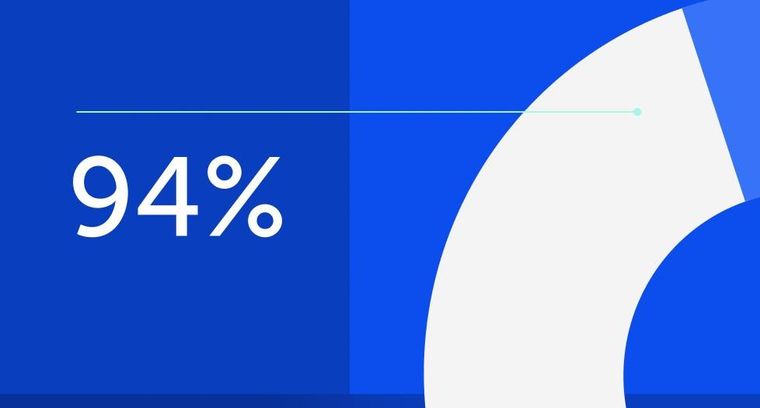
94% of researchers rate our articles as excellent or good
Learn more about the work of our research integrity team to safeguard the quality of each article we publish.
Find out more
ORIGINAL RESEARCH article
Front. Environ. Sci., 28 July 2023
Sec. Water and Wastewater Management
Volume 11 - 2023 | https://doi.org/10.3389/fenvs.2023.1212807
This article is part of the Research TopicDevelopments in Managing Emerging Pollutants in Natural Waters: A Focus on Low- and Middle-Income RegionsView all 6 articles
Ultraviolet disinfection has been extensively studied in recent years, especially in bacteria; however, there are still insufficient studies in fungal spores. Moreover, most studies use static batch reactors instead of continuous flow reactors, which are used mainly at the industrial scale. In the present work, the inactivation and reactivation characteristics of two species of filamentous fungi were studied using a single-pass flow-through UV-C reactor (FTR). For this purpose, Aspergillus niger and Penicillium sp. spores were suspended in water and circulated through the reactor at different UV-C doses. The effects on inactivation and reactivation after 24 in either light or dark conditions were studied. The two fungal strains studied show different UV-C treatment resistance and damage repair capacity. With the experimented FTR system, an inactivation efficiency of up to 2 log units (99% removal) was achieved with doses of 220.1 ± 24.3
Fungi are a class of heterotrophic eukaryotes, which mainly include molds (also known as filamentous fungi), yeasts (unicellular), and mushrooms (macroscopic fungi) (Mukherjee et al., 2018) and are ubiquitous in the environment (Al-Gabr et al., 2014). The main effect of these fungal spores is related to phytopathogenic diseases (Afonso et al., 2021). In addition, when present in specific environmental matrices, they can produce mycotoxins showing toxicities to invertebrates, plants, and microorganisms (Doggett, 2000). In recent years, the occurrence of filamentous fungi in the aquatic environment has been of growing interest because they can cause odor, taste, and turbidity problems in the water (Wan et al., 2023). Furthermore, studies report that once the fungi sprout rapidly, they would cause serious safety problems in the drinking water supply, causing asthma, allergic pneumonia, skin infection, and other diseases in people with low immunity (Al-Gabr et al., 2014). Several studies have reported the presence of filamentous fungi in drinking water distribution systems worldwide (Afonso et al., 2021). A first study of this type reported that fungi existed in systems of urban water supply biofilms, and the number of filamentous fungi ranged from 4.0 to 25.2 CFU
Disinfection of waterborne fungi is essential (Wan et al., 2023). Unfortunately, conventional drinking water treatment processes (coagulation-sedimentation-filtration) cannot remove fungi from water (Al-Gabr et al., 2014). Therefore, it will be necessary to explore further efficient, safe, and economical disinfection technologies for fungal inactivation in future studies. Under this context, studies have shown that applying ultraviolet (UV-C) radiation efficiently inactivates fungal spores (Wan et al., 2022; 2023; Wu et al., 2022). UV-C irradiation, with wavelengths between 200 and 320 nm, has been considered an effective disinfection technology in recent years (Hijnen et al., 2006; Kowalski and Kowalski, 2009). It is, therefore, widely used in water and wastewater treatment due to its versatility, efficiency, and low potential for forming harmful disinfection by-products (Song et al., 2016). The most commonly used UV-C sources are the low-pressure monochromatic emission (LPUV) with a wavelength of 254 nm and the medium-pressure mercury lamp (MPUV), which emits polychromatic light in a wide range of wavelengths, between 200 and 400 nm (Ao et al., 2018).
At the research-experimental level, UV-C disinfection can be applied using various devices, the most widely used being the collimated beam reactor (CBR). Studies have mainly focused on analyzing the application of UV-C radiation using static CBRs because it allows the maximum precision on the intensity calculation using a radiometer and direct control of the exposure time (Al-Gabr et al., 2014; Wen et al., 2017; Wan et al., 2020; Xu et al., 2022). However, their operation differs significantly from commercial UV-C disinfection systems, which commonly use flow-through reactors (FTRs). In this context, CBR often used as a reference and calibration for other more complex disinfection equipment (Bolton, 2000), while continuous FTRs are more similar to commercial UV-C systems (Lindenauer and Darby, 1994) and are therefore more focused on the application of full-scale treatments.
