- 1Animal and Grassland Research and Innovation Centre, Teagasc Moorepark, Fermoy, Ireland
- 2Civil Engineering and Ryan Institute, College of Science and Engineering, University of Galway, Galway, Ireland
- 3Environmental Research Centre, Teagasc Johnstown Castle, Wexford, Ireland
Introduction: On intensive grassland dairy farms in high rainfall areas with poorly drained soils, networks of open drainage channels linked to in-field drainage systems are needed to enable farm operations. Nitrogen and phosphorus point and diffuse sources may be connected to this open drainage channel network along surface and subsurface pathways, with negative impacts upon delivery to the downstream aquatic system.
Methods: This study developed a semi-quantitative risk assessment model by: (1) selecting parameters (categorical or continuous) representing the nutrient transfer continuum and (2) scoring (relative magnitude and impact) the risk of nutrient source connectivity and delivery for every open drainage channel section across seven dairy farms.
Results and Discussion: A Risk Index Classification System consisting of low, medium, high, or very high-risk class was developed, with high or above requiring a mitigation plan. Results showed that 23%, 68%, 9% and 0% of all open drainage channels on study farms were identified as low, moderate, high and very high-risk, respectively. A range from 2% to 25% per farm of the open drainage channels was classified as high-risk that potentially needed mitigation, although none was identified as very high-risk. Two-thirds of the high-risk open drainage channels were connected to the farmyards, with potential for high nutrient loss from point sources. A combined approach of source management and targeted breaking of the pathway (e.g., in-channel filters, water diversion bars) may help minimise nutrient losses from high risk open drainage channels on poorly draining soils.
1 Introduction
Agricultural landscapes in areas of high annual precipitation and heavy textured soils are characterised by high densities of open drainage channels, which provide outfalls for in-field drainage systems (Shore et al., 2015; Tuohy et al., 2018). Open drainage channels, comprising drainage ditches and smaller streams, are networked to collect and drain away excess water from different parts of a farm to larger water courses (Kröger et al., 2007). Within the open drainage channel network, streams exist as intermittent or perennial natural channels, whereas drainage ditches exist as man-made channels that may be intermittent or perennial, depending on their landscape position and their interplay with subsurface water and groundwater. These open drainage channels perform many functions (Daly et al., 2017; Ezzati et al., 2020) including storage and release of nutrients by sediments, transportation and interception of farm surface and subsurface runoff which may carry nutrients to the larger water courses.
It is important to minimise the source of nutrients and intercept instantaneous and legacy nutrients from farms in high rainfall areas (Fenton et al., 2021; Peyton et al., 2016; Valbuena-Parralejo et al., 2019). In these areas, open drainage channels form an integral part of the source, mobilisation, pathway, and receptor (S-M-P-R) component of the nutrient transfer continuum (Haygarth et al., 2005) (defined as the framework that captures the nutrient-loss influencing factors from source to receptor). Water drained in both natural and man-made open drainage channels may be nutrient-rich from different nutrient sources that are mobilised through point (e.g., farmyard (Martínez-Suller et al., 2010; Vero et al., 2020), farm roadway (Fenton et al., 2021; Rice et al., 2022) and diffuse (Daly et al., 2017; Roberts et al., 2017)) sources. Where hydrological connectivity exists with the surrounding environment, nutrients from these sources travel through different pathways (Wall et al., 2011) to enter open drainage channels. The nutrients are either transformed or remain unchanged along the pathway to the open drainage channel, before being transported to the adjoining waterways (Clagnan et al., 2018). Aside from nutrient transformation, these nutrients can be buffered and/or retained to prevent connectivity losses as they go through the processes and pathways (Deelstra et al., 2014). Understanding the nutrient dynamics and loss risks occurring within an open drainage channel system is critical to assessing, managing and mitigating nutrient losses from farms (Collins et al., 2016; Herzon and Helenius, 2008).
Moloney et al. (2020) ranked connectivity risk for phosphorus (P) loss along man-made open drainage channels and showed that varying levels of connectivity to nutrient source, depending on their geographical position, exist between man-made open drainage channels and surface waters. The highest to lowest connectivity for P loss was as follows: farmyard connection ditch, outlet (a ditch that connects the drainage network to a surface water body), outflow (a ditch that carries drainage water across the farm boundary through neighbouring land), secondary, or disconnected ditch. Opoku et al. (2024) further developed this concept by creating an integrated (i.e., P and nitrogen (N)) ranked connectivity risk incorporating nutrient loss from sources within open drainage channels. That study showed that other factors, i.e., farm management practices, landscape characteristics, and surface and subsurface hydrological connectivity of directly connecting areas, described the risk of P and N loss in categories of man-made open drainage channels. These factors vary spatially and temporally (Harrison et al., 2019; Mellander et al., 2017; Withers and Lord, 2002), even in a very small distance (Adams et al., 2022), and therefore may vary in the nutrient loss risk they pose for individual open drainage channels at different geographic locations on farm. Characterising these factors for individual open drainage channels is essential to assess the risk of connectivity for nutrient losses from an open drainage channel network, but is not well studied. In previous nutrient loss risk studies, open drainage channels were risk assessed largely as a (transport) pathway factor for nutrient loss based on either their presence, density, connectivity to high-risk fields or sloping conditions (Buczko and Kuchenbuch, 2007; Magette et al., 2007; Roberts et al., 2017; Schoumans and Chardon, 2003), thereby limiting a holistic assessment (Granger et al., 2010). Furthermore, in studies where these factors have been used in assessing farm nutrient loss connectivity (Deelstra et al., 2014; Gramlich et al., 2018), their influences on connectivity to open drainage channels under their respective nutrient transfer continuum sections to enable complete understanding of their nutrient loss risks (Haygarth et al., 2005; Murphy et al., 2015) and improve regulations (Wall et al., 2011) have not been evaluated. Such an evaluation could be achieved by exploring a risk assessment of the factors under the nutrient transfer continuum of open drainage channels and may allow mitigation efforts to be optimised to prevent nutrient losses to open drainage channels and transfer to adjoining water bodies.
Risk assessment provides an overall appraisal of the connectivity components for each element (S-M-P-R) of the nutrient transfer continuum to inform their combined implications and relationships for nutrient loss to open drainage channels on farms (Jordan et al., 2005). Risk can be assessed quantitatively (where data are sufficient; Adkin et al., 2014), qualitatively (where data are insufficient; Nag et al., 2020), and semi-quantitatively (a blend of the two, e.g., Rice et al., 2022)). Subjective expert judgment may be used to approximate risk values to inform decision-making (Redmill, 2002; Rice et al., 2022). Different assessment approaches to identify and characterise landscape hotspots for nutrient losses have been documented. These include direct nutrient concentration measurements in open drainage channels (Ezzati et al., 2020; Mattila and Ezzati, 2022), a combination of some nutrient transfer continuum parameters (Alder et al., 2015; Hayes et al., 2023; Fenton et al., 2022), or predictive models (Radcliffe et al., 2015; Vadas et al., 2007; Vadas et al., 2015). A risk assessment to identify open drainage channel sections associated with high-risk nutrient runoff connectivity using all possible field management data, and landscape and hydrological connectivity data across the nutrient transfer continuum for heavy textured farms has not been developed to date. Undertaking an appraisal incorporating these elements will help identify and rank high-risk areas (also known as critical source areas; McDowell et al., 2024) on the open drainage channel network for heavy textured grassland dairy farms for targeted mitigation.
The objective of this study was to develop a semi-quantitative risk model for heavy textured grassland dairy farms that identifies open drainage channel network sections that pose a risk of contributing nutrients to the adjoining aquatic water courses and which require mitigation. Instead of considering only nutrient source connectivity to classify open drainage channel risks for nutrient losses (as in Opoku et al. (2024)), the current study builds on this theory and captures all relevant S-M-P-R factors under the open drainage network nutrient transfer continuum to rank the nutrient loss risk in the open drainage channel network on a farm. To conduct this research, data were collected during field and desk-based studies across seven heavy textured grassland farms in Ireland. These farms are considered representative of heavy textured, poorly draining soils in Ireland, all receive high rainfall and were subjected to high-resolution data collection on a vast range of static and dynamic variables related to farm management.
2 Materials and methods
2.1 Nutrient transfer continuum framework
A semi-quantitative risk assessment model was developed based on seven intensive grassland heavy textured dairy farms. Using expert opinion and the literature, various parameters that best describe the nutrient transfer continuum between a source and an open drainage channel network (Dollinger et al., 2015; Kleinman et al., 2011; Needelman et al., 2007) were collated and categorised into S-M-P-R components as in Table 1.
