- 1Department of Surgery and Emory Critical Care Center, Emory University School of Medicine, Atlanta, GA, United States
- 2Department of Emergency, Critical Care and Disaster Medicine, Faculty of Medicine, Dentistry and Pharmaceutical Sciences, Okayama University, Okayama, Japan
Sepsis is a global health challenge marked by limited clinical options and high mortality rates. AMP-activated protein kinase (AMPK) is a cellular energy sensor that mediates multiple crucial metabolic pathways that may be an attractive therapeutic target in sepsis. Pre-clinical experimental studies have demonstrated that pharmacological activation of AMPK can offer multiple potential benefits during sepsis, including anti-inflammatory effects, induction of autophagy, promotion of mitochondrial biogenesis, enhanced phagocytosis, antimicrobial properties, and regulation of tight junction assembly. This review aims to discuss the existing evidence supporting the therapeutic potential of AMPK activation in sepsis management.
Introduction
Sepsis represents a significant global health challenge, impacting nearly 50 million people and accounting for approximately 20% of all deaths worldwide (1). Despite advances in understanding its pathophysiology, septic shock often results in mortalities of 30% or higher. Management of sepsis is generally supportive. While fluids and antibiotics improve outcome, no pharmacologic therapy has been shown to effectively target the dysregulated host response seen in sepsis (2, 3). While numerous preclinical studies have shown promise in improving outcomes based upon sound mechanistic insights, effective translation of specific treatments for sepsis into clinical practice at the bedside has proven to be an elusive goal (4). Consequently, there is a clear need to develop targeted and effective therapeutics to augment current strategies in sepsis management.
AMP-activated protein kinase (AMPK) is a central integrator of cellular energy and metabolic homeostasis (5). Once activated, AMPK participates in triggering downstream effector proteins involved in a range of biological responses, from glucose metabolism and lipid oxidation to autophagy (6, 7). Although AMPK activation is best known for its role in long-term treatment of diabetes through drugs such as metformin, growing evidence suggests that AMPK activation can exert anti-inflammatory effects through several mechanisms. As such, AMPK is increasingly viewed as a potential therapeutic target for a number of human conditions, including cardiovascular diseases, ischemia-reperfusion injuries, and various inflammatory diseases. Numerous pharmacological agents have been identified that can activate AMPK, ranging from widely used medications to innovative substrates to natural compounds.
In critically ill patients, multiple meta-analyses have demonstrated an association between preadmission use of metformin, an indirect AMPK activator, and lower mortality in adult septic patients who have diabetes mellitus (8, 9). Further, a propensity score matched cohort of 2,691 septic ICU patients with type 2 diabetes mellitus demonstrated decreased 90 day mortality, reduced severe kidney injury and increased renal recovery in patients exposed to metformin during their hospitalization (10). Additionally, metformin exposure during Staphylococcus aureus bacteremia (regardless of prior use) was shown to be an independent predictor of survival in a study of 452 patients with diabetes (11). Understanding the limitations of associative studies and that causation should not be inferred from these studies, activation of AMPK may represent a therapeutic strategy for the treatment of sepsis that is worthy of further study. In fact, a protocol has recently been published for a planned randomized clinical trial examining the safety and feasibility of metformin as a treatment for sepsis-associated acute kidney injury (12). While metformin is well known as a treatment for diabetes mellitus, AMPK activation has numerous effects above and beyond this, and the full spectrum of effects of AMPK activation during sepsis remains to be fully elucidated. This review explores the current understanding of the role of AMPK in sepsis, emphasizing the therapeutic implications of AMPK activators.
Overview of AMPK
AMPK functions as an energy sensor that is activated in response to cellular energy depletion. Activation is triggered by increased AMP/ATP or ADP/ATP ratios during conditions such as starvation, hypoxia, ischemic stress, or exercise. Structurally, AMPK is a heterotrimeric complex comprised of three subunits: the catalytic α-subunit, the scaffolding β-subunit, and the regulatory γ-subunit. There are two isoforms of the α and β subunits, while the γ-subunit has three isoforms (13, 14). The α1, β1, and γ1 subunits are ubiquitously expressed, whereas other combinations display tissue-specific expression. For instance, the α2 and β2 subunits are predominantly found in heart and skeletal muscle (15). Primary physiological AMPK activation is achieved by phosphorylation at Thr172 of the AMPK α-subunit predominantly by upstream kinases including liver kinase B1 (LKB1) and calcium/calmodulin-dependent protein kinase kinase-beta (CaMKKβ) (5, 14). Activation of the LKB1/AMPK signaling pathway reduces lung vascular permeability and the systemic inflammatory response following lipopolysaccharide (LPS) treatment (16). Macrophage LKB1 also helps control local Klebsiella pneumoniae growth during pneumonia by maintaining adequate alveolar macrophages in the lung (17). In contrast, CaMKKβ activates AMPK via an increase in intracellular Ca2+ concentration, independent of the AMP/ATP or ADP/ATP ratios. The CaMKKβ/AMPK pathway is pivotal in providing protection against LPS-induced neuroinflammation and cerebral ischemia/reperfusion injury (18, 19). Activation of AMPK then influences a number of downstream pathways related to carbohydrate, amino acid, and lipid metabolism, as well as mitochondrial function, autophagy, and cell growth (14, 16).
AMPK activators
AMPK can be activated directly or indirectly by a number of different pharmacological compounds. 5-aminoimidazole-4-carboxamide ribonucleoside (AICAR) is commonly utilized as an experimental direct AMPK activator. AICAR is transformed intracellularly into AICAR monophosphate, which acts as an AMP mimetic, consequently activating AMPK. However, it is worth noting that AICAR also activates other AMP-regulated enzymes, such as fructose-1,6-bisphosphatase through which it could play a role in regulating glycolysis and gluconeogenesis unrelated to AMPK (13). Over the past decade, more specific direct small molecule AMPK activators have been developed. For instance, A-769662 specifically activates AMPK by directly binding to the AMPK β-subunit, resulting in allosteric activation. In contrast, metformin, a frequently prescribed medication for type 2 diabetes mellitus, along with other biguanides, indirectly activates AMPK by inhibiting the mitochondrial respiratory chain complex I, leading to an increase in AMP levels. Additionally, IM156 is a novel biguanide more potent than metformin in activating AMPK. Several natural products derived from plants, such as berberine from traditional Chinese medicine and the polyphenol resveratrol, have also been identified as AMPK activators (20, 21).
Kinetics of AMPK activation during sepsis
AMPK activation changes during sepsis differ depending on both tissue and species. AMPK activation is increased at 12 hours and declines at 24 hours following CLP compared with sham control in mouse liver (22). In contrast, AMPK activation is elevated at 6 hours in rat heart and remains elevated at 24 and 72 hours following CLP compared with sham control (23). A trend towards lower AMPK activation is observed in mouse lung 24 hours after CLP (24) while AMPK activation trends higher by 6 hours in mouse kidney after CLP, before returning to baseline (25). AMPK activation in diaphragm and tibialis anterior is also increased 48 and 96 hours after CLP in mice (26). In contrast, human biopsy data demonstrated that in vastus lateral muscle, AMPK activity is reduced in critically ill ICU patients (not restricted to sepsis), with an interquartile range of 4 to 6 days following admission (27).
Potential beneficial effects of AMPK activation in treating sepsis
Experimental studies (described in further detail below) have demonstrated that metformin can potentially mitigate sepsis-induced organ failure via AMPK activation (28) via a number of physiological benefits (Figure 1).
