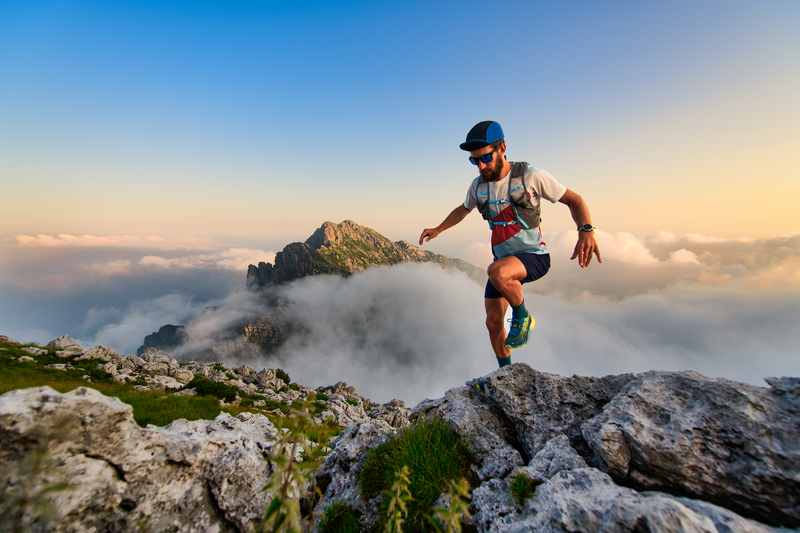
95% of researchers rate our articles as excellent or good
Learn more about the work of our research integrity team to safeguard the quality of each article we publish.
Find out more
MINI REVIEW article
Front. Environ. Health , 13 March 2025
Sec. Air Quality and Health
Volume 4 - 2025 | https://doi.org/10.3389/fenvh.2025.1466951
The 2015 policy to incorporate sex as a biological variable (SABV) enhanced biomedical research and allowed for better predictions to be made regarding clinical outcomes and environmental health risks. This review aims to make a case for the next SABV—stress as a biological variable. While the body is equipped to respond to acute stress, chronic stress can overwork physiologic systems, leading to allostatic load, or progressive wear and tear on the brain and body. Allostatic load has many implications on immune, cardiovascular, and metabolic function, and alters xenobiotic metabolism of environmental and pharmaceutical chemicals. However, historically disadvantaged communities and populations are at an increased risk of harm due to elevated exposure to psychosocial stressors and environmental pollutants. Therefore, the unique biological responses among populations that experience this double hit should be considered in toxicology risk assessments. Among current approaches, allostatic load measurements are optimal as a framework that captures health disparities and a tool that quantifies cumulative stress burdens that can be integrated into health data for better risk predictions.
In 2015, the National Institutes of Health (NIH) released the sex as a biological variable (SABV) policy to address the historical exclusion of female humans and animals from biomedical research. Many environmental health studies have demonstrated that sex is an important biological variable that influences xenobiotic exposure, metabolism, and health effects. In Rebuli et al., for example, we identified sex-specific inflammatory responses to woodsmoke exposure, that were not discernable when analyzing aggregate data (1). As a result of the SABV policy, which requires researchers to factor sex into both human and vertebrate animal study design, better predictions can be made regarding clinical outcomes and environmental health risks. However, stress, which affects all bodily systems and is another major biological variable that influences individual-level susceptibility to both clinical and environmental outcomes, is not considered in the design of clinical and pre-clinical studies. While the body is equipped to manage small or infrequent doses of stress, chronic stress can alter bodily processes, making individuals more vulnerable to other stressors, including environmental chemicals. Because of the broad impacts of stress on the body and the presence of persistent psychosocial stressors in minority and low-income communities, it is vital to understand how stress modifies responses to xenobiotic exposures, ranging from environmental exposures to pharmaceutical drugs, to improve comprehensive risk assessments. Additionally, as climate-related changes drive increased environmental exposures, it is crucial to integrate stress into environmental research to fully capture the complexities of environmental health risks and disparities. Therefore, this review discusses the importance of stress as a biological variable and the use of allostatic load to integrate chronic stress-related dysregulation into toxicology and risk assessments.
The neuroendocrine stress response is implicated in the physiological effects of chronic stress. Stressful stimuli activate the sympathetic-adrenal-medullary (SAM) to release catecholamines, primarily epinephrine and norepinephrine, from the adrenal medulla which interact with alpha- and beta-adrenergic receptors in the central nervous system and smooth muscle cells to exert a “fight or flight” response characterized by increased blood pressure, heart rate, cardiac output, oxygen consumption and lipolysis among other physiological responses (2). The hypothalamus-pituitary-adrenal (HPA) axis is also stimulated to produce corticotrophin-releasing hormone, which triggers adrenocorticotropic hormone (ACTH) synthesis from the pituitary gland. ACTH acts on adrenal glands to release glucocorticoids, primarily cortisol, into circulation. Glucocorticoids affect various systemic processes in response to stress, including altering lipid and glucose metabolism and immune responses according to the duration and intensity of exposure. While SAM and HPA activation is an adaptive response following acute stress, prolonged exposure to psychosocial and environmental stressors can chronically activate the HPA axis and stress responses, leading to excess glucocorticoid and catecholamine production and downstream adverse effects on immune, cardiovascular, metabolic, and nervous systems (3). Over time, chronic stressors impair the body's capacity for effective regulation and adaptation, increasing the risk for maladaptive responses, and allostatic load, the progressive physiological ‘wear and tear’ on the brain and body that pre-dispose individuals to disease through deterioration of physical and mental health. As sub-clinical changes to organ systems persist, allostatic overload occurs in which chronic stress results in morbidities and mortality (4, 5).
