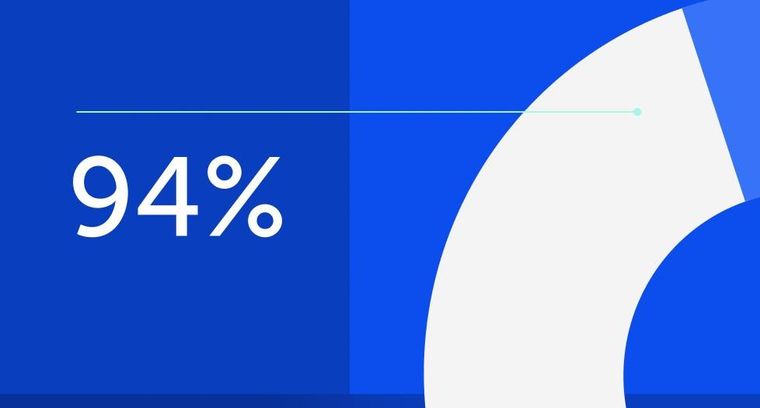
94% of researchers rate our articles as excellent or good
Learn more about the work of our research integrity team to safeguard the quality of each article we publish.
Find out more
MINI REVIEW article
Front. Endocrinol., 02 July 2024
Sec. Diabetes: Molecular Mechanisms
Volume 15 - 2024 | https://doi.org/10.3389/fendo.2024.1412411
This article is part of the Research TopicPancreatic Beta-Cell DedifferentiationView all 5 articles
Early in the development of Type 2 diabetes (T2D), metabolic stress brought on by insulin resistance and nutrient overload causes β-cell hyperstimulation. Herein we summarize recent studies that have explored the premise that an increase in the intracellular Ca2+ concentration ([Ca2+]i), brought on by persistent metabolic stimulation of β-cells, causes β-cell dysfunction and failure by adversely affecting β-cell function, structure, and identity. This mini-review builds on several recent reviews that also describe how excess [Ca2+]i impairs β-cell function.
T2D is a polygenic disease in which insulin resistance brought on by overnutrition, obesity, age, and a high genetic risk profile leads to the loss of glycemic control (1, 2). While many individuals exhibit insulin resistance, the loss of β-cell function in response to mounting metabolic stress determines whether euglycemia is maintained. Thus, to understand the pathogenesis of T2D, we must also know how the β-cell responds to metabolic stress and why it fails, especially in the pre-diabetic stage of the disease.
Compelling evidence exists that endoplasmic reticulum (ER) stress (3), mitochondrial dysfunction (4), cytokine signaling (5) and the loss of cell identity (6) all contribute to the loss of β-cell function in response to metabolic stress. Moreover, over 600 genetic risk loci for T2D have been identified by genome-wide association studies (GWAS) (7–9). While the target genes for most risk loci have not been unambiguously determined, islet-specific transcription factors often bind nearby, suggesting in many cases that they predispose β-cells to fail (10–12).
To develop a mechanism-based explanation for β-cell failure that integrates both genetic and biochemical knowledge, we build on several recent reviews (13–17) and summarize recent studies that point to the dysregulation of intracellular Ca2+ concentrations ([Ca2+]i) as a unifying explanation for the seemingly diverse mechanisms and genes that may contribute to β-cell failure in response to metabolic stress.
Ca2+ is a critical second messenger that regulates many cellular processes in β-cells, insulin exocytosis being foremost among them (18). Decades of studies have provided a now canonical model for metabolism-stimulated insulin secretion (19). Briefly, and as illustrated in Figure 1A, a rise in the plasma glucose concentration is sensed by the β-cell through the metabolism of glucose leading to an increase in the cellular ATP/ADP ratio. The rise in ATP/ADP ratio causes ATP-sensitive potassium (KATP) channel closure, plasma membrane depolarization, the opening of voltage‐gated Ca2+ channels (VDCC), and a rise in intracellular Ca2+ concentrations ([Ca2+]i). The transient spikes in [Ca2+]i stimulate docking and exocytosis of insulin vesicles (20).
Figure 1 A multifaceted role for [Ca2+]i in β-cell function. Hyperglycemia and other insulin secretagogues cause [Ca2+]i in β-cells to increase. (A) Metabolism-stimulated insulin secretion. Glucose and amino acid metabolism lead to an increase in the ATP/ADP ratio, causing KATP (ATP-sensitive) potassium channel closure, plasma membrane depolarization, and the opening of voltage‐gated Ca2+ channels (VDCC) triggering transient increases in intracellular Ca2+ concentrations ([Ca2+]i). The transient spikes in [Ca2+]i stimulate docking and exocytosis of insulin vesicles. The activity of KATP and VDCC channels can be blocked with tolbutamide (a sulfonylurea) and verapamil, respectively. (B) Adaptive regulation. Hormones, cytokines, neurotransmitters, and certain fatty acids and metabolites that signal through G protein-coupled receptors (GPCRs) activate intracellular signal transduction pathways that cause Ca2+ efflux/influx from intracellular stores/extracellular space. GPCR-induced alterations in [Ca2+]i modify other metabolism-based insulin secretory responses. In addition, Ca2+ signaling to the nucleus alters the expression Ca2+-dependent transcription factors that control many cellular functions.
Blocking KATP channel activity, either by the administration of sulfonylureas or by genetic disruptions, causes depolarization of the plasma membrane. Conversely, use of diazoxide, a molecule that opens the KATP channel, or expression of KATP channel subunits (encoded by Kcnj11 and Abcc8) that contain activating mutations, causes hyperpolarization of the plasma membrane (21–24). However, while the canonical model nicely links cell metabolism to insulin secretion and predicts an increase in [Ca2+]i in response to metabolic stress, it overlooks the now well-established fact that [Ca2+]i affects other β-cell organelles, such as the ER, mitochondria, and nucleus (15, 25, 26). Moreover, while the rise of [Ca2+]i is principally metabolism-driven, insulin exocytosis is modulated by many other agents including many hormones, fatty and amino acids, and neurotransmitters in so-called “amplifying pathways”. Many of these agents act by binding to G protein-coupled receptors (GPCRs), and the activation of phospholipase C (PLC)/protein kinase C (PKC) or adenylate cyclase (AC)/protein kinase A (PKA) pathways which rely on Ca2+ for signaling (27–30). An extended model (Figure 1B) recognizes both the role of other agents in modulating insulin secretion, and the critically important role of Ca2+ in other organelles.
[Ca2+]i is tightly regulated by transmembrane channels and pumps, Ca2+ buffering proteins, and by the uptake and release of Ca2+ from ER stores and mitochondria (31). Ca2+ concentrations vary considerably among different subcellular compartments, with concentrations in extracellular space (~1–2 mM) and ER and Golgi compartments (~200–700 µM) being more than ten thousand times higher than in the cytosol (~100 nM) (32, 33). Mitochondria Ca2+ concentrations vary between 50–500 nM in order to regulate metabolism and serve as a transient calcium buffer (34). Metabolic stimulation causes [Ca2+]i to sharply increase from basal levels of ~100 nM to stimulated levels of ~1–3 µM due to Ca2+ entry from the extracellular space or Ca2+ release from intracellular stores. These spikes in [Ca2+]i may start locally before propagating as cyclical Ca2+ oscillations throughout the islet (35, 36). While Ca2+ spikes are tightly linked to insulin secretion, an increase in [Ca2+]i also directly affects Ca2+ concentrations in various organelles, including the nucleus, through multiple influx/efflux pathways (37).