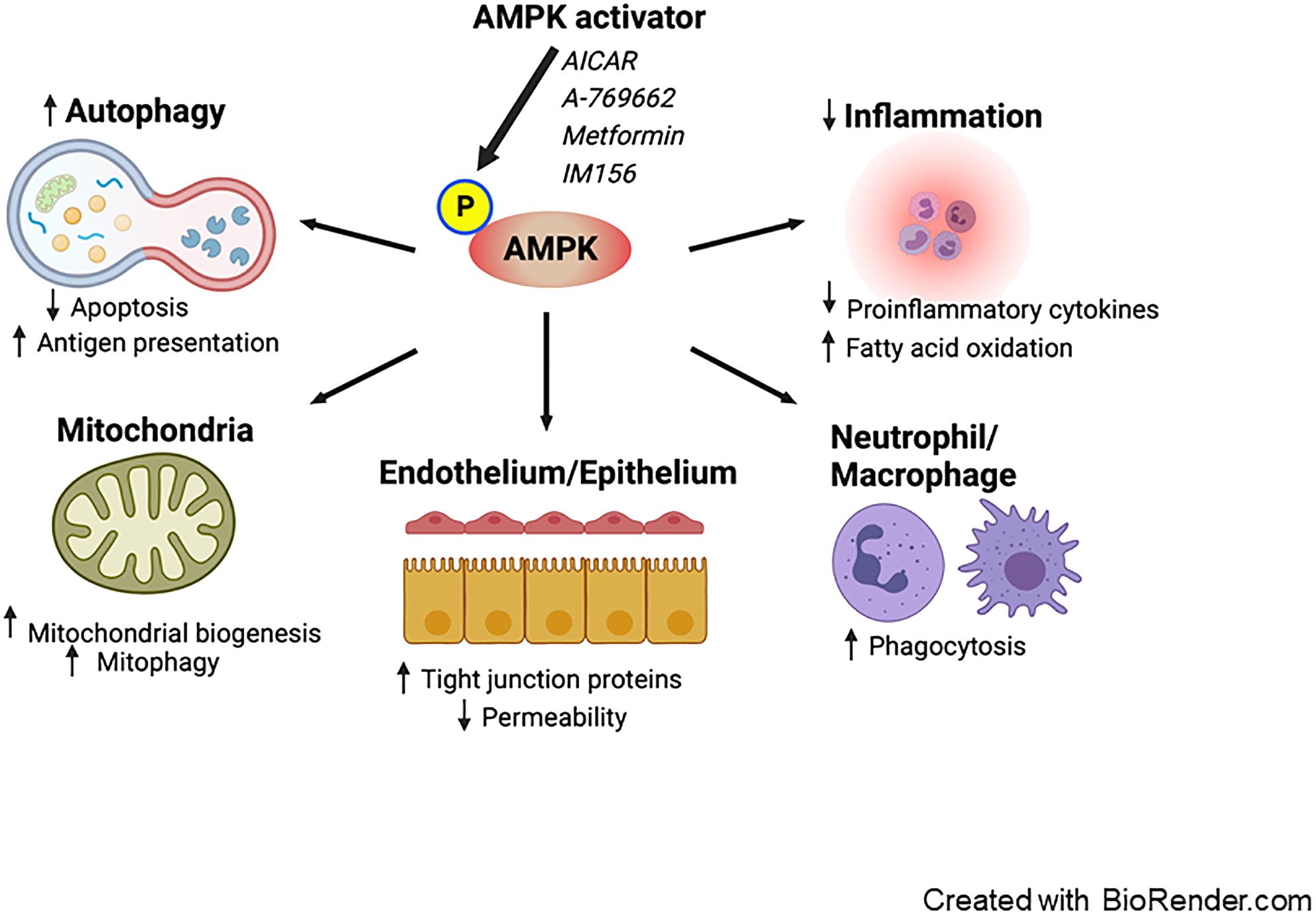
Figure 1. Proposed effects of AMPK activation on sepsis. Pharmacological activation of AMPK enhances autophagy, leading to a decrease in apoptosis and an increase in antigen presentation. AMPK activation promotes mitochondrial biogenesis and mitophagy. It exerts anti-inflammatory effects by reducing the production of pro-inflammatory cytokines and facilitating fatty acid oxidation. Furthermore, AMPK boosts the phagocytic activity of neutrophils and macrophages. Additionally, AMPK reduces vascular and intestinal permeability by restoring the integrity of tight junctions in the endothelium and epithelium. AMPK; AMP-activated protein kinase.
Anti-inflammatory effect
Sepsis is characterized as having dysregulated inflammation. While the balance between inflammation and immunosuppression is complex, modulating excessive inflammation – especially in the early stages of sepsis – and the resulting organ damage stands out as a potential therapeutic target (29). The role of AMPK in inflammation regulation has been extensively characterized. AMPK activation indirectly suppresses nuclear factor-κB, a pivotal transcriptional factor that induces the inflammatory response, through various mediators including SIRT1, FOXO, and PGC1α (30). Diminished AMPK activity in macrophages leads to a marked increase in the expression of pro-inflammatory cytokines IL-6 and TNF following LPS treatment with a simultaneous downregulation of the anti-inflammatory cytokine IL-10 (31). In contrast, AMPK activation causes a decrease in LPS-induced IL-6 and TNF production, coupled with a surge in IL-10 production. In addition, AMPK exhibits anti-inflammatory properties by modulating lipid metabolism. It is recognized that sepsis impacts fatty acid metabolism, with systemic inflammation and lipotoxicity stemming from reduced fatty acid oxidation and an accumulation of free fatty acids (32, 33). AMPK aids in enhancing fatty acid oxidation and mitigating excessive lipid accumulation via the phosphorylation of acetyl-CoA carboxylase (5).
Induction of autophagy
Autophagy is a dynamic process through which intracellular materials are degraded and subsequently recycled in lysosomes. In mouse cecal ligation and puncture (CLP), a model of polymicrobial intraabdominal sepsis, autophagy is accelerated 6 hours after the induction of sepsis, but this is followed by a decline in autophagy in the liver, heart, and spleen (34).
Some preclinical studies have indicated that autophagy offers protective effects against sepsis in multiple vital organs including lung, heart, kidneys and brain although it may be potentially harmful in skeletal muscle (35). The beneficial aspects of autophagy can be attributed, in part, to the preservation of mitochondrial integrity, the prevention of apoptosis, and the enhancement of MHC II antigen presentation (36). Interestingly, suppressing autophagy in the liver heightens mortality rates after experimental sepsis, primarily due to the induction of apoptosis and mitochondrial damage (37). Thus, autophagy modulation might be a promising therapeutic target for sepsis treatment. The Unc-51-like autophagy-activating kinase 1 (ULK1) protein kinase is pivotal in the initiation of autophagy. AMPK acts directly as an upstream signal facilitating ULK1 activation. Moreover, AMPK suppresses the mechanistic target of rapamycin (mTOR), another main regulator that exerts negative control over autophagy (38).
Mitochondrial homeostasis
Mitochondria are responsible for numerous physiological processes, including ATP generation through oxidative phosphorylation, reactive oxygen species (ROS) production, calcium homeostasis, and the initiation of apoptosis. Mitochondrial homeostasis hinges on two opposing yet harmoniously coordinated processes: mitochondrial biogenesis and mitochondrial selective autophagy (often referred to as mitophagy) (39). Mitochondrial biogenesis is the process through which mitochondria adapt by increasing both in number and size, ensuring their robust heath and functionality. In contrast, mitophagy is a form of specialized autophagy targeting the selective degradation of damaged mitochondria via lysosomes. Beyond general autophagy, ULK1 is believed to be integral to mitophagy under various conditions (6).
Sepsis frequently leads to mitochondrial dysfunction, which has been mechanistically linked to poor patient outcomes (40, 41). This dysfunction is mainly marked by disturbance in electron transport chain function, causing excessive ROS production. The surge in ROS is further exacerbated by pro-inflammatory cytokines, such as IL-1, IL-6, and TNF. This cascade eventually triggers apoptosis, induced by the release of cytochrome c and other pro-apoptotic proteins (42, 43). Emerging evidence highlights the protective roles of both mitochondrial biogenesis and mitophagy during sepsis. In response to mitochondrial dysfunction, AMPK activation serves as a key compensatory mechanism by stimulating both mitochondrial biogenesis and mitophagy, restoring function and reducing damage (41, 44–46). Notably A-769662, a potent AMPK activator, bolsters mitochondrial biogenesis, as evidenced by an increase in nuclear PGC-1α activity within the lungs (47). Additionally, pre-treatment with lycorine, a benzyl isoquinoline alkaloid, decreases mortality and mitigates cardiac injury following CLP, by activating AMPK and suppressing ROS production and oxidative stress (48). In contrast, inhibiting mitophagy in macrophages leads to enhanced survival following sepsis. This phenomenon is attributed to ROS production in mitochondria, facilitating bacterial clearance and host defense (49).
