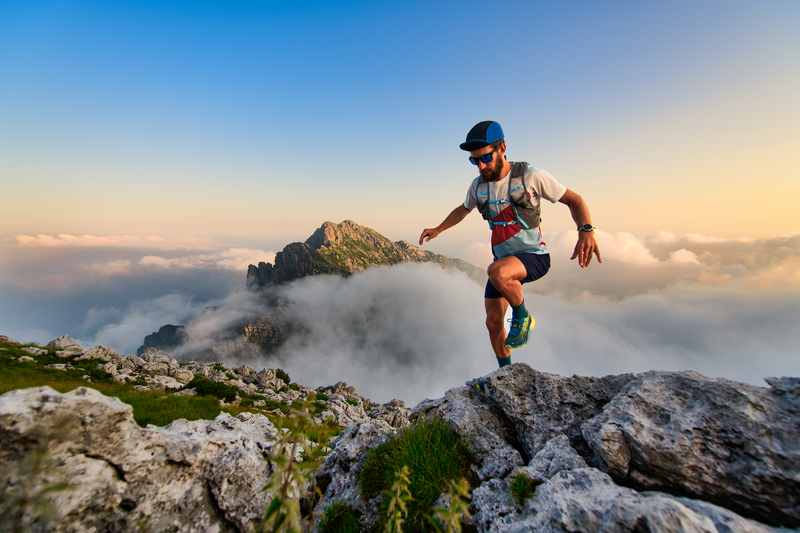
95% of researchers rate our articles as excellent or good
Learn more about the work of our research integrity team to safeguard the quality of each article we publish.
Find out more
ORIGINAL RESEARCH article
Front. Environ. Sci. , 23 August 2022
Sec. Freshwater Science
Volume 10 - 2022 | https://doi.org/10.3389/fenvs.2022.966418
This article is part of the Research Topic Hydropower: from Ecology to Policy View all 9 articles
A correction has been applied to this article in:
Corrigendum: Stranding of larval nase (Chondrostoma nasus L.) depending on bank slope, down-ramping rate and daytime
Rapid water level decreases due to hydropeaking are known to negatively affect riverine biota, mainly due to the stranding of organisms in the river bank area that becomes regularly dewatered. Even though studies of the last decades have focused on salmonid fish, also cyprinids may be affected. However, limited knowledge is available of this fish family. Therefore, we conducted mesocosm experiments under semi-natural conditions, simulating single hydropeaking events at two different lateral bank slopes (2% and 5%) with varying down-ramping rates (0.7–3.0 cm min−1) during day and night. As a response parameter, we quantified stranding rates of different larval stages (III-IV and V) of common nase (Chondrostoma nasus L.). The experiments revealed that lower sloped banks exhibited distinctly higher stranding rates than steeper ones. Daytime revealed a similar pattern, with more fish becoming stranded at night than during the day, and this was consistent for all down-ramping rates. The data also indicate increased stranding with higher down-ramping rates, particularly at low sloped riverbanks, and interaction effects between the tested parameters. Overall, this study, for the first time, quantifies the consequences of flow down-ramping on nase larvae, also revealing differences between larval stages. The gained information will, therefore, advance the ongoing discussion on hydropeaking mitigation by providing a deeper understanding of the effects of artificial sub-daily flow fluctuations on the early life stages of cyprinid fish. Our results can inform management and policy to sharpen existing mitigation concepts and fine-tune hydropower operations to reduce negative effects on riverine ecosystems.
Hydropower is the leading form of renewable energy, representing around 17% of the world’s total electricity generation (REN21, 2021). Although decades of research indicate that hydropower generation is accompanied by diverse consequences for riverine ecosystems (Cushman, 1985; Dynesius and Nilsson, 1994; Dudgeon et al., 2006; Hayes et al., 2018; Schmutz and Sendzimir, 2018), it is commonly considered to be a clean, sustainable and environmentally friendly energy source (Bejarano et al., 2018; Alfredsen et al., 2022; IHA, 2022). Due to the increase in global energy demand (Bejarano et al., 2018; van Ruijven et al., 2019) and the envisaged promotion for renewable energy sources (e.g., EU Renewable Energy Directive 2018/2001), hydropower production is expected to expand (Zarfl et al., 2019).
As a critical element of the power system, hydropower—in particular, peak-operating hydropower plants—serves as a load-balancing power source, e.g., for grid fluctuations caused by other renewable energies, as it allows to store and generate energy on demand (Harby et al., 2015; Greimel et al., 2016; Hayes et al., 2022). This mode of hydropower operation, referred to as “hydropeaking”, coincides with energy demand and stock market prices (Harby et al., 2015), and is characterized by frequent daily or sub-daily artificial flow fluctuations in the downstream water body (Greimel et al., 2016; Hayes et al., 2022).
Hydropeaking has been identified as one of the key stressors in mountainous rivers (Schmutz et al., 2015), as it alters the natural flow regime (Carolli et al., 2015; Greimel et al., 2016; Li and Pasternack, 2021) and hydraulic factors such as water level, flow velocity and bed shear stress (Shen & Diplas, 2010), river morphology (Tuhtan et al., 2012; Hauer et al., 2014, 2017; Vanzo et al., 2016b) and water temperature (Zolezzi et al., 2011; Vanzo et al., 2016a). Further, the related re-suspension of fine sediments increases turbidity and clogging risk of sediments (Hauer et al., 2019).
As a consequence, hydropeaking operation may lead to a reduction of physical habitat availability and habitat persistence (Freeman et al., 2001) and may affect connectivity of species and life-stage specific habitats (Person, 2013). These changes of habitat quality and quantity lead to biomass reduction (Hayes et al., 2021; Smokorowski, 2022) as a consequence of downstream displacement and drift of aquatic organisms (Auer et al., 2017; Schülting et al., 2019), lowered growth rate of fish in a long term (Puffer et al., 2015), reduced spawning and rearing success (Grabowski and Isely, 2007; Casas-Mulet et al., 2015), as well as stranding and trapping in dewatered areas (Perry and Perry, 1986; Nagrodski et al., 2012).
Regarding fish, multiple studies have highlighted that stranding affects riverine biota (Hamilton and Buell, 1976; Bauersfeld, 1978; Cushman, 1985; Hunter, 1992; Hauer et al., 2014; Auer et al., 2017) and depends on a wide range of biotic and abiotic factors (Larrieu et al., 2021). During flow increases, fish shift laterally towards the shoreline to avoid unsuitable hydraulic conditions (Greimel et al., 2018). However, in hydropeaked rivers, exactly this shoreline zone puts fish at risk of stranding at dewatering due to flow down-ramping (Young et al., 2011; Nagrodski et al., 2012; Harby and Noack, 2013; Auer et al., 2017). Particularly early life stages are highly sensitive to down-ramping events (Hunter, 1992; Harby and Noack, 2013; Hayes et al., 2019). Postemergence larvae and early juveniles exhibit relatively low swimming capacities (Heggenes and Traaen, 1988); thereby, they rely on shallow shore habitats of low flow velocity (Moore and Gregory, 1988; Baras and Nindaba, 1999).
Specifically, the stranding risk depends on the physical conditions of the riverbanks, including morphological characteristics (Young et al., 2011; Hauer et al., 2014; Auer et al., 2017), substrate grain size (Hunter, 1992; Hauer et al., 2014), bank slope with increased stranding risk at lower gradients (Bauersfeld, 1978; Hunter, 1992; Bell et al., 2008), season and daytime with contrasting direction of effects (Bradford et al., 1995; Saltveit et al., 2001; Puffer et al., 2015; Auer et al., 2017), peak amplitude and down-ramping rate with a significant decrease in the stranding risk with reduced down-ramping rates (Bradford et al., 1995; Halleraker et al., 2003; Auer et al., 2017).
Studies on fish stranding have so far focused on salmonid species (Nagrodski et al., 2012), since hydropeaking operations are predominant in high-elevation topographies, mostly impacting rivers in the trout and grayling fish regions (Schmutz et al., 2015; Hayes et al., 2021). However, hydropeaking may also be caused by run-off-the-river power plants (Greimel et al., 2016; Hayes et al., 2022), leading to lower-intensity but high-frequency hydropeaking, so-called hydro-fibrillation (Greimel et al., 2016); in Austria, this phenomenon is frequently found in regulated rivers, including rivers inhabited by cyprinids.
Recent studies underline the knowledge gap regarding non-salmonid fish species also affected by hydropeaking (Costa et al., 2018; Moreira et al., 2019); to date, however, the species-rich cyprinid fish family has hardly been considered in hydropeaking research (Moreira et al., 2019), despite such works being needed for mitigation efforts in non-salmonid rivers (Godinho et al., 2022). The common nase (Chondrostoma nasus L.), for example, is a cyprinid indicator species widespread throughout Europe and home to grayling and barbel zone of rivers (Schiemer et al., 2002). Considering that the nase is sensitive to hydroengineering activities (Peňáz, 1996), it is a suitable target species to quantify cyprinid fish stranding following hydropeaking down-ramping events, particularly regarding early life stages. Larvae and juvenile nase inhabit littoral habitats (Keckeis et al., 1997) of mainly medium to large rivers (Schiemer and Spindler, 1989; Copp et al., 1991; Kainz and Gollmann, 1999). Nase larvae inhabit shallow (0–30 cm) and slow-flowing (<10 cm s−1) shoreline habitats (Keckeis et al., 1997; Ovidio and Philippart, 2008; Schludermann et al., 2009) along gravel bars, as well as bay structures with fine sediments (Hofer and Kirchhofer, 1996; Keckeis et al., 1997; Baras and Nindaba, 1999).
To fill the knowledge gaps on cyprinid fish affected by hydropeaking, we quantified, for the first time, the stranding of nase larvae in response to down-ramping events. Specifically, we used semi-natural mesocosms, mimicking suitable larval habitats, to test the single and combined effects of 1) varying down-ramping rates at 2) two different lateral bank slopes, 3) each during day and night on stranding of 4) different larval stages of nase. We hypothesized that stranding is positively linked to increasing down-ramping rates and that a lower-sloped bank may exacerbate these effects. Moreover, we expected higher stranding during night experiments compared to daytime and higher sensitivity of earlier larval stages.