Traditionally, stress was defined as a threat to homeostasis, or the dynamic equilibrium maintained by physiological systems through immediate, short-term adjustments to restore balance to a fixed set point. However, allostasis, introduced by neuroscientist Peter Sterling and epidemiologist Joseph Eyer, expands on this concept by emphasizing the body's ability to achieve stability through adaptive changes in response to stress. Unlike homeostasis, which relies on adhering to a limited set point, allostasis describes the adaptive mechanisms that allow physiological systems to function within a dynamic operating range in response to stress (6, 7). However, both homeostasis and allostasis fail to account for the long-term effects of chronic stress, which causes long-term activation of allostatic processes. Over time, chronic or prolonged stress exert wear and tear on organs and tissues, where they are unable to recover from or adapt to ongoing stress. As a result, the body begins brace for prolonged exposure to stress by operating at new, altered set points (6, 8, 9). Allostatic load (AL) was defined by neuroscientist Bruce McEwen and physiological psychologist Eliot Stellar to encompass this stress-induced strain on the body that predisposes individuals to disease (Figure 1B) (6).
Figure 1. Graphical depictions of the interplay between (A) social determinants of health, (B) allostatic load, (C) environmental exposures and (D) stress induced susceptibility to environmental pollutants.
Allostatic load is both a framework and a tool to measure the effects of cumulative stress on the body. Teresa Seeman and colleagues were the first to develop an operational method for allostatic load which involved measuring biomarkers across various systems and integrating those markers into a composite score (10). These biomarkers include primary mediators (epinephrine, norepinephrine, cortisol, and DHEA-S), which have direct correlation with adrenal function, and secondary mediators, which represent the organ effects of stress on cardiovascular (systolic and diastolic blood pressure) and metabolic (waist-hip ratio, total cholesterol-HDL ratio, glycosylated hemoglobin, HDL cholesterol) systems. Higher allostatic load scores, measured in a cohort of older men and women, correlated with increased risk for poorer cognitive and physical function, cardiovascular disease, and mortality (10). These results were corroborated in a 5-year follow up study that also showed composite allostatic load scores were a better predictor of mortality and declines in physical functioning than metabolic markers or primary markers alone (11). Allostatic load continues to serve as an objective measure of cumulative stress in research and has been studied in the context of various physical and mental health outcomes including ageing, increased risk for cardiovascular diseases, diabetes, preeclampsia, musculoskeletal disorders, depression, epileptic seizures, cancer, anxiety disorders, and health risk behaviors (5, 12).
As illustrated in Figure 2, the physiological wear and tear from allostatic load can increase susceptibility to other physical and chemical stressors, including environmental pollutants, by impairing immune function, and increasing risks for metabolic and cardiovascular diseases (13). Allostatic load is characterized by the excess production or release of primary stress hormones, which regulate the immune system through mediating leukocyte distribution. Epinephrine and norepinephrine act together to mobilize immune cells into the bloodstream and epinephrine and cortisol influence the trafficking of immune cells to specific tissues or sites of injury (14). However, stress induced immune responses depend on the duration and severity of the stress response (3). Under acute stress, energy stores are mobilized, and the immune system ramps up as the body prepares for wound healing or defense against invading pathogens (15). This is characterized by the mobilization of leukocytes into the blood from various compartments, including spleen, bone marrow, lung, and lymph nodes, and the subsequent redistribution of leukocytes to tissues and organs where they recruit other immune mediators, such as cytokines. After the initial trafficking of immune cells, blood monocytes, lymphocytes, T helper cells, cytotoxic T cells, B and NK cells continue to decrease while blood neutrophil counts increase (14, 16). In contrast, chronic stress reduces the number of circulating lymphocytes and reduces immune cell responsivity, which may indicate glucocorticoid resistance (14). Additionally, chronic stress has been shown to suppress other immune mediators, including cell mediated immunity, antibody production, NK cell activity, and leukocyte proliferation, which is believed to be induced by chronic inflammation (17, 18). Compromised immune function can increase susceptibility to environmental exposures, which also have various harmful effects on the immune system, including generating oxidative stress that damage DNA or cellular proteins, activating pro-inflammatory pathways, or suppressing immune cell activity (19–21) (Figure 2).
Figure 2. Schematic illustrating biological mechanisms mediating potential interactions between chronic stress and environmental exposures.
Additionally, glucocorticoids have regulatory effects on cardiovascular and metabolic systems (22). For the cardiovascular system, glucocorticoids increase sensitivity to catecholamines, such as epinephrine and norepinephrine, which elevate heart rate and blood pressure, and directly affect the renin-angiotensin-aldosterone system (RAAS), which regulates blood volume, electrolyte balance, and endothelial function (22, 23). Excess glucocorticoids can also lead to metabolic syndrome, characterized by high blood pressure, obesity, low HDL cholesterol, elevated triglycerides, and hyperglycemia (24). Specifically, glucocorticoids drive changes in eating behaviors, promoting the consumption of less nutrient, but more palatable foods, increase visceral fat deposition in the liver and vascular tissue, and contribute to insulin resistance and elevated blood glucose levels (25, 26). Therefore, in a state of allostatic load, excess glucocorticoid production disrupts normal metabolic and cardiovascular function, driving the development of Type-2 diabetes, hypertension, obesity, atherosclerosis and cardiovascular-specific mortality (25, 27–29). Because chronic stress and environmental chemicals act through similar mechanisms, co-exposure amplifies their cumulative impact on cardiovascular and metabolic systems (3, 30, 31). This is demonstrated by studies linking transportation noise, a prevalent socioeconomic stressor, and air pollution to major adverse cardiovascular events (MACE) (32, 33). Chronic environmental stressors, such as transportation noise, stimulate stress-related neurobiological activity, including increased amygdala metabolic activity, which is central to stress perception (34–36). Elevated amygdala activity increases arterial inflammation, a critical driver of cardiovascular disease and metabolic conditions such as hypertension, type-2 diabetes and obesity (35, 37). Air pollution, such as PM 2.5, also exacerbates arterial inflammation and promotes leucopoietic tissue activity, contributing to MACE risk (38, 39). Air pollution also increases risks for metabolic diseases such as diabetes and stroke (39). When combined, exposure to both stressors and pollutants amplify these harmful pathways, significantly increasing susceptibility to cardiovascular and metabolic diseases (33). The combined impact of transportation noise and elevated PM 2.5 highlights how chronic stress amplifies susceptibility to environmental exposures, compounding their effects on already compromised systems and increasing the risk of harm.
