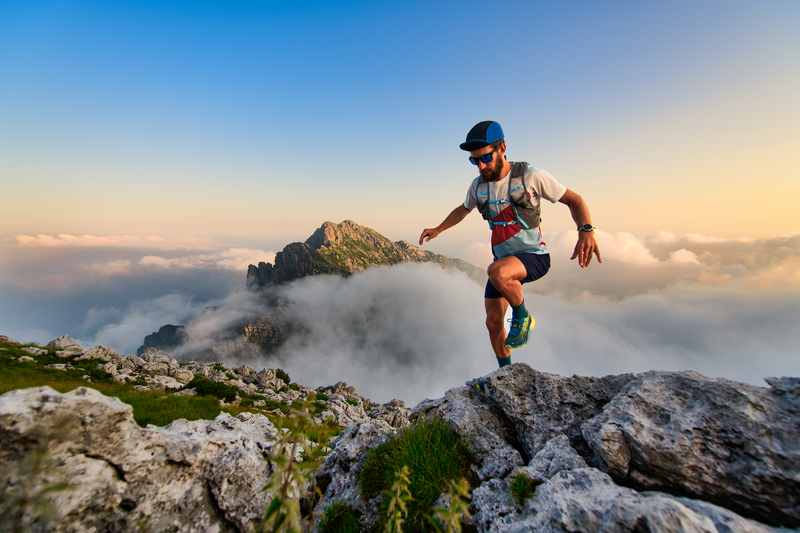
95% of researchers rate our articles as excellent or good
Learn more about the work of our research integrity team to safeguard the quality of each article we publish.
Find out more
ORIGINAL RESEARCH article
Front. Energy Res. , 09 July 2018
Sec. Bioenergy and Biofuels
Volume 6 - 2018 | https://doi.org/10.3389/fenrg.2018.00067
This article is part of the Research Topic Advancements in Biomass Feedstock Preprocessing: Conversion Ready Feedstocks View all 24 articles
This paper examines the efficacy of ionic liquid (IL) pretreatment on seven different commercially harvested biomass types: corn stover, miscanthus, pine, sorghum, sugarcane bagasse, switchgrass, and wheat straw in an effort to improve the production of renewable fuels and chemicals from biomass derived sugars. Initial experiments screened the pretreatment of lodgepole pine, a particularly recalcitrant biomass feedstock, with nine different imidazolium based ionic liquids. After screening, one hydrophilic and one hydrophobic ionic liquid was selected for pretreatment tests on six commercially harvested biomasses. Ultimately, the hydrophilic ionic liquid functioned better for biomass pretreatment than the hydrophobic ionic liquid. These results were then compared to a traditional dilute acid pretreatment to examine the relative effectiveness of ionic liquid pretreatment across a variety of biomass and ionic liquid types. Total theoretical sugar yields after IL pretreatment varied widely by IL and biomass type and ranged from 4.9 to 90.2%. Dilute acid pretreatment showed consistent sugar yields for herbaceous material (from 71.4 to 80.8%) but low yield for lodgepole pine (22.8%). Overall, ILs showed the potential to reach slightly higher sugar yields than dilute acid and were particularly effective for woody feedstocks. More importantly, the sugar release kinetics for IL pretreatment were three times faster than dilute acid and gave maximum sugar yields after about 24 h. Additional characterization of IL treated materials included scanning electron microscopy (SEM), x-ray diffraction (XRD), and compositional analysis. SEM and XRD showed qualitative and quantitative reductions in cellulose crystallinity (respectively) that correlated well to improved sugar release during enzymatic hydrolysis for hydrophilic ionic liquids. However, reductions in crystallinity associated with hydrophobic ionic liquids resulted in lower sugar release during enzymatic hydrolysis. Compositional analysis generally showed increased sugars content for hydrophilic ILs and increased lignin content for hydrophobic ILs.
Clean and domestic energy is an important issue for the development of sustainable and secure global communities. The United States has great opportunities to encourage the development of renewable energy as increased demand from residential, commercial, industrial, and transportation sectors, combined with the available billion tons of domestic biomass resources, (Perlack et al., 2011) could spur the growth of green fuels, chemicals, and energy. Lignocellulosic biomass is uniquely suited to address fuel and energy production challenges given that it can be transformed into liquid fuels and chemicals (Johnson et al., 2007; Floudas et al., 2012; Williams et al., 2012) and reduces GHG emissions through the capture of CO2 via photosynthesis. Additionally, utilizing waste biomass can create new jobs, as evidenced by the fact that in 2016 the Midwest US employed just over half a million people in clean energy industry jobs, (Trust, 2017) and second generation biorefineries are capable of generating further job growth (Miranowski, 2007). However, to achieve a robust second generation cellulosic refining industry two key factors need to be addressed: a stable supply of chemically consistent feedstock (Williams et al., 2016, 2017), and cost-effective pretreatment strategies due to greater hydrolysis recalcitrance than first generation feedstocks (Eranki et al., 2011; Banerjee et al., 2012).
While various biomass pretreatment strategies have been developed (Mosier et al., 2005; Alvira et al., 2010) this paper focuses on the comparison between a traditional dilute acid pretreatment and emerging ionic liquid pretreatments to understand, and evaluate, the effectiveness of ionic liquids. To date both dilute acid and IL pretreatment have been used for a variety of biomass types. A brief summary of previous work for both of these pretreatment strategies, and the results obtained, follows. Optimum dilute acid pretreatment conditions vary by feedstock (which explains the variety of conditions found in literature) but general conditions use millimeter size range particles, sulfuric acid at 0.5–2 wt%, temperatures from 100 to 190°C, and reaction times between 5 and 60 min. The percent of total sugars yielded from the combined pretreatment and enzymatic hydrolysis of these samples tends to be in the range of 40 to 90% but often averages 65%. Materials pretreated under this range of conditions include corn stover, (Lloyd and Wyman, 2005) miscanthus, (Sørensen et al., 2008; Yoshida et al., 2008) spruce, (Larsson et al., 1999) sugarcane bagasse, (Jackson De Moraes Rocha et al., 2011) switchgrass, (Dien et al., 2006) and wheat straw, (Saha et al., 2005) among others. The range of conditions and yields given above are represented in the references for the individual feedstocks.
