- 1Research Center of the Institut Universitaire en Santé Mentale de Montréal, Montreal, QC, Canada
- 2Department of Psychology, University of Quebec in Montreal, Montreal, QC, Canada
- 3Department of Medicine, University of Montreal, Montreal, QC, Canada
Background: Endogenous sex hormones and oral contraceptives (OCs) have been shown to influence key regions implicated in fear processing. While OC use has been found to impact brain morphology, methodological challenges remain to be addressed, such as avoiding selection bias between OC users and non-users, as well as examining potential lasting effects of OC intake.
Objective: We investigated the current and lasting effects of OC use, as well as the interplay between the current hormonal milieu and history of hormonal contraception use on structural correlates of the fear circuitry. We also examined the role of endogenous and exogenous sex hormones within this network.
Methods: We recruited healthy adults aged 23-35 who identified as women currently using (n = 62) or having used (n = 37) solely combined OCs, women who never used any hormonal contraceptives (n = 40), or men (n = 41). Salivary endogenous sex hormones and current users’ salivary ethinyl estradiol (EE) were assessed using liquid chromatography – tandem mass spectrometry. Using structural magnetic resonance imaging, we extracted surface-based gray matter volumes (GMVs) and cortical thickness (CT) for regions of interest of the fear circuitry. Exploratory whole-brain analyses were conducted with surface-based and voxel-based morphometry methods.
Results: Compared to men, all three groups of women exhibited a larger GMV of the dorsal anterior cingulate cortex, while only current users showed a thinner ventromedial prefrontal cortex. Irrespective of the menstrual cycle phase, never users exhibited a thicker right anterior insular cortex than past users. While associations with endogenous sex hormones remain unclear, we showed that EE dosage in current users had a greater influence on brain anatomy compared to salivary EE levels and progestin androgenicity, with lower doses being associated with smaller cortical GMVs.
Discussion: Our results highlight a sex difference for the dorsal anterior cingulate cortex GMV (a fear-promoting region), as well as a reduced CT of the ventromedial prefrontal cortex (a fear-inhibiting region) specific to current OC use. Precisely, this finding was driven by lower EE doses. These findings may represent structural vulnerabilities to anxiety and stress-related disorders. We showed little evidence of durable anatomical effects, suggesting that OC intake can (reversibly) affect fear-related brain morphology.
1 Introduction
Fear is a universal and adaptative emotion (1) but excessive fear reactions can be deleterious to the psychological and social functioning of individuals (2, 3). Dysfunctions resulting from poor fear regulation characterize anxiety disorders and post-traumatic stress disorder (PTSD) (4–6), where behavioral clinical treatments rely on the concept of fear extinction (i.e., safety learning, 4, 5, 7–11). As deficits in fear extinction have been identified in these psychopathologies, impaired extinction may promote the maintenance of anxiety symptoms (12–15).
Anxiety and stress-related disorders are more common in women (3, 16) but our understanding of this elevated vulnerability is limited. This knowledge gap is in part be due to the exclusion of females and women in animal and human science. Indeed, anxiety research has been oriented towards males and men (the so-called ‘male bias’ phenomenon). As of 2012, less than 2% of fear-related publications were conducted on female brains (6). This bias remains apparent in recent research where 65% of 2021 preclinical anxiety models were studied in males only (17). From 2009 to 2019, neuroscience and psychiatry publications showed a considerable increase in the inclusion of both sexes, although the proportion of male-only papers did not decrease. In fact, male-only manuscripts are 9 times more common than female-only papers in neuroscience and psychiatry journals (18). Female underrepresentation is mainly driven by the assumption that sex hormone fluctuations would lead to increased variability in results, albeit this argument has not been empirically supported (17, 19, 20).
1.1 Modulation of the fear circuitry by sex hormones
Scientific attention allocated to topics concerning women enabled researchers to connect endocrine activity to anxiety. Indeed, paradigms using naturally-fluctuating hormones (e.g., estradiol [E2] and progesterone [P]) or synthetic hormones (e.g., oral contraceptives [OCs]) have revealed that sex hormones modulate anxiety behaviors (for reviews see 16, 21, 22). Endogenous sex hormones are the end result of the endocrine cascade of the hypothalamic-pituitary-gonadal (HPG) axis and can fluctuate according to the menstrual cycle. That said, used by more than 150 million women worldwide (23), OCs act as a powerful disruptor of the menstrual cycle. Combined OCs (COCs) are the most common type and are made up of synthetic estrogen (e.g., ethinyl estradiol [EE]) with a progestin (e.g., levonogestrel). These exogenous hormones bind to estrogen and P receptors in brain regions involved in the HPG axis, thereby suppressing sex hormone secretion via negative feedback (24–26). Additionally, EE induces hepatic synthesis of the sex hormone binding globulin (SHBG), reducing not only free (unbound) E2 and P levels but also free testosterone (T) (27).
Notably, lowered endogenous E2 levels (either naturally occurring during the early follicular phase of the menstrual cycle or induced by OC use) is a key contributor to fear maintenance as evidenced using fear conditioning and extinction protocols (28–30). At the neural level, sex hormones are known to modulate the brain network implicated in fear processes (28, 31–34). Major brain regions of the fear circuitry include the amygdala, hippocampus, hypothalamus, anterior insular cortex (AIC), dorsal anterior cingulate cortex (dACC), rostral anterior cingulate cortex (rACC), and ventromedial prefrontal cortex (vmPFC) (32, 35–40). Particularly, the amygdala, hippocampus, and vmPFC express high levels of sex hormone receptors and as such, provide insight into how sex hormones exert their influence on fear (6, 28, 41, 42).
Fluctuations in sex hormones have been shown to influence brain activity of the fear circuitry (for reviews see 28, 31, 32). Human neuroimaging studies have associated high E2 with increased activations within this network (33, 43, 44), though fewer studies have investigated the role of sex hormones on its anatomy. The most consistent observation of the latter is the trophic anatomical effects of E2 in the hippocampus (45–48). Although less consistently reported, morphologic changes of fear-related regions have been reported throughout the menstrual cycle (e.g., changes in the dACC (49), AIC (50), and amygdala (51)). OC use has also been associated with a reduction of the hippocampus (52–54), amygdala (55), hypothalamus (56), and prefrontal cortical thickness (57, 58). Additionally, ‘acute’ sex hormone concentrations have been shown to correlate with brain structures (59). For instance, fear-related brain regions have been associated with salivary or serum E2 and T in various hormonal profiles (i.e., OC users, naturally cycling [NC] women, men) (49, 53, 57). Given the scarce literature on the topic and lack of replicability, these associations call for further empirical support. Nevertheless, these findings collectively support the involvement of endogenous and exogenous sex hormones on key regions implicated in fear processing and regulation, as well as their ability to engender significant structural alterations within this network.
1.2 Challenges in studying oral contraceptives
The impact of OCs on brain morphology has mainly been studied by comparing current users to NC women (according to specific phases of the menstrual cycle or not) and men (for reviews see 59–61). One of the most important methodological limitations in this type of design is the sample selection bias. Indeed, a ‘survivor effect’ may linger in samples of current OC users, knowing that women for whom the pill does not cause adverse effects are more likely to continue using it (62). Discontinuation of OCs has also been associated with psychiatric symptoms or disorders (63, 64), neuroticism (65), and mood-related side effects (66). Thereby, NC women that are previous OC users could constitute their own group that is distinct from never and current users. However, a recent investigation reported negligible differences between current, past, and never users in sociodemographic variables and personality in a matched Eastern European sample (67). Nonetheless, given that the study of OCs is normally done using cross-sectional designs between users and non-users, careful attention must be paid to the choice of comparison groups.
The vast majority of research on OCs has focused on acute (i.e., ‘here and now’, activational) effects underlying its use. Yet, hormonal events have been shown to exert long-lasting neural changes, as reported in the context of puberty (68, 69), pregnancy (70–72), and menopausal hormonal therapy (73, 74). Regarding OC use, a paucity of studies distinguishing current, past, and never users suggests that OC intake could lead to long-term effects on endogenous sex hormone concentrations (75), brain structures (76, 77), cognition (78, 79), and anxiety symptoms (as experienced during the COVID-19 pandemic, 80). It is still unclear whether such lasting effects exist and if so, by which mechanisms OCs exert their influence after cessation. Still, it appears obvious that the simple comparison of OC users and NC women would generate its share of heterogeneity, as grouping NC women would most likely comprise past and never users.
Generally, studies have homogenized their subset of OC users by selecting COC users only (i.e., excluding progestin-only OC users). If there has been a keen interest over time to refine methodologies to the specific use of COCs, very few studies have considered women’s lifetime history of hormonal contraceptives. Yet, as previously stated, an increasing body of research points to differential effects between current users, past users, and never users (77, 78, 80–82). Knowing that women commonly try several types of hormonal contraceptives in their lifetime, this factor may introduce unwanted noise. Therefore, when examining current and past COC users, rigorous methodological standards can be achieved by considering the history of hormonal contraceptive use. To our knowledge, only two studies have considered contraception history and restricted their sample to women who had only used OCs or COCs throughout their lifetime (76, 83).
Relatedly, COCs is a broad category composed of more than 30 formulations with varying doses and/or synthetic compound compositions (24, 84). Doses of EE range from 10 to 50μg, although COCs containing 35μg of EE or less are the most commonly used. While EE is typically the estrogenic constituent in COCs, progestins (and their dosage) are heterogenous and differ according to their chemical structure and pharmacodynamics (85). Androgenic activity is commonly used to classify progestins and as such, can be classified as high (e.g., levonogestrel), low (e.g., norgestimate, desogestrel), or anti-androgenic (e.g., drospirenone, cyproterone acetate) progestins (24, 86). Of note, androgenicity effects have been examined on cognitive functions (87–91) and neural correlates (79, 82, 83, 92). Anatomically, a larger volume of the bilateral fusiform face area was found in anti-androgenic users compared to androgenic users, while EE dose did not correlate with this region (82). Furthermore, the effects of exogenous hormone concentrations on brain structures have not been reported to date. Synthetic compounds (e.g., EE) are not captured by traditional immunoassays of circulating E2 in serum or saliva, meaning that the current literature has only partly depicted the endocrine activity of OC users (84).
Finally, many metrics to quantify brain morphology have emerged over the years. While this represents a fundamental strength for the field of neuroscience, it has also inevitably led to heterogeneous findings. Structural imaging studies have used different measures to assess brain tissues such as gray or white matter volume, cortical thickness (CT), cortical surface area, and folding/gyrification (61, 93, 94). Voxel-based morphometry (VBM) has traditionally been the most popular method to quantify gray matter volume (GMV), though it can also be measured using surfaced-based morphometry (SBM; as the product of CT and surface area for cortical GMV). However, it is well known that changes in GMV are unspecific and can result in differing interpretations (e.g., changes in CT, folding, or surface area of the cortex) (61, 95). Interestingly, GMV is the most frequent metric used to quantify OC changes in the brain (61) and only one group has investigated the impact of OCs on other gray matter (GM) phenotypes, namely CT (57, 58).
Despite the growing interest in studying women’s health, much remains to be discovered about the impact of sex hormones and OCs on the brain, cognition, behavior, and mental health. In psychoneuroendocrinology, there is a lack of consensus with regard to the effects of sex hormones and OCs, mostly due to the relatively small number of studies and heterogeneous methodologies used (96–102). This study aimed to investigate the current and potentially lasting effects of COC use, as well as the role of endogenous and exogenous sex hormones on structural correlates of the fear circuitry. We recruited women currently using or having used solely COCs in their lifetime, women who never used any hormonal contraceptives, and men. Using structural magnetic resonance imaging (MRI), we extracted GMV and CT of key regions of the fear circuitry. Comparing current users, past users, never users, and men allowed us to examine whether COC use was associated with acute (i.e., current users vs. never users = past users) or long-term (i.e., current users = past users vs. never users) morphologic alterations, as well as to detect sex differences. Additionally, we conducted analyses to explore the associations between endogenous sex hormones and brain morphology in past users, never users, and men, as well as with EE (salivary levels and prescribed doses) and androgenicity in current COC users.
2 Materials and methods
2.1 Participants and procedure
This study stems from a larger research project investigating the neural, cognitive, and endocrine correlates of COC use, which involved three laboratory sessions. Participants were all healthy adults aged between 23 and 35 years old. This minimal age criterion was set to limit brain variability due to late-adolescence maturation (103, 104) without compromising recruitment feasibility (i.e., undergraduates were still allowed to apply). According to their hormonal profile and COC history, participants were divided into groups of men, never users, past users, and current users. Eligibility was confirmed after a thorough phone screening, ensuring that participants from all groups met the following inclusion/exclusion criteria: French speakers, no past or current medical/psychological diagnosis, no current or past pregnancy, no usage of medication affecting the endocrine system (other than COCs), and no regular usage of drugs and alcohol. Participants also completed an MRI screening questionnaire for safety purposes (e.g., no metallic implant, claustrophobia). For COC criteria, past COC users were required to have used their COC and have stopped it for over a year (Mduration = 6.74 years ± 3.21; Mcessation= 3.37 years ± 2.15). Current COC users had to be using it for at least 3 months (M = 8.97 years ± 3.83). Regarding contraception history, past and current users must not have used other types of hormonal contraceptives than COCs in their lifetime (i.e., progestin-only pill, intra-uterine device, patch, vaginal ring, injection, or implant). Never users must not have used any hormonal contraceptives in their lifetime. As for NC women, never users and past users were expected to have a regular menstrual cycle (M = 29.26 days ± 3.01; normal cycle length of 21-35 days, 105). As these women monitored their menstrual cycles, laboratory sessions were scheduled during either the early follicular phase (day 1-5 of the menstrual cycle, where day 1 corresponds to the onset of menses) or the pre-ovulatory phase (1-4 days before ovulation, where ovulation day was calculated as average cycle length minus 14). One never user had a cycle length longer than 35 days and two women (one past user, one never user) were not able to quantify their cycle length, they were therefore scheduled during the early follicular phase. Given that E2 has been found to have a marked influence on the fear circuitry (see section 1.1.), these two cycle phases were selected due to their distinct E2 concentrations. This methodological detail allowed us to investigate the role of cycle phases in NC women, as well as to control for hormone levels within this group of women (i.e., equivalent distributions of cycle phases between both groups).
