- 1Mechanical Engineering Department, UPES, Dehradun, Uttarakhand, India
- 2Central Building Research Institute, Roorkee, India
- 3Mechanical Engineering Department, Institute of Engineering and Technology, GLA University, Mathura, Uttar Pradesh, India
- 4Future University in Egypt, New CairoCity, Egypt
Phase change materials (
1 Introduction
The best use of solar energy requires a storage facility because of the intermittent supply of solar energy. Various phase-changing materials (
The phase change of the
With accurate models for effective thermal conductivity, thermal dispersion coefficient of conductivity, permeability, inertial coefficient, and interstitial heat transfer coefficient, the volume-average method describes the melting and solidification of phase change materials in a graded metal foam. The total melting time of phase change material is reduced by 17.9% in the case of favourable gradient porosity and increased by 35.7% in the case of harmful gradient porosity. Positive gradients significantly impact temperature uniformity, raising it by 10.1%, whereas negative gradients lower it by 16.8%. Both the positive and negative gradients solidify considerably slower than the non-gradient structures5.7% and 38.5%, respectively) (Liu et al., 2022b).
For this reason, phase change materials (
1.1 Basic of thermal energy system (TES)
An overview of the many TES techniques is divided into physical processes, such as sensible and a combination of latent heat and chemical reactions, as shown in Figure 1. Numerous factors must be considered before picking TES content. The most important ones are chemical stability, mechanical toughness, low storage system corrosion density, and high energy storage density Jouhara et al., 2020. Peak load, duty cycle, operating temperature, deeper system integration, and simplicity of control are all things to consider while selecting
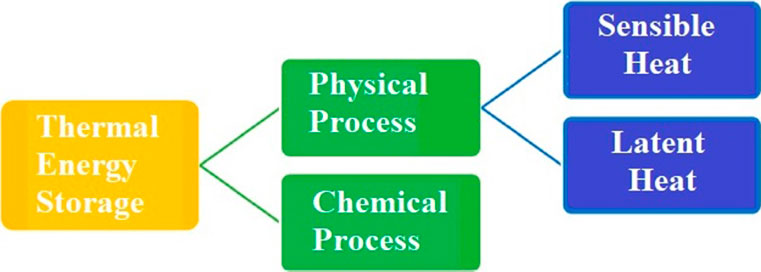
FIGURE 1. Thermal energy storage system (Jouhara et al., 2020).
1.2 Concept of latent heat storage (LHS)
The difference in enthalpy
According to the literature assessment, the
The latent heat capacity of a substance is described (Jouhara et al., 2020):
Where m is the mass of the PCMs (kg),
1.3 General equation for energy storage
The storage material must be chosen with consideration given to the TES’s operating temperature range, outside of which the material may undergo decomposition or an unfavourable phase change (Cingarapu et al., 2015b; Li et al., 2019b; Li et al., 2019d). The storage materials’ temperature-dependent material properties can significantly alter the storage’s behaviour, especially when large temperature swings are involved (Li et al., 2018c).
where
Under these conditions, the following equations describe the transient behaviour of a two-dimensional model.
Conservation of mass equation
Conservation of momentum equation
Where is the
Conservation of energyequation for air
where
Energy conservation equation for PCM capsules
Where
2 Previous experimental investigations on an energy storage system with various salt
Different salts have been used as energy storage in several practical analyses to evaluate solar energy storage’s effectiveness. Investigations were conducted on several characteristics, including stability, material performance, characterization, charging and discharging time. Various researchers conducted crucial studies on solar energy storage using salt as storage material, resulting in novel materials being developed. A combination of nanoparticles and salt, the manufacture of materials, and the confirmation of experimental findings with numerical models are studied and evaluated by researchers (Sharma et al., 1990; Zhao and Wu, 2011; Olivares and Edwards, 2013).
The temperature distributions and the heat transfer coefficients were calculated using steam as the
The eutectics of alkali chloride salts were regulated using 1% silica nanoparticles, which resulted in the heat capacity of the nanofluid increasing by 14.5%.
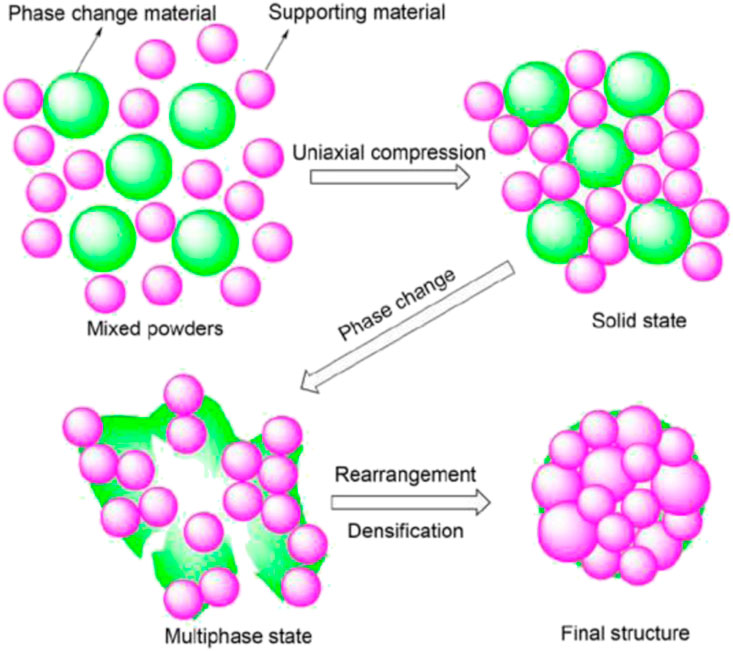
FIGURE 2. Schematic of the mechanism of microstructure development in
Researchers have identified and examined two crucial variables that control the storage system. Each significantly impacts reaction kinetics andhe reactor’s thermal power (Michel et al., 2014). A schematic and photographic representation of a prototype module is shown in Figure 3 (Michel et al., 2014).