We performed the ethohydraulic experiments from 19 May to 16 June 2021 at the “Hydromorphological and Temperature Experimental Channels” (HyTEC) in Lunz am See, Lower Austria (47°51′ 22.5″ N, 15°02′12.0″ E; https://hydropeaking.boku.ac.at). The HyTEC facility comprises (a) two parallel arranged and symmetrically designed outdoor experimental channels, (b) a control unit (Siemens Simetic S7-300) connected with ultrasonic sensors (Rittmeyer RISONIC 2000; Sensor Typ A) and pipe gate valves allowing to program, monitor and record discharge rates individually for both channels, and (c) fish rearing tanks. Nutrient-poor water is abstracted with up to 600 L s−1 from Lake Lunz via two pipes (DN 400) at two different depths (−0.75 and −10 m below the mean lake water level). The water temperature can be adjusted by controlled mixing of the water from both pipes in two separate basins (6 m × 4 m) before being discharged to each channel. Similarly, the inflow rate and water temperature for the fish rearing tanks can be individually adjusted via ball valves.
Nase larvae originated from wild fish stocks. Spawners were retrieved from the Schwechat River, Lower Austria, and eggs and sperm were stripped off manually. After hatching, individuals were reared in three circular flow-through tanks at the HyTEC facility (approx. 0.7 m³ each). Both, inflow magnitude (0.5 L s−1 per tank) and water mixing ratio of surface and deep water remained constant. Fish larvae were fed with zooplankton obtained daily from the lake water according to their natural feeding needs (Reckendorfer et al., 2001; Schiemer et al., 2002). Additionally, brine shrimp (Artemia sp.) prey was cultured and fed alive several times a day. Feedings were done randomly throughout the day to minimize habituation effects. Rearing tanks were partially covered to provide shade and shelter, and small stones were used as structural elements to provide cover and different flow patterns. After the trials, larvae were kept in separate tanks and later transferred to a rearing pond where they continued to grow before being released at the end of all experimental runs.
We examined the increasing body size of larvae by measuring the total fish length (TL ± 0.1 mm) of randomly selected larvae (30 ind. per measurement) out of the rearing tanks using the image-analysis tool ImageJ (Schneider et al., 2012). In the experimental period, the length of larvae ranged from 11.0 mm to 19.2 mm (mean TL: 14.1 mm ± 1.0 SD), with a mean growth rate of 0.13 mm per day. In addition, larval development stages were classified according to Peňáz (1974), with larvae in the third (III) to fifth (V) larval stage (Figure 1B).
FIGURE 1. (A) Side view of both experimental channels with the four embedded mesocosms: two each with a bank slope I of 2% and 5%, respectively; blue shadings indicate the wetted area at low flows and high flows during the experiments (B) Larvae stages used in the experiments; top: stage III-IV with TL = 15.0 mm, bottom: stage V with TL = 19.2 mm. For details, see Peňáz (1974); (C) Larval nase (Chondrostoma nasus L.) stranded by rapid down-ramping during the experiments.
Due to the vulnerability of the small-sized study organisms (see Section 2.1), experiments were conducted in four customized mesocosms (2.25 m × 2 m) embedded in the shore area of the channels (Figure 1). The transition between the channel bed and the mesocosms were gently ramped with the substrate to homogenize the flow within the mesocosms. The mesocosms consisted of 1) a platform made of a steel frame and formwork panels on top and 2) a small aluminum channel (width: 25 cm, depth: 7.5 cm) mounted to the platform representing the deepest part allowing easy clearing of fish at low flow conditions (further named “low flow channel”). 3) Frames made of thin-walled structural profiles covered with fine nets (mesh size: 0.75 mm) were bolted to the outer edge of the platform. The construction was mounted laterally rotatabe 4) on an outer base frame made of profiles, allowing a precise adjustment of the bank slope (for a detailed illustration, see the Supplementary Material).
The flat gravel bank area (the ramping zone) of each mesocosm was filled with sediments (height of layer approx. 1.5 cm), dominated by sand and fine gravel (dmax<10 mm; d10 = 0.6 mm; d50 = 2.2 mm; d90 = 6.0 mm). The substrate remained the same for all mesocosms and replicates. To ensure constant experimental conditions over time, the sediments were smoothed and the nets were checked and cleaned before each experimental run.
The discharge rate during the experiments was 80 L s−1 at high flow and 10 L s−1 at low flow for each channel (Figure 2). In each of the two mesocosms per experimental channel, two different lateral bank slopes were tested: 1) 2% and 2) 5% (Figure 1A). These values were based on previous experiments (Auer et al., 2014) and represent typical shoreline habitats inhabited by early fish life stages (e.g., Bauersfeld, 1978; Pander et al., 2017). All mesocosms had a longitudinal slope of 0.5%, identical to the slope of the two facilities’ channels. At high flow conditions, the water depth at the shoreline was 4 cm for all mesocosms. The water depth in the low flow channel was 17 cm in the 2% sloped mesocosms and 23 cm in the 5% sloped during high flow. The water depth was around 10 cm at the low flow channel for both bank slope setups during low flow conditions. The dewatering width—which corresponds to the zone of potential stranding during down-ramping (i.e., the ramping zone)—was 1.75 m in all mesocosms (Figure 2). Flow velocity and water depths were measured in all mesocosms using a 2-D ADV flow meter (SonTek FlowTracker 2) and a micro propeller (Höntzsch FT25GFE-MN20/100/P6). Flow velocity close to the shoreline at high flow was <0.075 m s−1 (Figure 2). Overall, the hydraulic setting in the mesocosms provided preferred habitats for nase larvae, deeming the mesocosms suitable for hydropeaking down-ramping experiments for this species and life stage (e.g., Hofer and Kirchhofer, 1996; Keckeis et al., 1997; Flore and Keckeis, 1998; Flore et al., 2001; Keckeis and Schiemer, 2002; Zens et al., 2017).
FIGURE 2. Cross-sectional plot of flow velocity distribution in the mesocosms at high flow rate of 80 L s−1 (top) and low flow rate at 10 L s−1 (bottom) for the 2% (left) and 5% bank slope (right) setups.
In total, we tested 22 experimental scenarios to analyze the effects of 1) varying down-ramping rates, 2) two different lateral bank slopes, 3) daytime, and 4) different larval stages of nase (Table 1). To assess the effects of varying vertical down-ramping rates, we tested five down-ramping rates: 0.7 cm min−1, 1.1 cm min−1, 1.5 cm min−1, 2.0 cm min−1, and 3.0 cm min−1. Down-ramping rates were programmed using the facilities’ control unit and verified with pressure probes (Aquitronic ATP05; resolution 5 s) for each mesocosm separately. There was no interdependence between the two mesocosms within a channel (5% setup upstream; 2% setup downstream; see Figure 1A), as evidenced by flow measurements (see Figure 2). Considering that the experiments were performed simultaneously in the mesocosms (see Figure 1A), the down-ramping rates of 1.1 cm min−1, 1.5 cm min−1, and 2.0 cm min−1 were achieved for both tested bank slope setups (2%; 5%). The lowest down-ramping rate (0.7 cm min−1), however, was achieved only at the downstream mesocosms (2% bank slope setup), while the highest (3.0 cm min−1) was achieved only at the upstream mesocosms (5% bank slope setup). This is due to the channels’ retention effect, lowering the programmed down-ramping rate for the downstream mesocosms. In later experiments, more developed larval stages were tested with rather high down-ramping rates. Here, down-ramping rates of 1.1 cm min−1 and 1.5 cm min−1 were tested only during the day and with only a few replicates.
TABLE 1. Overview of the experimental scenarios with the number of independent replicates (n) used for statistical analysis dependent on tested parameters: daytime, bank slope, down-ramping rate, and larval stage.
The majority of experiments were performed during the day (i.e., under daylight conditions, 10:00–18:30) and at night (i.e., after sunset, 22:15–02:00) to assess the influence of daytime (i.e., the photoperiod). At night, meticulous care was taken to avoid shadowing and light pollution. Therefore, e.g., we used headlamps with red lights at low levels for counting and stocking fish.
Down-ramping experiments were performed according to a repeatable design to quantify the stranding rate of early life stages of nase: 1) Immediately before being stocked into the mesocosms, 100 individuals were separated from the rearing tanks using a 1 L bucket. 2) Larvae were stocked at high flow (80 L s−1) in the upper part of the mesocosms around 20 cm from the shore, offering suitable larvae habitats (Figure 2). The water temperature was kept constant between the rearing tanks and mesocosms. To avoid a flight response of larvae, stocking was done gently inclined against the flow, waiting until all larvae had left the stocking bucket. To prevent stocking-related responses during the down-ramping experiment, 3) a period of 15 min with unchanged flow was specified as acclimation time (based on preliminary experiments). 4) Subsequently, the high flow was automatically reduced (down-ramping) by the control unit of HyTEC-facility to the low flow rate of 10 L s−1. To quantify stranded individuals after the down-ramping, 5) the mesocosms were surveyed according to a defined scheme: First, larvae stranded on the dewatered substrate (see Figure 1C) were quantified and removed immediately. Secondly, larvae found close to the downstream net (≤1 cm) were counted together with larvae retrieved from the nets. These specimens were excluded from stranding calculations in a later step, as observations revealed that most of them were displaced into the net before down-ramping and were therefore not available for possible stranding (Heggenes and Traaen, 1988). Thirdly, larvae that remained in the low flow channel at the end of an experiment were cleared using small dip nets. Finally, sediments were re-flushed by increasing the flow to 60 L s−1, and the nets and sediments were examined for larvae once again. If it could not be clearly assigned whether such a larva was stranded or flushed from the nets, it was classified as non-stranded. Each step was performed according to the four-eyes principle. To ensure a high recapture rate and avoid that larvae remained in the mesocosms, sediments were also re-flushed and cleared from single remaining specimens before each experimental run. Overall, this elaborate assessment allowed a mean recapture rate of 99.1% ± 1 SD. Each larva participated in only one experiment.
As water temperature affects metabolism, growth, and tissue differentiation (Seikai et al., 1986; Pepin, 1991) and influences the behavior of fish (Volkoff and Rønnestad, 2020) the water temperature was recorded separately for each channel and all rearing tanks by the HyTEC’s control unit and HOBO Pendant Temperature/Light 64K Data Logger (UA-002-64). Water temperature remained constant within each experimental run (i.e., no thermopeaking) but varied within the experimental scenarios due to natural fluctuations of the lake water. Water temperatures were consistent with seasonal temperatures in typical habitats of nase, ranging from 8.5°C to 11.4°C (Figure 3).
FIGURE 3. Overview of experimental replicates (n = 126) regarding the history of the water temperature grouped by larval stage (senu Peňáz, 1974) and daytime (D = day; N = night).