Data for the present manuscript were collected during the second session of the larger study. Before entering the MRI scanner, saliva samples were collected for later quantification of sex hormone levels. In the MRI scanner, participants first underwent a structural sequence before going through a task for functional sequences. All sessions were scheduled between 8:15AM and 7PM. This study was reviewed and approved by the research ethics board of the Centre intégré universitaire de santé et de services sociaux de l’Est-de-l’Île-de-Montréal. Individuals provided written consent before participating in this study, had the right to terminate the experiment at any time, and were offered monetary compensation for their time. A total of 181 participants completed the procedure for the present study.
2.2 Questionnaires
The following questionnaires were completed by participants to evaluate potential confounds between our groups and on structural outcomes. Importantly, these constructs have all been associated with COC use and/or brain morphology (65, 106–111). Participants completed the 1) Beck Depression Inventory-II (BDI), a 21-item questionnaire assessing physiological and psychological symptoms related to depression (range 0-63, 112, 113), 2) Brief Trauma Questionnaire (BTQ), a 10-item questionnaire derived from the Brief Trauma Interview (114) that evaluates previous exposure to stressful/traumatic events (115), 3) Neuroticism scale from the NEO-Five Factor Inventory-3, a 12-item scale measuring the tendency towards negative affect and emotional instability (range 12-60, 116), 4) Santa Clara Strength of Religions Faith Questionnaire (SCSRFQ), 10 questions regarding religiosity (range 10-40, 117), and 5) trait form of the State and Trait Anxiety Questionnaire (STAI-T), which measures anxiety as a personality trait through 20 items (range 20-80, 118, 119). All questionnaires (except the BDI) were answered online on Qualtrics XM between the first and second sessions of the larger study. The BDI was completed at the end of the third session and participants scoring ≥ 14 (i.e., mild depression symptomatology) were offered psychological resources.
2.3 Sex hormone assessment
Salivary sex hormone concentrations were collected using the passive drool method. A 2mL sample was provided in a salivette by all participants and stored temporarily in a -20°C freezer at the MRI facility. Within a period of 2 months, samples were transferred to a -80°C freezer at our research center for long-term conservation. Upon the time of analysis, samples were shipped with dry ice to ZRT Laboratory (Beaverton, Oregon, USA), a lab specialized in salivary assays of sex hormones using liquid chromatography – tandem mass spectrometry (LC-MS/MS). Assays were run with an AB Sciex Triple Quad 5500 system. Saliva was mixed with internal standards, then extraction of steroids was performed by Cl 8 column chromatography. Steroids were eluted from the solid phase extraction and dried under nitrogen. Derivatization was carried out on dried samples (120), after which they were diluted and injected for LC-MS/MS analysis with analytical separation performed on an Agilent Poroshell 120 EC-C8 column and ionization by atmospheric pression chemical ionization (121). The lower limit of quantification (LLOQ) was 0.30 pg/mL for E2, 5 pg/mL for P, 3 pg/mL for T, and 0.40 pg/mL for EE. Given that we had data on which participants were expected to truly have circulating EE levels (i.e., COC users in the active phase of their regimen), we used the lower limit of detection (LOD) of 0.11 pg/mL for EE values below the LLOQ.
2.4 MRI data acquisition
Structural images were acquired on a Siemens Magnetom Prisma 3T scan using a 64-channel coil. A T1-weighted Multi-Echo Magnetization-Prepared Rapid Gradient-Echo (MEMPRAGE) sequence was used to collect 176 high-resolution images (TR = 2530ms, TE = 1.62ms, FOV = 176mm, flip angle = 7°, voxel size = 1mm3). Visual inspection for motion artifacts was performed on raw data for each participant based on a 4-point scale protocol (122; https://github.com/CoBrALab/documentation/wiki/Motion-Quality-Control-(QC)-Manual). This procedure resulted in the exclusion of one participant (past COC user).
2.5 Surface-based morphometry
Raw anatomical images were processed using FreeSurfer (http://surfer.nmr.mgh.harvard.edu). The recon-all pipeline of Freesurfer 6.0 was performed on CBRAIN, an online open-source software (123) and allowed for the automatic generation of a 3D cortical surface model. Segmentation of white matter (WM) and GM (pial) surfaces was visually inspected for every participant. No major flaws were detected by visual inspection (e.g., WM/pial surface segmentation into the skull or ventricles).
We extracted both GMV and CT phenotypes for regions of interest (ROI)-based analyses. The Destrieux atlas (124, 125) was used for the parcellation of cortical ROIs. To select precise parcels of the fear circuitry, we inspected the brain map for the term ‘fear’ on Neurosynth (neurosynth.org), an online platform for the automated synthesis of functional MRI data. Using peak coordinates, we identified which Destrieux’s labels overlapped with fear-related clusters. The dACC was best delimited by the anterior part of the middle cingulate gyrus and sulcus (aMCC, label#7), which was further validated by Vogt et al. (126) as being involved in fear expression. We defined the rACC as the anterior part of the cingulate gyrus and sulcus (ACC, label #6). For the AIC, we combined the short insular gyrus (label #18) and anterior segment of the circular sulcus of the insula (label #47). Finally, the vmPFC was defined as the sum of the straight gyrus (gyrus rectus, label #31) and suborbital sulcus (label #70). At the subcortical level, GMV of the amygdala and hippocampus were obtained from FreeSurfer’s segmentation. The hypothalamus was segmented using the automated tool of Billot et al. (127). GMV of the whole structure was used. All ROIs were extracted by hemisphere. Exploratory vertex-by-vertex whole-brain analyses (WBA) were conducted for cortical GMV and CT using a smoothing full-width half maximum (FWHM) kernel of 10mm and 20mm, respectively.
2.6 Voxel-based morphometry
Additionally, we performed VBM as a secondary analytic method. Data were analyzed using Matlab (R2018b, Mathworks Inc.) and SPM12 (http://www.fil.ion.ucl.ac.uk/spm). Images were reoriented along the anterior-posterior commissure line and the anterior commissure was set as the coordinate origin. Images were segmented into GM, WM, and cerebrospinal fluid (Ashburner and Friston, 2005). The Diffeomorphic Anatomical Registration Through Lie Algebra (DARTEL) algorithm was used for estimating deformations and increasing the accuracy of between-subjects alignment (Ashburner, 2007). Images were modulated and spatially normalized to the Montreal Neurological Institute (MNI) space. Normalized GM images were smoothed with a FWHM kernel of 8 mm. GM voxels with a value < 0.2 were excluded from statistical analyses. Global normalization was set at proportional scaling to account for TIV.
2.7 Analytic approach
In the present study, we examined two phenotypes of the GM, namely volume and thickness. As volume is an unspecific feature of the GM (95), we also measured CT to refine the interpretation of the results. Further, we used two volumetric methods to generate surface-based and voxel-based GMV. Given the well-documented difficulty of low reproducibility in MRI studies (128–130), this motivated our team to conduct analyses not only in FreeSurfer but in SPM using VBM to evaluate the robustness of analytical variability of GMV results. As such, between-software convergence would strengthen confidence in the obtained results (130). Moreover, given that FreeSurfer’s volumetric WBA is restricted to the cortex, the use of SPM’s VBM allowed us to examine cortical, subcortical, and cerebellar voxels.
Regarding endocrine quantification, the use of LC-MS/MS resulted in a considerable rate of levels falling under the LLOQ. Left-censored undetected values were high for E2 (< 0.3 pg/mL: 110/180, 61.1%), P (< 5 pg/mL: 125/180, 69.4%), and moderate for T (< 3 pg/mL: 51/180, 28.3%). This small proportion of detected values was also reported with LC-MS/MS for salivary E2 (131). Nonetheless, undetected values followed a coherent pattern with nearly all of them being distributed in expected low hormonal profiles. Frequencies of undetected E2 were distributed as follows: 57 COC users, 27 NC women in the early follicular phase, and 23 men (107/110, 97.27%). Given that the luteal phase of the menstrual cycle was not targeted in NC women, all our participants were recruited in a low state of P. This provides insight into the high undetected P rate in every group (≥ 64.5%). For T, 39 COC users, 11 early follicular NC women, and 1 pre-ovulatory NC woman accounted for the undetected values. For COC users in the active phase of their regimen (n = 55), EE levels were below the LOD for 8 women (14.5%). Given that our missing values were not missing at random (NMAR) and as recommended by Herbers et al. (132), we addressed this issue with multiple imputation from a fitted lognormal distribution using their same procedure implemented in R with the fitdistrplus and EnvStats packages (133, 134; the code used by Hebers et al. is accessible at https://osf.io/spgtv/). This imputation method was found to be valid, despite the high proportion of missingness in left-censored datasets (135). Using the SPSS toolbox, we generated 10 datasets with simulated values between 0.01 pg/mL and the LLOQ for each endogenous hormone (e.g., 0.01-0.29 pg/mL for E2). The LOD was used as the upper bound for EE. For statistics, we reported the mean value of each metric (e.g., FM = average of the 10 Fs). Due to highly unbalanced distributions and to be coherent with the imputation procedure performed, we then log-transformed endocrine variables. Finally, outliers were examined. Z-scores were derived for men, early follicular NC women, pre-ovulatory NC women, and COC users on log-transformed variables. Using a criterion of ≥ ± 3.29 (136, 137), we identified two outliers for E2 (COC users), one outlier for P (COC user), two outliers for T (pre-ovulatory NC woman and man), and one outlier for EE. All outliers were winsorized with respect to groups, using the next highest value of each group (138) on the imputed log-transformed variables. Additionally, we removed endocrine values for one participant (man) due to an invalid assessment (technical issue/low internal standard). For descriptive purposes only, we winsorized imputed raw (untransformed) values and reported endocrine concentrations in pg/mL.
All ROI analyses were performed using SPSS 27 (IBM). We conducted general linear models (GLMs) for each ROI with Group (men, never users, past users, current users) as the between-subjects factor and Hemisphere (left, right) as the within-subjects factor. We controlled for age for both GMV and CT analyses, as well as for TIV in GMV analyses (for a justification, see 139, 140). Given our between-group design, we examined further potential confounders thereafter. Covariates were selected from analyses performed on various sociodemographic and psychological outcomes with a threshold set at p <.10. First, study groups were compared using ANOVAs and chi-square tests for continuous and categorical variables, respectively. Second, correlations were performed between potential confounders and TIV/global CT. Using the ‘disjunctive cause criterion’ approach for covariate selection (141), we selected variables that were either associated with the study groups, brain morphology (TIV/global CT), or both. Thus, we decided to initially run the minimally adjusted models (e.g., age and TIV for GMV) and re-run the fully adjusted models for additional confounders (e.g., ethnicity, relationship status, religiosity). To investigate the interplay between current dynamics of the menstrual cycle and history of COC use, we focused on both groups of NC women (namely, never users and past users, as well as the cycle phase they were in during data collection). Accordingly, we performed Group (past users, never users) x Cycle phase (early follicular, pre-ovulatory) GLMs. Similar to the previous model, we implemented the same covariate selection plan.
To investigate the impact of endogenous sex hormones, we re-ran previous Group x Hemisphere GLMs for each ROI with added hormonal concentrations. Given that these specific analyses relied on the continuous nature of hormonal data, we excluded variables showing a proportion of imputation higher than 60%. This procedure led to the exclusion of COC users in this set of analyses and P levels for the three remaining groups (i.e., men, never users, past users). Conceptually, this decision is supported by the fact that COC users should be in an endogenous ‘shut-down’ state and that P variability was expected to be minimal across participants (due to the nature of our study design). As such, models were built to explore the effects of Group (3) x Hemisphere (2) x E2 and Group (3) x Hemisphere (2) x T, all while controlling for age (and TIV for GMV). In a second step, significant results were re-examined by running a sensitivity analysis with subcategorized hormonal data (e.g., ‘low levels’ = undetected imputed values, ‘medium levels’ = detected but low values, ‘high’ = detected and higher values). This was performed to assess the robustness of the results obtained with multiple imputations. No WBA was performed for this analysis subset given that multiple imputation could not be implemented in FreeSurfer and SPM.