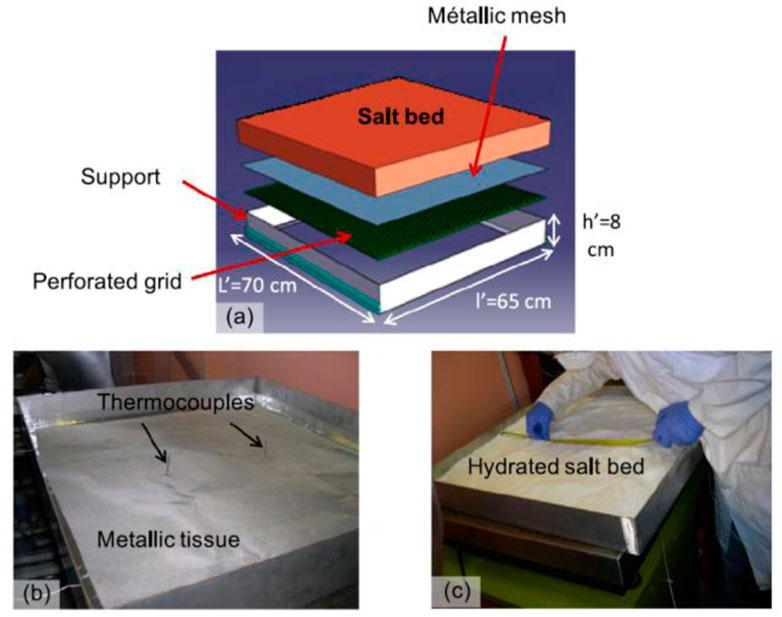
FIGURE 3. Schematic and photographic view of a module of the prototype (Michel et al., 2014). License Number: 5526331096176.
High-temperature heat transmission characteristics of an
An experimentally and numerically generated extremely high-temperature latent
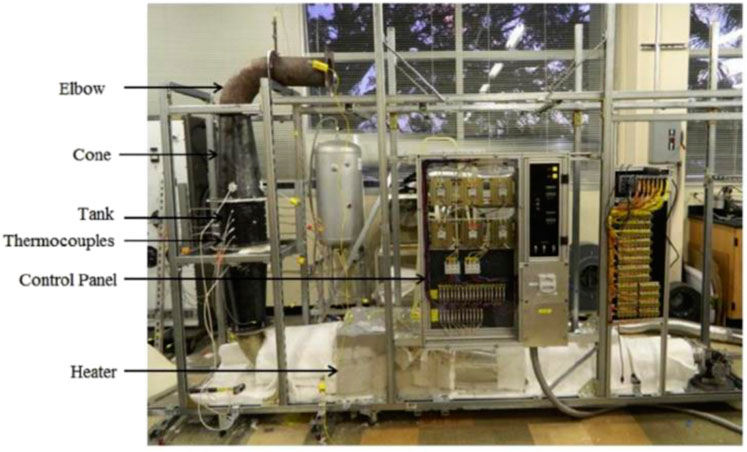
FIGURE 4. Energy storage system laboratory using PCM capsules made of molten salt (Bellan et al., 2015). License Number: 5526331356459.
The eutectic
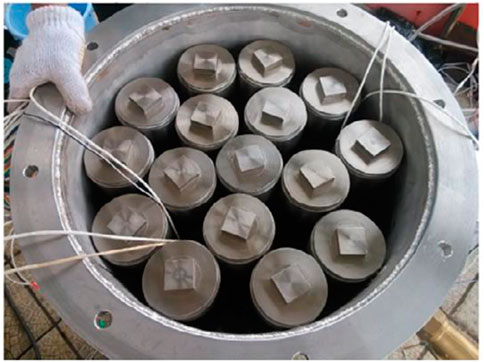
FIGURE 5. Photographic view of lab facilities of the TES tank (Zhang et al., 2016). License Number: 5526340217542.
A modest amount of hydrated salt, which is a
Thermochemical energy storage compounds of magnesium chloride hydrates show the potential for higher energy storage. With a relative humidity of less than 30%, it has been determined that magnesium chloride hydrates are the sole option (Kohler et al., 2018).
A novel packed bed thermal energy storage (
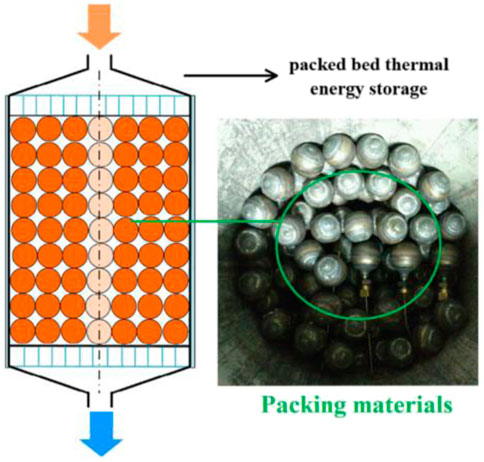
FIGURE 6. Packed bed TES system and PCM (Li et al., 2018c). License Number: 5526340562731.
Utilizing thermal analytic techniques for
The thermal system was analyzed experimentally (Li M. J. et al., 2018) to determine the fusion enthalpy change, specific heat, density, and other thermo-physical parameters of the eutectic salt mixture. Figure 7 illustrates the system’s latent heat storage and transport principle (Li et al., 2018b).
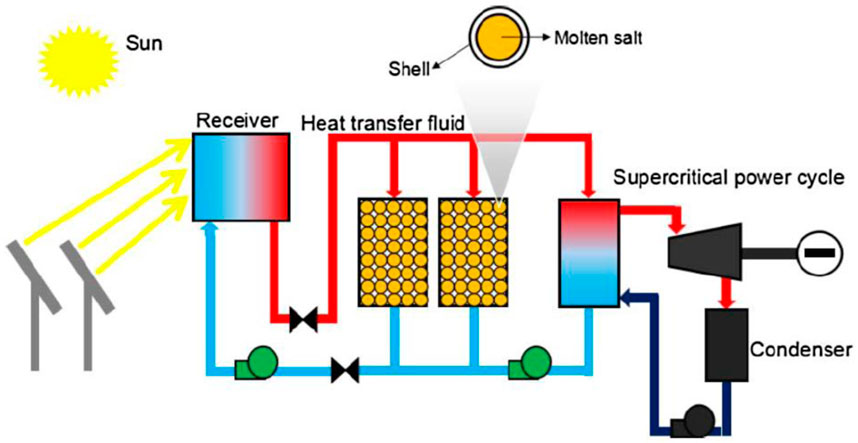
FIGURE 7. Representation of a heat transfer and latent heat storage system (Li et al., 2018b). License Number: 5526340717330.