Many different ionic liquids have been investigated for lignocellulose degradation due to their tunable properties (based on a multitude of possible anion and cation combinations) (Brandt et al., 2013). IL properties reported to be important for pretreatment of lignocellulosic biomass include viscosity, melting point, dipolarity, hydrogen bond basicity, and hydrophobicity/hydrophilicity (Mäki-Arvela et al., 2010). Among the tested ILs, imidazolium based ILs have demonstrated high capability to dissolve/degrade lignocellulose (Cheng et al., 2012; Brandt et al., 2013; Gräsvik et al., 2014). Reaction conditions typically have 10 wt% solids with reaction temperatures from 70 to 160°C and reaction times ranging from 30 min to a few hours. The percent of total sugars yielded ranges from about 60 to 90% (with an average around 70%) for most materials and ionic liquids tested. Materials treated with IL are more varied, and studies often treat blended feedstocks, such as; miscanthus/corn stover, (Shill et al., 2011) maple wood, (Lee et al., 2009) corn stover/pine/sugarcane bagasse, (Li et al., 2008) sugarcane bagasse with varying enzymes, (Qiu et al., 2012) wheat straw, (Li et al., 2009) and miscanthus (Brandt et al., 2011) (with a variety of ionic liquids that showed a strong dependence on the cation). Again, the range of conditions and yields given above are represented in the references for the individual feedstocks.
Biomass tested in this study includes corn stover, miscanthus, lodgepole pine, sorghum, sugarcane bagasse, switchgrass, and wheat straw, since they are commercially harvested and show great potentials for application in future biorefineries. The diversity represented in this selection spans pine as a softwood, sorghum, and corn stover stalks as representatives of grains, sugarcane bagasse as a special grass, with the other three feedstocks being traditional grasses. Nine imidazolium based ILs were selected for investigating performance variations between different types of ILs as listed in Table 1. These ILs cover a wide range of hydrophobicity. Table 1 is organized first by the most hydrophilic cations, decreasing in the series, ethyl, butyl, and then arranged by the perceived increasing hydrophobicity of anions from Cl−, I−, OTf−, , to . The four ILs with Bmim+ exhibit increasingly hydrophobic behavior as the anion varies from simple Cl−, to bulky OTf −, BF4−, and PF6−. On the other hand, if anions are same, the hydrophobicity of ILs depends on the length and structure of their cations' alkyl chain (Huddleston et al., 2001; Yee et al., 2013). The ILs with Cl− enable us to examine the effect of increasing alkyl chain length and structure from ethyl, to butyl, allyl, and butyl with two methyls on the performance of these ILs. Emim Ac has the highest basicity and has been demonstrated high capability to dissolve cellulose (Sun et al., 2009; Zavrel et al., 2009; Cheng et al., 2012; Brandt et al., 2013). Bmim Cl is one of the most studied ILs for dissolving cellulose and serves as a good reference (Sun et al., 2009; Gräsvik et al., 2014).
Published results encompass various biomass types with different treatment conditions including: type of ILs, feedstock type, feedstock particle size, particle water content, solids loading, dissolution time, and temperature (Mäki-Arvela et al., 2010; Gräsvik et al., 2014). This wide variety of reaction conditions and feedstocks causes great difficulty in comparing results. Attempts have been made to solve this problem for the ubiquitous dilute acid treatment by developing a generalized reaction severity parameters that takes into account for variations in pH, temperature, and reaction time (Pedersen and Meyer, 2010). However, a similar metric for ionic liquid pretreatment would be much more difficult to make given the almost infinite variety of ionic liquids available. Confounding this issue further is the wide array of conditions for enzymatic hydrolysis (with varying enzyme types and loadings) that occurs after pretreatment. This paper addresses these comparison issues by evaluating pretreatment effects of different ILs on different types of lignocellulosic biomass under identical conditions. Additionally, to aid the comparison with dilute acid pretreatment the IL pretreatment has been performed under conditions similar to what is now considered standard pretreatment conditions for dilute acid (Wolfrum et al., 2013). To date, only one paper has made a direct comparison between dilute acid and IL pretreatment for switchgrass, which showed that IL pretreatment has the potential to be far superior to dilute acid (Li et al., 2010).
This paper begins by screening nine different imidazolium based ILs for pretreatment of lodgepole pine. Next, the most promising and diverse candidates were tested across the variety of commercially harvested feedstocks and compared to a traditional dilute acid pretreatment. Additionally, a variety of physical and chemical characterization methods have been utilized to further understand the effects that diverse ionic liquids can have on this variety of biomass types.
Corn stover was collected by a single pass harvester in Boone County Iowa, Miscanthus was collected from Tift County Goergia, Sorghum was collected from Garvin County Oklahoma, Sugarcane Baggasse was collected from Pointe Coupee County Lousiana, switchgrass was collected from Garvin County Oklahoma, wheat straw was collected from Jefferson County Idaho, and Lodgepole pine was collected from Mineral County Montana. All biomass materials were processed on a Shute Buffalo mill through a ¼” screen before being ground to 500 μm using a Wiley Mill and finally size reduced to 200 μm on a Retsch Ultra Centrifugal Mill ZM 200. Ground samples were divided using a Retsch Sample Divider PT 100 to obtain a representative sample size for IL pretreatment. After deconstruction to such fine particle sizes biomass moisture content is ~5% on a wet basis.
Dilute-acid pretreatment was performed using an ASE 350 (Accelerated Solvent Extractor, ThermoFisher Scientific, Waltham, MA, USA) using procedures developed at National Renewable Energy Laboratory (NREL) (Wolfrum et al., 2013). Experiments were performed using 66-mL zirconium cells, and a 10% (w/w) solids loading with an acid-to-biomass loading of 0.08 g g−1. Each cell was filled with 3.0 ± 0.03 g biomass and 30 mL of 1% sulfuric acid (w/w). Cells were subjected to a 7-min heating period followed by a 7-min static time with a reaction temperature of 160°C, followed by 200 s of N2 purge. The temperature was then reduced to 100°C and 100 to 150-mL of nanopure water was rinsed through the cell with a 200 s N2 gas purge and the rinsate was collected. Aliquots of the rinsate were collected for determination of total and monomeric sugars and organic acids using High Performance Liquid Chromatography.
Lodgepole pine samples were treated with nine different ionic liquids, summarized in Table 1, at 160°C for 3 h. The dry biomass solids loadings was 10 wt% for all experiments at 15 g dry biomass and 135 g ionic liquid. After the reaction samples were washed three times with 300 mL of water (or ethanol in the case of ionic liquid BmimPF6 due to the hydrophobic nature of the hexafluorophosphate anion), filtered through 5 μm filter paper, and then dried at 40°C for at least 48 h to recover the pretreated solids. After the initial IL screening experiments with pine the washing procedure was altered to 300 mL each of ethanol, acetone, then water for the feedstock screening experiments to better wash the biomass (particularly for the more hydrophobic ILs).