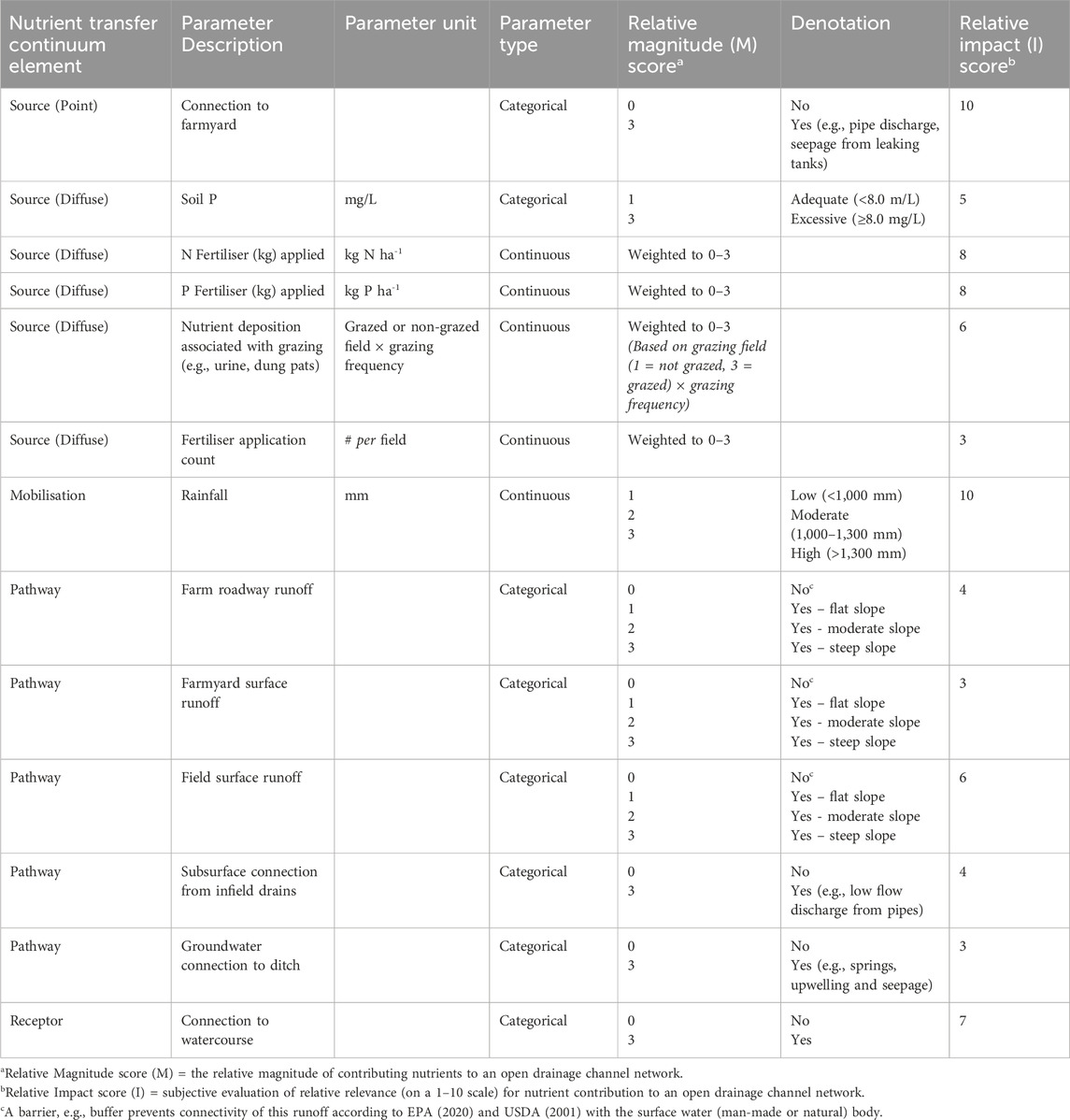
Table 1. Nutrient transfer continuum element, parameter description, units, type, relative magnitude score, relative impact score, and denotation.
2.1.1 Justification to S-M-P-R parameters
2.1.1.1 Source
In a nutrient loss risk assessment, identifying potential sources and their characteristics is critical (Carton et al., 2008; McDowell et al., 2024). Farmyards are largely associated with potential nutrient sources, and connection to them imposes high-risk of direct or indirect discharges of point source nutrients into the open drainage channel network (Moloney et al., 2020; Opoku et al., 2024; Vero et al., 2020). Soil P status of fields directly connected to open drainage channels offers a potential source contribution of soil nutrients that can be readily lost, and dictates the amount of P that can be applied in a mineral or organic soil (Moloney et al., 2020), and is therefore essential as a source parameter. The organic matter proportion in mineral and organic soils determines the adsorption or repulsion of dissolved nutrients unto soil particles (Roberts et al., 2017; Tejada and Gonzalez, 2008) and therefore influences the soil P status. Soil P Indices of 1, 2 and 3 are defined as low risk, while index 4 is defined as high-risk, with all organic soils categorised as index 4 by default (Daly, 2005; Wall and Plunkett, 2016). The amount of P and N fertiliser (kg) applied is one of the major nutrient sources that influences surface and subsurface nutrient losses in open drainage channels (Hart et al., 2004; Ibrahim et al., 2013; Richards et al., 2015; Watson and Foy, 2001). The rate of fertiliser application increases soluble reactive P (SRP) and total P (TP) concentrations in overland flow and drainage water (Watson et al., 2007). On these connecting fields, fertiliser application count is another source parameter that contributes nutrient loss to open drainage channels and may increase nutrient losses especially under wet soil conditions. The grazing status of a field connecting to open drainage channel specifies the risk of another major nutrient source that determines probability of livestock wastes (faeces and urine) being deposited near an open drainage channel (Bilotta et al., 2007; Gary et al., 1983; Hubbard et al., 2004) and damage to soils (that may be high nutrient rich) by trafficking and poaching to runoff into open drainage channels (Cassidy et al., 2017; Doody et al., 2014; Pietola et al., 2005). Its impact varies with grazing frequency (the number of times a grazing field is accessed by animals for grazing), with frequently grazed fields more susceptible to increase nutrient losses (Cassidy et al., 2017; Doody et al., 2014; Hubbard et al., 2004).
2.1.1.2 Mobilisation
Rainfall is the prime mobilising parameter that controls the transfer of nutrients within and around the open drainage channel (Pérez-Gutiérrez et al., 2020; Vadas et al., 2011; Yao et al., 2021).
2.1.1.3 Pathway
Farm roadways that are connected to open drainage channels under the nutrient transfer continuum acts as pathway by which runoff, carrying nutrients, is transferred into the open drain (Maher et al., 2023; Rice et al., 2022). Along the farm roadway network, nutrients may be contributed from the road surface (Davison et al., 2008; Edwards and Withers, 2008; Fenton et al., 2022). The farmyard is another pathway, which comprises hard standing areas that collect rainfall that becomes runoff to the adjacent open drainage channels (Edwards et al., 2008; Vero et al., 2020). The field surface influences runoff to connecting open drainage channels. Field surface is dependent of the soil drainage class (well, moderate, imperfect, and poorly-draining soils) and this dictates the runoff pathway between surface and subsurface pathways (Houlbrooke and Monaghan, 2009). There is high P loss risk from overland flow in poorly drained soils, moderate P loss risk from imperfectly drained soils, low P loss risk from both moderate and well-drained soils (Magette et al., 2007). The subsurface in-field drain pathway influences soil drainage capacity and subsequently the surface and subsurface pathways (Houlbrooke and Monaghan, 2009). Subsurface in-field drains enhance infiltration and other processes in soils (Opoku et al., 2024). Groundwater upwelling or seeping pathways introduces nitrate (NO3-N) and P into open drainage channels, but depends on many factors such as landscape position and soil type (Opoku et al., 2024). Groundwater composition may be high in nitrate concentrations, especially if the soil processes are modified by drainage (Edwards and Withers, 2008).
2.1.1.4 Receptor
The receptor is associated with the final direct impact on a watercourse (Wall et al., 2011). Watercourse in this regard is defined as any natural river, stream, or lake (but not a man-made drainage channel) (Department of Agriculture Food and the Marine, 2018) identifiable on an Ordnance Survey Ireland 6-inch map (www.osi.ie). In this study, all natural open drainage channels were assumed to have a final connection to a watercourse, with or without any proximity observed during the ground survey.
2.2 Scoring continuous and categorical parameters
The parameters were assigned individual risk scores that were scored arithmetically in a magnitude-impact matrix (Teunis and Schijven, 2019). For each open drainage channel, the risk score for every parameter was calculated by multiplying the score for magnitude (M) for contributing nutrients to an open drainage channel by the score for its relative impact (I) (Table 1) (after Shariff and Zaini, 2013).
Within the risk assessment, data for some parameters were measured quantitatively as continuous data (e.g., N fertiliser (kg) rate applied; Table 1), while others were assessed qualitatively as categorical data during field observation (e.g., connection to a farmyard; Table 1). As such, the M value for each parameter differed depending on the parameter type.
For continuous parameters, the M value was weighted between 0 and 3 using the formula (Equation 1):
where Xi is the on-farm observed data value; Xmin and Xmax are the minimum and maximum values observed across all farms.