Role of AMPK in immune cells
AMPK has been demonstrated to play a crucial role in the immune response, particularly within myeloid and T cells. Activating AMPK with either metformin or AICAR leads to reduced bacterial loads in peritoneal lavage following peritonitis-induced sepsis, associated with the amplification of neutrophil chemotaxis, phagocytosis, and bacterial neutralization (50). Additionally, metformin-induced AMPK activation inhibits the proliferation of Listeria pneumophilia within macrophages through enhanced mitochondrial ROS production (51). Moreover, AMPK is an important regulator of high mobility group box 1 (a consequential late mediator in lethal sepsis) release within stimulated macrophages and monocytes (52). AMPK is also crucial for T cell-mediated immunity as mice with a T cell-specific deletion of AMPKα1 have reduced counts of CD4+ and CD8+ T cells within bronchoalveolar lavage and lung tissues following pulmonary infections caused by either Influenza A or Listeria monocytogenes (53). While viral load in the lung remains consistent in mice with T cell-specific AMPKα1 deletion following Influenza A, knockout mice exhibit elevated bacterial loads within the liver (with a trend towards an increase in the spleen) following Listeria monocytogenes.
Antimicrobial effect
AMPK plays a complex role in infectious diseases, especially viral infections, with its impact varying depending on the specific pathogen. AMPK activation also plays a key role in defending the host against Mycobacterium tuberculosis. This bacterium bypasses the host’s autophagic defense system in part by activating the mTOR pathway. AMPK therefore has been highlighted as a potential therapeutic target in the fight against mycobacterium infection (54). Within severe acute respiratory syndrome coronavirus 2 (SARS-CoV-2), AMPK activation leads to the phosphorylation and stabilization of angiotensin-converting enzyme 2 (ACE2) (55). While ACE2 facilitates SARS-CoV-2 entry into host cells, it also offers protection against lung injury by reducing inflammation, fibrosis, and pulmonary arterial hypertension (56). Metformin was extensively studied for its potential anti-COVID-19 effects. Based on lack of efficacy in the TOGETHER and COVID-OUT trials, metformin is not recommended for treatment of patients with COVID-19 in international guidelines; however, interest remains in identifying subsets of patients in whom it may be beneficial (57–60). IM156, a novel biguanide more potent than metformin in activating AMPK, offers protection following CLP by enhancing bacterial clearance, regulating cytokine release, and preventing lymphocyte apoptosis (61). IM156 also inhibits ROS generation and formation of neutrophil extracellular traps in response to LPS, so the mechanism underlying its survival benefit following sepsis is likely to be multifactorial.
Cytomegalovirus (CMV) reactivation is frequently seen in critically ill patients without primary immunodeficiency diseases (62). CMV relies on AMPK for glycolytic activation during its replication and suppressing AMPK activity can hinder CMV replication (63). Additionally, the effects of AMPK activation on bacterial infections vary based on the specific host cells infected and the nature of the pathogen. A comprehensive review of the impact of host AMPK activation on various microbial species has been conducted and can be found elsewhere (64).
Regulation of epithelial/endothelial tight junction assembly
AMPK activation exerts beneficial impacts on tight junction assembly. For instance, AMPK activation using metformin and AICAR has been shown to counteract airway epithelial barrier disruption caused by Pseudomonas aeruginosa, a significant microorganism linked to hospital-acquired infections in critically ill patients (65).
The intestinal epithelium serves as a dynamic protective barrier, separating the host from its external environment. This barrier’s integrity is regulated by both tight junction-dependent and independent mechanisms (66, 67). Gut barrier dysfunction characterized by intestinal hyperpermeability is associated with various diseases and systemic inflammation (68). AICAR bolsters ZO-1 formation, leading to a reduction in intestinal paracellular permeability following heat stress in rats (69). In a model of experimental colitis, metformin-induced AMPK activation upregulates the expression of occludin, ZO-1, and claudin-1, mitigating colonic damage (70). Current evidence suggests that sepsis impairs tight junctions in two different pathways (pore and leak), associated with intestinal hyperpermeability and unfavorable outcomes (67, 71). To our knowledge, there have been no studies investigating the impact of AMPK activation on intestinal barrier function in sepsis.
Disruption of endothelial tight junction proteins also worsens microvascular permeability and exacerbates multiple organ dysfunction (72). A number of investigations have centered on the interplay between AMPK activity and endothelial permeability in the context of LPS stimulation. AMPKα1-deficient mice display elevated cardiac vascular permeability, reduced endothelial ZO-1 expression, and myocardial edema post-LPS exposure (73). Conversely, in wild-type mice, these adverse effects are counteracted by AICAR. Additionally, both metformin and AICAR are effective in alleviating LPS-induced pulmonary endothelial permeability in rats (74).
AMPK activation as a therapeutic target in sepsis
Regardless of the degree of AMPK activation in tissues following sepsis, a large number of pre-clinical studies have demonstrated beneficial effects of AMPK activation following either CLP-induced sepsis or sterile inflammation in LPS-induced endotoxemia. Table 1 summarizes the effects of AMPK activators or drugs/agents that can positively mediate AMPK pathway in animal studies (22, 23, 25, 47, 61, 74–104). Notably, a single dose of metformin administered 1 hour after CLP improves survival associated with preservation of metabolic fitness (25). Additionally, Dexmedetomidine, a sedative commonly used for critically ill patients, enhances hepatic autophagy through AMPK activation and leads to improved survival compared to vehicle in mice subjected to CLP (22).
Drawbacks of AMPK activation
While a substantial body of evidence highlights the beneficial effects of AMPK activation, data also suggest that inhibiting AMPK can reduce liver injury. The AMPK inhibitor compound C reduces hepatocyte apoptosis and mitigates liver damage by inhibiting the pro-apoptotic protein JNK in a model of LPS/D-galactosamine-induced fulminant hepatitis (105). Additionally, inhibiting AMPK improves LPS-induced liver damage by suppressing the ROS/NF-κB signaling pathway (106). The role of AMPK in myocardial ischemic injury remains debated, with conflicting views on whether it is beneficial or harmful (107). Furthermore, in certain tumor types or under conditions of limited nutrients or hypoxia, AMPK has been observed to promote tumor growth (13).
Discussion
Although AMPK activation shows promise in the treatment of sepsis, several barriers remain prior to translation to bedside use above and beyond the absence of clinical trials supporting the efficacy of this approach. First, sepsis is a highly heterogeneous condition characterized by the presence of multiple suphenotypes, each with distinct pathophysiological mechanisms and clinical outcomes (108–116). Additionally, subtype strategies using clinical, biomarker and transcriptomic data do not identify comparable patient populations with sepsis (117), suggesting precision medicine approaches to identifying which patients might respond to AMPK-targeted therapies would be challenging. Next, the diversity of cell types involved in the septic response further complicates the potential usage of AMPK activation strategies due to potential of unwanted off-target effects. Additionally, immunometabolic paralysis – a state of metabolic dysfunction in immune cells – is another potential barrier to effective sepsis treatment with AMPK activation. While AMPK is a central regulator of cellular metabolism, its role in reversing immunometabolic paralysis in sepsis is not yet fully understood. Emerging evidence suggests that AMPK activation may influence immune cell function, but the effects appear to be context-dependent, requiring more precise targeting strategies. Future research should focus on elucidating the specific pathways through which AMPK modulates immune metabolism during sepsis and developing biomarkers to guide the use of AMPK-targeted therapies in overcoming immunometabolic paralysis (44).