The first set of stranding experiments, conducted from 19th May to 30th May, focused on very early developmental stages of nase classified as larval stage III-IV sensu Peňáz (1974) (see Table 1). Larval stages III and IV were pooled due to their similar morphological characteristics and associated challenges in classification and timing the experiments. For both stages, in contrast to larval stage V, the differentiation of the finfold is still not very advanced. As for swimming ability, the dorsal, caudal, ventral and anal fins are distinctly less developed compared to larval stage V. The second set of experiments focused on larval stage V. Trials with selected down-ramping rates were performed from 3rd June to 16th June (see Table 1). For readability, larval stage III-IV is coded “1” and larval stage V is coded “2” in Table 1 (see column “Experimental scenario”) and Figure 5.
For each trial, the frequency of stranded larvae (Strcalc) was calculated as follows:
whereby N is the count of larvae retrieved from the nets, N′ those found on the substrate close to the downstream net (≤1 cm), and C is the number of larvae cleared from the low flow channel. Missing individuals were assumed to be stranded, but hidden in the substrate and were therefore included for stranding quantifications.
The frequency of stranded larvae was standardized (Strrate) as follows:
This study assesses the variability in stranding of larval nase (target variable) in the context of different experimental conditions (independent variables: 1) varying down-ramping rates, 2) two bank slopes, 3) day and night, 4) different larval stages). In detail, this work aims to answer if differences in stranding rate can be detected and, if so, by which effect sizes (main and interaction effects) these differences can be explained. Such questions are commonly answered with general linear models. These, however, pose minimum requirements, such as multidimensional normal distribution or homogeneity of variance, which are not fully met.
Therefore, we used odds and odds ratios (OR) to identify variation in nase stranding. Odds and OR are widely used to analyze binary data in epidemiology (Schmidt and Kohlmann, 2008) and neuroscience (Sandercock, 1989) and are statistically appropriate approaches for the present data (see also Morris and Gardner, 1988; Sachs and Hedderich, 2006). Odds were calculated by stranded (Strcalc) and non-stranded (C) fish for each scenario [see also (1)]. Based on these, OR were calculated for pairs of experimental scenarios (A vs. B). Each experimental scenario consisted of an assortment of four parameters: daytime, bank slope, down-ramping rate, larval stage (see Table 1).
Yule (1912) was used to standardize OR to −1 to +1. Negative values are based on a lower ratio of stranded to non-stranded fish in scenario A, while positive values are based on a lower ratio of stranded to non-stranded individuals in scenario B. For Yule’s Q, we assumed the equivalence of the compared scenarios between −0.08 and 0.07. This equivalence assumption is common in medicine, but may vary between disciplines. To provide a proxy for the presence of statistical significance, with a significance level α of 0.05, we calculated the confidence interval (CI) of Yule’s Q using the logit method (see Woolf, 1955; Bewick et al., 2004). If the CI of a scenario combination does not overlap with the equivalence assumption, the treatment pairs can be regarded as different (Sandercock, 1989). To additionally express significance by the widely used p-values, the Cochran–Mantel–Haenszel test (CMH; Cochran, 1954; Mantel and Haenszel, 1959) and the power coefficient Phi were calculated. The significance level α was assumed to be 0.05.
To relate stranding rate to fish length, Spearman’s rank correlation coefficients were determined separately by bank slope and daytime, although the assumptions, e.g., normal distribution and homogeneity of variance were not met. The effect size was described according to Cohen (1988).
To explore the hierarchical structure of the independent variables (i.e., down-ramping rate, lateral bank slope, daytime, larval stage), we applied a decision tree-based model using the RPART-routine (CART-algorithm using Gini Index; see Therneau and Atkinson, 2022) with the dichotomized stranding rate as the target variable.
In total, 126 trials from 22 experimental scenarios were conducted with larval nase (Table 1). The number of replicates varied by scenario (Figure 4) for several reasons, including limited experimental period due to larval growth or less time at night due to longer daylight hours. Also, some replicates had to be excluded due to external factors such as heavy rain showers or other unintentional disturbances during trials.
FIGURE 4. Stranding rate for the 22 experimental scenarios depending on the down-ramping rate (0.7 cm min−1; 1.1 cm min−1; 1.5 cm min−1; 2.0 cm min−1; 3.0 cm min−1) with number of replicates (n). Experiments were performed with with larval stages III-IV (A,B) and V (C,D) at 2% (A,C) and 5% (B,D) bank slope, both during the day (D) and during the night (N). The boxplots with the associated bold lines and whiskers refer to median values and interquartile ranges. White squares indicate the mean value. The gray dots represent individual trials.
There was no evidence of a difference in stranding risk between both experimental channels (Q: −0.01; CI: −0.07–0.06; p = 0.799). Combining all trials, the median stranding rate was 0.05, ranging from 0.0 to 0.62 (see also Figures 4, 7). Figure 4 shows that the stranding rate varies depending on bank slope, daytime, larval stage and down-ramping rate. Therefore, we conducted pairwise odds ratio (OR) analysis of all experimental scenarios (one scenario consists of an assortment of these four parameters) to test for differences between them (Figure 5).
FIGURE 5. Pairwise comparisons of the experimental scenarios (for coding explanation, see Table 1): Yule’s Q and confidence interval (CI); complementary with Cochran–Mantel–Haenszel (CMH) test and power coefficient (Phi); sorted by Yule’s Q.
Stranding risk differed significantly comparing both bank slopes (Q: 0.52; CI: 0.47–0.58; p = 0) (see Figure 4). For the 2% bank slope (n = 61), the median stranding rate was 0.11, almost four times higher than the median stranding rate of 0.03 at the 5% bank slope (n = 65) (Figures 4, 7).
We found significantly higher stranding rates at night compared to day across all scenarios (Q: −0.48; CI: −0.54 to −0.43; p = 0) (Figure 4), with about three times higher stranding rates during trials at day (median = 0.036; n = 76) than during night experiments (median = 0.10; n = 50). When splitting the data by bank slope, larval stage and down-ramping rate, differences between stranding rates at day and night was significant for all comparisons (p = 0 for all scenarios at larval stage III-IV; p < 0.01 for scenarios at larval stage V), except for the scenario comparing day and night at the 5% bank slope with larval stage V and a down-ramping rate of 2.0 cm min−1 (Q: −0.30; CI: −0.74–0.15; p = 0.210) (see Figures 4D, 5).
Overall, larval stage III-IV exhibited stronger stranding differences between day and night (Q: −0.56; CI: −0.61 to −0.51; p = 0) than larval stage V (Q: −0.36; CI: −0.48 to −0.24; p < 0.001). Daytime trials with larval stage III-IV resulted in a median stranding rate of 0.05 (n = 43), while night experiments showed a median stranding rate of 0.24 (n = 26). For larval stage V, this was 0.02 (n = 33) and 0.06 (n = 24), respectively.
The strongest differences between day and night stranding (∆ = 0.24) were detected for larval stage III-IV when aggregating all down-ramping rates at 2% bank slope (Q: −0.52; CI: −0.59 to −0.45; p = 0), showing a median stranding rate of 0.16 during daytime trials (n = 22) compared to 0.40 at night experiments (n = 13) (see Figures 4A, 7).
The difference between day and night stranding was also comparably high at 5% bank slope and larval stage III-IV at the highest down-ramping rate of 2 cm min−1 (∆ = 0.19; see Figure 4B). When aggregating all down-ramping rates at 5% bank slope for larval stage III-IV, the median stranding rate was 0.04 during daytime trials (n = 21) compared to 0.17 at night experiments (n = 13) (see Figures 4B, 7), and this difference was statistically significant (Q: −0.7; CI: −0.78 to −0.62; p = 0). Although the stranding rates were generally low at 5% bank slope and larval stage V (Figure 4D), the difference between day and night stranding was significant for the higher down-ramping rate of 3 cm min−1 (Q: −0.35; CI: −0.57 to −0.13; p = 0.004), with stranding at night being more than 3-fold higher compared to day.
We found significantly higher stranding rates for larval stage III-IV compared to larval stage V (Q: 0.58; CI: 0.53–0.63; p = 0) (see Figure 4), with stranding rates about four times higher for trials with larval stage III-IV (median = 0.13; n = 69) compared to experiments with larval stage V (median = 0.03; n = 57). The differences in stranding rate between both larval stages were significant at 2% bank slope (Q: 0.59; CI: 0.53–0.65; p = 0) and at 5% bank slope (Q: 0.58; CI: 0.49–0.68; p = 0).
When splitting the data by bank slope, daytime, and down-ramping rate, the difference between both larval stages was significant for all feasible comparisons (p < 0.001 for all scenarios except for day experiments at 5% bank slope and a down-ramping rate of 2.0 cm min−1 with p < 0.05) (see Figures 4, 5). During the day at 2% bank slope and a down-ramping rate of 1.5 cm min−1, the median stranding rate was 0.19 for trials with larval stage III-IV (n = 8) and 0,02 for trials with larval stage V (n = 5), which is about nine times lower (Q: 0.84; CI: 0.75–0.94) (see Figures 4A,C, 5). During the night at 2% bank slope and a down-ramping rate of 1.5 cm min−1, the median stranding rate was 0.48 for trials with larval stage III-IV (n = 5) and 0.06 for trials with larval stage V (n = 4), which is eight times lower (Q: 0.86; CI: 0.79–0.92) (see Figures 4A,C, 5).
During the day at 5% bank slope and a down-ramping rate of 2.0 cm min−1, the median stranding rate was 0.012 for trials with larval stage III-IV (n = 7) and 0,01 for trials with larval stage V (n = 5) (Q: 0.42; CI: 0.07–0.78) (see Figures 4B,C, 5). During the night at 5% bank slope and a down-ramping rate of 2.0 cm min−1, the median stranding rate was 0.21 for trials with larval stage III-IV (n = 5) and 0.03 for trials with larval stage V (n = 4), which is about seven times higher at trials with larval stage III-IV compared to larval stage V (Q: 0.81; CI: 0.7–0.930) (see Figures 4B,C, 5).
We found differences between individual down-ramping rates, with the strongest differences at 2% bank slope and larval stage III-IV (Figure 4). At a down-ramping rate of 0.7 cm min−1, the median stranding rate was 0.11 (n = 12) compared to 0.17 at a down-ramping rate of 1.1 cm min−1 (n = 10) and 0.33 at a down-ramping rate of 1.5 cm min−1 (n = 13); which was significant for all comparisons (p < 0.001).
When splitting the data at the 2% bank slope by daytime, the median stranding rate during day trials at a down-ramping rate of 0.7 cm min−1 was 0.06 (n = 8) compared to 0.16 at a down-ramping rate of 1.1 cm min−1 (n = 6) and 0.19 at a down-ramping rate of 1.5 cm min−1 (n = 8). During night experiments, the median stranding rate at a down-ramping rate of 0.7 cm min−1 was 0.24 (n = 4) compared to 0.43 at a down-ramping rate of 1.1 cm min−1 (n = 4) and 0.48 at a down-ramping rate of 1.5 cm min−1 (n = 5) (Figure 4A).