For categorical parameters, the value was based on literature and/or expert judgement. Either “0” or “1” was scored as the “lowest” and “3” as the “highest” values (Table 1). For each open drainage channel, a total risk score was calculated by summing up all the risk scores for each continuous and/or categorical nutrient transfer continuum parameter for that open drainage channel. A total risk score represents the degree of risk (i.e., the scale of likelihood or propensity at which an open drainage channel contributes nutrients to a watercourse) associated with the blend of complex parameters (Table 1) for nutrient loss across all the open drainage channels on a given farm. Although the risk assessment takes into account the influence of the contributing area to an open drainage channel, the approach of weighting the contributions over the area rather than adding their impacts ensured an unbiased assessment where a larger area of fields surrounding the stretch of an open drainage channel could have led to high-risk. The risk assessment is simple to use, relying on easily accessible farm data, and can be used to assess the relative risk agricultural open drainage channels pose to water quality, without quantifying the nutrient loss.
2.3 Fieldwork to collect nutrient transfer continuum parameter data
Seven farms, dominated by heavy textured soils of a wide variety of bio-physical settings, were selected. These farms represented varying open drainage channel network density and connectivity risk compositions. During winter (November 2021 to March 2022), a field survey was conducted in which all open drainage channel networks were mapped as per Opoku et al. (2024) and Moloney et al. (2020). Open drainage channel network features such as connection to the farmyard, field slope, the proximity to water bodies, and connectivity pathways for nutrients into the open drainage channel network from in-field drains, farm roadways, groundwater springs, seepage and upwelling throughout the open drainage channel network, were noted on each farm. All the information characterising the open drainage channel network was recorded using an electronic device with ESRI ArcGIS Field Maps mobile software (version 21.4.0) (ESRI, 2024) during the field survey. This information was transferred to ‘geographic information system’ (GIS) mapping software, ArcMap GIS software (version 10.5). Data on other parameters for the nutrient transfer continuum elements was obtained from previous studies (Corbett et al., 2022a; Corbett et al., 2022b; Tuohy et al., 2021) and ongoing data collection by participating farmers and field agents. The data were downloaded and collated with data from the field survey, and the parameters in Table 1 were assigned an M score for every open drainage channel network across the farms.
In applying nutrient loss risk magnitude to areas that have never been calibrated, errors may prevail due to the unknowns in parameter settings and adjustments, and reliance on experts’ opinions to set model parameters without calibration (Sharpley et al., 2017). However, the adoption of systems that are assessed and approved (as suggested by Bhandari et al. (2017); Nelson et al. (2017)) enhanced the robust calibration of the parameters for the risk assessment.
2.4 Formation of risk classification system
Total risk score values for every open drainage channel for all seven farms were split into four categories of equal intervals to produce four potential risk classes (i.e., low risk, moderate risk, high-risk, and very high-risk). The range was determined by the possible highest and lowest score that could occur as per the risk assessment scoring system developed. The risk classes were developed by Equation 2:
where TRShigh and TRSlow are the highest and lowest total risk score values recorded across the seven farms, and I.e., is the interval between the four risk classes. These were colour-coded as green, yellow, orange, and red, respectively, on farm maps. Such maps provide information on the open drainage channels that are potential critical hotspots for nutrient losses on heavy-textured dairy farms. Risk classes in high and very high-risk ranges are identified as hotspots that may require mitigation measures.
2.5 Synoptic water sampling across dairy farms
Water quality parameters change over time, depending on the local climatic conditions and farming practices (Huebsch et al., 2013). At 105 sampling points throughout the drainage network across all farms, a total of 210 water samples (a pair of filtered and unfiltered at each sampling point) were collected during each season (sampling event) for 4 seasons (Spring (March) 2022 to Winter (January) 2023). The sampling was carried out across all 4 seasons to capture hydrological fluctuations and conditions, including surface and subsurface connectivity as per Opoku et al. (2024). As this study aimed to assess the risk of the open drainage channels, the water N and P chemistry only validated the potential nutrient losses from the open drainage channel network surroundings and did not aim to elucidate the load or impact of this connection. Except for disconnected ditches (which were mostly dry), all man-made open drainage channels (farmyard connection, outlet, outflow, and secondary ditches; Moloney et al., 2020) and natural open drainage channels were sampled. At each water sample location, two 50 mL samples (filtered on-site using 0.45 μm filter paper and unfiltered) were collected for dissolved and total P analyses, respectively. All water samples were kept in an ice box during sampling and transportation, and then tested within 1 day of sample collection.
Filtered water samples were analysed for dissolved reactive phosphorus (DRP) and total dissolved phosphorus (TDP) using a Gallery discrete analyser (Gallery reference manual, 2016) and a Hach Ganimede P analyser, respectively. Total dissolved phosphorus (TDP) was measured by acid persulphate oxidation, under high temperature and pressure. The unfiltered water samples were analysed for nitrite (NO2-N), NH4-N, total oxidised nitrogen (TON), and total reactive phosphorus (TRP) using a Gallery analyser. Total phosphorus was analysed using the Ganimede P analyser. Phosphorus was measured colourimetrically by the ascorbic acid reduction method (Askew and Smith, 2005), where the 12-molybdophosphoric acid complex is formed by the reaction of orthophosphate ion with ammonium molybdate and antimony potassium tartrate (catalyst) and reduced ascorbic acid. All samples, reagent blanks, and check standards were analysed following the Standard Methods (APHA, 2005). All quality control (QC) samples/check standards are made from certified stock standards from a different source than calibration standards. Quality control samples were analysed at the beginning and end of every batch, and every 10 samples within a batch, and if the QC fell outside limits, samples were repeated back to the last correct QC. Blanks were included in every batch and approximately 10% of samples were repeated. Tolerances range up to a maximum of ±7.5% of nominal value. All instruments used were calibrated in line with manufacturers’ recommendations. Nitrate-N was calculated by subtracting NO2-N from TON, particulate phosphorus (PP) was the difference between TP and TDP, and dissolved unreactive phosphorus (DUP) was the difference between TDP and DRP.
3 Results and discussion
3.1 Open drainage channel characteristics
The total length and the number of open drainage channels in the farms are shown in Table 2. The length of an open drainage channel characterised the field area of contribution influencing the connectivity and potential risk of nutrient loss to an open drainage channel. Opoku et al. (2024) reported that multiple connectivity pathways may exist on a single open drainage channel. Although the relationship between the presence of connectivity pathways in open drainage channels and the length of the open drainage channels was not assessed in that study, longer open drainage channel lengths may have high connectivity, resulting in a potentially higher risk of nutrient loss. However, other parameters such as soil chemistry (Daly et al., 2017; Ezzati et al., 2020), slope, design (Hodaj et al., 2017), and vegetation (Soana et al., 2017) may also influence nutrient loss.
3.2 Risk classification system
Table 3 presents the risk classification system ranges based on the minimum and maximum possible total risk score from the risk assessment scoring system. These risk classification ranges were the basis on which risk class output maps for open drainage channel networks on each farm were developed (Figure 1).
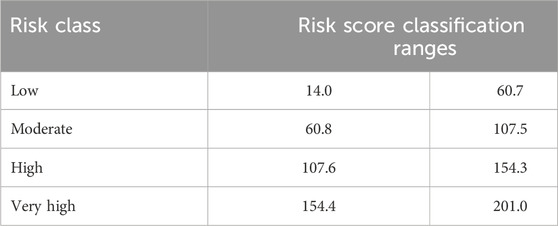
Table 3. Risk classification system (risk class and score ranges) for risk assessment model for open drainage channels on heavy textured dairy farms.
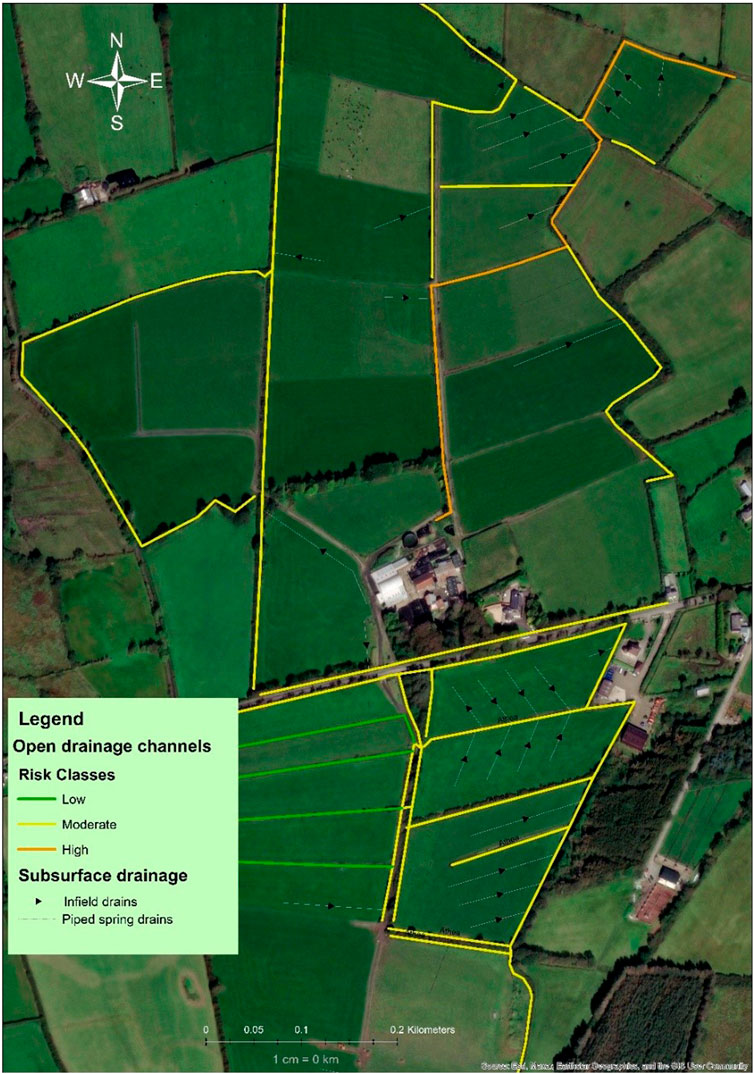
Figure 1. A map of a heavy textured grassland dairy farm (Farm #1 from Table 2) showing the risk classes of the open drainage channel network.
