis, G., Cottier, F., Last, K., Gabrielsen, T. M., et al. (2015). In the dark: a review of ecosystem processes during the Arctic polar night. Prog. Oceanogr. 139, 258–271. doi:10.1016/j.pocean.2015.08.005
Bergmann, M., Collard, F., Fabres, J., Gabrielsen, G. W., Provencher, J. F., Rochman, C. M., et al. (2022). Plastic pollution in the arctic. Nat. Rev. Earth Environ. 3, 323–337. doi:10.1038/s43017-022-00279-8
Bergstad, O. A., Johannesen, E., Høines, Å., Ellingsen, K. E., Lien, V. S., Byrkjedal, I., et al. (2018). Demersal fish assemblages in the boreo-Arctic shelf waters around Svalbard during the warm period 2007–2014. Polar Biol. 41, 125–142. doi:10.1007/s00300-017-2176-2
Berline, L., Spitz, Y. H., Ashjian, C. J., Campbell, R. G., Maslowski, W., and Moore, S. E. (2008). Euphausiid transport in the western Arctic Ocean. Mar. Ecol. Prog. Ser. 360, 163–178. doi:10.3354/meps07387
Bernardo, C., Pecuchet, L., Santos, J., Dolgov, A., Husson, B., Fossheim, M., et al. (2024). Warming changes the life history composition of marine fish communities at high latitudes. Mar. Ecol. Prog. Ser. 732, 119–133. doi:10.3354/meps14537
Beuchel, F., Gulliksen, B., and Carroll, M. L. (2006). Long-term patterns of rocky bottom macrobenthic community structure in an Arctic fjord (Kongsfjorden, Svalbard) in relation to climate variability (1980–2003). J. Mar. Syst. 63, 35–48. doi:10.1016/j.jmarsys.2006.05.002
Bezzubova, E. M., Romanova, N. D., Shchuka, A. S., Seliverstova, A. M., Plotnikov, N. A., and Volodina, A. A. (2024). Free-living bacterial diversity in the Kara and Laptev seas: spatial variability and environmental control. Polar Biol. 47, 209–226. doi:10.1007/s00300-023-03221-w
Bienhold, C., Schourup-Kristensen, V., Krumpen, T., Nöthig, E.-M., Wenzhöfer, F., Korhonen, M., et al. (2022). Effects of sea ice retreat and ocean warming on the Laptev Sea continental slope ecosystem (1993 vs 2012). Front. Mar. Sci. 9. doi:10.3389/fmars.2022.1004959
Blais, M., Ardyna, M., Gosselin, M., Dumont, D., Bélanger, S., Tremblay, J.-É., et al. (2017). Contrasting interannual changes in phytoplankton productivity and community structure in the coastal Canadian Arctic Ocean. Limnol. Oceanogr. 62, 2480–2497. doi:10.1002/lno.10581
Blanchard, J. L., and Novaglio, C. (2024). Climate change risks to marine ecosystems and fisheries. China, FAO; Available at: https://openknowledge.fao.org/handle/20.500.14283/cd1379en (Accessed August 13, 2024).
Blanchet, M.-A., Primicerio, R., Frainer, A., Kortsch, S., Skern-Mauritzen, M., Dolgov, A. V., et al. (2019). The role of marine mammals in the Barents Sea foodweb. ICES J. Mar. Sci. 76, i37–i53. doi:10.1093/icesjms/fsz136
Bluhm, B. A., Ambrose, W. G., Bergmann, M., Clough, L. M., Gebruk, A. V., Hasemann, C., et al. (2011a). Diversity of the arctic deep-sea benthos. Mar. Biodiv 41, 87–107. doi:10.1007/s12526-010-0078-4
Bluhm, B. A., Gebruk, A. V., Gradinger, R., Hopcroft, R. R., Huettmann, F., Kosobokova, K. N., et al. (2011b). Arctic marine biodiversity: an update of species richness and examples of biodiversity change. Oceanography 24, 232–248. doi:10.5670/oceanog.2011.75
Bluhm, B. A., Janout, M. A., Danielson, S. L., Ellingsen, I., Gavrilo, M., Grebmeier, J. M., et al. (2020). The pan-arctic continental slope: sharp gradients of physical processes affect pelagic and benthic ecosystems. Front. Mar. Sci. 7. doi:10.3389/fmars.2020.544386
Bluhm, B. A., Kosobokova, K. N., and Carmack, E. C. (2015). A tale of two basins: an integrated physical and biological perspective of the deep Arctic Ocean. Prog. Oceanogr. 139, 89–121. doi:10.1016/j.pocean.2015.07.011
Boetius, A., Albrecht, S., Bakker, K., Bienhold, C., Felden, J., Fernández-Méndez, M., et al. (2013). Export of algal biomass from the melting arctic sea ice. Science 339, 1430–1432. doi:10.1126/science.1231346
Bonsell, C., and Dunton, K. H. (2018). Long-term patterns of benthic irradiance and kelp production in the central Beaufort sea reveal implications of warming for Arctic inner shelves. Prog. Oceanogr. 162, 160–170. doi:10.1016/j.pocean.2018.02.016
Bouchard, C., Chawarski, J., Geoffroy, M., Klasmeier, A., Møller, E. F., Mohn, C., et al. (2022). Resource partitioning may limit interspecific competition among Arctic fish species during early life. Elem. Sci. Anthropocene 10, 00038. doi:10.1525/elementa.2021.00038
Bouchard, C., Farnole, P., Lynge-Pedersen, K., Dahl, P. E., and Christiansen, H. (2023). Arctic cod (Boreogadus saida) in fjord and glacial habitats: a collaborative study with Uummannap Kangerlua Fishers. Arct. Sci. 9, 781–795. doi:10.1139/as-2023-0014
Bouchard, C., and Fortier, L. (2020). The importance of Calanus glacialis for the feeding success of young polar cod: a circumpolar synthesis. Polar Biol. 43, 1095–1107. doi:10.1007/s00300-020-02643-0
Bouchard, C., Geoffroy, M., LeBlanc, M., and Fortier, L. (2018). Larval and adult fish assemblages along the Northwest Passage: the shallow Kitikmeot and the ice-covered Parry Channel as potential barriers to dispersal. Arct. Sci. 4, 781–793. doi:10.1139/as-2018-0003
Bouchard, C., Geoffroy, M., LeBlanc, M., Majewski, A., Gauthier, S., Walkusz, W., et al. (2017). Climate warming enhances polar cod recruitment, at least transiently. Prog. Oceanogr. 156, 121–129. doi:10.1016/j.pocean.2017.06.008
Box, J. E., Colgan, W. T., Christensen, T. R., Schmidt, N. M., Lund, M., Parmentier, F.-J. W., et al. (2019). Key indicators of Arctic climate change: 1971–2017. Environ. Res. Lett. 14, 045010. doi:10.1088/1748-9326/aafc1b
Brandt, S., Wassmann, P., and Piepenburg, D. (2023). Revisiting the footprints of climate change in Arctic marine food webs: an assessment of knowledge gained since 2010. Front. Mar. Sci. 10. doi:10.3389/fmars.2023.1096222
Brodeur, R. D., Decker, M. B., Ciannelli, L., Purcell, J. E., Bond, N. A., Stabeno, P. J., et al. (2008). Rise and fall of jellyfish in the eastern Bering Sea in relation to climate regime shifts. Prog. Oceanogr. 77, 103–111. doi:10.1016/j.pocean.2008.03.017
Brodeur, R. D., Sugisaki, H., and Jr, G. L. H. (2002). Increases in jellyfish biomass in the Bering Sea: implications for the ecosystem. Mar. Ecol. Prog. Ser. 233, 89–103. doi:10.3354/meps233089
Brown, K. A., Holding, J. M., and Carmack, E. C. (2020). Understanding regional and seasonal variability is key to gaining a pan-arctic perspective on Arctic Ocean freshening. Front. Mar. Sci. 7. doi:10.3389/fmars.2020.00606
Brown, T. A., Assmy, P., Hop, H., Wold, A., and Belt, S. T. (2017). Transfer of ice algae carbon to ice-associated amphipods in the high-Arctic pack ice environment. J. Plankton Res. 39, 664–674. doi:10.1093/plankt/fbx030
Bruhn, C. S., Wohlrab, S., Krock, B., Lundholm, N., and John, U. (2021). Seasonal plankton succession is in accordance with phycotoxin occurrence in Disko Bay, West Greenland. Harmful Algae 103, 101978. doi:10.1016/j.hal.2021.101978
Brussaard, C. P. D., Noordeloos, A. a. M., Witte, H., Collenteur, M. C. J., Schulz, K., Ludwig, A., et al. (2013). Arctic microbial community dynamics influenced by elevated CO2 levels. Biogeosciences 10, 719–731. doi:10.5194/bg-10-719-2013
Bryndum-Buchholz, A., Prentice, F., Tittensor, D. P., Blanchard, J. L., Cheung, W. W. L., Christensen, V., et al. (2020). Differing marine animal biomass shifts under 21st century climate change between Canada’s three oceans. FACETS 5, 105–122. doi:10.1139/facets-2019-0035
Bryndum-Buchholz, A., Tittensor, D. P., Blanchard, J. L., Cheung, W. W. L., Coll, M., Galbraith, E. D., et al. (2019). Twenty-first-century climate change impacts on marine animal biomass and ecosystem structure across ocean basins. Glob. Change Biol. 25, 459–472. doi:10.1111/gcb.14512
Burrows, M. T., Bates, A. E., Costello, M. J., Edwards, M., Edgar, G. J., Fox, C. J., et al. (2019). Ocean community warming responses explained by thermal affinities and temperature gradients. Nat. Clim. Chang. 9, 959–963. doi:10.1038/s41558-019-0631-5
Byrkjedal, I., Godø, O. R., and Heino, M. (2004). Northward range extensions of some mesopelagic fishes in the Northeastern Atlantic. Sarsia 89, 484–489. doi:10.1080/00364820410009265
Calvet, N., Bluhm, B. A., Yoccoz, N., and Altenburger, A. (2024). Shifting invertebrate distributions in the Barents Sea since pre-1900. Front. Mar. Sci. 11. doi:10.3389/fmars.2024.1421475
Carmack, E., and Wassmann, P. (2006). Food webs and physical–biological coupling on pan-Arctic shelves: unifying concepts and comprehensive perspectives. Prog. Oceanogr. 71, 446–477. doi:10.1016/j.pocean.2006.10.004
Carmack, E. C., Yamamoto-Kawai, M., Haine, T. W. N., Bacon, S., Bluhm, B. A., Lique, C., et al. (2016). Freshwater and its role in the Arctic Marine System: sources, disposition, storage, export, and physical and biogeochemical consequences in the Arctic and global oceans. J. Geophys. Res. Biogeosciences 121, 675–717. doi:10.1002/2015JG003140
Cautain, I. J., Last, K. S., Bluhm, B. A., Renaud, P. E., McKee, D., and Narayanaswamy, B. E. (2024). High uptake of sympagic organic matter by benthos on an Arctic outflow shelf. PLOS ONE 19, e0308562. doi:10.1371/journal.pone.0308562
Cautain, I. J., Last, K. S., McKee, D., Bluhm, B. A., Renaud, P. E., Ziegler, A. F., et al. (2022). Uptake of sympagic organic carbon by the Barents Sea benthos linked to sea ice seasonality. Front. Mar. Sci. 9. doi:10.3389/fmars.2022.1009303
Chambault, P., Albertsen, C. M., Patterson, T. A., Hansen, R. G., Tervo, O., Laidre, K. L., et al. (2018). Sea surface temperature predicts the movements of an Arctic cetacean: the bowhead whale. Sci. Rep. 8, 9658–9712. doi:10.1038/s41598-018-27966-1
Chambault, P., Tervo, O. M., Garde, E., Hansen, R. G., Blackwell, S. B., Williams, T. M., et al. (2020). The impact of rising sea temperatures on an Arctic top predator, the narwhal. Sci. Rep. 10, 18678. doi:10.1038/s41598-020-75658-6
Chambellant, M., Stirling, I., and Ferguson, S. H. (2013). Temporal variation in western Hudson Bay ringed seal Phoca hispida diet in relation to environment. Mar. Ecol. Prog. Ser. 481, 269–287. doi:10.3354/meps10134
Chawarski, J., Klevjer, T. A., Coté, D., and Geoffroy, M. (2022). Evidence of temperature control on mesopelagic fish and zooplankton communities at high latitudes. Front. Mar. Sci. 9. doi:10.3389/fmars.2022.917985
Cheung, W. W. L., Brodeur, R. D., Okey, T. A., and Pauly, D. (2015). Projecting future changes in distributions of pelagic fish species of Northeast Pacific shelf seas. Prog. Oceanogr. 130, 19–31. doi:10.1016/j.pocean.2014.09.003
Chila, Z., Dunmall, K. M., Proverbs, T. A., Lantz, T. C., Hunters, A., Committee, T., et al. (2022). Inuvialuit knowledge of Pacific salmon range expansion in the western Canadian Arctic. Can. J. Fish. Aquat. Sci. 79, 1042–1055. doi:10.1139/cjfas-2021-0172
Christiansen, J. S., Bonsdorff, E., Byrkjedal, I., Fevolden, S.-E., Karamushko, O. V., Lynghammar, A., et al. (2016). Novel biodiversity baselines outpace models of fish distribution in Arctic waters. Sci. Nat. 103, 8. doi:10.1007/s00114-016-1332-9
Christiansen, J. S., Mecklenburg, C. W., and Karamushko, O. V. (2014). Arctic marine fishes and their fisheries in light of global change. Glob. Change Biol. 20, 352–359. doi:10.1111/gcb.12395
Coello-Camba, A., Agustí, S., Holding, J., Arrieta, J. M., and Duarte, C. M. (2014). Interactive effect of temperature and CO2 increase in Arctic phytoplankton. Front. Mar. Sci. 1. doi:10.3389/fmars.2014.00049
Colatriano, D., Tran, P. Q., Guéguen, C., Williams, W. J., Lovejoy, C., and Walsh, D. A. (2018). Genomic evidence for the degradation of terrestrial organic matter by pelagic Arctic Ocean Chloroflexi bacteria. Commun. Biol. 1, 90–99. doi:10.1038/s42003-018-0086-7
Comeau, A. M., Li, W. K. W., Tremblay, J.-É., Carmack, E. C., and Lovejoy, C. (2011). Arctic Ocean microbial community structure before and after the 2007 record sea ice minimum. PLOS ONE 6, e27492. doi:10.1371/journal.pone.0027492
Comeau, S., Gorsky, G., Jeffree, R., Teyssié, J.-L., and Gattuso, J.-P. (2009). Impact of ocean acidification on a key Arctic pelagic mollusc (Limacina helicina). Biogeosciences 6, 1877–1882. doi:10.5194/bg-6-1877-2009