Raw and pretreated solids were examined for structural and compositional alterations as well as altered reactivity. Structural analysis was performed by scanning electron microscopy (SEM) and x-ray diffraction (XRD). Biomass composition was analyzed using the National Renewable Energy Laboratory's standard laboratory analytical procedure (Sluiter et al., 2008) with the modification that the sample size was 0.2 mm instead of the usual 2 mm. Reactivity characterization was quantified using enzymatic hydrolysis. More detail about these procedures can be found below.
SEM images were taken for both untreated and pretreated materials using a JEOL Ltd. JSM 6610LV microscope. Prior to SEM analysis samples were prepared by mounting on standard aluminum pin stub mounts with biomass powder adhered via copper tape. Mounted samples were sputter coated with gold prior to analysis using a Hummer 6.2 Sputter System operating at 15 mA under 60 mTorr vacuum for 1 min. SEM images were acquired using a 15 kV accelerating voltage.
XRD was performed using a Rigaku SmartLab diffractometer. Scans were collected at 40 kV and 44 mA with a step size of 0.01°. The degree of cellulose crystallinity can be inferred by the ratio of peak heights for the cellulose I in the 002 plane at 2θ = 22.5 and the amorphous cellulose at 2θ = 16.6 (Segal et al., 1959).
Enzymatic hydrolysis was conducted using a modified version of the procedure described in Selig et al. (2008). Pretreated solids were enzymatically hydrolyzed by adding biomass up to an equivalent of 1.0 g of dry solids to a 50 mL incubation flask with 5 mL of 0.1 M citric acid buffer (pH 4.8), 100 μL of 2 % sodium azide solution, and enough nanopure water to reach a final reaction volume of 10 mL. Enzymes were added at 40 mg g−1 dry biomass for Cellic® CTec2 (Novozymes, Franklin, NC, USA) and 4 mg g−1 biomass for Cellic® HTec2 with enzyme and substrate blanks prepared as controls. To investigate sugar release kinetics, 150 μL aliquots of liquor were removed after 2, 4, 8, 24, 48, and 72 h of incubation at 50°C, filtered through a 0.2 μm filter, and analyzed for monomeric sugars using high performance liquid chromatography (Agilent HPLC Model 1260; Agilent Technologies; Santa Clara, CA). Sugars were analyzed on an Aminex HPX-87P column (BioRad Laboratories; Hercules, CA) with a column temperature of 85 °C using a refractive index detector, a mobile phase of 18 MΩ ultrapure water, and a flow rate of 0.6 mL min−1. The sugars evaluated for this study, defined as the “total sugar yield” included the sum of released glucose, xylose, galactose, arabinose, mannose, and cellobiose divided by those sugars present in the pretreated material multiplied by 100. Duplicate injections were performed for each sample.
Initial IL screening experiments used lodgepole pine as a feedstock because of its recalcitrant nature. The pine samples were treated with the nine ILs shown in Table 1 which was comprised of a set of imidazolium based ionic liquids. After IL treatment the pine was characterized using SEM (Figure 1) and XRD (Figure 2) for physical changes and examined for reactivity enhancement using enzymatic hydrolysis (Figure 3).
Figure 1. SEM images of IL treated lodgepole pine. (A) Raw pine, (B) EmimAc, (C) EmimCl, (D) BmimCl, (E) AmimCl, (F) BdimCl, (G) MpimI, (H) BmimOTf, (I) BmimBF4, and (J) BmimPF6. The scale bar in each image is 50 μm.
Figure 2. XRD images of raw and ionic liquid treated lodgepole pine. Ionic liquid abbreviations can be seen in Table 1.
Figure 3. Enzymatic hydrolysis of lodgepole pine. Theoretical sugar yield is the sum of glucan, xylan, galactose, arabinose, mannose, and cellobiose released divided by the sugars in the pretreated material.
The raw pine shown in Figure 1A demonstrates clear wood fibers and texture, which serves as a point of comparison for the IL treated samples, while other ILs show different alterations on biomass structure. Emim Ac (Figure 1B) showed a significant amount of degradation. This degradation likely has a strong link to the high hydrogen-bond basicity of the IL. Hydrogen-bond basicity is strongly linked to the IL's capability to dissolve or swell cellulose/lignocellulose because it is important for the hydrogen-bond accepting ability of the IL anions (Mäki-Arvela et al., 2010; Zhang et al., 2010; Brandt et al., 2013; Cláudio et al., 2014; Gräsvik et al., 2014).
Cation hydrophobicity was another key parameter varied in this study. Four Cl− based ILs, Emim Cl, Bmin Cl, Amim Cl, and Bdim Cl, as seen in Figures 1C–F respectively, showed increasing cation hydrophobicity with increasing length and branching structure of alkyl substituents (Huddleston et al., 2001; Yee et al., 2013). It is clear that the IL with the shortest alkyl chain (Emim Cl) altered the biomass structure by the greatest degree. The effect of the other ILs could not be clearly distinguished from the SEM images. The final IL structural trend investigated was that of anion hydrophobicity. As seen in Figures 1G–J, Mpim I, Bmin OTf, Bmin BF4, and Bmim PF6, exhibit increasingly hydrophobic behavior as the anion varies from I, through OTf − and BF4− to PF6− (Huddleston et al., 2001; Yee et al., 2013). None of these bulky or increasingly hydrophobic anions appeared to have any significant effect on the biomass crystallinity. In fact, Bmim BF4 and Bmim PF6 appear to be even more crystalline than the original biomass.
These observations have demonstrated that hydrophilic ILs exhibit better capability for dissolving biomass than hydrophobic ones, when altering either the cation or anion. Hydrogen bond basicity also appears to have a significant impact on IL effectiveness. Clear trends emerge when comparing the hydrogen-bond basicity (seen in Table 1) across the suite of ILs tested. The hydrogen bond basicity of Emim Ac is 0.95, which is slightly higher than Emim Cl (0.87) and much higher than Bmim PF6 (0.41), which is in the lowest part of range of ILs applied in this study and made material that appeared very crystalline. It is clear that the structure and components of the IL plays an important role in biomass dissolution by modulating the hydrophobicity/philicity and hydrogen-bond basicity of the IL.