Both the ER and mitochondria require Ca2+ for their function, and both serve as intracellular Ca2+ reservoirs. The ER is critical for protein synthesis and folding, lipid synthesis, and Ca2+ storage and release, and β-cells require optimal ER functionality to support the production of insulin and to maintain [Ca2+]i homeostasis (25). The entry and release of Ca2+ from and to the ER is mainly regulated by SERCA pumps, or by inositol 1,4,5-triphosphate (IP3R) and by ryanodine receptors (RyR), respectively. Moreover, store-operated Ca2+ entry from the extracellular space plays a critical role in maintaining ER Ca2+ concentrations. Dysfunctions in any of these processes can change ER susceptibility to stress (38, 39). Not only does ER Ca2+ affect the unfolded protein stress response (40), persistent ER stress likely causes β-cell demise in both Type 1 and T2D (25, 41). Indeed, cytokine-induced depletion of Ca2+ from the ER may directly trigger apoptosis (42).
Mitochondrial activity and metabolic enzymes are also regulated by Ca2+ (43, 44). Glucose-stimulated insulin secretion is directly linked to mitochondrial function, and Ca2+ flows between the ER and mitochondria via mitochondria-associated ER membrane (MAMs) contact sites. Since Ca2+ is released from the ER and directly taken up by mitochondria, intraluminal ER, mitochondrial matrix, and cytoplasmic Ca2+ concentrations are closely interrelated (45, 46). ER-mitochondria interplay may also be a critical cellular adaptive mechanism for restoring [Ca2+]i homeostasis after episodes of Ca2+ overload, thereby also contributing to β-cell dysfunction (47, 48).
Ca2+ signaling to the nucleus links signaling cues with gene expression, enabling cellular adaptations to both internal and external stimuli (49). This process, known as excitation-transcription coupling, is well-described in neurons and myocytes (50–53). Ca2+ influx in response to membrane depolarization and GPCR activation triggers multiple intracellular signaling pathways that regulate cell identity, proliferation, autophagy and cell death (54). Glucose stimulation leads to increase in β-cell nuclear Ca2+ concentration (55, 56). [Ca2+]i is sensed by Ca2+-binding proteins (CBPs), with calmodulin being the most well-studied (57). These molecules in turn activate downstream targets, including the protein phosphatase calcineurin and Ca2+/calmodulin-dependent kinases (e.g. CamKII and CamKIV), which in turn modulate the activity of Ca2+ responsive transcription factors such as NFAT and CREB (58–62), as well as a number of other transcription factors, transcriptional co-regulators and chromatin modifying enzymes (63, 64). While excitation-transcription coupling is a part of a normal response to changes in the cell environment, chronic and sustained activation of Ca2+ signaling pathways is detrimental to cell function (65–67).
While the harmful effects of excess [Ca2+]i have been extensively investigated in other excitable cell types, and a critical role for Ca2+ signaling in β-cell function has long been clear, we lack a clear understanding of how Ca2+ signaling is linked to β-cell failure (13–17). Prior studies have shown that basal [Ca2+]i is increased in rat islets cultured in high glucose (68), in mouse islets from obese (db/db) mice (69–71), in islets from mice fed a high-fat diet (HFD) (72), and in β-cells that exhibit chronic membrane depolarization due to the loss of KATP channel subunit Abcc8 (73). Conversely, lowering [Ca2+]i in β-cells by blocking Ca2+ influx with verapamil (74–78), other compounds (79), or by a genetic deletion of Cavβ3, a voltage-dependent calcium channel subunit (80), attenuates β-cell loss and diabetes in mice and humans. Moreover, studies in which β cells are “rested” through the use of diazoxide, which opens the KATP channel thereby impairing membrane depolarization, or other therapies that lower the blood glucose concentration or reduce glucokinase activity, may all work in large part by limiting increases in [Ca2+]i (41, 71). However, while there is ample evidence showing a correlation between a sustained increase in [Ca2+]i and impairments in β-cell function, we do not know how metabolic stress-induced increases in [Ca2+]i, which are likely to only be transient during the pre-diabetic phase of T2D, initiate events that lead to β-cell failure, as illustrated in Figure 2. Similarly, we do not know how specific Ca2+-regulated processes are affected by T2D-associated risk loci, either individually or in combination.
Figure 2 A model for the development of T2D that considers the role of metabolic-stress and [Ca2+]i. This model illustrates the stepwise failure of β-cells in response to metabolic stress. 1) In healthy individuals β-cells have normal [Ca2+]i levels. 2) In pre-diabetes, environmental factors such as age, sex, genetic makeup, obesity, and overnutrition cause insulin resistance and an increase in insulin demand resulting in mild metabolic stress. Transient elevations of the blood glucose and other insulin secretagogues cause the hyperstimulation of β-cells and small increases in [Ca2+]i. Initially, the increase in Ca2+-signaling stimulate insulin secretion, β-cell proliferation and other adaptive responses that continue to maintain glycemia and compensate for increased insulin demand. 3) The limited ability of β-cells to compensate together with a continuing rise in metabolic stress cause further increases in [Ca2+]i. A tipping point occurs where a network of Ca2+-regulated genes crucial for maintaining Ca2+ homeostasis and β-cell function becomes maladaptive. 4) The maladaptive changes brought on by chronically elevated [Ca2+]i cause the loss of β-cell identity and function, with β-cells entering a decompensation stage where they can no longer secrete enough insulin to maintain normal blood glucose. Glucolipotoxicity further accelerates the loss of β-cell function, identity and viability, therefore resulting in overt T2D. The increased gray shading of β-cells from left to right indicates increasing loss of β-cell identity and function. The * indicates a tipping point.
Consistent with the canonical model (Figure 1), depolarization of the plasma membrane is predicted to cause Ca2+ influx, a rise in [Ca2+]i, and an increase in insulin secretion. Abcc8 and Kcnj11 knockout mice, both of which lack functional KATP channels and exhibit a sustained elevation in [Ca2+]i, display mild hypoglycemia as young animals but develop diabetes as they age (73, 81–85). Similarly, in humans, several individuals with inactivating mutations of the KATP channel that cause hyperinsulinism in infancy have been reported to develop diabetes in adolescence (86, 87). The findings that both mice and humans with genetically-driven increases in [Ca2+]i maintain euglycemia for several months before they cross over to being overtly diabetic has important implications (73, 82). First, it clearly separates the effects of a sustained pathological increase in [Ca2+]i, often referred to as excitotoxity, from glucotoxicity, which occurs after the onset of hyperglycemia. Second, the delay has enabled studies of how β-cell gene expression and function is affected by a chronic increase in [Ca2+]i without the confounding effects of hyperglycemia (73, 82, 88).
Studies of Abcc8 knockout mice revealed alterations in islet morphology and glucose intolerance prior to the development of hyperglycemia. In addition, they revealed a loss of β-cell identity that correlated with a marked alteration of a network of Ca2+ regulated genes (73). Recently, studies of β-cell-specific Kcnj11 knockout mice, which also exhibit an increase in [Ca2+]i and glucose intolerance, revealed a Gs/Gq signaling switch (89).
Since overnutrition is common in pre-diabetes, we compared transcriptomes of FACS-purified β-cells of Abcc8 knockout mice, which serve as a model for excitotoxicity, with mice fed a HFD (88). Both excitotoxicity and overnutrition were found to affect overlapping sets of genes, and to exert an additively negative effect on β-cell function (88). The commonalities in transcriptional response are not surprising since overnutrition, by elevating circulating free fatty acids (FFAs), contributes to insulin resistance and hyperglycemia (90) by causing the release of Ca2+ from ER stores, increase in [Ca2+]i and accentuating both ER and oxidative stress (16, 91–95).