In addition to increasing environmental susceptibility through modifying organ function, stress can also modify xenobiotic metabolism through altering cytochrome P-450 (CYP) enzymes, the primary enzymes involved in the metabolism of endogenous and exogenous chemicals (Figure 1C) (40). Glucocorticoids are known regulators of CYP enzymes, specifically belonging to the CYP1A family, which metabolizes polycyclic aromatic hydrocarbons, persistent organic pollutants present in air, water, and soil (41, 42). Cortisol suppresses the hypothalamic-pituitary-thyroid (HPT) axis through stimulating the release of somatostatin, which inhibits the secretion of thyrotropin releasing hormone (TRH) and thyroid stimulating hormone (TSH), modifying thyroid-mediated regulation of CYPs. Somatostatin also suppresses growth hormone secretion, which further down-regulates insulin-like growth factor-1 and, thus, alters insulin-mediated regulation of CYP enzymes (43). Catecholamines are also capable of modifying CYP enzymes through alpha- and beta-adrenergic receptor pathways (40). Animal studies have demonstrated that psychological stress suppressed CYP1A2 in rat liver and upregulated CYP2A5 in mice (42). Another study showed stress increased the inducibility of CYP1A1 and CYP1A2 by Benzo[a]pyrene, a polycyclic aromatic hydrocarbon prevalent in urban and industrial areas (44–46). The consequences of altered CYP function depend on the location of the enzyme and the activity of the parent compound; however, a major risk is the potential of induced enzymes to accelerate the formation of carcinogenic or highly reactive species, increasing the toxicity of the parent compound (47) (Figure 2). Overall, more research is needed to elucidate the role of stress in modifying xenobiotic metabolism and assess differences between the effects of acute and chronic stress.
Factors that alter the expression and function of metabolizing enzymes can also affect the pharmacodynamics of various drugs. Glucocorticoids can affect drug absorption by influencing gastrointestinal function and blood flow. Stress decreases blood flow to visceral organs while increasing blood flow to working muscles, which may decrease drug absorption by the GI track for orally administered drugs and increase absorption for drugs administered intramuscularly (43). Additionally, because distribution occurs most rapidly into tissues with increased blood flow and least rapidly in tissue with decreased blood flow, changes in blood flow can alter the distribution of drugs from circulation into target tissues (48). Further, because cortisol mobilizes free fatty acids, which have a strong binding affinity for the same binding sites as drugs on human serum albumin, it may displace drugs from albumin binding sites, increasing the amount of unbound drug in circulation, which can result in subtherapeutic or toxic plasma concentrations of drugs (49–51). This effect has been reported for various drug types including anesthetics, anticoagulants, and antibiotics (48). Pharmacologic implications of stress may influence clinical trial success and drug efficacy and safety, especially among racial and ethnic groups that have higher circulating levels of glucocorticoids and higher baseline allostatic load.
Many studies have reported racial, ethnic, and socioeconomic disparities in allostatic load, which are driven by “weathering,” the hypothesis that minority populations experience early health deterioration because of socioeconomic disadvantage (27, 52–56). This accelerated health decline is often overlooked in research studies, which can misinform health and disease predictions. For example, one study observed that cohort selection bias in the Study of Women's Health Across the Nation (SWAN) resulted in poor age of onset predictions for cardio-metabolic diseases, which were significantly lower for Black and Hispanic women compared to White women (57). Weathering is an outcome of experiencing adverse social determinants of health (SDOH), non-medical factors (neighborhood quality, access to education and health care, economic stability, and social and community support) that impact disease risk, mental health, life expectancy, education and development, productivity, economic stability, and overall quality of life (Figure 1A) (58–62). Adverse SDOH are the legacy of discriminatory practices, such as redlining and disinvestment, which created housing, economic, educational and health inequalities in minority communities that are still felt today (63, 64). Historically redlined neighborhoods across the U.S. are not only associated with current markers of neighborhood disadvantage and elevated social stressors (discrimination, crime, violence, and noise), but also correlate with elevated exposure to pollutants (63, 65). Therefore, allostatic load is useful for capturing the weathering effects of SDOH to assess how they affect pollutant susceptibility and responses.
Because of the effects allostatic load has on xenobiotic metabolism, immune dysfunction, and cardiometabolic health, it is plausible that allostatic load increases susceptibility to environmental pollutants. This is further supported by epidemiological studies reporting a link between adverse psychosocial experiences, toxicant exposures, and greater risks for adverse health outcomes. A study in infants reported that prenatal exposure to both air pollution and maternal stress were associated with lower orienting and regulation (OR) at 4 months, which predicted lower competence and increased behavioral problems at 12 months (66). Other studies have found strong associations between air pollution and asthma in children exposed to community violence (67, 68). Similar associations were observed among children from mothers with less than a high school education, black children, and children living in geographic areas with a higher percentage of black residents (69). Additionally, in adults, strong associations have been found between high psychosocial stress, PM2.5 exposure and systolic blood pressure (70). Among the most impactful studies highlighting the link between allostatic load and environmental disparities is a study associated with the Baltimore Housing Mobility Program, which was designed to address historic and contemporary housing discrimination. This study demonstrated that removing factors causing allostatic load, by moving families from high to low poverty neighborhoods with increased access to resources (food, safe schools, etc.), decreased symptoms and improved disease outcomes in children with asthma (71). Interestingly, indoor PM2.5 and PM10 concentrations, as well as levels of allergens, were less associated with changes in asthma outcomes than markers of stress. These examples underscore the impact stressful events, before and during one's lifespan, can have on susceptibility to environmental exposures and suggest that interventions addressing causes of allostatic load can significantly improve environmental health disparities.