There is some speculation that
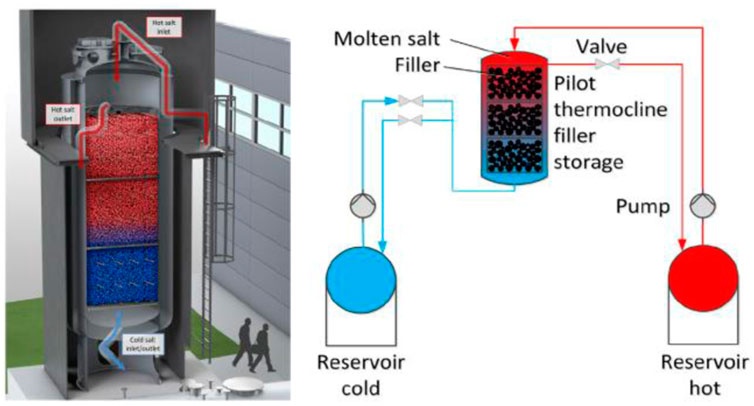
FIGURE 8. Schematic solar latent heat solar storage system (Martin et al., 2018). License Number: 5526341024752.
The possibility for salt-based
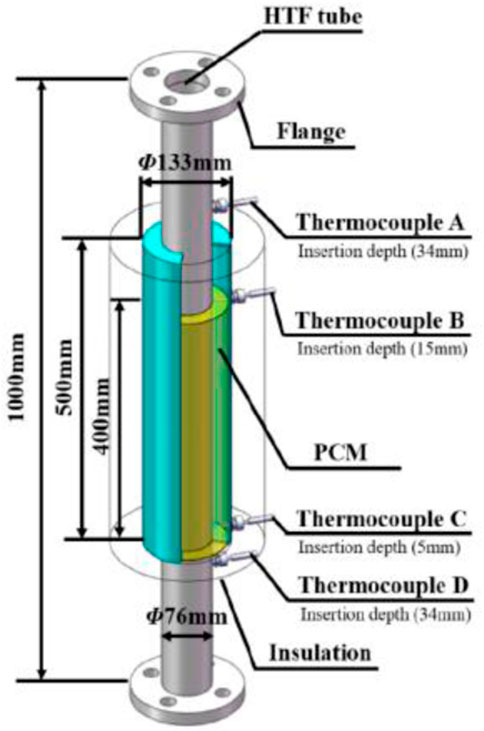
FIGURE 9. Photographic view of the investigational setup of PCM thermal energy storage system (Yuan et al., 2018). License Number: 5526341186605.
Phase change materials (
3 Previous numerical analysis on TES with different salt as storage materials
Numerous researchers used a numerical approach to examine various salt
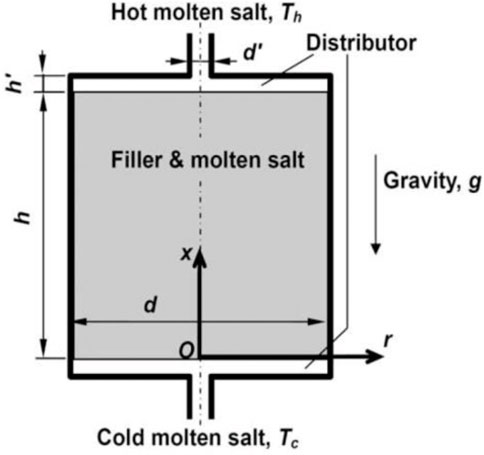
FIGURE 10. Diagrammatic representation of the thermocline TES system (Yang and Garimella, 2010). License Number: 5526341331974.
First and second-law efficiency ideas and first-law efficiency with an outflow temperature condition are utilized to evaluate storage performance (Flueckiger et al., 2013). According to the results, the
Lower melt flow rates, more excellent length ratios, and higher tank heights all increase cycle productivity. Cycle effectiveness is significantly impacted by filler particle size and container volume [67]. A thorough transient and
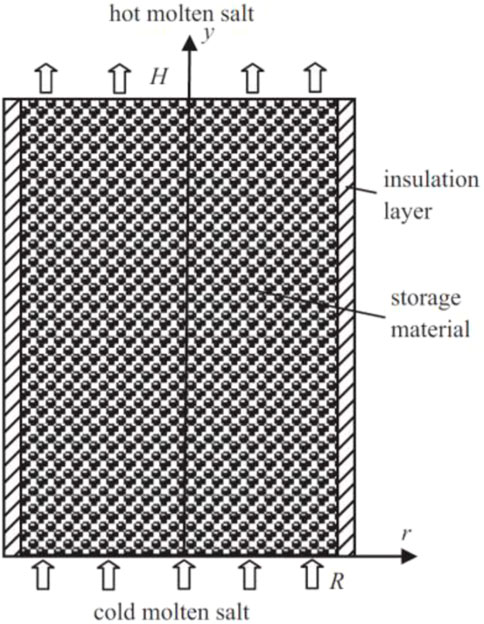
FIGURE 11. Schematic of molten salt thermocline TES (Lu et al., 2015). License Number: 5526341492967.
The importance of using molten salt in high-temperature concentrating solar power (
The effect of design and operating parameters on
According to several research studies, salt hydrates may be used in
The researchers also suggested that the molten-salt packed-bed
A practical
As temperatures rise, the radiative heat transfer takes a larger share of the total heat transfer. Deliberation of radiative heat transfer leads to improved temperature transmission between the
4 Comparative analysis of different properties and parameters of TES
The capacity to store and release substantial amounts of thermal energy at constant temperatures utilising Phase Change Materials (
Latent Heat Storage Capacity: The amount of thermal energy stored depends primarily on the
Thermal Stability: Another crucial aspect to consider is the
Thermal Conductivity: How efficiently heat is transferred from the
Cost-effectiveness: The
Compatibility with Environment: When deciding on a thermal energy storage system, it is essential to consider the
In conclusion,
5 Comparative analysis on with salt as energy storage material
Different salts utilised in
The ability to store heat at various temperature variations of the
6 Applications of TES
• Heating and cooling buildings: Through thermal energy storage, extra daytime heat can be stored and then released to assist with nighttime heating needs. In a similar vein, thermal energy storage can be used to store nighttime cold air and release it throughout the day to help with cooling buildings.