While excitotoxicity and overnutrition individually perturb the expression in β-cells of several thousand genes (88), a meta-analysis revealed that many of the upregulated genes were involved in oxidative phosphorylation, mitochondrial organization, metabolic pathways, and oxidative stress response whereas downregulated genes were involved in cell organization, secretory function, cell adhesion, cell junctions, cilia, cytoskeleton, and regulation of β-cell epigenetic and transcriptional program (88). Furthermore, many genes that are dysregulated excitotoxicity and overnutrition are altered in pre-diabetic and diabetic β-cells from db/db mice (96), also suggesting a strong correlation between chronic alterations in [Ca2+]i and the loss of β-cell function.
A chronic increase in [Ca2+]i stimulates expression of mitochondrial structural and metabolic genes (Me3, Cox7a) that is parallelled by increased oxygen consumption and mitogenesis in islets (88). Chronic stimulation of the electron transport chain leads to an increase in reactive oxygen species and to mitochondrial dysfunction (97). Furthermore, the combination of increased [Ca2+]i and overnutrition not only impairs mitochondrial function, but it may also impair the replacement of metabolically damaged mitochondria (88) due to the downregulation of mitophagy associated genes (Clec16a, Prkn) (88, 98–100).
Lysosomes are of note since they are involved in maintaining the mitochondrial biogenesis/mitophagy balance (101), and stressed β-cells showed an increase in regulators for mitochondrial (Ppargc1a) and lysosomal (Tfeb) biogenesis (88), both known to be activated by Ca2+ in other cell types (102, 103). Ppargc1a, a key transcriptional regulator of energy metabolism, FA β-oxidation and mitochondrial biogenesis is implicated in β-cell dysfunction and T2D (104–106).
Metabolic stress, by increasing the expression of genes that contribute to metabolic inflexibility, may also impair the ability of β-cells to utilize glucose, which would impair their metabolic response to glucose (107). Consistent with this, an increase in the expression of FA β-oxidation genes, as well as Pdk4, a kinase that inhibits pyruvate flux into the TCA cycle (108), and decrease in mitochondrial respiration response to glucose also suggests that stressed β-cells switch to FAs and ketones as mitochondrial fuels (88).
Together, these findings suggest that metabolic-stress induced elevations in [Ca2+]i cause impairments in mitochondrial function that reduce the ability of β-cells to respond to glucose, an unambiguous sign of β-cell failure.
Excitotoxicity and overnutrition also cause an increase in the expression of genes associated with ER secretory stress (88, 109). Glycosylation, the process during which glycans (mono- or oligosaccharides) are attached to proteins in the ER and Golgi, is a critical quality control signal in ER protein folding (110). Since excess protein glycosylation brought on by ER stress is linked to cellular apoptosis (111), the observed increases in expression of genes associated with ER protein folding and N- and O-linked protein glycosylation are highly noteworthy as they suggest that the stability, localization, trafficking, and function of glycosylated receptors, ion channels, nutrient transporters, and transcription factors in β-cells may all be adversely affected (112).
Since islet architecture in Abcc8 knock-out mice is abnormal (73), it is not surprising that many genes important for β-cell structure and function downregulated (88), including those necessary for cell adhesion and cell-cell junctions (113), cilia (114), and cytoskeletal and vesicular trafficking (115). Our transcriptomic analysis also predicts changes in β-cell polarity since genes essential for apical domain formation, primary cilia, and the lateral domain are all downregulated while genes associated with the vasculature-facing basal domain are upregulated (116). Together, the many changes we observed suggest that a chronic increase in [Ca2+]i disrupts cell polarity, exocytotic machinery, and critical cell-cell contacts, thereby physically disrupting islet architecture and insulin secretion.
Cellular dedifferentiation and the resulting loss of β-cell identity are fundamentally important contributors to β-cell dysfunction in T2D (117, 118). In β-cells that are stressed by excitotoxicity and overnutrition, many transcription factors that are essential for maintaining β-cell identity are downregulated (88). Similarly, epigenetic modifiers, including a DNA methyltransferase (Dnmt1) important for silencing of developmental or “disallowed” metabolic genes in mature β-cells (119), are decreased. This likely explains the upregulation of multiple disallowed genes (120) in response to metabolic stress (88). Several lncRNAs which contribute to the maintenance of the epigenetic and transcriptional landscape of β-cells (121), are also down-regulated. Importantly, Aldh1a3 (122) and Bach2 (123), two well-established markers and drivers of β-cell dedifferentiation, are upregulated, suggesting that they too are regulated by Ca2+ (88). Thus, the continued expression of key transcription factors necessary to maintain β-cell identity likely also depends on the maintenance of Ca2+-signaling and homeostatic regulation.
While the expression of many genes in β-cells is altered by metabolic stress, the chronic nature of the analyses performed to date limits the establishment of direct cause-and-effect relationships. For this reason, we sought to identify a Ca2+-regulated gene that could be studied in detail. Ascl1 (Achaete-scute homolog 1) stood out since many of the genes putatively upregulated by [Ca2+]i contain binding sites for ASCL1 (73). In addition, Ascl1 is a pioneer transcription factor critical for neural cell differentiation (124–126) and is necessary for the formation of neuroendocrine cells in multiple tissues (127–129). Importantly, ASCL1 is also expressed in human islets (130).
To investigate how Ascl1 contributes to β-cell dysfunction during metabolic stress, we generated β-cell-specific Ascl1 knockout mice and studied their responses to both excitotoxicity and overnutrition. We found that Ascl1 is indeed induced by stimuli that cause Ca2+-signaling to the nucleus, and that it contributes in multiple ways to the loss of β-cell function. Remarkably, the removal of Ascl1 from β-cells improved their function in response to metabolic stress by HFD feeding (131). Transcriptional profiling of islets under different experimental conditions revealed that ASCL1 contributes to a loss of β-cell function both by activating a dedifferentiation program and by suppressing the expression of secretory and innervation genes in response to overnutrition.
Interestingly, β-specific Ascl1 knockout islets from HFD mice have increased expression of parasympathetic neuronal markers, increased insulin secretion in response to acetylcholine, and an increased islet innervation. While additional studies of the role of Ascl1 in stressed β-cells are necessary, our experiments clearly demonstrate that a metabolic stress-induced increase in Ca2+-signaling to the nucleus alters both β-cell function and identity in an ASCL1-dependent manner. Our studies also point to a role for other Ca2+-regulated transcription factors, suggesting that a Ca2+-dependent gene regulatory network is critical for the proper function of β-cells, and that metabolic-stress profoundly modifies this network by invoking both adaptive and maladaptive transcriptional changes.
Although multiple lines of evidence point to Ca2+-signaling being intimately involved in metabolic stress-induced β-cell failure, the mechanisms whereby an increase in [Ca2+]i leads to a loss of β-cell function are not understood and need further investigation.
1. Temporal causality between changes in [Ca2+]i, the expression of key transcription factors, and the loss of β-cell function needs to be established.
2. We need to better understand how specific Ca2+-regulated processes modulate β-cell function. Cleverly designed studies are required to distinguish between the effects of elevated metabolic flux and closely linked [Ca2+]i.
3. We need to determine how overnutrition and insulin resistance affect Ca2+ spiking activity in pre-diabetic setting.
4. We need to better understand how Ca2+-mediated transcriptional reprogramming impairs β-cell function and identity.
5. Finally, we need to determine how specific T2D genetic risk loci affect Ca2+-dependent processes in β-cells.
While our assertions for the importance of Ca2+-signaling have strong experimental support, we do not understand how genetic risk loci, either individually or in aggregate, may contribute to β-cell dysfunction.