The inactivation of microorganisms by UV-C radiation emission is explained by the fact that it affects the formation of cyclobutane pyrimidine dimers (CPD) and six to four pyrimidine dimers in the DNA strands of microbial cells after irradiation, so the existence of these UV-C-induced lesions would prevent standard DNA transcription and replication, leading to the inactivation of microorganisms (Setlow and Carrier, 1964). However, microorganisms inactivated by UV-C light have a high risk of reactivation by means of two main processes, photoreactivation and dark repair (Lindenauer and Darby, 1994), thus significantly weakening the efficiency of disinfection. Photoreactivation is a conventional repair system widely present in organisms in which the enzyme photolyase is activated by photon energy with wavelengths from 330 to 480 nm and binds specifically to cyclobutane-pyrimidine dimers and reverses the damage (Song et al., 2019). On the other hand, dark repair is a light-independent process that replaces damaged DNA with new and intact nucleotides (Sinha and Häder, 2002). Among both methods, photoreactivation is the main one in terms of the damage that can be repaired (Nebot Sanz et al., 2007).
The present study aimed to explore the inactivation and reactivation characteristics of Aspergillus niger and Penicillium sp. spores present in water after being irradiated by UV-C at different doses through a continuous flow laboratory reactor. Viable fungal spores were measured immediately after UV-C irradiation and after a 24-h incubation period in an illuminated or dark environment. Inactivation curves were fitted to inactivation models to determine kinetic parameters that allowed quantitative comparison of UV-C-resistance and damage repair potential of the two fungal strains and post-treatment conditions tested.
This study used two strains of the fungal species A. niger and Penicillium sp., acquired from the Fungi Collection of the University of Azuay (Ecuador). These strains were chosen as model microbes because they are the dominant genera of fungal spores isolated from water sources; in addition, they are representative genera of water contamination.
The biological procedures were based on previous studies (Pereira et al., 2013; Wen et al., 2017; Wan et al., 2022). First, the strains delivered were reactivated in 30 mL of potato dextrose agar (PDA) culture medium at 27°C for 2 weeks. They were then inoculated on dichloran rose bengal chloramphenicol agar (DRBC) and cultured in an incubator at 27°C for 2 weeks. Subsequently, the grown spores were scraped from DRBC agar and washed three times with sterile phosphate buffer solution (PBS) at pH = 7.3 by centrifugation (8000 rpm, 10 min).
The spores concentration was determined using a Neubauer chamber. Then a volume of distilled with a phosphate-buffer solution water was added, aiming to obtain a concentration between 107—108 spores
The surface spreading and plate counting technique was used to quantify the concentration of viable microorganisms after the experiments (Hoben and Somasegaran, 1982). Plates with a concentration between 20 and 300 spores were selected as representatives. To achieve this concentration, serial dilutions of each sample were made at a ratio of 1:10.100 μL of each dilution was then taken and evenly dispersed in 90 mm Petri dishes with DRBC agar. These plates were incubated at 27°C for 3 days. This culture time was left, due to the space they begin to occupy once they start to develop, determining that this is sufficient time to obtain adequate development and an accurate estimate of the number of spores in the sample. After incubation, the spore concentration was counted and expressed as spores
The UV-C treatment was applied by means of a single-pass through a ring-type continuous flow-through reactor, built at laboratory scale. The reactor was equipped with a low-pressure (LP) mercury vapor lamp (Phillips 1GPM - in/out 1/4″monochromatic emission at 254 nm) covered with a quartz sleeve and inserted in an aluminum housing (Figure 1; Table 1). The reactor was inserted in a laboratory rig composed of a 20 L plastic tank, a centrifugal pump, manual valves to manipulate the flow rate and, finally, the UV-C reactor.
The UV-C dose applied (Eq. 1; abbreviations and values in Table 1) was determined according to USEPA specifications (USEPA, 1986), as the product of the mean intensity (Im) and the theoretical retention time (TRT). The power output at 254 nm (P254) was calculated as one-third of the total lamp power (Figawa, 2009). The TRT was calculated as the quotient between the volume of water exposed to UV-C light (VR) and the flow rate (Q). Im was calculated according to a geometrical model of radiation scattering for ring reactors (Eq. 2), validated by biodosimetry in previous studies (Romero-Martínez et al., 2014; Moreno-Andrés et al., 2017). Water transmittance at 254 nm (TW) was measured at the beginning of each test using a Genesys 20 spectrophotometer, providing values between 93.5% ± 0.5%. Depending on the value of TW, Im 8.73 ± 0.34
The stock solution containing the fungal spores was placed in the 20 L plastic tank containing distilled buffered water, obtaining a concentration between 105 and 106
The sample volume was divided into three 20 mL test tubes. One of these tubes underwent immediate plate counting on the same day of the test to determine the number of surviving spores after UV-C inactivation (0 d). Another tube was covered with aluminum foil to replicate dark conditions (1 d—dark), while the remaining tube was left uncovered to simulate light conditions (1 d—light). These two tubes were incubated in a shaking incubator (model FS-70B) at 20°C for 24 h. The incubator was equipped with a fluorescent lamp emitting 36
Survival (S) in each sample was determined as the quotient of the culturable spore concentration in the irradiated sample between the spore concentration in control without irradiation and without being subjected to post-treatment incubation. Inactivation curves were obtained by plotting Log(S) versus the UV-C dose applied in each case. For determining the existence of significant differences between the UV-C treatment for the different organisms under the different post-treatment conditions, the inactivation curves were subjected to ANCOVA analysis with Statgraphics Centurion (version.16.1.03), using Log(S) as the dependent variable, UV-C dose as covariable, and the target organism (A. niger or Penicillium sp.) and the post-treatment (0 days, 1 day in illuminated conditions or 1 day in dark conditions) as factors. The inactivation kinetics parameters were obtained by modeling the inactivation curves with the GInaFiT tool for MS Excel (Geeraerd et al., 2005). These parameters were used to calculate the dose required to reduce the initial concentration by two orders of magnitude (D2), that is, the inactivation of the 99% of the target organisms, as an estimator of treatment efficacy.