Constantin, A., and Johnson, R. S. (2023). On the dynamics of the near-surface currents in the Arctic Ocean. Nonlinear Anal. Real World Appl. 73, 103894. doi:10.1016/j.nonrwa.2023.103894
Copeman, L. A., Salant, C. D., Stowell, M. A., Spencer, M. L., Kimmel, D. G., Pinchuk, A. I., et al. (2022). Annual and spatial variation in the condition and lipid storage of juvenile Chukchi Sea gadids during a recent period of environmental warming (2012 to 2019). Deep Sea Res. Part II Top. Stud. Oceanogr. 205, 105180. doi:10.1016/j.dsr2.2022.105180
Csapó, H. K., Grabowski, M., and Węsławski, J. M. (2021). Coming home - boreal ecosystem claims Atlantic sector of the Arctic. Sci. Total Environ. 771, 144817. doi:10.1016/j.scitotenv.2020.144817
Daase, M., Søreide, J. E., and Martynova, D. (2011). Effects of food quality on naupliar development in Calanus glacialis at subzero temperatures. Mar. Ecol. Prog. Ser. 429, 111–124. doi:10.3354/meps09075
Dahlke, F. T., Wohlrab, S., Butzin, M., and Pörtner, H.-O. (2020). Thermal bottlenecks in the life cycle define climate vulnerability of fish. Science 369, 65–70. doi:10.1126/science.aaz3658
Dalpadado, P., Arrigo, K. R., Hjøllo, S. S., Rey, F., Ingvaldsen, R. B., Sperfeld, E., et al. (2014). Productivity in the Barents Sea - response to recent climate variability. PLOS ONE 9, e95273. doi:10.1371/journal.pone.0095273
Dalpadado, P., Arrigo, K. R., van Dijken, G. L., Skjoldal, H. R., Bagøien, E., Dolgov, A. V., et al. (2020). Climate effects on temporal and spatial dynamics of phytoplankton and zooplankton in the Barents Sea. Prog. Oceanogr. 185, 102320. doi:10.1016/j.pocean.2020.102320
Dalpadado, P., Ingvaldsen, R., and Hassel, A. (2003). Zooplankton biomass variation in relation to climatic conditions in the Barents Sea. Polar Biol. 26, 233–241. doi:10.1007/s00300-002-0470-z
Dalpadado, P., Prokopchuk, I. P., Bogstad, B., Skaret, G., Ingvaldsen, R. B., Dolgov, A. V., et al. (2024). Zooplankton link climate to capelin and polar cod in the Barents Sea. Prog. Oceanogr. 226, 103302. doi:10.1016/j.pocean.2024.103302
Danielson, S. L., Ahkinga, O., Ashjian, C., Basyuk, E., Cooper, L. W., Eisner, L., et al. (2020). Manifestation and consequences of warming and altered heat fluxes over the Bering and Chukchi Sea continental shelves. Deep Sea Res. Part II Top. Stud. Oceanogr. 177, 104781. doi:10.1016/j.dsr2.2020.104781
Danielson, S. L., Eisner, L., Ladd, C., Mordy, C., Sousa, L., and Weingartner, T. J. (2017). A comparison between late summer 2012 and 2013 water masses, macronutrients, and phytoplankton standing crops in the northern Bering and Chukchi Seas. Deep Sea Res. Part II Top. Stud. Oceanogr. 135, 7–26. doi:10.1016/j.dsr2.2016.05.024
Darnis, G., Geoffroy, M., Dezutter, T., Aubry, C., Massicotte, P., Brown, T., et al. (2022). Zooplankton assemblages along the North American arctic: ecological connectivity shaped by ocean circulation and bathymetry from the Chukchi Sea to labrador sea. Elem. Sci. Anthropocene 10, 00053. doi:10.1525/elementa.2022.00053
David, C., Lange, B., Krumpen, T., Schaafsma, F., van Franeker, J. A., and Flores, H. (2016). Under-ice distribution of polar cod Boreogadus saida in the central Arctic Ocean and their association with sea-ice habitat properties. Polar Biol. 39, 981–994. doi:10.1007/s00300-015-1774-0
Deary, A. L., Vestfals, C. D., Mueter, F. J., Logerwell, E. A., Goldstein, E. D., Stabeno, P. J., et al. (2021). Seasonal abundance, distribution, and growth of the early life stages of polar cod (Boreogadus saida) and saffron cod (Eleginus gracilis) in the US Arctic. Polar Biol. 44, 2055–2076. doi:10.1007/s00300-021-02940-2
Decker, M. B., Brodeur, R. D., Ciannelli, L., Britt, L. L., Bond, N. A., DiFiore, B. P., et al. (2023). Cyclic variability of eastern Bering Sea jellyfish relates to regional physical conditions. Prog. Oceanogr. 210, 102923. doi:10.1016/j.pocean.2022.102923
de la Vega, C., Mahaffey, C., Yurkowski, D. J., Norman, L., Simpson, E., Smout, S., et al. (2021). Biomarkers in ringed seals reveal recent onset of borealization in the high- compared to the mid-latitude Canadian arctic. Front. Mar. Sci. 8. Available at:. doi:10.3389/fmars.2021.700687
Demidov, A. B., Sukhanova, I. N., Belevich, T. A., Flint, M. V., Gagarin, V. I., Sergeeva, V. M., et al. (2021). Size-fractionated surface phytoplankton in the Kara and Laptev Seas: environmental control and spatial variability. Mar. Ecol. Prog. Ser. 664, 59–77. doi:10.3354/meps13652
Descamps, S., and Strøm, H. (2021). As the Arctic becomes boreal: ongoing shifts in a high-Arctic seabird community. Ecology 102, e03485. doi:10.1002/ecy.3485
Descamps, S., Wojczulanis-Jakubas, K., Jakubas, D., Vihtakari, M., Steen, H., Karnovsky, N. J., et al. (2022). Consequences of atlantification on a zooplanktivorous arctic seabird. Front. Mar. Sci. 9. doi:10.3389/fmars.2022.878746
Descôteaux, R., Huserbråten, M., Jørgensen, L. L., Renaud, P. E., Ingvaldsen, R. B., Ershova, E. A., et al. (2022). Origin of marine invertebrate larvae on an Arctic inflow shelf. Mar. Ecol. Prog. Ser. 699, 1–17. doi:10.3354/meps14170
Dittmar, T., and Kattner, G. (2003). The biogeochemistry of the river and shelf ecosystem of the Arctic Ocean: a review. Mar. Chem. 83, 103–120. doi:10.1016/S0304-4203(03)00105-1
Donelson, J. M., Sunday, J. M., Figueira, W. F., Gaitán-Espitia, J. D., Hobday, A. J., Johnson, C. R., et al. (2019). Understanding interactions between plasticity, adaptation and range shifts in response to marine environmental change. Philosophical Trans. R. Soc. B Biol. Sci. 374, 20180186. doi:10.1098/rstb.2018.0186
Dörr, J., Årthun, M., Eldevik, T., and Madonna, E. (2021). Mechanisms of regional winter sea-ice variability in a warming arctic. J. Clim. 34, 8635–8653. doi:10.1175/JCLI-D-21-0149.1
Dörr, J., Årthun, M., Eldevik, T., and Sandø, A. B. (2024). Expanding influence of atlantic and pacific ocean heat transport on winter sea-ice variability in a warming arctic. J. Geophys. Res. Oceans 129, e2023JC019900. doi:10.1029/2023JC019900
Dorrell, R. G., Kuo, A., Füssy, Z., Richardson, E. H., Salamov, A., Zarevski, N., et al. (2023). Convergent evolution and horizontal gene transfer in Arctic Ocean microalgae. Life Sci. Alliance 6, e202201833. doi:10.26508/lsa.202201833
Drinkwater, K. F., Harada, N., Nishino, S., Chierici, M., Danielson, S. L., Ingvaldsen, R. B., et al. (2021). Possible future scenarios for two major Arctic Gateways connecting Subarctic and Arctic marine systems: I. Climate and physical–chemical oceanography. ICES J. Mar. Sci. 78, 3046–3065. doi:10.1093/icesjms/fsab182
Drits, A., Pasternak, A., Arashkevich, E., Amelina, A., and Flint, M. (2023). Timing of ice retreat determines summer state of zooplankton community in the ob estuary (the Kara Sea, siberian arctic). Diversity 15, 674. doi:10.3390/d15050674
Drost, H. E., Carmack, E. C., and Farrell, A. P. (2014). Upper thermal limits of cardiac function for Arctic cod Boreogadus saida, a key food web fish species in the Arctic Ocean. J. Fish Biol. 84, 1781–1792. doi:10.1111/jfb.12397
Drost, H. E., Lo, M., Carmack, E. C., and Farrell, A. P. (2016). Acclimation potential of Arctic cod (Boreogadus saida) from the rapidly warming Arctic Ocean. J. Exp. Biol. 219, 3114–3125. doi:10.1242/jeb.140194
Duarte, P., Meyer, A., and Moreau, S. (2021). Nutrients in water masses in the atlantic sector of the Arctic Ocean: temporal trends, mixing and links with primary production. J. Geophys. Res. Oceans 126, e2021JC017413. doi:10.1029/2021JC017413
Dulvy, N. K., Rogers, S. I., Jennings, S., Stelzenmüller, V., Dye, S. R., and Skjoldal, H. R. (2008). Climate change and deepening of the North Sea fish assemblage: a biotic indicator of warming seas. J. Appl. Ecol. 45, 1029–1039. doi:10.1111/j.1365-2664.2008.01488.x
Dunmall, K. M., Langan, J. A., Cunningham, C. J., Reist, J. D., Melling, H., Hunters, A., et al. (2024). Pacific salmon in the Canadian Arctic highlight a range-expansion pathway for sub-Arctic fishes. Glob. Change Biol. 30, e17353. doi:10.1111/gcb.17353
Dunton, K. H., Weingartner, T., and Carmack, E. C. (2006). The nearshore western Beaufort Sea ecosystem: circulation and importance of terrestrial carbon in arctic coastal food webs. Prog. Oceanogr. 71, 362–378. doi:10.1016/j.pocean.2006.09.011
Dupont, N., Durant, J. M., Langangen, Ø., Gjøsæter, H., and Stige, L. C. (2020). Sea ice, temperature, and prey effects on annual variations in mean lengths of a key Arctic fish, Boreogadus saida, in the Barents Sea. ICES J. Mar. Sci. 77, 1796–1805. doi:10.1093/icesjms/fsaa040
Dvoretsky, A. G., and Dvoretsky, V. G. (2024). Filling knowledge gaps in arctic marine biodiversity: environment, plankton, and benthos of Franz Josef land, Barents Sea. Ocean and Coast. Manag. 249, 106987. doi:10.1016/j.ocecoaman.2023.106987
Eerkes-Medrano, L., and Huntington, H. P. (2021). Untold stories: indigenous knowledge beyond the changing arctic cryosphere. Front. Clim. 3. doi:10.3389/fclim.2021.675805
Einarsson, S. V., Lowry, K. E., Lin, P., Pickart, R. S., Ashjian, C. J., and Chappell, P. D. (2022). Alexandrium on the alaskan Beaufort Sea shelf: impact of upwelling in a warming arctic. Harmful Algae 120, 102346. doi:10.1016/j.hal.2022.102346
Eisner, L. B., Napp, J. M., Mier, K. L., Pinchuk, A. I., and Andrews, A. G. (2014). Climate-mediated changes in zooplankton community structure for the eastern Bering Sea. Deep Sea Res. Part II Top. Stud. Oceanogr. 109, 157–171. doi:10.1016/j.dsr2.2014.03.004
Emblemsvåg, M., Pecuchet, L., Velle, L. G., Nogueira, A., and Primicerio, R. (2022a). Recent warming causes functional borealization and diversity loss in deep fish communities east of Greenland. Divers. Distributions 28, 2071–2083. doi:10.1111/ddi.13604
Emblemsvåg, M., Werner, K. M., Núñez-Riboni, I., Frelat, R., Torp Christensen, H., Fock, H. O., et al. (2022b). Deep demersal fish communities respond rapidly to warming in a frontal region between Arctic and Atlantic waters. Glob. Change Biol. 28, 2979–2990. doi:10.1111/gcb.16113
Emelin, P. O., Maznikova, O. A., Benzik, A. N., Sheibak, A.Yu., Trofimova, A. O., and Orlov, A. M. (2022). Invader’s portrait: biological characteristics of walleye pollock Gadus chalcogrammus in the western Chukchi Sea. Deep Sea Res. Part II Top. Stud. Oceanogr. 206, 105211. doi:10.1016/j.dsr2.2022.105211
Endo, C. A. K., Skogen, M. D., Stige, L. C., Hjøllo, S. S., and Vikebø, F. B. (2024). The effects of spatial and temporal variations in spawning on offspring survival in Northeast Arctic cod. ICES J. Mar. Sci. 81, 616–626. doi:10.1093/icesjms/fsad034
Engelhard, G. H., Ellis, J. R., Payne, M. R., ter Hofstede, R., and Pinnegar, J. K. (2011). Ecotypes as a concept for exploring responses to climate change in fish assemblages. ICES J. Mar. Sci. 68, 580–591. doi:10.1093/icesjms/fsq183
Eriksen, E. (2016). Do scyphozoan jellyfish limit the habitat of pelagic species in the Barents Sea during the late feeding period? ICES J. Mar. Sci. 73, 217–226. doi:10.1093/icesjms/fsv183
Eriksen, E., Bagøien, E., Strand, E., Primicerio, R., Prokhorova, T., Trofimov, A., et al. (2020). The record-warm Barents Sea and 0-group fish response to abnormal conditions. Front. Mar. Sci. 7. doi:10.3389/fmars.2020.00338
Eriksen, E., Bogstad, B., Dolgov, A., and Beck, I. M. (2018). Cod diet as an indicator of Ctenophora abundance dynamics in the Barents Sea. Mar. Ecol. Prog. Ser. 591, 87–100. doi:10.3354/meps12199
Eriksen, E., and Dalpadado, P. (2011). Long-term changes in Krill biomass and distribution in the Barents Sea: are the changes mainly related to capelin stock size and temperature conditions? Polar Biol. 34, 1399–1409. doi:10.1007/s00300-011-0995-0
Eriksen, E., Ingvaldsen, R. B., Nedreaas, K., and Prozorkevich, D. (2015). The effect of recent warming on polar cod and beaked redfish juveniles in the Barents Sea. Regional Stud. Mar. Sci. 2, 105–112. doi:10.1016/j.rsma.2015.09.001
Eriksen, E., Skjoldal, H. R., Gjøsæter, H., and Primicerio, R. (2017). Spatial and temporal changes in the Barents Sea pelagic compartment during the recent warming. Prog. Oceanogr. 151, 206–226. doi:10.1016/j.pocean.2016.12.009