The cellulose crystallinity, shown in the XRD spectrum of Figure 2, supports the visible changes seen with the SEM images. The XRD spectrum are color coded to denote the different aspects of the ILs in terms of altering the cation chain length or the anion hydrophobicity. The raw biomass spectrum can be seen in black while the most effective IL (with a short functional groups and hydrophilic anion, Emim Ac) can be seen in red. The ILs representing increasing cation hydrophobicity, and a constant Cl− anion, can be seen in blue progressing from Emim Cl, through Bmim Cl and Amim Cl to Bdim Cl.) The least effective ILs for biomass dissolution contained longer functional groups and hydrophobic anions. This can be seen in the green hued XRD spectra for Mpim I, Bmim OTf, Bmim BF4, and Bmim PF6. These XRD spectrum confirm that hydrophilic ILs with higher hydrogen bond basicity are more efficient in dissolving biomass than hydrophobic ILs.
Quantitative values for cellulose crystallinity for pine treated with nine different ionic liquids can be seen in Table 2. These values were calculated based on the method outlined by Segal et al. (1959). This analysis is based on evaluating the ratio of peak heights between the cellulose 002 peak (~22°) and the amorphous cellulose minimum (~18°). This method is useful for comparing differences between samples and is the most frequently used throughout the literature (at least in part due to the simplicity of use). However, this method has three shortcomings in its quantitative analysis as outlined by Park et al. (2010). These shortcoming include: (1) underestimation of the amorphous peak height (which leads to an overestimation of the CI), (2) contributions from crystalline cellulose, other than the 002 peak, are not accounted for, and (3) variation in peak width, which is also impacted by cellulose crystallinity, for the 002 peak is neglected. In terms of this work the Segal method can be used to compare differences by caution should be used when evaluating small differences in CI as they relate to enzymatic hydrolysis. The CI values in Table 2 show that crystallinity is reduced for the Cl− anion ionic liquids and generally increased for the hydrophobic ionic liquids except, interestingly, for treatment with Bmim PF6 where it appears that there was a slight decrease in crystallinity. However, it should be noted that a crystallinity index for the Emim Ac, Emim Cl, and Bmim Cl could not be effectively calculated because the amorphous region has been convoluted with the cellulose 002 peak. It should be noted that directly relating cellulose crystallinity to enzymatic hydrolysis yields can be difficult given that cellulose accessibility can also be influenced by lignin and hemicellulose content/distribution, porosity, and particle size.
After examining the results of the SEM images and XRD patterns three ILs were selected for further reactivity characterization using enzymatic hydrolysis. The ILs used included Emim Ac, Emim Cl, and BmimPF6. Emim Ac and Emim Cl were selected to represent hydrophilic ILs because they showed the greatest overall amount of reduction in cellulose crystallinity and Bmim PF6 was chosen as representative of hydrophobic ILs due to a longer functional groups, a hydrophobic anion, and the lowest hydrogen-bond basicity. The results from the enzymatic hydrolysis study can be seen in Figure 3. As expected, the untreated pine released very little sugar (about 14% of the theoretical maximum) and the pine treated with Emim Ac and Emim Cl released significant amounts of sugar (73 and 52% respectively). Interestingly, the pine treated with Bmim PF6 actually became more recalcitrant and showed a decreased sugar yield (9%).
Results from SEM, XRD and enzymatic hydrolysis show that the most effective ionic liquids for biomass dissolution has three attributes: (1) fewer functional groups (as seen with the ethyl instead of butyl groups on Emim Cl vs. Bmim Cl and only one methyl group instead of two on Bmim Cl vs. Bdim Cl), (2) polar protic anions (as seen with the acetate anion compared with the chlorine anion for Emim Ac vs. Emim Cl, and (3) hydrophilic anions (as seen with the acetate and chlorine anions compared with BF4, PF6, and to a lesser degree the OTf). These characteristics are in accordance with other studies where ILs that have relatively small cations (Zavrel et al., 2009; Mäki-Arvela et al., 2010) and small hydrogen-bond acceptor anions, (Zhang et al., 2005) are often efficient in dissolving cellulose. After initial screening tests two ILs were selected for further study: Emim Ac because it was highly effective for biomass dissolution, and Bmim PF6 because of its' unique hydrophobic properties and to see if the decrease in sugar yields during enzymatic hydrolysis is consistent across many biomass types.
The screened ILs, EminAc and BminPF6, were used to pretreat seven biomass samples including corn stover, miscanthus, pine, sorghum, sugar cane bagasse, switchgrass, and wheat straw. These samples were examined by SEM, XRD, compositional analysis, and enzymatic hydrolysis in order to elucidate the different effect of hydrophilic and hydrophobic IL on various biomass types. The results from the IL pretreatment of the seven biomass types with Emim Ac and BmimPF6 showed the same general trends as the pine sample. The SEM images for all of the IL treated biomass are shown in Figure 4 where the raw biomass is in the left hand column, biomass treated with Emim Ac is in the middle column, and biomass treated with BmimPF6 is in the right hand column. Untreated biomass shows a high degree of filamentous structure while Emim Ac treated biomass, for the most part, has a much more amorphous structure. Treatment with BmimPF6 appears to make the material more crystalline for all biomass types tested in this study.
Figure 4. SEM images of biomass that is untreated (left), treated with Emim Ac (middle), or treated with Bmim PF6 (right). Biomass types are corn stover (A), miscanthus (B), pine (C), sorghum (D), sugar cane bagasse (E), switchgrass (F), and wheat straw (G). The scale bar in each image is 50 μm.
XRD spectrums for all untreated biomass, biomass pretreated with Emim Ac, and biomass pretreated with BmimPF6 are presented in Figures 5A–C, respectively. Untreated biomass clearly exhibits a cellulose I peak in the 002 crystalline plane at 2Θ = 22.5°. Treatment with Emim Ac clearly shifts the main peak to 20.7° as cellulose I is transformed to cellulose II.(Sun et al., 2009) Interestingly, reacting higher ash biomass samples (lodgepole pine is low in ash) with BmimPF6 adds several new peaks to the XRD spectrum as seen in Figure 5C. These peaks are likely the result of the hexafluorophosphate anion complexing with ash species (like sodium and potassium; Huber et al., 1997) in the biomass samples (Wang et al., 2017) which eliminates the recovery of the ionic liquid for recycle and would severely limit the use of these types of IL anions for biomass pretreatment.