To evaluate the effect of photoreactivation, the photoreactivation percentage (PPR) was calculated according to Eq. 3 (Lindenauer and Darby, 1994), where: Np = bacterial concentration of the photoreactivated sample (CFU
The inactivation curves obtained for A. niger and Penicillium sp. (Figure 3) showed a dependence between the Log(S) and the applied UV-C dose. The ANCOVA analysis indicated that the organism (p < 0.001) and the UV-C dose applied (p < 0.001) influence significantly the UV-C treatment outcome. In the case of A. niger, although the inactivation curves indicate a slightly lower efficacy when the treated organisms were incubated under light for 24 h, the ANCOVA analysis indicated no significant differences between the three post-treatment conditions (p = 0.683). On the other hand, there were significant differences between the post-treatment conditions in the case of Penicillium sp. (p < 0.001), determining one homogeneous group with both 0 days and 1 day under dark conditions and another homogeneous group with 1 day under illuminated conditions. Therefore, according to the inactivation curves analysis, the spores of A. niger are more UV-C-resistant with respect to the Penicillium sp. spores. The photoreactivation is significant in the case of Penicillium sp. These facts have been evaluated quantitatively by determining the inactivation kinetics and the percentage of photoreactivation.
FIGURE 3. Inactivation curves of (A) Aspergillus niger and (B) Penicillium sp. for different post-treatment conditions: absence of post-treatment incubation (0 d), one-day incubation in dark environment (1 d—dark) and one-day incubation in illuminated environment (1 d—light). Symbols represent the experimental data and lines represent the inactivation model fitted (solid line: 0 d, dashed line: 1 d—dark, dotted line: 1 d—light).
First-order kinetics was observed between the S and the UV-C dose applied up to 180
TABLE 2. Estimated kinetic values for the two strains studied in different post-treatment conditions and statistical values of the fit to the models.
In the present study, no tails were present for the UV-C range experimented in the three treatments (inactivation and reactivation in light and dark) (Figure 3), nor were shoulders observed. Comparing with previous studies (Wen et al., 2017; Wan et al., 2020) experimented with these two genera in a collimated reactor with 254 nm low-pressure mercury lamps and found tailing and shoulder phenomena; they concluded that this phenomenon is present regardless of incubation time and emission wavelength. Therefore, we also support the hypothesis that these phenomena are probably due to spore aggregation (Mattle and Kohn, 2012), hydraulic problems in treatment with recirculation and/or screening by suspended solids and flocs, as well as achieving inactivation levels close to the detection limit (Azimi et al., 2017; Carré et al., 2018; Romero-Martínez et al., 2019). In this study, the non-significant values of S0 indicate absence of shoulder and thus, suitability of the log-linear model for fitting the obtained data. On the other hand, tailing cannot be discarded at UV-C dose beyond the experimental range of UV-C doses applied.
The results obtained revealed significant differences in UV-C resistance between the two strains. Comparing the inactivation profiles (d 0), Penicillium sp. showed higher sensitivity to UV-C light in comparison with A. niger. This fact was mainly attributed to differences in enzymes, intracellular structure diversity and pigment content within the fungal cells (Guo et al., 2011; Liu et al., 2014). The genus Aspergillus has been reported to have as its main defense mechanism the production of melanins and other pigments (Clauß, 2006; Wu et al., 2022), which makes it more tolerant compared to other species studied (Narita et al., 2020). In the case of Penicillium sp., carotenoids play a protective role against UV-C exposure (Guo et al., 2011). However, this defense system is weaker when compared to that of the genus Aspergillus. Moreover, these defense mechanisms are more significant, especially in wild-type species versus laboratory strains (Esbelin et al., 2013). Betzalel et al. (2020) reported that spores of fungi such as Penicillium, Aspergillus and Acremonium are hydrophobic and tend to cluster together, which makes them resistant to UV-C inactivation. Nourmoradi et al. (2012) investigated the effects of UV-C irradiation on Aspergillus sp. and found that a dose of 20.7
Comparing the reactor configuration, studies (Wen et al., 2019) found that k for A. niger was relatively lower than for Penicillium polonicum; the results of this study agree with this fact. However, the reported values are considerably higher (0.0638–0.0368
It was found that a higher UV-C dose was required to achieve fungal spore reduction, compared to other microorganisms; for example, typical indicator organisms such as Escherichia coli, in a study performed with the same reactor type, it was found that E. coli ATCC 8739, required a dose of 15
The inactivation curves analysis indicated that the dark repair after UV-C irradiation was not significant, and the photoreactivation was significant in the case of Penicillium sp. This implies that the values of D2 (Table 2) obtained for samples incubated for 24 h in dark conditions were similar to those obtained without incubation, indicating that the inactivation achieved by the UV-C treatment is maintained for at least 1 day is the irradiated spores are kept in a dark environment. On the other hand, the Penicillium sp. samples incubated for 24 h in illuminated conditions showed a D2 value that increased a 53.8% with respect to the D2 right after the UV-C exposure, due to the photoreactivation.