All comparisons between individual down-ramping rates were significant at 2% bank slope and larval stage III-IV during the day and at night (p < 0.001 for the comparison between the down-ramping rates of 0.7 cm min−1 and 1.5 cm min−1 during day and between down-ramping rates of 0.7 cm min−1 and 1.5 cm min−1 as well as 1.1 cm min−1 and 1.5 cm min−1, both during night; p < 0.01 for the comparison between the down-ramping rates of 0.7 cm min−1 and 1.1 cm min−1 during day as well at night; see Figure 5), except for the comparison of the down-ramping rates of 1.1 cm min−1 and 1.5 cm min−1 during day trials (Q: −0.16; CI: −0.33–0; p = 0.054) (see Figure 4A, 5). At 5% bank slope and larval stage III-IV, the comparisons between individual down-ramping rates were significant at night between the down-ramping rates of 1.1 cm min−1 and 2.0 cm min−1 (Q: −0.32; CI: −0.5 to −0.14; p = 0.001) as well as 1.5 cm min−1 and 2.0 cm min−1 (Q: −0.2; CI: −0.39 to −0.02; p = 0.033) (see Figure 4B, 5). For larval stage V, differences between individual down-ramping rates during day and night were evident only at 2% bank slope (p = 0.001 for the comparison between the down-ramping rates of 1.5 cm min−1 and 2.0 cm min−1 during day (Q: −0.6; CI: −0.82 to −0.39); p < 0.01 for the comparison between the down-ramping rates of 1.5 cm min−1 and 2.0 cm min−1 during night (Q: −0.33; CI: −0.55 to −0.11) (see Figure 4C, 5).
When the fish length is considered instead of the larval stage and plotted against the stranding rate, a decrease in stranding incidences is evident for both bank slopes during day and night (Figure 6); and this is statistically significant (p < 0.05). The day experiments revealed a medium effect (|ρ|>0.30; Figures 6A,B), and the night experiments a strong effect (|ρ|>0.50; Figures 6C,D). In general, the effect of bank slope and daytime on the stranding rate diminishes with increasing fish length.
FIGURE 6. Stranding rate depending on mean fish length for 2% (A,C) and 5% (B,D) bank slope, both during day (A,B) and during night (C,D) with the number of replicates (n) per setup combination. The red dots represent trials with larval stage III-IV, the gray dots represent trials with larval stage V. Trends in stranding rate are indicated by the black polynomial lines with the gray shading marking the confidence interval (95% CI).
The decision tree model selected bank slope in the first level as main effect (Figure 7). For the 2% bank slope, the stranding rate is almost four times as high (median = 0.11) than at the 5% bank slope (median = 0.03). The second level decision rule is split by daytime for both bank slopes. Trials conducted at night (median = 0.23 for the 2% bank slope; median = 0.07 for the 5% bank slope) led to median stranding rates around three times higher as compared to day experiments (median = 0.07 for the 2% bank slope; median = 0.02 for the 5% bank slope). In the third stage, the decision tree split day trials into the larval stage at both bank slopes, with higher stranding rates at larval stage III-IV. At the 2% bank slope, the stranding rate of larval stage III-IV is about five times higher (median = 0.16) than those of experiments with larval stage V (median = 0.03). At the 5% bank slope, the stranding rate of larval stage III-IV (median = 0.04) is four times higher than at experiments with larval stage V (median = 0.01). Across all effect variables, the tree shows the lowest ranked rule for the down-ramping rates, with consistently higher stranding rates at higher down-ramping rates (Figure 7).
FIGURE 7. Decision tree model including bank slope, daytime, larval stage and down-ramping rate as effect variables to disentangle the target variable stranding rate of nase larvae. Values in the boxes represent the median of the stranding rate.
This study aimed to assess the single and combined effects of 1) varying down-ramping rates, ranging from 0.7 to 3.0 cm min−1 at 2) two different lateral bank slopes (2% and 5%), 3) each during day and night on stranding of 4) different larval stages of common nase. This paper, therefore, establishes a solid baseline for ecologically-based management of peak-operating hydropower plants in rivers inhabited by cyprinid fish (Moreira et al., 2019; Godinho et al., 2022).
Our results demonstrate that, similar to salmonid fish (Young et al., 2011; Auer et al., 2017; Hayes et al., 2019), bank dewatering due to artificial flow reduction causes larval nase to strand. Thereby, this study is the first to quantify stranding for nase as a cyprinid indicator species. In detail, the data showed that bank slope is a major determinant for the stranding risk of nase, with more stranding being documented on lower sloped banks. Also, time of day and larval developmental stages were decisive parameters. Generally, more fish became stranded during the night than during the day, particularly during earlier life cycle stages. The down-ramping rate was of subordinate importance to the parameters mentioned above, but showed interaction effects with the other parameters.
The tested bank slopes are common in shoreline habitats inhabited by early fish life stages (e.g., Bauersfeld, 1978; Pander et al., 2017). The data showed four times higher stranding rates at 2% sloped banks compared to 5% sloped banks. Indeed, this result is in line with the findings of several other studies, showing higher fish stranding on gently sloped banks than on steeper sloped banks (Beck and associates, 1989; Monk, 1989; Hunter, 1992; Bradford et al., 1995). Even though steeper banks may cause less stranding, we emphasize that steep shorelines (>10%) do not necessarily constitute suitable larval and juvenile habitats of cyprinid fishes (see also Pander et al., 2017). Instead, post-emergence larvae and early juveniles rely on shallow habitats with low flow velocities (Moore and Gregory, 1988; Baras and Nindaba, 1999), which are at risk of becoming dewatered over vast areas in hydropeaked rivers. Therefore, to ensure self-sustaining fish populations in such rivers, fish stranding in these key habitats must be prevented.
Our results revealed that time of day significantly affected fish stranding across all scenarios and are in line with several studies, underlining the effect of photophase on fish stranding (Heggenes et al., 1993; Bradford et al., 1995; Bradford, 1997; Saltveit et al., 2001; Puffer et al., 2015; Auer et al., 2017). Nighttime trials lead to almost three times higher stranding than daytime experiments. According to the literature, we expected higher stranding rates during night experiments. Unsurprisingly, the highest stranding rate of over 60% was observed at night. During the day, however, the highest observed stranding was half as high as at the night. In this regard, our study underlines that dewatering of larval habitats during the night will significantly increase stranding. This pattern can be explained by behavioral observations conducted during the experiments. We observed that nase larvae tended to remain in shallow habitats near the shoreline at night. However, during the daytime, fish used deeper parts of the mesocosms more quickly. Therefore, during nighttime down-ramping, larvae must conduct longer lateral shifts to avoid stranding compared to daytime down-ramping. This is likely the cause of increased night stranding, especially in combination with reduced visual cues and high down-ramping rates. In any case, nocturnal stranding risk seems more pronounced for early larval stages.
Anyhow, diurnal patterns may vary depending on the season, seasonal temperature, and species, and contrasting directions of effects have been reported in the literature (see also Bradford, 1997). For example, Bradford (1997) reported lower stranding rates during the night for chinook fry (Oncorhynchus tshawytscha) on a gravel bar (6°C). Similarly, Halleraker et al. (2003) found lower stranding rates at night than during day for 0+ and 1+ brown trout (Salmo trutta) at warmer water temperatures in summer (>10°C). Both studies linked the lower nighttime stranding to increased fish activity during night. However, for 1+ Atlantic salmon (Salmo salar) and 0+ brown trout in autumn (>9°C) almost no difference in stranding between day and night could be detected (Saltveit et al., 2001). Also, winter conditions (<4.5°C) did not reveal diurnal differences for brown trout. This was in contrast to 1+ Atlantic salmon with distinctively lower stranding at night compared to the day (∆ of about 30%) during winter conditions (<4.5°C). Also here, the authors linked this pattern to the prevalence of nocturnal behavior (Saltveit et al., 2001).
Contrasting diurnal effects have also been found by Auer et al. (2014, 2017), who conducted flume experiments during spring and summer. In these cases, the authors reported higher stranding during the night for larvae (4°C–10°C) and juveniles (11°C–15°C) of grayling (Thymallus thymallus) and brown trout. Similarly, Bradford (1997) found higher stranding for juveniles of Coho salmon (Oncorhynchus kisutch) during night in a trapping experiment (10°C), and this diurnal pattern was consistent for three tested down-ramping rates (0.1–1.0 cm min−1). Overall, these contrasting results from the literature indicate that environmental factors such as water temperature can influence behavior and thus the frequency of stranding at different times of the day. Therefore, we propose to conduct further experiments addressing seasonal patterns and varying water temperatures (see below).
Previous studies pointed out that early life stages are particularly sensitive to flow fluctuations (Hunter, 1992; Harby and Noack, 2013; Hayes et al., 2019). This conclusion is reasonable, as the critical swimming speed positively correlats to developmental stage and body size (Flore and Keckeis 1998; Flore et al., 2001; Kopf et al., 2014). Accordingly, we assumed, that earlier nase larval stages with incompletely developed fins are more sensitive to stranding than later stages. The experiments confirmed this assumption, as we documented a clear difference in stranding between larval stages III-IV and V. In detail, the smaller larval stage (range of mean length: 12.9–14.3 mm) showed about four times higher stranding rates than the larger one (range of mean length: 14.8–16.5 mm). This is also evident as stranding rates decrease with increasing fish length, which is consistent with other studies (Stoll and Beeck, 2011; Harby et al., 2015). We suggest conducting further experiments on earlier stages (e.g., I-II). In addition, under different conditions than tested in this study, stranding may be higher at stage V and beyond. Lower bank slope, coarser substrate, structured riverbanks, higher downramping rates, and multiple peaking events (see below) may cause increased stranding of larger nase, and should be subject to future studies.
Nevertheless, as water temperature affects tissue differentiation and growth (Seikai et al., 1986; Pepin, 1991), fish growth and life stage development may not always be synchronous. For example, Peňáz (1974) reported a fish length of 15.6–18.0 mm for the fourth larval stage, while Lechner et al. (2018) noted a mean fish length of 12.7 mm ± 1.8 SD in 2011 and 14.3 mm ± 0.9 SD in 2012 for the same larval stage. This has significant implications for defining mitigation measures for early life stages and can be applied, for example, to define the so-called “emergence window”, a mitigation concept suggested by Hayes et al. (2019). The authors propose two approaches to determine this ecologically-sensitive period where stricter flow thresholds should be enforced. The first calculates this period based on the water temperature. The second is based on in-situ observations to determine river (reach)-specific emergence periods and stranding patterns. Based on the present results, we suggest complementing field observations by measuring the larval length and determining larval stage to minimize the risk of incorrect timing and duration of the emergence window mitigation approach. In addition, restrictions regarding, e.g., down-ramping to prevent stranding, could be based on the actual larval stages occurring (more on down-ramping, see below).