Figure 5. XRD of several biomass types before reaction (A) and after reaction with Emim Ac (B) or Bmim PF6 (C).
Crystallinity index data for the herbaceous biomass can be seen in Table 3. As expected, the cellulose crystallinity for material treated with Emim Ac could not be calculated due to a complete removal of the amorphous cellulose minimum so this data was not added to the table. Interestingly, the crystallinity as measured by the method of Segal et al. generally decreased for the Bmim PF6 samples. This indicates that an alternative method of measuring cellulose crystallinity would be useful in more carefully evaluating overall cellulose accessibility. Other potential methods include XRD peak deconvolution, and XRD based amorphous peak subtraction, and an NMR C4 peak subtraction method as outlined by Park et al. (2010).
Table 3. Crystallinity Index of herbaceous biomass types that are either untreated, or treated with Bmim PF6.
The compositional analysis for the raw biomass, and the biomass after pretreatment with IL can be seen in Table 4. The changes in composition for the various biomass types can be generalized into two different groups. The first group comprises corn stover, miscanthus, sugarcane, switchgrass, and wheat straw. Interestingly, the second group contains pine and sorghum. General changes within these two groups, from the untreated case, is discussed for each type of ionic liquid.
Table 4. Compositional analysis of several raw and IL treated biomasses where the abbreviations are as follows: Cnst, corn stover; Misc, miscanthus; Pine, lodgepole pine; Srgm, sorghum; SugCn, sugarcane bagasse; Swgr, switchgrass; Wht Str, wheat straw.
After treatment with BmimPF6 the first group of biomass showed an average decrease in glucan content of 31% with a standard deviation of 12%. Xylan content showed the same trend as glucan, but to a greater degree, and decreased by an average of 87 ± 12%. Given the large decreases in sugars after treatment with BmimPF6 it can be expected that the lignin content should increase, and in fact it does increase by an average of 108%. Treatment with Emim Ac produced results that were opposite from the BmimPF6. In this case the lignin decreased by an average of 43 ± 15% while the glucan and xylan increased by 19.0 ± 15 and 39 ± 16% respectively. These compositional changes are generally in line with what previous literature has seen for treatment with Emim Ac (Brandt et al., 2013).
For the second group of biomass types (pine and sorghum) the Emim Ac and Bmim PF6 showed similar trends. Both of these materials saw an increase (or very little change) in glucan, xylan, and lignin content that corresponds well with the loss of the extractive from the untreated biomass (which is often around 10% for sorghum and 5% for pine) (Inl, 2015). Sorghum has rarely been investigated in the literature and appears to be resistant to dissolution by Bmim Cl (though the authors of that study also saw an increase in lignin content) (Zhang et al., 2011). While somewhat speculative, it is possible that the recalcitrance of sorghum is related to high amounts of p-coumaric and ferulic acids associated with the cell walls creating cross linkages between the lignin and hemicellulose (Billa et al., 1997). The p-coumaric acid would contribute to the formation of the p-hydroxyphenyl (H) lignol and provide recalcitrance in much the same way that the high guaiacyl (G) to syringyl (S) ratio causes recalcitrance in softwoods (Brandt et al., 2013).
While glucan, xylan, and lignin are important components of biomass structure another influential, but lower wt%, component is ash content. It can be seen in Table 4 that the ash content of the biomass increased drastically for the majority of the samples treated with Bmim PF6. This increase in ash is concomitant with the new peaks appearing in the XRD data in Figure 5. These peaks are likely due to the fact that hydrophobic ILs exhibit a strong metal-complexing ability (Mehdi et al., 2010; Wang et al., 2017) which could lead to the hexafluorophosphate anion binding with the ash present in the biomass, resulting in an increased ash content by making complexes like potassium hexafluorophosphate (Huber et al., 1997). However, while this metal complexation is a detriment to biomass processing the effect could have useful applications in other areas, like the recovery of rare earth elements (Wang et al., 2017).
Overall it can be seen that for less recalcitrant biomass treatment with Emim Ac generally delignifies biomass and increases sugar content while treatment with BmimPF6 decreases sugars and increases lignin content. It has also been shown that sorghum is recalcitrant in much the same way as pine for treatment with both hydrophilic and hydrophobic ionic liquids. It was also shown that treatment with an IL that contains a metal complexing anion like PF6 will increase overall ash content by binding with ash species and eliminating the possibility of recovering the IL.
Past research has shown that biomass structure and cellulose crystallinity play an important role in the effectiveness of enzymatic saccharification, where material with a less ordered structure often has a higher sugar release (Li et al., 2010). This is because cellulose crystallinity, lignin content, and hemicellulose-lignin linkages all effect cell wall structure and altering these facets permits enzymatic access for hydrolysis. To investigate the effects of how much the altered structure of the biomass in this study would alter sugar release we performed enzymatic hydrolysis. This enzymatic hydrolysis was also compared to that of untreated biomass and a standard dilute acid pretreatment to assess the overall effectiveness of ionic liquid pretreatment. The enzymatic hydrolysis data for untreated, dilute acid treated, Bmim PF6 treated, and Emim Ac treated biomass can be seen in Figures 6A–D, respectively. Two points should be noted in this figure: (1) that the legend visible in panel C applies to all of the panels in the figure, and (2) that the theoretical maximum for the sugar yield is the sum of release glucan, xylan, and cellobiose based on the composition data of the pretreated material. If the composition of the untreated material was used in all cases (assuming that the act of pretreating the material reduces the accuracy of the compositional analysis) then the data presented in Figure 6 would change in the following ways: (1) the dilute acid pretreatment data would essentially remain the same, (2) the Bmim PF6 data would decrease in yield by about 50%, and (3) the Emim Ac data would increase in overall yield by about 20%.
Figure 6. Enzymatic hydrolysis data points (markers) and trends (lines) for raw biomass (A), dilute acid pretreated biomass (B), Bmin PF6 pretreated biomass (C), and Emin Ac pretreated biomass (D). The legend visible in (C) applies to all of the panels in the figure. Theoretical sugar yield is the sum of glucan, xylan, galactose, arabinose, mannose, and cellobiose released divided by the sugars in the pretreated material.