The percentage of photoreactivation (PPR) was determined for both organisms and correlated with the UV-C dose according to a first order kinetics (Log(PPR) = a + b · UV-C dose) (Figure 4). In the case of A. niger, the PPR ranged between the 1.5% and 20%; the regression analysis reported not significant linear correlation between Log(PPR) and the UV-C dose applied (p = 0.572). On the other hand, PPR ranged between the 0.2 and the 30% for Penicillium sp., with a significant linear correlation between Log(PPR) and the UV-C dose (p = 0.011) (Figure 4). According to the regression parameters determined for Penicillium sp., a 13.7% of the spores can be photoreactivated after 1-log reduction, a 3.7% after 2-log reductions and a 1.0% after 3-log reductions. Therefore, treating the Penicillium sp. spores with higher UV-C doses increases the inactivation level as well as reduces the potential photoreactivation.
The process of repair involves DNA damage reversal mechanisms, such as photoreactivation and dark repair. In this study, it was found that using a low-pressure mercury lamp for UV-C treatment with emission of 254 nm, in a continuous flow reactor, did not completely prevent fungal strains from repairing some of the damage if they were exposed to environmental light after treatment. Previous studies (Wen et al., 2017; Wu et al., 2022; Xu et al., 2022) have highlighted the importance of photoreactivation in the inactivation process. In this study, irradiated samples were exposed to light in a culture chamber to enhance photorepair, the percentage of photoreactivation varied among fungal spores at different UV-C doses ranging from 23 to 260
The rate of photoreactivation is influenced by the storage conditions of the irradiated water (Bohrerova and Linden, 2007; Maghsoodi et al., 2022). For instance, if the water is stored in sunlight, there is a trade-off between photoreactivation and subsequent inactivation caused by UV-C-A and UV-C-B radiation (Lindenauer and Darby, 1994; Kashimada et al., 1996; Tosa and Hirata, 1999; Salcedo et al., 2007; Marugán et al., 2008; Quek and Hu, 2008; Rubio et al., 2013; Rodríguez-Chueca et al., 2015). During the reactivation process, a fluorescent lamp was used whose emission range includes the optimal wavelengths for photolyase activation, from 350 to 500 nm (Xu et al., 2022). In the case of Penicillium sp, it was observed to show signs of successful reactivation. In this sense, it can be assumed that the light used in incubation is effective for photoreactivation, and thus, the lack of photoreactivation in A. niger may be due to the specific characteristics of that organism, which make it less prone to be photoreactivated.
The results obtained in this study indicated the absence of dark repair within 24 h after UV-C irradiation, which is consistent with previous studies (Wan et al., 2020). Although not significant, this can influence the photoreactivation processes if conjugated during the application of UV-C radiation; it is known that a 3 h delay in light exposure after UV-C irradiation significantly reduces the photoreactivation fractions (Hallmich and Gehr, 2010). Therefore, this dark delay can be introduced as part of water treatment to prevent risks due to photoreactivation. However, this is validated for bacteria; it is unknown if the same will be valid for fungal spores. These results support the need to consider the post-treatment management of the water once treated with UV-C; since higher doses of UV-C will be required to maintain, for example, the same goal of 3 log reductions if the treated water is exposed to light conditions after treatment (Figure 2), or else consider the possibility of keeping the water in darkness after treatment and before use. It is therefore proposed that future studies are needed to determine the hours of dark storage necessary for the fungal strains not to reactivate, or to reactivate a minimum target percentage, once exposed to light.
It is of interest to study the mechanisms of UV-C inactivation and reactivation in different microorganisms and environmental conditions to ensure the effectiveness of disinfection and to avoid the selection of resistant strains. The implication of these mechanisms in reducing fungal spores in water is significant since water treatment systems must consider not only microbial inactivation but also the possibility of reactivation. Exposure of fungal spores to UV-C radiation may not be sufficient to completely eliminate them if adequate light conditions are present for photoreactivation. Thus, the importance of taking into account storage conditions after UV-C treatment is directly related to the photoreactivation ability of fungal spores. As previous research has shown, some types of fungal spores may be more resistant to UV-C radiation and have a higher photoreactivation capacity than others (Wan et al., 2020; 2022). In the case of Penicillium sp. it is requires less UV-C dose. However, it has a higher photoreactivation capacity, but even considering photoreactivation, it requires less UV-C dose than A. niger. This suggests that in the treatment of water contaminated with Penicillium sp. spores, the storage conditions after treatment will not significantly impact the treatment’s effectiveness. On the other hand, in the case of A. niger, storage conditions after treatment may be more important. In addition, these studies are important for optimizing the design and scale-up of UV-C radiation reactors at the commercial level, considering the different inactivation and photoreactivation conditions in fungal spores and storage conditions.
Spore inactivation is a key factor in ensuring the microbiological safety of water and other products, especially in the food and pharmaceutical industry. However, photoreactivation can decrease the effectiveness of treatment, which can increase the risk of microbiological contamination. Therefore, it is important to consider the specific characteristics of each fungal spore species when designing water treatment systems and other products.
Based on the findings of this study, it is essential to conduct further research to examine the resistance and repair capacity of diverse fungal strains in response to UV-C treatment. Additionally, conducting targeted experiments to explore the photoreactivation process in Penicillium sp. is recommended. This experimentation should encompass varying wavelengths and exposure durations to determine the optimal effects. Lastly, assessing the impact of environmental factors beyond light, such as temperature and the presence of chemical compounds, is crucial on the fungi’s photoreactivation ability. These recommendations will contribute to a more comprehensive understanding of the repair and photoreactivation mechanisms, ultimately enhancing the efficacy of UV-C treatment.