We hope that this mini-review stimulates investigations by others as there is much to learn about how alterations in [Ca2+]i affect β-cell function and contribute to T2D.
MM: Writing – original draft, Writing – review & editing. AO: Writing – original draft, Writing – review & editing.
The author(s) declare financial support was received for the research, authorship, and/or publication of this article. The studies described herein were supported by institutional and philanthropic funds from Vanderbilt University.
We thank Pamela Uttz for skillfully proofing of the text.
The authors declare that the research was conducted in the absence of any commercial or financial relationships that could be construed as a potential conflict of interest.
All claims expressed in this article are solely those of the authors and do not necessarily represent those of their affiliated organizations, or those of the publisher, the editors and the reviewers. Any product that may be evaluated in this article, or claim that may be made by its manufacturer, is not guaranteed or endorsed by the publisher.
1. Chang-Chen KJ, Mullur R, Bernal-Mizrachi E. Beta-cell failure as a complication of diabetes. Rev Endocr Metab Disord. (2008) 9:329–43. doi: 10.1007/s11154-008-9101-5
2. Muoio DM, Newgard CB. Mechanisms of disease: molecular and metabolic mechanisms of insulin resistance and beta-cell failure in type 2 diabetes. Nat Rev Mol Cell Biol. (2008) 9:193–205. doi: 10.1038/nrm2327
3. Fonseca SG, Gromada J, Urano F. Endoplasmic reticulum stress and pancreatic beta-cell death. Trends Endocrinol Metab. (2011) 22:266–74. doi: 10.1016/j.tem.2011.02.008
4. Sha W, Hu F, Bu S. Mitochondrial dysfunction and pancreatic islet beta-cell failure (Review). Exp Ther Med. (2020) 20:266. doi: 10.3892/etm
5. Rohm TV, Meier DT, Olefsky JM, Donath MY. Inflammation in obesity, diabetes, and related disorders. Immunity. (2022) 55:31–55. doi: 10.1016/j.immuni.2021.12.013
6. Swisa A, Glaser B, Dor Y. Metabolic stress and compromised identity of pancreatic beta cells. Front Genet. (2017) 8:21. doi: 10.3389/fgene.2017.00021
7. Torres JM, Abdalla M, Payne A, Fernandez-Tajes J, Thurner M, Nylander V, et al. A multi-omic integrative scheme characterizes tissues of action at loci associated with type 2 diabetes. Am J Hum Genet. (2020) 107:1011–28. doi: 10.1016/j.ajhg.2020.10.009
8. Mahajan A, Taliun D, Thurner M, Robertson NR, Torres JM, Rayner NW, et al. Fine-mapping type 2 diabetes loci to single-variant resolution using high-density imputation and islet-specific epigenome maps. Nat Genet. (2018) 50:1505–13. doi: 10.1016/j.ceca.2014.09.001
9. Suzuki K, Hatzikotoulas K, Southam L, Taylor HJ, Yin X, Lorenz KM, et al. Genetic drivers of heterogeneity in type 2 diabetes pathophysiology. Nature. (2024) 627:347–57. doi: 10.3390/ijms20246110
10. Pasquali L, Gaulton KJ, Rodriguez-Segui SA, Mularoni L, Miguel-Escalada I, Akerman I, et al. Pancreatic islet enhancer clusters enriched in type 2 diabetes risk-associated variants. Nat Genet. (2014) 46:136–43. doi: 10.1038/ng.2870
11. Rosengren AH, Braun M, Mahdi T, Andersson SA, Travers ME, Shigeto M, et al. Reduced insulin exocytosis in human pancreatic beta-cells with gene variants linked to type 2 diabetes. Diabetes. (2012) 61:1726–33. doi: 10.2337/db11-1516
12. Miguel-Escalada I, Bonas-Guarch S, Cebola I, Ponsa-Cobas J, Mendieta-Esteban J, Atla G, et al. Human pancreatic islet three-dimensional chromatin architecture provides insights into the genetics of type 2 diabetes. Nat Genet. (2019) 51:1137–48. doi: 10.1038/s41588-019-0457-0
13. Gilon P, Chae HY, Rutter GA, Ravier MA. Calcium signaling in pancreatic beta-cells in health and in Type 2 diabetes. Cell Calcium. (2014) 56:340–61. doi: 10.1016/j.ceca.2014.09.001
14. Klec C, Ziomek G, Pichler M, Malli R, Graier WF. Calcium signaling in ss-cell physiology and pathology: A revisit. Int J Mol Sci. (2019) 20:6110. doi: 10.3390/ijms20246110
15. Sabatini PV, Speckmann T, Lynn FC. Friend and foe: beta-cell Ca(2+) signaling and the development of diabetes. Mol Metab. (2019) 21:1–12. doi: 10.1016/j.molmet.2018.12.007
16. Idevall-Hagren O, Tengholm A. Metabolic regulation of calcium signaling in beta cells. Semin Cell Dev Biol. (2020) 103:20–30. doi: 10.1016/j.semcdb.2020.01.008
17. Jacobson DA, Shyng SL. Ion channels of the islets in type 2 diabetes. J Mol Biol. (2020) 432:1326–46. doi: 10.1016/j.jmb.2019.08.014
18. Berridge MJ. Calcium signalling remodelling and disease. Biochem Soc Trans. (2012) 40:297–309. doi: 10.1042/BST20110766
19. Newsholme P, Gaudel C, McClenaghan NH. Nutrient regulation of insulin secretion and beta-cell functional integrity. Adv Exp Med Biol. (2010) 654:91–114. doi: 10.1007/978-90-481-3271-3_6
20. Henquin JC. Triggering and amplifying pathways of regulation of insulin secretion by glucose. Diabetes. (2000) 49:1751–60. doi: 10.2337/diabetes.49.11.1751
21. Nichols CG, York NW, Remedi MS. ATP-sensitive potassium channels in hyperinsulinism and type 2 diabetes: inconvenient paradox or new paradigm? Diabetes. (2022) 71:367–75. doi: 10.2337/db21-0755
22. Remedi MS, Koster JC. K(ATP) channelopathies in the pancreas. Pflugers Arch. (2010) 460:307–20. doi: 10.1007/s00424-009-0756-x
23. Aguilar-Bryan L, Clement J, Gonzalez G, Kunjilwar K, Babenko A, Bryan J. Toward understanding the assembly and structure of KATP channels. Physiol Rev. (1998) 78:227–45. doi: 10.1152/physrev.1998.78.1.227
24. Brereton MF, Ashcroft FM. Mouse models of beta-cell K(ATP) channel dysfunction. Drug Discovery Today Dis Models. (2013) 10:e101–e9. doi: 10.1016/j.ddmod.2013.02.001
25. Zhang IX, Raghavan M, Satin LS. The endoplasmic reticulum and calcium homeostasis in pancreatic beta cells. Endocrinology. (2020) 161:bqz028. doi: 10.1210/endocr/bqz028
26. Weiser A, Feige JN, De Marchi U. Mitochondrial calcium signaling in pancreatic beta-cell. Int J Mol Sci. (2021) 22(5):2515. doi: 10.3390/ijms22052515
27. Keane K, Newsholme P. Metabolic regulation of insulin secretion. Vitam Horm. (2014) 95:1–33. doi: 10.1016/B978-0-12-800174-5.00001-6