Although the possible lowest and highest total risk score are 14.0 and 201.0 according to the risk assessment scoring system (Table 3), the actual lowest and highest total risk scores recorded for the open drainage channels for the farms studied were 35.9 (Farm 4) and 144.4 (Farm 4), respectively. This indicates the highest total risk score across the farms reached only about 72% of the potential maximum total risk score. Of the 171 open drainage channels on all seven farms, 23%, 68%, 9%, and 0% were ranked as low, moderate, high, and very high-risk classes, respectively (Figure 2). Data from individual farms were similar to the overall trend (Figure 2), except for Farm 6, where the majority (57%) of the open drainage channels ranked as low-risk.
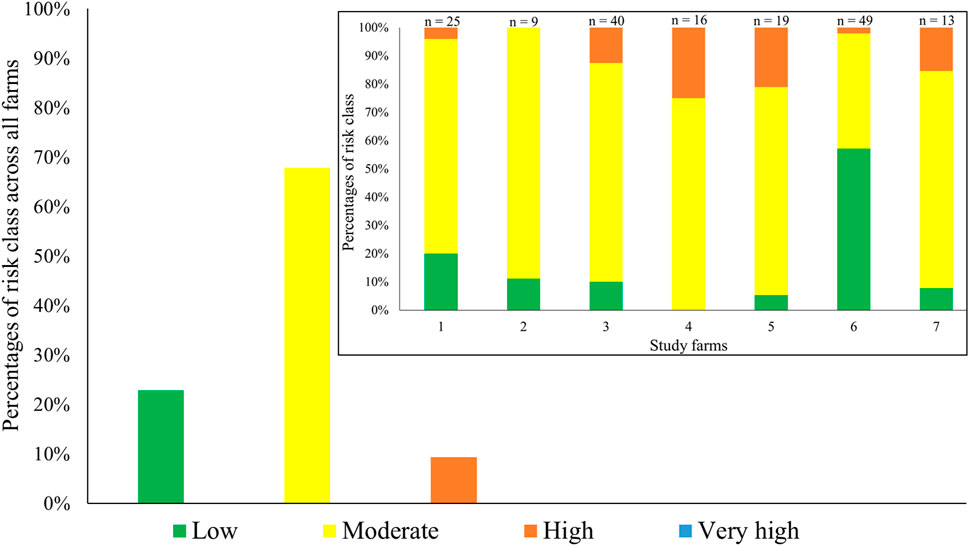
Figure 2. Percentages of risk classes for open drainage channels across all farms and within farms (inset).
Across the high-risk open drainage channels, the total risk score varied, with 144.4 being the highest recorded (a farmyard connection ditch) on Farm 4 and 109.9 being the lowest (a farmyard connection ditch) on Farm 7. The 9% high-risk open drainage channels across the study farms were mostly on farmyard connection and outlet ditches (Table 4). This result is similar to Opoku et al. (2024) and Moloney et al. (2020), who found that farmyard connection ditches were potentially the riskiest.
Agricultural pressures on waterbodies in Ireland are associated with excess nutrients, mainly present as NO3-N or DRP (EPA, 2023a). Phosphorus dominates in poorly drained soils, such as those included in this study, while N loss is more likely to vary depending on other specific site conditions (EPA, 2023a). In Ireland, the EPA considers good water in rivers to have NO3-N concentrations of less than 1.8 mg L-1 and DRP concentrations of less than 0.035 mg P L-1 (EPA, 2023b). While open drainage channels assessed in these study farms are different water bodies from rivers as defined on national ordnance survey maps (6-inch maps) (www.osi.ie), comparisons of NO3-N and DRP concentrations on the open drainage channels with the water quality standards for rivers act as a guide to show if a water sample is high or low.
The annual mean DRP concentrations in the open drainage channels, which ranged from 0.09 mg L-1 in moderate-risk class to 0.40 mg L-1 in high-risk class (Figure 3), were higher than the surface water standard of 0.035 mg L-1. The annual mean NO3-N concentrations on the open drainage channels were lower across the risk classes, with ranges of 0.59 mg L-1 in low-risk class to 1.18 mg L-1 in moderate-risk class (Figure 3) relative to the standard of 1.8 mg NO3-N L-1. This is consistent with the poorly draining conceptual model of the EPA in Ireland, as P losses dominate nutrients relative to N losses. While this may be beyond the scope of the present study, 32% of sampling locations had high NO3-N concentrations, indicating the N connectivity pathways that may be introducing NO3-N into these open drainage channels (Opoku et al., 2024). Average P and N concentrations per risk class increased as the risk of the open drainage channels increased, except for average P concentrations for the moderate-risk class (Figure 3). This could be due to the anthropogenic and natural characteristics that create hydrochemical variation in the farm landscapes that contribute nutrients to the open drainage channels. With this caveat, this showed that the water quality seasonal grab samples validated the total risk score.
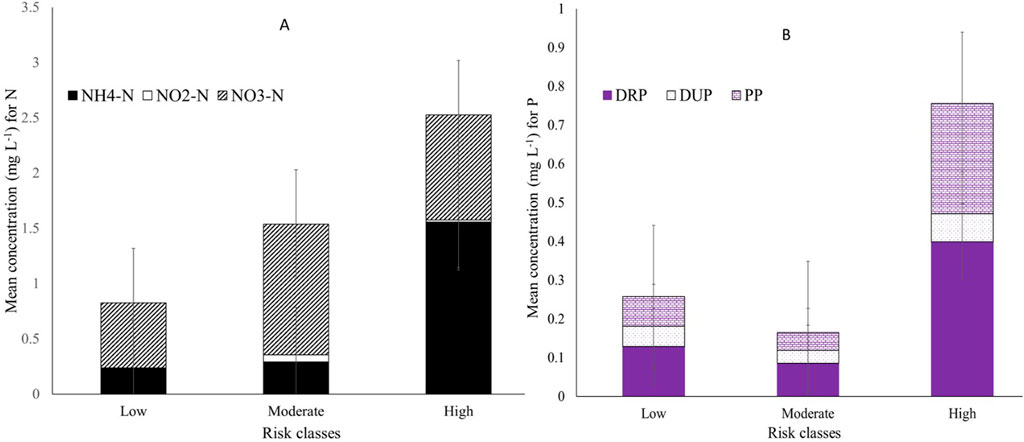
Figure 3. (A) Nitrogen and (B) phosphorus mean plus standard error concentrations from seasonal water sampling from within open drainage channels as per the risk classes across the case study farms.
3.3 Assessment of the nutrient transfer continuum elements on the open drainage channels
The contribution of the source to the average total risk score of open drainage channels per farm ranged from 44.2% (Farm 2) to 63.5% (Farm 5) (Figure 4). Similarly, the contribution of the source to the total risk score of each of the high-risk open drainage channels ranged from 40.3%–70.2% (Figure 5).
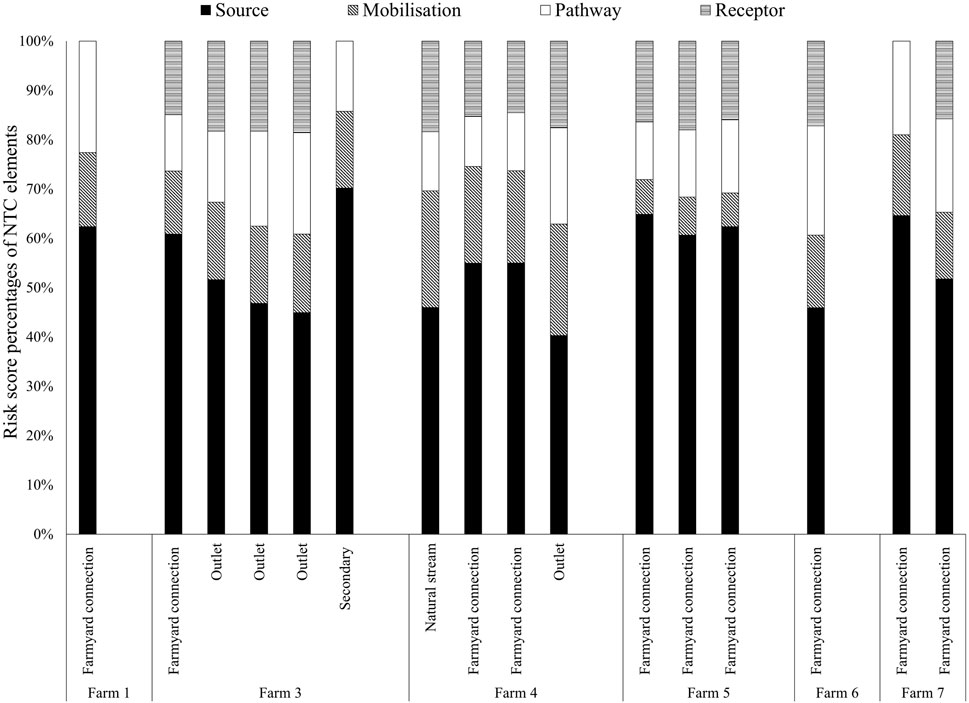
Figure 5. Risk score percentages of nutrient transfer continuum elements for farms with high-risk open drainage channels, excluding Farm 2 which had no high-risk open drainage channels.