• Solar power plants:
• Industrial processes: Heat created in industrial processes can be stored in
• Transportation: Electric vehicles can employ
• Agriculture: Greenhouses can use
7 Conclusion
The current paper offers a thorough analysis of the literature on the effects of different salts on performance, stability, and other elements employed as
• The
• A densely packed phase transition bed stores latent heat in the
• The phase change material’s melting point must be between the effective outlet and starting temperatures for optimum thermal storage performance. The high content and latent heat of phase transition materials may also benefit the thermal storage system.
• To assess the effectiveness of the
• The
• The
Experiments with thermal energy storage involve the controlled release of previously stored heat energy. High heat capacity materials, phase transition materials, and sensible heat storage systems are just a few options for storing thermal energy. Phase change materials are frequently used because of their ability to absorb or release vast amounts of heat energy during their phase transitions from solid to liquid or liquid to gas. Overall, the thermal energy storage experiments aim to increase the efficiency and efficacy of storing and utilizing heat energy, which has important implications in renewable energy systems and lowering conventional energy consumption.
Author contributions
Conceptualization, AK, RM, TA, NG, and AD; methodology, AK, RM, and SS, Software, AK, RM, and SS, Resources, TA, NG, and AD, writing—original draft preparation, AK, RM, SS, and AD writing—review and editing, TA, NG, and AD; visualization AK, RM, and SS, Supervision, AK and TA.
Conflict of interest
The authors declare that the research was conducted in the absence of any commercial or financial relationships that could be construed as a potential conflict of interest.
Publisher’s note
All claims expressed in this article are solely those of the authors and do not necessarily represent those of their affiliated organizations, or those of the publisher, the editors and the reviewers. Any product that may be evaluated in this article, or claim that may be made by its manufacturer, is not guaranteed or endorsed by the publisher.
Abbreviation
DSC, Differential scanning calorimetry; PCM, Phase change material; PBTES, Packed bed thermal energy storage; TES, Thermal Energy Storage; MS, Molten salt; LHTES, Latest Heat Thermal Energy Storage.
References
Abdulla, A., and Reddy, K. S. (2017). Effect of operating parameters on thermal performance of molten salt packed-bed thermocline thermal energy storage system for concentrating solar power plants. Int. J. Therm. Sci. 121, 30–44. doi:10.1016/j.ijthermalsci.2017.07.004
Agarwala, S., and Prabhu, N. K. (2019). Characterization of metals and salts-based thermal energy storage materials using energy balance method. Heat Transf. - Asian Res. 48 (5), 1889–1898. doi:10.1002/htj.21461
Al-Abbasi, O., Abdelkefi, A., and Ghommem, M. (2017). Modeling and assessment of a thermochemical energy storage using salt hydrates. Int. J. Energy Res. 41 (14), 2149–2161. doi:10.1002/er.3776
Awad, A., Burns, A., Waleed, M., Al-Yasiri, M., and Wen, D. (2018). Latent and sensible energy storage enhancement of nano-nitrate molten salt. Sol. Energy 172, 191–197. doi:10.1016/j.solener.2018.04.012
Balasubramanian, G., Ghommem, M., Hajj, M. R., Wong, W. P., Tomlin, J. A., and Puri, I. K. (2010). Modeling of thermochemical energy storage by salt hydrates. Int. J. Heat Mass Transf. 53 (25–26), 5700–5706. doi:10.1016/j.ijheatmasstransfer.2010.08.012
Bellan, S., Alam, T. E., González-Aguilar, J., Romero, M., Rahman, M. M., Goswami, D. Y., et al. (2015). Numerical and experimental studies on heat transfer characteristics of thermal energy storage system packed with molten salt PCM capsules. Appl. Therm. Eng. 90, 970–979. doi:10.1016/j.applthermaleng.2015.07.056
Bonk, A., Knoblauch, N., Braun, M., Bauer, T., and Schmücker, M. (2020). An inexpensive storage material for molten salt based thermocline concepts: Stability of AlferRock in solar salt. Sol. Energy Mater. Sol. Cells 212, 110578. doi:10.1016/j.solmat.2020.110578
Calderón-Vásquez, I., Segovia, V., Cardemil, J. M., and Barraza, R. (2021). Assessing the use of copper slags as thermal energy storage material for packed-bed systems. Energy 227, 120370. doi:10.1016/j.energy.2021.120370
Cingarapu, S., Singh, D., Timofeeva, E. v., and Moravek, M. R. (2015a). Use of encapsulated zinc particles in a eutectic chloride salt to enhance thermal energy storage capacity for concentrated solar power. Renew. Energy 80, 508–516. doi:10.1016/j.renene.2015.02.026
Cingarapu, S., Singh, D., Timofeeva, E. V., and Moravek, M. R. (2015b). Use of encapsulated zinc particles in a eutectic chloride salt to enhance thermal energy storage capacity for concentrated solar power. Renew. Energy 80, 508–516. doi:10.1016/J.RENENE.2015.02.026
Du, L., Ding, J., Tian, H., Wang, W., Wei, X., and Song, M. (2017). Thermal properties and thermal stability of the ternary eutectic salt NaCl-CaCl2-MgCl2 used in high-temperature thermal energy storage process. Appl. Energy 204, 1225–1230. doi:10.1016/j.apenergy.2017.03.096
Flueckiger, S. M., Yang, Z., and Garimella, S. v. (2013). Review of molten-salt thermocline tank modeling for solar thermal energy storage. Heat. Transf. Eng. 34 (10), 787–800. doi:10.1080/01457632.2012.746152
Fonseca-Aten, M., Salvatore, C. M., Mejías, A., Ríos, A. M., Chávez-Bueno, S., Katz, K., et al. (2005). Evaluation of LBM415 (NVP PDF-713), a novel peptide deformylase inhibitor, for treatment of experimental Mycoplasma pneumoniae pneumonia. Antimicrob. Agents Chemother. 49 (10), 4128–4136. doi:10.1128/AAC.49.10.4128-4136.2005