28. Kalwat MA, Cobb MH. Mechanisms of the amplifying pathway of insulin secretion in the beta cell. Pharmacol Ther. (2017) 179:17–30. doi: 10.1016/j.pharmthera.2017.05.003
29. Kebede MA, Alquier T, Latour MG, Poitout V. Lipid receptors and islet function: therapeutic implications? Diabetes Obes Metab. (2009) 11 Suppl 4:10–20. doi: 10.1111/j.1463-1326.2009.01114.x
30. Varney MJ, Benovic JL. The role of G protein-coupled receptors and receptor kinases in pancreatic beta-cell function and diabetes. Pharmacol Rev. (2024) 76:267–99. doi: 10.1124/pharmrev.123.001015
31. Bagur R, Hajnoczky G. Intracellular ca(2+) sensing: its role in calcium homeostasis and signaling. Mol Cell. (2017) 66:780–8. doi: 10.1016/j.molcel.2017.05.028
32. Berridge MJ, Lipp P, Bootman MD. The versatility and universality of calcium signalling. Nat Rev Mol Cell Biol. (2000) 1:11–21. doi: 10.1038/35036035
33. Zampese E, Pizzo P. Intracellular organelles in the saga of Ca2+ homeostasis: different molecules for different purposes? Cell Mol Life Sci. (2012) 69:1077–104. doi: 10.1007/s00018-011-0845-9
34. Romero-Garcia S, Prado-Garcia H. Mitochondrial calcium: Transport and modulation of cellular processes in homeostasis and cancer (Review). Int J Oncol. (2019) 54:1155–67. doi: 10.3892/ijo
35. Fridlyand LE, Tamarina N, Philipson LH. Bursting and calcium oscillations in pancreatic beta-cells: specific pacemakers for specific mechanisms. Am J Physiol Endocrinol Metab. (2010) 299:E517–32. doi: 10.1152/ajpendo.00177.2010
36. Fletcher PA, Thompson B, Liu C, Bertram R, Satin LS, Sherman AS. Ca(2+) release or Ca(2+) entry, that is the question: what governs Ca(2+) oscillations in pancreatic beta cells? Am J Physiol Endocrinol Metab. (2023) 324:E477–E87. doi: 10.1152/ajpendo.00030.2023
37. Laude AJ, Simpson AW. Compartmentalized signalling: Ca2+ compartments, microdomains and the many facets of Ca2+ signalling. FEBS J. (2009) 276:1800–16. doi: 10.1111/j.1742-4658.2009.06927.x
38. Luciani DS, Gwiazda KS, Yang TL, Kalynyak TB, Bychkivska Y, Frey MH, et al. Roles of IP3R and RyR Ca2+ channels in endoplasmic reticulum stress and beta-cell death. Diabetes. (2009) 58:422–32. doi: 10.2337/db07-1762
39. Sabourin J, Le Gal L, Saurwein L, Haefliger JA, Raddatz E, Allagnat F. Store-operated ca2+ Entry mediated by orai1 and TRPC1 participates to insulin secretion in rat beta-cells. J Biol Chem. (2015) 290:30530–9. doi: 10.1074/jbc.M115.682583
40. Groenendyk J, Agellon LB, Michalak M. Calcium signaling and endoplasmic reticulum stress. Int Rev Cell Mol Biol. (2021) 363:1–20. doi: 10.1016/bs.ircmb.2021.03.003
41. Kalwat MA, Scheuner D, Rodrigues-Dos-Santos K, Eizirik DL, Cobb MH. The pancreatic ss-cell response to secretory demands and adaption to stress. Endocrinology. (2021) 162(11):bqab173. doi: 10.1210/endocr/bqab173
42. Ramadan JW, Steiner SR, O’Neill CM, Nunemaker CS. The central role of calcium in the effects of cytokines on beta-cell function: implications for type 1 and type 2 diabetes. Cell Calcium. (2011) 50:481–90. doi: 10.1016/j.ceca.2011.08.005
43. Wiederkehr A, Wollheim CB. Impact of mitochondrial calcium on the coupling of metabolism to insulin secretion in the pancreatic beta-cell. Cell Calcium. (2008) 44:64–76. doi: 10.1016/j.ceca.2007.11.004
44. Tarasov AI, Griffiths EJ, Rutter GA. Regulation of ATP production by mitochondrial Ca(2+). Cell Calcium. (2012) 52:28–35. doi: 10.1016/j.ceca.2012.03.003
45. Huang DX, Yu X, Yu WJ, Zhang XM, Liu C, Liu HP, et al. Calcium signaling regulated by cellular membrane systems and calcium homeostasis perturbed in alzheimer’s disease. Front Cell Dev Biol. (2022) 10:834962. doi: 10.3389/fcell.2022.834962
46. Barazzuol L, Giamogante F, Cali T. Mitochondria Associated Membranes (MAMs): Architecture and physiopathological role. Cell Calcium. (2021) 94:102343. doi: 10.1016/j.ceca.2020.102343
47. Vig S, Lambooij JM, Zaldumbide A, Guigas B. Endoplasmic reticulum-mitochondria crosstalk and beta-cell destruction in type 1 diabetes. Front Immunol. (2021) 12:669492. doi: 10.3389/fimmu.2021.669492
48. Madec AM, Perrier J, Panthu B, Dingreville F. Role of mitochondria-associated endoplasmic reticulum membrane (MAMs) interactions and calcium exchange in the development of type 2 diabetes. Int Rev Cell Mol Biol. (2021) 363:169–202. doi: 10.1016/bs.ircmb.2021.06.001
49. van Haasteren G, Li S, Muda M, Susini S, Schlegel W. Calcium signalling and gene expression. J Recept Signal Transduct Res. (1999) 19:481–92. doi: 10.3109/10799899909036666
50. Wamhoff BR, Bowles DK, Owens GK. Excitation-transcription coupling in arterial smooth muscle. Circ Res. (2006) 98:868–78. doi: 10.1161/01.RES.0000216596.73005.3c
51. Dolmetsch R. Excitation-transcription coupling: signaling by ion channels to the nucleus. Sci STKE. (2003) 2003:PE4. doi: 10.1126/scisignal.1662003pe4
52. Ma H, Khaled HG, Wang X, Mandelberg NJ, Cohen SM, He X, et al. Excitation-transcription coupling, neuronal gene expression and synaptic plasticity. Nat Rev Neurosci. (2023) 24:672–92. doi: 10.1038/s41583-023-00742-5
53. Dewenter M, von der Lieth A, Katus HA, Backs J. Calcium signaling and transcriptional regulation in cardiomyocytes. Circ Res. (2017) 121:1000–20. doi: 10.1161/CIRCRESAHA.117.310355
54. Patergnani S, Danese A, Bouhamida E, Aguiari G, Previati M, Pinton P, et al. Various aspects of calcium signaling in the regulation of apoptosis, autophagy, cell proliferation, and cancer. Int J Mol Sci. (2020) 21(21):8323. doi: 10.3390/ijms21218323
55. Quesada I, Rovira JM, Martin F, Roche E, Nadal A, Soria B. Nuclear KATP channels trigger nuclear Ca(2+) transients that modulate nuclear function. Proc Natl Acad Sci U S A. (2002) 99:9544–9. doi: 10.1073/pnas.142039299
56. Quesada I, Martin F, Roche E, Soria B. Nutrients induce different Ca(2+) signals in cytosol and nucleus in pancreatic beta-cells. Diabetes. (2004) 53 Suppl 1:S92–5. doi: 10.2337/diabetes.53.2007.S92