Currently, operationalizing allostatic load is limited by the substantial variability in scoring approaches used throughout the literature. Since the introduction of the allostatic load score, the number of primary and secondary biomarkers included in allostatic load indices has grown from the original 10 to approximately 60, with individual scores now incorporating a variable range of 6–25 biomarkers (72). Most commonly, biomarkers are converted into dichotomous variables using high-risk quartiles to assign risk. Typically, biomarkers in either the highest or lowest 25% of the sample distribution are deemed high-risk and assigned a value of 1, while “low-risk” biomarkers are assigned a value of 0. The total allostatic load score then reflects the sum of high-risk biomarkers for an individual (73). Calculating high-risk quartiles based on the sample distribution may skew results by baselessly weighing biomarkers from certain biological systems more than others. Additionally, because the sample distribution is study-specific, results can vary significantly across studies due to study demographics, making it harder to compare findings or establish thresholds for high allostatic load (73). Alternatively, some studies use clinically-relavant cutoffs to dichotomize biomarkers into high vs. low risk and compute scores. However, many biomarkers lack established clinical cutoffs and this approach may fail to detect subclinical changes that are characteristic of allostatic load (73). Less common approaches include calculating the sum or average of biomarker Z-scores and summing dichotomous systems-level scores to generate an overall allostaic load score (73). Because biomarker Z-scores are weighted based on its deviation from the mean, biomarkers with greater deviation will have a greater weight and impact on the overall allostatic load score without proper justification (5). Translating allostatic load scores into meaningful interpretations poses another challenge. Allostatic load scores are often reported as either continuous variables or categories (low, medium, or high) using a variety of approaches, such as assigning scores above the median, or in the highest quartile, as high or using arbitrary thresholds based on score distributions (73). Despite these limitations, the utility of allostatic load justifies greater efforts to develop standardized methods. To improve the validity and comparability of allostatic load, researchers suggest using a core set of biomarkers to improve comparability. An individual participant data (IPD) meta-analysis identified a panel of five biomarkers—resting heart rate (RHR), high density lipoprotein (HDL), waist-to-height ratio (WtHR), C-reactive protein (CRP), and hemoglobin A1C (HbA1C)- that predicted health outcomes and mortality similar to longer allostatic load measures (74). Using a small set of biomarkers consistently across studies is a first step toward standardizing allostatic load scoring. This framework can be re-evaluated with additional markers as new research emerges.
Integrating allostatic load into environmental exposure assessments is an emerging area of research. Previous studies have commonly used high-risk thresholds to calculate allostatic load scores, followed by regression models to examine relationships between allostatic load and environmental pollutants such as PFAS, traffic-related air pollution, and PM 2.5 (75–77). More complex approaches, like multilevel mixed-effects generalized linear models, have been used to examine associations between residential greenspace and allostatic load (78). Association studies provide valuable insight into the relationship between stress and pollutant health effects; however, mechanistic studies are needed to better understand the underlying factors that contribute to increased susceptibility. Animal models have proven useful for studying stress-induced susceptibility to air pollutants (79). In these models, allostatic load can be integrated using tools like the rat cumulative allostatic load measure (rCALM) to explore stress and pollutant interactions (80, 81). In human exposure studies, allostatic load can be incorporated as critical variable, much like sex, to better understand its role in stress-induced susceptibility. By evaluating the mechanisms described earlier, researchers can gain insight into how allostatic load interacts with environmental exposures to influence health outcomes. Furthermore, longitudinal studies on allostatic load would help characterize the development of allostatic load over a lifespan as well as potential consequence of persistent exposures. For example, a longitudinal study by Mair et al., found that long-term proximity to petrochemical plants in Texas City was associated with higher allostatic load, particularly among women (82). Together, these approaches will help to enhance the precision of risk evaluations and guide more targeted interventions for vulnerable populations.
As allostatic load assessments become increasingly standardized and research on the environmental risks linked to allostatic load continues to grow, there is a greater opportunity to integrate these assessments into clinical practice. This integration can enhance patient evaluations, identify underlying stress-related health risks, and inform more personalized interventions to improve both mental and physical health. Incorporating allostatic load into clinical settings will also allow for a more comprehensive understanding of patients’ health, not only addressing the physical symptoms, but also the psychological and environmental factors that contribute to health and well-being (83).
In summary, epidemiological and experimental evidence support the relationship between stress and greater susceptibility to chemical stressors. Therefore, it is important to consider more rigorously stress as a biological variable in toxicological studies. Allostatic load is a powerful framework and tool that captures health disparities and socioeconomic disadvantages, while providing a cumulative stress score that can be integrated into risk assessments. Allostatic load overcomes the limitations of traditional stress assessment methods, including questionnaires, which are susceptible to bias or underreporting among racial and ethnic minorities, or using a single measure of cortisol, which naturally operate on a day-night rhythm and fail to account for the downstream effects of stress on the body (84, 85). Additionally, it is important that allostatic load is integrated into health data, to assess how chronic stress modifies biology and disease. The consequences of not accounting for allostatic load include having poorly representative research cohorts that fail to provide cumulative risk assessments for environmental and pharmaceutical chemicals. Therefore, future work is needed to refine allostatic load score measurements, establish a framework for incorporating allostatic load into risk assessment studies and clinical settings, and uncover the mechanisms underlying stress-induced susceptibility.
AB: Conceptualization, Visualization, Writing – original draft, Writing – review & editing. IJ: Conceptualization, Resources, Supervision, Writing – review & editing.
The author(s) declare that financial support was received for the research and/or publication of this article. AB was supported by the Environmental Protection Agency Cooperative Agreement with the University of North Carolina at Chapel Hill (UNC) CR 84033801, a pre-doctoral traineeship [National Research Service Award T32 ES007126] from the National Institute of Environmental Health Sciences, National Institutes of Health. This work was supported in part by a grant to UNC Chapel Hill from the Howard Hughes Medical Institute through the Gilliam Fellows Program.
The authors declare that the research was conducted in the absence of any commercial or financial relationships that could be construed as a potential conflict of interest.
All claims expressed in this article are solely those of the authors and do not necessarily represent those of their affiliated organizations, or those of the publisher, the editors and the reviewers. Any product that may be evaluated in this article, or claim that may be made by its manufacturer, is not guaranteed or endorsed by the publisher.