The results show that the two strains of fungi studied, A. niger and Penicillium sp. offer different resistance to UV-C treatment and capacity for damage repair. The effect of repair in darkness is insignificant, while the photoreactivation process is relevantin the case Penicillium sp. In general, A. niger is more resistant than Penicillium sp.; however, the latter has a greater capacity for photoreactivation.
The FTR is effective in inactivating fungal spores in water. It was demonstrated that inactivation could be up to 2 log-reductions with UV-C doses of 251.4 ± 38.5 and 190.4 ± 16.7
The use of FTR demonstrates that it could be a valuable tool for the investigation and optimization of fungal spore inactivation at the laboratory level. This type of reactor allows a better reproduction of the processes at full scale, resulting in a more accurate extrapolation of the results obtained in the laboratory. In addition, the more precise control of process parameters in a continuous flow reactor can improve the efficiency and reproducibility of the results, which is crucial for the research and development of microorganism inactivation technologies in practical applications.
Finally, the method used, including the photoreactivation procedure, can be used to evaluate the microbiological quality of UV-C-disinfected water for filamentous fungal strains. The increased UV-C dose should be considered during the design of UV-C disinfection when UV-C-disinfected water is discharged into open receiving systems, such as rivers or lakes, where they are exposed to light conditions, which may favor photoreactivation processes.
The datasets presented in this study can be found in online repositories. The names of the repository/repositories and accession number(s) can be found below: https://estliveupsedu-my.sharepoint.com/:x:/g/personal/pduque_ups_edu_ec/EfNtGcPEMrZGvgWTDcRahMQBj9bJhB-sC76Ld_9pDlV30w?e=0KgYoS
PD-S writing—original draft, writing-review and editing, methodology, investigation, data curation, conceptualization, project administration, funding acquisition. ND-A methodology, investigation, conceptualization. LR-M writing—review and editing, conceptualization, supervision. VP-V review, editing, supervision, formal analysis. All authors contributed to the article and approved the submitted version.
The Salesian Polytechnic University research funds, project number 010-005-2021-07-01 have funded this research.
We would like to express our gratitude to Blgo. Rodrigo Caróca for kindly providing us with the fungal strains used in this study. We also extend our thanks to the Life Sciences Laboratory at UPS for the use of their facilities and the support provided during our research.
The authors declare that the research was conducted in the absence of any commercial or financial relationships that could be construed as a potential conflict of interest.
All claims expressed in this article are solely those of the authors and do not necessarily represent those of their affiliated organizations, or those of the publisher, the editors and the reviewers. Any product that may be evaluated in this article, or claim that may be made by its manufacturer, is not guaranteed or endorsed by the publisher.
Afonso, T. B., Simões, L. C., and Lima, N. (2021). Occurrence of filamentous fungi in drinking water: Their role on fungal-bacterial biofilm formation. Res. Microbiol. 172, 103791. doi:10.1016/J.RESMIC.2020.11.002
Al-Gabr, H. M., Zheng, T., and Yu, X. (2014). Fungi contamination of drinking water. Rev. Environ. Contam. Toxicol. 228, 121–139. doi:10.1007/978-3-319-01619-1_6
Ao, X., Liu, W., Sun, W., Cai, M., Ye, Z., Yang, C., et al. (2018). Medium pressure UV-activated peroxymonosulfate for ciprofloxacin degradation: Kinetics, mechanism, and genotoxicity. Chem. Eng. J. 345, 87–97. doi:10.1016/J.CEJ.2018.03.133
Azimi, Y., Liu, Y., Tan, T. C., Allen, D. G., and Farnood, R. R. (2017). The tail of two models: Impact of circularity and biomass non-homogeneity on UV disinfection of wastewater flocs. Water Res. 126, 70–78. doi:10.1016/J.WATRES.2017.09.011
Beck, S. E., Ryu, H., Boczek, L. A., Cashdollar, J. L., Jeanis, K. M., Rosenblum, J. S., et al. (2017). Evaluating UV-C LED disinfection performance and investigating potential dual-wavelength synergy. Water Res. 109, 207–216. doi:10.1016/j.watres.2016.11.024
Ben Said, M., Masahiro, O., and Hassen, A. (2010). Detection of viable but non cultivable Escherichia coli after UV irradiation using a lytic Qβ phage. Ann. Microbiol. 60, 121–127. doi:10.1007/s13213-010-0017-4
Betzalel, Y., Gerchman, Y., Cohen-Yaniv, V., and Mamane, H. (2020). Multiwell plates for obtaining a rapid microbial dose-response curve in UV-LED systems. J. Photochem Photobiol. B 207, 111865. doi:10.1016/j.jphotobiol.2020.111865
Bohrerova, Z., and Linden, K. G. (2007). Standardizing photoreactivation: Comparison of DNA photorepair rate in Escherichia coli using four different fluorescent lamps. Water Res. 41, 2832–2838. doi:10.1016/J.WATRES.2007.03.015
Bolton, J. R. (2000). Calculation of ultraviolet fluence rate distributions in an annular reactor: Significance of refraction and reflection. Water Res. 34, 3315–3324. doi:10.1016/S0043-1354(00)00087-7
Carlos Rivera y Liliana Ochoa (2018). Caracterización microbiológica de las aguas de los ríos de la ciudad de Cuenca. Available at: https://dspace.ucuenca.edu.ec/bitstream/123456789/31338/1/Trabajo%20de%20Titulaci%C3%B3n.pdf (Accessed December 5, 2022).