57. Niki I, Hidaka H. Roles of intracellular Ca2+ receptors in the pancreatic beta-cell in insulin secretion. Mol Cell Biochem. (1999) 190:119–24. doi: 10.1023/a:1006997822987
58. Heit JJ, Apelqvist AA, Gu X, Winslow MM, Neilson JR, Crabtree GR, et al. Calcineurin/NFAT signalling regulates pancreatic beta-cell growth and function. Nature. (2006) 443:345–9. doi: 10.1038/nature05097
59. Soleimanpour SA, Crutchlow MF, Ferrari AM, Raum JC, Groff DN, Rankin MM, et al. Calcineurin signaling regulates human islet {beta}-cell survival. J Biol Chem. (2010) 285:40050–9. doi: 10.1074/jbc.M110.154955
60. Dadi PK, Vierra NC, Ustione A, Piston DW, Colbran RJ, Jacobson DA. Inhibition of pancreatic beta-cell Ca2+/calmodulin-dependent protein kinase II reduces glucose-stimulated calcium influx and insulin secretion, impairing glucose tolerance. J Biol Chem. (2014) 289:12435–45. doi: 10.1074/jbc.M114.562587
61. Blanchet E, Van de Velde S, Matsumura S, Hao E, LeLay J, Kaestner K, et al. Feedback inhibition of CREB signaling promotes beta cell dysfunction in insulin resistance. Cell Rep. (2015) 10:1149–57. doi: 10.1016/j.celrep.2015.01.046
62. Yu X, Murao K, Sayo Y, Imachi H, Cao WM, Ohtsuka S, et al. The role of calcium/calmodulin-dependent protein kinase cascade in glucose upregulation of insulin gene expression. Diabetes. (2004) 53:1475–81. doi: 10.2337/diabetes.53.6.1475
63. Hernandez-Oliveras A, Zarain-Herzberg A. The role of Ca(2+)-signaling in the regulation of epigenetic mechanisms. Cell Calcium. (2024) 117:102836. doi: 10.1016/j.ceca.2023.102836
64. Bading H. Nuclear calcium signalling in the regulation of brain function. Nat Rev Neurosci. (2013) 14:593–608. doi: 10.1038/nrn3531
65. Kiessling M, Djalinac N, Voglhuber J, Ljubojevic-Holzer S. Nuclear calcium in cardiac (Patho)Physiology: small compartment, big impact. Biomedicines. (2023) 11:960. doi: 10.3390/biomedicines11030960
66. Roca-Lapirot O, Radwani H, Aby F, Nagy F, Landry M, Fossat P. Calcium signalling through L-type calcium channels: role in pathophysiology of spinal nociceptive transmission. Br J Pharmacol. (2018) 175:2362–74. doi: 10.1111/bph.13747
67. Mozolewski P, Jeziorek M, Schuster CM, Bading H, Frost B, Dobrowolski R. The role of nuclear Ca2+ in maintaining neuronal homeostasis and brain health. J Cell Sci. (2021) 134:jcs254904. doi: 10.1242/jcs.254904
68. Khaldi MZ, Guiot Y, Gilon P, Henquin JC, Jonas JC. Increased glucose sensitivity of both triggering and amplifying pathways of insulin secretion in rat islets cultured for 1 wk in high glucose. Am J Physiol Endocrinol Metab. (2004) 287:E207–17. doi: 10.1152/ajpendo.00426.2003
69. Do OH, Low JT, Gaisano HY, Thorn P. The secretory deficit in islets from db/db mice is mainly due to a loss of responding beta cells. Diabetologia. (2014) 57:1400–9. doi: 10.1007/s00125-014-3226-8
70. Roe MW, Philipson LH, Frangakis CJ, Kuznetsov A, Mertz RJ, Lancaster ME, et al. Defective glucose-dependent endoplasmic reticulum Ca2+ sequestration in diabetic mouse islets of Langerhans. J Biol Chem. (1994) 269:18279–82. doi: 10.1016/S0021-9258(17)32299-8
71. Corbin KL, Waters CD, Shaffer BK, Verrilli GM, Nunemaker CS. Islet hypersensitivity to glucose is associated with disrupted oscillations and increased impact of proinflammatory cytokines in islets from diabetes-prone male mice. Endocrinology. (2016) 157:1826–38. doi: 10.1210/en.2015-1879
72. Chen C, Chmelova H, Cohrs CM, Chouinard JA, Jahn SR, Stertmann J, et al. Alterations in beta-cell calcium dynamics and efficacy outweigh islet mass adaptation in compensation of insulin resistance and prediabetes onset. Diabetes. (2016) 65:2676–85. doi: 10.2337/db15-1718
73. Stancill JS, Cartailler JP, Clayton HW, O’Connor JT, Dickerson MT, Dadi PK, et al. Chronic beta-Cell Depolarization Impairs beta-Cell Identity by Disrupting a Network of Ca2+-Regulated Genes. Diabetes. (2017) 66(8):2175–87. doi: 10.2337/db16-1355
74. Xu G, Chen J, Jing G, Shalev A. Preventing beta-cell loss and diabetes with calcium channel blockers. Diabetes. (2012) 61:848–56. doi: 10.2337/db11-0955
75. Arefanian H, Koti L, Sindhu S, Ahmad R, Al Madhoun A, Al-Mulla F. Verapamil chronicles: advances from cardiovascular to pancreatic beta-cell protection. Front Pharmacol. (2023) 14:1322148. doi: 10.3389/fphar.2023.1322148
76. Malayeri A, Zakerkish M, Ramesh F, Galehdari H, Hemmati AA, Angali KA. The effect of verapamil on TXNIP gene expression, GLP1R mRNA, FBS, hbA1c, and lipid profile in T2DM patients receiving metformin and sitagliptin. Diabetes Ther. (2021) 12:2701–13. doi: 10.1007/s13300-021-01145-4
77. Xu G, Chen J, Lu B, Sethupathy P, Qian WJ, Shalev A. Verapamil prevents decline of IGF-I in subjects with type 1 diabetes and promotes beta-cell IGF-I signaling. Diabetes. (2023) 72:1460–9. doi: 10.2337/db23-0256
78. Zhao D, Cao Y, Yu CG, Yuan SS, Zhang N, Zhang YY, et al. The association of calcium channel blockers with beta-cell function in type 2 diabetic patients: A cross-sectional study. J Clin Hypertens (Greenwich). (2019) 21:638–47. doi: 10.1111/jch.13517
79. Vogel J, Yin J, Su L, Wang SX, Zessis R, Fowler S, et al. A phenotypic screen identifies calcium overload as a key mechanism of beta-cell glucolipotoxicity. Diabetes. (2020) 69:1032–41. doi: 10.2337/db19-0813
80. Lee K, Kim J, Kohler M, Yu J, Shi Y, Yang SN, et al. Blocking ca(2+) channel beta3 subunit reverses diabetes. Cell Rep. (2018) 24:922–34. doi: 10.1016/j.celrep.2018.06.086
81. Miki T, Nagashima K, Tashiro F, Kotake K, Yoshitomi H, Tamamoto A, et al. Defective insulin secretion and enhanced insulin action in KATP channel-deficient mice. Proc Natl Acad Sci U S A. (1998) 95:10402–6. doi: 10.1073/pnas.95.18.10402
82. Shiota C, Larsson O, Shelton KD, Shiota M, Efanov AM, Hoy M, et al. Sulfonylurea receptor type 1 knock-out mice have intact feeding-stimulated insulin secretion despite marked impairment in their response to glucose. J Biol Chem. (2002) 277:37176–83. doi: 10.1074/jbc.M206757200