1. Rebuli ME, Speen AM, Martin EM, Addo KA, Pawlak EA, Glista-Baker E, et al. Wood smoke exposure alters human inflammatory responses to viral infection in a sex-specific manner. A randomized, placebo-controlled study. Am J Respir Crit Care Med. (2019) 199:996–1007. doi: 10.1164/rccm.201807-1287OC
2. Chu B, Marwaha K, Sanvictores T, Awosika AO, Ayers D. Physiology, Stress Reaction, in: StatPearls. Treasure Island, FL: StatPearls Publishing (2024).
3. Thomson EM. Air pollution, stress, and allostatic load: linking systemic and central nervous system impacts. J Alzheimers Dis. (2019) 69:597–614. doi: 10.3233/JAD-190015
4. Gee GC, Payne-Sturges DC. Environmental health disparities: a framework integrating psychosocial and environmental concepts. Environ Health Perspect. (2004) 112:1645–53. doi: 10.1289/ehp.7074
5. Juster R-P, McEwen BS, Lupien SJ. Allostatic load biomarkers of chronic stress and impact on health and cognition. Neurosci Biobehav Rev. (2010) 35:2–16. doi: 10.1016/j.neubiorev.2009.10.002
6. McEwen BS, Stellar E. Stress and the individual. Mechanisms leading to disease. Arch Intern Med. (1993) 153:2093–101. doi: 10.1001/archinte.1993.00410180039004
7. Sterling P, Eyer J. Allostasis: a new paradigm to explain arousal pathology. In: Fisher S, Reason J, editors. Handbook of Life Stress, Cognition and Health. New York: John Wiley & Sons (1988). p. 629–49.
8. Lee DY, Kim E, Choi MH. Technical and clinical aspects of cortisol as a biochemical marker of chronic stress. BMB Rep. (2015) 48:209–16. doi: 10.5483/BMBRep.2015.48.4.275
9. McEwen BS, Wingfield JC. What’s in a name? Integrating homeostasis, allostasis and stress. Horm Behav. (2010) 57:105. doi: 10.1016/j.yhbeh.2009.09.011
10. Seeman TE, Singer BH, Rowe JW, Horwitz RI, McEwen BS. Price of adaptation–allostatic load and its health consequences. MacArthur studies of successful aging. Arch Intern Med. (1997) 157:2259–68. doi: 10.1001/archinte.1997.00440400111013
11. Seeman TE, McEwen BS, Rowe JW, Singer BH. Allostatic load as a marker of cumulative biological risk: MacArthur studies of successful aging. Proc Natl Acad Sci. (2001) 98:4770–5. doi: 10.1073/pnas.081072698
12. Guidi J, Lucente M, Sonino N, Fava GA. Allostatic load and its impact on health: a systematic review. Psychother Psychosom. (2021) 90:11–27. doi: 10.1159/000510696
13. Zota AR, Shenassa ED, Morello-Frosch R. Allostatic load amplifies the effect of blood lead levels on elevated blood pressure among middle-aged U.S. adults: a cross-sectional study. Environ Health. (2013) 12:64. doi: 10.1186/1476-069X-12-64
14. Dhabhar FS, Malarkey WB, Neri E, McEwen BS. Stress-induced redistribution of immune cells - from barracks to boulevards to battlefields: a tale of three hormones - Curt Richter award winner. Psychoneuroendocrinology. (2012) 37:1345–68. doi: 10.1016/j.psyneuen.2012.05.008
15. McEwen BS. The neurobiology of stress: from serendipity to clinical relevance. Brain Res. (2000) 886:172–89. doi: 10.1016/s0006-8993(00)02950-4
16. Dhabhar FS, McEwen BS. Acute stress enhances while chronic stress suppresses cell-mediated immunity in vivo: a potential role for leukocyte trafficking. Brain Behav Immun. (1997) 11:286–306. doi: 10.1006/brbi.1997.0508
17. Dhabhar FS. Effects of stress on immune function: the good, the bad, and the beautiful. Immunol Res. (2014) 58:193–210. doi: 10.1007/s12026-014-8517-0
18. Kanterman J, Sade-Feldman M, Baniyash M. New insights into chronic inflammation-induced immunosuppression. Semin Cancer Biol. (2012) 22:307–18. doi: 10.1016/j.semcancer.2012.02.008
19. Glencross DA, Ho T-R, Camiña N, Hawrylowicz CM, Pfeffer PE. Air pollution and its effects on the immune system. Free Radic Biol Med. (2020) 151:56–68. doi: 10.1016/j.freeradbiomed.2020.01.179
20. Jin X, Chen Y, Xu B, Tian H. Exercise-Mediated protection against air pollution-induced immune damage: mechanisms, challenges, and future directions. Biology (Basel). (2024) 13:247. doi: 10.3390/biology13040247
21. Suzuki T, Hidaka T, Kumagai Y, Yamamoto M. Environmental pollutants and the immune response. Nat Immunol. (2020) 21:1486–95. doi: 10.1038/s41590-020-0802-6
22. McEwen BS. Interacting mediators of allostasis and allostatic load: towards an understanding of resilience in aging. Metab Clin Exp. (2003) 52:10–6. doi: 10.1016/S0026-0495(03)00295-6
23. Lagraauw HM, Kuiper J, Bot I. Acute and chronic psychological stress as risk factors for cardiovascular disease: insights gained from epidemiological, clinical and experimental studies. Brain Behav Immun. (2015) 50:18–30. doi: 10.1016/j.bbi.2015.08.007
24. Osei F, Block A, Wippert P-M. Association of primary allostatic load mediators and metabolic syndrome (MetS): a systematic review. Front Endocrinol. (2022) 13. doi: 10.3389/fendo.2022.946740
25. Di Dalmazi G, Pagotto U, Pasquali R, Vicennati V. Glucocorticoids and type 2 diabetes: from physiology to pathology. J Nutr Metab. (2012) 2012:525093. doi: 10.1155/2012/525093
26. Ryan KK. Stress and Metabolic Disease, in: Sociality, Hierarchy, Health: Comparative Biodemography: A Collection of Papers. US: National Academies Press (2014).