Recent studies have identified itaconate, a metabolite derived from the TCA cycle, as a significant modulator of immune responses, contributing to disease tolerance in sepsis (118). Itaconate is mainly produced by activated macrophages through the enzyme immune-responsive gene 1 (IRG1) and inhibits ferroptosis of macrophages via Nrf2 pathways in sepsis-induced acute lung injury (119). As a central regulator of cellular energy homeostasis, AMPK interacts with multiple metabolic pathways, including those linked to the TCA cycle. This interaction suggests that AMPK and itaconate may work together to modulate immune responses and metabolic reprogramming in sepsis given the role of mitochondrial TCA cycle metabolites in physiology and disease (120). Additionally, AMPK may influence itaconate production by regulating IRG1 expression, as AMPK can modulate inflammatory responses through altering macrophage polarization (121). Given that itaconate has been demonstrated to regulate AMPK signaling in hepatocytes (122, 123), this suggests a complementary approach to direct AMPK activation in modulating metabolic and inflammatory responses in sepsis.
Ultimately, AMPK plays a crucial role in numerous biological processes that are relevant to sepsis pathogenesis, and numerous studies suggest that either AMPK activation or deficiency can influence susceptibility to sepsis in ways that vary by age or sex (124, 125). It is exciting to consider the possibility of AMPK as a potential therapeutic target in human sepsis given the numerous pre-clinical studies demonstrating a benefit of AMPK activation and associative studies suggesting a potential benefit of metformin in critical illness. However, there is currently insufficient human evidence to support using metformin or other AMPK activators to treat septic patients in the ICU. Ongoing and future clinical trials may clarify the role (if any) in activating AMPK in human sepsis while future research focused on tissue-specific and condition-specific AMPK activators will hopefully clarify mechanisms underlying potential efficacy.
Author contributions
TY: Writing – original draft, Writing – review & editing. CC: Writing – original draft, Writing – review & editing.
Funding
The author(s) declare that financial support was received for the research, authorship, and/or publication of this article. This work was supported by the National Institutes of Health (GM148217).
Conflict of interest
The authors declare that the research was conducted in the absence of any commercial or financial relationships that could be construed as a potential conflict of interest.
Generative AI statement
The authors declare that no Generative AI was used in the creation of this manuscript.
Publisher’s note
All claims expressed in this article are solely those of the authors and do not necessarily represent those of their affiliated organizations, or those of the publisher, the editors and the reviewers. Any product that may be evaluated in this article, or claim that may be made by its manufacturer, is not guaranteed or endorsed by the publisher.
References
1. Rudd KE, Johnson SC, Agesa KM, Shackelford KA, Tsoi D, Kievlan DR, et al. Global, regional, and national sepsis incidence and mortality, 1990-2017: analysis for the Global Burden of Disease Study. Lancet. (2020) 395:200–11. doi: 10.1016/S0140-6736(19)32989-7
2. Evans L, Rhodes A, Alhazzani W, Antonelli M, Coopersmith CM, French C, et al. Surviving sepsis campaign: international guidelines for management of sepsis and septic shock 2021. Crit Care Med. (2021) 49:e1063–e143. doi: 10.1097CCM.0000000000005337
3. Singer M, Deutschman CS, Seymour CW, Shankar-Hari M, Annane D, Bauer M, et al. The third international consensus definitions for sepsis and septic shock (Sepsis-3). JAMA. (2016) 315:801–10. doi: 10.1001/jama.2016.0287
4. De Backer D, Deutschman CS, Hellman J, Myatra SN, Ostermann M, Prescott HC, et al. Surviving sepsis campaign research priorities 2023. Crit Care Med. (2024) 52:268–96. doi: 10.1097/CCM.0000000000006135
5. Steinberg GR, Carling D. AMP-activated protein kinase: the current landscape for drug development. Nat Rev Drug Discovery. (2019) 18:527–51. doi: 10.1038/s41573-019-0019-2
6. Herzig S, Shaw RJ. AMPK: guardian of metabolism and mitochondrial homeostasis. Nat Rev Mol Cell Biol. (2018) 19:121–35. doi: 10.1038/nrm.2017.95
7. Sharma A, Anand SK, Singh N, Dwivedi UN, Kakkar P. AMP-activated protein kinase: An energy sensor and survival mechanism in the reinstatement of metabolic homeostasis. Exp Cell Res. (2023) 428:113614. doi: 10.1016/j.yexcr.2023.113614
8. Liang H, Ding X, Li L, Wang T, Kan Q, Wang L, et al. Association of preadmission metformin use and mortality in patients with sepsis and diabetes mellitus: a systematic review and meta-analysis of cohort studies. Crit Care. (2019) 23:50. doi: 10.1186/s13054-019-2346-4
9. Li Y, Zhao H, Guo Y, Duan Y, Guo Y, Ding X. Association of preadmission metformin use and prognosis in patients with sepsis and diabetes mellitus: A systematic review and meta-analysis. Front Endocrinol (Lausanne). (2021) 12:811776. doi: 10.3389/fendo.2021.811776
10. Gómez H, Del Rio-Pertuz G, Priyanka P, Manrique-Caballero CL, Chang CH, Wang S, et al. Association of metformin use during hospitalization and mortality in critically ill adults with type 2 diabetes mellitus and sepsis. Crit Care Med. (2022) 50:935–44. doi: 10.1097/CCM.0000000000005468
11. Lee JY, Kim ES, Chang E, Bae S, Jung J, Kim MJ, et al. Clinical impact of metformin exposure during Staphylococcus aureus bacteremia in patients with diabetes mellitus. Eur J Clin Microbiol Infect Dis. (2023) 42:1439–47. doi: 10.1007/s10096-023-04679-6
12. Saraiva IE, Hamahata N, Huang DT, Kane-Gill SL, Rivosecchi RM, Shiva S, et al. Metformin for sepsis-associated AKI: a protocol for the Randomized Clinical Trial of the Safety and FeasibiLity of Metformin as a Treatment for sepsis-associated AKI (LiMiT AKI). BMJ Open. (2024) 14:e081120. doi: 10.1136/bmjopen-2023-081120
13. Kim J, Yang G, Kim Y, Kim J, Ha J. AMPK activators: mechanisms of action and physiological activities. Exp Mol Med. (2016) 48:e224. doi: 10.1038/emm.2016.16
14. Steinberg GR, Hardie DG. New insights into activation and function of the AMPK. Nat Rev Mol Cell Biol. (2023) 24:255–72. doi: 10.1038/s41580-022-00547-x
15. Garcia D, Shaw RJ. AMPK: mechanisms of cellular energy sensing and restoration of metabolic balance. Mol Cell. (2017) 66:789–800. doi: 10.1016/j.molcel.2017.05.032
16. Yang Y, Dong R, Hu D, Chen Z, Fu M, Wang DW, et al. Liver kinase B1/AMP-activated protein kinase pathway activation attenuated the progression of endotoxemia in the diabetic mice. Cell Physiol Biochem. (2017) 42:761–79. doi: 10.1159/000478068