83. Seghers V, Nakazaki M, DeMayo F, Aguilar-Bryan L, Bryan J. Sur1 knockout mice. A model for K(ATP) channel-independent regulation of insulin secretion. J Biol Chem. (2000) 275:9270–7. doi: 10.1074/jbc.275.13.9270
84. Ravier MA, Nenquin M, Miki T, Seino S, Henquin JC. Glucose controls cytosolic Ca2+ and insulin secretion in mouse islets lacking adenosine triphosphate-sensitive K+ channels owing to a knockout of the pore-forming subunit Kir6.2. Endocrinology. (2009) 150:33–45. doi: 10.1210/en.2008-0617
85. Remedi MS, Rocheleau JV, Tong A, Patton BL, McDaniel ML, Piston DW, et al. Hyperinsulinism in mice with heterozygous loss of K(ATP) channels. Diabetologia. (2006) 49:2368–78. doi: 10.1007/s00125-006-0367-4
86. Abdulhadi-Atwan M, Bushman J, Tornovsky-Babaey S, Perry A, Abu-Libdeh A, Glaser B, et al. Novel de novo mutation in sulfonylurea receptor 1 presenting as hyperinsulinism in infancy followed by overt diabetes in early adolescence. Diabetes. (2008) 57:1935–40. doi: 10.2337/db08-0159
87. Vieira TC, Bergamin CS, Gurgel LC, Moises RS. Hyperinsulinemic hypoglycemia evolving to gestational diabetes and diabetes mellitus in a family carrying the inactivating ABCC8 E1506K mutation. Pediatr Diabetes. (2010) 11:505–8. doi: 10.1111/pdi.2010.11.issue-7
88. Osipovich AB, Stancill JS, Cartailler JP, Dudek KD, Magnuson MA. Excitotoxicity and overnutrition additively impair metabolic function and identity of pancreatic beta-cells. Diabetes. (2020) 69:1476–91. doi: 10.2337/db19-1145
89. Oduori OS, Murao N, Shimomura K, Takahashi H, Zhang Q, Dou H, et al. Gs/Gq signaling switch in beta cells defines incretin effectiveness in diabetes. J Clin Invest. (2020) 130:6639–55. doi: 10.1172/JCI140046
90. Imai Y, Cousins RS, Liu S, Phelps BM, Promes JA. Connecting pancreatic islet lipid metabolism with insulin secretion and the development of type 2 diabetes. Ann N Y Acad Sci. (2020) 1461(1):53–72. doi: 10.1111/nyas.14037
91. Cnop M, Ladriere L, Igoillo-Esteve M, Moura RF, Cunha DA. Causes and cures for endoplasmic reticulum stress in lipotoxic beta-cell dysfunction. Diabetes Obes Metab. (2010) 12 Suppl 2:76–82. doi: 10.1111/j.1463-1326.2010.01279.x
92. Keane KN, Cruzat VF, Carlessi R, de Bittencourt PI Jr., Newsholme P. Molecular events linking oxidative stress and inflammation to insulin resistance and beta-cell dysfunction. Oxid Med Cell Longev. (2015) 2015:181643. doi: 10.1155/2015/181643
93. Vilas-Boas EA, Almeida DC, Roma LP, Ortis F, Carpinelli AR. Lipotoxicity and β-cell failure in type 2 diabetes: Oxidative stress linked to NADPH oxidase and ER stress. Cells. (2021) 10:3328. doi: 10.3390/cells10123328
94. Hara T, Mahadevan J, Kanekura K, Hara M, Lu S, Urano F. Calcium efflux from the endoplasmic reticulum leads to beta-cell death. Endocrinology. (2014) 155:758–68. doi: 10.1210/en.2013-1519
95. Ly LD, Ly DD, Nguyen NT, Kim JH, Yoo H, Chung J, et al. Mitochondrial ca(2+) uptake relieves palmitate-induced cytosolic ca(2+) overload in MIN6 cells. Mol Cells. (2020) 43:66–75. doi: 10.14348/molcells.2019.0223
96. Motomura K, Matsuzaka T, Shichino S, Ogawa T, Pan H, Nakajima T, et al. Single-cell transcriptome profiling of pancreatic islets from early diabetic mice identifies anxa10 for ca2+ Allostasis toward beta-cell failure. Diabetes. (2024) 73:75–92. doi: 10.2337/db23-0212
97. Bano D, Ankarcrona M. Beyond the critical point: An overview of excitotoxicity, calcium overload and the downstream consequences. Neurosci Lett. (2018) 663:79–85. doi: 10.1016/j.neulet.2017.08.048
98. Kaufman BA, Li C, Soleimanpour SA. Mitochondrial regulation of beta-cell function: maintaining the momentum for insulin release. Mol Aspects Med. (2015) 42:91–104. doi: 10.1016/j.mam.2015.01.004
99. Ma ZA, Zhao Z, Turk J. Mitochondrial dysfunction and beta-cell failure in type 2 diabetes mellitus. Exp Diabetes Res. (2012) 2012:703538. doi: 10.1155/2012/703538
100. Perrone M, Patergnani S, Di Mambro T, Palumbo L, Wieckowski MR, Giorgi C, et al. Calcium homeostasis in the control of mitophagy. Antioxid Redox Signal. (2023) 38:581–98. doi: 10.1089/ars.2022.0122
101. Rovira-Llopis S, Banuls C, Diaz-Morales N, Hernandez-Mijares A, Rocha M, Victor VM. Mitochondrial dynamics in type 2 diabetes: Pathophysiological implications. Redox Biol. (2017) 11:637–45. doi: 10.1016/j.redox.2017.01.013
102. Medina DL, Di Paola S, Peluso I, Armani A, De Stefani D, Venditti R, et al. Lysosomal calcium signalling regulates autophagy through calcineurin and TFEB. Nat Cell Biol. (2015) 17:288–99. doi: 10.1038/ncb3114
103. Lira VA, Benton CR, Yan Z, Bonen A. PGC-1alpha regulation by exercise training and its influences on muscle function and insulin sensitivity. Am J Physiol Endocrinol Metab. (2010) 299:E145–61. doi: 10.1152/ajpendo.00755.2009
104. Besseiche A, Riveline JP, Gautier JF, Breant B, Blondeau B. Metabolic roles of PGC-1alpha and its implications for type 2 diabetes. Diabetes Metab. (2015) 41:347–57. doi: 10.1016/j.diabet.2015.02.002
105. Oropeza D, Jouvet N, Bouyakdan K, Perron G, Ringuette LJ, Philipson LH, et al. PGC-1 coactivators in beta-cells regulate lipid metabolism and are essential for insulin secretion coupled to fatty acids. Mol Metab. (2015) 4:811–22. doi: 10.1016/j.molmet.2015.08.001
106. Besseiche A, Riveline JP, Delavallee L, Foufelle F, Gautier JF, Blondeau B. Oxidative and energetic stresses mediate beta-cell dysfunction induced by PGC-1alpha. Diabetes Metab. (2018) 44:45–54. doi: 10.1016/j.diabet.2017.01.007
107. Kim-Muller JY, Zhao S, Srivastava S, Mugabo Y, Noh HL, Kim YR, et al. Metabolic inflexibility impairs insulin secretion and results in MODY-like diabetes in triple FoxO-deficient mice. Cell Metab. (2014) 20:593–602. doi: 10.1016/j.cmet.2014.08.012
108. Zhang S, Hulver MW, McMillan RP, Cline MA, Gilbert ER. The pivotal role of pyruvate dehydrogenase kinases in metabolic flexibility. Nutr Metab (Lond). (2014) 11:10. doi: 10.1186/1743-7075-11-10
109. Evans-Molina C, Hatanaka M, Mirmira RG. Lost in translation: endoplasmic reticulum stress and the decline of beta-cell health in diabetes mellitus. Diabetes Obes Metab. (2013) 15 Suppl 3:159–69. doi: 10.1111/dom.12163