Gage, S. H., Kesseli, D., Dupree, J., Kimbal, C., Rigby, J., Yates, J., et al. (2021). Technical and economic feasibility of molten chloride salt thermal energy storage systems. Sol. Energy Mater. Sol. Cells 226, 111099. doi:10.1016/j.solmat.2021.111099
Ge, Z., Ye, F., Cao, H., Leng, G., Qin, Y., and Ding, Y. (2014). Carbonate-salt-based composite materials for medium- and high-temperature thermal energy storage. Particuology 15, 77–81. doi:10.1016/j.partic.2013.09.002
Grosu, Y., Anagnostopoulos, A., Balakin, B., Krupanek, J., Navarro, M. E., González-Fernández, L., et al. (2021). Nanofluids based on molten carbonate salts for high-temperature thermal energy storage: Thermophysical properties, stability, compatibility and life cycle analysis. Sol. Energy Mater. Sol. Cells 220, 110838. doi:10.1016/j.solmat.2020.110838
Hu, Y., He, Y., Zhang, Z., and Wen, D. (2019). Enhanced heat capacity of binary nitrate eutectic salt-silica nanofluid for solar energy storage. Sol. Energy Mater. Sol. Cells 192, 94–102. doi:10.1016/j.solmat.2018.12.019
Huang, J., Wang, T., Zhu, P., and Xiao, J. (2013). Preparation, characterization, and thermal properties of the microencapsulation of a hydrated salt as phase change energy storage materials. Thermochim. Acta 557, 1–6. doi:10.1016/j.tca.2013.01.019
Jiang, Y., Sun, Y., Bruno, F., and Li, S. (2017). Thermal stability of Na2CO3-Li2CO3 as a high temperature phase change material for thermal energy storage. Thermochim. Acta 650, 88–94. doi:10.1016/j.tca.2017.01.002
Jo, B., and Banerjee, D. (2015). Effect of solvent on specific heat capacity enhancement of binary molten salt-based carbon nanotube nanomaterials for thermal energy storage. Int. J. Therm. Sci. 98, 219–227. doi:10.1016/j.ijthermalsci.2015.07.020
Jouhara, H., Żabnieńska-Góra, A., Khordehgah, N., Ahmad, D., and Lipinski, T. (2020). Latent thermal energy storage technologies and applications: A review. Int. J. Thermofluids 5, 100039. doi:10.1016/j.ijft.2020.100039
Kheradmand, M., Azenha, M., de Aguiar, J. L. B., and Castro-Gomes, J. (2016). Experimental and numerical studies of hybrid PCM embedded in plastering mortar for enhanced thermal behaviour of buildings. Energy 94, 250–261. doi:10.1016/J.ENERGY.2015.10.131
Kohler, T., Biedermann, T., and Müller, K. (2018). Experimental study of MgCl2 ⋅ 6 H2O as thermochemical energy storage material. Energy Technol. 6 (10), 1935–1940. doi:10.1002/ente.201800042
Lappalainen, J., Hakkarainen, E., Sihvonen, T., Rodríguez-García, M. M., and Alopaeus, V. (2019). Modelling a molten salt thermal energy system – a validation study. Appl. Energy 233–234, 126–145. doi:10.1016/j.apenergy.2018.10.009
Lee, D., and Jo, B. (2021). Thermal energy storage characteristics of binary molten salt nanofluids: Specific heat and latent heat. Int. J. Energy Res. 45 (2), 3231–3241. doi:10.1002/er.6019
Li, B., Tan, H., Liu, Y., Liu, Q., Zhang, G., Feng, X., et al. (2020a). Experimental investigations on the thermal stability of Na2CO3–K2CO3 eutectic salt/ceramic composites for high temperature energy storage. Renew. Energy 146, 2556–2565. doi:10.1016/j.renene.2019.08.027
Li, C., Li, Q., Cong, L., Li, Y., Liu, X., Xuan, Y., et al. (2019a). Carbonate salt based composite phase change materials for medium and high temperature thermal energy storage: A microstructural study. Sol. Energy Mater. Sol. Cells 196, 25–35. doi:10.1016/J.SOLMAT.2019.03.035
Li, C., Li, Q., and Ding, Y. (2019b). Investigation on the effective thermal conductivity of carbonate salt based composite phase change materials for medium and high temperature thermal energy storage. Energy 176, 728–741. doi:10.1016/j.energy.2019.04.029
Li, C., Li, Q., and Ding, Y. (2019c). Investigation on the thermal performance of a high temperature packed bed thermal energy storage system containing carbonate salt based composite phase change materials. Appl. Energy 247, 374–388. doi:10.1016/J.APENERGY.2019.04.031
Li, C., Li, Q., Li, Y., She, X., Cao, H., Zhang, P., et al. (2019d). Heat transfer of composite phase change material modules containing a eutectic carbonate salt for medium and high temperature thermal energy storage applications. Appl. Energy 238, 1074–1083. doi:10.1016/j.apenergy.2019.01.184
Li, M. J., Jin, B., Ma, Z., and Yuan, F. (2018a). Experimental and numerical study on the performance of a new high-temperature packed-bed thermal energy storage system with macroencapsulation of molten salt phase change material. Appl. Energy 221, 1–15. doi:10.1016/j.apenergy.2018.03.156
Li, T. X., Wu, D. L., He, F., and Wang, R. Z. (2017a). Experimental investigation on copper foam/hydrated salt composite phase change material for thermal energy storage. Int. J. Heat Mass Transf. 115, 148–157. doi:10.1016/j.ijheatmasstransfer.2017.07.056
Li, T. X., Xu, J. X., Wu, D. L., He, F., and Wang, R. Z. (2019e). High energy-density and power-density thermal storage prototype with hydrated salt for hot water and space heating. Appl. Energy 248, 406–414. doi:10.1016/j.apenergy.2019.04.114
Li, X., Wang, Y., Wu, S., and Xie, L. (2018b). Preparation and investigation of multicomponent alkali nitrate/nitrite salts for low temperature thermal energy storage. Energy 160, 1021–1029. doi:10.1016/j.energy.2018.07.078
Li, X., Wu, S., Wang, Y., and Xie, L. (2018c). Experimental investigation and thermodynamic modeling of an innovative molten salt for thermal energy storage (TES). Appl. Energy 212, 516–526. doi:10.1016/J.APENERGY.2017.12.069