17. Otto NA, de Vos AF, van Heijst JWJ, Roelofs J, van der Poll T. Association of myeloid liver kinase B1 depletion with a reduction in alveolar macrophage numbers and an impaired host defense during gram-negative pneumonia. J Infect diseases. (2022) 225:1284–95. doi: 10.1093/infdis/jiaa416
18. Li C, Zhang C, Zhou H, Feng Y, Tang F, Hoi MPM, et al. Inhibitory effects of betulinic acid on LPS-induced neuroinflammation involve M2 microglial polarization via caMKKβ-dependent AMPK activation. Front Mol Neurosci. (2018) 11:98. doi: 10.3389/fnmol.2018.00098
19. Yao Y, Ji Y, Ren J, Liu H, Khanna R, Sun L. Inhibition of autophagy by CRMP2-derived peptide ST2-104 (R9-CBD3) via a CaMKKβ/AMPK/mTOR pathway contributes to ischemic postconditioning-induced neuroprotection against cerebral ischemia-reperfusion injury. Mol Brain. (2021) 14:123. doi: 10.1186/s13041-021-00836-0
20. Bernard GR, Wheeler AP, Russell JA, Schein R, Summer WR, Steinberg KP, et al. The effects of ibuprofen on the physiology and survival of patients with sepsis. The Ibuprofen in Sepsis Study Group. N Engl J Med. (1997) 336:912–8. doi: 10.1056/NEJM199703273361303
21. Heidary Moghaddam R, Samimi Z, Asgary S, Mohammadi P, Hozeifi S, Hoseinzadeh-Chahkandak F, et al. Natural AMPK activators in cardiovascular disease prevention. Front Pharmacol. (2021) 12:738420. doi: 10.3389/fphar.2021.738420
22. Yu Q, Zou L, Yuan X, Fang F, Xu F. Dexmedetomidine protects against septic liver injury by enhancing autophagy through activation of the AMPK/SIRT1 signaling pathway. Front Pharmacol. (2021) 12:658677. doi: 10.3389/fphar.2021.658677
23. Liu J, Chen D, Liu X, Liu Z. Cyclosporine A attenuates cardiac dysfunction induced by sepsis via inhibiting calcineurin and activating AMPK signaling. Mol Med Rep. (2017) 15:3739–46. doi: 10.3892/mmr.2017.6421
24. Liu Z, Bone N, Jiang S, Park DW, Tadie JM, Deshane J, et al. AMP-activated protein kinase and glycogen synthase kinase 3β Modulate the severity of sepsis-induced lung injury. Mol Med (Cambridge Mass). (2016) 21:937–50. doi: 10.2119/molmed.2015.00198
25. Jin K, Ma Y, Manrique-Caballero CL, Li H, Emlet DR, Li S, et al. Activation of AMP-activated protein kinase during sepsis/inflammation improves survival by preserving cellular metabolic fitness. FASEB J. (2020) 34:7036–57. doi: 10.1096/fj.201901900R
26. Stana F, Vujovic M, Mayaki D, Leduc-Gaudet JP, Leblanc P, Huck L, et al. Differential regulation of the autophagy and proteasome pathways in skeletal muscles in sepsis. Crit Care Med. (2017) 45:e971–e9. doi: 10.1097/CCM.0000000000002520
27. Weber-Carstens S, Schneider J, Wollersheim T, Assmann A, Bierbrauer J, Marg A, et al. Critical illness myopathy and GLUT4: significance of insulin and muscle contraction. Am J Respir Crit Care Med. (2013) 187:387–96. doi: 10.1164/rccm.201209-1649OC
28. Ismail Hassan F, Didari T, Khan F, Niaz K, Mojtahedzadeh M, Abdollahi M. A review on the protective effects of metformin in sepsis-induced organ failure. Cell J. (2020) 21:363–70. doi: 10.22074/cellj.2020.6286
29. Hotchkiss RS, Moldawer LL, Opal SM, Reinhart K, Turnbull IR, Vincent JL. Sepsis and septic shock. Nat Rev Dis Primers. (2016) 2:16045. doi: 10.1038/nrdp.2016.45
30. Jeon SM. Regulation and function of AMPK in physiology and diseases. Exp Mol Med. (2016) 48:e245. doi: 10.1038/emm.2016.81
31. Sag D, Carling D, Stout RD, Suttles J. Adenosine 5'-monophosphate-activated protein kinase promotes macrophage polarization to an anti-inflammatory functional phenotype. J Immunol. (2008) 181:8633–41. doi: 10.4049/jimmunol.181.12.8633
32. Idrovo JP, Yang WL, Jacob A, Corbo L, Nicastro J, Coppa GF, et al. Inhibition of lipogenesis reduces inflammation and organ injury in sepsis. J Surg Res. (2016) 200:242–9. doi: 10.1016/j.jss.2015.06.059
33. Van Wyngene L, Vandewalle J, Libert C. Reprogramming of basic metabolic pathways in microbial sepsis: therapeutic targets at last? EMBO. (2018) 10:e8712. doi: 10.15252/emmm.201708712
34. Takahashi W, Watanabe E, Fujimura L, Watanabe-Takano H, Yoshidome H, Swanson PE, et al. Kinetics and protective role of autophagy in a mouse cecal ligation and puncture-induced sepsis. Crit Care. (2013) 17:R160. doi: 10.1186/cc12839
35. Yin X, Xin H, Mao S, Wu G, Guo L. The role of autophagy in sepsis: protection and injury to organs. Front Physiol. (2019) 10:1071. doi: 10.3389/fphys.2019.01071
36. Ho J, Yu J, Wong SH, Zhang L, Liu X, Wong WT, et al. Autophagy in sepsis: Degradation into exhaustion? Autophagy. (2016) 12:1073–82. doi: 10.1080/15548627.2016.1179410
37. Oami T, Watanabe E, Hatano M, Teratake Y, Fujimura L, Sakamoto A, et al. Blocking liver autophagy accelerates apoptosis and mitochondrial injury in hepatocytes and reduces time to mortality in a murine sepsis model. Shock. (2018) 50:427–34. doi: 10.1097/SHK.0000000000001040
38. Kim J, Kundu M, Viollet B, Guan KL. AMPK and mTOR regulate autophagy through direct phosphorylation of Ulk1. Nat Cell Biol. (2011) 13:132–41. doi: 10.1038/ncb2152
39. Ma K, Chen G, Li W, Kepp O, Zhu Y, Chen Q. Mitophagy, mitochondrial homeostasis, and cell fate. Front Cell Dev Biol. (2020) 8:467. doi: 10.3389/fcell.2020.00467
40. Kumar S, Srivastava VK, Kaushik S, Saxena J, Jyoti A. Free radicals, mitochondrial dysfunction and sepsis-induced organ dysfunction: A mechanistic insight. Curr Pharm Des. (2024) 30:161–8. doi: 10.2174/0113816128279655231228055842
41. Lira Chavez FM, Gartzke LP, van Beuningen FE, Wink SE, Henning RH, Krenning G, et al. Restoring the infected powerhouse: Mitochondrial quality control in sepsis. Redox Biol. (2023) 68:102968. doi: 10.1016/j.redox.2023.102968
42. Zhang H, Feng YW, Yao YM. Potential therapy strategy: targeting mitochondrial dysfunction in sepsis. Mil Med Res. (2018) 5:41. doi: 10.1186/s40779-018-0187-0
43. Preau S, Vodovar D, Jung B, Lancel S, Zafrani L, Flatres A, et al. Energetic dysfunction in sepsis: a narrative review. Ann Intensive Care. (2021) 11:104. doi: 10.1186/s13613-021-00893-7
44. McBride MA, Owen AM, Stothers CL, Hernandez A, Luan L, Burelbach KR, et al. The metabolic basis of immune dysfunction following sepsis and trauma. Front Immunol. (2020) 11:1043. doi: 10.3389/fimmu.2020.01043
45. Zhu CL, Yao RQ, Li LX, Li P, Xie J, Wang JF, et al. Mechanism of mitophagy and its role in sepsis induced organ dysfunction: A review. Front Cell Dev Biol. (2021) 9:664896. doi: 10.3389/fcell.2021.664896
46. Mohsin M, Tabassum G, Ahmad S, Ali S, Ali Syed M. The role of mitophagy in pulmonary sepsis. Mitochondrion. (2021) 59:63–75. doi: 10.1016/j.mito.2021.04.009