In COC users, we explored the role of exogenous hormones as assessed by salivary EE levels or EE prescribed dose (contained in each pill, in μg) and androgenicity. As EE levels were accounted for, EE models were restricted to women tested in the active phase of their COC (n = 55). Among them, 48 used monophasic regimens, while seven used triphasic regimens with a constant dose of EE across all active pills. Given the few and unevenly distributed data points for EE dose (as reported in Table 1), we grouped users into low (10-25μg) or high (30-35μg) dosages. In our sample, no COC users used 50μg of EE. For each ROI, EE GLMs were tested with Hemisphere (left, right) x EE dose (low, high) and Hemisphere x EE levels as variables of interest, where age and TIV (for GMV only) were used as covariates. Of note, we did not investigate the impact of lifetime EE dose in current users or previous EE dose within past users given that these calculations led to insufficient sample sizes (i.e., 33/62 current users and 14/37 past users having used the same dosage over lifetime use). As for progestin androgenicity, we classified users into high (n = 27), low (n = 18), and anti-androgenic (n = 17) COCs categories (24) (see Table 1). The influence of androgenicity was explored with Hemisphere (right, left) x Androgenicity (anti, low, high) GLMs, while controlling for age and TIV (for GMV only). Considering the small sample size, we first ran separate models for EE and progestin androgenicity. However, EE dosage and progestin androgenicity can vary within formulations and potentially interact with each other. Indeed, the chi-square test showed that a higher proportion of low EE doses were classified as high androgenic COCs, while high EE doses were more likely to be classified as low androgenic COCs (p = .004). Therefore, in a second step, we adjusted for one or the other in their respective set of analyses. Although statistically underpowered, this allowed us to verify the stability of our results when covarying out an important confounder.
For ROI-based analyses, significance levels were set at p <.05. Trend-level results were defined as.05 ≤ p ≤.08. Bonferroni corrections were applied to p-values in post hoc analyses. Based on the novelty of the study, uncorrected results were presented for multiple comparisons; however, adjustment for multiple comparisons was applied for the seven GMV ROIs and four CT ROIs using the False Discovery Rate (FDR) correction (142). For exploratory volumetric and thickness WBA in FreeSurfer, the Monte Carlo Null-Z Simulation was carried out to control for multiple comparisons (10 000 iterations, cluster-forming two-tailed p <.05, cluster-wise p <.05). A Bonferroni correction was applied as we tested both hemispheres. For VBM, we used a primary uncorrected threshold of p <.001 with a cluster extent of k > 10 voxels and secondary cluster-level FDR-corrected threshold of q <.05. Groups were compared using a F-test orthogonal contrast. Effect sizes were reported as partial eta squared (ηp2). Error bars in figures denote ± standard error of the mean.
3 Results
3.1 Preliminary analyses
To account for potential diurnal variations in sex hormone levels (143–145), we examined whether the timing of study sessions, which ranged from 8:15AM to 7PM as a matter of feasibility, influenced endocrine concentrations. Our analysis revealed no significant influence of session timing (AM/PM) on hormone levels within each group (p ≥.130). Groups were also equally distributed for AM/PM testing (p = .190). To confirm hormonal status, GLMs were conducted on groups based on their current hormonal state. Levels of E2, P, and T were compared between men (n = 40), early follicular NC women (n = 45), pre-ovulatory NC women (n = 32), and COC users (n = 62). Analyses revealed a group difference for E2 [FM(3, 175) = 34.80, pM <.001, ηp2M = .369] and T [FM(3, 175) = 213.98, pM <.001, ηp2M = .784]. Games-Howell post hoc tests showed higher E2 concentrations in pre-ovulatory NC women compared to the three other groups (pMs <.001). Early follicular NC women and men had similar E2 levels (pM = .898), which were both comparable to COC users (pM = .267 and.117 respectively). For T, men showed higher concentrations than the three groups of women (pMs <.001). Pre-ovulatory NC women exhibited greater T levels than early follicular NC women (pM = .033) and both NC groups had more T levels than COC users (pMs ≤.006). Further, a Group (2) x Cycle phase (2) GLM was conducted between past users and never users. For the three endogenous hormones, no differences were found between the two groups nor for the interaction term (pMs ≥.185). For cycle phase, irrespective of groups, E2 and T were significant and followed the same pattern as in the first set of hormonal analyses. Untransformed hormonal means are reported in Table 1, while both sets of group analyses are summarized in Figure 1. In sum, the salivary endocrine assessment revealed expected variations in our study groups. Never and past users were equivalent in terms of their hormonal profile.
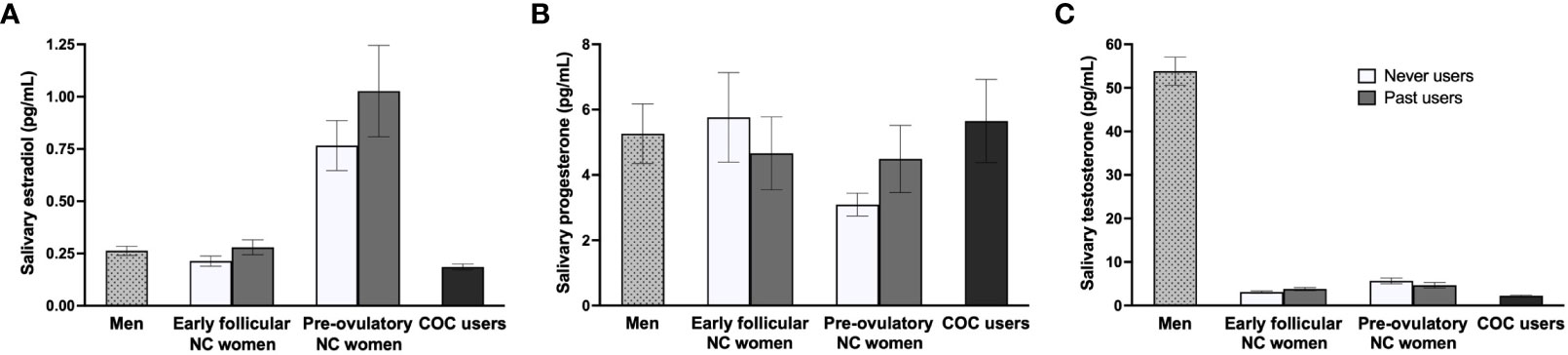
Figure 1 Sex hormone profiles across study groups. (A) As expected, pre-ovulatory naturally-cycling (NC) women had higher estradiol concentrations compared to the three other groups. Estradiol levels were similar for early follicular NC women, men, and combined oral contraceptive (COC) users. (B) Groups did not differ in progesterone levels. (C) For testosterone, men showed higher concentrations than the three groups of women, pre-ovulatory NC women exhibited greater levels than early follicular NC women, and both NC groups had more testosterone levels than COC users. No differences were found between never users and past users across the three hormones.
Sample characteristics are presented in Table 1. As expected, men had greater TIV than the three other groups of women (p <.001). No differences were observed for global CT. Regarding sociodemographic variables, men, never users, past users, and current COC users did not differ on age, mother tongue, body mass index, and physical activity practice (ps ≥.101). However, relationship status was disproportionately distributed across study groups (p <.001). Based on Bonferroni-adjusted pairwise Z-tests, current users were significantly more in relationships than the three other groups and the proportion of individuals in a relationship was higher in both current and past users than never users and men. Moreover, groups tended to differ on ethnicity (p = .059), where never users were less of Caucasian origin than expected. Although non-significant, a greater proportion of past users were of Caucasian origin (78.4%) than never users (52.5%) in our sample. Years of education were also marginally different between the groups (p = .072), although post hocs did not yield significant group differences (ps ≥.156). Concerning psychological constructs, groups did not differ regarding religiosity, neuroticism, trait anxiety, depressive symptoms, and exposure to adverse events (ps ≥.151). For endocrine-related variables (Table 2), no differences were found for the age at menarche between the three groups of women (p = .498), nor for cycle phase between past and never users (p = .524), and reasons for COC use between current and past users (p = .329).
Correlations between TIV/global CT and potential confounders across the whole sample are reported in Table 3. According to the disjunctive cause criterion (141), fully adjusted models for GMV included age (conceptually), TIV, ethnicity, years of education, relationship status, and religiosity, whereas age, ethnicity, years of education, relationship status, and practicing physical activity were controlled for in fully adjusted CT models. When restricted to past users and never users, fully adjusted models included age and TIV (conceptually), mother tongue, body mass index, ethnicity, relationship status, religiosity, and adverse events for GMV analyses and age, mother tongue, body mass index, ethnicity, relationship status, and neuroticism for CT analyses (data not shown).
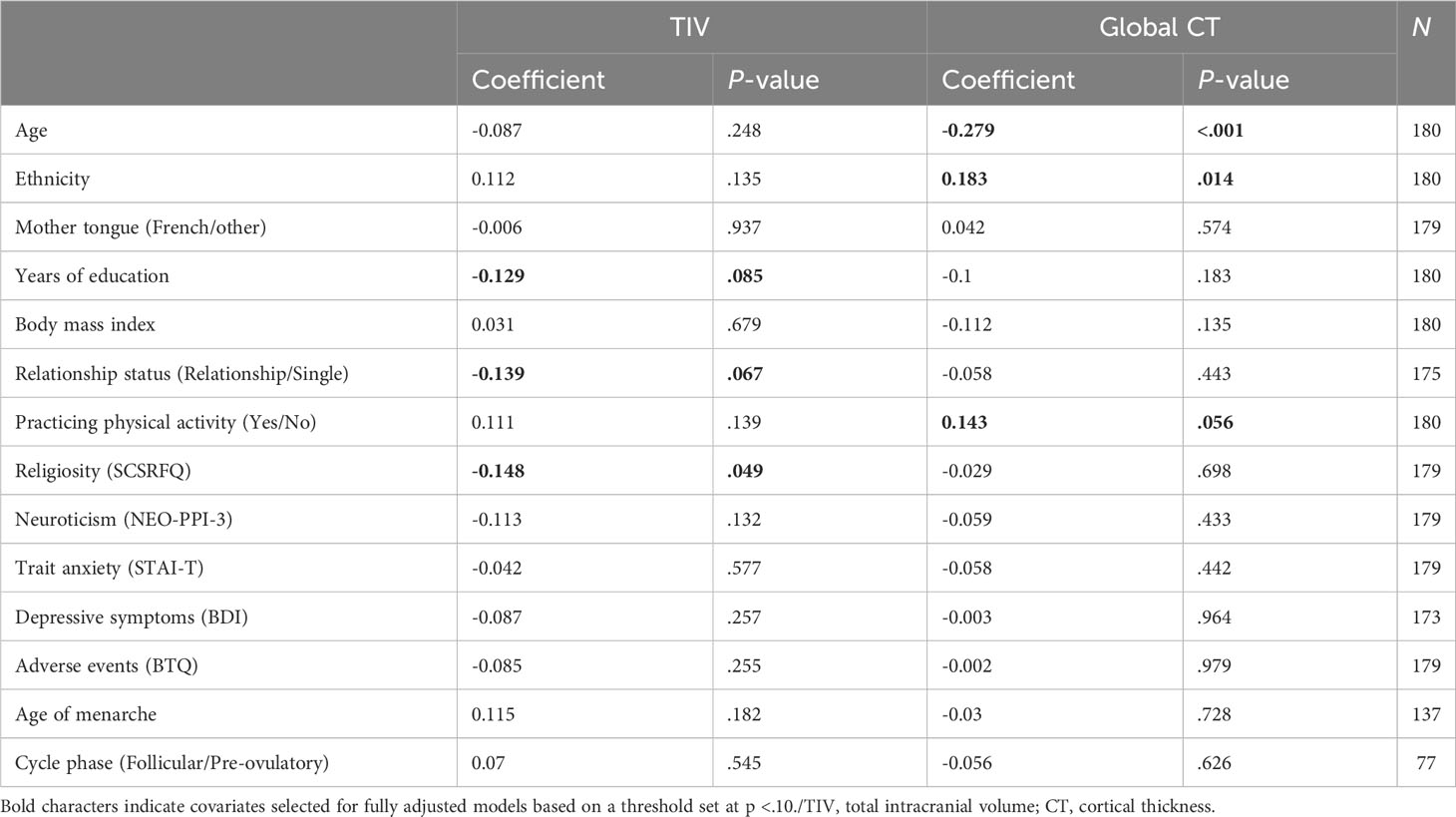
Table 3 Associations between total intracranial volume, global cortical thickness, and potential confounders across the whole sample.
3.2 Main analyses
3.2.1 Are there acute or long-term effects of COCs on structural correlates of the fear circuitry?
To examine the acute or long-term effects of COC use, we performed Group (4) x Hemisphere (2) GLMs. In ROI-based GMV analyses controlling for age and TIV, a main effect of Group was observed for the dACC [F(3,174) = 4.63, p = .004, ηp2 = .074, qFDR = .027], with all three groups of women exhibiting a larger bilateral volume than men (ps ≤.037; Figure 2A). In ROI-based CT analyses controlling for age, groups also differed regarding vmPFC thickness [F(3,175) = 3.71, p = .013, ηp2 = .060, qFDR = .051]. Current COC users, but not past nor never users (ps ≥.229), had thinner bilateral vmPFCs than men (p = .007; Figure 2B). Results remained the same using fully adjusted models.