Fish stranding is a major issue for individual fish and their populations (Hamilton & Buell, 1976; Cushman, 1985; Hauer et al., 2014; Auer et al., 2017) and has been observed for several species and life stages to decrease with reduced down-ramping rates (Bauersfeld, 1978; Hunter, 1992; Bradford et al., 1995; Zeiringer et al., 2014; Schmutz et al., 2015; Greimel et al., 2018). Our experiments emphasize the relationship between stranding risk and down-ramping rate for the tested larval stages during both, day and night, however, only at the lower sloped bank. Whereas at the 2% bank slope and larval stage III-IV, the median stranding rate was three times higher at the highest down-ramping rate (1.5 cm min−1) compared to the lowest down-ramping rate (0.7 cm min−1). In contrast, almost no difference in stranding could be observed between the down-ramping rates at the 5% bank slope, neither during the day nor at night. These results are surprising, since the tested down-ramping rates are considered high regarding the fish size and larval stages. For example, at a down-ramping rate of 3.0 cm min−1, we observed during day and night stranding rates of larval stage V (TL < 20 mm) comparable to stranding experiments with juvenile grayling (TL ≈ 50 mm), testing the same down-ramping rate and lateral bank slope (Auer et al., 2014). Similar stranding rates were also observed for juvenile brown trout (TL almost 70 mm) (Auer et al., 2014). This indicates that nase, in contrast to salmonids, can avoid stranding at high down-ramping rates at a comparatively early stage. This is also consistent with our observations during down-ramping. Larval nase were able to avoid stranding during the day. Interestingly, they shifted back and forth laterally close to the shoreline with the receding flow. This is remarkable, since we assumed that such behavior would significantly increase the vulnerability for stranding. The experiments further supported the diminishing effect of down-ramping rates at the steeper bank (5% bank slope), as larvae exhibited stranding rates at a similar level regardless of the tested down-ramping rates.
In conclusion, our experiments shed light on distinct interaction effects between daytime and the other parameters, whereby the effects were generally amplified at night (see Figure 4). For example, the differences between the lower and steeper sloped banks were strongest during night experiments. Clear differences in stranding at different down-ramping rates were only evident at the lower sloped bank, especially for experiments at night and with larval stage III-IV. Although the down-ramping was a parameter subordinated to bank slope, time of day, and larval stage regarding fish stranding (see Figure 7), it will nonetheless be crucial for mitigation frameworks to restrict ramping rates at times of the day or year (Hayes et al., 2019), such as after emergence and during the night. Overall, the results of the present study emphasize the need to understand combined effects to improve management actions.
Experiments provide the opportunity to quantify the effect of individual factors under controlled conditions and can, therefore, directly elucidate causal mechanisms (Melcher et al., 2017). However, even in semi-natural mesocosms, using small-sized fish (here: TL < 20 mm) may pose difficulties retrieving all stocked fish and determining stranded or non-stranded fish. However, this was not the case in our setups, as we achieved a mean post-trial fish recapture rate of 99.1% ± 1 SD.
The mesocosms mimicked typical nase larvae habitats, enabling transferability of the results to real-world conditions (Tran et al., 2015; Gelwick and McIntyre, 2017). For example, the flow velocities in the shoreline habitats stocked during high flow (Figure 2) were comparable to those reported in the field (e.g., Hofer and Kirchhofer, 1996; Keckeis et al., 1997; Flore and Keckeis, 1998; Flore et al., 2001; Keckeis and Schiemer, 2002). Also, sediments in the mesocosms, dominated by sand and fine gravel, were loose and not fixed (e.g., by the use of glue, grout wash, or foil), allowing not only lateral dewatering but also vertical water drainage during down-ramping, as it can be observed in natural conditions. Nevertheless, sediments consisted of homogeneous grain sizes to ensure standardized experimental conditions and were smoothed before each experimental run. In the field, however, sediment composition and grain size could vary in larval habitats depending, e.g., on river region and water level. Hence, stranding rates are likely to be even higher in hydropeaked rivers than quantified in the experimental channels, as fish may also conceal themselves in the interstitial of a river’s substrate (e.g., Bradford et al., 1995). Moreover, a coarser substrate generally leads to increased trapping during bank dewatering (Hunter, 1992; Young et al., 2011; Hauer et al., 2014). Similar effects with exacerbated stranding rates compared to flat gravel bars as tested in our experiments have been reported for morphologically diverse bank structures, such as potholes or ditches, which are known to trap juvenile fish (Bradford, 1997; Auer et al., 2017).
It is worth noting that the number of repetitions varies and is rather low for some scenarios. These uncertainties should be considered when interpreting the results. Further, we quantified larvae according to the location where they were found after down-ramping: 1) stranded on the dewatered substrate, 2) stranded, but found close to the downstream net, 3) stuck to the nets, and 4) swimming in the low flow channel. We excluded groups 2–3 from the calculation of stranded individuals (see Eq. 2). This is based firstly on the observation that many fish found in the nets were displaced before the onset of down-ramping and were therefore unavailable for possible stranding. Secondly, we observed individual larvae dropping from the downstream net onto the dewatered substrate. Therefore, we classified larvae found on the substrate close to the nets (≤1 cm) as non-stranded. However, we also observed real stranding in this area. Hence, this conservative approach of not counting fish close to the net as stranded may have led to an underestimation of true stranding rates. Further, we assumed that all larvae from the nets (group 2–3) and the low flow channel (group 4) were retrieved after the completion of each experiment. Therefore, missing larvae (e.g., those concealed in the substrate) were considered stranded. However, this potential uncertainty in the stranding calculation seems negligible, as it may lead to an overestimation of only 1% on average compared to the observed stranding.
This study determined stranding rates based on single down-ramping events. However, in hydropeaked rivers, artificial flow fluctuations often occur multiple times a day (Greimel et al., 2018). According to Bauersfeld (1978), who estimated a loss of 1.5% of salmon fry in each down-ramping and a total loss of 59% of all salmon fry within a season, even a small percentage of stranded fish in a single event can accumulate to a substantial loss affecting the entire fish population (Young et al., 2011). Therefore, we believe that studies on consecutive hydropeaks are highly relevant to further investigate hydropeaking effects on the population level.
Hydropeaking causes changes in the hydrological regime, and the ecological effects are associated with the river’s morphological features (Hauer et al., 2014; Schmutz et al., 2015). Different direct and indirect measures have been proposed to mitigate the negative effects of hydropeaking on river ecosystems (e.g., Greimel et al., 2018; Hayes et al., 2022). These measures include changes in hydropower plant’s operation mode, the construction of peak retention basins, or reducing hydropeaking effects by adapting river morphology, to name the most prominent ones.
Our results may feed into defining specific mitigation criteria for cyprinid species. Specifically, bank slope was identified as a major factor determining the stranding rate of larval nase, underlining the notion of focusing on critical habitats to define overall restoration approaches (Moreira et al., 2020). This could be done on a river-specific basis by assessing the downstream development of hydropeaking waves (Greimel et al., 2022) and linking peak intensity parameters to habitat locations. The strong diurnal differences in stranding indicate that under certain conditions (e.g., after emergence and during the following period when larvae inhabit shallow nursery habitat at the shoreline), temporal restrictions during the night may be necessary to reduce stranding. Most existing management recommendations emphasize the reduction of down-ramping rates to prevent the stranding of salmonids (Moreira et al., 2019), which have been extensively studied in the last decades. Although hydropower management can easily manipulate flow rates (Nagrodski et al., 2012), operational restrictions (e.g., limitations on the maximum turbine flow or drawdown range) may cause high costs (Person et al., 2014). Therefore, in practice, such measures are considered on a river-specific cost-benefit basis to achieve the most substantial improvements by combining various mitigation measures, including retention basins and power plant outflow diversions (Greimel et al., 2018). Implementing flow thresholds, particularly during sensitive life cycle phases (Hayes et al., 2019) is a more cost-effective approach than year-round limitations, and may constitute a critical step in rehabilitating and conserving fish populations in hydropeaked rivers. For non-salmonid fish families, e.g., cyprinids including the nase, information on mitigation targets or flow thresholds is yet non-existent (Moreira et al., 2019), but urgently needed as cyprinids inhabit many hydropeaked rivers.
When establishing flow thresholds, also water temperature should be considered as particularly thermopeaking (i.e., sudden alterations of water temperatures in the downstream stretch related to hydropeaking) (Zolezzi et al., 2011) can affect fish stranding (Auer et al., submitted). Critical water temperatures can induce adverse physiological and behavioral responses, including reduced swimming ability, delayed/accelerated development and growth, and lower activity (Seikai et al., 1986; Pepin, 1991; Muir et al., 1994; Bradford, 1997; Robinson and Childs, 2001; Smith and Hubert, 2003), potentially encouraging increased fish stranding. Therefore, stranding experiments addressing critical water temperatures are needed to better understand the ecological implications of this phenomenon.
Our data showed that the larval stage is a key component in influencing stranding rates, with younger fish being more susceptible to flow down-ramping than older ones. We assume that larvae are even more sensitive directly after emerging (larval stage I-II) from the interstitial due to the small size and limited mobility. Therefore, we recommend that the reduction of down-ramping rates should focus on early life stages up to larval stage IV. In connection with the effects of varying down-ramping rates, a similar approach as the proposed “emergence window” concept (Hayes et al., 2019), targeting a restricted period to mitigate stranding of sensitive life stages, may be useful to reduce hydropeaking-induced effects on cyprinids, too. For nase, this time frame could likely be shorter compared to salmonids due to the fast growth in spring. In any case, strict ramping thresholds should be enforced within the first weeks after gravel emergence, especially at night. However, as spawning is mainly triggered by water temperature that varies over the years, case-specific monitoring or longer emergence windows are required to make mitigation measures effective.
Finally, ecological thresholds, such as limiting down-ramping rates during sensitive life stages, should be implemented in hydropeaking mitigation programs (Greimel et al., 2021). The efficiency of experimentally developed thresholds for hydropeaking mitigation should be monitored and analyzed to learn more about their effectiveness in re-establishing fish populations in hydropeaked rivers.