Ershova, E. A., Descoteaux, R., Wangensteen, O. S., Iken, K., Hopcroft, R. R., Smoot, C., et al. (2019). Diversity and distribution of meroplanktonic larvae in the pacific arctic and connectivity with adult benthic invertebrate communities. Front. Mar. Sci. 6. doi:10.3389/fmars.2019.00490
Ershova, E. A., Hopcroft, R. R., Kosobokova, K. N., Matsuno, K., Nelson, R. J., Yamaguchi, A., et al. (2015). Long-term changes in summer zooplankton communities of the western Chukchi Sea, 1945–2012. Oceanography 28, 100–115. doi:10.5670/oceanog.2015.60
Ershova, E. A., Kosobokova, K. N., Banas, N. S., Ellingsen, I., Niehoff, B., Hildebrandt, N., et al. (2021). Sea ice decline drives biogeographical shifts of key Calanus species in the central Arctic Ocean. Glob. Change Biol. 27, 2128–2143. doi:10.1111/gcb.15562
Fachon, E., Pickart, R. S., Sheffield, G., Pate, E., Pathare, M., Brosnahan, M. L., et al. (2024). Tracking a large-scale and highly toxic Arctic algal bloom: rapid detection and risk communication. Limnol. Oceanogr. Lett. doi:10.1002/lol2.10421
Falardeau, M., and Bennett, E. M. (2020). Towards integrated knowledge of climate change in Arctic marine systems: a systematic literature review of multidisciplinary research. Arct. Sci. 6, 1–23. doi:10.1139/as-2019-0006
Falardeau, M., Bennett, E. M., Else, B., Fisk, A., Mundy, C. J., Choy, E. S., et al. (2022). Biophysical indicators and Indigenous and Local Knowledge reveal climatic and ecological shifts with implications for Arctic Char fisheries. Glob. Environ. Change 74, 102469. doi:10.1016/j.gloenvcha.2022.102469
Falardeau, M., Bouchard, C., Robert, D., and Fortier, L. (2017). First records of pacific sand lance (ammodytes hexapterus) in the Canadian arctic Archipelago. Polar Biol. 40, 2291–2296. doi:10.1007/s00300-017-2141-0
Falardeau, M., Robert, D., and Fortier, L. (2014). Could the planktonic stages of polar cod and Pacific sand lance compete for food in the warming Beaufort Sea? ICES J. Mar. Sci. 71, 1956–1965. doi:10.1093/icesjms/fst221
Fang, C., Zheng, R., Zhang, Y., Hong, F., Mu, J., Chen, M., et al. (2018). Microplastic contamination in benthic organisms from the Arctic and sub-Arctic regions. Chemosphere 209, 298–306. doi:10.1016/j.chemosphere.2018.06.101
Ferguson, S. H., Higdon, J. W., and Chmelnitsky, E. G. (2010). “The rise of killer whales as a major arctic predator,” in A little less arctic: top predators in the world’s largest northern inland sea Editors S. H. Ferguson, L. L. Loseto, and M. L. Mallory Hudson Bay (Dordrecht: Springer Netherlands), 117–136. doi:10.1007/978-90-481-9121-5_6
Florko, K. R. N., Tai, T. C., Cheung, W. W. L., Ferguson, S. H., Sumaila, U. R., Yurkowski, D. J., et al. (2021). Predicting how climate change threatens the prey base of Arctic marine predators. Ecol. Lett. 24, 2563–2575. doi:10.1111/ele.13866
Fossheim, M., Primicerio, R., Johannesen, E., Ingvaldsen, R. B., Aschan, M. M., and Dolgov, A. V. (2015). Recent warming leads to a rapid borealization of fish communities in the Arctic. Nat. Clim. Change 5, 673–677. doi:10.1038/nclimate2647
Fox, R. J., Donelson, J. M., Schunter, C., Ravasi, T., and Gaitán-Espitia, J. D. (2019). Beyond buying time: the role of plasticity in phenotypic adaptation to rapid environmental change. Philosophical Trans. R. Soc. B Biol. Sci. 374, 20180174. doi:10.1098/rstb.2018.0174
Frainer, A., Primicerio, R., Dolgov, A., Fossheim, M., Johannesen, E., Lind, S., et al. (2021). Increased functional diversity warns of ecological transition in the Arctic. Proc. R. Soc. B Biol. Sci. 288, 20210054. doi:10.1098/rspb.2021.0054
Frainer, A., Primicerio, R., Kortsch, S., Aune, M., Dolgov, A. V., Fossheim, M., et al. (2017). Climate-driven changes in functional biogeography of Arctic marine fish communities. PNAS 114, 12202–12207. doi:10.1073/pnas.1706080114
Freer, J. J., Daase, M., and Tarling, G. A. (2022). Modelling the biogeographic boundary shift of Calanus finmarchicus reveals drivers of Arctic Atlantification by subarctic zooplankton. Glob. Change Biol. 28, 429–440. doi:10.1111/gcb.15937
Frey, K. E., Moore, G. W. K., Cooper, L. W., and Grebmeier, J. M. (2015). Divergent patterns of recent sea ice cover across the bering, Chukchi, and Beaufort seas of the pacific arctic region. Prog. Oceanogr. 136, 32–49. doi:10.1016/j.pocean.2015.05.009
Freyria, N. J., Joli, N., and Lovejoy, C. (2021). A decadal perspective on north water microbial eukaryotes as Arctic Ocean sentinels. Sci. Rep. 11, 8413. doi:10.1038/s41598-021-87906-4
Gall, A. E., Morgan, T. C., Day, R. H., and Kuletz, K. J. (2017). Ecological shift from piscivorous to planktivorous seabirds in the Chukchi Sea, 1975–2012. Polar Biol. 40, 61–78. doi:10.1007/s00300-016-1924-z
Gaston, A. J., and Elliott, K. H. (2014). Seabird diet changes in northern Hudson Bay, 1981-2013, reflect the availability of schooling prey. Mar. Ecol. Prog. Ser. 513, 211–223. doi:10.3354/meps10945
Gaston, A. J., Woo, K., and Hipfner, J. M. (2003). Trends in forage fish populations in northern Hudson Bay since 1981, as determined from the diet of nestling thick-billed murres Uria lomvia. ARCTIC 56, 227–233. doi:10.14430/arctic618
Geoffroy, M., Berge, J., Majaneva, S., Johnsen, G., Langbehn, T. J., Cottier, F., et al. (2018). Increased occurrence of the jellyfish Periphylla periphylla in the European high Arctic. Polar Biol. 41, 2615–2619. doi:10.1007/s00300-018-2368-4
Geoffroy, M., Bouchard, C., Flores, H., Robert, D., Gjøsæter, H., Hoover, C., et al. (2023). The circumpolar impacts of climate change and anthropogenic stressors on Arctic cod (Boreogadus saida) and its ecosystem. Elem. Sci. Anthropocene 11, 00097. doi:10.1525/elementa.2022.00097
Geoffroy, M., Daase, M., Cusa, M., Darnis, G., Graeve, M., Santana Hernández, N., et al. (2019). Mesopelagic sound scattering layers of the high arctic: seasonal variations in biomass, species assemblage, and trophic relationships. Front. Mar. Sci. 6. doi:10.3389/fmars.2019.00364
Geoffroy, M., and Priou, P. (2020). “Fish ecology during the polar night,” in POLAR NIGHT marine ecology: life and light in the dead of night. Editors J. Berge, G. Johnsen, and J. H. Cohen (Cham: Springer International Publishing), 181–216. doi:10.1007/978-3-030-33208-2_7
Gerland, S., Barber, D., Meier, W., Mundy, C. J., Holland, M., Kern, S., et al. (2019). Essential gaps and uncertainties in the understanding of the roles and functions of Arctic sea ice. Environ. Res. Lett. 14, 043002. doi:10.1088/1748-9326/ab09b3
Gjøsæter, H., Huserbråten, M., Vikebø, F., and Eriksen, E. (2020). Key processes regulating the early life history of Barents Sea polar cod. Polar Biol. 43, 1015–1027. doi:10.1007/s00300-020-02656-9
Gluchowska, M., Dalpadado, P., Beszczynska-Möller, A., Olszewska, A., Ingvaldsen, R. B., and Kwasniewski, S. (2017). Interannual zooplankton variability in the main pathways of the atlantic water flow into the Arctic Ocean (Fram Strait and Barents Sea branches). ICES J. Mar. Sci. 74, 1921–1936. doi:10.1093/icesjms/fsx033
Goethel, C. L., Grebmeier, J. M., Cooper, L. W., and Miller, T. J. (2017). Implications of ocean acidification in the Pacific Arctic: experimental responses of three Arctic bivalves to decreased pH and food availability. Deep Sea Res. Part II Top. Stud. Oceanogr. 144, 112–124. doi:10.1016/j.dsr2.2017.08.013
Grebmeier, J. M. (2012). Shifting patterns of life in the pacific arctic and sub-arctic seas. Annu. Rev. Mar. Sci. 4, 63–78. doi:10.1146/annurev-marine-120710-100926
Grebmeier, J. M., Bluhm, B. A., Cooper, L. W., Danielson, S. L., Arrigo, K. R., Blanchard, A. L., et al. (2015a). Ecosystem characteristics and processes facilitating persistent macrobenthic biomass hotspots and associated benthivory in the Pacific Arctic. Prog. Oceanogr. 136, 92–114. doi:10.1016/j.pocean.2015.05.006
Grebmeier, J. M., Bluhm, B. A., Cooper, L. W., Denisenko, S. G., Iken, K., Kędra, M., et al. (2015b). Time-series benthic community composition and biomass and associated environmental characteristics in the Chukchi Sea during the RUSALCA 2004–2012 program. Oceanography 28, 116–133. doi:10.5670/oceanog.2015.61
Grebmeier, J. M., Frey, K. E., Cooper, L. W., and Kędra, M. (2018). Trends in benthic macrofaunal populations, seasonal sea ice persistence, and bottom water temperatures in the Bering Strait region. Oceanography 31, 136–151. doi:10.5670/oceanog.2018.224
Grebmeier, J. M., Overland, J. E., Moore, S. E., Farley, E. V., Carmack, E. C., Cooper, L. W., et al. (2006). A major ecosystem shift in the northern Bering Sea. Science 311, 1461–1464. doi:10.1126/science.1121365
Greene, C. H., Pershing, A. J., Cronin, T. M., and Ceci, N. (2008). Arctic climate change and its impacts on the ecology of the North atlantic. Ecology 89, S24–S38. doi:10.1890/07-0550.1
Hansen, M. L. S., Piepenburg, D., Pantiukhin, D., and Kraan, C. (2020). Unraveling the effects of environmental drivers and spatial structure on benthic species distribution patterns in Eurasian-Arctic seas (Barents, Kara and Laptev Seas). Polar Biol. 43, 1693–1705. doi:10.1007/s00300-020-02737-9
Haug, T., Bogstad, B., Chierici, M., Gjøsæter, H., Hallfredsson, E. H., Høines, Å. S., et al. (2017). Future harvest of living resources in the Arctic Ocean north of the Nordic and Barents Seas: a review of possibilities and constraints. Fish. Res. 188, 38–57. doi:10.1016/j.fishres.2016.12.002
Heide-Jørgensen, M. P., Chambault, P., Jansen, T., Gjelstrup, C. V. B., Rosing-Asvid, A., Macrander, A., et al. (2023). A regime shift in the Southeast Greenland marine ecosystem. Glob. Change Biol. 29, 668–685. doi:10.1111/gcb.16494
Heintz, R. A., Siddon, E. C., Farley, E. V., and Napp, J. M. (2013). Correlation between recruitment and fall condition of age-0 pollock (Theragra chalcogramma) from the eastern Bering Sea under varying climate conditions. Deep Sea Res. Part II Top. Stud. Oceanogr. 94, 150–156. doi:10.1016/j.dsr2.2013.04.006
Hendrix, A. M., Lefebvre, K. A., Quakenbush, L., Bryan, A., Stimmelmayr, R., Sheffield, G., et al. (2021). Ice seals as sentinels for algal toxin presence in the Pacific Arctic and subarctic marine ecosystems. Mar. Mammal Sci. 37, 1292–1308. doi:10.1111/mms.12822
Henley, S. F., Porter, M., Hobbs, L., Braun, J., Guillaume-Castel, R., Venables, E. J., et al. (2020). Nitrate supply and uptake in the Atlantic Arctic sea ice zone: seasonal cycle, mechanisms and drivers. Philosophical Trans. R. Soc. A Math. Phys. Eng. Sci. 378, 20190361. doi:10.1098/rsta.2019.0361
Hill, V., Ardyna, M., Lee, S. H., and Varela, D. E. (2018). Decadal trends in phytoplankton production in the pacific arctic region from 1950 to 2012. Deep Sea Res. Part II Top. Stud. Oceanogr. 152, 82–94. doi:10.1016/j.dsr2.2016.12.015
Hill, V. J., Matrai, P. A., Olson, E., Suttles, S., Steele, M., Codispoti, L. A., et al. (2013). Synthesis of integrated primary production in the Arctic Ocean: II. in situ and remotely sensed estimates. Prog. Oceanogr. 110, 107–125. doi:10.1016/j.pocean.2012.11.005
Hinzman, L. D., Bettez, N. D., Bolton, W. R., Chapin, F. S., Dyurgerov, M. B., Fastie, C. L., et al. (2005). Evidence and implications of recent climate change in northern Alaska and other arctic regions. Clim. Change 72, 251–298. doi:10.1007/s10584-005-5352-2
Hirche, H., Ershova, E., Kosobokova, K., and Hopcroft, R. (2024). From fringe to basin: unravelling the survival strategies of Calanus hyperboreus and C. glacialis in the Arctic Ocean. Mar. Ecol. Prog. Ser. 745, 41–57. doi:10.3354/meps14665
Hirche, H. J., Kosobokova, K. N., Gaye-Haake, B., Harms, I., Meon, B., and Nöthig, E.-M. (2006). Structure and function of contemporary food webs on Arctic shelves: a panarctic comparison. Prog. Oceanogr. 71, 288–313. doi:10.1016/j.pocean.2006.09.010
Höffle, H., Solemdal, P., Korsbrekke, K., Johannessen, M., Bakkeplass, K., and Kjesbu, O. S. (2014). Variability of northeast Arctic cod (Gadus morhua) distribution on the main spawning grounds in relation to biophysical factors. ICES J. Mar. Sci. 71, 1317–1331. doi:10.1093/icesjms/fsu126
Hoffmann, A. A., and Parsons, P. A. (1990). Evolutionary genetics and environmental stress. Oxford University Press. Available at: https://academic.oup.com/book/52930 (Accessed July 4, 2024).