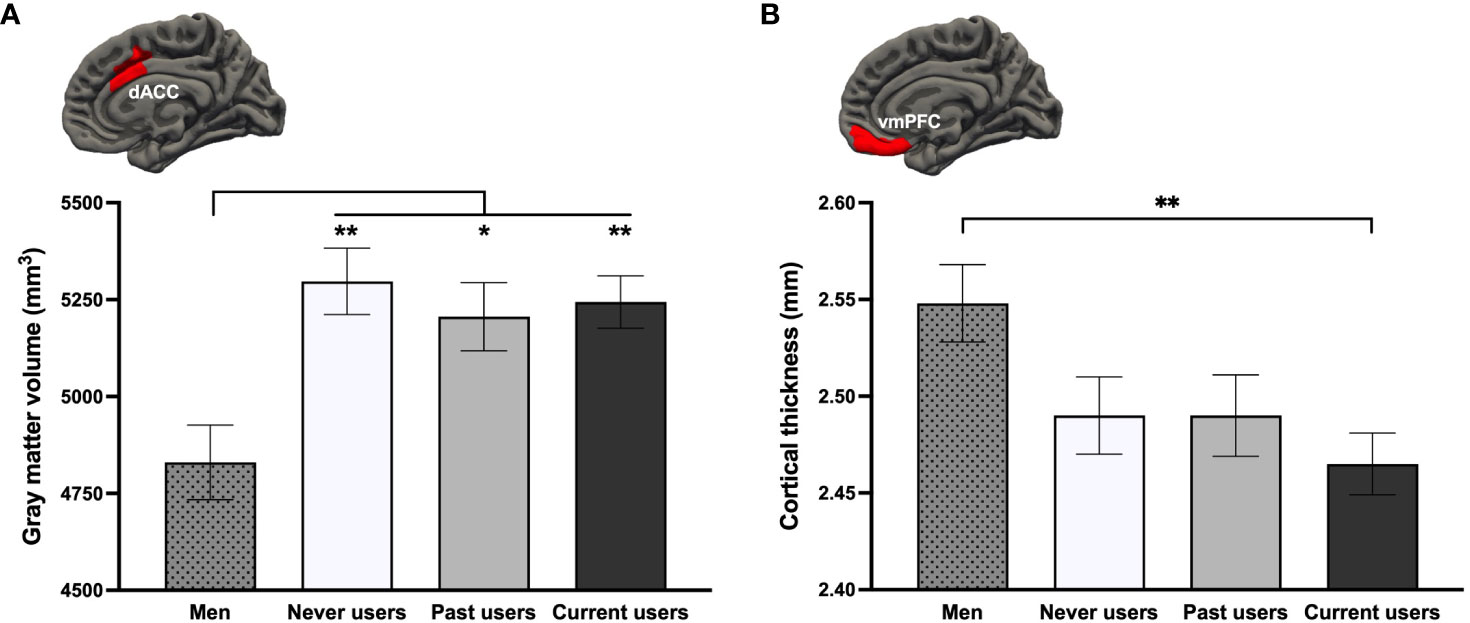
Figure 2 Group differences on the (A) bilateral dorsal anterior cingulate cortex (dACC) and (B) bilateral ventromedial prefrontal cortex (vmPFC) based on a region-of-interest approach. **p <.01, *p <.05.
We found no significant clusters in volumetric and thickness WBA. However, SPM’s VBM revealed several clusters surviving multiple comparisons at the cluster level. Results are presented in Table 4 and visually represented in Supplementary Figures 1–3.
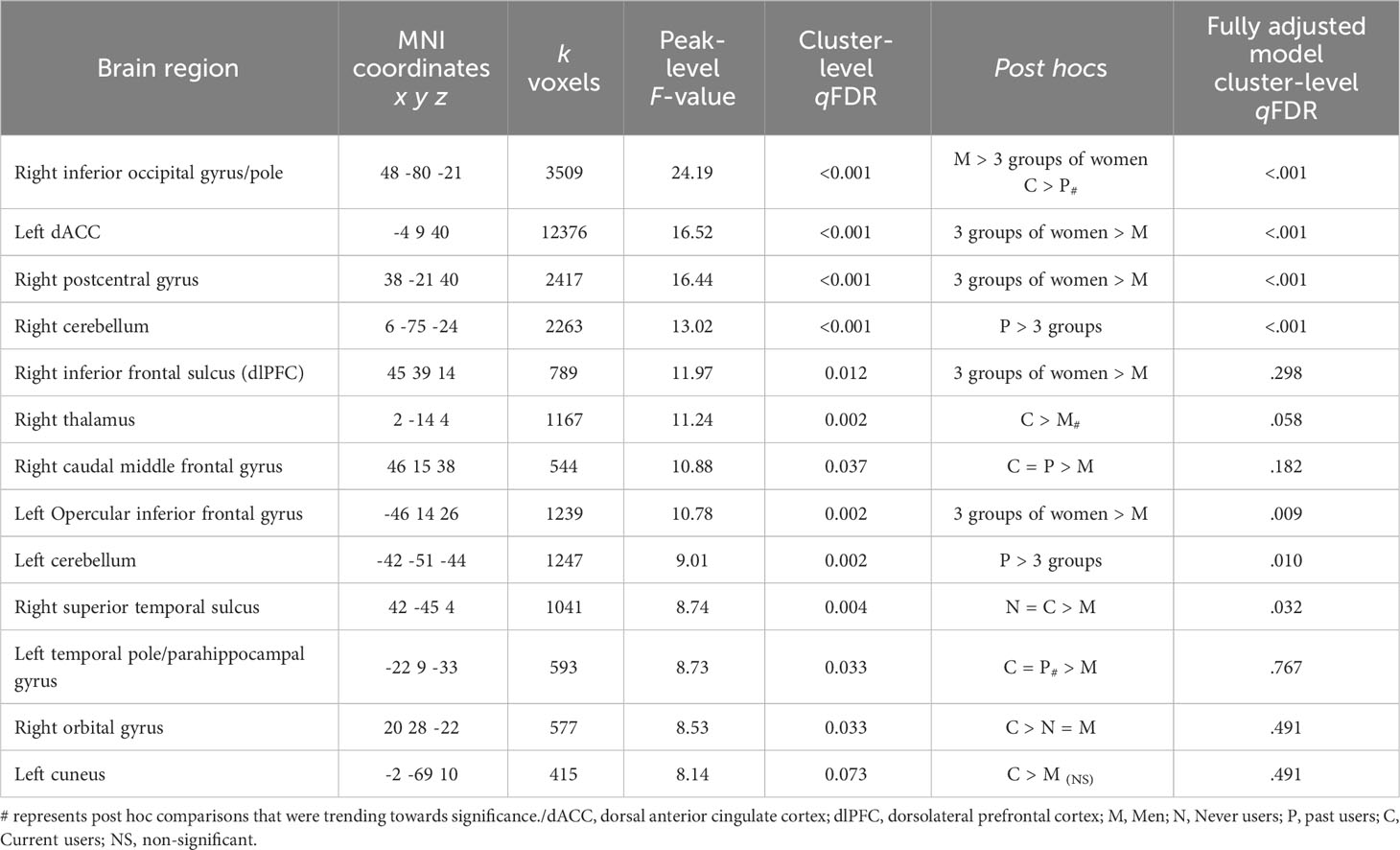
Table 4 Voxel-based morphometry clusters emerged between the four groups using a whole-brain approach, adjusted for age and scaled for total intracranial volume.
3.2.2 Does the current hormonal milieu interact with the history of contraception use in NC women?
When looking at both groups of NC women, GMV analyses revealed no effects of Group (past users, never users), Cycle phase (early follicular, pre-ovulatory), or Group x Cycle phase across all ROIs. For CT, a Group x Hemisphere interaction effect was found for the AIC [F(1, 72) = 6.40, p = .014, ηp2 = .082, qFDR = .054]. Irrespective of cycle phase, never users tended to have a thicker right AIC compared to past users (p = .057; Figure 3). This difference reached significance (p = .039) with the fully adjusted model.
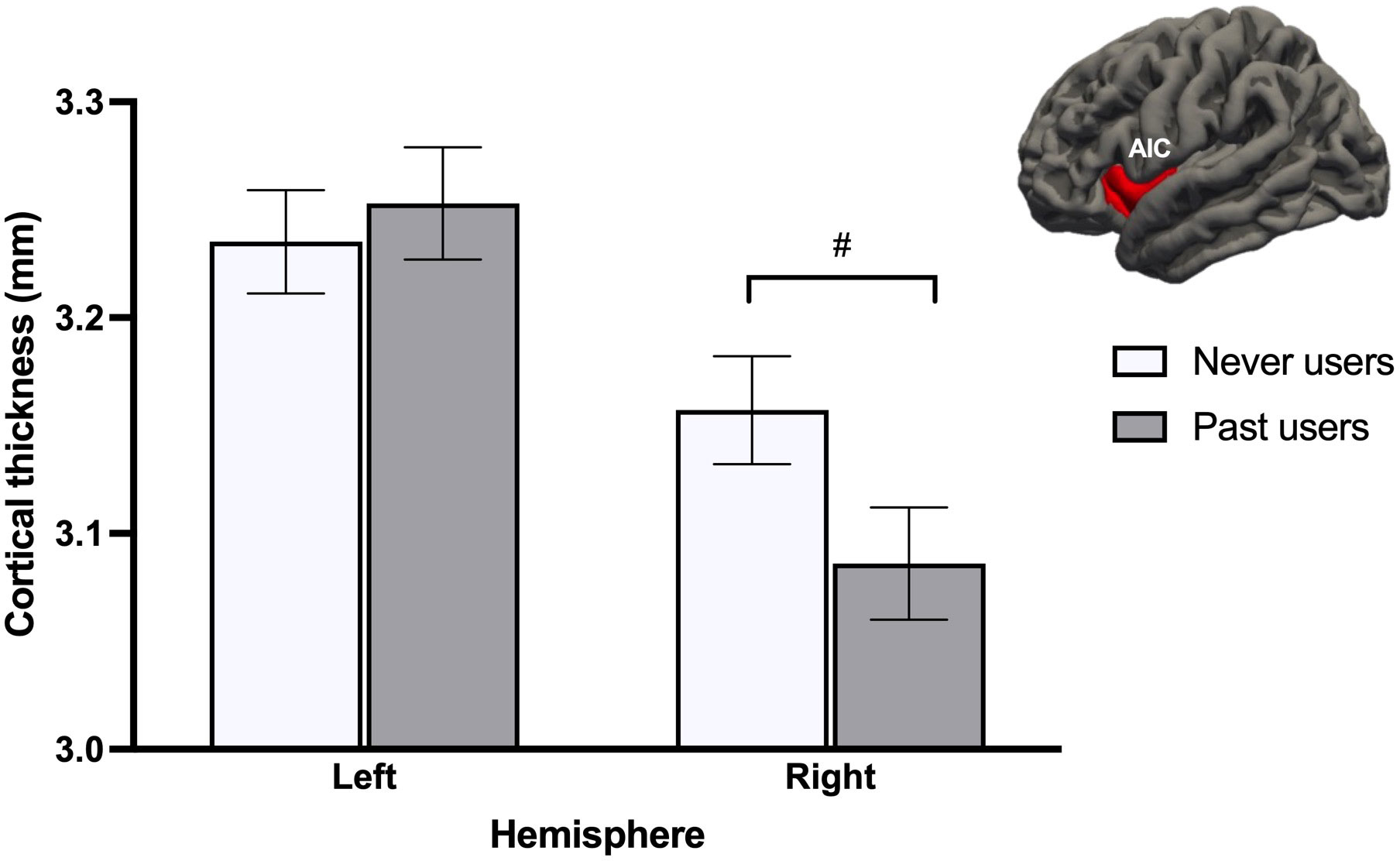
Figure 3 Never users tended to show thicker right anterior insular cortex (AIC) than past users, after adjusting for age and menstrual cycle phase. This difference became significant after adjusting for additional confounds. #p <.08.
We found no significant clusters in volumetric and thickness WBA. Using VBM, WBA yielded no significant results, though we found a trend for a cluster in the right orbital gyrus for the Group x Cycle phase interaction term [F(1, 72) = 11.77, qFDR = .072, k = 606, MNIxyz = 24, 39, -12]. This cluster fell at qFDR = 0.95 with the fully adjusted model.
3.2.3 Are endogenous estradiol and testosterone linked to structural correlates of the fear circuitry? Are those associations specific to men, never users, or past COC users?
To evaluate the role of acute endogenous levels and their potential interaction with our study groups, we conducted Group (3) x Hemisphere (2) x Hormone (E2 or T) GLMs on our imputed and log-transformed dataset. ROI-based GMV analyses revealed a Group x E2 interaction [FM(2, 108) = 4.22, pM = .029 (.003 ≤ ps ≤.086), ηp2M = .073, qFDR = .203] for the hippocampus. Post hocs showed that bilateral hippocampal GMV correlated negatively with E2 levels (BM = -124.33, βM = -.345, pM = .040, fraction missing info = .185) in never users, specifically. A main effect of T was also trending [FM(2, 108) = 4.61, pM = .052 (.007 ≤ ps ≤.145), ηp2M = .041, qFDR = .196], where T concentrations tended to positively correlate with the GMV of the bilateral hippocampus in all participants (BM = 48.58, βM = .181, pM = .067, fraction missing info = .078; Figures 4A, B).