47. Kitzmiller L, Ledford JR, Hake PW, O'Connor M, Piraino G, Zingarelli B. Activation of AMP-activated protein kinase by A769662 ameliorates sepsis-induced acute lung injury in adult mice. Shock. (2019) 52:540–9. doi: 10.1097/SHK.0000000000001303
48. Zhao H, Chen Y, Qian L, Du L, Wu X, Tian Y, et al. Lycorine protects against septic myocardial injury by activating AMPK-related pathways. Free Radic Biol Med. (2023) 197:1–14. doi: 10.1016/j.freeradbiomed.2023.01.010
49. Patoli D, Mignotte F, Deckert V, Dusuel A, Dumont A, Rieu A, et al. Inhibition of mitophagy drives macrophage activation and antibacterial defense during sepsis. J Clin Invest. (2020) 130:5858–74. doi: 10.1172/JCI130996
50. Park DW, Jiang S, Tadie JM, Stigler WS, Gao Y, Deshane J, et al. Activation of AMPK enhances neutrophil chemotaxis and bacterial killing. Mol Med (Cambridge Mass). (2013) 19:387–98. doi: 10.2119/molmed.2013.00065
51. Kajiwara C, Kusaka Y, Kimura S, Yamaguchi T, Nanjo Y, Ishii Y, et al. Metformin Mediates Protection against Legionella Pneumonia through Activation of AMPK and Mitochondrial Reactive Oxygen Species. J Immunol. (2018) 200:623–31. doi: 10.4049/jimmunol.1700474
52. Huang J, Liu K, Zhu S, Xie M, Kang R, Cao L, et al. AMPK regulates immunometabolism in sepsis. Brain Behav Immun. (2018) 72:89–100. doi: 10.1016/j.bbi.2017.11.003
53. Blagih J, Coulombe F, Vincent EE, Dupuy F, Galicia-Vázquez G, Yurchenko E, et al. The energy sensor AMPK regulates T cell metabolic adaptation and effector responses in vivo. Immunity. (2015) 42:41–54. doi: 10.1016/j.immuni.2014.12.030
54. Silwal P, Paik S, Kim JK, Yoshimori T, Jo EK. Regulatory mechanisms of autophagy-targeted antimicrobial therapeutics against mycobacterial infection. Front Cell Infect Microbiol. (2021) 11:633360. doi: 10.3389/fcimb.2021.633360
55. Zhang J, Dong J, Martin M, He M, Gongol B, Marin TL, et al. AMP-activated protein kinase phosphorylation of angiotensin-converting enzyme 2 in endothelium mitigates pulmonary hypertension. Am J Respir Crit Care Med. (2018) 198:509–20. doi: 10.1164/rccm.201712-2570OC
56. Ni W, Yang X, Yang D, Bao J, Li R, Xiao Y, et al. Role of angiotensin-converting enzyme 2 (ACE2) in COVID-19. Crit Care. (2020) 24:422. doi: 10.1186/s13054-020-03120-0
57. Reis G, Dos Santos Moreira Silva EA, Medeiros Silva DC, Thabane L, Cruz Milagres A, Ferreira TS, et al. Effect of early treatment with metformin on risk of emergency care and hospitalization among patients with COVID-19: The TOGETHER randomized platform clinical trial. Lancet Reg Health Am. (2022) 6:100142. doi: 10.1016/j.lana.2021.100142
58. Bramante CT, Buse JB, Liebovitz DM, Nicklas JM, Puskarich MA, Cohen K, et al. Outpatient treatment of COVID-19 and incidence of post-COVID-19 condition over 10 months (COVID-OUT): a multicentre, randomised, quadruple-blind, parallel-group, phase 3 trial. Lancet Infect diseases. (2023) 23:1119–29. doi: 10.1016/S1473-3099(23)00299-2
59. Bramante CT, Huling JD, Tignanelli CJ, Buse JB, Liebovitz DM, Nicklas JM, et al. Randomized trial of metformin, ivermectin, and fluvoxamine for covid-19. N Engl J Med. (2022) 387:599–610. doi: 10.1056/NEJMoa2201662
60. De Jesús-González LA, Del Ángel RM, Palacios-Rápalo SN, Cordero-Rivera CD, Rodríguez-Carlos A, Trujillo-Paez JV, et al. A dual pharmacological strategy against COVID-19: the therapeutic potential of metformin and atorvastatin. Microorganisms. (2024) 12:383. doi: 10.3390/microorganisms12020383
61. Kang JH, Lee SK, Yun NJ, Kim YS, Song JJ, Bae YS. IM156, a new AMPK activator, protects against polymicrobial sepsis. J Cell Mol Med. (2022) 26:3378–86. doi: 10.1111/jcmm.v26.12
62. Tassaneeyasin T, Sungkanuparph S, Srichatrapimuk S, Charoensri A, Thammavaranucupt K, Jayanama K, et al. Prevalence and risk factors of cytomegalovirus reactivation in critically Ill patients with COVID-19 pneumonia. PLoS One. (2024) 19:e0303995. doi: 10.1371/journal.pone.0303995
63. McArdle J, Moorman NJ, Munger J. HCMV targets the metabolic stress response through activation of AMPK whose activity is important for viral replication. PLoS pathogens. (2012) 8:e1002502. doi: 10.1371/journal.ppat.1002502
64. Silwal P, Kim JK, Yuk JM, Jo EK. AMP-activated protein kinase and host defense against infection. Int J Mol Sci. (2018) 19:3495. doi: 10.3390/ijms19113495
65. Patkee WR, Carr G, Baker EH, Baines DL, Garnett JP. Metformin prevents the effects of Pseudomonas aeruginosa on airway epithelial tight junctions and restricts hyperglycaemia-induced bacterial growth. J Cell Mol Med. (2016) 20:758–64. doi: 10.1111/jcmm.2016.20.issue-4
66. Horowitz A, Chanez-Paredes SD, Haest X, Turner JR. Paracellular permeability and tight junction regulation in gut health and disease. Nat Rev Gastroenterol Hepatol. (2023) 20:417–32. doi: 10.1038/s41575-023-00766-3
67. Oami T, Abtahi S, Shimazui T, Chen CW, Sweat YY, Liang Z, et al. Claudin-2 upregulation enhances intestinal permeability, immune activation, dysbiosis, and mortality in sepsis. Proc Natl Acad Sci United States America. (2024) 121:e2217877121. doi: 10.1073/pnas.2217877121
68. Zhang L, Li J, Young LH, Caplan MJ. AMP-activated protein kinase regulates the assembly of epithelial tight junctions. Proc Natl Acad Sci United States America. (2006) 103:17272–7. doi: 10.1073/pnas.0608531103
69. Xia Z, Huang L, Yin P, Liu F, Liu Y, Zhang Z, et al. L-Arginine alleviates heat stress-induced intestinal epithelial barrier damage by promoting expression of tight junction proteins via the AMPK pathway. Mol Biol Rep. (2019) 46:6435–51. doi: 10.1007/s11033-019-05090-1
70. Chen L, Wang J, You Q, He S, Meng Q, Gao J, et al. Activating AMPK to restore tight junction assembly in intestinal epithelium and to attenuate experimental colitis by metformin. Front Pharmacol. (2018) 9:761. doi: 10.3389/fphar.2018.00761
71. Yoseph BP, Klingensmith NJ, Liang Z, Breed ER, Burd EM, Mittal R, et al. Mechanisms of intestinal barrier dysfunction in sepsis. Shock. (2016). 46:52-9. doi: 10.1097/SHK.0000000000000565
72. Vermette D, Hu P, Canarie MF, Funaro M, Glover J, Pierce RW. Tight junction structure, function, and assessment in the critically ill: a systematic review. Intensive Care Med Exp. (2018) 6:37. doi: 10.1186/s40635-018-0203-4
73. Castanares-Zapatero D, Bouleti C, Sommereyns C, Gerber B, Lecut C, Mathivet T, et al. Connection between cardiac vascular permeability, myocardial edema, and inflammation during sepsis: role of the α1AMP-activated protein kinase isoform. Crit Care Med. (2013) 41:e411–22. doi: 10.1097/CCM.0b013e31829866dc
74. Jian MY, Alexeyev MF, Wolkowicz PE, Zmijewski JW, Creighton JR. Metformin-stimulated AMPK-α1 promotes microvascular repair in acute lung injury. Am J Physiol Lung Cell Mol Physiol. (2013) 305:L844–55. doi: 10.1152/ajplung.00173.2013