The high proportion for source total risk score indicates that the multiple sources of nutrients, either from connection to farmyard, legacy soil P, fertiliser application, and grazing input parameters, primarily influenced the risk of nutrient losses (Cassidy et al., 2017; Moloney et al., 2020) to these open drainage channels. While most (62.5%) of the high-risk open drainage channels connect farmyards, the high-risk open drainage channel with the highest source contribution to a total risk score recorded (70.2%) (i.e., secondary ditch on Figure 5) had no farmyard connection. This could be attributed to the open drainage channel’s connectivity with high soil P-status fields, which received high fertiliser application for the duration of this study. This, together with surface and subsurface sources, may have led to the high total risk score on the other 37.5% of the whole high-risk open drainage channels with no connection to farmyards.
Along a connected pathway to the open drainage channel, the mobilisation of nutrients from the source was integral in most of the open drainage channels. The percentage of mobilisation contribution to the average total risk score of the open drainage channels per farm ranged from 10.2% to 31.5% (Figure 4). Rainfall is the primary factor by which mobilisation occurs for nutrient losses (Wang et al., 2020). Rainfall characteristics, including the intensity, duration and frequency, may influence the hydrological conditions that are critical to the surface and subsurface nutrient movement (Pérez-Gutiérrez et al., 2020). This necessitates the need to break the pathway to prevent the mobilised nutrient from the source to the receptor.
Nutrients enter the open drainage channels through multiple (surface, shallow subsurface and groundwater) pathways. The pathway contribution to the average total risk score per farm ranged from 10.5% to 18.4% (Figure 4). Heavy textured farms have multiple subsurface and surface connectivity pathways through which nutrients are lost (Clagnan et al., 2019; Granger et al., 2010; Opoku et al., 2024), and these may have contributed to the high-risk open drainage channels. Eighteen-point-six percent and 18.6% of the high-risk open drainage channels received risk scores from roadway and farmyard runoff surface connectivity pathways to the total risk score, respectively, while 87.5% and 31.3% of the high-risk open drainage channels received risk scores from in-field drains and groundwater subsurface connectivity pathways, respectively. Although the pathway percentage contribution to the total risk score of the high-risk open drainage channels ranged from 10.1%–22.6%, the highest pathway contribution to total risk score for an open drainage channel was 44.9% which was a moderate-risk open drainage channel on Farm 6.
The connection to the receptor was not present on all high-risk open drainage channels. However, contributions from 14.5% to 18.6% of the total risk score of high-risk open drainage channels with connection to receptor for the study farms (Figure 5). This informs the importance of considering the delivery of the final nutrient loss through the open drainage channels and may inform the mitigation type.
3.4 Mitigation of the high-risk open drainage channels
In Ireland, the EU Nitrates Directive is implemented through the Nitrates Action Plan (NAP), which applies to all farms in the country. This programme of measures outlines best farming practices to achieve good water quality outcomes for different farm enterprises. The EPA in Ireland identifies “breaking the pathway” on poorly draining soils, such as those in the present study, as an effective way to break the connectivity of surface or near-surface runoff between sources and waters. Opoku et al. (2024) classified the open drainage channel network into different ditch categories. Building on this work, the present study identifies open drainage channel sections within these large networks to be of higher risk and which may need mitigation. A combination of targeted measures is therefore necessary to improve water quality. This may include (1) source management (2) breaking the pathway (stopping runoff or near-runoff being delivered to waters), and (3) installation of in-channel filters (to slow the flow and attenuate a proportion of nutrients in dissolved and particulate forms from discharging through that open drainage channel section). On poorly draining soils this combined treatment train (Bourke et al., 2022) may prevent high nutrient-content water discharging from high-risk open drainage channel sections to the broader aquatic environment. Scrutiny of individual high-risk total risk score for different open drainage channel sections enables an advisor and farmer to identify specific sources, pathways, and in-channel actions as required. These may differ due to site-specific factors and cannot therefore be generic. Farmers are more inclined to accept less costly measures (van den Berg et al., 2023), and therefore these should be considered during the selection of mitigation measures (McDowell et al., 2024; King et al., 2015).
Opoku et al. (2024) and Fenton et al. (2021) detailed potential mitigation measures and costs available in terms of “break the pathway” mitigation options and costs. A few examples include: re-directing runoff away from internal roadways and the farmyard to collection or buffer areas with low-cost diversion bars or water bars (Fenton et al., 2021); installation of riparian (spatially targeted and linear) buffers along natural streams (Stutter et al., 2021) to control nutrient losses from the upslope field and connected internal farm roadways (Palmer, 2012; Yuan et al., 2009); targeted engineered mitigation measures including low-grade weirs (Faust et al., 2018), bunded drains, filter cells (Teagasc, 2022); and management of in-channel sediments through maintenance or characterisation of soil/sub-soil layer chemistry (Shore et al., 2015), which is both a sink and source of nutrients (Daly et al., 2017).
4 Conclusion
Assessments of nutrient loss from open drainage channels on poorly draining (heavy textured) soils are largely associated with predictions of surface runoff from critical hotspots. The risk assessment developed in this study combines potential water quality impacts from surface, subsurface, and groundwater characteristics of connecting fields to produce a colour-coded model of different potential water quality risk levels by which open drainage channels can be risk assessed. This risk assessment enables the production of risk maps that identify potential high- or very-high risk open drainage channels on dairy farms with heavy textured soils and assesses the nutrient transfer continuum elements to inform mitigation. Unlike previous open drainage channel risk assessment studies of Moloney et al. (2020) and Opoku et al. (2024), this study critically assesses all the source-mobilisation-pathway-receptor multi-parameters of the open drainage channel nutrient transfer continuum framework, provides in-depth information regarding high-risk open drainage channels to elucidate which parameters require attention during mitigation. The findings of this study apply to dairy farms on heavy textured soils in high rainfall areas, and may (or may not) differ in other geographic areas with different soils, climates and agricultural practices. However, it should be noted that the same methodology can be applied anywhere to develop a semi-quantitative risk assessment that will inform mitigation management. Future work incorporating varying risks encountered over time across wider farm characteristics will improve the risk scoring system to produce a more robust model that can be applied more generally on farms.
Data availability statement
The original contributions presented in the study are included in the article/supplementary material, further inquiries can be directed to the corresponding author.
Author contributions
DO: Conceptualization, Data curation, Formal Analysis, Investigation, Methodology, Validation, Visualization, Writing–original draft, Writing–review and editing. MH: Conceptualization, Funding acquisition, Investigation, Methodology, Supervision, Validation, Visualization, Writing–review and editing. OF: Conceptualization, Funding acquisition, Investigation, Methodology, Supervision, Visualization, Writing–review and editing. KD: Conceptualization, Investigation, Methodology, Validation, Visualization, Writing–review and editing. TC: Funding acquisition, Methodology, Project administration, Writing–review and editing. PT: Conceptualization, Funding acquisition, Investigation, Methodology, Project administration, Resources, Supervision, Validation, Visualization, Writing–review and editing.
Funding
The author(s) declare that financial support was received for the research, authorship, and/or publication of this article. The authors are grateful to Teagasc for the award of a Walsh Scholarship to the first author [grant number: RMIS-1381] to conduct this research.
Acknowledgments
The authors are grateful to Teagasc for the award of a Walsh Scholarship to the first author [grant number: RMIS-1381] to conduct this research, and to Simon Leach, Asaf Shnel and Denis Brennan for GIS, fieldwork and laboratory assistance provided.
Conflict of interest
The authors declare that the research was conducted in the absence of any commercial or financial relationships that could be construed as a potential conflict of interest.
Publisher’s note
All claims expressed in this article are solely those of the authors and do not necessarily represent those of their affiliated organizations, or those of the publisher, the editors and the reviewers. Any product that may be evaluated in this article, or claim that may be made by its manufacturer, is not guaranteed or endorsed by the publisher.