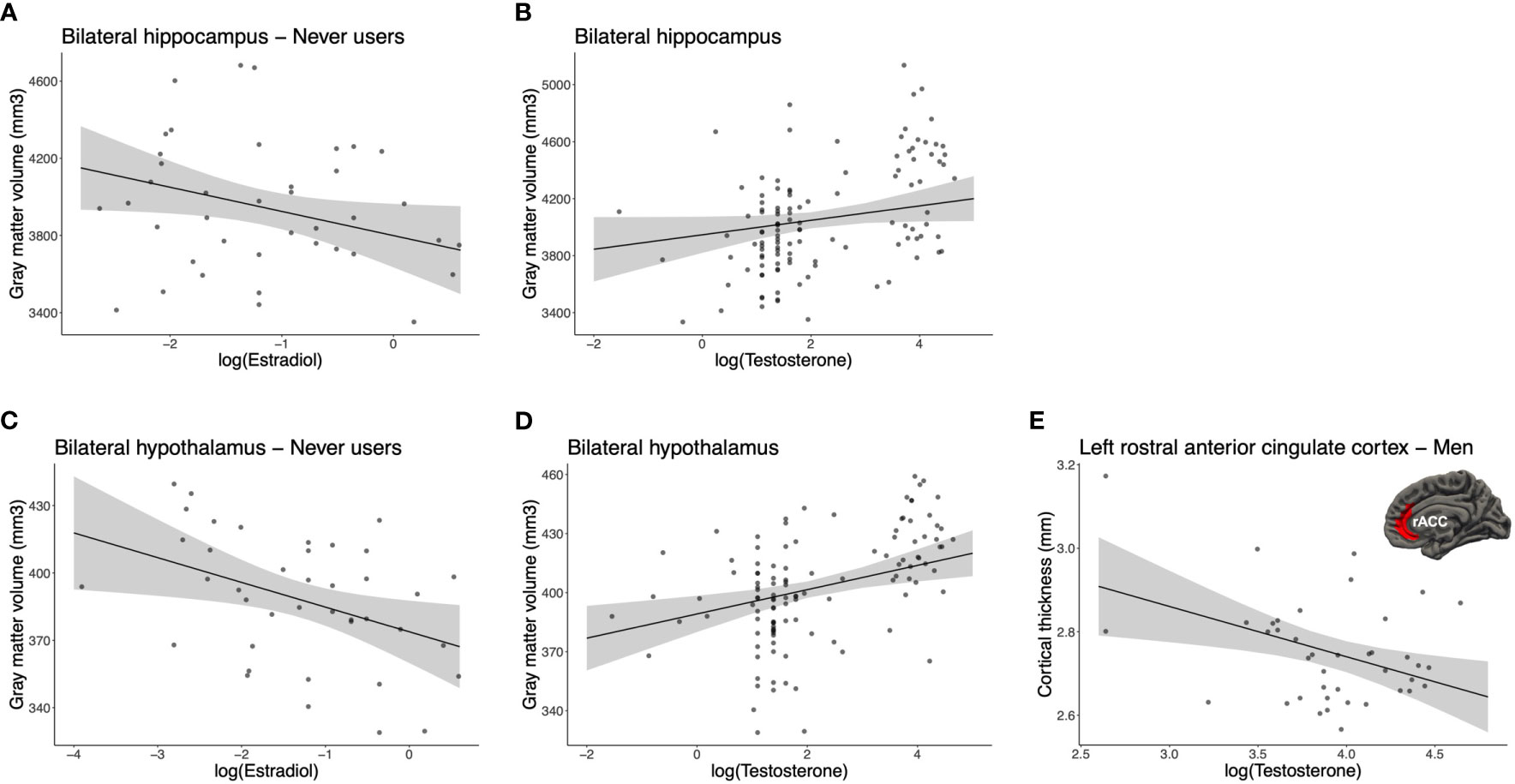
Figure 4 Associations between endogenous sex hormones and brain morphology. For the bilateral hippocampus, (A) estradiol was negatively correlated in never users (pM = .040, fraction missing info = .185) and (B) testosterone tended to positively correlate in all participants (pM = .067, fraction missing info = .078). For the bilateral hypothalamus, (C) estradiol was negatively correlated in never users (pM = .014, fraction missing info = .197) and (D) testosterone was positively correlated in all participants (pM = .002, fraction missing info = .128). (E) For the left rostral anterior cingulate cortex (rACC), testosterone was negatively correlated to the cortical thickness in men (pM = .006, fraction missing info = .021). For illustrative purposes, each scatter plot depicts the imputation dataset being the most similar to the pooled p-value of the post hoc result. Error bars represent 95% confidence intervals.
For the hypothalamus, we found a marginal interaction for Group x E2 [FM(2, 108) = 2.98, pM = .079 (.016 ≤ ps ≤.233), ηp2M = .053, qFDR = .277]. GMV of the bilateral hypothalamus was inversely correlated to E2 levels in never users (BM = -13.02, βM = -.429, pM = .014, fraction missing info = .197). Also, we found a trend towards a main effect of T [F(1, 108) = 4.09, pM = .056 (.015 ≤ ps ≤.151), ηp2M = .037, qFDR = .196], where T concentrations across all groups were positively associated with the bilateral hypothalamus (BM = 6.15, βM = .314, pM = .002, fraction missing info = .128; Figures 4C, D).
For CT analyses, we found a marginal Group x Hemisphere x T interaction [FM(2, 109) = 3.31, pM = .067 (.007 ≤ ps ≤.158), ηp2M = .058, qFDR = .268] for the rACC. When examining post hocs, the only significant result pertained to men, where thickness of their left rACC was inversely associated with T levels (BM = -.12, βM = -.421, pM = .006, fraction missing info = .021; Figure 4E). Of note, potential outliers were visually acknowledged. Using a Cook’s distance >.5, we excluded two influential data points and observed that the relationship survived in trend (BM = -.089, βM = -.334, pM = .077, n = 38). No other comparison was related to the left or right rACC thickness for T in never and past users.
Sensitivity analyses for all post hocs are further presented in Supplementary Figures 4–8.
3.2.4 Are exogenous sex hormones linked to structural correlates of the fear circuitry in current COC users? Identifying the most influential factor between salivary EE levels, EE dosage, and progestin androgenicity
In COC users, we performed GLMs for each ROI with Hemisphere (2), EE dose (2), and Salivary EE levels, as well as Hemisphere x EE dose and Hemisphere x EE levels. For GMV, Hemisphere x EE dose interactions were found in the AIC [FM(1, 50) = 5.08, pM = .029 (.026 ≤ ps ≤.032), ηp2M = .092, qFDR = .098], the dACC [FM(1, 50) = 4.92, pM = .031 (.027 ≤ ps ≤.035), ηp2M = .090, qFDR = .098], and the vmPFC [FM(1, 50) = 4.21, pM = .046 (.042 ≤ ps ≤.051), ηp2M = .078, qFDR = .098]. Post hocs showed that women using COCs with a higher EE dose (30-35μg, n = 27) exhibited a larger right AIC (pM = .020), left dACC (pM = .037) and left vmPFC (pM = .033) than women using a lower dose of EE (10-25μg, n = 28; Figures 5A–C). This interaction term was also trending in the rACC [FM(1, 50) = 3.84, pM = .056 (.047 ≤ ps ≤.066), ηp2M = .071, qFDR = .098], although no post hocs emerged significant (pMs ≥.101). For CT, a main effect of EE dose was trending in the vmPFC [FM(1, 51) = 3.46, pM = .069 (.056 ≤ ps ≤.084), ηp2M = .064, qFDR = .276], with 30-35μg doses being marginally associated with thicker bilateral vmPFC compared to 10-25μg doses (Figure 5D). Results were similar when adjusting for androgenicity, where higher doses were still associated with greater GMV (though statistical significance was not met for all ROIs).
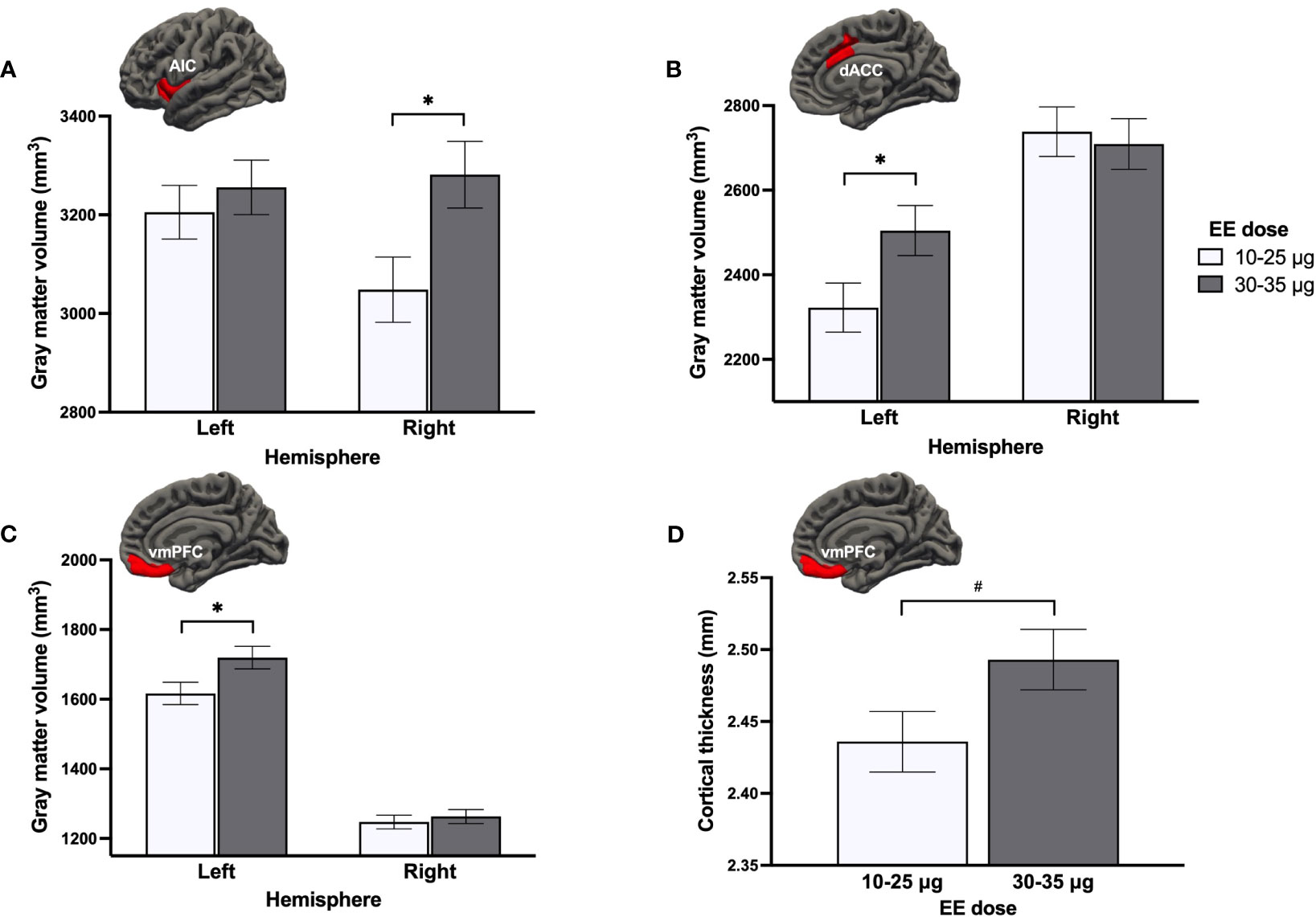
Figure 5 Effect of ethinyl estradiol (EE) doses on the gray matter volume of the (A) anterior insular cortex (AIC), (B) dorsal anterior cingulate cortex (dACC), (C) ventromedial prefrontal cortex (vmPFC), and (D) the cortical thickness of the vmPFC. *p <.05, #p <.08.
To better understand the directionality of the influence of EE dose, we compared both groups of EE dose users to never users and men on GMV of the right AIC, left dACC, left vmPFC, and CT of the bilateral vmPFC. A main effect of Group was found for the right AIC [F(3, 137) = 2.98, p = .034, ηp2 = .061], left dACC [F(3, 137) = 5.38, p = .002, ηp2 = .105], and bilateral vmPFC thickness [F(3, 138) = 4.03, p = .009, ηp2 = .080] (Figures 6A–C). Low EE dose users tended to have a smaller GMV of the right AIC compared to men (p = .051). For the left dACC GMV, a region previously shown to be larger in all groups of women (section 3.2.1.), only never users and high EE dose users presented greater GMV than men (ps ≤.031), with low EE dose users being most similar to men and having significantly less GMV than high EE dose users (p = .037). For the CT of the bilateral vmPFC (where current use of COCs was linked to vmPFC thinning compared to men; section 3.2.1.), this more in-depth investigation revealed that this difference was specific to COC users taking low doses of EE (p = .006), with high EE dose users being most similar to never users. Finally, albeit no group effect was found for the left vmPFC (p = .434), GMV appeared to be smaller in women using COCs with a lower dose compared to the three other groups (Figure 6D).