The data supporting the findings of this study are available from the corresponding author upon reasonable request. Requests to access the datasets should be directed to simon.fuehrer@boku.ac.at.
Ethical review and approval was not required for the animal study because no invasive procedures were used and the fish larvae were stocked under conditions that frequently occur in many water bodies (hydropeaking). All fish handling was done in strict accordance with international and national ethical guidelines to ensure animal welfare.
Conceptualization: SF, DH, SS, and SA; Methodology: SF, DH, TH, DG, EF, DM, SS, and SA; Formal analysis: SF; Experimental investigation: SF, DH, TH, DG, EF, DM, and SA; Data curation: SF, DH, TH, DG, EF, DM, and SA; Writing—original draft: SF; Writing—review and editing: DH, SS, and SA; Visualization: SF; Funding acquisition: SF, DH, SS, and SA.
This research was funded in whole by the Austrian Science Fund (FWF) (P 34061-B). For the purpose of open access, the author has applied a CC BY public copyright licence to any Author Accepted Manuscript version arising from this submission. DM was supported by a Ph.D. scholarship from the FLUVIO—River Restoration and Management program funded by Fundação para a Ciência e a Tecnologia I.P. (FCT) (PD/BD/142885/2018).
The authors wish to thank Erwin Lautsch for his valuable comments regarding data analysis. We also want to thank Lisa Schülting, Patrick Leitner, and Bernhard Zeiringer for expert discussions and Georg Fürnweger for his support in larval rearing.
The authors declare that the research was conducted in the absence of any commercial or financial relationships that could be construed as a potential conflict of interest.
All claims expressed in this article are solely those of the authors and do not necessarily represent those of their affiliated organizations, or those of the publisher, the editors and the reviewers. Any product that may be evaluated in this article, or claim that may be made by its manufacturer, is not guaranteed or endorsed by the publisher.
The Supplementary Material for this article can be found online at: https://www.frontiersin.org/articles/10.3389/fenvs.2022.966418/full#supplementary-material
Alfredsen, K., Helland, I. P., Martins, E. G., and Power, M. (2022). Perspectives on the environmental implications of sustainable hydro-power: comparing countries, problems and approaches. Hydrobiologia 849 (2), 261–268. doi:10.1007/s10750-021-04700-z
Auer, S., Fohler, N., Zeiringer, B., Führer, S., and Schmutz, S. (2014). Experimentelle Untersuchungen zur Schwallproblematik—Drift und Stranden von Äschen und Bachforellen während der ersten Lebensstadien (p. 109) [Forschungsbericht]. Wien: Im Auftrag von: Bundesamt für Umwelt (BAFU), Abt. Wasser. CH-3003 Bern.
Auer, S., Hayes, D. S., Führer, S., Zeiringer, B., and Schmutz, S. (submitted). Effects of cold and warm thermopeaking on drift and stranding of juvenile European grayling (Thymallus thymallus). River Research and Applications.
Auer, S., Zeiringer, B., Führer, S., Tonolla, D., and Schmutz, S. (2017). Effects of river bank heterogeneity and time of day on drift and stranding of juvenile European grayling (Thymallus thymallus L.) caused by hydropeaking. Sci. Total Environ. 575, 1515–1521. doi:10.1016/j.scitotenv.2016.10.029
Baras, E., and Nindaba, J. (1999). Diel dynamics of habitat use by riverine young-of-the-year Barbus barbus and Chondrostoma nasus (Cyprinidae). Fundam. Appl. Limnol. 146 (4), 431–448. doi:10.1127/archiv-hydrobiol/146/1999/431
Bauersfeld, K. (1978). Stranding of juvenile salmon by flow reductions at mayfield dam on the cowlitz river, 1976. Tacoma, Washington: State of Washington, Department of Fisheries, 42. Technical Report No. 36.
Beck and associates, (1989). Skagit river salmon and steelhead fry stranding studies. Seattle, Washington: R.W. Beck and AssociatesSeattle City Light Environmental Affairs Division, 237. [Study Report].
Bejarano, M. D., Jansson, R., and Nilsson, C. (2018). The effects of hydropeaking on riverine plants: a review. Biol. Rev. 93 (1), 658–673. doi:10.1111/brv.12362
Bell, E., Kramer, S., Zajanc, D., and Aspittle, J. (2008). Salmonid fry stranding mortality associated with daily water level fluctuations in trail bridge reservoir, Oregon. North Am. J. Fish. Manag. 28 (5), 1515–1528. doi:10.1577/M07-026.1
Bewick, V., Cheek, L., and Ball, J. (2004). Statistics review 11: assessing risk. Crit. Care 8 (4), 287–291. doi:10.1186/cc2908
Bradford, M. J. (1997). An experimental study of stranding of juvenile salmonids on gravel bars and in sidechannels during rapid flow decreases. Regul. Rivers Res. Manag., 13. 3952–4401. doi:10.1002/(SICI)1099-1646(199709/10)13:5<395::AID-RRR464>3.0
Bradford, M. J., Taylor, G. C., Allan, J. A., and Higgins, P. S. (1995). An experimental study of the stranding of juvenile Coho salmon and rainbow trout during rapid flow decreases under winter conditions, North Am. J. Fish. Manag., 15, 4732–5479. doi:10.1577/1548-8675(1995)015<0473:AESOTS>2.32
Carolli, M., Vanzo, D., Siviglia, A., Zolezzi, G., Bruno, M. C., Alfredsen, K., et al. (2015). A simple procedure for the assessment of hydropeaking flow alterations applied to several european streams. Aquat. Sci. 77 (4), 639–653. doi:10.1007/s00027-015-0408-5
Casas-Mulet, R., Saltveit, S. J., and Alfredsen, K. (2015). The survival of atlantic salmon (Salmo salar) eggs during dewatering in a river subjected to hydropeaking. River Res. Appl. 31 (4), 433–446. doi:10.1002/rra.2827
Cochran, W. G. (1954). Some methods for strengthening the common χ2 tests. Biometrics 10 (4), 417. doi:10.2307/3001616
Cohen, J. (1988). Statistical power analysis for the behavioral Sciences. 2nd ed. Routledge. doi:10.4324/9780203771587
Copp, G. H., Oliver, J. M., Peňáz, M., and Roux, A. L. (1991). Juvenile fishes as functional describers of fluvial ecosystem dynamics: applications on the river rhǒne, France. Regul. Rivers Res. Mgmt. 6 (2), 135–145. doi:10.1002/rrr.3450060209
Costa, M. j., Boavida, I., Almeida, V., Cooke, S. j., and Pinheiro, A. n. (2018). Do artificial velocity refuges mitigate the physiological and behavioural consequences of hydropeaking on a freshwater Iberian cyprinid? Ecohydrology 11 (7), e1983. doi:10.1002/eco.1983
Cushman, R. M. (1985). Review of ecological effects of rapidly varying flows downstream from hydroelectric facilities. North Am. J. Fish. Manag., 5, 3302–3339. doi:10.1577/1548-8659(1985)5<330
Dudgeon, D., Arthington, A. H., Gessner, M. O., Kawabata, Z.-I., Knowler, D. J., Lévêque, C., et al. (2006). Freshwater biodiversity: Importance, threats, status and conservation challenges. Biol. Rev. 81 (2), 163. doi:10.1017/S1464793105006950
Dynesius, M., and Nilsson, C. (1994). Fragmentation and flow regulation of river systems in the northern third of the world. Science 266 (5186), 753–762. doi:10.1126/science.266.5186.753
Flore, L., Keckeis, H., and Schiemer, F. (2001). Feeding, energetic benefit and swimming capabilities of 0+ nase (Chondrostoma nasus L.) in flowing water: an integrative laboratory approach. rs. 135, 409–424. doi:10.1127/lr/12/2001/409
Flore, L., and Keckeis, H. (1998). The effect of water current on foraging behaviour of the rheophilic cyprinid Chondrostoma nasus (L.) during ontogeny: Evidence of a trade-off between energetic gain and swimming costs, Regul. Rivers Res. Manag., 14, 1412–1544. doi:10.1002/(SICI)1099-1646(199801/02)14:1<141::AID-RRR492>3.0
Freeman, M. C., Bowen, Z. H., Bovee, K. D., and Irwin, E. R. (2001). Flow and habitat effects on juvenile fish abundance in natural and altered flow regimes. Ecol. Appl. 11 (1), 179–190. doi:10.1890/1051-0761(2001)011[0179:FAHEOJ]2.0.CO;2
Gelwick, F. P., and McIntyre, P. B. (2017). “Chapter 22—trophic relations of stream fishes,” in Methods in stream ecology. Editors F. R. Hauer, and G. A. Lamberti Third Edition (San Diego, California: Academic Press), Vol. 1, 457–479. doi:10.1016/B978-0-12-416558-8.00022-6
Godinho, F. N., Alexandre, C., Almeida, P. R., Martínez-Capel, F., Cortes, R. M. V., Quintella, B. R., et al. (2022). Hydropeaking impact assessment for iberian cyprinids and leuciscids: an adaptation of the hydropeaking tool method. River Res. Appl., 1–9. doi:10.1002/rra.3943
Grabowski, T. B., and Isely, J. J. (2007). Effects of flow fluctuations on the spawning habitat of a riverine fish. Southeast. Nat. 6 (3), 471–478. doi:10.1656/1528-7092(2007)6[471:eoffot]2.0.co;2
Greimel, F., Grün, B., Hayes, D. S., Höller, N., Haider, J., Zeiringer, B., et al. (2022). PeakTrace: Routing of hydropeaking waves using multiple hydrographs—a novel approach. River Res. Appl.,
Greimel, F., Neubarth, J., Fuhrmann, M., Zoltan, L., Zeiringer, B., Schülting, L., et al. (2021). SuREmMa+: Entwicklung einer Methode zur ökologischen und energiewirtschaftlichen Bewertung von Maßnahmen zur Minderung von negativen schwall- und sunkbedingten ökologischen Auswirkungen, 323. [Forschungsbericht] Available at: https://oesterreichsenergie.at/fileadmin/user_upload/Oesterreichs_Energie/Publikationsdatenbank/Diverses/2021/SuREmMa__kl.pdf.