75. Zhao X, Zmijewski JW, Lorne E, Liu G, Park YJ, Tsuruta Y, et al. Activation of AMPK attenuates neutrophil proinflammatory activity and decreases the severity of acute lung injury. Am J Physiol Lung Cell Mol Physiol. (2008) 295:L497–504. doi: 10.1152/ajplung.90210.2008
76. Escobar DA, Botero-Quintero AM, Kautza BC, Luciano J, Loughran P, Darwiche S, et al. Adenosine monophosphate-activated protein kinase activation protects against sepsis-induced organ injury and inflammation. J Surg Res. (2015) 194:262–72. doi: 10.1016/j.jss.2014.10.009
77. Inata Y, Kikuchi S, Samraj RS, Hake PW, O'Connor M, Ledford JR, et al. Autophagy and mitochondrial biogenesis impairment contribute to age-dependent liver injury in experimental sepsis: dysregulation of AMP-activated protein kinase pathway. FASEB J. (2018) 32:728–41. doi: 10.1096/fj.201700576R
78. Vaez H, Rameshrad M, Najafi M, Barar J, Barzegari A, Garjani A. Cardioprotective effect of metformin in lipopolysaccharide-induced sepsis via suppression of toll-like receptor 4 (TLR4) in heart. Eur J Pharmacol. (2016) 772:115–23. doi: 10.1016/j.ejphar.2015.12.030
79. Tian R, Li R, Liu Y, Liu J, Pan T, Zhang R, et al. Metformin ameliorates endotoxemia-induced endothelial pro-inflammatory responses via AMPK-dependent mediation of HDAC5 and KLF2. Biochim Biophys Acta Mol basis disease. (2019) 1865:1701–12. doi: 10.1016/j.bbadis.2019.04.009
80. Gao Y, Liu J, Li K, Li T, Li R, Zhang W, et al. Metformin alleviates sepsis-associated myocardial injury by enhancing AMP-activated protein kinase/mammalian target of rapamycin signaling pathway-mediated autophagy. J Cardiovasc Pharmacol. (2023) 82:308–17. doi: 10.1097/FJC.0000000000001463
81. Zhang J, Zhao P, Quan N, Wang L, Chen X, Cates C, et al. The endotoxemia cardiac dysfunction is attenuated by AMPK/mTOR signaling pathway regulating autophagy. Biochem Biophys Res Commun. (2017) 492:520–7. doi: 10.1016/j.bbrc.2017.08.034
82. Liu LJ, Xu M, Zhu J, Li N, Zhao XZ, Gao HM. Adiponectin alleviates liver injury in sepsis rats through AMPK/MTOR pathway. Eur Rev Med Pharmacol Sci. (2020) 24:10745–52. doi: 10.26355/eurrev_202010_23435
83. Lin SP, Wei JX, Hu JS, Bu JY, Zhu LD, Li Q, et al. Artemisinin improves neurocognitive deficits associated with sepsis by activating the AMPK axis in microglia. Acta Pharmacol Sin. (2021) 42:1069–79. doi: 10.1038/s41401-021-00634-3
84. Pan T, Chang Y, He M, He Z, Jiang J, Ren X, et al. [amp]]beta;-Hydroxyisovalerylshikonin regulates macrophage polarization via the AMPK/Nrf2 pathway and ameliorates sepsis in mice. Pharm Biol. (2022) 60:729–42. doi: 10.1080/13880209.2022.2046111
85. Chang KC. Cilostazol inhibits HMGB1 release in LPS-activated RAW 264.7 cells and increases the survival of septic mice. Thromb Res. (2015) 136:456–64. doi: 10.1016/j.thromres.2015.06.017
86. Tan C, Gu J, Chen H, Li T, Deng H, Liu K, et al. Inhibition of aerobic glycolysis promotes neutrophil to influx to the infectious site via CXCR2 in sepsis. Shock. (2020) 53:114–23. doi: 10.1097/SHK.0000000000001334
87. Tan C, Gu J, Li T, Chen H, Liu K, Liu M, et al. Inhibition of aerobic glycolysis alleviates sepsis−induced acute kidney injury by promoting lactate/Sirtuin 3/AMPK−regulated autophagy. Int J Mol Med. (2021) 47:19. doi: 10.3892/ijmm.2021.4852
88. Zhou BY, Yang J, Luo RR, Sun YL, Zhang HT, Yang AX, et al. Dexmedetomidine alleviates ischemia/reperfusion-associated acute kidney injury by enhancing autophagic activity via the α2-AR/AMPK/mTOR pathway. Front Biosci (Landmark Ed). (2023) 28:323. doi: 10.31083/j.fbl2812323
89. Xing W, Yang L, Peng Y, Wang Q, Gao M, Yang M, et al. Ginsenoside Rg3 attenuates sepsis-induced injury and mitochondrial dysfunction in liver via AMPK-mediated autophagy flux. Biosci Rep. (2017) 37:BSR20170934. doi: 10.1042/BSR20170934
90. Li X, Jamal M, Guo P, Jin Z, Zheng F, Song X, et al. Irisin alleviates pulmonary epithelial barrier dysfunction in sepsis-induced acute lung injury via activation of AMPK/SIRT1 pathways. BioMed Pharmacother. (2019) 118:109363. doi: 10.1016/j.biopha.2019.109363
91. Cao J, Mai H, Chen Y, Yuan R, Fang E, Liu M, et al. Ketamine promotes LPS-induced pulmonary autophagy and reduces apoptosis through the AMPK/mTOR pathway. Contrast Media Mol Imaging. (2022) 2022:8713701. doi: 10.1155/2022/8713701
92. Bai X, Zhu Y, Jie J, Li D, Song L, Luo J. Maackiain protects against sepsis via activating AMPK/Nrf2/HO-1 pathway. Int Immunopharmacol. (2022) 108:108710. doi: 10.1016/j.intimp.2022.108710
93. Wu Q, Wang Y, Li Q. Matairesinol exerts anti-inflammatory and antioxidant effects in sepsis-mediated brain injury by repressing the MAPK and NF-κB pathways through up-regulating AMPK. Aging (Albany NY). (2021) 13:23780–95. doi: 10.18632/aging.203649
94. Di W, Jin Z, Lei W, Liu Q, Yang W, Zhang S, et al. Protection of melatonin treatment and combination with traditional antibiotics against septic myocardial injury. Cell Mol Biol Lett. (2023) 28:35. doi: 10.1186/s11658-022-00415-8
95. Chang KC, Ko YS, Kim HJ, Nam DY, Lee DU. 13-Methylberberine reduces HMGB1 release in LPS-activated RAW264.7 cells and increases the survival of septic mice through AMPK/P38 MAPK activation. Int Immunopharmacol. (2016) 40:269–76. doi: 10.1016/j.intimp.2016.08.022
96. Zhuang X, Yu Y, Jiang Y, Zhao S, Wang Y, Su L, et al. Molecular hydrogen attenuates sepsis-induced neuroinflammation through regulation of microglia polarization through an mTOR-autophagy-dependent pathway. Int Immunopharmacol. (2020) 81:106287. doi: 10.1016/j.intimp.2020.106287
97. Ni YL, Shen HT, Su CH, Chen WY, Huang-Liu R, Chen CJ, et al. Nerolidol Suppresses the Inflammatory Response during Lipopolysaccharide-Induced Acute Lung Injury via the Modulation of Antioxidant Enzymes and the AMPK/Nrf-2/HO-1 Pathway. Oxid Med Cell Longev. (2019) 2019:9605980. doi: 10.1155/2019/9605980
98. Li K, Liu TX, Li JF, Ma YR, Liu ML, Wang YQ, et al. rhEPO inhibited cell apoptosis to alleviate acute kidney injury in sepsis by AMPK/SIRT1 activated autophagy. Biochem Biophys Res Commun. (2019) 517:557–65. doi: 10.1016/j.bbrc.2019.07.027
99. Quan H, Yin M, Kim J, Jang EA, Yang SH, Bae HB, et al. Resveratrol suppresses the reprogramming of macrophages into an endotoxin-tolerant state through the activation of AMP-activated protein kinase. Eur J Pharmacol. (2021) 899:173993. doi: 10.1016/j.ejphar.2021.173993
100. Qi Z, Zhang Y, Qi S, Ling L, Gui L, Yan L, et al. Salidroside Inhibits HMGB1 Acetylation and Release through Upregulation of SirT1 during Inflammation. Oxid Med Cell Longev. (2017) 2017:9821543. doi: 10.1155/2017/9821543