Carré, E., Pérot, J., Jauzein, V., and Lopez-Ferber, M. (2018). Impact of suspended particles on UV disinfection of activated-sludge effluent with the aim of reclamation. J. Water Process Eng. 22, 87–93. doi:10.1016/J.JWPE.2018.01.016
Clauß, M. (2006). Higher effectiveness of photoinactivation of bacterial spores, UV resistant vegetative bacteria and mold spores with 222 nm compared to 254 nm wavelength. Acta hydrochimica hydrobiologica 34, 525–532. doi:10.1002/AHEH.200600650
Doggett, M. S. (2000). Characterization of fungal biofilms within a municipal water distribution system. Appl. Environ. Microbiol. 66, 1249–1251. doi:10.1128/AEM.66.3.1249-1251.2000
Duque, P., et al. (2022). (PDF) proceedings of the V ibero-American congress of smart cities (ICSC-cities 2022), 135–148. Available at: https://www.researchgate.net/publication/368567663_Proceedings_of_the_V_Ibero-American_Congress_of_Smart_Cities_ICSC-CITIES_2022 (Accessed April 22, 2023).
Esbelin, J., Mallea, S., Ram, A. F. J., and Carlin, F. (2013). Role of pigmentation in protecting Aspergillus Niger conidiospores against pulsed light radiation. Photochem Photobiol. 89, 758–761. doi:10.1111/PHP.12037
Figawa (2009). Ultraviolet disinfection in water treatment. Technical Report 01 | 08 – Revised Version of Technical.
Geeraerd, A. H., Valdramidis, V. P., and Van Impe, J. F. (2005). GInaFiT, a freeware tool to assess non-log-linear microbial survivor curves. Int. J. Food Microbiol. 102, 95–105. doi:10.1016/J.IJFOODMICRO.2004.11.038
Guo, M., Huang, J., Hu, H., and Liu, W. (2011). Growth and repair potential of three species of bacteria in reclaimed wastewater after UV disinfection. Biomed. Environ. Sci. 24, 400–407. doi:10.3967/0895-3988.2011.04.011
Hageskal, G., Gaustad, P., Heier, B. T., and Skaar, I. (2007). Occurrence of moulds in drinking water. J. Appl. Microbiol. 102, 774–780. doi:10.1111/J.1365-2672.2006.03119.X
Hallmich, C., and Gehr, R. (2010). Effect of pre- and post-UV disinfection conditions on photoreactivation of fecal coliforms in wastewater effluents. Water Res. 44, 2885–2893. doi:10.1016/J.WATRES.2010.02.003
Hijnen, W. A. M., Beerendonk, E. F., and Medema, G. J. (2006). Inactivation credit of UV radiation for viruses, bacteria and protozoan (oo)cysts in water: A review. Water Res. 40, 3–22. doi:10.1016/j.watres.2005.10.030
Hoben, H. J., and Somasegaran, P. (1982). Comparison of the pour, spread, and drop plate methods for enumeration of rhizobium spp. in inoculants made from presterilized peat. Appl. Environ. Microbiol. 44, 1246–1247. doi:10.1128/AEM.44.5.1246-1247.1982
Kashimada, K., Kamiko, N., Yamamoto, K., and Ohgaki, S. (1996). Assessment of photoreactivation following ultraviolet light disinfection. Water Sci. Technol. 33, 261–269. doi:10.2166/wst.1996.0683
Keshavarzfathy, M., Hosoi, Y., Oguma, K., and Taghipour, F. (2021). Experimental and computational evaluation of a flow-through UV-LED reactor for MS2 and adenovirus inactivation. Chem. Eng. J. 407, 127058. doi:10.1016/J.CEJ.2020.127058
Kowalski, W., and Kowalski, W. (2009). “Pulsed UV systems,” in Ultraviolet germicidal irradiation handbook (Springer Berlin Heidelberg), 383–398. doi:10.1007/978-3-642-01999-9_16
Li, X., Cai, M., Wang, L., Niu, F., Yang, D., and Zhang, G. (2019). Evaluation survey of microbial disinfection methods in UV-LED water treatment systems. Sci. Total Environ. 659, 1415–1427. doi:10.1016/J.SCITOTENV.2018.12.344
Lindenauer, K. G., and Darby, J. L. (1994). Ultraviolet disinfection of wastewater: Effect of dose on subsequent photoreactivation. Water Res. 28, 805–817. doi:10.1016/0043-1354(94)90087-6
Liu, J., Zhou, L., Chen, J. H., Mao, W., Li, W. J., Hu, W., et al. (2014). Role of ozone in UV-C disinfection, demonstrated by comparison between wild-type and mutant conidia of Aspergillus Niger. Photochem Photobiol. 90, 615–621. doi:10.1111/PHP.12217
Maghsoodi, M., Lowry, G. L., Smith, I. M., and Snow, S. D. (2022). Evaluation of parameters governing dark and photo-repair in UVC-irradiated Escherichia coli. Environ. Sci. (Camb) 8, 407–418. doi:10.1039/D1EW00644D
Mamane-Gravetz, H., Linden, K. G., Cabaj, A., and Sommer, R. (2005). Spectral sensitivity of Bacillus subtilis spores and MS2 coliphage for validation testing of ultraviolet reactors for water disinfection. Environ. Sci. Technol. 39, 7845–7852. doi:10.1021/ES048446T
Marugán, J., van Grieken, R., Sordo, C., and Cruz, C. (2008). Kinetics of the photocatalytic disinfection of Escherichia coli suspensions. Appl. Catal. B 82, 27–36. doi:10.1016/J.APCATB.2008.01.002
Mattle, M. J., and Kohn, T. (2012). Inactivation and tailing during UV254 disinfection of viruses: Contributions of viral aggregation, light shielding within viral aggregates, and recombination. Environ. Sci. Technol. 46, 10022–10030. doi:10.1021/ES302058V
Moreno-Andrés, J., Romero-Martínez, L., Acevedo-Merino, A., and Nebot, E. (2017). UV-based technologies for marine water disinfection and the application to ballast water: Does salinity interfere with disinfection processes? Sci. Total Environ. 581–582, 144–152. doi:10.1016/J.SCITOTENV.2016.12.077