References
Adams, R., Doody, D. G., Anderson, A., Fenton, O., Tuohy, P., and Vadas, P. (2022). Evaluating scenarios to reduce phosphorus transport in surface waters from slurry applications in temperate grasslands. Hydrological Sci. J. 67 (8), 1216–1227. doi:10.1080/02626667.2022.2072221
Adkin, A., Jones, D. L., Eckford, R. L., Edwards-Jones, G., and Williams, A. P. (2014). A quantitative risk assessment for the safety of carcase storage systems for scrapie infected farms. J. Appl. Microbiol. 117 (4), 940–948. doi:10.1111/jam.12596
Alder, S., Prasuhn, V., Liniger, H., Herweg, K., Hurni, H., Candinas, A., et al. (2015). A high-resolution map of direct and indirect connectivity of erosion risk areas to surface waters in Switzerland—a risk assessment tool for planning and policy-making. Land Use Policy 48, 236–249. doi:10.1016/j.landusepol.2015.06.001
APHA (2005). Standard methods for the examination of water and wastewater. Washington, DC: American Public Health Association.
Askew, F. E., and Smith, R. K. (2005). “Inorganic non metallic constituents; Phosphorus; Method 4500-P F. Automated ascorbic acid reduction method,” in Standard methods for the examination of waters and waste water. Editor A. Bryman 21st edn (Washington, DC: American Public Health Association), 4–155.
Bhandari, A. B., Nelson, N. O., Sweeney, D. W., Baffaut, C., Lory, J. A., Senaviratne, A., et al. (2017). Calibration of the APEX model to simulate management practice effects on runoff, sediment, and phosphorus loss. J. Environ. Qual. 46 (6), 1332–1340. doi:10.2134/jeq2016.07.0272
Bilotta, G., Brazier, R., and Haygarth, P. (2007). The impacts of grazing animals on the quality of soils, vegetation, and surface waters in intensively managed grasslands. Adv. Agron. 94, 237–280. doi:10.1016/S0065-2113(06)94006-1
Bourke, M., Wilkinson, M. E., and Srdjevic, Z. (2022). Nature-based solutions for flow reduction in catchment headwaters. Spatial Flood Risk Manag. Implement. Catchment-Based Retent. Resil. Private Land Front, 13–32. doi:10.4337/9781800379534.00009
Buczko, U., and Kuchenbuch, R. O. (2007). Review Article: Phosphorus indices as risk-assessment tools in the U.S.A. and Europe - A review. J. Plant Nutr. Soil Sci. 170 (4), 445–460. doi:10.1002/jpln.200725134
Carton, O. T., Tunney, H., Daly, K., Ryan, M., Kurz, I., Doody, D., et al. (2008). Eutrophication from Agricultural Sources.
Cassidy, R., Doody, D. G., and Watson, C. J. (2017). Impact of legacy soil phosphorus on losses in drainage and overland flow from grazed grassland soils. Sci. Total Environ. 575, 474–484. doi:10.1016/j.scitotenv.2016.07.063
Clagnan, E., Thornton, S. F., Rolfe, S. A., Tuohy, P., Peyton, D., Wells, N. S., et al. (2018). Influence of artificial drainage system design on the nitrogen attenuation potential of gley soils: Evidence from hydrochemical and isotope studies under field-scale conditions. J. Environ. Manag. 206, 1028–1038. doi:10.1016/j.jenvman.2017.11.069
Clagnan, E., Thornton, S. F., Rolfe, S. A., Wells, N. S., Knoeller, K., Murphy, J., et al. (2019). An integrated assessment of nitrogen source, transformation and fate within an intensive dairy system to inform management change. PLoS ONE 14 (7), 1–22. doi:10.1371/journal.pone.0219479
Collins, S. D., Shukla, S., and Shrestha, N. K. (2016). Drainage ditches have sufficient adsorption capacity but inadequate residence time for phosphorus retention in the Everglades. Ecol. Eng. 92, 218–228. doi:10.1016/j.ecoleng.2016.04.003
Corbett, D., Lynch, B., Wall, D. P., and Tuohy, P. (2022a). The response of finely textured and organic soils to lime and phosphorus application: Results from an incubation experiment. Soil Use Manag. 39, 368–384. doi:10.1111/sum.12825
Corbett, D., Wall, D. P., Lynch, M. B., and Tuohy, P. (2022b). The influence of phosphorus application and varying soil pH on soil and herbage properties across a range of grassland soils with impeded drainage. J. Agric. Sci. 160, 516–527. doi:10.1017/s0021859622000363
Daly, K. (2005). Evaluating Morgan ’ s Phosphorus Test as an Environmental Indicator. Available at: http://www.teagasc.ie/research/reports/environment/4976/eopr4976.pdf.1, 1–19.
Daly, K., Tuohy, P., Peyton, D., Wall, D. P., and Fenton, O. (2017). Field soil and ditch sediment phosphorus dynamics from two artificially drained fields on poorly drained soils. Agric. Water Manag. 192, 115–125. doi:10.1016/j.agwat.2017.07.005
Davison, P. S., Withers, P. J. A., Lord, E. I., Betson, M. J., and Strömqvist, J. (2008). PSYCHIC - A process-based model of phosphorus and sediment mobilisation and delivery within agricultural catchments. Part 1: Model description and parameterisation. J. Hydrology 350 (3–4), 290–302. doi:10.1016/j.jhydrol.2007.10.036
Deelstra, J., Iital, A., Povilaitis, A., Kyllmar, K., Greipsland, I., Blicher-Mathiesen, G., et al. (2014). Reprint of “Hydrological pathways and nitrogen runoff in agricultural dominated catchments in Nordic and Baltic countries.”. Agric. Ecosyst. Environ. 198, 65–73. doi:10.1016/j.agee.2014.06.032
Department of Agriculture Food and the Marine (2018). Woodland for Water: Creating new native woodlands to protect and enhance Ireland’s waters. Available at: www.agriculture.gov.ie/forestservice.
Dollinger, J., Dagès, C., Bailly, J. S., Lagacherie, P., and Voltz, M. (2015). Managing ditches for agroecological engineering of landscape. A review. Agron. Sustain. Dev. 35 (3), 999–1020. doi:10.1007/s13593-015-0301-6
Doody, D. G., Ferris, C. P., and Watson, C. J. (2014). Nutrient losses from two contrasting dairy cow grazing systems. Biol. Environ. Proc. R. Ir. Acad. 114B (2), 61. doi:10.3318/bioe.2014.10
Edwards, A. C., Kay, D., McDonald, A. T., Francis, C., Watkins, J., Wilkinson, J. R., et al. (2008). Farmyards, an overlooked source for highly contaminated runoff. J. Environ. Manag., 87(4), 551–559. doi:10.1016/j.jenvman.2006.06.027
Edwards, A. C., and Withers, P. J. A. (2008). Transport and delivery of suspended solids, nitrogen and phosphorus from various sources to freshwaters in the UK. J. Hydrology 350 (3–4), 144–153. doi:10.1016/j.jhydrol.2007.10.053
EPA. (2020). Council Directive of 12 December 1991 concerning the protection of waters against pollution caused by nitrates from agricultural sources (91/676/EEC) Article 10 Report for Ireland for the Period 2016-2019: Vol. 375/1 (Issue December).
EPA (2023a). Ireland’s national water quality monitoring programme 2022–2027. Ireland: Environmental Protection Agency. Available at: http://www.ctic.org/media/Detailed NWQMC Agenda.pdf.
EPA (2023b). “Water Quality in 2022,” in Water quality in 2022 an indicators report. Available at: https://www.epa.ie/publications/monitoring--assessment/freshwater--marine/water-quality-in-2020.php.
ESRI (2024). ArcGIS field maps mobile. Software. Available at: https://www.esri.com/en-us/arcgis/products/arcgis-field-maps.
Ezzati, G., Fenton, O., Healy, M. G., Christianson, L., Feyereisen, G. W., Thornton, S., et al. (2020). Impact of P inputs on source-sink P dynamics of sediment along an agricultural ditch network. J. Environ. Manag. 257 (March), 109988. doi:10.1016/j.jenvman.2019.109988
Faust, D. R., Kröger, R., Moore, M. T., and Rush, S. A. (2018). Management Practices Used in Agricultural Drainage Ditches to Reduce Gulf of Mexico Hypoxia. Bull. Environ. Contam. Toxicol. 100 (1), 32–40. doi:10.1007/s00128-017-2231-2
Fenton, O., Rice, P., Murnane, J. G., Tuohy, P., and Daly, K. (2022). Dairy farm roadway surface materials as a P-source within the nutrient transfer continuum framework. Front. Environ. Sci. 10 (October), 1–13. doi:10.3389/fenvs.2022.878166
Fenton, O., Tuohy, P., Daly, K., Moloney, T., Rice, P., and Murnane, J. G. (2021). A Review of On-farm Roadway Runoff Characterisation and Potential Management Options for Ireland. Water, Air, Soil Pollut. 232 (Issue 3), 89. doi:10.1007/s11270-021-05027-0
Gary, H. L., Johnson, S. R., and Ponce, S. L. (1983). Cattle grazing impact on surface water quality in a Colorado Front Range stream. J. Soil & Water Conservation 38 (2), 124–128.