Greimel, F., Schülting, L., Graf, W., Bondar-Kunze, E., Auer, S., Zeiringer, B., et al. (2018). “Hydropeaking impacts and mitigation,” in Riverine ecosystem management: Science for governing towards a sustainable future. Editors S. Schmutz, and J. Sendzimir (Springer International Publishing), 91–110. doi:10.1007/978-3-319-73250-3_5
Greimel, F., Zeiringer, B., Höller, N., Grün, B., Godina, R., Schmutz, S., et al. (2016). A method to detect and characterize sub-daily flow fluctuations. Hydrol. Process. 30 (13), 2063–2078. doi:10.1002/hyp.10773
Halleraker, J. H., Saltveit, S. J., Harby, A., Arnekleiv, J. V., Fjeldstad, H.-P., Kohler, B., et al. (2003). Factors influencing stranding of wild juvenile Brown trout (Salmo trutta) during rapid and frequent flow decreases in an artificial stream. River Res. Appl. 19 (5–6), 589–603. doi:10.1002/rra.752
Hamilton, R., and Buell, J. W. (1976). Effects of modified hydrology on Campbell River salmonids. Vancouver, British Columbia: Department of the Environment Fisheries and Marine Service Habitat Protection Directorate Vancouver. Technical Report PAC/T-76-20; p. 184).
Harby, A., and Noack, M. (2013). “Rapid flow fluctuations and impacts on fish and the aquatic ecosystem,” in Ecohydraulics (Chichester, West Sussex: John Wiley & Sons), 323–335. doi:10.1002/9781118526576.ch19
Harby, A., Sauterleute, J., Killingtveit, Å., and Solvang, E. (2015). Hydropower for energy storage and balancing renewables. Dehradun: International Conference on Hydropower for Sustainable Development.
Hauer, C., Holzapfel, P., Tonolla, D., Habersack, H., and Zolezzi, G. (2019). In situ measurements of fine sediment infiltration (FSI) in gravel-bed rivers with a hydropeaking flow regime. Earth Surf. Process. Landf. 44 (2), 433–448. doi:10.1002/esp.4505
Hauer, C., Holzapfel, P., Leitner, P., and Graf, W. (2017). Longitudinal assessment of hydropeaking impacts on various scales for an improved process understanding and the design of mitigation measures. Sci. Total Environ. 575, 1503–1514. doi:10.1016/j.scitotenv.2016.10.031
Hauer, C., Unfer, G., Holzapfel, P., Haimann, M., and Habersack, H. (2014). Impact of channel bar form and grain size variability on estimated stranding risk of juvenile Brown trout during hydropeaking. Earth Surf. Process. Landf. 39 (12), 1622–1641. doi:10.1002/esp.3552
Hayes, D. S., Brändle, J. M., Seliger, C., Zeiringer, B., Ferreira, T., Schmutz, S., et al. (2018). Advancing towards functional environmental flows for temperate floodplain rivers. Sci. Total Environ. 633, 1089–1104. doi:10.1016/j.scitotenv.2018.03.221
Hayes, D. S., Lautsch, E., Unfer, G., Greimel, F., Zeiringer, B., Höller, N., et al. (2021). Response of European grayling, Thymallus thymallus, to multiple stressors in hydropeaking rivers. J. Environ. Manag. 292, 112737. doi:10.1016/j.jenvman.2021.112737
Hayes, D. S., Moreira, M., Boavida, I., Haslauer, M., Unfer, G., Zeiringer, B., et al. (2019). Life stage-specific hydropeaking flow rules. Sustainability 11 (6), 1547. doi:10.3390/su11061547
Hayes, D. S., Schülting, L., Carolli, M., Greimel, F., Batalla, R. J., and Casas-Mulet, R. (2022). “Hydropeaking: processes, effects, and mitigation,” in Reference module in Earth systems and environmental Sciences (Elsevier). doi:10.1016/B978-0-12-819166-8.00171-7
Heggenes, J., Krog, O. M., Lindås, O. R., Dokk, J. G., and Bremnes, T. (1993). Homeostatic behavioural responses in a changing environment: Brown trout (Salmo trutta) become nocturnal during winter. J. Anim. Ecol. 62, 295. doi:10.2307/5361
Heggenes, J., and Traaen, T. (1988). Downstream migration and critical water velocities in stream channels for fry of four salmonid species. J. Fish. Biol. 32 (5), 717–727. doi:10.1111/j.1095-8649.1988.tb05412.x
Hofer, K., and Kirchhofer, A. (1996). Drift, habitat choice and growth of the nase (Chondrostoma nasus, Cyprinidae) during early life stages", in Conservation of endangered freshwater fish in Europe Editors A. Kirchhofer, and D. Hefti Birkhäuser Basel, 269–278. doi:10.1007/978-3-0348-9014-4_26
Hunter, M. A. (1992). Hydropower flow fluctuations and salmonids: a review of the biological effects, mechanical causes, and options for mitigation. Department of Fisheries, State of Washington. [Technical Report No. 119.
IHA (2022). International hydropower association. Annual Report 2022 [Report] Available at: https://assets-global.website-files.com/5f749e4b9399c80b5e421384/6231efcd04624321c3031033_IHA-Annual-report-2022a.pdf.
Kainz, E., and Gollmann, H. P. (1999). Ein Beitrag zur Biologie der Nase (Chondrostoma nasus L.): Aufzucht und Vorkommen in Österreich. Fisch. Jahrg. 52/1999, 265
Keckeis, H., and Schiemer, F. (2002). “Understanding conservation issues of the danube river,” in Fishery science. Editors Fuiman, and Werner (John Wiley & Sons), 272–288.
Keckeis, H., Winkler, G., Flore, L., Reckendorfer, W., and Schiemer, F. (1997). Spatial and seasonal characteristics of O+ fish nursery habitats of nase, Chondrostoma nasus in the River Danube, Austria. Folia Zool. -Praha 146, 143–150.
Kopf, S. M., Humphries, P., and Watts, R. J. (2014). Ontogeny of critical and prolonged swimming performance for the larvae of six Australian freshwater fish species. J. Fish Biol. 84 (6), 1820–1841. doi:10.1111/jfb.12399
Larrieu, K. G., Pasternack, G. B., and Schwindt, S. (2021). Automated analysis of lateral river connectivity and fish stranding risks—Part 1: review, theory and algorithm. Ecohydrology 14 (2), e2268. doi:10.1002/eco.2268
Lechner, A., Keckeis, H., Glas, M., Tritthart, M., Habersack, H., Andorfer, L., et al. (2018). The influence of discharge, current speed, and development on the downstream dispersal of larval nase (Chondrostoma nasus) in the River Danube. Can. J. Fish. Aquat. Sci. 75 (2), 247–259. doi:10.1139/cjfas-2016-0340
Li, T., and Pasternack, G. B. (2021). Revealing the diversity of hydropeaking flow regimes. J. Hydrology 598, 126392. doi:10.1016/j.jhydrol.2021.126392
Mantel, N., and Haenszel, W. (1959). Statistical aspects of the analysis of data from retrospective studies of disease. J. Natl. Cancer Inst. 22 (4), 719–748. doi:10.1093/jnci/22.4.719
Melcher, A. H., Bakken, T. H., Friedrich, T., Greimel, F., Humer, N., Schmutz, S., et al. (2017). Drawing together multiple lines of evidence from assessment studies of hydropeaking pressures in impacted rivers. Freshw. Sci. 36 (1), 220–230. doi:10.1086/690295
Monk, C. L. (1989). Factors that influence Stranding of juvenile chinook Salmon and steelhead trout. [Master’s thesis]. Seattle: University of Washington.
Moore, K. M. S., and Gregory, S. V. (1988). Response of young-of-the-year cutthroat trout to manipulation of habitat structure in a small stream. Trans. Am. Fish. Soc. 117, 162–170. doi:10.1577/1548-8659(1988)117<0162:royoty>2.3.co;2
Moreira, M., Hayes, D. S., Boavida, I., Schletterer, M., Schmutz, S., Pinheiro, A., et al. (2019). Ecologically-based criteria for hydropeaking mitigation: a review. Sci. Total Environ. 657, 1508–1522. doi:10.1016/j.scitotenv.2018.12.107
Moreira, M., Schletterer, M., Quaresma, A., Boavida, I., and Pinheiro, A. (2020). New insights into hydropeaking mitigation assessment from a diversion hydropower plant: the GKI project (Tyrol, Austria). Ecol. Eng. 158, 106035. doi:10.1016/j.ecoleng.2020.106035
Morris, J. A., and Gardner, M. J. (1988). Statistics in Medicine: calculating confidence intervals for relative risks (odds ratios) and standardised ratios and rates. Br. Med. J. Clin. Res. Ed. 296 (6632), 1313–1316. doi:10.1136/bmj.296.6632.1313
Muir, W. D., Zaugg, W. S., Giorgi, A. E., and McCutcheon, S. (1994). Accelerating smolt development and downstream movement in yearling chinook salmon with advanced photoperiod and increased temperature. Aquaculture 123 (3), 387–399. doi:10.1016/0044-8486(94)90073-6
Nagrodski, A., Raby, G. D., Hasler, C. T., Taylor, M. K., and Cooke, S. J. (2012). Fish stranding in freshwater systems: Sources, consequences, and mitigation. J. Environ. Manag. 103, 133–141. doi:10.1016/j.jenvman.2012.03.007
Ovidio, M., and Philippart, J. C. (2008). Movement patterns and spawning activity of individual nase Chondrostoma nasus (L.) in flow-regulated and weir-fragmented rivers. J. Appl. Ichthyol. 24 (3), 256–262. doi:10.1111/j.1439-0426.2008.01050.x
Pander, J., Mueller, M., Knott, J., Egg, L., and Geist, J. (2017). Is it worth the money? the functionality of engineered shallow stream banks as habitat for juvenile fishes in heavily modified water bodies. River Res. applic. 33 (1), 63–72. doi:10.1002/rra.3065
Peňáz, M. (1996). “Chondrostoma nasus—its reproduction strategy and possible reasons for a widely observed population decline—a review,” in Conservation of endangered freshwater fish in Europe. ALS advances in life Sciences. Editors A. Kirchhofer, and D. Hefti (Birkhäuser Basel). doi:10.1007/978-3-0348-9014-4_27
Peňáz, M. (1974). Early development of the nase carp, Chondrostoma nasus (Linnaeus, 1758). Zool. Listy 23, 275
Pepin, P. (1991). Effect of temperature and size on development, mortality, and survival rates of the pelagic early life history stages of marine fish. Can. J. Fish. Aquat. Sci. 48 (3), 503–518. doi:10.1139/f91-065
Perry, S. A., and Perry, W. B. (1986). Effects of experimental flow regulation on invertebrate drift and stranding in the flathead and kootenai rivers, montana, USA. Hydrobiologia 134 (2), 171–182. doi:10.1007/BF00006739
Person, E., Bieri, M., Peter, A., and Schleiss, A. J. (2014). Mitigation measures for fish habitat improvement in alpine rivers affected by hydropower operations. Ecohydrology 7 (2), 580–599. doi:10.1002/eco.1380
Person, E. (2013). Impact of hydropeaking on fish and their habitat. PhD thesis, École polytechnique fédérale de Lausanne.