The data in Figure 6A indicates that the ability to release sugars from untreated biomass using enzymatic hydrolysis varies widely by biomass type. However, straight EH was more effective for corn stover and sorghum while being almost completely ineffective for lodgepole pine, miscanthus, and switchgrass. Dilute acid pretreatment on the other hand (panel B) is very consistent for herbaceous materials (including sorghum), yielding about 70% of the available sugars, while being almost completely ineffective for woody feedstocks like pine. Treatment with the hydrophobic ionic liquid Bmim PF6 (Figure 6C) actually reduces the amount the amount of sugar recovered from the biomass. This is particularly interesting given the fact that the cellulose crystallinity, as determined by the XRD spectrum, showed a reduction in cellulose crystallinity. Traditionally, a decrease in crystallinity is thought to be correlated with an increase in hydrolytic sugar release. However, this result contributes to the evidence that this trend does not always hold true (Park et al., 2010). It is possible that while the cellulose crystallinity has been disrupted the increase in apparent lignin content, as measured by the composition in Table 4, contributes to the resistance to enzymatic hydrolysis. Biomass pretreatment with Emim Ac (Figure 6D) shows significant potential across all biomass types (although the treatment seems to be highly variable compared with dilute acid). Perhaps most favorable aspect of IL pretreatment is the rapid sugar release kinetics. While the biomass treated with dilute acid continues to release sugar over the course of 3 days the Emim Ac treated material has reached the full sugar release in only 1 day (Figure 6). Shorter reaction time could reduce the size of the equipment needed which would improve mixing and heat transfer issues often associated with biomass processing. It is also worth noting that the Emim Ac treated biomass also had more sugars available, based on the compositional analysis, so the fact that it reached such high theoretical yields, in such a short time, is even more impressive. Overall, it appears that using IL as a pretreatment instead of dilute acid provides significant improvements in sugar release kinetics and modest improvements in sugar yield.
Pretreatment of pine, with a wide array of ionic liquids that contained varying cation and anion hydrophobicity, confirmed that hydrophilic ILs with polar protic anions work well for biomass deconstruction. The hydrophilic IL Emim Ac increases sugar content and decreases lignin content while the hydrophobic IL Bmim PF6 decreases sugar content and increases lignin content for most herbaceous feedstocks. Interestingly, sorghum behaves similar to pine in terms of recalcitrance to IL pretreatment with both feedstocks not undergoing a large shift in the sugar content to lignin ratio. For all biomass types, treatment with Bmim PF6 showed metal complexation with naturally occurring ash that increases the difficulty of IL recovery. In a three way comparison between hydrophilic IL, hydrophobic IL, and dilute acid pretreatment four conclusions can be drawn: (1) hydrophilic ILs exhibit sugar release rates that are three times faster than dilute acid, (2) total sugar release from hydrophilic IL treatment is highly variable, compared with dilute acid, but is better for recalcitrant feedstocks like pine, (3) hydrophilic ILs exhibit a slightly greater amount of cellulosic sugars release than dilute acid, and (4) hydrophobic ILs increase biomass recalcitrance which reduces sugar release.
CW idea conception, majority of the writing and data analysis, some experiments. CL and HH idea conception, some writing. JA data analysis, majority of the experiments. BT some experiments.
The US Government retains and the publisher, by accepting the article for publication, acknowledges that the US Government retains a nonexclusive, paid-up, irrevocable, worldwide license to publish or reproduce the published form of this manuscript, or allow others to do so, for US Government purposes. The authors have no relevant affiliations, or financial involvement, with any organization or entity with a financial interest in, or financial conflict with, the subject matter or materials discussed in the manuscript.
The views and opinions of the authors expressed herein do not necessarily state or reflect those of the United States Government or any agency thereof. Neither the United States Government nor any agency thereof, nor any of their employees, makes any warranty, expressed or implied, or assumes any legal liability or responsibility for the accuracy, completeness, or usefulness of any information, apparatus, product, or process disclosed, or represents that its use would not infringe privately owned rights.
The authors would like to thank Kastli Schaller and Eric Fillerup for running the enzymatic hydrolysis and compositional analysis experiments. This research was supported by the US Department of Energy under Department of Energy Idaho Operations Office Contract No. DE-AC07-05ID14517.
Alvira, P., Tomás-Pejó, E., Ballesteros, M., and Negro, M. J. (2010). Pretreatment technologies for an efficient bioethanol production process based on enzymatic hydrolysis: a review. Bioresour. Technol. 101, 4851–4861. doi: 10.1016/j.biortech.2009.11.093
Banerjee, G., Car, S., Liu, T., Williams, D. L., Meza, S. L., Walton, J. D., et al. (2012). Scale-up and integration of alkaline hydrogen peroxide pretreatment, enzymatic hydrolysis, and ethanolic fermentation. Biotechnol. Bioeng. 109, 922–931. doi: 10.1002/bit.24385
Billa, E., Koullas, D. P., Monties, B., and Koukios, E. G. (1997). Structure and composition of sweet sorghum stalk components. Indus. Crops Prod. 6, 297–302. doi: 10.1016/S0926-6690(97)00031-9
Brandt, A., Gräsvik, J., Hallett, J. P., and Welton, T. (2013). Deconstruction of lignocellulosic biomass with ionic liquids. Green Chem. 15, 550–583. doi: 10.1039/c2gc36364j
Brandt, A., Ray, M. J., To, T. Q., Leak, D. J., Murphy, R. J., and Welton, T. (2011). Ionic liquid pretreatment of lignocellulosic biomass with ionic liquid–water mixtures. Green Chem. 13, 2489–2499. doi: 10.1039/c1gc15374a
Cheng, G., Varanasi, P., Arora, R., Stavila, V., Simmons, B. A., Kent, M. S., et al. (2012). Impact of ionic liquid pretreatment conditions on cellulose crystalline structure using 1-ethyl-3-methylimidazolium acetate. J. Phys. Chem. B 116, 10049–10054. doi: 10.1021/jp304538v
Cláudio, A. F., Swift, L., Hallett, J. P., Welton, T., Coutinho, J. A., and Freire, M. G. (2014). Extended scale for the hydrogen-bond basicity of ionic liquids. Phys. Chem. Chem. Phys. 16, 6593–6601. doi: 10.1039/c3cp55285c
Dien, B. S., Jung, H.-J. G., Vogel, K. P., Casler, M. D., Lamb, J. F. S., Iten, L., et al. (2006). Chemical composition and response to dilute-acid pretreatment and enzymatic saccharification of alfalfa, reed canarygrass, and switchgrass. Biomass Bioenergy 30, 880–891. doi: 10.1016/j.biombioe.2006.02.004
Eranki, P. L., Bals, B. D., and Dale, B. E. (2011). Advanced regional biomass processing depots: a key to the logistical challenges of the cellulosic biofuel industry. Biofuels Bioproducts Biorefining 5, 621–630. doi: 10.1002/bbb.318
Floudas, C. A., Elia, J. A., and Baliban, R. C. (2012). Hybrid and single feedstock energy processes for liquid transportation fuels: a critical review. Comput. Chem. Eng. 41, 24–51. doi: 10.1016/j.compchemeng.2012.02.008
Gräsvik, J., Winestrand, S., Normark, M., Jönsson, L. J., and Mikkola, J. P. (2014). Evaluation of four ionic liquids for pretreatment of lignocellulosic biomass. BMC Biotechnol. 14:34. doi: 10.1186/1472-6750-14-34
Huber, P., Krummeck, H., Baller, J., Krüger, J. K., Knorr, K., and Haussühl, S. (1997). Phases and phase transitions of KPF6. Ferroelectrics 203, 211–219. doi: 10.1080/00150199708012847
Huddleston, J. G., Visser, A. E., Reichert, W. M., Willauer, H. D., Broker, G. A., and Rogers, R. D. (2001). Characterization and comparison of hydrophilic and hydrophobic room temperature ionic liquids incorporating the imidazolium cation. Green Chem. 3, 156–164. doi: 10.1039/b103275p
Inl (2015). DOE Biomass Feedstock Library [Online]. Available online at: bioenergy.inl.gov (Accessed October 3, 2015).