27. Borrell LN, Rodríguez-Álvarez E, Dallo FJ. Racial/ethnic inequities in the associations of allostatic load with all-cause and cardiovascular-specific mortality risk in U.S. adults. PLoS One. (2020) 15:e0228336. doi: 10.1371/journal.pone.0228336
28. Felix AS, Nolan TS, Glover LM, Sims M, Addison D, Smith SA, et al. The modifying role of resilience on allostatic load and cardiovascular disease risk in the Jackson heart study. J Racial Ethn Health Disparities. (2023) 10:2124–35. doi: 10.1007/s40615-022-01392-6
29. Park C, Ringel JB, Pinheiro LC, Morris AA, Sterling M, Balkan L, et al. Allostatic load and incident heart failure in the reasons for geographic and racial differences in stroke (REGARDS) study. BMC Cardiovasc Disord. (2023) 23:340. doi: 10.1186/s12872-023-03371-z
30. Kodavanti UP. Stretching the stress boundary: linking air pollution health effects to a neurohormonal stress response. Biochim Biophys Acta. (2016) 1860:2880–90. doi: 10.1016/j.bbagen.2016.05.010
31. Snow SJ, Henriquez AR, Costa DL, Kodavanti UP. Neuroendocrine regulation of air pollution health effects: emerging insights. Toxicol Sci. (2018) 164:9–20. doi: 10.1093/toxsci/kfy129
32. Abohashem S, Aldosoky W, Hahad O, Civieri G, Assefa A, Lau HC, et al. Additive effect of high transportation noise exposure and socioeconomic deprivation on stress-associated neural activity, atherosclerotic inflammation, and cardiovascular disease events. J Expo Sci Environ Epidemiol. (2024). doi: 10.1038/s41370-024-00734-2
33. Osborne MT, Abohashem S, Naddaf N, Abbasi T, Zureigat H, Mezue K, et al. The combined effect of air and transportation noise pollution on atherosclerotic inflammation and risk of cardiovascular disease events. J Nucl Cardiol. (2023) 30:665–79. doi: 10.1007/s12350-022-03003-7
34. Dar T, Osborne MT, Abohashem S, Abbasi T, Choi KW, Ghoneem A, et al. Greater neurobiological resilience to chronic socioeconomic or environmental stressors associates with lower risk for cardiovascular disease events. Circ Cardiovasc Imaging. (2020) 13:e010337. doi: 10.1161/CIRCIMAGING.119.010337
35. Osborne MT, Naddaf N, Abohashem S, Radfar A, Ghoneem A, Dar T, et al. A neurobiological link between transportation noise exposure and metabolic disease in humans. Psychoneuroendocrinology. (2021) 131:105331. doi: 10.1016/j.psyneuen.2021.105331
36. Osborne MT, Radfar A, Hassan MZO, Abohashem S, Oberfeld B, Patrich T, et al. A neurobiological mechanism linking transportation noise to cardiovascular disease in humans. Eur Heart J. (2020) 41:772–82. doi: 10.1093/eurheartj/ehz820
37. Tawakol A, Ishai A, Takx RA, Figueroa AL, Ali A, Kaiser Y, et al. Relation between resting amygdalar activity and cardiovascular events: a longitudinal and cohort study. Lancet Lond Engl. (2017) 389:834–45. doi: 10.1016/S0140-6736(16)31714-7
38. Abohashem S, Osborne MT, Dar T, Naddaf N, Abbasi T, Ghoneem A, et al. A leucopoietic-arterial axis underlying the link between ambient air pollution and cardiovascular disease in humans. Eur Heart J. (2021) 42:761–72. doi: 10.1093/eurheartj/ehaa982
39. Sagheer U, Al-Kindi S, Abohashem S, Phillips CT, Rana JS, Bhatnagar A, et al. Environmental pollution and cardiovascular disease: part 1 of 2: air pollution. JACC Adv. (2024) 3:100805. doi: 10.1016/j.jacadv.2023.100805
40. Daskalopoulos EP, Malliou F, Rentesi G, Marselos M, Lang MA, Konstandi M. Stress is a critical player in CYP3A, CYP2C, and CYP2D regulation: role of adrenergic receptor signaling pathways. Am J Physiol-Endocrinol Metab. (2012) 303:E40–54. doi: 10.1152/ajpendo.00545.2011
41. Choi H, Harrison R, Komulainen H, Saborit JMD. Polycyclic Aromatic Hydrocarbons, in: WHO Guidelines for Indoor Air Quality: Selected Pollutants. Bonn, Germany: World Health Organization Regional Office for Europe (2010).