Gramlich, A., Stoll, S., Stamm, C., Walter, T., and Prasuhn, V. (2018). Effects of artificial land drainage on hydrology, nutrient and pesticide fluxes from agricultural fields – A review. Agric. Ecosyst. Environ. 266 (August), 84–99. doi:10.1016/j.agee.2018.04.005
Granger, S. J., Bol, R., Anthony, S., Owens, P. N., White, S. M., and Haygarth, P. M. (2010). “Towards a holistic classification of diffuse agricultural water pollution from intensively managed grasslands on heavy soils,”. Advances in agronomy. 1st ed., Vol. 105 (Elsevier Inc), 83–115. doi:10.1016/S0065-2113(10)05003-0
Harrison, S., McAree, C., Mulville, W., and Sullivan, T. (2019). The problem of agricultural ‘diffuse’ pollution: Getting to the point. Sci. Total Environ. 677, 700–717. doi:10.1016/j.scitotenv.2019.04.169
Hart, M. R., Quin, B. F., and Nguyen, M. L. (2004). Phosphorus Runoff from Agricultural Land and Direct Fertilizer Effects: A Review. J. Environ. Qual. 33 (6), 1954–1972. doi:10.2134/jeq2004.1954
Hayes, E., Higgins, S., Geris, J., Nicholl, G., and Mullan, D. (2023). Weighted risk assessment of critical source areas for soil phosphorus losses through surface runoff mechanisms. Catena 225, 107027. doi:10.1016/j.catena.2023.107027
Haygarth, P. M., Condron, L. M., Heathwaite, A. L., Turner, B. L., and Harris, G. P. (2005). The phosphorus transfer continuum: Linking source to impact with an interdisciplinary and multi-scaled approach. Sci. Total Environ. 344 (1-3 SPEC. ISS.), 5–14. doi:10.1016/j.scitotenv.2005.02.001
Herzon, I., and Helenius, J. (2008). Agricultural drainage ditches, their biological importance and functioning. Biol. Conserv. 141 (5), 1171–1183. doi:10.1016/j.biocon.2008.03.005
Hodaj, A., Bowling, L. C., Frankenberger, J. R., and Chaubey, I. (2017). Impact of a two-stage ditch on channel water quality. Agric. Water Manag. 192, 126–137. doi:10.1016/j.agwat.2017.07.006
Houlbrooke, D. J., and Monaghan, R. M. (2009) The influence of soil drainage characteristics on contaminant leakage risk associated with the land application of farm dairy effluent Prepared for Environment Southland, 32. October.
Hubbard, R. K., Newton, G. L., and Hill, G. M. (2004). Water Quality and the Grazing Animal. J. Anim. Sci. 82 E-Suppl, E255–E263. doi:10.2527/2004.8213_supplE255x
Huebsch, M., Horan, B., Blum, P., Richards, K. G., Grant, J., and Fenton, O. (2013). Impact of agronomic practices of an intensive dairy farm on nitrogen concentrations in a karst aquifer in Ireland. Agric. Ecosyst. Environ. 179, 187–199. doi:10.1016/j.agee.2013.08.021
Ibrahim, T. G., Fenton, O., Richards, K. G., Fealy, R. M., and Healy, M. G. (2013). Spatial and temporal variations of nutrient loads in overland flow and subsurface drainage from a marginal land site in south-east Ireland. Biol. Environ. 113 B (2), 1–18. doi:10.3318/BIOE.2013.13
Jordan, P., Menary, W., Daly, K., Kiely, G., Morgan, G., Byrne, P., et al. (2005). Patterns and processes of phosphorus transfer from Irish grassland soils to rivers - Integration of laboratory and catchment studies. J. Hydrology 304 (1–4), 20–34. doi:10.1016/j.jhydrol.2004.07.021
Kleinman, P. J. A., Sharpley, A. N., McDowell, R. W., Flaten, D. N., Buda, A. R., Tao, L., et al. (2011). Managing agricultural phosphorus for water quality protection: Principles for progress. Plant Soil 349 (1–2), 169–182. doi:10.1007/s11104-011-0832-9
Kröger, R., Holland, M. M., Moore, M. T., and Cooper, C. M. (2007). Hydrological Variability and Agricultural Drainage Ditch Inorganic Nitrogen Reduction Capacity. J. Environ. Qual. 36 (6), 1646–1652. doi:10.2134/jeq2006.0506
Magette, W., Hallissey, R., Hughes, K., and Cosgrave, E. (2007). Eutrophication from Agricultural Sources: Field- and Catchment-Scale Risk Assessment. Environ. Prot. Agency. Available at: https://www.epa.ie/publications/research/water/ERTDI-Report-79---Web1.pdf.
Maher, P. J., Murphy, M. D., Egan, M., and Tuohy, P. (2023). An evaluation of parameters that affect dairy herd movement on Irish pasture-based farms. J. Agric. Sci., 1–10. doi:10.1017/S0021859623000497
Martínez-Suller, L., Provolo, G., Carton, O. T., Brennan, D., Kirwan, L., and Richards, K. G. (2010). The composition of dirty water on dairy farms in Ireland. Ir. J. Agric. Food Res. 49 (1), 67–80.
Mattila, T. J., and Ezzati, G. (2022). A common agricultural soil test can identify legacy P hotspots in a drainage ditch network. J. Environ. Manag. 302 (Pt A), 113876. doi:10.1016/j.jenvman.2021.113876
McDowell, R., Kleinman, P. J. A., Haygarth, P., McGrath, J. M., Smith, D., Heathwaite, L., et al. (2024). A review of the development and implementation of the critical source area concept: A reflection of Andrew Sharpley’s role in improving water quality. J. Environ. Qual., 1–20. doi:10.1002/jeq2.20551
Mellander, P., Jordan, P., Mcdonald, N., and Vero, S. (2017). Nutrient mobilisation and transfer pathways.
Moloney, T., Fenton, O., and Daly, K. (2020). Ranking connectivity risk for phosphorus loss along agricultural drainage ditches. Sci. Total Environ. 703, 134556. doi:10.1016/j.scitotenv.2019.134556
Murphy, P. N. C., Mellander, P. E., Melland, A. R., Buckley, C., Shore, M., Shortle, G., et al. (2015). Variable response to phosphorus mitigation measures across the nutrient transfer continuum in a dairy grassland catchment. Agric. Ecosyst. Environ. 207, 192–202. doi:10.1016/j.agee.2015.04.008
Nag, R., Whyte, P., Markey, B. K., O’Flaherty, V., Bolton, D., Fenton, O., et al. (2020). Ranking hazards pertaining to human health concerns from land application of anaerobic digestate. Sci. Total Environ. 710, 136297. doi:10.1016/j.scitotenv.2019.136297
Needelman, B. A., Kleinman, P. J. A., Strock, J. S., and Allen, A. L. (2007). Improved management of agricultural drainage ditches for water quality protection: an overview. J. soil water conservation 62 (4), 171–178.
Nelson, N. O., Baffaut, C., Lory, J. A., Anomaa Senaviratne, G. M. M. M., Bhandari, A. B., Udawatta, R. P., et al. (2017). Multisite Evaluation of APEX for Water Quality: II. Regional Parameterization. J. Environ. Qual. 46 (6), 1349–1356. doi:10.2134/jeq2016.07.0254
Opoku, D. G., Healy, M. G., Fenton, O., Daly, K., Condon, T., and Tuohy, P. (2024). An integrated connectivity risk ranking for phosphorus and nitrogen along agricultural open ditches to inform targeted and specific mitigation management. Front. Environ. Sci., 12, 1–16. doi:10.3389/fenvs.2024.1337857
Palmer, M. (2012). Agricultural fine sediment: sources, pathways and mitigation: a thesis submitted for the degree of doctor of philosophy at school of civil engineering and geosciences,. UK: [University of Newcastle upon Tyne]. Available at: https://theses.ncl.ac.uk/jspui/bitstream/10443/1518/1/Palmer12.pdf.