Puffer, M., Berg, O. K., Huusko, A., Vehanen, T., Forseth, T., Einum, S., et al. (2015). Seasonal effects of hydropeaking on growth, energetics and movement of juvenile atlantic Salmon (Salmo salar). River Res. Appl. 31 (9), 1101–1108. doi:10.1002/rra.2801
Reckendorfer, W., Keckeis, H., Tutu, V., Winkler, G., Zornig, H., Schiemer, F., et al. (2001). Diet shifts in 0+ nase, chondrostoma nasus: Size-specific differences and the effect of food availability. rs. 12 (2–4), 425–440. doi:10.1127/lr/12/2001/425
Ren21, (2021). Renewables 2021 global status report. [Report] Available at: https://www.ren21.net/wp-content/uploads/2019/05/GSR2021_Full_Report.pdf.
Robinson, A. T., and Childs, M. R. (2001). Juvenile growth of native fishes in the little colorado river and in a thermally modified portion of the colorado river. North Am. J. Fish. Manag. 21 (4), 809–815. doi:10.1577/1548-8675(2001)021<0809:JGONFI>2.0.CO;2
Sachs, L., and Hedderich, J. (2006). Angewandte Statistik: Methodensammlung mit R. 12th ed. Berlin/Heidelberg: Springer. Available at: https://link.springer.com/book/10.1007/978-3-540-32161-3.
Saltveit, S. j., Halleraker, J. h., Arnekleiv, J. v., and Harby, A. (2001). Field experiments on stranding in juvenile atlantic salmon (Salmo salar) and Brown trout (Salmo trutta) during rapid flow decreases caused by hydropeaking. Regul. Rivers Res. Mgmt. 17 (4–5), 609–622. doi:10.1002/rrr.652
Sandercock, P. (1989). The odds ratio: A useful tool in neurosciences. J. Neurology, Neurosurg. Psychiatry 52 (7), 817–820. doi:10.1136/jnnp.52.7.817
Schiemer, F., Keckeis, H., and Kamler, E. (2002). The early life history stages of riverine fish: ecophysiological and environmental bottlenecks. Comp. Biochem. Physiology Part A Mol. Integr. Physiology 133 (3), 439–449. doi:10.1016/S1095-6433(02)00246-5
Schiemer, F., and Spindler, T. (1989). Endangered fish species of the danube river in austria. Regul. Rivers Res. Mgmt. 4 (4), 397–407. doi:10.1002/rrr.3450040407
Schludermann, E., Keckeis, H., and Nemeschkal, H.-L. (2009). Effect of initial size on daily growth and survival in freshwater Chondrostoma nasus larvae: A field survey. J. Fish. Biol. 74 (4), 939–955. doi:10.1111/j.1095-8649.2009.02182.x
Schmidt, C. O., and Kohlmann, T. (2008). When to use the odds ratio or the relative risk? Int. J. Public Health 53 (3), 165–167. doi:10.1007/s00038-008-7068-3
Schmutz, S., Bakken, T. H., Friedrich, T., Greimel, F., Harby, A., Jungwirth, M., et al. (2015). Response of fish communities to hydrological and morphological alterations in hydropeaking rivers of Austria. River Res. Appl. 31 (8), 919–930. doi:10.1002/rra.2795
S. Schmutz, and J. Sendzimir (Editors) (2018). Riverine ecosystem management: Science for governing towards a sustainable future (Cham: Springer International Publishing). doi:10.1007/978-3-319-73250-3
Schneider, C. A., Rasband, W. S., and Eliceiri, K. W. (2012). NIH image to ImageJ: 25 years of image analysis. Nat. Methods 9 (7), 671–675. doi:10.1038/nmeth.2089
Schülting, L., Feld, C. K., Zeiringer, B., Huđek, H., and Graf, W. (2019). Macroinvertebrate drift response to hydropeaking: an experimental approach to assess the effect of varying ramping velocities: macroinvertebrate drift response to hydropeaking with varying ramping velocities. Ecohydrology 12 (1), e2032. doi:10.1002/eco.2032
Seikai, T., Tanangonan, J. B., and Tanaka, M. (1986). Temperature influence on larval growth and metamorphosis of the Japanese flounder Palalichthys olivaceus in the laboratory. Nippon. Suisan Gakkaishi 52 (6), 977–982. doi:10.2331/suisan.52.977
Shen, Y., and Diplas, P. (2010). Modeling unsteady flow characteristics of hydropeaking operations and their implications on fish habitat. J. Hydraul. Eng. 136, 1053–1066. doi:10.1061/(ASCE)HY.1943-7900.0000112
Smith, M. A., and Hubert, W. A. (2003). Simulated thermal tempering versus sudden temperature change and short-term survival of fingerling rainbow trout. North Am. J. Aquac. 65 (1), 67–69. doi:10.1577/1548-8454(2003)065<0067:STTVST>2.0.CO;2
Smokorowski, K. E. (2022). The ups and downs of hydropeaking: a canadian perspective on the need for, and ecological costs of, peaking hydropower production. Hydrobiologia 849 (2), 421–441. doi:10.1007/s10750-020-04480-y
Stoll, S., and Beeck, P. (2011). Post-release stranding rates of stocked allis shad (Alosa alosa) larvae exposed to surface wave action. J. Appl. Ichthyology 27 (3), 41–44. doi:10.1111/j.1439-0426.2011.01879.x
Therneau, T., and Atkinson, E. (2022). An introduction to Recursive Partitioning Using the RPART Routines, 60. Sine loco: Mayo Foundation. https://cran.r-project.org/web/packages/rpart/vignettes/longintro.pdf.
Tran, T. N. Q., Jackson, M. C., Sheath, D., Verreycken, H., and Britton, J. R. (2015). Patterns of trophic niche divergence between invasive and native fishes in wild communities are predictable from mesocosm studies. J. Anim. Ecol. 84 (4), 1071–1080. doi:10.1111/1365-2656.12360
Tuhtan, J., Noack, M., and Wieprecht, S. (2012). Estimating stranding risk due to hydropeaking for juvenile European grayling considering river morphology. KSCE J. Civ. Eng. 16, 197–206. doi:10.1007/s12205-012-0002-5
van Ruijven, B. J., De Cian, E., and Sue Wing, I. (2019). Amplification of future energy demand growth due to climate change. Nat. Commun. 10 (1), 2762. doi:10.1038/s41467-019-10399-3
Vanzo, D., Siviglia, A., Carolli, M., and Zolezzi, G. (2016a). Characterization of sub-daily thermal regime in alpine rivers: Quantification of alterations induced by hydropeaking. Hydrol. Process. 30 (7), 1052–1070. doi:10.1002/hyp.10682
Vanzo, D., Zolezzi, G., and Siviglia, A. (2016b). Eco-hydraulic modelling of the interactions between hydropeaking and river morphology: Eco-hydraulic Modelling of Hydropeaking. Ecohydrology 9 (3), 421–437. doi:10.1002/eco.1647
Volkoff, H., and Rønnestad, I. (2020). Effects of temperature on feeding and digestive processes in fish. Temp. (Austin, Tex.) 7 (4), 307–320. doi:10.1080/23328940.2020.1765950
Woolf, B. (1955). On estimating the relation between blood group and disease. Ann. Hum. Genet. 19 (4), 251–253. doi:10.1111/j.1469-1809.1955.tb01348.x
Young, P. S., Cech, J. J., and Thompson, L. C. (2011). Hydropower-related pulsed-flow impacts on stream fishes: a brief review, conceptual model, knowledge gaps, and research needs. Rev. Fish. Biol. Fish. 21 (4), 713–731. doi:10.1007/s11160-011-9211-0
Yule, G. U. (1912). On the methods of measuring association between two attributes. J. R. Stat. Soc. 75 (6), 579. doi:10.2307/2340126
Zarfl, C., Berlekamp, J., He, F., Jähnig, S. C., Darwall, W., Tockner, K., et al. (2019). Future large hydropower dams impact global freshwater megafauna. Sci. Rep. 9 (1), 18531. doi:10.1038/s41598-019-54980-8
Zeiringer, B., Fohler, N., Auer, S., Greimel, F., and Schmutz, S. (2014). “Experiments on drifting and stranding of juvenile grayling during fluctuating flow in nature-like channels with different morphological structures,” in 10th International Symposium on Ecohydraulics 2014 Norwegian university of science and technology (Norway: E-Proceedings. Trondheim)
Zens, B., Glas, M., Tritthart, M., Habersack, H., and Keckeis, H. (2017). Movement patterns and rheoreaction of larvae of a fluvial specialist (nase, Chondrostoma nasus, Linnaeus 1758): the role of active versus passive components of behaviour in dispersal. Can. J. Fish. Aquat. Sci. 75, 193–200. doi:10.1139/cjfas-2016-0276
Keywords: flow ramping, young-of-the-year, experimental flumes, storage hydropower, hydro-peaking, river restoration
Citation: Führer S, Hayes DS, Hasler T, Graf DRM, Fauchery E, Mameri D, Schmutz S and Auer S (2022) Stranding of larval nase (Chondrostoma nasus L.) depending on bank slope, down-ramping rate and daytime. Front. Environ. Sci. 10:966418. doi: 10.3389/fenvs.2022.966418
Received: 10 June 2022; Accepted: 13 July 2022;
Published: 23 August 2022.
Edited by:
Riccardo Fornaroli, University of Milano-Bicocca, ItalyReviewed by:
Luca Bonacina, University of Milano-Bicocca, ItalyCopyright © 2022 Führer, Hayes, Hasler, Graf, Fauchery, Mameri, Schmutz and Auer. This is an open-access article distributed under the terms of the Creative Commons Attribution License (CC BY). The use, distribution or reproduction in other forums is permitted, provided the original author(s) and the copyright owner(s) are credited and that the original publication in this journal is cited, in accordance with accepted academic practice. No use, distribution or reproduction is permitted which does not comply with these terms.
*Correspondence: Simon Führer, simon.fuehrer@boku.ac.at
Disclaimer: All claims expressed in this article are solely those of the authors and do not necessarily represent those of their affiliated organizations, or those of the publisher, the editors and the reviewers. Any product that may be evaluated in this article or claim that may be made by its manufacturer is not guaranteed or endorsed by the publisher.
Research integrity at Frontiers
Learn more about the work of our research integrity team to safeguard the quality of each article we publish.