Hoffmann, A. A., and Sgrò, C. M. (2011). Climate change and evolutionary adaptation. Nature 470, 479–485. doi:10.1038/nature09670
Hofstede, R. ter, Hiddink, J. G., and Rijnsdorp, A. D. (2010). Regional warming changes fish species richness in the eastern North Atlantic Ocean. Mar. Ecol. Prog. Ser. 414, 1–9. doi:10.3354/meps08753
Hollowed, A. B., Barange, M., Beamish, R. J., Brander, K., Cochrane, K., Drinkwater, K., et al. (2013a). Projected impacts of climate change on marine fish and fisheries. ICES J. Mar. Sci. 70, 1023–1037. doi:10.1093/icesjms/fst081
Hollowed, A. B., Planque, B., and Loeng, H. (2013b). Potential movement of fish and shellfish stocks from the sub-Arctic to the Arctic Ocean. Fish. Oceanogr. 22, 355–370. doi:10.1111/fog.12027
Holt, R. E., Bogstad, B., Durant, J. M., Dolgov, A. V., and Ottersen, G. (2019). Barents Sea cod (Gadus morhua) diet composition: long-term interannual, seasonal, and ontogenetic patterns. ICES J. Mar. Sci. 76, 1641–1652. doi:10.1093/icesjms/fsz082
Holt, R. E., Hvingel, C., Agnalt, A.-L., Dolgov, A. V., Hjelset, A. M., and Bogstad, B. (2021). Snow crab (Chionoecetes opilio), a new food item for North-east Arctic cod (Gadus morhua) in the Barents Sea. ICES J. Mar. Sci. 78, 491–501. doi:10.1093/icesjms/fsaa168
Hop, H., Falk-Petersen, S., Svendsen, H., Kwasniewski, S., Pavlov, V., Pavlova, O., et al. (2006). Physical and biological characteristics of the pelagic system across Fram Strait to Kongsfjorden. Prog. Oceanogr. 71, 182–231. doi:10.1016/j.pocean.2006.09.007
Hop, H., and Gjøsæter, H. (2013). Polar cod (Boreogadus saida) and capelin (Mallotus villosus) as key species in marine food webs of the Arctic and the Barents Sea. Mar. Biol. Res. 9, 878–894. doi:10.1080/17451000.2013.775458
Hop, H., Wold, A., Meyer, A., Bailey, A., Hatlebakk, M., Kwasniewski, S., et al. (2021). Winter-spring development of the zooplankton community below sea ice in the Arctic Ocean. Front. Mar. Sci. 8. doi:10.3389/fmars.2021.609480
Huang, J., Zhang, X., Zhang, Q., Lin, Y., Hao, M., Luo, Y., et al. (2017). Recently amplified arctic warming has contributed to a continual global warming trend. Nat. Clim. Change 7, 875–879. doi:10.1038/s41558-017-0009-5
Hunt, B. P. V., Nelson, R. J., Williams, B., McLaughlin, F. A., Young, K. V., Brown, K. A., et al. (2014). Zooplankton community structure and dynamics in the Arctic Canada Basin during a period of intense environmental change (2004–2009). J. Geophys. Res. Oceans 119, 2518–2538. doi:10.1002/2013JC009156
Hunt, G. L., Ressler, P. H., Gibson, G. A., De Robertis, A., Aydin, K., Sigler, M. F., et al. (2016). Euphausiids in the eastern Bering Sea: a synthesis of recent studies of euphausiid production, consumption and population control. Deep Sea Res. Part II Top. Stud. Oceanogr. 134, 204–222. doi:10.1016/j.dsr2.2015.10.007
Huntington, H. P., Danielson, S. L., Wiese, F. K., Baker, M., Boveng, P., Citta, J. J., et al. (2020). Evidence suggests potential transformation of the Pacific Arctic ecosystem is underway. Nat. Clim. Change 10, 342–348. doi:10.1038/s41558-020-0695-2
Huse, G., and Ellingsen, I. (2008). Capelin migrations and climate change–a modelling analysis. Clim. Change 87, 177–197. doi:10.1007/s10584-007-9347-z
Huserbråten, M. B. O., Eriksen, E., Gjøsæter, H., and Vikebø, F. (2019). Polar cod in jeopardy under the retreating Arctic sea ice. Commun. Biol. 2, 407–408. doi:10.1038/s42003-019-0649-2
Hussey, N. E., Hedges, K. J., Barkley, A. N., Treble, M. A., Peklova, I., Webber, D. M., et al. (2017). Movements of a deep-water fish: establishing marine fisheries management boundaries in coastal Arctic waters. Ecol. Appl. 27, 687–704. doi:10.1002/eap.1485
Husson, B., Certain, G., Filin, A., and Planque, B. (2020). Suitable habitats of fish species in the Barents Sea. Fish. Oceanogr. 29, 526–540. doi:10.1111/fog.12493
Ibarbalz, F. M., Henry, N., Mahé, F., Ardyna, M., Zingone, A., Scalco, E., et al. (2023). Pan-Arctic plankton community structure and its global connectivity. Elem. Sci. Anthropocene 11, 00060. doi:10.1525/elementa.2022.00060
Ingvaldsen, R. B., Assmann, K. M., Primicerio, R., Fossheim, M., Polyakov, I. V., and Dolgov, A. V. (2021). Physical manifestations and ecological implications of Arctic Atlantification. Nat. Rev. Earth Environ. 2, 874–889. doi:10.1038/s43017-021-00228-x
Ingvaldsen, R. B., Eriksen, E., Gjøsæter, H., Engås, A., Schuppe, B. K., Assmann, K. M., et al. (2023). Under-ice observations by trawls and multi-frequency acoustics in the Central Arctic Ocean reveals abundance and composition of pelagic fauna. Sci. Rep. 13, 1000. doi:10.1038/s41598-023-27957-x
Irrgang, A. M., Bendixen, M., Farquharson, L. M., Baranskaya, A. V., Erikson, L. H., Gibbs, A. E., et al. (2022). Drivers, dynamics and impacts of changing Arctic coasts. Nat. Rev. Earth Environ. 3, 39–54. doi:10.1038/s43017-021-00232-1
Isaksen, K., Nordli, Ø., Ivanov, B., Køltzow, M. A. Ø., Aaboe, S., Gjelten, H. M., et al. (2022). Exceptional warming over the Barents area. Sci. Rep. 12, 9371. doi:10.1038/s41598-022-13568-5
Ivanov, V., Alexeev, V., Koldunov, N. V., Repina, I., Sandø, A. B., Smedsrud, L. H., et al. (2016). Arctic Ocean heat impact on regional ice decay: a suggested positive feedback. J. Phys. Oceanogr. 46, 1437–1456. doi:10.1175/JPO-D-15-0144.1
Jordà-Molina, È., Renaud, P. E., Silberberger, M. J., Sen, A., Bluhm, B. A., Carroll, M. L., et al. (2023). Seafloor warm water temperature anomalies impact benthic macrofauna communities of a high-Arctic cold-water fjord. Mar. Environ. Res. 189, 106046. doi:10.1016/j.marenvres.2023.106046
Jordán, F., Capelli, G., Primicerio, R., Hidas, A., Fábián, V., Patonai, K., et al. (2024). Spatial food webs in the Barents Sea: atlantification and the reorganization of the trophic structure. Philosophical Trans. R. Soc. B Biol. Sci. 379, 20230164. doi:10.1098/rstb.2023.0164
Jørgensen, L. L., Logerwell, E. A., Strelkova, N., Zakharov, D., Roy, V., Nozères, C., et al. (2022). International megabenthic long-term monitoring of a changing arctic ecosystem: baseline results. Prog. Oceanogr. 200, 102712. doi:10.1016/j.pocean.2021.102712
Jørgensen, L. L., Primicerio, R., Ingvaldsen, R. B., Fossheim, M., Strelkova, N., Thangstad, T. H., et al. (2019). Impact of multiple stressors on sea bed fauna in a warming Arctic. Mar. Ecol. Prog. Ser. 608, 1–12. doi:10.3354/meps12803
Jueterbock, A., Tyberghein, L., Verbruggen, H., Coyer, J. A., Olsen, J. L., and Hoarau, G. (2013). Climate change impact on seaweed meadow distribution in the North Atlantic rocky intertidal. Ecol. Evol. 3, 1356–1373. doi:10.1002/ece3.541
Juranek, L. W. (2022). Changing biogeochemistry of the Arctic Ocean: surface nutrient and Co₂ cycling in a warming, melting north. Oceanography 35, 144–155. doi:10.5670/oceanog.2022.120
Kaartvedt, S. (2008). Photoperiod may constrain the effect of global warming in arctic marine systems. J. Plankton Res. 30, 1203–1206. doi:10.1093/plankt/fbn075
Kahru, M., Brotas, V., Manzano-Sarabia, M., and Mitchell, B. G. (2011). Are phytoplankton blooms occurring earlier in the Arctic? Glob. Change Biol. 17, 1733–1739. doi:10.1111/j.1365-2486.2010.02312.x
Kahru, M., Lee, Z., Mitchell, B. G., and Nevison, C. D. (2016). Effects of sea ice cover on satellite-detected primary production in the Arctic Ocean. Biol. Lett. 12, 20160223. doi:10.1098/rsbl.2016.0223
Kaiser, P., Hagen, W., Bode-Dalby, M., and Auel, H. (2022). Tolerant but facing increased competition: arctic zooplankton versus Atlantic invaders in a warming ocean. Front. Mar. Sci. 9. doi:10.3389/fmars.2022.908638
Kavan, J., and Strzelecki, M. C. (2023). Glacier decay boosts the formation of new Arctic coastal environments—perspectives from Svalbard. Land Degrad. and Dev. 34, 3467–3474. doi:10.1002/ldr.4695
Kędra, M., Moritz, C., Choy, E. S., David, C., Degen, R., Duerksen, S., et al. (2015). Status and trends in the structure of Arctic benthic food webs. Polar Res. 34, 23775. doi:10.3402/polar.v34.23775
Kelly, B. P., Whiteley, A., and Tallmon, D. (2010). The Arctic melting pot. Nature 468, 891. doi:10.1038/468891a
Khosravi, N., Wang, Q., Koldunov, N., Hinrichs, C., Semmler, T., Danilov, S., et al. (2022). The Arctic Ocean in CMIP6 models: biases and projected changes in temperature and salinity. Earth’s Future 10, e2021EF002282. doi:10.1029/2021EF002282
Kimmel, D. G., Eisner, L. B., and Pinchuk, A. I. (2023). The northern Bering Sea zooplankton community response to variability in sea ice: evidence from a series of warm and cold periods. Mar. Ecol. Prog. Ser. 705, 21–42. doi:10.3354/meps14237
Kimmel, D. G., Eisner, L. B., Wilson, M. T., and Duffy-Anderson, J. T. (2018). Copepod dynamics across warm and cold periods in the eastern Bering Sea: implications for walleye pollock (Gadus chalcogrammus) and the Oscillating Control Hypothesis. Fish. Oceanogr. 27, 143–158. doi:10.1111/fog.12241
Knutsen, T., Wiebe, P. H., Gjøsæter, H., Ingvaldsen, R. B., and Lien, G. (2017). High latitude epipelagic and mesopelagic scattering layers—a reference for future arctic ecosystem change. Front. Mar. Sci. 4, 334. doi:10.3389/fmars.2017.00334
Ko, E., Gorbunov, M. Y., Jung, J., Joo, H. M., Lee, Y., Cho, K.-H., et al. (2020). Effects of nitrogen limitation on phytoplankton physiology in the western Arctic Ocean in summer. J. Geophys. Res. Oceans 125, e2020JC016501. doi:10.1029/2020JC016501
Koch, C. W., Cooper, L. W., Grebmeier, J. M., Frey, K., and Brown, T. A. (2020). Ice algae resource utilization by benthic macro- and megafaunal communities on the Pacific Arctic shelf determined through lipid biomarker analysis. Mar. Ecol. Prog. Ser. 651, 23–43. doi:10.3354/meps13476
Koenigk, T., Key, J., and Vihma, T. (2020). “Climate change in the arctic,” in Physics and chemistry of the arctic atmosphere. Editors A. Kokhanovsky, and C. Tomasi (Cham: Springer International Publishing), 673–705. doi:10.1007/978-3-030-33566-3_11
Kohlbach, D., Graeve, M., A. Lange, B., David, C., Peeken, I., and Flores, H. (2016). The importance of ice algae-produced carbon in the central Arctic Ocean ecosystem: food web relationships revealed by lipid and stable isotope analyses. Limnol. Oceanogr. 61, 2027–2044. doi:10.1002/lno.10351
Kokarev, V. N., Vedenin, A. A., Basin, A. B., and Azovsky, A. I. (2017). Taxonomic and functional patterns of macrobenthic communities on a high-Arctic shelf: a case study from the Laptev Sea. J. Sea Res. 129, 61–69. doi:10.1016/j.seares.2017.08.011
Kortsch, S., Primicerio, R., Aschan, M., Lind, S., Dolgov, A. V., and Planque, B. (2019). Food-web structure varies along environmental gradients in a high-latitude marine ecosystem. Ecography 42, 295–308. doi:10.1111/ecog.03443
Kortsch, S., Primicerio, R., Beuchel, F., Renaud, P. E., Rodrigues, J., Lønne, O. J., et al. (2012). Climate-driven regime shifts in Arctic marine benthos. PNAS 109, 14052–14057. doi:10.1073/pnas.1207509109
Kortsch, S., Primicerio, R., Fossheim, M., Dolgov, A. V., and Aschan, M. (2015). Climate change alters the structure of arctic marine food webs due to poleward shifts of boreal generalists. Proc. R. Soc. B Biol. Sci. 282, 20151546. doi:10.1098/rspb.2015.1546
Kosobokova, K. N., Hopcroft, R. R., and Hirche, H.-J. (2011). Patterns of zooplankton diversity through the depths of the Arctic’s central basins. Mar. Biodiv 41, 29–50. doi:10.1007/s12526-010-0057-9
Kovacs, K. M., and Lydersen, C. (2008). Climate change impacts on seals and whales in the North atlantic arctic and adjacent shelf seas. Sci. Prog. 91, 117–150. doi:10.3184/003685008X324010
Kovacs, K. M., Lydersen, C., Overland, J. E., and Moore, S. E. (2011). Impacts of changing sea-ice conditions on Arctic marine mammals. Mar. Biodiv 41, 181–194. doi:10.1007/s12526-010-0061-0
Kraft, A., Nöthig, E.-M., Bauerfeind, E., Wildish, D. J., Pohle, G. W., Bathmann, U. V., et al. (2013). First evidence of reproductive success in a southern invader indicates possible community shifts among Arctic zooplankton. Mar. Ecol. Prog. Ser. 493, 291–296. doi:10.3354/meps10507
Krause-Jensen, D., Archambault, P., Assis, J., Bartsch, I., Bischof, K., Filbee-Dexter, K., et al. (2020). Imprint of climate change on pan-arctic marine vegetation. Front. Mar. Sci. 7. doi:10.3389/fmars.2020.617324
Kritzberg, E. S., Duarte, C. M., and Wassmann, P. (2010). Changes in Arctic marine bacterial carbon metabolism in response to increasing temperature. Polar Biol. 33, 1673–1682. doi:10.1007/s00300-010-0799-7
Kuletz, K., Cushing, D., and Labunski, E. (2020). Distributional shifts among seabird communities of the Northern Bering and Chukchi seas in response to ocean warming during 2017–2019. Deep Sea Res. Part II Top. Stud. Oceanogr. 181–182, 104913. doi:10.1016/j.dsr2.2020.104913
Kuletz, K. J., Ferguson, S., Frederiksen, M., Gallagher, C. P., Hauser, D. D., Hop, H., et al. (2024). A review of climate change impacts on migration patterns of marine vertebrates in Arctic and Subarctic ecosystems. Front. Environmen. Sci. 12. Available at: https://www.frontiersin.org/journals/environmental-science/articles/10.3389/fenvs.2024.1434549/abstract(Accessed October 17, 2024).