110. Wang Q, Groenendyk J, Michalak M. Glycoprotein quality control and endoplasmic reticulum stress. Molecules. (2015) 20:13689–704. doi: 10.3390/molecules200813689
111. Liu K, Paterson AJ, Chin E, Kudlow JE. Glucose stimulates protein modification by O-linked GlcNAc in pancreatic beta cells: linkage of O-linked GlcNAc to beta cell death. Proc Natl Acad Sci U S A. (2000) 97:2820–5. doi: 10.1073/pnas.97.6.2820
112. Hart GW. Nutrient regulation of signaling and transcription. J Biol Chem. (2019) 294:2211–31. doi: 10.1074/jbc.AW119.003226
113. Dissanayake WC, Sorrenson B, Shepherd PR. The role of adherens junction proteins in the regulation of insulin secretion. Biosci Rep. (2018) 38(2):BSR20170989. doi: 10.1042/BSR20170989
114. Idevall-Hagren O, Incedal Nilsson C, Sanchez G. Keeping pace: the primary cilium as the conducting baton of the islet. Diabetologia. (2024) 67:773–82. doi: 10.1007/s00125-024-06096-6
115. Wang Z, Thurmond DC. Mechanisms of biphasic insulin-granule exocytosis - roles of the cytoskeleton, small GTPases and SNARE proteins. J Cell Sci. (2009) 122:893–903. doi: 10.1242/jcs.034355
116. Gan WJ, Zavortink M, Ludick C, Templin R, Webb R, Webb R, et al. Cell polarity defines three distinct domains in pancreatic beta-cells. J Cell Sci. (2017) 130:143–51. doi: 10.1242/jcs.185116
117. Talchai C, Xuan S, Lin HV, Sussel L, Accili D. Pancreatic beta cell dedifferentiation as a mechanism of diabetic beta cell failure. Cell. (2012) 150:1223–34. doi: 10.1016/j.cell.2012.07.029
118. Cinti F, Bouchi R, Kim-Muller JY, Ohmura Y, Sandoval PR, Masini M, et al. Evidence of beta-cell dedifferentiation in human type 2 diabetes. J Clin Endocrinol Metab. (2016) 101:1044–54. doi: 10.1210/jc.2015-2860
119. Dhawan S, Tschen SI, Zeng C, Guo T, Hebrok M, Matveyenko A, et al. DNA methylation directs functional maturation of pancreatic beta cells. J Clin Invest. (2015) 125:2851–60. doi: 10.1172/JCI79956
120. Pullen TJ, Rutter GA. When less is more: the forbidden fruits of gene repression in the adult beta-cell. Diabetes Obes Metab. (2013) 15:503–12. doi: 10.1111/dom.12029
121. Esguerra JL, Eliasson L. Functional implications of long non-coding RNAs in the pancreatic islets of Langerhans. Front Genet. (2014) 5:209. doi: 10.3389/fgene.2014.00209
122. Son J, Du W, Esposito M, Shariati K, Ding H, Kang Y, et al. Genetic and pharmacologic inhibition of ALDH1A3 as a treatment of beta-cell failure. Nat Commun. (2023) 14:558. doi: 10.1038/s41467-023-36315-4
123. Son J, Ding H, Farb TB, Efanov AM, Sun J, Gore JL, et al. BACH2 inhibition reverses beta cell failure in type 2 diabetes models. J Clin Invest. (2021) 131(24):e153876. doi: 10.1172/JCI153876
124. Vasconcelos FF, Castro DS. Transcriptional control of vertebrate neurogenesis by the proneural factor Ascl1. Front Cell Neurosci. (2014) 8:412. doi: 10.3389/fncel.2014.00412
125. Imayoshi I, Isomura A, Harima Y, Kawaguchi K, Kori H, Miyachi H, et al. Oscillatory control of factors determining multipotency and fate in mouse neural progenitors. Science. (2013) 342:1203–8. doi: 10.1126/science.1242366
126. Park NI, Guilhamon P, Desai K, McAdam RF, Langille E, O’Connor M, et al. ASCL1 reorganizes chromatin to direct neuronal fate and suppress tumorigenicity of glioblastoma stem cells. Cell Stem Cell. (2017) 21:209–24 e7. doi: 10.1016/j.stem.2017.06.004
127. Ball DW. Achaete-scute homolog-1 and Notch in lung neuroendocrine development and cancer. Cancer Lett. (2004) 204:159–69. doi: 10.1016/S0304-3835(03)00452-X
128. Kokubu H, Ohtsuka T, Kageyama R. Mash1 is required for neuroendocrine cell development in the glandular stomach. Genes Cells. (2008) 13:41–51. doi: 10.1111/j.1365-2443.2007.01146.x
129. Huber K, Bruhl B, Guillemot F, Olson EN, Ernsberger U, Unsicker K. Development of chromaffin cells depends on MASH1 function. Development. (2002) 129:4729–38. doi: 10.1242/dev.129.20.4729
130. Ackermann AM, Wang Z, Schug J, Naji A, Kaestner KH. Integration of ATAC-seq and RNA-seq identifies human alpha cell and beta cell signature genes. Mol Metab. (2016) 5:233–44. doi: 10.1016/j.molmet.2016.01.002
131. Osipovich AB, Zhou FY, Chong JJ, Trinh LT, Cottam MA, Shrestha S, et al. Deletion of Ascl1 in pancreatic beta-cells improves insulin secretion, promotes parasympathetic innervation, and attenuates dedifferentiation during metabolic stress. Mol Metab. (2023) 78:101811. doi: 10.1016/j.molmet.2023.101811
Keywords: type 2 diabetes, pancreatic β-cells, Ca2+ signaling, metabolic stress, dedifferentiation, gene expression
Citation: Magnuson MA and Osipovich AB (2024) Ca2+ signaling and metabolic stress-induced pancreatic β-cell failure. Front. Endocrinol. 15:1412411. doi: 10.3389/fendo.2024.1412411
Received: 04 April 2024; Accepted: 10 June 2024;
Published: 02 July 2024.
Edited by:
Xiaoqing Tang, Michigan Technological University, United StatesReviewed by:
Zong Wei, Mayo Clinic Arizona, United StatesCopyright © 2024 Magnuson and Osipovich. This is an open-access article distributed under the terms of the Creative Commons Attribution License (CC BY). The use, distribution or reproduction in other forums is permitted, provided the original author(s) and the copyright owner(s) are credited and that the original publication in this journal is cited, in accordance with accepted academic practice. No use, distribution or reproduction is permitted which does not comply with these terms.
*Correspondence: Mark A. Magnuson, bWFyay5tYWdudXNvbkB2YW5kZXJiaWx0LmVkdQ==
Disclaimer: All claims expressed in this article are solely those of the authors and do not necessarily represent those of their affiliated organizations, or those of the publisher, the editors and the reviewers. Any product that may be evaluated in this article or claim that may be made by its manufacturer is not guaranteed or endorsed by the publisher.
Research integrity at Frontiers
Learn more about the work of our research integrity team to safeguard the quality of each article we publish.