42. Konstandi M, Marselos M, Radon-Camus AM, Johnson E, Lang MA. The role of stress in the regulation of drug metabolizing enzymes in mice. Eur J Drug Metab Pharmacokinet. (1998) 23:483–90. doi: 10.1007/BF03189999
43. Konstandi M, Johnson EO, Lang MA. Consequences of psychophysiological stress on cytochrome P450-catalyzed drug metabolism. Neurosci Biobehav Rev. (2014) 45:149–67. doi: 10.1016/j.neubiorev.2014.05.011
44. Konstandi M, Kostakis D, Harkitis P, Johnson EO, Marselos M, Adamidis K, et al. Benzo(α)pyrene-induced up-regulation of CYP1A2 gene expression: role of adrenoceptor-linked signaling pathways. Life Sci. (2006) 79:331–41. doi: 10.1016/j.lfs.2006.01.012
45. Konstandi M, Kostakis D, Harkitis P, Marselos M, Johnson EO, Adamidis K, et al. Role of adrenoceptor-linked signaling pathways in the regulation of CYP1A1 gene expression. Biochem Pharmacol. (2005) 69:277–87. doi: 10.1016/j.bcp.2004.09.024
46. Konstandi M, Johnson EO, Marselos M, Kostakis D, Fotopoulos A, Lang MA. Stress-mediated modulation of B(α)P-induced hepatic CYP1A1: role of catecholamines. Chem Biol Interact. (2004) 147:65–77. doi: 10.1016/j.cbi.2003.10.007
47. Fuhr U. Induction of drug metabolising enzymes: pharmacokinetic and toxicological consequences in humans. Clin Pharmacokinet. (2000) 38:493–504. doi: 10.2165/00003088-200038060-00003
48. Antonia K, Anastasia A, Tesseromatis C. Stress can affect drug pharmacokinetics via serum/tissues protein binding and blood flow rate alterations. Eur J Drug Metab Pharmacokinet. (2012) 37:1–7. doi: 10.1007/s13318-011-0077-2
49. Currie GM. Pharmacology, part 2: introduction to pharmacokinetics. J Nucl Med Technol. (2018) 46:221–30. doi: 10.2967/jnmt.117.199638
50. Czock D, Keller F, Rasche FM, Häussler U. Pharmacokinetics and pharmacodynamics of systemically administered glucocorticoids. Clin Pharmacokinet. (2005) 44:61–98. doi: 10.2165/00003088-200544010-00003
51. Zemanova N, Anzenbacher P, Anzenbacherova E. The role of cytochromes P450 in the metabolism of selected antidepressants and anxiolytics under psychological stress. Biomed Pap. (2022) 166:140–9. doi: 10.5507/bp.2022.019
52. Beckie TM. A systematic review of allostatic load, health, and health disparities. Biol Res Nurs. (2012) 14:311–46. doi: 10.1177/1099800412455688
53. Duru OK, Harawa NT, Kermah D, Norris KC. Allostatic load burden and racial disparities in mortality. J Natl Med Assoc. (2012) 104(1–2):89–95. doi: 10.1016/s0027-9684(15)30120-6
54. Geronimus AT. The weathering hypothesis and the health of African-American women and infants: evidence and speculations. Ethn Dis. (1992) 2:207–21.1467758
55. Geronimus AT, Hicken M, Keene D, Bound J. “Weathering” and age patterns of allostatic load scores among blacks and whites in the United States. Am J Public Health. (2006) 96:826–33. doi: 10.2105/AJPH.2004.060749
56. Rodriquez EJ, Kim EN, Sumner AE, Nápoles AM, Pérez-Stable EJ. Allostatic load: importance, markers, and score determination in minority and disparity populations. J Urban Health. (2019) 96:3–11. doi: 10.1007/s11524-019-00345-5
57. Reeves A, Elliott MR, Lewis TT, Karvonen-Gutierrez CA, Herman WH, Harlow SD. Study selection bias and racial or ethnic disparities in estimated age at onset of cardiometabolic disease among midlife women in the US. JAMA Netw Open. (2022) 5:e2240665. doi: 10.1001/jamanetworkopen.2022.40665
58. Embrett MG, Randall GE. Social determinants of health and health equity policy research: exploring the use, misuse, and nonuse of policy analysis theory. Soc Sci Med. (2014) 108:147–55. doi: 10.1016/j.socscimed.2014.03.004
59. Gustafsson PE, San Sebastian M, Janlert U, Theorell T, Westerlund H, Hammarström A. Life-course accumulation of neighborhood disadvantage and allostatic load: empirical integration of three social determinants of health frameworks. Am J Public Health. (2014) 104:904–10. doi: 10.2105/AJPH.2013.301707
60. Hickson DA, Diez Roux AV, Gebreab SY, Wyatt SB, Dubbert PM, Sarpong DF, et al. Social patterning of cumulative biological risk by education and income among African Americans. Am J Public Health. (2012) 102:1362–9. doi: 10.2105/AJPH.2011.300444
61. Schulkin J, McEwen BS, Gold PW. Allostasis, amygdala, and anticipatory angst. Neurosci Biobehav Rev. (1994) 18:385–96. doi: 10.1016/0149-7634(94)90051-5
62. Singh GK, Daus GP, Allender M, Ramey CT, Martin EK, Perry C, et al. Social determinants of health in the United States: addressing major health inequality trends for the nation, 1935–2016. Int J MCH AIDS 6. (2017) 6(2):139–64. doi: 10.21106/ijma.236
63. Hwa Jung K, Pitkowsky Z, Argenio K, Quinn JW, Bruzzese J-M, Miller RL, et al. The effects of the historical practice of residential redlining in the United States on recent temporal trends of air pollution near New York city schools. Environ Int. (2022) 169:107551. doi: 10.1016/j.envint.2022.107551
64. Martin CL, Ward-Caviness CK, Dhingra R, Zikry TM, Galea S, Wildman DE, et al. Neighborhood environment, social cohesion, and epigenetic aging. Aging. (2021) 13:7883–99. doi: 10.18632/aging.202814
65. Motairek I, Lee EK, Janus S, Farkouh M, Freedman D, Wright J, et al. Historical neighborhood redlining and contemporary cardiometabolic risk. J Am Coll Cardiol. (2022) 80:171–5. doi: 10.1016/j.jacc.2022.05.010
66. Liu R, DeSerisy M, Fox NA, Herbstman JB, Rauh VA, Beebe B, et al. Prenatal exposure to air pollution and maternal stress predict infant individual differences in reactivity and regulation and socioemotional development. J Child Psychol Psychiatry. (2022) 63:1359–67. doi: 10.1111/jcpp.13581
67. Clougherty JE, Levy JI, Kubzansky LD, Ryan PB, Suglia SF, Canner MJ, et al. Synergistic effects of traffic-related air pollution and exposure to violence on urban asthma etiology. Environ Health Perspect. (2007) 115:1140–6. doi: 10.1289/ehp.9863