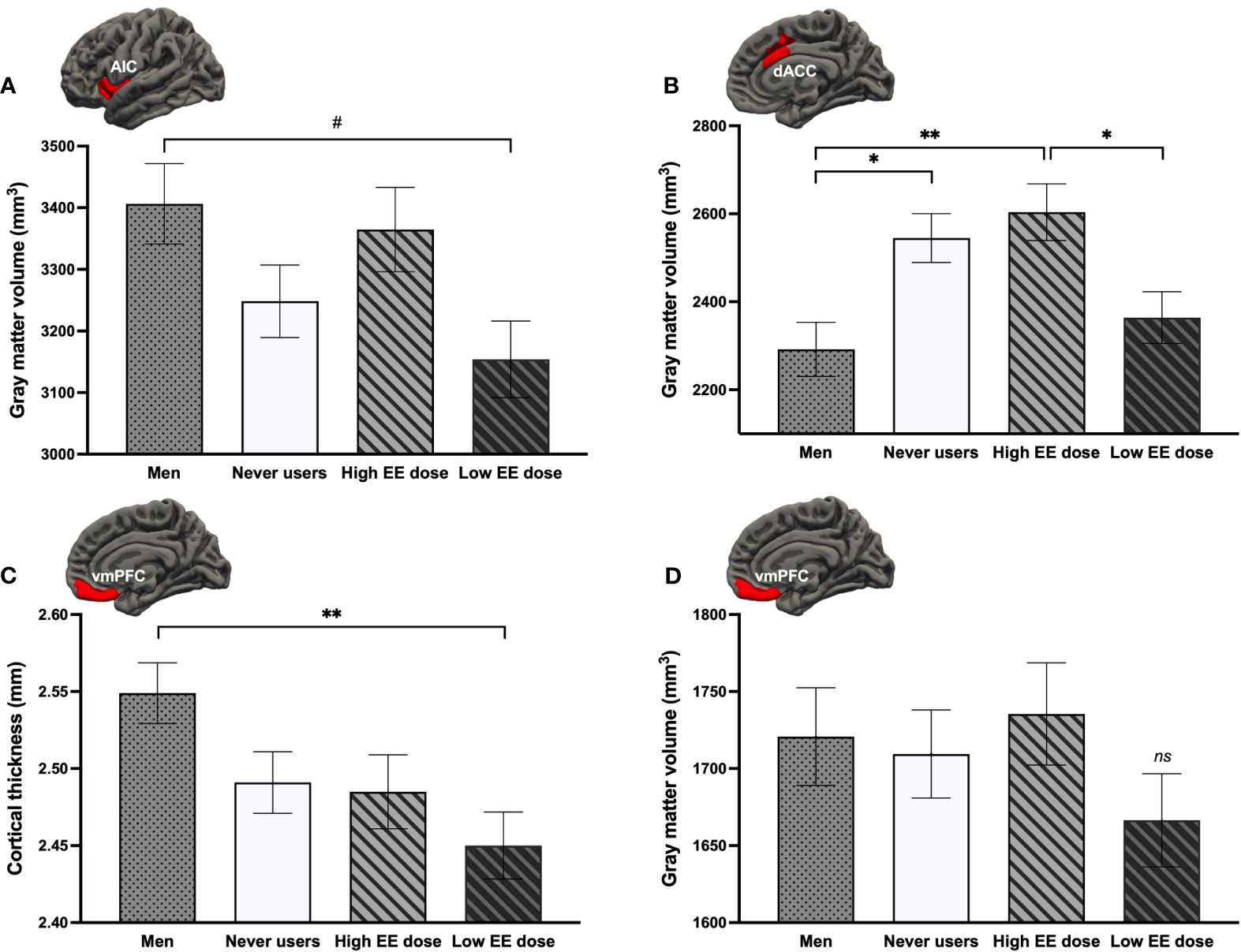
Figure 6 Comparison of current users either taking low or high doses of ethinyl estradiol (EE) with never users and men regarding the (A) right anterior insular cortex (AIC) volume, (B) left dorsal anterior cingulate cortex (dACC) volume, (C) bilateral ventromedial prefrontal cortex (vmPFC) thickness, and (D) left vmPFC volume. **p <.01, *p <.05, #p <.08. ns, non-significant.
Looking at progestin androgenicity, Hemisphere (2) x Androgenicity (3) GLMs revealed no significant effects related to androgenicity. A marginal main effect of androgenicity was found for the dACC GMV [F(2, 57) = 3.04, p = .056, ηp2 = .096, qFDR = .389] and dACC CT [F(2, 58) = 2.95, p = .060, ηp2 = .092, qFDR = .241]. Women taking low androgenic COCs (n = 18) tended to have greater bilateral GMV than those taking high androgenic COCs (n = 27; p = .055) and greater bilateral CT than those taking anti-androgenic COCs (n = 17; p = .055; Figures 7A, B). Results remained similar when adjusting for EE dosage. When further comparing androgenicity groups to never users and men for the bilateral dACC, groups significantly differed for the GMV [F(4, 136) = 5.73, p <.001, ηp2 = .144], where men exhibited less GMV than never users (p = .004; as previously shown above) but also than low androgenic COC users (p <.001). Though no significant group effect was found for the dACC CT (p = .086), low androgenic COC users appeared to have a thicker bilateral dACC than all groups (ps ≥.082; Figures 7C, D).
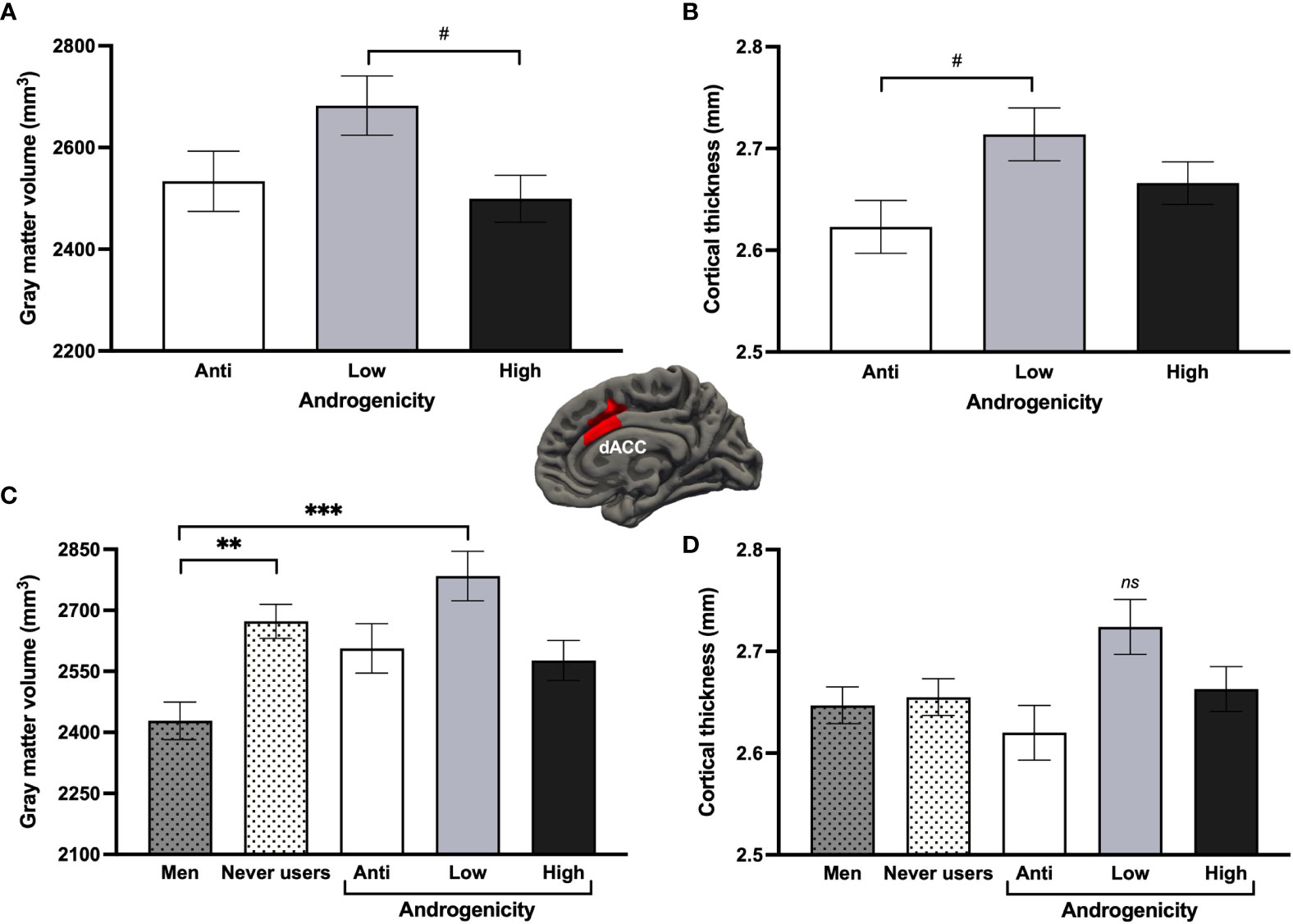
Figure 7 Effects of progestin androgenicity on the dorsal anterior cingulate cortex (dACC). Results are displayed for (A) gray matter volume and (B) cortex thickness of the three androgenicity groups, as well as additional comparison with never users and past users for (C) gray matter volume and (D) cortex thickness of this region. ***p <.001, **p <.01, #p <.08. ns, non-significant.
For EE models, no significant clusters emerged for all WBA (volume, thickness, VBM). For androgenicity models, we found significant clusters for CT of the right middle/superior frontal cortex [cluster-wise p = .0085, MNIxyz = 21.9, 52.3, 25.6, max = 3.38, size (surface area) = 2976.14mm2, kvertices = 4928] and GMV of the right caudal middle temporal gyrus [cluster-wise p = .023, MNIxyz = 58.9, -54.4, 0.1, max = 3.17, size (surface area) = 946.08mm2, kvertices = 1875]. T-test post hocs showed that low androgenic COC users had thicker cortex of the right frontal cluster than anti-androgenic and high androgenic COC users, while GMV of the right temporal cluster was larger in anti-androgenic COC users than both low and high subgroups. Both results survived when controlling for EE dose. VBM yielded no significant clusters.
4 Discussion
This study examined the modulation of endogenous and exogenous sex hormones on structural properties of key regions implicated in fear expression and regulation. We used rigorous methodology to decipher whether acute or long-term effects of COCs were detectable and if we could document an influence related to menstrual cycle phase, endogenous sex hormone concentrations, and exogenous endocrine factors of COCs. Comparisons of current COC users, past COC users, never users, and men were carried out on surface-based and voxel-based morphometry, as well as with both region-of-interest and whole-brain approaches.
Irrespective of their hormonal contraceptive history, we found that women showed greater GMV than men in the dACC. This ROI-based sex difference aligns with previous reports (146–149) and was further replicated in this study with VBM, among other sex differences found in the right occipital pole, right postcentral gyrus, and left inferior frontal gyrus. These results suggest a predominant role the organizational effects of sex steroids. Indeed, exposure to sex hormones can confer permanent structural modelling of the nervous system when occurring in a specific developmental window (150). Given that COCs are often initiated during adolescence (111, 151–153) and that this developmental stage is considered to be a rich (re-)organizational period (150, 154–156), we can assume that GMV of certain regions like dACC are particularly sensitive to the influence of sex hormones in an earlier developmental stage. This may explain why later endocrine disruption such as via initiation of COCs did not yield anatomical alteration. Naturally, other non-hormonal factors may underlie this finding, such as gender (53). Given that this result survived the adjustment for many socio-cultural factors, we are inclined to lean towards a biological explanation. That said, as we did not measure all relevant gendered confounds, we are unable to support our claim with the current data. Furthermore, we wish to acknowledge the complexity of sex and gender science and the interaction between both dimensions (157). Moreover, it is worth noting that many cluster-corrected findings emerged from SPM’s VBM analysis, though not from FreeSurfer’s surface-based volumetric WBA. Beyond the discrepancy across software, this provides insight into VBM’s sensitivity to sex-sensitive discoveries versus SBM.
The dACC is a region associated with fear expression and appraisal (39, 158). It was found to be involved in the neural signature of trait anxiety, where its volume was reported to be a significant predictor (106). A larger GMV of the dACC was also found to be a discriminant region between drug-naïve anxiety patients compared to healthy controls (159). While the role of the dACC in anxiety appears quite clear, it has also been associated with positive emotion regulation constructs including the use of cognitive reappraisal (160), the ability to identify and describing one’s feelings (161), and effortful control (162, 163). These conflicting results may stem from the broad role of dACC in emotion processing and the fact that these complex mental states are sustained by large networks rather than a single brain region. They can also be reconciled with regard to paradoxical traditional women-oriented stereotypes, where women are expected to carry heavier household responsibilities, plan ahead, have emotional intelligence, and care and worry about others (164–166).