Kunisch, E. H., Bluhm, B. A., Daase, M., Gradinger, R., Hop, H., Melnikov, I. A., et al. (2020). Pelagic occurrences of the ice amphipod Apherusa glacialis throughout the Arctic. J. Plankton Res. 42, 73–86. doi:10.1093/plankt/fbz072
Kvernvik, A. C., Hoppe, C. J. M., Lawrenz, E., Prášil, O., Greenacre, M., Wiktor, J. M., et al. (2018). Fast reactivation of photosynthesis in arctic phytoplankton during the polar night1. J. Phycol. 54, 461–470. doi:10.1111/jpy.12750
Lacour, T., Morin, P.-I., Sciandra, T., Donaher, N., Campbell, D. A., Ferland, J., et al. (2019). Decoupling light harvesting, electron transport and carbon fixation during prolonged darkness supports rapid recovery upon re-illumination in the Arctic diatom Chaetoceros neogracilis. Polar Biol. 42, 1787–1799. doi:10.1007/s00300-019-02507-2
Laidre, K. L., Stern, H., Kovacs, K. M., Lowry, L., Moore, S. E., Regehr, E. V., et al. (2015). Arctic marine mammal population status, sea ice habitat loss, and conservation recommendations for the 21st century. Conserv. Biol. 29, 724–737. doi:10.1111/cobi.12474
Lalande, C., Bauerfeind, E., Nöthig, E.-M., and Beszczynska-Möller, A. (2013). Impact of a warm anomaly on export fluxes of biogenic matter in the eastern Fram Strait. Prog. Oceanogr. 109, 70–77. doi:10.1016/j.pocean.2012.09.006
Lalande, C., Bélanger, S., and Fortier, L. (2009). Impact of a decreasing sea ice cover on the vertical export of particulate organic carbon in the northern Laptev Sea, Siberian Arctic Ocean. Geophys. Res. Lett. 36. doi:10.1029/2009GL040570
Lam, V. W. Y., Cheung, W. W. L., Reygondeau, G., and Sumaila, U. R. (2016). Projected change in global fisheries revenues under climate change. Sci. Rep. 6, 32607. doi:10.1038/srep32607
Langangen, Ø., Stige, L. C., Kvile, K. Ø., Yaragina, N. A., Skjæraasen, J. E., Vikebø, F. B., et al. (2018). Multi-decadal variations in spawning ground use in Northeast Arctic haddock (Melanogrammus aeglefinus). Fish. Oceanogr. 27, 435–444. doi:10.1111/fog.12264
Langbehn, T. J., Aksnes, D. L., Kaartvedt, S., Fiksen, Ø., Ljungström, G., and Jørgensen, C. (2022). Poleward distribution of mesopelagic fishes is constrained by seasonality in light. Glob. Ecol. Biogeogr. 31, 546–561. doi:10.1111/geb.13446
Lannuzel, D., Tedesco, L., van Leeuwe, M., Campbell, K., Flores, H., Delille, B., et al. (2020). The future of Arctic sea-ice biogeochemistry and ice-associated ecosystems. Nat. Clim. Chang. 10, 983–992. doi:10.1038/s41558-020-00940-4
Lee, Y., Min, J.-O., Yang, E. J., Cho, K.-H., Jung, J., Park, J., et al. (2019). Influence of sea ice concentration on phytoplankton community structure in the Chukchi and East siberian seas, pacific Arctic Ocean. Deep Sea Res. Part I Oceanogr. Res. Pap. 147, 54–64. doi:10.1016/j.dsr.2019.04.001
Lefebvre, K. A., Fachon, E., Bowers, E. K., Kimmel, D. G., Snyder, J. A., Stimmelmayr, R., et al. (2022). Paralytic shellfish toxins in Alaskan Arctic food webs during the anomalously warm ocean conditions of 2019 and estimated toxin doses to Pacific walruses and bowhead whales. Harmful Algae 114, 102205. doi:10.1016/j.hal.2022.102205
Le Fouest, V., Babin, M., and Tremblay, J.-É. (2013a). The fate of riverine nutrients on Arctic shelves. Biogeosciences 10, 3661–3677. doi:10.5194/bg-10-3661-2013
Le Fouest, V., Zakardjian, B., Xie, H., Raimbault, P., Joux, F., and Babin, M. (2013b). Modeling plankton ecosystem functioning and nitrogen fluxes in the oligotrophic waters of the Beaufort Sea, Arctic Ocean: a focus on light-driven processes. Biogeosciences 10, 4785–4800. doi:10.5194/bg-10-4785-2013
Levine, R. M., De Robertis, A., Grünbaum, D., Wildes, S., Farley, E. V., Stabeno, P. J., et al. (2023). Climate-driven shifts in pelagic fish distributions in a rapidly changing Pacific Arctic. Deep Sea Res. Part II Top. Stud. Oceanogr. 208, 105244. doi:10.1016/j.dsr2.2022.105244
Lewis, K. M., Dijken, G. L. van, and Arrigo, K. R. (2020). Changes in phytoplankton concentration now drive increased Arctic Ocean primary production. Science 369, 198–202. doi:10.1126/science.aay8380
Li, W. K. W., McLaughlin, F. A., Lovejoy, C., and Carmack, E. C. (2009). Smallest algae thrive as the Arctic Ocean freshens. Science 326, 539. doi:10.1126/science.1179798
Lim, S. M., Payne, C. M., van Dijken, G. L., and Arrigo, K. R. (2022). Increases in Arctic sea ice algal habitat, 1985–2018. Elem. Sci. Anthropocene 10, 00008. doi:10.1525/elementa.2022.00008
Lin, L., Chen, Y., Liao, Y., Zhang, J., Song, P., Yu, X., et al. (2014). Composition of fish species in the Bering and Chukchi Seas and their responses to changes in the ecological environment. Acta Oceanol. Sin. 33, 63–73. doi:10.1007/s13131-014-0490-x
Liu, Q., Li, Y., Wang, H., Yang, G., Kan, J., Yang, M., et al. (2023). Assembly and network stability of planktonic microorganisms under the influence of salinity gradient: an arctic case study from the lena river estuary to the Laptev Sea. Microbiol. Spectr. 11, e0211522. doi:10.1128/spectrum.02115-22
Livingston, P. A., and Tjelmeland, S. (2000). Fisheries in boreal ecosystems. ICES J. Mar. Sci. 57, 619–627. doi:10.1006/jmsc.2000.0728
Ljungström, G., Claireaux, M., Fiksen, Ø., and Jørgensen, C. (2020). Body size adaptions under climate change: zooplankton community more important than temperature or food abundance in model of a zooplanktivorous fish. Mar. Ecol. Prog. Ser. 636, 1–18. doi:10.3354/meps13241
Ljungström, G., Langbehn, T. J., and Jørgensen, C. (2021). Light and energetics at seasonal extremes limit poleward range shifts. Nat. Clim. Chang. 11, 530–536. doi:10.1038/s41558-021-01045-2
Logerwell, E. A., Wang, M., Jörgensen, L. L., and Rand, K. (2022). Winners and losers in a warming Arctic: potential habitat gain and loss for epibenthic invertebrates of the Chukchi and Bering Seas, 2008–2100. Deep Sea Res. Part II Top. Stud. Oceanogr. 206, 105210. doi:10.1016/j.dsr2.2022.105210
Lotze, H. K., Tittensor, D. P., Bryndum-Buchholz, A., Eddy, T. D., Cheung, W. W. L., Galbraith, E. D., et al. (2019). Global ensemble projections reveal trophic amplification of ocean biomass declines with climate change. PNAS 116, 12907–12912. doi:10.1073/pnas.1900194116
Lovejoy, C., Massana, R., and Pedrós-Alió, C. (2006). Diversity and distribution of marine microbial eukaryotes in the Arctic Ocean and adjacent seas. Appl. Environ. Microbiol. 72, 3085–3095. doi:10.1128/AEM.72.5.3085-3095.2006
Lovejoy, C., Vincent, W. F., Bonilla, S., Roy, S., Martineau, M.-J., Terrado, R., et al. (2007). Distribution, phylogeny, and growth of cold-adapted picoprasinophytes in arctic Seas1. J. Phycol. 43, 78–89. doi:10.1111/j.1529-8817.2006.00310.x
Lydersen, C., Assmy, P., Falk-Petersen, S., Kohler, J., Kovacs, K. M., Reigstad, M., et al. (2014). The importance of tidewater glaciers for marine mammals and seabirds in Svalbard, Norway. J. Mar. Syst. 129, 452–471. doi:10.1016/j.jmarsys.2013.09.006
Mańko, M. K., Gluchowska, M., and Weydmann-Zwolicka, A. (2020). Footprints of atlantification in the vertical distribution and diversity of gelatinous zooplankton in the Fram Strait (Arctic Ocean). Prog. Oceanogr. 189, 102414. doi:10.1016/j.pocean.2020.102414
Mańko, M. K., Merchel, M., Kwasniewski, S., and Weydmann-Zwolicka, A. (2022). Oceanic fronts shape biodiversity of gelatinous zooplankton in the European arctic. Front. Mar. Sci. 9. doi:10.3389/fmars.2022.941025
Marsh, J. M., and Mueter, F. J. (2020). Influences of temperature, predators, and competitors on polar cod (Boreogadus saida) at the southern margin of their distribution. Polar Biol. 43, 995–1014. doi:10.1007/s00300-019-02575-4
Matishov, G., Moiseev, D., Lyubina, O., Zhichkin, A., Dzhenyuk, S., Karamushko, O., et al. (2012). Climate and cyclic hydrobiological changes of the Barents Sea from the twentieth to twenty-first centuries. Polar Biol. 35, 1773–1790. doi:10.1007/s00300-012-1237-9
Mayot, N., Matrai, P. A., Arjona, A., Bélanger, S., Marchese, C., Jaegler, T., et al. (2020). Springtime export of arctic sea ice influences phytoplankton production in the Greenland sea. J. Geophys. Res. Oceans 125, e2019JC015799. doi:10.1029/2019JC015799
Mazanowski, K., Mańko, M. K., Møller, E. F., and Weydmann-Zwolicka, A. (2023). Gelatinous zooplankton off the Northeast Greenland coast. Prog. Oceanogr. 219, 103173. doi:10.1016/j.pocean.2023.103173
Maznikova, O. A., Emelin, P. O., Sheibak, A. Y., Nosov, M. A., and Orlov, A. M. (2023). Can an invader support commercial fishing? A case study of walleye pollock Gadus chalcogrammus in the western Chukchi Sea. Deep Sea Res. Part II Top. Stud. Oceanogr. 207, 105222. doi:10.1016/j.dsr2.2022.105222
McBride, M. M., Dalpadado, P., Drinkwater, K. F., Godø, O. R., Hobday, A. J., Hollowed, A. B., et al. (2014). Krill, climate, and contrasting future scenarios for Arctic and Antarctic fisheries. ICES J. Mar. Sci. 71, 1934–1955. doi:10.1093/icesjms/fsu002
McCrystall, M. R., Stroeve, J., Serreze, M., Forbes, B. C., and Screen, J. A. (2021). New climate models reveal faster and larger increases in Arctic precipitation than previously projected. Nat. Commun. 12, 6765. doi:10.1038/s41467-021-27031-y
McNicholl, D. G., Davoren, G. K., Majewski, A. R., and Reist, J. D. (2018). Isotopic niche overlap between co-occurring capelin (Mallotus villosus) and polar cod (Boreogadus saida) and the effect of lipid extraction on stable isotope ratios. Polar Biol. 41, 423–432. doi:10.1007/s00300-017-2199-8
McNicholl, D. G., Walkusz, W., Davoren, G. K., Majewski, A. R., and Reist, J. D. (2016). Dietary characteristics of co-occurring polar cod (Boreogadus saida) and capelin (Mallotus villosus) in the Canadian Arctic, Darnley Bay. Polar Biol. 39, 1099–1108. doi:10.1007/s00300-015-1834-5
Mecklenburg, C. W., Møller, P. R., and Steinke, D. (2011). Biodiversity of arctic marine fishes: taxonomy and zoogeography. Mar. Biodiv 41, 109–140. doi:10.1007/s12526-010-0070-z
Meire, L., Mortensen, J., Meire, P., Juul-Pedersen, T., Sejr, M. K., Rysgaard, S., et al. (2017). Marine-terminating glaciers sustain high productivity in Greenland fjords. Glob. Change Biol. 23, 5344–5357. doi:10.1111/gcb.13801
Merkel, F. R., Linnebjerg, J. F., Andersen, O. G. N., Huffeldt, N. P., Jansen, T., Hedeholm, R., et al. (2021). Changing winter diet of Thick-billed Murres (Uria lomvia) in southwest Greenland, 1990s versus 2010s. Can. J. Zool. 99, 1080–1088. doi:10.1139/cjz-2021-0120
Meyer-Kaiser, K. S., Schrage, K. R., von Appen, W.-J., Hoppmann, M., Lochthofen, N., Sundfjord, A., et al. (2022). Larval dispersal and recruitment of benthic invertebrates in the Arctic Ocean. Prog. Oceanogr. 203, 102776. doi:10.1016/j.pocean.2022.102776
Michel, C., Hamilton, J., Hansen, E., Barber, D., Reigstad, M., Iacozza, J., et al. (2015). Arctic Ocean outflow shelves in the changing Arctic: a review and perspectives. Prog. Oceanogr. 139, 66–88. doi:10.1016/j.pocean.2015.08.007
Michel, C., Ingram, R. G., and Harris, L. R. (2006). Variability in oceanographic and ecological processes in the Canadian Arctic Archipelago. Prog. Oceanogr. 71, 379–401. doi:10.1016/j.pocean.2006.09.006
Mills, M. M., Brown, Z. W., Laney, S. R., Ortega-Retuerta, E., Lowry, K. E., van Dijken, G. L., et al. (2018). Nitrogen limitation of the summer phytoplankton and heterotrophic prokaryote communities in the Chukchi Sea. Front. Mar. Sci. 5. doi:10.3389/fmars.2018.00362
Min, C., Yang, Q., Chen, D., Yang, Y., Zhou, X., Shu, Q., et al. (2022). The emerging arctic shipping corridors. Geophys. Res. Lett. 49, e2022GL099157. doi:10.1029/2022GL099157
Min Joo, H., Lee, S. H., Won Jung, S., Dahms, H.-U., and Hwan Lee, J. (2012). Latitudinal variation of phytoplankton communities in the western Arctic Ocean. Deep Sea Res. Part II Top. Stud. Oceanogr. 81–84, 3–17. doi:10.1016/j.dsr2.2011.06.004
Møller, E. F., and Nielsen, T. G. (2020). Borealization of Arctic zooplankton—smaller and less fat zooplankton species in Disko Bay, Western Greenland. Limnol. Oceanogr. 65, 1175–1188. doi:10.1002/lno.11380
Moore, S. E., Clarke, J. T., Okkonen, S. R., Grebmeier, J. M., Berchok, C. L., and Stafford, K. M. (2022). Changes in gray whale phenology and distribution related to prey variability and ocean biophysics in the northern Bering and eastern Chukchi seas. PLOS ONE 17, e0265934. doi:10.1371/journal.pone.0265934
Mousing, E. A., Ellingen, I., Hjøllo, S. S., Husson, B., Skogen, M. D., and Wallhead, P. (2023). Why do regional biogeochemical models produce contrasting future projections of primary production in the Barents Sea? J. Sea Res. 192, 102366. doi:10.1016/j.seares.2023.102366
Mueter, F. J., Iken, K., Cooper, L. W., Grebmeier, J. M., Kuletz, K. J., Hopcroft, R. R., et al. (2021a). Changes in diversity and species composition across multiple assemblages in the eastern Chukchi Sea during two contrasting years are consistent with borealization. Oceanography 34, 38–51. doi:10.5670/oceanog.2021.213
Mueter, F. J., and Litzow, M. A. (2008). Sea ice retreat alters the biogeography of the bering Sea continental shelf. Ecol. Appl. 18, 309–320. doi:10.1890/07-0564.1
Mueter, F. J., Nahrgang, J., John Nelson, R., and Berge, J. (2016). The ecology of gadid fishes in the circumpolar Arctic with a special emphasis on the polar cod (Boreogadus saida). Polar Biol. 39, 961–967. doi:10.1007/s00300-016-1965-3
Mueter, F. J., Planque, B., Hunt, G. L., Alabia, I. D., Hirawake, T., Eisner, L., et al. (2021b). Possible future scenarios in the gateways to the Arctic for Subarctic and Arctic marine systems: II. prey resources, food webs, fish, and fisheries. ICES J. Mar. Sci. 78, 3017–3045. doi:10.1093/icesjms/fsab122
Mullowney, D. R. J., Baker, K. D., Szuwalski, C. S., Boudreau, S. A., Cyr, F., and Kaiser, B. A. (2023). Sub-Arctic no more: short- and long-term global-scale prospects for snow crab (Chionoecetes opilio) under global warming. PLOS Clim. 2, e0000294. doi:10.1371/journal.pclm.0000294
Nascimento, M. C., Husson, B., Guillet, L., and Pedersen, T. (2023). Modelling the spatial shifts of functional groups in the Barents Sea using a climate-driven spatial food web model. Ecol. Model. 481, 110358. doi:10.1016/j.ecolmodel.2023.110358
Nelson, R. J., Ashjian, C. J., Bluhm, B. A., Conlan, K. E., Gradinger, R. R., Grebmeier, J. M., et al. (2014). “Biodiversity and biogeography of the lower trophic taxa of the pacific arctic region: sensitivities to climate change,” in The pacific arctic region. Editors J. M. Grebmeier, and W. Maslowski (Dordrecht: Springer Netherlands), 269–336. doi:10.1007/978-94-017-8863-2_10
Neukermans, G., Oziel, L., and Babin, M. (2018). Increased intrusion of warming Atlantic water leads to rapid expansion of temperate phytoplankton in the Arctic. Glob. Change Biol. 24, 2545–2553. doi:10.1111/gcb.14075
Nielsen, J. M., Sigler, M. F., Eisner, L. B., Watson, J. T., Rogers, L. A., Bell, S. W., et al. (2024). Spring phytoplankton bloom phenology during recent climate warming on the Bering Sea shelf. Prog. Oceanogr. 220, 103176. doi:10.1016/j.pocean.2023.103176
Niemi, A., Bednaršek, N., Michel, C., Feely, R. A., Williams, W., Azetsu-Scott, K., et al. (2021). Biological impact of ocean acidification in the Canadian arctic: widespread severe pteropod shell dissolution in amundsen gulf. Front. Mar. Sci. 8. doi:10.3389/fmars.2021.600184
Niemi, A., Bluhm, B. A., Juul-Pedersen, T., Kohlbach, D., Reigstad, M., Søgaard, D. H., et al. (2024). Ice algae contributions to the benthos during a time of sea ice change: a review of supply, coupling, and fate. Front. Environmen. Sci. 12. Available at: https://www.frontiersin.org/journals/environmental-science/articles/10.3389/fenvs.2024.1432761/full(Accessed October 17, 2024).