Pérez-Gutiérrez, J. D., Paz, J. O., Tagert, M. L. M., and Sepehrifar, M. (2020). Impact of rainfall characteristics on the NO3 – N concentration in a tailwater recovery ditch. Agric. Water Manag. 233 (3), 106079. doi:10.1016/j.agwat.2020.106079
Peyton, D. P., Healy, M. G., Fleming, G. T. A., Grant, J., Wall, D., Morrison, L., et al. (2016). Nutrient, metal and microbial loss in surface runoff following treated sludge and dairy cattle slurry application to an Irish grassland soil. Sci. Total Environ. 541, 218–229. doi:10.1016/j.scitotenv.2015.09.053
Pietola, L., Horn, R., and Yli-Halla, M. (2005). Effects of trampling by cattle on the hydraulic and mechanical properties of soil. Soil Tillage Res. 82 (1), 99–108. doi:10.1016/j.still.2004.08.004
Radcliffe, D. E., Reid, D. K., Blombäck, K., Bolster, C. H., Collick, A. S., Easton, Z. M., et al. (2015). Applicability of Models to Predict Phosphorus Losses in Drained Fields: A Review. J. Environ. Qual. 44 (2), 614–628. doi:10.2134/jeq2014.05.0220
Redmill, F. (2002). Risk analysis - a subjective process. Eng. Manag. J. 12 (2), 91–96. doi:10.1049/em:20020206
Rice, P., Daly, K., Tuohy, P., Murnane, J. G., Nag, R., and Fenton, O. (2022). Evaluating connectivity risk of farm roadway runoff with waters - Development and sensitivity analysis of a semi quantitative risk model. Sci. Total Environ. 851 (August), 158114. doi:10.1016/j.scitotenv.2022.158114
Richards, K. G., Jahangir, M. M. R., Drennan, M., Lenehan, J. J., Connolly, J., Brophy, C., et al. (2015). Effect of an agri-environmental measure on nitrate leaching from a beef farming system in Ireland. Agric. Ecosyst. Environ. 202 (April), 17–24. doi:10.1016/j.agee.2014.12.020
Roberts, W. M., Gonzalez-Jimenez, J. L., Doody, D. G., Jordan, P., and Daly, K. (2017). Assessing the risk of phosphorus transfer to high ecological status rivers: Integration of nutrient management with soil geochemical and hydrological conditions. Sci. Total Environ. 589, 25–35. doi:10.1016/j.scitotenv.2017.02.201
Schoumans, O. F., and Chardon, W. J. (2003). Risk assessment methodologies for predicting phosphorus losses. J. Plant Nutr. Soil Sci. 166 (4), 403–408. doi:10.1002/jpln.200321222
Shariff, A. M., and Zaini, D. (2013). Inherent risk assessment methodology in preliminary design stage: A case study for toxic release. J. Loss Prev. Process Industries, 26(4), 605–613. doi:10.1016/j.jlp.2012.12.003
Sharpley, A., Kleinman, P., Baffaut, C., Beegle, D., Bolster, C., Collick, A., et al. (2017). Evaluation of Phosphorus Site Assessment Tools: Lessons from the USA. J. Environ. Qual. 46 (6), 1250–1256. doi:10.2134/jeq2016.11.0427
Shore, M., Jordan, P., Mellander, P. E., Kelly-Quinn, M., and Melland, A. R. (2015). An agricultural drainage channel classification system for phosphorus management. Agric. Ecosyst. Environ. 199, 207–215. doi:10.1016/j.agee.2014.09.003
Soana, E., Balestrini, R., Vincenzi, F., Bartoli, M., and Castaldelli, G. (2017). Mitigation of nitrogen pollution in vegetated ditches fed by nitrate-rich spring waters. Agric. Ecosyst. Environ. 243 (November 2016), 74–82. doi:10.1016/j.agee.2017.04.004
Stutter, M., Barros Costa, F., and O Huallachain, D. (2021). Riparian buffer zone quantitative effectiveness review database. doi:10.17632/t64dbpv63x.3
Teagasc (2022). “GROUNDWATER DRAINAGE SYSTEMS,” in Teagasc manual on drainage - a best practice manual for Ireland’s farmers. Editors P. Tuohy, O. Fenton, and M. Moore (Carlow, Ireland: Teagasc), 79–88.
Tejada, M., and Gonzalez, J. L. (2008). Influence of two organic amendments on the soil physical properties, soil losses, sediments and runoff water quality. Geoderma 145 (3–4), 325–334. doi:10.1016/j.geoderma.2008.03.020
Teunis, P., and Schijven, J. F. (2019). Generic Guidance to Quantitative Microbial Risk Assessment for Food And Water.
Tuohy, P., O’ Loughlin, J., Peyton, D., and Fenton, O. (2018). The performance and behavior of land drainage systems and their impact on field scale hydrology in an increasingly volatile climate. Agric. Water Manag. 210 (August), 96–107. doi:10.1016/j.agwat.2018.07.033
Tuohy, P., O’Sullivan, L., and Fenton, O. (2021). Field scale estimates of soil carbon stocks on ten heavy textured farms across Ireland. J. Environ. Manag. 281 (December 2020), 111903. doi:10.1016/j.jenvman.2020.111903
Vadas, P. A., Busch, D. L., Powell, J. M., and Brink, G. E. (2015). Monitoring runoff from cattle-grazed pastures for a phosphorus loss quantification tool. Agric. Ecosyst. Environ. 199, 124–131. doi:10.1016/j.agee.2014.08.026
Vadas, P. A., Gburek, W. J., Sharpley, A. N., Kleinman, P. J. A., Moore, P. A., Cabrera, M. L., et al. (2007). A Model for Phosphorus Transformation and Runoff Loss for Surface-Applied Manures. J. Environ. Qual. 36 (1), 324–332. doi:10.2134/jeq2006.0213
Vadas, P. A., Jokela, W. E., Franklin, D. H., and Endale, D. M. (2011). The effect of rain and runoff when assessing timing of manure application and dissolved phosphorus loss in runoff. J. Am. Water Resour. Assoc. 47 (4), 877–886. doi:10.1111/j.1752-1688.2011.00561.x
Valbuena-Parralejo, N., Fenton, O., Tuohy, P., Williams, M., Lanigan, G. J., and Humphreys, J. (2019). Phosphorus and nitrogen losses from temperate permanent grassland on clay-loam soil after the installation of artificial mole and gravel mole drainage. Sci. Total Environ. 659, 1428–1436. doi:10.1016/j.scitotenv.2018.12.173
van den Berg, L. M., Dingkuhn, E. L., Meehan, N., and O’Sullivan, L. (2023). Investigating bottlenecks hampering the adoption of water quality-enhancing practices for sustainable land management in Ireland. J. Environ. Manag. 345 (January), 118741. doi:10.1016/j.jenvman.2023.118741
Vero, S. E., Sherry, E., and Doody, D. (2020). Evidence and perception of phosphorus loss risk factors in farmyards. Environ. Sci. Policy 114, 542–548. doi:10.1016/j.envsci.2020.09.025
Wall, D., Jordan, P., Melland, A. R., Mellander, P.-E., Buckley, C., Reaney, S. M., et al. (2011). Using the nutrient transfer continuum concept to evaluate the European Union Nitrates Directive National Action Programme. Environ. Sci. & Policy, 14(6), 664–674. doi:10.1016/j.envsci.2011.05.003
Wang, Z.-J., Li, S.-L., Yue, F.-J., Qin, C.-Q., Buckerfield, S., and Zeng, J. (2020). Rainfall driven nitrate transport in agricultural karst surface river system: Insight from high resolution hydrochemistry and nitrate isotopes. Agric. Ecosyst. & Environ., 291, 106787. doi:10.1016/j.agee.2019.106787
Watson, C. J., and Foy, R. H. (2001). Environmental impacts of nitrogen and phosphorus cycling in grassland systems. Outlook Agric. 30 (2), 117–127. doi:10.5367/000000001101293562
Watson, C. J., Smith, R. V., and Matthews, D. I. (2007). Increase in Phosphorus Losses from Grassland in Response to Olsen-P Accumulation. J. Environ. Qual. 36 (5), 1452–1460. doi:10.2134/jeq2006.0207
Withers, P. J. A., and Lord, E. I. (2002). Agricultural nutrient inputs to rivers and groundwaters in the UK: Policy, environmental management and research needs. Sci. Total Environ. 282–283, 9–24. doi:10.1016/S0048-9697(01)00935-4
Yao, Y., Dai, Q., Gao, R., Gan, Y., and Yi, X. (2021). Effects of rainfall intensity on runoff and nutrient loss of gently sloping farmland in a karst area of SW China. PLoS ONE 16 (3 March), e0246505–e0246518. doi:10.1371/journal.pone.0246505
Keywords: water quality, agriculture, nitrogen, phosphorus, environment, mitigation
Citation: Opoku DG, Healy MG, Fenton O, Daly K, Condon T and Tuohy P (2024) A semi-quantitative risk model for dairy farms to pinpoint and break source-pathway connections between nutrient sources and open drainage channel sections. Front. Environ. Sci. 12:1435418. doi: 10.3389/fenvs.2024.1435418
Received: 20 May 2024; Accepted: 09 September 2024;
Published: 23 September 2024.
Edited by:
Paweł Tomczyk, Wroclaw University of Environmental and Life Sciences, PolandReviewed by:
Laura Christianson, University of Illinois at Urbana-Champaign, United StatesPankaj Tiwari, University of Kalyani, India
Copyright © 2024 Opoku, Healy, Fenton, Daly, Condon and Tuohy. This is an open-access article distributed under the terms of the Creative Commons Attribution License (CC BY). The use, distribution or reproduction in other forums is permitted, provided the original author(s) and the copyright owner(s) are credited and that the original publication in this journal is cited, in accordance with accepted academic practice. No use, distribution or reproduction is permitted which does not comply with these terms.
*Correspondence: P. Tuohy, cGF0cmljay50dW9oeUB0ZWFnYXNjLmll