101. Wang Y, Xu Y, Zhang P, Ruan W, Zhang L, Yuan S, et al. Smiglaside A ameliorates LPS-induced acute lung injury by modulating macrophage polarization via AMPK-PPARγ pathway. Biochem Pharmacol. (2018) 156:385–95. doi: 10.1016/j.bcp.2018.09.002
102. Quan H, Bae HB, Hur YH, Lee KH, Lee CH, Jang EA, et al. Stearoyl lysophosphatidylcholine inhibits LPS-induced extracellular release of HMGB1 through the G2A/calcium/CaMKKβ/AMPK pathway. Eur J Pharmacol. (2019) 852:125–33. doi: 10.1016/j.ejphar.2019.02.038
103. Chen J, Wang B, Lai J, Braunstein Z, He M, Ruan G, et al. Trimetazidine attenuates cardiac dysfunction in endotoxemia and sepsis by promoting neutrophil migration. Front Immunol. (2018) 9:2015. doi: 10.3389/fimmu.2018.02015
104. Sun M, Wang R, Han Q. Inhibition of leukotriene B4 receptor 1 attenuates lipopolysaccharide-induced cardiac dysfunction: role of AMPK-regulated mitochondrial function. Sci Rep. (2017) 7:44352. doi: 10.1038/srep44352
105. Hu K, Gong X, Ai Q, Lin L, Dai J, Cai L, et al. Endogenous AMPK acts as a detrimental factor in fulminant hepatitis via potentiating JNK-dependent hepatocyte apoptosis. Cell Death disease. (2017) 8:e2637. doi: 10.1038/cddis.2017.62
106. Guo Y, Zhang Y, Hong K, Luo F, Gu Q, Lu N, et al. AMPK inhibition blocks ROS-NFκB signaling and attenuates endotoxemia-induced liver injury. PLoS One. (2014) 9:e86881. doi: 10.1371/journal.pone.0086881
107. Dyck JR, Lopaschuk GD. AMPK alterations in cardiac physiology and pathology: enemy or ally? J Physiol. (2006) 574:95–112. doi: 10.1113/jphysiol.2006.109389
108. Wong HR, Cvijanovich NZ, Anas N, Allen GL, Thomas NJ, Bigham MT, et al. Developing a clinically feasible personalized medicine approach to pediatric septic shock. Am J Respir Crit Care Med. (2015) 191:309–15. doi: 10.1164/rccm.201410-1864OC
109. Davenport EE, Burnham KL, Radhakrishnan J, Humburg P, Hutton P, Mills TC, et al. Genomic landscape of the individual host response and outcomes in sepsis: a prospective cohort study. Lancet Respir Med. (2016) 4:259–71. doi: 10.1016/S2213-2600(16)00046-1
110. Scicluna BP, van Vught LA, Zwinderman AH, Wiewel MA, Davenport EE, Burnham KL, et al. Classification of patients with sepsis according to blood genomic endotype: a prospective cohort study. Lancet Respir Med. (2017) 5:816–26. doi: 10.1016/S2213-2600(17)30294-1
111. Seymour CW, Kennedy JN, Wang S, Chang CH, Elliott CF, Xu Z, et al. Derivation, validation, and potential treatment implications of novel clinical phenotypes for sepsis. Jama. (2019) 321:2003–17. doi: 10.1001/jama.2019.5791
112. Bhavani SV, Semler M, Qian ET, Verhoef PA, Robichaux C, Churpek MM, et al. Development and validation of novel sepsis subphenotypes using trajectories of vital signs. Intensive Care Med. (2022) 48:1582–92. doi: 10.1007/s00134-022-06890-z
113. Baghela A, Pena OM, Lee AH, Baquir B, Falsafi R, An A, et al. Predicting sepsis severity at first clinical presentation: The role of endotypes and mechanistic signatures. EBioMedicine. (2022) 75:103776. doi: 10.1016/j.ebiom.2021.103776
114. Cano-Gamez E, Burnham KL, Goh C, Allcock A, Malick ZH, Overend L, et al. An immune dysfunction score for stratification of patients with acute infection based on whole-blood gene expression. Sci Trans Med. (2022) 14:eabq4433. doi: 10.1126/scitranslmed.abq4433
115. Cummings MJ, Bakamutumaho B, Tomoiaga AS, Owor N, Jain K, Price A, et al. A transcriptomic classifier model identifies high-risk endotypes in a prospective study of sepsis in Uganda. Crit Care Med. (2024) 52:475–82. doi: 10.1097/CCM.0000000000006023
116. Sinha P, Kerchberger VE, Willmore A, Chambers J, Zhuo H, Abbott J, et al. Identifying molecular phenotypes in sepsis: an analysis of two prospective observational cohorts and secondary analysis of two randomised controlled trials. Lancet Respir Med. (2023) 11:965–74. doi: 10.1016/S2213-2600(23)00237-0
117. van Amstel RBE, Kennedy JN, Scicluna BP, Bos LDJ, Peters-Sengers H, Butler JM, et al. Uncovering heterogeneity in sepsis: a comparative analysis of subphenotypes. Intensive Care Med. (2023) 49:1360–9. doi: 10.1007/s00134-023-07239-w
118. Li Z, Zheng W, Kong W, Zeng T. Itaconate: A potent macrophage immunomodulator. Inflammation. (2023) 46:1177–91. doi: 10.1007/s10753-023-01819-0
119. He R, Liu B, Xiong R, Geng B, Meng H, Lin W, et al. Itaconate inhibits ferroptosis of macrophage via Nrf2 pathways against sepsis-induced acute lung injury. Cell Death Discovery. (2022) 8:43. doi: 10.1038/s41420-021-00807-3
120. Martínez-Reyes I, Chandel NS. Mitochondrial TCA cycle metabolites control physiology and disease. Nat Commun. (2020) 11:102. doi: 10.1038/s41467-019-13668-3
121. Phair IR, Nisr RB, Howden AJM, Sovakova M, Alqurashi N, Foretz M, et al. AMPK integrates metabolite and kinase-based immunometabolic control in macrophages. Mol Metab. (2023) 68:101661. doi: 10.1016/j.molmet.2022.101661
122. Fan K, Chen K, Zan X, Zhi Y, Zhang X, Zhang X, et al. Negative regulation of pro-apoptotic AMPK/JNK pathway by itaconate in mice with fulminant liver injury. Cell Death disease. (2023) 14:486. doi: 10.1038/s41419-023-06001-w
123. Chu X, Li L, Yan W, Ma H. 4-octyl itaconate prevents free fatty acid-induced lipid metabolism disorder through activating nrf2-AMPK signaling pathway in hepatocytes. Oxid Med Cell Longev. (2022) 2022:5180242. doi: 10.1155/2022/5180242
124. Inata Y, Piraino G, Hake PW, O'Connor M, Lahni P, Wolfe V, et al. Age-dependent cardiac function during experimental sepsis: effect of pharmacological activation of AMP-activated protein kinase by AICAR. Am J Physiol Heart Circulatory Physiol. (2018) 315:H826–h37. doi: 10.1152/ajpheart.00052.2018
Keywords: AMP activated kinase, metformin, AICAR, sepsis, treatment
Citation: Yumoto T and Coopersmith CM (2024) Targeting AMP-activated protein kinase in sepsis. Front. Endocrinol. 15:1452993. doi: 10.3389/fendo.2024.1452993
Received: 21 June 2024; Accepted: 30 September 2024;
Published: 14 October 2024.
Edited by:
Ashu Johri, Independent Researcher, New York, NY, United StatesReviewed by:
Charles E. McCall, Wake Forest Baptist Medical Center, United StatesPhilip Alexander Efron, University of Florida, United States
Copyright © 2024 Yumoto and Coopersmith. This is an open-access article distributed under the terms of the Creative Commons Attribution License (CC BY). The use, distribution or reproduction in other forums is permitted, provided the original author(s) and the copyright owner(s) are credited and that the original publication in this journal is cited, in accordance with accepted academic practice. No use, distribution or reproduction is permitted which does not comply with these terms.
*Correspondence: Craig M. Coopersmith, Y21jb29wM0BlbW9yeS5lZHU=