Jackson De Moraes Rocha, G., Martin, C., Soares, I. B., Souto Maior, A. M., Baudel, H. M., and Moraes De Abreu, C. A. (2011). Dilute mixed-acid pretreatment of sugarcane bagasse for ethanol production. Biomass Bioenergy 35, 663–670. doi: 10.1016/j.biombioe.2010.10.018
Johnson, J. M., Coleman, M. D., Gesch, R., Jaradat, A., Mitchell, R., Reicosky, D., et al. (2007). Biomass-bioenergy crops in the United States: A changing paradigm. Am. J. Plant. Sci. Biotechnol. 1, 1–28.
Larsson, S., Palmqvist, E., Hahn-Hägerdal, B., Tengborg, C., Stenberg, K., Zacchi, G., et al. (1999). The generation of fermentation inhibitors during dilute acid hydrolysis of softwood. Enzyme Microb. Technol. 24, 151–159. doi: 10.1016/S0141-0229(98)00101-X
Lee, S. H., Doherty, T. V., Linhardt, R. J., and Dordick, J. S. (2009). Ionic liquid-mediated selective extraction of lignin from wood leading to enhanced enzymatic cellulose hydrolysis. Biotechnol. Bioeng. 102, 1368–1376. doi: 10.1002/bit.22179
Li, C., Knierim, B., Manisseri, C., Arora, R., Scheller, H. V., Auer, M., et al. (2010). Comparison of dilute acid and ionic liquid pretreatment of switchgrass: biomass recalcitrance, delignification and enzymatic saccharification. Bioresour. Technol. 101, 4900–4906. doi: 10.1016/j.biortech.2009.10.066
Li, C., Wang, Q., and Zhao, Z. K. (2008). Acid in ionic liquid: an efficient system for hydrolysis of lignocellulose. Green Chem. 10, 177–182. doi: 10.1039/B711512A
Li, Q., He, Y. C., Xian, M., Jun, G., Xu, X., Yang, J. M., et al. (2009). Improving enzymatic hydrolysis of wheat straw using ionic liquid 1-ethyl-3-methyl imidazolium diethyl phosphate pretreatment. Bioresour. Technol. 100, 3570–3575. doi: 10.1016/j.biortech.2009.02.040
Lloyd, T. A., and Wyman, C. E. (2005). Combined sugar yields for dilute sulfuric acid pretreatment of corn stover followed by enzymatic hydrolysis of the remaining solids. Bioresour. Technol. 96, 1967–1977. doi: 10.1016/j.biortech.2005.01.011
Mäki-Arvela, P., Anugwom, I., Virtanen, P., Sjöholm, R., and Mikkola, J. P. (2010). Dissolution of lignocellulosic materials and its constituents using ionic liquids—A review. Ind. Crops Prod. 32, 175–201. doi: 10.1016/j.indcrop.2010.04.005
Mehdi, H., Binnemans, K., Van Hecke, K., Van Meervelt, L., and Nockemann, P. (2010). Hydrophobic ionic liquids with strongly coordinating anions. Chem. Commun. 46, 234–236. doi: 10.1039/B914977E
Miranowski, J. A. (2007). Biofuel incentives and the energy title of the 2007 farm bill. Ames Am. Enter. Inst. 1–30. Available online at: https://lib.dr.iastate.edu/econ_las_pubs/567
Mosier, N., Wyman, C., Dale, B., Elander, R., Lee, Y. Y., Holtzapple, M., et al. (2005). Features of promising technologies for pretreatment of lignocellulosic biomass. Bioresour. Technol. 96, 673–686. doi: 10.1016/j.biortech.2004.06.025
Park, S., Baker, J. O., Himmel, M. E., Parilla, P. A., and Johnson, D. K. (2010). Cellulose crystallinity index: measurement techniques and their impact on interpreting cellulase performance. Biotechnol. Biofuels 3:10. doi: 10.1186/1754-6834-3-10
Pedersen, M., and Meyer, A. S. (2010). Lignocellulose pretreatment severity – relating pH to biomatrix opening. N. Biotechnol. 27, 739–750. doi: 10.1016/j.nbt.2010.05.003
Perlack, R. D., Eaton, L. M., Turhollow, A. F. Jr, Langholtz, M. H., Brandt, C. C., Downing, M. E., et al. (2011). US Billion-Ton Update: Biomass Supply for a Bioenergy and Bioproducts Industry. Oak Ridge, TN: Oak Ridge National laboratory.
Qiu, Z., Aita, G. M., and Walker, M. S. (2012). Effect of ionic liquid pretreatment on the chemical composition, structure and enzymatic hydrolysis of energy cane bagasse. Bioresour. Technol. 117, 251–256. doi: 10.1016/j.biortech.2012.04.070
Saha, B. C., Iten, L. B., Cotta, M. A., and Wu, Y. V. (2005). Dilute acid pretreatment, enzymatic saccharification and fermentation of wheat straw to ethanol. Process Biochem. 40, 3693–3700. doi: 10.1016/j.procbio.2005.04.006
Segal, L., Creely, J. J., Martin, A. E. Jr., and Conrad, C. M. (1959). An Empirical method for estimating the degree of crystallinity of native cellulose using the x-ray diffractometer. Text. Res. J. 29, 786–794. doi: 10.1177/004051755902901003
Selig, M., Weiss, N., and Ji, Y. (2008). Enzymatic Saccharification of Lignocellulosic Biomass: Laboratory Analytical Procedure (LAP): Issue Date, 3/21/2008. National Renewable Energy Laboratory.