Li, X., Xu, E., Song, S., Wang, X., and Yuan, G. (2017b). Dynamic simulation of two-tank indirect thermal energy storage system with molten salt. Renew. Energy 113, 1311–1319. doi:10.1016/j.renene.2017.06.024
Li, Z., Li, B., Du, X., and Wu, H. (2020b). Experimental investigation on stability of thermal performances of solar salt based nanocomposite. Renew. Energy 146, 816–827. doi:10.1016/j.renene.2019.07.009
Liu, G., Du, Z., Xiao, T., Guo, J., Lu, L., Yang, X., et al. (2022a). Design and assessments on a hybrid pin fin-metal foam structure towards enhancing melting heat transfer: An experimental study. Int. J. Therm. Sci. 182, 107809. doi:10.1016/J.IJTHERMALSCI.2022.107809
Liu, G., Xiao, T., Guo, J., Wei, P., Yang, X., and Hooman, K. (2022b). Melting and solidification of phase change materials in metal foam filled thermal energy storage tank: Evaluation on gradient in pore structure. Appl. Therm. Eng. 212, 118564. doi:10.1016/J.APPLTHERMALENG.2022.118564
Liu, Y., Xie, M., Gao, X., Yang, Y., and Sang, Y. (2018). Experimental exploration of incorporating form-stable hydrate salt phase change materials into cement mortar for thermal energy storage. Appl. Therm. Eng. 140, 112–119. doi:10.1016/j.applthermaleng.2018.05.042
Liu, Y., and Yang, Y. (2017). Preparation and thermal properties of Na2CO3·10H2O-Na2HPO4·12H2O eutectic hydrate salt as a novel phase change material for energy storage. Appl. Therm. Eng. 112, 606–609. doi:10.1016/j.applthermaleng.2016.10.146
Liu, Z., Chen, Z., and Yu, F. (2019). Preparation and characterization of microencapsulated phase change materials containing inorganic hydrated salt with silica shell for thermal energy storage. Sol. Energy Mater. Sol. Cells 200, 110004. doi:10.1016/j.solmat.2019.110004
Lu, J., Yu, T., Ding, J., and Yuan, Y. (2015). Thermal storage performance of molten salt thermocline system with packed phase change bed. Energy Convers. Manag. 102, 267–274. doi:10.1016/j.enconman.2014.10.049
Lu, J., Zhang, Z., Wang, W., and Ding, J. (2021). Effects of mgo nanoparticles on thermo-physical properties of lino3-nano3-kno3 for thermal energy storage. Energies 14 (3), 677. doi:10.3390/en14030677
Mamani, V., Gutiérrez, A., and Ushak, S. (2018). Development of low-cost inorganic salt hydrate as a thermochemical energy storage material. Sol. Energy Mater. Sol. Cells 176, 346–356. doi:10.1016/j.solmat.2017.10.021
Mao, A., Park, J. H., Han, G. Y., Seo, T., and Kang, Y. (2010). Heat transfer characteristics of high temperature molten salt for storage of thermal energy. Korean J. Chem. Eng. 27 (5), 1452–1457. doi:10.1007/s11814-010-0260-1
Martin, C., Bonk, A., Braun, M., Odenthal, C., and Bauer, T. (2018). Investigation of the long-term stability of quartzite and basalt for a potential use as filler materials for a molten-salt based thermocline storage concept. Sol. Energy 171, 827–840. doi:10.1016/j.solener.2018.06.090
Meng, E., Yu, H., and Zhou, B. (2017). Study of the thermal behavior of the composite phase change material (PCM) room in summer and winter. Appl. Therm. Eng. 126, 212–225. doi:10.1016/J.APPLTHERMALENG.2017.07.110
Mi, X., Liu, R., Cui, H., Memon, S. A., Xing, F., and Lo, Y. (2016). Energy and economic analysis of building integrated with PCM in different cities of China. Appl. Energy 175, 324–336. doi:10.1016/J.APENERGY.2016.05.032
Michel, B., Mazet, N., and Neveu, P. (2014). Experimental investigation of an innovative thermochemical process operating with a hydrate salt and moist air for thermal storage of solar energy: Global performance. Appl. Energy 129, 177–186. doi:10.1016/j.apenergy.2014.04.073
Mohan, G., Venkataraman, M., Gomez-Vidal, J., and Coventry, J. (2018). Assessment of a novel ternary eutectic chloride salt for next generation high-temperature sensible heat storage. Energy Convers. Manag. 167, 156–164. doi:10.1016/j.enconman.2018.04.100
Myers, P. D., Alam, T. E., Kamal, R., Goswami, D. Y., and Stefanakos, E. (2016). Nitrate salts doped with CuO nanoparticles for thermal energy storage with improved heat transfer. Appl. Energy 165, 225–233. doi:10.1016/j.apenergy.2015.11.045
Navarrete, N., Mondragón, R., Wen, D., Navarro, M. E., Ding, Y., and Juliá, J. E. (2019). Thermal energy storage of molten salt –based nanofluid containing nano-encapsulated metal alloy phase change materials. Energy 167, 912–920. doi:10.1016/j.energy.2018.11.037
Niedermeier, K., Marocco, L., Flesch, J., Mohan, G., Coventry, J., and Wetzel, T. (2018). Performance of molten sodium vs. molten salts in a packed bed thermal energy storage. Appl. Therm. Eng. 141, 368–377. doi:10.1016/j.applthermaleng.2018.05.080
Olivares, R. I., and Edwards, W. (2013). LiNO3-NaNO3-KNO3 salt for thermal energy storage: Thermal stability evaluation in different atmospheres. Thermochim. Acta 560, 34–42. doi:10.1016/j.tca.2013.02.029
Olivares, R. I. (2012). The thermal stability of molten nitrite/nitrates salt for solar thermal energy storage in different atmospheres. Sol. Energy 86 (9), 2576–2583. doi:10.1016/J.SOLENER.2012.05.025
Ong, T. C., Sarvghad, M., Lippiatt, K., Griggs, L., Ryan, H., Will, G., et al. (2020). Review of the solubility, monitoring, and purification of impurities in molten salts for energy storage in concentrated solar power plants. Renew. Sustain. Energy Rev. 131, 110006. doi:10.1016/j.rser.2020.110006
Pan, G., Wei, X., Yu, C., Lu, Y., Li, J., Ding, J., et al. (2020). Thermal performance of a binary carbonate molten eutectic salt for high-temperature energy storage applications. Appl. Energy 262, 114418. doi:10.1016/j.apenergy.2019.114418
Pereira da Cunha, J., and Eames, P. (2016). Thermal energy storage for low and medium temperature applications using phase change materials – a review. Appl. Energy 177, 227–238. doi:10.1016/J.APENERGY.2016.05.097