Mukherjee, D., Singh, S., Kumar, M., Kumar, V., Datta, S., and Dhanjal, D. S. (2018). “Fungal biotechnology: Role and aspects,” in Fungi and their role in sustainable development: Current perspective, 91–103. doi:10.1007/978-981-13-0393-7_6/TABLES/3
Narita, K., Asano, K., Naito, K., Ohashi, H., Sasaki, M., Morimoto, Y., et al. (2020). Ultraviolet C light with wavelength of 222 nm inactivates a wide spectrum of microbial pathogens. J. Hosp. Infect. 105, 459–467. doi:10.1016/J.JHIN.2020.03.030
Nebot Sanz, E., Salcedo Dávila, I., Andrade Balao, J. A., and Quiroga Alonso, J. M. (2007). Modelling of reactivation after UV disinfection: Effect of UV-C dose on subsequent photoreactivation and dark repair. Water Res. 41, 3141–3151. doi:10.1016/j.watres.2007.04.008
Nourmoradi, H., Nikaeen, M., Stensvold, C. R., and Mirhendi, H. (2012). Ultraviolet irradiation: An effective inactivation method of Aspergillus spp. in water for the control of waterborne nosocomial aspergillosis. Water Res. 46, 5935–5940. doi:10.1016/J.WATRES.2012.08.015
Nyangaresi, P. O., Qin, Y., Chen, G., Zhang, B., Lu, Y., and Shen, L. (2018). Effects of single and combined UV-LEDs on inactivation and subsequent reactivation of E. coli in water disinfection. Water Res. 147, 331–341. doi:10.1016/j.watres.2018.10.014
Pereira, V. J., Marques, R., Marques, M., Benoliel, M. J., and Barreto Crespo, M. T. (2013). Free chlorine inactivation of fungi in drinking water sources. Water Res. 47, 517–523. doi:10.1016/J.WATRES.2012.09.052
Quek, P. H., and Hu, J. (2008). Influence of photoreactivating light intensity and incubation temperature on photoreactivation of Escherichia coli following LP and MP UV disinfection. J. Appl. Microbiol. 105, 124–133. doi:10.1111/j.1365-2672.2008.03723.x
Rattanakul, S., and Oguma, K. (2018). Inactivation kinetics and efficiencies of UV-LEDs against Pseudomonas aeruginosa, Legionella pneumophila, and surrogate microorganisms. Water Res. 130, 31–37. doi:10.1016/j.watres.2017.11.047
Rodríguez-Chueca, J., Ormad, M. P., Mosteo, R., and Ovelleiro, J. L. (2015). Kinetic modeling of Escherichia coli and Enterococcus sp. inactivation in wastewater treatment by photo-Fenton and H2O2/UV–vis processes. Chem. Eng. Sci. 138, 730–740. doi:10.1016/J.CES.2015.08.051
Romero-Martínez, L., Moreno-Andrés, J., Acevedo-Merino, A., and Nebot, E. (2014). Improvement of ballast water disinfection using a photocatalytic (UV-C + TiO2) flow-through reactor for saltwater treatment. J. Chem. Technol. Biotechnol. 89, 1203–1210. doi:10.1002/JCTB.4385
Romero-Martínez, L., Duque-Sarango, P., Acevedo-Merino, A., and Nebot, E. (2019). Comparing the inactivating efficacy of enteric bacteria in seawater treated with different configurations of continuous flow-through ultraviolet devices: Single-pass and recirculation. J. Chem. Technol. Biotechnol. 94, 2980–2989. doi:10.1002/jctb.6108
Romero-Martínez, L., Duque-Sarango, P., González-Martín, C., Moreno-Andrés, J., Acevedo-Merino, A., and Nebot, E. (2023). Inactivation efficacy and reactivation of fecal bacteria with a flow-through LED ultraviolet reactor: Intraspecific response prevails over interspecific differences. J. Water Process Eng. 52, 103497. doi:10.1016/J.JWPE.2023.103497
Rubio, D., Casanueva, J. F., and Nebot, E. (2013). Improving UV seawater disinfection with immobilized TiO2: Study of the viability of photocatalysis (UV254/TiO2) as seawater disinfection technology. J. Photochem Photobiol. A Chem. 271, 16–23. doi:10.1016/J.JPHOTOCHEM.2013.08.002
Salcedo, I., Andrade, J. A., Quiroga, J. M., and Nebot, E. (2007). Photoreactivation and dark repair in UV-treated microorganisms: Effect of temperature. Appl. Environ. Microbiol. 73, 1594–1600. doi:10.1128/AEM.02145-06
Setlow, R. B., and Carrier, W. L. (1964). The disappearance of thymine dimers from DNA: AN error-correcting. Proc. Natl. Acad. Sci. U. S.51, 226–231. doi:10.1073/pnas.51.2.226
Silva, A. B., Lima Filho, N. M., Palha, M. A. P. F., and Sarmento, S. M. (2013). Kinetics of water disinfection using UV-C radiation. Fuel 110, 114–123. doi:10.1016/J.FUEL.2012.11.026
Sinha, R. P., and Häder, D. P. (2002). UV-Induced DNA damage and repair: A review. Photochem. Photobiological Sci. 1, 225–236. doi:10.1039/B201230H
Song, K., Mohseni, M., and Taghipour, F. (2016). Application of ultraviolet light-emitting diodes (UV-LEDs) for water disinfection: A review. Water Res. 94, 341–349. doi:10.1016/j.watres.2016.03.003
Song, K., Taghipour, F., and Mohseni, M. (2019). Microorganisms inactivation by wavelength combinations of ultraviolet light-emitting diodes (UV-LEDs). Sci. Total Environ. 665, 1103–1110. doi:10.1016/J.SCITOTENV.2019.02.041
Tosa, K., and Hirata, T. (1999). Photoreactivation of enterohemorrhagic Escherichia coli following UV disinfection. Water Res. 33, 361–366. doi:10.1016/S0043-1354(98)00226-7
UNICEF, and WHO (2017). Progress on drinking water, sanitation and hygiene. Available at: https://apps.who.int/iris/bitstream/handle/10665/258617/9?sequence=1 (Accessed March 7, 2023).