Niiranen, S., Richter, A., Blenckner, T., Stige, L. C., Valman, M., and Eikeset, A.-M. (2018). Global connectivity and cross-scale interactions create uncertainty for Blue Growth of Arctic fisheries. Mar. Policy 87, 321–330. doi:10.1016/j.marpol.2017.10.024
Nilsson, A. E., Carson, M., Cost, D. S., Forbes, B. C., Haavisto, R., Karlsdottir, A., et al. (2021). Towards improved participatory scenario methodologies in the Arctic. Polar Geogr. 44, 75–89. doi:10.1080/1088937X.2019.1648583
Nishio, S., Sasaki, H., Waga, H., and Yamamura, O. (2020). Effects of the timing of sea ice retreat on demersal fish assemblages in the northern bering and Chukchi Seas. Deep Sea Res. Part II Top. Stud. Oceanogr. 181–182, 104910. doi:10.1016/j.dsr2.2020.104910
Noh, K.-M., Oh, J.-H., Lim, H.-G., Song, H., and Kug, J.-S. (2024). Role of atlantification in enhanced primary productivity in the Barents Sea. Earth’s Future 12, e2023EF003709. doi:10.1029/2023EF003709
Nummelin, A., Ilicak, M., Li, C., and Smedsrud, L. H. (2016). Consequences of future increased Arctic runoff on Arctic Ocean stratification, circulation, and sea ice cover. J. Geophys. Res. Oceans 121, 617–637. doi:10.1002/2015JC011156
Okolodkov, Y. B. (2005). The global distributional patterns of toxic, bloom dinoflagellates recorded from the Eurasian Arctic. Harmful Algae 4, 351–369. doi:10.1016/j.hal.2004.06.016
Olsen, T., Frøysa, H. G., Yaragina, N. A., Titelman, J., Durant, J. M., and Langangen, Ø. (2024). Predator biomass affects west–east shifts in Barents Sea capelin (Mallotus villosus) spawning ground use. Fish. Oceanogr. 33, e12685. doi:10.1111/fog.12685
Onarheim, I. H., Eldevik, T., Smedsrud, L. H., and Stroeve, J. C. (2018). Seasonal and regional manifestation of arctic sea ice loss. J. Clim. 31, 4917–4932. doi:10.1175/JCLI-D-17-0427.1
Ono, J., Watanabe, M., Komuro, Y., Tatebe, H., and Abe, M. (2022). Enhanced Arctic warming amplification revealed in a low-emission scenario. Commun. Earth Environ. 3, 27–29. doi:10.1038/s43247-022-00354-4
Opdal, A. F., and Jørgensen, C. (2015). Long-term change in a behavioural trait: truncated spawning distribution and demography in Northeast Arctic cod. Glob. Change Biol. 21, 1521–1530. doi:10.1111/gcb.12773
Orlov, A. M., and Volvenko, I. V. (2024). Ongoing borealization of Siberian Arctic marine ichthyofauna: further evidence. Prog. Oceanogr. 225, 103288. doi:10.1016/j.pocean.2024.103288
Orlova, E. L., Dolgov, A. V., Renaud, P. E., Boitsov, V. D., Prokopchuk, I. P., and Zashihina, M. V. (2013). Structure of the macroplankton–pelagic fish–cod trophic complex in a warmer Barents Sea. Mar. Biol. Res. 9, 851–866. doi:10.1080/17451000.2013.775453
Orlova, E. L., Dolgov, A. V., Renaud, P. E., Greenacre, M., Halsband, C., and Ivshin, V. A. (2015). Climatic and ecological drivers of euphausiid community structure vary spatially in the Barents Sea: relationships from a long time series (1952â€2009). Front. Mar. Sci. 1, 74. doi:10.3389/fmars.2014.00074
Orlova, E. L., Rudneva, G. B., Renaud, P. E., Eiane, K., Savinov, V., Yurko, A. S., et al. (2010). Climate impacts on feeding and condition of capelin Mallotus villosus in the Barents Sea: evidence and mechanisms from a 30 year data set. Aquat. Biol. 10, 105–118. doi:10.3354/ab00265
Orlova, S.Yu., Volkov, A. A., Shcepetov, D. M., Maznikova, O. A., Chernova, N. V., Chikurova, E. A., et al. (2019). Inter- and intra-species relationships of Greenland halibut Reinhardtius hippoglossoides (pleuronectidae) based on the analysis of nuclear and mitochondrial genetic markers. J. Ichthyol. 59, 65–77. doi:10.1134/S0032945219010119
Overland, J., Dunlea, E., Box, J. E., Corell, R., Forsius, M., Kattsov, V., et al. (2019). The urgency of Arctic change. Polar Sci. 21, 6–13. doi:10.1016/j.polar.2018.11.008
Oviatt, C., Smith, L., McManus, M. C., and Hyde, K. (2015). Decadal patterns of westerly winds, temperatures, ocean gyre circulations and fish abundance: a review. Climate 3, 833–857. doi:10.3390/cli3040833
Oziel, L., Baudena, A., Ardyna, M., Massicotte, P., Randelhoff, A., Sallée, J.-B., et al. (2020). Faster Atlantic currents drive poleward expansion of temperate phytoplankton in the Arctic Ocean. Nat. Commun. 11, 1705. doi:10.1038/s41467-020-15485-5
Oziel, L., Neukermans, G., Ardyna, M., Lancelot, C., Tison, J.-L., Wassmann, P., et al. (2017). Role for Atlantic inflows and sea ice loss on shifting phytoplankton blooms in the Barents Sea. J. Geophys. Res. Oceans 122, 5121–5139. doi:10.1002/2016JC012582
Oziel, L., Schourup-Kristensen, V., Wekerle, C., and Hauck, J. (2022). The pan-arctic continental slope as an intensifying conveyer belt for nutrients in the central Arctic Ocean (1985–2015). Glob. Biogeochem. Cycles 36, e2021GB007268. doi:10.1029/2021GB007268
Pantiukhin, D., Verhaegen, G., and Havermans, C. (2024). Pan-Arctic distribution modeling reveals climate-change-driven poleward shifts of major gelatinous zooplankton species. Limnol. Oceanogr. 69, 1316–1334. doi:10.1002/lno.12568
Pantiukhin, D., Verhaegen, G., Kraan, C., Jerosch, K., Neitzel, P., Hoving, H.-J. T., et al. (2023). Optical observations and spatio-temporal projections of gelatinous zooplankton in the Fram Strait, a gateway to a changing Arctic Ocean. Front. Mar. Sci. 10. doi:10.3389/fmars.2023.987700
Pecuchet, L., Blanchet, M.-A., Frainer, A., Husson, B., Jørgensen, L. L., Kortsch, S., et al. (2020). Novel feeding interactions amplify the impact of species redistribution on an Arctic food web. Glob. Change Biol. 26, 4894–4906. doi:10.1111/gcb.15196
Pécuchet, L., Mohamed, B., Hayward, A., Alvera-Azcarate, A., Dörr, J., Filbee-Dexter, K., et al. (2024). Arctic and Subarctic marine heatwaves and their ecological impacts. Front. Environmen. Sci. 12.
Pedersen, T., Mikkelsen, N., Lindstrøm, U., Renaud, P. E., Nascimento, M. C., Blanchet, M.-A., et al. (2021). Overexploitation, recovery, and warming of the Barents Sea ecosystem during 1950–2013. Front. Mar. Sci. 8. Available at:. doi:10.3389/fmars.2021.732637
Pedro, S., Fisk, A. T., Ferguson, S. H., Hussey, N. E., Kessel, S. T., and McKinney, M. A. (2020). Broad feeding niches of capelin and sand lance may overlap those of polar cod and other native fish in the eastern Canadian Arctic. Polar Biol. 43, 1707–1724. doi:10.1007/s00300-020-02738-8
Pedro, S., Lemire, M., Hoover, C., Saint-Béat, B., Janjua, M. Y., Herbig, J., et al. (2023). Structure and function of the western Baffin Bay coastal and shelf ecosystem. Elem. Sci. Anthropocene 11, 00015. doi:10.1525/elementa.2022.00015
Pilfold, N. W., McCall, A., Derocher, A. E., Lunn, N. J., and Richardson, E. (2017). Migratory response of polar bears to sea ice loss: to swim or not to swim. Ecography 40, 189–199. doi:10.1111/ecog.02109
Pinsky, M. L., Worm, B., Fogarty, M. J., Sarmiento, J. L., and Levin, S. A. (2013). Marine taxa track local climate velocities. Science 341, 1239–1242. doi:10.1126/science.1239352
Poloczanska, E. S., Burrows, M. T., Brown, C. J., García Molinos, J., Halpern, B. S., Hoegh-Guldberg, O., et al. (2016). Responses of marine organisms to climate change across oceans. Front. Mar. Sci. 3. doi:10.3389/fmars.2016.00062
Polyakov, I. V., Alkire, M. B., Bluhm, B. A., Brown, K. A., Carmack, E. C., Chierici, M., et al. (2020a). Borealization of the Arctic Ocean in response to anomalous advection from sub-arctic seas. Front. Mar. Sci. 7. doi:10.3389/fmars.2020.00491
Polyakov, I. V., Ingvaldsen, R. B., Pnyushkov, A. V., Bhatt, U. S., Francis, J. A., Janout, M., et al. (2023). Fluctuating Atlantic inflows modulate Arctic atlantification. Science 381, 972–979. doi:10.1126/science.adh5158
Polyakov, I. V., Pnyushkov, A. V., Alkire, M. B., Ashik, I. M., Baumann, T. M., Carmack, E. C., et al. (2017). Greater role for atlantic inflows on seasea-ice loss in the Eurasian Basin of the Arctic Ocean. Science 356, 285–291. doi:10.1126/science.aai8204
Polyakov, I. V., Rippeth, T. P., Fer, I., Alkire, M. B., Baumann, T. M., Carmack, E. C., et al. (2020b). Weakening of cold halocline layer exposes sea ice to oceanic heat in the eastern Arctic Ocean. J. Clim. 33, 8107–8123. doi:10.1175/JCLI-D-19-0976.1
Polyakova, Ye. I., Kryukova, I. M., Martynov, F. M., Novikhin, A. E., Abramova, E. N., Kassens, H., et al. (2021). Community structure and spatial distribution of phytoplankton in relation to hydrography in the Laptev Sea and the East Siberian Sea (autumn 2008). Polar Biol. 44, 1229–1250. doi:10.1007/s00300-021-02873-w
Pörtner, H.-O., Roberts, D. C., Masson-Delmotte, V., Zhai, P., Tignor, M., Poloczanska, E., et al. (2019). IPCC special report on the ocean and cryosphere in a changing climate. Cambridge, UK and New York, NY, USA: IPCC, 3–35. Available at: https://www.ipcc.ch/srocc/(Accessed August 15, 2024).