Our results also showed a reduced CT of the vmPFC in current COC users compared to men. This aligns with Petersen et al. (57, 58) who also showed a reduction of CT in prefrontal areas, although they found significant disparities between NC women and COC users and did not have a group of men for comparison (57). Previous studies have reported thicker vmPFC in men compared to women (167, 168), however, this difference has not been replicated enough to support a reliable sex difference (169–171). Thus, a specific comparison of COC users and men may elucidate the mixed results regarding a potential sex difference of the vmPFC thickness. Moreover, the vmPFC is consensually viewed as a critical node for fear regulation (13, 38, 39, 158). Thicker vmPFC has been associated with greater fear extinction learning and recall (172, 173) and lesser fear generalization (174), as well as resilience following trauma exposure (175) and remission in treatment-naïve patients with obsessive-compulsive disorder (176). Reduced vmPFC thickness is thought to reflect top-down inhibition deficits, particularly of amygdala reactivity (177, 178). Interestingly, poorer extinction recall has been reported in COC users compared to NC women in a high E2 state (30). COC users also showed reduced responsiveness to exposure therapy compared to NC women irrespective of their E2 levels (8, 179). In light of these findings, our vmPFC result suggests that COCs may confer a risk factor for extinction deficits during their current use but not after their discontinuation. Indeed, it is well known that women are more susceptible to suffering from fear-related psychopathologies than men, including anxiety and stress-related disorders (3, 16). Given our results that men have smaller dACC volume than women and thicker vmPFC than COC users, these findings may represent structural vulnerabilities to psychopathologies that predominantly affect women. Specifically, a larger dACC could represent a female predisposition to fear promotion, whereas COC use could exacerbate this vulnerability by potentially inducing a thinning of a fear-inhibiting region such as the vmPFC. However, as tempting as it may be to settle on this conclusion, caution must be exercised when interpreting interrelations between brain morphology and behavioral or psychological data. Despite the high reliability of structural imaging (180), the validity of brain-behavior associations has been criticized (181, 182).
Interestingly, no lasting effects of COC use were detected when comparing the four groups. The sole difference pointing towards such effects was in the right AIC, where never users had a greater CT than past users when controlling for the ‘here and now’ influence of the menstrual cycle. The right AIC has been found to be engaged in bodily-arousing anxiety-related processing such as heartbeat awareness, sympathetic nervous system activities, and unpredictable aversiveness (183–186). Knowing that its volume has been negatively associated with reactivity to uncertain threat (187), thinner CT in past users could possibly be interpreted as deleterious. Most importantly, our overall findings support the reversibility of the impact of COCs on brain morphology, especially on vmPFC thickness. Yet, our conceptualization of the past user group may not have been optimal for detecting long-term effects. Indeed, as the literature provided little guidance, we used an arbitrary (yet stringent) criterion of 12 months for a minimal duration of use and time since discontinuation. Greater variability for cessation duration (i.e., setting a criterion to a shorter elapsed time) would have allowed a finer investigation of lasting effects. Therefore, our results prompt future research to explore the reversibility of COC use within the first year of discontinuation. In addition, pooling all past users together may have camouflaged potential long-term effects. Given that adolescence is a sensitive period for brain development and that OC use at this age has been linked to a higher vulnerability to depression (111, 188–191), OC parameters (e.g., duration of use and age of onset) may be other relevant and possibly more sensitive factors for studying durable effects (192). Of note, our team is currently investigating the effects of these parameters.
For endogenous sex hormone concentrations, we observed similar patterns for the hippocampus and hypothalamus, where these two structures are highly susceptible to the influence of sex hormones (193–195). However, the inverse relationships found for E2 in never users were quite surprising and opposed the scientific consensus on the trophic effects of E2 on the hippocampus (45–48). Considering our unique methodological approach, our hormone-brain associations should be interpreted cautiously and require replication. Statistical significance varied considerably across imputations, as observed by wide p-value ranges and the ‘fraction missing information’ metric. The latter indicates the variance proportion pertaining to the multiple imputation procedure. Specifically, in our study, the variation of one dataset to another amounted to nearly 20% and was the result of a high non-detection rate using LC-MS/MS. Importantly, LC-MS/MS provides better assay validity than immunoassay methods for salivary E2 and P (especially for low ranges) and is on its way to becoming the gold standard in endocrine measurement (196–199). Our study shows that for a normative adult population (i.e., without pathology, naturally-circulating and low sex hormone levels), data from LC-MS/MS are relevant and valid for categorization purposes (mean comparisons). However, according to our study, the main drawback of this method relates to statistics based on the continuous nature of sex steroid levels. Knowing that saliva accounts for free (unbound) steroids and represents less than 5% of total serum concentrations (200, 201), we recommend the use of serum samples for future studies conducting correlational analyses on steroid data obtained via LC-MS/MS. Visibly, the future of LC-MS/MS is promising and efforts in refining actual methods for studying sex steroids are within reach. Improvement in detection rates would certainly allow the scientific community to better grasp the influence of endogenous sex hormones on the brain.
For exogenous steroids, we first determined that EE dose (rather than circulating EE levels) modulated cortical fear-related ROIs in COC users. This observation is fairly unsurprising, as we expect brain tissues to adapt over a certain period of time (202). For instance, we expect this adaptation with constant and chronic exposure to EE dose, rather than with the rapid fluctuations inherent to pharmacokinetics. Indeed, GMV of the right AIC, left dACC, and left vmPFC were significantly larger in COC users using higher doses of EE compared to lower doses. We were able to contextualize the direction of this effect by comparing these groups to men and never users, revealing the atrophic effects of a lower EE dose rather than the trophic effect of a higher EE dose. This also allowed us to refine the result regarding CT of the vmPFC in current COC users, where thinning was driven by lower doses of EE. Interestingly, the potency of EE is approximately two times greater than that of E2 on the estrogen receptor alpha (ERα), while mixed findings have been reported for the estrogen receptor beta (ERβ) (85, 203, 204). Both ERs have been detected in the cortex (205, 206), with a larger proportion of ERα reported in the vmPFC (28). Given that endogenous E2 is suppressed by any kind of COCs, one could hypothesize that only low EE intake (i.e., 10-25μg) may lead to underactivation of ERα (and potentially ERβ to a lesser degree) and therefore, prevent cortical trophic effects of estrogenic activity (207, 208). Though we were unable to empirically explore this option, the impact of EE dosage on brain volumes may follow a curvilinear trajectory where higher EE doses (e.g., 50 μg) may induce atrophic effects. Inverted-U relationships of E2 have been reported on fear extinction (209) and hippocampal activity (210). Despite its speculative nature, this hypothesis could be further tested in animal models to deepen our understanding of basic endocrinology knowledge. We however strongly advise against testing this hypothesis in women considering the known risk of much higher EE doses on safety and tolerability of COCs (26, 211). Yet, based on our results, we propose that a hypoestrogenic state may only occur when low endogenous E2 is combined with low EE intake. Conversely, higher EE doses may provide adequate ERα binding to simulate moderate-to-high estrogenic activity, similar to NC women. Recent reviews in the field have highlighted the importance of disentangling OC mechanisms with regard to their endogenous (i.e., inhibition of the HPG axis) versus exogenous actions (i.e., intake of synthetic molecules) (84, 85, 212, 213). While this line of interpretation remains novel, our results provide insight into how COC users may be under a hypoestrogenic state when using regimens containing lower EE doses.
In contrast, our investigation on progestin androgenicity did not yield convincing effects on the fear circuitry. We reported a possible inverted-U relationship in the dACC, where low androgenic activity tended to show trophic influence over this cortical region. This curvilinear relationship appears to be more largely spread and stronger in the upper part of the frontal cortex, as observed by the means of an exploratory WBA. However, even after statistically controlling for EE dosage, these findings could still potentially be confounded by higher EE doses in low androgenic COC users. Due to the small sample size for these models, it is difficult to clearly depict the impact of androgenicity on the fear circuitry. To do so, it would be essential to conduct analyses on a larger sample and to focus on the interaction between EE dose and androgenicity.
We measured two phenotypes of the GM that have been previously linked to OC use, namely GMV and CT (59–61). In our study, some results pertaining to cortical ROIs were found for GMV, CT, or both. On the one hand, effects specific to CT (e.g., thinner vmPFC in current users) or found for both phenotypes (e.g., marginal influence of androgenicity on the dACC) suggest changes in cell restructuring among cortical layers. On the other hand, effects in GMV but not in CT (e.g., sex difference in the dACC) suggest that volumetric alterations are consequent to other properties of the GM such as folding, surface area, or an interplay between CT, folding and/or surface area. Further investigation of various GM phenotypes could help singularize which aspect of GM is particularly influenced by volumetric findings.
For the ROI approach, we opted for an uncorrected significance threshold for the multiple ROIs, even though qFDRs were presented for completeness. As multiple testing is an important issue in MRI studies, it is typically handled using FDR or Bonferroni corrections. Yet, when applied to ROIs as opposed to WBA, this procedure is highly dependent on the number of ROIs and may give the false impression of non-significant results (93). Given the strong a priori rationale for ROIs defined in our study, we deemed that all (uncorrected) results were relevant. By looking at effect sizes and qFDRs, our findings clearly show that the results of medium-to-large effect size did not survive the FDR adjustment of q <.05 for ROIs of kGMV = 7 and kCT = 4. Despite filtering out results with the smallest p-values, this highlights the weakness of a posteriori corrections (i.e., inflation of false negative rate) for ROI-based analyses.
Throughout the discussion, few limitations of the present study were identified. Although the non-randomized cross-sectional design was informative considering the novelty of this research, it also warrants caution when making causal inferences. Moreover, the inclusion of other menstrual cycle phases (e.g., luteal phase) would have allowed for a more holistic portrait of NC women. Relatedly, our study is characterized by low external validity due to stringent sample criteria (e.g., no current or previous psychiatric/physical diagnosis). Thus, even if we were able to rule out any ‘survivor effect’ among current users (by having a group of past users), we had a very pure sample of individuals that may be distinct from other subgroups. Therefore, generalization of the results is limited and could explain discrepancies with the literature.
In addition, we identified several future directions and recommendations for the field. Above, we highlighted the relevance of exploring the following avenues: the relative role of sex and gender in terms of OC use and brain correlates, the lasting-but-reversible OC effects in women having recently stopped using OCs, and other OC parameters relevant to the discovery of long-term effects including duration of use and age of onset. Exploration of other structural properties and replication would also be of great interest. Moreover, replicating this study with other imaging methods (e.g., functional MRI) during a fear conditioning and extinction task would certainly allow for a better understanding of the current anatomical findings.
While our research does not have a direct clinical focus, it nonetheless contributes to advancing our fundamental understanding of the structural brain correlates of COC use. Our aim is to stimulate applied research by providing valuable insights and knowledge in the field of psychoneuroendocrinology. We first recommend evaluating the dose-dependent EE effects on cortical GM, then the clinical significance of our results, especially the atrophic effect of low EE doses. While our data may encourage prescribing COCs containing 30-35μg of EE for women presenting emotion-dysregulation psychopathologies, future research should prospectively investigate (in first-time COC users) the clinical effect size of such atrophy on fear-related symptomatology.
5 Conclusion
As OC use is so widespread, it is important to better understand its current and long-term effects on brain anatomy and emotional regulation. Our study demonstrated that COC intake can affect fear-related brain morphology, but that these effects can be reversible over time. Studying the brain circuitry underlying fear regulation and its modulation by endogenous and exogenous sex hormones could deepen our understanding of the etiology and maintenance of fear-related psychopathologies predominantly affecting women such as anxiety disorders and post-traumatic stress disorder.
Data availability statement
The raw data supporting the conclusions of this article will be made available by the authors, without undue reservation.
Ethics statement
The studies involving human participants were reviewed and approved by the research ethics board of the Centre intégré universitaire de santé et de services sociaux de l’Est-de-l’Île-de-Montréal. The patients/participants provided their written informed consent to participate in this study.
Author contributions
AB and MFM contributed to the conception and design of the study. AB, LMD, and AMT managed data collection. AB organized the database, performed the statistical analyses, and wrote the first draft of the manuscript. AMT wrote a section of the manuscript. All authors contributed to manuscript revision, read, and approved the submitted version.
Acknowledgments
We would like to thank Tristin P. Best, Élise B. Barbeau, Étienne Vachon-Presseau, and Mathieu Roy for their scientific insight and support, Charles-Édouard Giguère and Eugénie Samson-Daoust for their statistical aid, and Rebecca Cernik for linguistic revision. Finally, we want to acknowledge the considerable work devoted to data collection that was provided by Alexandra Laliberté, Anabelle Guérard, Gabrielle Wester, Shany Girouard, and Jessie Provencher.
Conflict of interest
The authors declare that the research was conducted in the absence of any commercial or financial relationships that could be construed as a potential conflict of interest.
Publisher’s note
All claims expressed in this article are solely those of the authors and do not necessarily represent those of their affiliated organizations, or those of the publisher, the editors and the reviewers. Any product that may be evaluated in this article, or claim that may be made by its manufacturer, is not guaranteed or endorsed by the publisher.
Supplementary material
The Supplementary Material for this article can be found online at: https://www.frontiersin.org/articles/10.3389/fendo.2023.1228504/full#supplementary-material
Abbreviations
AIC, Anterior insular cortex; BDI, Beck Depression Inventory; BTQ, Brief Trauma Questionnaire; COC, Combined oral contraceptives; CT, Cortical thickness; dACC, Dorsal anterior cingulate cortex; DARTEL, Diffeomorphic Anatomical Registration Through Lie Algebra; E2, Estradiol; EE, Ethinyl estradiol; ERα, Estrogen receptor alpha; FDR, False Discovery Rate; FWHM, Full-width half maximum; GLM, General linear model; GM, Gray matter; GMV, Gray matter volume; HPG, Hypothalamic-pituitary-gonadal; LC-MS/MS, Liquid chromatography – tandem mass spectrometry; LLOQ, Lower limit of quantification; LOD, Lower limit of detection; MEMPRAGE, Multi-Echo Magnetization-Prepared Rapid Gradient-Echo; MNI, Montreal Neurological Institute; MRI, Magnetic resonance imaging; NC, Naturally cycling; OCs, Oral contraceptives; P, Progesterone; PTSD, Post-traumatic stress disorder; rACC, Rostral anterior cingulate cortex; ROI, Region of interest; SBM, Surface-based morphometry; SCSRFQ, Santa Clara Strength of Religions Faith Questionnaire; SHBG, Sex hormone binding globulin; STAI-T, State and Trait Anxiety Questionnaire-Trait form; T, Testosterone; TIV, Total intracranial volume; VBM, Voxel-based morphometry; vmPFC, Ventromedial prefrontal cortex; WBA, Whole-brain analysis; WM, White matter.