Vélez-Colmenares, J. J., Acevedo, A., and Nebot, E. (2011). Effect of recirculation and initial concentration of microorganisms on the disinfection kinetics of Escherichia coli. Desalination 280, 20–26. doi:10.1016/J.DESAL.2011.06.041
Wan, Q., Wen, G., Cao, R., Xu, X., Zhao, H., Li, K., et al. (2020). Comparison of UV-LEDs and LPUV on inactivation and subsequent reactivation of waterborne fungal spores. Water Res. 173, 115553. doi:10.1016/J.WATRES.2020.115553
Wan, Q., Cao, R., Wen, G., Xu, X., Xia, Y., Wu, G., et al. (2022). Efficacy of UV-LED based advanced disinfection processes in the inactivation of waterborne fungal spores: Kinetics, photoreactivation, mechanism and energy requirements. Sci. Total Environ. 803, 150107. doi:10.1016/J.SCITOTENV.2021.150107
Wan, Q., Wen, G., Cui, Y., Cao, R., Xu, X., Wu, G., et al. (2023). Occurrence and control of fungi in water: New challenges in biological risk and safety assurance. Sci. Total Environ. 860, 160536. doi:10.1016/J.SCITOTENV.2022.160536
Wen, G., Xu, X., Zhu, H., Huang, T., and Ma, J. (2017). Inactivation of four genera of dominant fungal spores in groundwater using UV and UV/PMS: Efficiency and mechanisms. Chem. Eng. J. 328, 619–628. doi:10.1016/J.CEJ.2017.07.055
Wen, G., Deng, X., Wan, Q., Xu, X., and Huang, T. (2019). Photoreactivation of fungal spores in water following UV disinfection and their control using UV-based advanced oxidation processes. Water Res. 148, 1–9. doi:10.1016/J.WATRES.2018.10.028
Wu, G., Zhao, H., Wan, Q., Xu, X., Cao, R., Li, K., et al. (2022). Inactivation and subsequent reactivation of Aspergillus species by the combination of UV and monochloramine: Comparisons with UV/chlorine. J. Environ. Sci. (China) 117, 105–118. doi:10.1016/J.JES.2022.03.021
Xu, X., Zuo, J., Wan, Q., Cao, R., Xu, H., Li, K., et al. (2022). Effective inactivation of fungal spores by the combined UV/PAA: Synergistic effect and mechanisms. J. Hazard Mater 430, 128515. doi:10.1016/J.JHAZMAT.2022.128515
Keywords: ultraviolet disinfection, fungal spores, photoreactivation, aspergillus, penicillium, flow-through UV-C reactor
Citation: Duque-Sarango P, Delgado-Armijos N, Romero-Martínez L and Pinos-Vélez V (2023) Assessing the potential of ultraviolet irradiation for inactivating waterborne fungal spores: kinetics and photoreactivation studies. Front. Environ. Sci. 11:1212807. doi: 10.3389/fenvs.2023.1212807
Received: 26 April 2023; Accepted: 17 July 2023;
Published: 28 July 2023.
Edited by:
Rui C. Martins, University of Coimbra, PortugalReviewed by:
Samira Nahim Granados, Environmental and Technological Research, SpainCopyright © 2023 Duque-Sarango, Delgado-Armijos, Romero-Martínez and Pinos-Vélez. This is an open-access article distributed under the terms of the Creative Commons Attribution License (CC BY). The use, distribution or reproduction in other forums is permitted, provided the original author(s) and the copyright owner(s) are credited and that the original publication in this journal is cited, in accordance with accepted academic practice. No use, distribution or reproduction is permitted which does not comply with these terms.
*Correspondence: Paola Duque-Sarango, cGR1cXVlQHVwcy5lZHUuZWM=
Disclaimer: All claims expressed in this article are solely those of the authors and do not necessarily represent those of their affiliated organizations, or those of the publisher, the editors and the reviewers. Any product that may be evaluated in this article or claim that may be made by its manufacturer is not guaranteed or endorsed by the publisher.
Research integrity at Frontiers
Learn more about the work of our research integrity team to safeguard the quality of each article we publish.