Post, S., Werner, K. M., Núñez-Riboni, I., Chafik, L., Hátún, H., and Jansen, T. (2021). Subpolar gyre and temperature drive boreal fish abundance in Greenland waters. Fish Fish. 22, 161–174. doi:10.1111/faf.12512
Poulin, M., Daugbjerg, N., Gradinger, R., Ilyash, L., Ratkova, T., and von Quillfeldt, C. (2011). The pan-Arctic biodiversity of marine pelagic and sea-ice unicellular eukaryotes: a first-attempt assessment. Mar. Biodiv 41, 13–28. doi:10.1007/s12526-010-0058-8
Previdi, M., Smith, K. L., and Polvani, L. M. (2021). Arctic amplification of climate change: a review of underlying mechanisms. Environ. Res. Lett. 16, 093003. doi:10.1088/1748-9326/ac1c29
Prokopchuk, I. P., and Trofimov, A. G. (2019). Interannual dynamics of zooplankton in the Kola Section of the Barents Sea during the recent warming period. ICES J. Mar. Sci. 76, i10–i23. doi:10.1093/icesjms/fsz206
Provencher, J. F., Gaston, A. J., O’Hara, P. D., and Gilchrist, H. G. (2012). Seabird diet indicates changing Arctic marine communities in eastern Canada. Mar. Ecol. Prog. Ser. 454, 171–182. doi:10.3354/meps09299
Qi, D., Ouyang, Z., Chen, L., Wu, Y., Lei, R., Chen, B., et al. (2022). Climate change drives rapid decadal acidification in the Arctic Ocean from 1994 to 2020. Science 377, 1544–1550. doi:10.1126/science.abo0383
Questel, J. M., Clarke, C., and Hopcroft, R. R. (2013). Seasonal and interannual variation in the planktonic communities of the northeastern Chukchi Sea during the summer and early fall. Cont. Shelf Res. 67, 23–41. doi:10.1016/j.csr.2012.11.003
Ramirez-Llodra, E., Argentino, C., Baker, M., Boetius, A., Costa, C., Dahle, H., et al. (2023). Hot vents beneath an icy ocean: the aurora vent field, gakkel ridge, revealed. Oceanography 36, 6–17. doi:10.5670/oceanog.2023.103
Ramondenc, S., Nöthig, E.-M., Hufnagel, L., Bauerfeind, E., Busch, K., Knüppel, N., et al. (2023). Effects of Atlantification and changing sea-ice dynamics on zooplankton community structure and carbon flux between 2000 and 2016 in the eastern Fram Strait. Limnol. Oceanogr. 68, S39–S53. doi:10.1002/lno.12192
Randall, J. R., Busby, M. S., Spear, A. H., and Mier, K. L. (2019). Spatial and temporal variation of late summer ichthyoplankton assemblage structure in the eastern Chukchi Sea: 2010–2015. Polar Biol. 42, 1811–1824. doi:10.1007/s00300-019-02555-8
Rantanen, M., Karpechko, A. Y., Lipponen, A., Nordling, K., Hyvärinen, O., Ruosteenoja, K., et al. (2022). The Arctic has warmed nearly four times faster than the globe since 1979. Commun. Earth Environ. 3, 168–210. doi:10.1038/s43247-022-00498-3
Ravelo, A. M., Konar, B., Bluhm, B., and Iken, K. (2017). Growth and production of the brittle stars Ophiura sarsii and Ophiocten sericeum (Echinodermata: ophiuroidea). Cont. Shelf Res. 139, 9–20. doi:10.1016/j.csr.2017.03.011
Reid, P. C., Johns, D. G., Edwards, M., Starr, M., Poulin, M., and Snoeijs, P. (2007). A biological consequence of reducing Arctic ice cover: arrival of the Pacific diatom Neodenticula seminae in the North Atlantic for the first time in 800 000 years. Glob. Change Biol. 13, 1910–1921. doi:10.1111/j.1365-2486.2007.01413.x
Renaud, P. E., Ambrose, W. G., Vanreusel, A., and Clough, L. M. (2006). Nematode and macrofaunal diversity in central Arctic Ocean benthos. J. Exp. Mar. Biol. Ecol. 330, 297–306. doi:10.1016/j.jembe.2005.12.035
Renaud, P. E., Daase, M., Banas, N. S., Gabrielsen, T. M., Søreide, J. E., Varpe, Ø., et al. (2018). Pelagic food-webs in a changing Arctic: a trait-based perspective suggests a mode of resilience. ICES J. Mar. Sci. 75, 1871–1881. doi:10.1093/icesjms/fsy063
Renaud, P. E., Wallhead, P., Kotta, J., Włodarska-Kowalczuk, M., Bellerby, R. G. J., Rätsep, M., et al. (2019). Arctic sensitivity? Suitable habitat for benthic taxa is surprisingly robust to climate change. Front. Mar. Sci. 6. doi:10.3389/fmars.2019.00538
Renaud, P. E., Włodarska-Kowalczuk, M., Trannum, H., Holte, B., Węsławski, J. M., Cochrane, S., et al. (2007). Multidecadal stability of benthic community structure in a high-Arctic glacial fjord (van Mijenfjord, Spitsbergen). Polar Biol. 30, 295–305. doi:10.1007/s00300-006-0183-9
Richman, S. E., and Lovvorn, J. R. (2003). Effects of clam species dominance on nutrient and energy acquisition by spectacled eiders in the Bering Sea. Mar. Ecol. Prog. Ser. 261, 283–297. doi:10.3354/meps261283
Riedlinger, D., and Berkes, F. (2001). Contributions of traditional knowledge to understanding climate change in the Canadian Arctic. Polar Rec. 37, 315–328. doi:10.1017/S0032247400017058
Rysgaard, S., and Nielsen, T. G. (2006). Carbon cycling in a high-arctic marine ecosystem – young Sound, NE Greenland. Prog. Oceanogr. 71, 426–445. doi:10.1016/j.pocean.2006.09.004
Sameoto, D. (1989). Feeding ecology of the lantern fish Benthosema glaciale in a subarctic region. Polar Biol. 9, 169–178. doi:10.1007/BF00297172
Sanders, T., Fiencke, C., Fuchs, M., Haugk, C., Juhls, B., Mollenhauer, G., et al. (2022). Seasonal nitrogen fluxes of the lena river delta. Ambio 51, 423–438. doi:10.1007/s13280-021-01665-0
Sauser, C., Angelier, F., Blévin, P., Chastel, O., Gabrielsen, G. W., Jouanneau, W., et al. (2023). Demographic responses of Arctic seabirds to spring sea-ice variations. Front. Ecol. Evol. 11. doi:10.3389/fevo.2023.1107992
Schiøtt, S., Tejsner, P., and Rysgaard, S. (2022). Inuit and local knowledge on the marine ecosystem in ilulissat icefjord, Greenland. Hum. Ecol. 50, 167–181. doi:10.1007/s10745-021-00277-2
Schmid, M. K., Piepenburg, D., Golikov, A. A., Juterzenka, K. von, Petryashov, V. V., and Spindler, M. (2006). Trophic pathways and carbon flux patterns in the Laptev Sea. Prog. Oceanogr. 71, 314–330. doi:10.1016/j.pocean.2006.09.002
Schwoerer, T., Spellman, K. V., Davis, T. J., Lee, O., Martin, A., Mulder, C. P. H., et al. (2021). Harnessing the power of community science to address data gaps in arctic observing: invasive species in Alaska as case examples. Arctic 74, 1–14. doi:10.14430/arctic73773
Serreze, M. C., and Barry, R. G. (2011). Processes and impacts of Arctic amplification: a research synthesis. Glob. Planet. Change 77, 85–96. doi:10.1016/j.gloplacha.2011.03.004
Shogren, A. J., Zarnetske, J. P., Abbott, B. W., Iannucci, F., and Bowden, W. B. (2020). We cannot shrug off the shoulder seasons: addressing knowledge and data gaps in an Arctic headwater. Environ. Res. Lett. 15, 104027. doi:10.1088/1748-9326/ab9d3c
Shu, Q., Wang, Q., Song, Z., and Qiao, F. (2021). The poleward enhanced Arctic Ocean cooling machine in a warming climate. Nat. Commun. 12, 2966. doi:10.1038/s41467-021-23321-7
Skjoldal, H. R., Eriksen, E., Gjøsæter, H., Skagseth, Ø., Prozorkevich, D., and Lien, V. S. (2022). Recruitment variability of fish stocks in the Barents Sea: spatial and temporal variation in 0-group fish length of six commercial species during recent decades of warming (1980–2017). Prog. Oceanogr. 206, 102845. doi:10.1016/j.pocean.2022.102845
Slagstad, D., Wassmann, P. F. J., and Ellingsen, I. (2015). Physical constrains and productivity in the future Arctic Ocean. Front. Mar. Sci. 2. Available at:. doi:10.3389/fmars.2015.00085
Smedsrud, L. H., Muilwijk, M., Brakstad, A., Madonna, E., Lauvset, S. K., Spensberger, C., et al. (2022). Nordic seas heat loss, atlantic inflow, and arctic sea ice cover over the last century. Rev. Geophys. 60, e2020RG000725. doi:10.1029/2020RG000725
Snoeijs-Leijonmalm, P., Flores, H., Sakinan, S., Hildebrandt, N., Svenson, A., Castellani, G., et al. (2022). Unexpected fish and squid in the central Arctic deep scattering layer. Sci. Adv. 8, eabj7536. doi:10.1126/sciadv.abj7536
Sora, K. J., Wabnitz, C. C. C., Steiner, N. S., Sumaila, U. R., Hoover, C., Niemi, A., et al. (2024). Historical climate drivers and species’ ecological niche in the Beaufort Sea food web. ICES J. Mar. Sci., fsae062. doi:10.1093/icesjms/fsae062
Søreide, J. E., Leu, E., Berge, J., Graeve, M., and Falk-Petersen, S. (2010). Timing of blooms, algal food quality and Calanus glacialis reproduction and growth in a changing Arctic. Glob. Change Biol. 16, 3154–3163. doi:10.1111/j.1365-2486.2010.02175.x
Sou, T., and Flato, G. (2009). Sea ice in the Canadian arctic Archipelago: modeling the past (1950–2004) and the future (2041–60). J. Clim. 22, 2181–2198. doi:10.1175/2008JCLI2335.1
Spear, A., Duffy-Anderson, J., Kimmel, D., Napp, J., Randall, J., and Stabeno, P. (2019). Physical and biological drivers of zooplankton communities in the Chukchi Sea. Polar Biol. 42, 1107–1124. doi:10.1007/s00300-019-02498-0
Spear, A., Napp, J., Ferm, N., and Kimmel, D. (2020). Advection and in situ processes as drivers of change for the abundance of large zooplankton taxa in the Chukchi Sea. Deep Sea Res. Part II Top. Stud. Oceanogr. 177, 104814. doi:10.1016/j.dsr2.2020.104814
Spotowitz, L., Johansen, T., Hansen, A., Berg, E., Stransky, C., and Fischer, P. (2022). New evidence for the establishment of coastal cod Gadus morhua in Svalbard fjords. Mar. Ecol. Prog. Ser. 696, 119–133. doi:10.3354/meps14126
Stabeno, P. J., and Bell, S. W. (2019). Extreme conditions in the Bering Sea (2017–2018): record-breaking low seasea-ice extent. Geophys. Res. Lett. 46, 8952–8959. doi:10.1029/2019GL083816
Stafford, K. M. (2019). Increasing detections of killer whales (Orcinus orca), in the Pacific Arctic. Mar. Mammal Sci. 35, 696–706. doi:10.1111/mms.12551
Steiner, N. S., Cheung, W. W. L., Cisneros-Montemayor, A. M., Drost, H., Hayashida, H., Hoover, C., et al. (2019). Impacts of the changing ocean-sea ice system on the key forage fish arctic cod (Boreogadus saida) and subsistence fisheries in the western Canadian arctic—evaluating linked climate, ecosystem and economic (CEE) models. Front. Mar. Sci. 6. doi:10.3389/fmars.2019.00179
Stewart, J. D., Joyce, T. W., Durban, J. W., Calambokidis, J., Fauquier, D., Fearnbach, H., et al. (2023). Boom-bust cycles in gray whales associated with dynamic and changing Arctic conditions. Science 382, 207–211. doi:10.1126/science.adi1847
Stige, L. C., Eriksen, E., Dalpadado, P., and Ono, K. (2019). Direct and indirect effects of sea ice cover on major zooplankton groups and planktivorous fishes in the Barents Sea. ICES J. Mar. Sci. 76, i24–i36. doi:10.1093/icesjms/fsz063
Stoecker, D. K., and Lavrentyev, P. J. (2018). Mixotrophic plankton in the polar seas: a pan-arctic review. Front. Mar. Sci. 5. doi:10.3389/fmars.2018.00292
Sugie, K., Fujiwara, A., Nishino, S., Kameyama, S., and Harada, N. (2020). Impacts of temperature, CO2, and salinity on phytoplankton community composition in the western Arctic Ocean. Front. Mar. Sci. 6. doi:10.3389/fmars.2019.00821
Sukhanova, I. N., Flint, M. V., Fedodov, A. V., Sakharova, E. G., Makkaveev, P. N., Polukhin, A. A., et al. (2021). First data on the structure of phytoplankton communities of the East Siberian sea. Oceanology 61, 909–929. doi:10.1134/S0001437021060151
Svavarsson, J., Guls, H. D., Sham, R. C., Leung, K. M. Y., and Halldórsson, H. P. (2021). Pollutants from shipping - new environmental challenges in the subarctic and the Arctic Ocean. Mar. Pollut. Bull. 164, 112004. doi:10.1016/j.marpolbul.2021.112004
Tai, T. C., Steiner, N. S., Hoover, C., Cheung, W. W. L., and Sumaila, U. R. (2019). Evaluating present and future potential of arctic fisheries in Canada. Mar. Policy 108, 103637. doi:10.1016/j.marpol.2019.103637
Tank, S. E., McClelland, J. W., Spencer, R. G. M., Shiklomanov, A. I., Suslova, A., Moatar, F., et al. (2023). Recent trends in the chemistry of major northern rivers signal widespread Arctic change. Nat. Geosci. 16, 789–796. doi:10.1038/s41561-023-01247-7
Tarling, G. A., Freer, J. J., Banas, N. S., Belcher, A., Blackwell, M., Castellani, C., et al. (2022). Can a key boreal Calanus copepod species now complete its life-cycle in the Arctic? Evidence and implications for Arctic food-webs. Ambio 51, 333–344. doi:10.1007/s13280-021-01667-y
Terhaar, J., Kwiatkowski, L., and Bopp, L. (2020). Emergent constraint on Arctic Ocean acidification in the twenty-first century. Nature 582, 379–383. doi:10.1038/s41586-020-2360-3
Terhaar, J., Lauerwald, R., Regnier, P., Gruber, N., and Bopp, L. (2021). Around one third of current Arctic Ocean primary production sustained by rivers and coastal erosion. Nat. Commun. 12, 169. doi:10.1038/s41467-020-20470-z
Thiemann, G. W., Iverson, S. J., and Stirling, I. (2008). Polar bear diets and arctic marine food webs: insights from fatty acid analysis. Ecol. Monogr. 78, 591–613. doi:10.1890/07-1050.1
Thoisen, C., Riisgaard, K., Lundholm, N., Nielsen, T. G., and Hansen, P. J. (2015). Effect of acidification on an arctic phytoplankton community from disko Bay, west Greenland. Mar. Ecol. Prog. Ser. 520, 21–34. doi:10.3354/meps11123
Thyrring, J., Wegeberg, S., Blicher, M. E., Krause-Jensen, D., Høgslund, S., Olesen, B., et al. (2021). Latitudinal patterns in intertidal ecosystem structure in West Greenland suggest resilience to climate change. Ecography 44, 1156–1168. doi:10.1111/ecog.05381