Pethurajan, V., Suresh, S., Mojiri, A., and Konatt, A. J. (2020). Microencapsulation of nitrate salt for solar thermal energy storage-synthesis, characterisation and heat transfer study. Sol. Energy Mater. Sol. Cells 206, 110308. doi:10.1016/j.solmat.2019.110308
Pu, W., Yang, N., Yue, C., Bai, S., and Chen, Y. (2019). Simulation on direct contact heat transfer in gas-molten salt bubble column for high temperature solar thermal storage. Int. Commun. Heat Mass Transf. 104, 51–59. doi:10.1016/j.icheatmasstransfer.2019.02.019
Raud, R., Bell, S., Ong, T. C., Will, G., and Steinberg, T. A. (2018). Optimized salt selection for solar thermal latent heat energy storage. Adv. Sustain. Syst. 2 (11), 1800074. doi:10.1002/adsu.201800074
Rong, Z., Pan, G., Lu, J., Liu, S., Ding, J., Wang, W., et al. (2021). Ab-initio molecular dynamics study on thermal property of NaCl–CaCl2 molten salt for high-temperature heat transfer and storage. Renew. Energy 163, 579–588. doi:10.1016/j.renene.2020.08.152
Saranprabhu, M. K., and Rajan, K. S. (2019b). Enhancement of solid-phase thermal conductivity and specific heat of solar salt through addition of MWCNT: New observations and implications for thermal energy storage. Appl. Nanosci. 9 (8), 2117–2126. doi:10.1007/s13204-019-01107-0
Saranprabhu, M. K., and Rajan, K. S. (2019a). Magnesium oxide nanoparticles dispersed solar salt with improved solid phase thermal conductivity and specific heat for latent heat thermal energy storage. Renew. Energy 141, 451–459. doi:10.1016/j.renene.2019.04.027
Seo, J., and Shin, D. (2016). Size effect of nanoparticle on specific heat in a ternary nitrate (LiNO3-NaNO3-KNO3) salt eutectic for thermal energy storage. Appl. Therm. Eng. 102, 144–148. doi:10.1016/j.applthermaleng.2016.03.134
Sharma, S. K., Jotshi, C. K., and Kumar, S. (1990). Thermal stability of sodium salt hydrates for solar energy storage applications. Sol. Energy 45, 177–181. doi:10.1016/0038-092x(90)90051-D
Shen, C., Li, X., Yang, G., Wang, Y., Zhao, L., Mao, Z., et al. (2020). Shape-stabilized hydrated salt/paraffin composite phase change materials for advanced thermal energy storage and management. Chem. Eng. J. 385, 123958. doi:10.1016/j.cej.2019.123958
Shere, L., Trivedi, S., Roberts, S., Sciacovelli, A., and Ding, Y. (2019). Synthesis and characterization of thermochemical storage material combining porous zeolite and inorganic salts. Heat. Transf. Eng. 40 (13–14), 1176–1181. doi:10.1080/01457632.2018.1457266
Shin, D., and Banerjee, D. (2011). Enhancement of specific heat capacity of high-temperature silica-nanofluids synthesized in alkali chloride salt eutectics for solar thermal-energy storage applications. Int. J. Heat Mass Transf. 54 (5–6), 1064–1070. doi:10.1016/j.ijheatmasstransfer.2010.11.017
Sötz, V. A., Bonk, A., and Bauer, T. (2020). With a view to elevated operating temperatures in thermal energy storage - reaction chemistry of Solar Salt up to 630°C. Sol. Energy Mater. Sol. Cells 212, 110577. doi:10.1016/j.solmat.2020.110577
Sun, G., Liu, Y., Dong, S., and Wang, J. (2020). Study on novel molten salt-ceramics composite as energy storage material. J. Energy Storage 28, 101237. doi:10.1016/j.est.2020.101237
Sun, Z., Hu, C., Ni, H., Lu, G., Song, X., and Yu, J. (2018). Influence of impurity SO42− on the thermal performance of molten nitrates used for thermal energy storage. Energy Technol. 6 (10), 2065–2073. doi:10.1002/ente.201800172
Tao, Y. B., He, Y. L., and Qu, Z. G. (2012). Numerical study on performance of molten salt phase change thermal energy storage system with enhanced tubes. Sol. Energy 86 (5), 1155–1163. doi:10.1016/j.solener.2012.01.004
Vaka, M., Walvekar, R., Jagadish, P., Khalid, M., Mubarak, N. M., and Panchal, H. (2020a). High-temperature molten salts optimisation using mixture design for energy storage application. J. Energy Storage 32, 101981. doi:10.1016/j.est.2020.101981
Vaka, M., Walvekar, R., Khalid, M., Jagadish, P., Mubarak, N. M., and Panchal, H. (2020b). Synthesis of hybrid graphene/TiO2 nanoparticles based high-temperature quinary salt mixture for energy storage application. J. Energy Storage 31, 101540. doi:10.1016/j.est.2020.101540
Wan, Z., Wei, J., Qaisrani, M. A., Fang, J., and Tu, N. (2020). Evaluation on thermal and mechanical performance of the hot tank in the two-tank molten salt heat storage system. Appl. Therm. Eng. 167, 114775. doi:10.1016/j.applthermaleng.2019.114775
Wang, G., Yu, S., Niu, S., Chen, Z., and Hu, P. (2020a). A comprehensive parametric study on integrated thermal and mechanical performances of molten-salt-based thermocline tank. Appl. Therm. Eng. 170, 115010. doi:10.1016/j.applthermaleng.2020.115010
Wang, K., Li, M. J., Zhang, Z. D., Min, C. H., and Li, P. (2021). Evaluation of alternative eutectic salt as heat transfer fluid for solar power tower coupling a supercritical CO2 Brayton cycle from the viewpoint of system-level analysis. J. Clean. Prod. 279, 123472. doi:10.1016/j.jclepro.2020.123472
Wang, X., Yu, H., Li, L., and Zhao, M. (2016). Experimental assessment on the use of phase change materials (PCMs)-bricks in the exterior wall of a full-scale room. Energy Convers. Manag. 120, 81–89. doi:10.1016/J.ENCONMAN.2016.04.065
Wang, Y., Yu, K., and Ling, X. (2019). Experimental and modeling study on thermal performance of hydrated salt latent heat thermal energy storage system. Energy Convers. Manag. 198, 111796. doi:10.1016/j.enconman.2019.111796
Wang, Y., Yu, K., and Ling, X. (2020b). Experimental study on thermal performance of a mobilized thermal energy storage system: A case study of hydrated salt latent heat storage. Energy Build. 210, 109744. doi:10.1016/j.enbuild.2019.109744