68. Landeo-Gutierrez J, Celedón JC. Chronic stress and asthma in adolescents. Ann Allergy Asthma Immunol. (2020) 125:393–8. doi: 10.1016/j.anai.2020.07.001
69. Zanobetti A, Ryan PH, Coull BA, Luttmann-Gibson H, Datta S, Blossom J, et al. Early-life exposure to air pollution and childhood asthma cumulative incidence in the ECHO CREW consortium. JAMA Netw Open. (2024) 7:e240535. doi: 10.1001/jamanetworkopen.2024.0535
70. Hicken MT, Dvonch JT, Schulz AJ, Mentz G, Max P. Fine particulate matter air pollution and blood pressure: the modifying role of psychosocial stress. Environ Res. (2014) 133:195–203. doi: 10.1016/j.envres.2014.06.001
71. Pollack CE, Roberts LC, Peng RD, Cimbolic P, Judy D, Balcer-Whaley S, et al. Association of a housing mobility program with childhood asthma symptoms and exacerbations. JAMA. (2023) 329:1671–81. doi: 10.1001/jama.2023.6488
72. Johnson SC, Cavallaro FL, Leon DA. A systematic review of allostatic load in relation to socioeconomic position: poor fidelity and major inconsistencies in biomarkers employed. Soc Sci Med. (2017) 192:66–73. doi: 10.1016/j.socscimed.2017.09.025
73. Carbone JT, Clift J, Alexander N. Measuring allostatic load: approaches and limitations to algorithm creation. J Psychosom Res. (2022) 163:111050. doi: 10.1016/j.jpsychores.2022.111050
74. McCrory C, McLoughlin S, Layte R, NiCheallaigh C, O’Halloran AM, Barros H, et al. Towards a consensus definition of allostatic load: a multi-cohort, multi-system, multi-biomarker individual participant data (IPD) meta-analysis. Psychoneuroendocrinology. (2023) 153:106117. doi: 10.1016/j.psyneuen.2023.106117
75. Bashir T, Obeng-Gyasi E. The association between multiple per- and polyfluoroalkyl substances’ serum levels and allostatic load. Int J Environ Res Public Health. (2022) 19:5455. doi: 10.3390/ijerph19095455
76. Montresor-López JA, Reading SR, Yanosky JD, Mittleman MA, Bell RA, Crume TL, et al. The relationship between traffic-related air pollution exposures and allostatic load score among youth with type 1 diabetes in the SEARCH cohort. Environ Res. (2021) 197:111075. doi: 10.1016/j.envres.2021.111075
77. Xu H, Yang T, Guo B, Silang Y, Dai Y, Baima K, et al. Increased allostatic load associated with ambient air pollution acting as a stressor: cross-sectional evidence from the China multi-ethnic cohort study. Sci Total Environ. (2022) 831:155658. doi: 10.1016/j.scitotenv.2022.155658
78. Lai KY, Kumari S, Gallacher J, Webster CJ, Sarkar C. Association between residential greenness and allostatic load: a cohort study. Environ Sci Technol. (2024) 58(11):4884–93. doi: 10.1021/acs.est.3c04792
79. Clougherty JE, Rossi CA, Lawrence J, et al. Chronic social stress and susceptibility to concentrated ambient fine particles in rats. Environ Health Perspect. (2010) 118(6):769–75. doi: 10.1289/ehp.0901631
80. Fiamingo M, Bailey A, Toler S, Lee K, Oshiro W, Yoo B, et al. Enriched housing differentially alters allostatic load and cardiopulmonary responses to wildfire-related smoke in male and female mice. J Toxicol Environ Health A. (2024) 87(14):561–78. doi: 10.1080/15287394.2024.2346582
81. McCreary JK, Erickson ZT, Paxman E, Kiss D, Montina T, Olson DM, et al. The rat cumulative allostatic load measure (rCALM): a new translational assessment of the burden of stress. Environ Epigenetics. (2019) 5:dvz005. doi: 10.1093/eep/dvz005
82. Mair CA, Cutchin MP, Peek MK. Allostatic load in an environmental riskscape: the role of stressors and gender. Health Place. (2011) 17:978–87. doi: 10.1016/j.healthplace.2011.03.009
83. Fava GA, Sonino N, Lucente M, Guidi J. Allostatic load in clinical practice. Clin Psychol Sci. (2023) 11:345–56. doi: 10.1177/21677026221121601
84. Brown LL, Mitchell UA, Ailshire JA. Disentangling the stress process: race/ethnic differences in the exposure and appraisal of chronic stressors among older adults. J Gerontol B Psychol Sci Soc Sci. (2020) 75:650–60. doi: 10.1093/geronb/gby072
Keywords: allostatic load, stress, health disparities, environmental susceptibility, risk assessment
Citation: Bailey A and Jaspers I (2025) The next SABV—stress as a biological variable. Front. Environ. Health 4:1466951. doi: 10.3389/fenvh.2025.1466951
Received: 18 July 2024; Accepted: 25 February 2025;
Published: 13 March 2025.
Edited by:
Araceli Camargo, Centric Lab, United KingdomReviewed by:
Shady Abohashem, Massachusetts General Hospital and Harvard Medical School, United StatesCopyright: © 2025 Bailey and Jaspers. This is an open-access article distributed under the terms of the Creative Commons Attribution License (CC BY). The use, distribution or reproduction in other forums is permitted, provided the original author(s) and the copyright owner(s) are credited and that the original publication in this journal is cited, in accordance with accepted academic practice. No use, distribution or reproduction is permitted which does not comply with these terms.
*Correspondence: Ilona Jaspers, aWxvbmFfamFzcGVyc0BtZWQudW5jLmVkdQ==
†Present Address: Ilona Jaspers, NIEHS/NHLBI, Research Triangle Park, NC, United States
‡ORCID:
Aleah Bailey
orcid.org/0000-0002-5096-3716
Ilona Jaspers
orcid.org/0000-0001-8728-0305
Disclaimer: All claims expressed in this article are solely those of the authors and do not necessarily represent those of their affiliated organizations, or those of the publisher, the editors and the reviewers. Any product that may be evaluated in this article or claim that may be made by its manufacturer is not guaranteed or endorsed by the publisher.
Research integrity at Frontiers
Learn more about the work of our research integrity team to safeguard the quality of each article we publish.