Timmermans, M.-L., and Marshall, J. (2020). Understanding arctic Ocean Circulation: a review of ocean dynamics in a changing climate. J. Geophys. Res. Oceans 125, e2018JC014378. doi:10.1029/2018JC014378
Tittensor, D. P., Novaglio, C., Harrison, C. S., Heneghan, R. F., Barrier, N., Bianchi, D., et al. (2021). Next-generation ensemble projections reveal higher climate risks for marine ecosystems. Nat. Clim. Chang. 11, 973–981. doi:10.1038/s41558-021-01173-9
Torres-Valdés, S., Tsubouchi, T., Bacon, S., Naveira-Garabato, A. C., Sanders, R., McLaughlin, F. A., et al. (2013). Export of nutrients from the Arctic Ocean. J. Geophys. Res. Oceans 118, 1625–1644. doi:10.1002/jgrc.20063
Torres-Valdés, S., Tsubouchi, T., Davey, E., Yashayaev, I., and Bacon, S. (2016). Relevance of dissolved organic nutrients for the Arctic Ocean nutrient budget. Geophys. Res. Lett. 43, 6418–6426. doi:10.1002/2016GL069245
Tremblay, J.-É., Anderson, L. G., Matrai, P., Coupel, P., Bélanger, S., Michel, C., et al. (2015). Global and regional drivers of nutrient supply, primary production and CO2 drawdown in the changing Arctic Ocean. Prog. Oceanogr. 139, 171–196. doi:10.1016/j.pocean.2015.08.009
Tremblay, J.-É., and Gagnon, J. (2009). “The effects of irradiance and nutrient supply on the productivity of Arctic waters: a perspective on climate change,” in Influence of climate change on the changing arctic and sub-arctic conditions. Editors J. C. J. Nihoul, and A. G. Kostianoy (Dordrecht: Springer Netherlands), 73–93. doi:10.1007/978-1-4020-9460-6_7
Tremblay, J.-É., Hattori, H., Michel, C., Ringuette, M., Mei, Z.-P., Lovejoy, C., et al. (2006). Trophic structure and pathways of biogenic carbon flow in the eastern North Water Polynya. Prog. Oceanogr. 71, 402–425. doi:10.1016/j.pocean.2006.10.006
Trudnowska, E., Szczucka, J., Hoppe, L., Boehnke, R., Hop, H., and Blachowiak-Samolyk, K. (2012). Multidimensional zooplankton observations on the northern west spitsbergen shelf. J. Mar. Syst. 98 (99), 18–25. doi:10.1016/j.jmarsys.2012.03.001
Tuerena, R. E., Mahaffey, C., Henley, S. F., de la Vega, C., Norman, L., Brand, T., et al. (2022). Nutrient pathways and their susceptibility to past and future change in the Eurasian Arctic Ocean. Ambio 51, 355–369. doi:10.1007/s13280-021-01673-0
Ulrich, K. L., and Tallman, R. F. (2021). The Capelin invasion: evidence for a trophic shift in Arctic Char populations from the Cumberland Sound region, Nunavut, Canada. Arct. Sci. 7, 413–435. doi:10.1139/as-2020-0001
van de Poll, W. H., Abdullah, E., Visser, R. J. W., Fischer, P., and Buma, A. G. J. (2020). Taxon-specific dark survival of diatoms and flagellates affects Arctic phytoplankton composition during the polar night and early spring. Limnol. Oceanogr. 65, 903–914. doi:10.1002/lno.11355
Van Engeland, T., Bagøien, E., Wold, A., Cannaby, H. A., Majaneva, S., Vader, A., et al. (2023). Diversity and seasonal development of large zooplankton along physical gradients in the Arctic Barents Sea. Prog. Oceanogr. 216, 103065. doi:10.1016/j.pocean.2023.103065
Van Hemert, C., Harley, J. R., Baluss, G., Smith, M. M., Dusek, R. J., Lankton, J. S., et al. (2022). Paralytic shellfish toxins associated with Arctic Tern mortalities in Alaska. Harmful Algae 117, 102270. doi:10.1016/j.hal.2022.102270
van Leeuwe, M. A., Tedesco, L., Arrigo, K. R., Assmy, P., Campbell, K., Meiners, K. M., et al. (2018). Microalgal community structure and primary production in Arctic and Antarctic sea ice: a synthesis. Elem. Sci. Anthropocene 6, 4. doi:10.1525/elementa.267
Verde, C., Giordano, D., Bellas, C. M., di Prisco, G., and Anesio, A. M. (2016). “Chapter four - polar marine microorganisms and climate change,” in Advances in microbial physiology. Editor R. K. Poole (Academic Press), 187–215. doi:10.1016/bs.ampbs.2016.07.002
Vermeij, G. J., and Roopnarine, P. D. (2008). The coming arctic invasion. Science 321, 780–781. doi:10.1126/science.1160852
Vihtakari, M., Welcker, J., Moe, B., Chastel, O., Tartu, S., Hop, H., et al. (2018). Black-legged kittiwakes as messengers of atlantification in the arctic. Sci. Rep. 8, 1178. doi:10.1038/s41598-017-19118-8
Villarino, E., Chust, G., Licandro, P., Butenschön, M., Ibaibarriaga, L., Larrañaga, A., et al. (2015). Modelling the future biogeography of North Atlantic zooplankton communities in response to climate change. Mar. Ecol. Prog. Ser. 531, 121–142. doi:10.3354/meps11299
von Biela, V. R., Laske, S. M., Stanek, A. E., Brown, R. J., and Dunton, K. H. (2023). Borealization of nearshore fishes on an interior Arctic shelf over multiple decades. Glob. Change Biol. 29, 1822–1838. doi:10.1111/gcb.16576
Waga, H., Hirawake, T., and Grebmeier, J. M. (2020). Recent change in benthic macrofaunal community composition in relation to physical forcing in the Pacific Arctic. Polar Biol. 43, 285–294. doi:10.1007/s00300-020-02632-3
Wassmann, P., Carmack, E. C., Bluhm, B. A., Duarte, C. M., Berge, J., Brown, K., et al. (2020). Towards a unifying pan-arctic perspective: a conceptual modelling toolkit. Prog. Oceanogr. 189, 102455. doi:10.1016/j.pocean.2020.102455
Wassmann, P., Kosobokova, K. N., Slagstad, D., Drinkwater, K. F., Hopcroft, R. R., Moore, S. E., et al. (2015). The contiguous domains of Arctic Ocean advection: trails of life and death. Prog. Oceanogr. 139, 42–65. doi:10.1016/j.pocean.2015.06.011
Wassmann, P., Reigstad, M., Haug, T., Rudels, B., Carroll, M. L., Hop, H., et al. (2006a). Food webs and carbon flux in the Barents Sea. Prog. Oceanogr. 71, 232–287. doi:10.1016/j.pocean.2006.10.003
Wassmann, P., Slagstad, D., Riser, C. W., and Reigstad, M. (2006b). Modelling the ecosystem dynamics of the Barents Sea including the marginal ice zone: II. Carbon flux and interannual variability. J. Mar. Syst. 59, 1–24. doi:10.1016/j.jmarsys.2005.05.006
Węsławski, J. M., Dragańska-Deja, K., Legeżyńska, J., and Walczowski, W. (2018). Range extension of a boreal amphipod Gammarus oceanicus in the warming Arctic. Ecol. Evol. 8, 7624–7632. doi:10.1002/ece3.4281
Weydmann, A., Carstensen, J., Goszczko, I., Dmoch, K., Olszewska, A., and Kwasniewski, S. (2014). Shift towards the dominance of boreal species in the Arctic: inter-annual and spatial zooplankton variability in the West Spitsbergen Current. Mar. Ecol. Prog. Ser. 501, 41–52. doi:10.3354/meps10694
Whitehouse, G. A., Aydin, K. Y., Hollowed, A. B., Holsman, K. K., Cheng, W., Faig, A., et al. (2021). Bottom–up impacts of forecasted climate change on the eastern Bering Sea food web. Front. Mar. Sci. 8, 624301. doi:10.3389/fmars.2021.624301
Wiedmann, I., Ceballos-Romero, E., Villa-Alfageme, M., Renner, A. H. H., Dybwad, C., van der Jagt, H., et al. (2020). Arctic observations identify phytoplankton community composition as driver of carbon flux attenuation. Geophys. Res. Lett. 47, e2020GL087465. doi:10.1029/2020GL087465
Wildes, S., Whittle, J., Nguyen, H., Marsh, M., Karpan, K., D’Amelio, C., et al. (2022). Walleye Pollock breach the Bering Strait: a change of the cods in the arctic. Deep Sea Res. Part II Top. Stud. Oceanogr. 204, 105165. doi:10.1016/j.dsr2.2022.105165
Williams, P. L., Burgess, D. O., Waterman, S., Roberts, M., Bertrand, E. M., and Bhatia, M. P. (2021). Nutrient and carbon export from a tidewater glacier to the coastal ocean in the Canadian arctic Archipelago. J. Geophys. Res. Biogeosciences 126, e2021JG006289. doi:10.1029/2021JG006289
Williams, W. J., and Carmack, E. C. (2015). The ‘interior’ shelves of the Arctic Ocean: physical oceanographic setting, climatology and effects of sea-ice retreat on cross-shelf exchange. Prog. Oceanogr. 139, 24–41. doi:10.1016/j.pocean.2015.07.008
Winter, A., Henderiks, J., Beaufort, L., Rickaby, R. E. M., and Brown, C. W. (2014). Poleward expansion of the coccolithophore Emiliania huxleyi. J. Plankton Res. 36, 316–325. doi:10.1093/plankt/fbt110
Wisz, M. S., Broennimann, O., Grønkjær, P., Møller, P. R., Olsen, S. M., Swingedouw, D., et al. (2015). Arctic warming will promote Atlantic–Pacific fish interchange. Nat. Clim. Change 5, 261–265. doi:10.1038/nclimate2500
Wold, A., Hop, H., Svensen, C., Søreide, J. E., Assmann, K. M., Ormanczyk, M., et al. (2023). Atlantification influences zooplankton communities seasonally in the northern Barents Sea and Arctic Ocean. Prog. Oceanogr. 219, 103133. doi:10.1016/j.pocean.2023.103133
Wood, H. L., Spicer, J. I., Kendall, M. A., Lowe, D. M., and Widdicombe, S. (2011). Ocean warming and acidification; implications for the Arctic brittlestar Ophiocten sericeum. Polar Biol. 34, 1033–1044. doi:10.1007/s00300-011-0963-8
Wood, K. R., Bond, N. A., Danielson, S. L., Overland, J. E., Salo, S. A., Stabeno, P. J., et al. (2015). A decade of environmental change in the Pacific Arctic region. Prog. Oceanogr. 136, 12–31. doi:10.1016/j.pocean.2015.05.005
Woodgate, R. A. (2018). Increases in the Pacific inflow to the Arctic from 1990 to 2015, and insights into seasonal trends and driving mechanisms from year-round Bering Strait mooring data. Prog. Oceanogr. 160, 124–154. doi:10.1016/j.pocean.2017.12.007
Woodgate, R. A., Weingartner, T., and Lindsay, R. (2010). The 2007 Bering Strait oceanic heat flux and anomalous Arctic sea-ice retreat. Geophys. Res. Lett. 37. doi:10.1029/2009GL041621
Xu, G., Rencurrel, M. C., Chang, P., Liu, X., Danabasoglu, G., Yeager, S. G., et al. (2024). High-resolution modelling identifies the Bering Strait’s role in amplified Arctic warming. Nat. Clim. Chang. 14, 615–622. doi:10.1038/s41558-024-02008-z
Yool, A., Popova, E. E., and Coward, A. C. (2015). Future change in ocean productivity: is the Arctic the new Atlantic? J. Geophys. Res. Oceans 120, 7771–7790. doi:10.1002/2015JC011167
Yunda-Guarin, G., Brown, T. A., Michel, L. N., Saint-Béat, B., Amiraux, R., Nozais, C., et al. (2020). Reliance of deep-sea benthic macrofauna on ice-derived organic matter highlighted by multiple trophic markers during spring in Baffin Bay, Canadian Arctic. Elem. Sci. Anthropocene 8, 047. doi:10.1525/elementa.2020.047
Yurkowski, D. J., Brown, T. A., Blanchfield, P. J., and Ferguson, S. H. (2020). Atlantic walrus signal latitudinal differences in the long-term decline of sea ice-derived carbon to benthic fauna in the Canadian Arctic. Proc. R. Soc. B Biol. Sci. 287, 20202126. doi:10.1098/rspb.2020.2126
Yurkowski, D. J., Ferguson, S., Choy, E. S., Loseto, L. L., Brown, T. M., Muir, D. C. G., et al. (2016a). Latitudinal variation in ecological opportunity and intraspecific competition indicates differences in niche variability and diet specialization of Arctic marine predators. Ecol. Evol. 6, 1666–1678. doi:10.1002/ece3.1980
Yurkowski, D. J., Ferguson, S. H., Semeniuk, C. A. D., Brown, T. M., Muir, D. C. G., and Fisk, A. T. (2016b). Spatial and temporal variation of an ice-adapted predator’s feeding ecology in a changing Arctic marine ecosystem. Oecologia 180, 631–644. doi:10.1007/s00442-015-3384-5
Zakharov, D. V., Jørgensen, L. L., Manushin, I. E., and Strelkova, N. A. (2020). Barents Sea megabenthos: spatial and temporal distribution and production. mbj 5, 19–37. doi:10.21072/mbj.2020.05.2.03
Zhang, R., Song, P., Li, H., Wang, R., Li, Y., Miao, X., et al. (2022). Spatio-temporal characteristics of demersal fish community in the Chukchi and northern Bering Seas: significant distributional records and interannual variations in species composition and biodiversity. Polar Biol. 45, 259–273. doi:10.1007/s00300-021-02980-8
Zhuang, Y., Jin, H., Cai, W.-J., Li, H., Jin, M., Qi, D., et al. (2021). Freshening leads to a three-decade trend of declining nutrients in the western Arctic Ocean. Environ. Res. Lett. 16, 054047. doi:10.1088/1748-9326/abf58b
Keywords: Climate change, Arctic, biodiversity, ecosystem functioning, species distribution, Blue Growth
Citation: Husson B, Bluhm BA, Cyr F, Danielson SL, Eriksen E, Fossheim M, Geoffroy M, Hopcroft RR, Ingvaldsen RB, Jørgensen LL, Lovejoy C, Meire L, Mueter F, Primicerio R and Winding M (2024) Borealization impacts shelf ecosystems across the Arctic. Front. Environ. Sci. 12:1481420. doi: 10.3389/fenvs.2024.1481420
Received: 15 August 2024; Accepted: 14 October 2024;
Published: 24 October 2024.
Edited by:
Torben Røjle Christensen, Aarhus University, DenmarkReviewed by:
Jan Kavan, Masaryk University, CzechiaOran Young, University of California, Santa Barbara, United States
Copyright © 2024 Husson, Bluhm, Cyr, Danielson, Eriksen, Fossheim, Geoffroy, Hopcroft, Ingvaldsen, Jørgensen, Lovejoy, Meire, Mueter, Primicerio and Winding. This is an open-access article distributed under the terms of the Creative Commons Attribution License (CC BY). The use, distribution or reproduction in other forums is permitted, provided the original author(s) and the copyright owner(s) are credited and that the original publication in this journal is cited, in accordance with accepted academic practice. No use, distribution or reproduction is permitted which does not comply with these terms.
*Correspondence: Bérengère Husson, YmVyZW5nZXJlLmh1c3NvbkBoaS5ubw==