Wu, S., Yan, T., Kuai, Z., and Pan, W. (2020). Experimental and numerical study of modified expanded graphite/hydrated salt phase change material for solar energy storage. Sol. Energy 205, 474–486. doi:10.1016/j.solener.2020.05.052
Wu, Y. T., Li, Y., Ren, N., Zhi, R. P., and Ma, C. F. (2018). Experimental study on the thermal stability of a new molten salt with low melting point for thermal energy storage applications. Sol. Energy Mater. Sol. Cells 176, 181–189. doi:10.1016/j.solmat.2017.12.001
Xiao, T., Liu, G., Guo, J., Shu, G., Lu, L., and Yang, X. (2022). Effect of metal foam on improving solid–liquid phase change in a multi-channel thermal storage tank. Sustain. Energy Technol. Assessments 53, 102533. doi:10.1016/J.SETA.2022.102533
Xiao, X., Jia, H., Wen, D., and Zhao, X. (2020). Thermal performance analysis of a solar energy storage unit encapsulated with HITEC salt/copper foam/nanoparticles composite. Energy 192, 116593. doi:10.1016/j.energy.2019.116593
Xiong, Y., Wang, Z., Sun, M., Wu, Y., Xu, P., Qian, X., et al. (2021). Enhanced thermal energy storage of nitrate salts by silica nanoparticles for concentrating solar power. Int. J. Energy Res. 45 (4), 5248–5262. doi:10.1002/er.6142
Yang, X., Yang, X., Qin, F. G. F., and Jiang, R. (2016). Experimental investigation of a molten salt thermocline storage tank. Int. J. Sustain. Energy 35 (6), 606–614. doi:10.1080/14786451.2014.930465
Yang, Z., and Garimella, S. (2013). Cyclic operation of molten-salt thermal energy storage in thermoclines for solar power plants. Appl. Energy 103, 256–265. doi:10.1016/j.apenergy.2012.09.043
Yang, Z., and Garimella, S. (2010). Thermal analysis of solar thermal energy storage in a molten-salt thermocline. Sol. Energy 84 (6), 974–985. doi:10.1016/j.solener.2010.03.007
Yin, H., Ding, J., Jiang, R., and Yang, X. (2017). Thermocline characteristics of molten-salt thermal energy storage in porous packed-bed tank. Appl. Therm. Eng. 110, 855–863. doi:10.1016/j.applthermaleng.2016.08.214
Yuan, F., Li, M. J., Ma, Z., Jin, B., and Liu, Z. (2018). Experimental study on thermal performance of high-temperature molten salt cascaded latent heat thermal energy storage system. Int. J. Heat Mass Transf. 118, 997–1011. doi:10.1016/j.ijheatmasstransfer.2017.11.024
Zhang, P., Ma, F., and Xiao, X. (2016). Thermal energy storage and retrieval characteristics of a molten-salt latent heat thermal energy storage system. Appl. Energy 173, 255–271. doi:10.1016/j.apenergy.2016.04.012
Zhang, S., and Yan, Y. (2021). Melting and thermodynamic properties of nanoscale binary chloride salt as high-temperature energy storage material. Case Stud. Therm. Eng. 25, 100973. doi:10.1016/j.csite.2021.100973
Zhao, B., Cheng, M., song, Liu, C., and Dai, Z. (2018a). Conceptual design and preliminary performance analysis of a hybrid nuclear-solar power system with molten-salt packed-bed thermal energy storage for on-demand power supply. Energy Convers. Manag. 166, 174–186. doi:10.1016/j.enconman.2018.04.015
Zhao, B., Cheng, M., song, Liu, C., and Dai, Z. (2018b). System-level performance optimization of molten-salt packed-bed thermal energy storage for concentrating solar power. Appl. Energy 226, 225–239. doi:10.1016/j.apenergy.2018.05.081
Zhao, B. C., and Wang, R. Z. (2019). Perspectives for short-term thermal energy storage using salt hydrates for building heating. Energy 189, 116139. doi:10.1016/j.energy.2019.116139
Zhao, C. Y., and Wu, Z. G. (2011). Thermal property characterization of a low melting-temperature ternary nitrate salt mixture for thermal energy storage systems. Sol. Energy Mater. Sol. Cells 95 (12), 3341–3346. doi:10.1016/j.solmat.2011.07.029
Zheng, H., Li, Y., Shi, D., Cheng, X., Gong, S., and Wang, X. (2020). Preparation and thermal property of unusual morphology NaNO3 modified by solution combustion for thermal energy storage. J. Energy Storage 29, 101366. doi:10.1016/j.est.2020.101366
Zhou, G., and Han, Y. (2017). Numerical simulation on thermal characteristics of supercooled salt hydrate PCM for energy storage: Multiphase model. Appl. Therm. Eng. 125, 145–152. doi:10.1016/j.applthermaleng.2017.07.010
Zhu, Y., Yuan, Y., Zhang, C., Xie, M., and Tan, H. (2019). Numerical study on heat transfer enhancement of thermal energy storage systems considering radiation of molten salt. Sol. Energy 183, 337–344. doi:10.1016/j.solener.2019.03.044
Keywords: thermal energy storage, phase change material (PCM), charging and discharing, heat, sensible
Citation: Kumar A, Maithani R, Sharma S, Alam T, Gupta NK and Deifalla AF (2023) A review of the effects of different parameters on salt-based solar thermal energy storage systems. Front. Energy Res. 11:1152714. doi: 10.3389/fenrg.2023.1152714
Received: 28 January 2023; Accepted: 06 April 2023;
Published: 18 April 2023.
Edited by:
R. Parameshwaran, Birla Institute of Technology and Science, IndiaReviewed by:
Xiaohu Yang, Xi’an Jiaotong University, ChinaShaopeng Guo, Xi’an University of Architecture and Technology, China
Copyright © 2023 Kumar, Maithani, Sharma, Alam, Gupta and Deifalla. This is an open-access article distributed under the terms of the Creative Commons Attribution License (CC BY). The use, distribution or reproduction in other forums is permitted, provided the original author(s) and the copyright owner(s) are credited and that the original publication in this journal is cited, in accordance with accepted academic practice. No use, distribution or reproduction is permitted which does not comply with these terms.
*Correspondence: Ahmed Farouk Deifalla, YWhtZWQuZGFpZmFsbGFAZnVlLmVkdS5lZw==