Shill, K., Padmanabhan, S., Xin, Q., Prausnitz, J. M., Clark, D. S., and Blanch, H. W. (2011). Ionic liquid pretreatment of cellulosic biomass: enzymatic hydrolysis and ionic liquid recycle. Biotechnol. Bioeng. 108, 511–520. doi: 10.1002/bit.23014
Sluiter, A., Hames, B., Ruiz, R., Scarlata, C., Sluiter, J., Templeton, D., et al. (2008). Determination of Structural Carbohydrates and Lignin in Biomass. Laboratory analytical procedure.
Sørensen, A., Teller, P. J., Hilstrøm, T., and Ahring, B. K. (2008). Hydrolysis of miscanthus for bioethanol production using dilute acid presoaking combined with wet explosion pre-treatment and enzymatic treatment. Bioresour. Technol. 99, 6602–6607. doi: 10.1016/j.biortech.2007.09.091
Sun, N., Rahman, M., Qin, Y., Maxim, M. L., Rodríguez, H., and Rogers, R. D. (2009). Complete dissolution and partial delignification of wood in the ionic liquid 1-ethyl-3-methylimidazolium acetate. Green Chem. 11, 646–655. doi: 10.1039/b822702k
Trust, C. E. (2017). Clean Jobs Midwest [Online]. Available online at: http://www.cleanjobsmidwest.com/ (Accessed September 1, 2017).
Wang, K., Adidharma, H., Radosz, M., Wan, P., Xu, X., Russell, C. K., et al. (2017). Recovery of rare earth elements with ionic liquids. Green Chem. 19, 4469–4493. doi: 10.1039/C7GC02141K
Williams, C. L., Chang, C.-C., Do, P., Nikbin, N., Caratzoulas, S., Vlachos, D. G., et al. (2012). Cycloaddition of biomass-derived furans for catalytic production of Renewablep-Xylene. ACS Catal. 2, 935–939. doi: 10.1021/cs300011a
Williams, C. L., Emerson, R. M., and Tumuluru, J. S. (2017).“Biomass volume estimation and valorization for energy” in Biomass Compositional Analysis for Conversion to Renewable Fuels and Chemicals, ed J. S. Tumuluru (Rijeka: InTech), 251–270.
Williams, C. L., Westover, T. L., Emerson, R. M., Tumuluru, J. S., and Li, C. (2016). Sources of biomass feedstock variability and the potential impact on biofuels production. Bioenergy Res. 9, 1–14. doi: 10.1007/s12155-015-9694-y
Wolfrum, E. J., Ness, R. M., Nagle, N. J., Peterson, D. J., and Scarlata, C. J. (2013). A laboratory-scale pretreatment and hydrolysis assay for determination of reactivity in cellulosic biomass feedstocks. Biotechnol. Biofuels 6:162. doi: 10.1186/1754-6834-6-162
Yee, P., Shah, J. K., and Maginn, E. J. (2013). State of hydrophobic and hydrophilic ionic liquids in aqueous solutions: are the ions fully dissociated? J. Phys. Chem. B 117, 12556–12566. doi: 10.1021/jp405341m
Yoshida, M., Liu, Y., Uchida, S., Kawarada, K., Ukagami, Y., Ichinose, H., et al. (2008). Effects of cellulose crystallinity, hemicellulose, and lignin on the enzymatic hydrolysis of Miscanthus sinensis to monosaccharides. Biosci. Biotechnol. Biochem. 72, 805–810. doi: 10.1271/bbb.70689
Zavrel, M., Bross, D., Funke, M., Büchs, J., and Spiess, A. C. (2009). High-throughput screening for ionic liquids dissolving (ligno-)cellulose. Bioresour. Technol. 100, 2580–2587. doi: 10.1016/j.biortech.2008.11.052
Zhang, H., Wu, J., Zhang, J., and He, J. (2005). 1-Allyl-3-methylimidazolium chloride room temperature ionic liquid: a new and powerful nonderivatizing solvent for cellulose. Macromolecules 38, 8272–8277. doi: 10.1021/ma0505676
Zhang, J., Ma, X., Yu, J., Zhang, X., and Tan, T. (2011). The effects of four different pretreatments on enzymatic hydrolysis of sweet sorghum bagasse. Bioresour. Technol. 102, 4585–4589. doi: 10.1016/j.biortech.2010.12.093
Keywords: ionic liquid, hydrophilic, hydrophobic, dilute acid, biomass pretreatment, herbaceous, woody
Citation: Williams CL, Li C, Hu H, Allen JC and Thomas BJ (2018) Three Way Comparison of Hydrophilic Ionic Liquid, Hydrophobic Ionic Liquid, and Dilute Acid for the Pretreatment of Herbaceous and Woody Biomass. Front. Energy Res. 6:67. doi: 10.3389/fenrg.2018.00067
Received: 15 March 2018; Accepted: 20 June 2018;
Published: 09 July 2018.
Edited by:
Timothy G. Rials, University of Tennessee, Knoxville, United StatesReviewed by:
Shishir P. S. Chundawat, Rutgers University, The State University of New Jersey, United StatesCopyright © 2018 Williams, Li, Hu, Allen and Thomas. This is an open-access article distributed under the terms of the Creative Commons Attribution License (CC BY). The use, distribution or reproduction in other forums is permitted, provided the original author(s) and the copyright owner(s) are credited and that the original publication in this journal is cited, in accordance with accepted academic practice. No use, distribution or reproduction is permitted which does not comply with these terms.
*Correspondence: C. Luke Williams, bHVrZS53aWxsaWFtc0BpbmwuZ292
Disclaimer: All claims expressed in this article are solely those of the authors and do not necessarily represent those of their affiliated organizations, or those of the publisher, the editors and the reviewers. Any product that may be evaluated in this article or claim that may be made by its manufacturer is not guaranteed or endorsed by the publisher.
Research integrity at Frontiers
Learn more about the work of our research integrity team to safeguard the quality of each article we publish.