References
1. Ekman P. An argument for basic emotions. Cogn Emotion (1992) 6(3/4):169–200. doi: 10.1080/02699939208411068
2. Hartley CA, Phelps EA. Changing fear: the neurocircuitry of emotion regulation. Neuropsychopharmacology (2010) 35(1):136–46. doi: 10.1038/npp.2009.121
3. McLean CP, Asnaani A, Litz BT, Hofmann SG. Gender differences in anxiety disorders: prevalence, course of illness, comorbidity and burden of illness. J Psychiatr Res (2011) 45(8):1027–35. doi: 10.1016/j.jpsychires.2011.03.006
4. Craske MG, Sandman CF, Stein MB. How can neurobiology of fear extinction inform treatment? Neurosci Biobehav Rev (2022) 143:104923. doi: 10.1016/j.neubiorev.2022.104923
5. Foa EB, McLean CP. The efficacy of exposure therapy for anxiety-related disorders and its underlying mechanisms: the case of OCD and PTSD. Annu Rev Clin Psychol (2016) 12(1):1–28. doi: 10.1146/annurev-clinpsy-021815-093533
6. Lebron-Milad K, Milad MR. Sex differences, gonadal hormones and the fear extinction network: implications for anxiety disorders. Biol Mood Anxiety Disord (2012) 2:3. doi: 10.1186/2045-5380-2-3
7. Forcadell E, Torrents-Rodas D, Vervliet B, Leiva D, Tortella-Feliu M, Fullana MA. Does fear extinction in the laboratory predict outcomes of exposure therapy? A treatment analog study. Int J Psychophysiol (2017) 121:63–71. doi: 10.1016/j.ijpsycho.2017.09.001
8. Graham BM, Li SH, Black MJ, Ost LG. The association between estradiol levels, hormonal contraceptive use, and responsiveness to one-session-treatment for spider phobia in women. Psychoneuroendocrinology (2018) 90:134–40. doi: 10.1016/j.psyneuen.2018.02.019
9. Knowles KA, Tolin DF. Mechanisms of action in exposure therapy. Curr Psychiatry Rep (2022) 24(12):861–9. doi: 10.1007/s11920-022-01391-8
10. McLean CP, Levy HC, Miller ML, Tolin DF. Exposure therapy for PTSD: A meta-analysis. Clin Psychol Review. (2022) 91:102115. doi: 10.1016/j.cpr.2021.102115
11. Scheveneels S, Boddez Y, Vervliet B, Hermans D. The validity of laboratory-based treatment research: Bridging the gap between fear extinction and exposure treatment. Behav Res Ther (2016) 86:87–94. doi: 10.1016/j.brat.2016.08.015
12. Duits P, Baas JMP, Engelhard IM, Richter J, Huisman - van Dijk HM, Limberg-Thiesen A, et al. Latent class growth analyses reveal overrepresentation of dysfunctional fear conditioning trajectories in patients with anxiety-related disorders compared to controls. J Anxiety Disord (2021) 78:102361. doi: 10.1016/j.janxdis.2021.102361
13. Greco JA, Liberzon I. Neuroimaging of fear-associated learning. Neuropsychopharmacology (2016) 41(1):320–34. doi: 10.1038/npp.2015.255
14. Marin MF, Hammoud MZ, Klumpp H, Simon NM, Milad MR. Multimodal categorical and dimensional approaches to understanding threat conditioning and its extinction in individuals with anxiety disorders. JAMA Psychiatry (2020) 77(6):618–27. doi: 10.1001/jamapsychiatry.2019.4833
15. Milad MR, Pitman RK, Ellis CB, Gold AL, Shin LM, Lasko NB, et al. Neurobiological basis of failure to recall extinction memory in posttraumatic stress disorder. Biol Psychiatry (2009) 66(12):1075–82. doi: 10.1016/j.biopsych.2009.06.026
16. Li SH, Graham BM. Why are women so vulnerable to anxiety, trauma-related and stress-related disorders? The potential role of sex hormones. Lancet Psychiatry (2017) 4(1):73–82. doi: 10.1016/S2215-0366(16)30358-3
17. Kaluve AM, Le JT, Graham BM. Female rodents are not more variable than male rodents: A meta-analysis of preclinical studies of fear and anxiety. Neurosci Biobehav Rev (2022) 143:104962. doi: 10.1016/j.neubiorev.2022.104962
18. Rechlin RK, Splinter TF, Hodges TE, Albert AY, Galea LAM. An analysis of neuroscience and psychiatry papers published from 2009 and 2019 outlines opportunities for increasing discovery of sex differences. Nat Commun (2022) 13(2137). doi: 10.1038/s41467-022-29903-3
19. Rocks D, Cham H, Kundakovic M. Why the estrous cycle matters for neuroscience. Biol Sex Differ (2022) 13(1):62. doi: 10.1186/s13293-022-00466-8
20. Weigard A, Loviska AM, Beltz AM. Little evidence for sex or ovarian hormone influences on affective variability. Sci Rep (2021) 11:20925. doi: 10.1038/s41598-021-00143-7
21. Graham BM. The impact of hormonal contraceptives on anxiety treatments: From preclinical models to clinical settings. Front Neuroendocrinol (2022) 67:101030. doi: 10.1016/j.yfrne.2022.101030
22. Hammoud MZ, Foa EB, Milad MR. Oestradiol, threat conditioning and extinction, post-traumatic stress disorder, and prolonged exposure therapy: A common link. J Neuroendocrinol (2020) 32(1):e12800. doi: 10.1111/jne.12800
23. United Nations. Contraceptive Use by Method 2019 (2019). Available at: https://www.un-ilibrary.org/content/books/9789210046527.
24. Dickey RP, Seymour ML. Managing Contraceptive Pill Patients and Other Hormonal Contraceptives. 17th ed. Fort Collins, CO: EMIS Medical Publishers (2021).
25. Rivera R, Yacobson I, Grimes D. The mechanism of action of hormonal contraceptives and intrauterine contraceptive devices. Am J Obstet Gynecol. (1999) 181(5):7. doi: 10.1016/S0002-9378(99)70120-1
26. Stanczyk FZ, Archer DF, Bhavnani BR. Ethinyl estradiol and 17β-estradiol in combined oral contraceptives: pharmacokinetics, pharmacodynamics and risk assessment. Contraception (2013) 87(6):706–27. doi: 10.1016/j.contraception.2012.12.011
27. Zimmerman Y, Eijkemans MJC, Coelingh Bennink HJT, Blankenstein MA, Fauser BCJM. The effect of combined oral contraception on testosterone levels in healthy women: a systematic review and meta-analysis. Hum Reprod Update (2014) 20(1):76–105. doi: 10.1093/humupd/dmt038
28. Cover KK, Maeng LY, Lebron-Milad K, Milad MR. Mechanisms of estradiol in fear circuitry: implications for sex differences in psychopathology. Transl Psychiatry (2014) 4:e422. doi: 10.1038/tp.2014.67
29. Glover EM, Jovanovic T, Mercer KB, Kerley K, Bradley B, Ressler KJ, et al. Estrogen levels are associated with extinction deficits in women with posttraumatic stress disorder. Biol Psychiatry (2012) 72(1):19–24. doi: 10.1016/j.biopsych.2012.02.031
30. Graham BM, Milad MR. Blockade of estrogen by hormonal contraceptives impairs fear extinction in female rats and women. Biol Psychiatry (2013) 73(4):371–8. doi: 10.1016/j.biopsych.2012.09.018
31. van Wingen GA, Ossewaarde L, Bäckström T, Hermans EJ, Fernández G. Gonadal hormone regulation of the emotion circuitry in humans. Neuroscience (2011) 191:38–45. doi: 10.1016/j.neuroscience.2011.04.042
32. Velasco ER, Florido A, Milad MR, Andero R. Sex differences in fear extinction. Neurosci Biobehav Rev (2019) 103:81–108. doi: 10.1016/j.neubiorev.2019.05.020
33. Hwang MJ, Zsido RG, Song H, Pace-Schott EF, Miller KK, Lebron-Milad K, et al. Contribution of estradiol levels and hormonal contraceptives to sex differences within the fear network during fear conditioning and extinction. BMC Psychiatry (2015) 15:295. doi: 10.1186/s12888-015-0673-9
34. Merz CJ, Tabbert K, Schweckendiek J, Klucken T, Vaitl D, Stark R, et al. Neuronal correlates of extinction learning are modulated by sex hormones. Soc Cogn Affect Neurosci (2012) 7(7):819–30. doi: 10.1093/scan/nsr063
35. Day HLL, Stevenson CW. The neurobiological basis of sex differences in learned fear and its inhibition. Eur J Neurosci (2020) 52(1):2466–86. doi: 10.1111/ejn.14602
36. Fullana MA, Harrison BJ, Soriano-Mas C, Vervliet B, Cardoner N, Àvila-Parcet A, et al. Neural signatures of human fear conditioning: an updated and extended meta-analysis of fMRI studies. Mol Psychiatry (2016) 21(4):500–8. doi: 10.1038/mp.2015.88
37. Fullana MA, Albajes-Eizagirre A, Soriano-Mas C, Vervliet B, Cardoner N, Benet O, et al. Fear extinction in the human brain: A meta-analysis of fMRI studies in healthy participants. Neurosci Biobehav Rev (2018) 88:16–25. doi: 10.1016/j.neubiorev.2018.03.002
38. Laing PAF, Felmingham KL, Davey CG, Harrison BJ. The neurobiology of Pavlovian safety learning: Towards an acquisition-expression framework. Neurosci Biobehav Rev (2022) 142:104882. doi: 10.1016/j.neubiorev.2022.104882
39. Milad MR, Quirk GJ. Fear extinction as a model for translational neuroscience: ten years of progress. Annu Rev Psychol (2012) 63:129–51. doi: 10.1146/annurev.psych.121208.131631
40. Shin LM, Liberzon I. The neurocircuitry of fear, stress, and anxiety disorders. Neuropsychopharmacology (2010) 35(1):169–91. doi: 10.1038/npp.2009.83
41. Marrocco J, McEwen BS. Sex in the brain: hormones and sex differences. Dialogues Clin Neurosci (2016) 18(4):373–83. doi: 10.31887/DCNS.2016.18.4/jmarrocco
42. Osterlund MK. Estrogen Receptor (ER ) Messenger Ribonucleic Acid (mRNA) Expression within the Human Forebrain: Distinct Distribution Pattern to ER mRNA. J Clin Endocrinol Metab (2000) 85(10):3840–6. doi: 10.1210/jc.85.10.3840
43. Wen Z, Hammoud MZ, Scott JC, Jimmy J, Brown L, Marin MF, et al. Impact of exogenous estradiol on task-based and resting-state neural signature during and after fear extinction in healthy women. Neuropsychopharmacol (2021) 46:2278–87. doi: 10.1038/s41386-021-01158-4
44. Zeidan MA, Igoe SA, Linnman C, Vitalo A, Levine JB, Klibanski A, et al. Estradiol modulates medial prefrontal cortex and amygdala activity during fear extinction in women and female rats. Biol Psychiatry (2011) 70(10):920–7. doi: 10.1016/j.biopsych.2011.05.016
45. Borgsted C, Hoegsted E, Henningsson S, Pinborg A, Ganz M, Frokjaer VG. Hippocampal volume changes in a pharmacological sex-hormone manipulation risk model for depression in women. Hormones Behavior (2022) 145:105234. doi: 10.1016/j.yhbeh.2022.105234
46. Lisofsky N, Mårtensson J, Eckert A, Lindenberger U, Gallinat J, Kühn S. Hippocampal volume and functional connectivity changes during the female menstrual cycle. NeuroImage (2015) 118:154–62. doi: 10.1016/j.neuroimage.2015.06.012
47. Pletzer B, Harris T, Hidalgo-Lopez E. Subcortical structural changes along the menstrual cycle: beyond the hippocampus. Sci Rep (2018) 8(1):16042. doi: 10.1038/s41598-018-34247-4
48. Protopopescu X, Butler T, Pan H, Root J, Altemus M, Polanecsky M, et al. Hippocampal structural changes across the menstrual cycle. Hippocampus (2008) 18(10):985–8. doi: 10.1002/hipo.20468
49. De Bondt T, Jacquemyn Y, Van Hecke W, Sijbers J, Sunaert S, Parizel PM. Regional gray matter volume differences and sex-hormone correlations as a function of menstrual cycle phase and hormonal contraceptives use. Brain Res (2013) 1530:22–31. doi: 10.1016/j.brainres.2013.07.034
50. De Bondt T, Pullens P, Van Hecke W, Jacquemyn Y, Parizel PM. Reproducibility of hormone-driven regional grey matter volume changes in women using SPM8 and SPM12. Brain Struct Funct (2016) 221(9):4631–41. doi: 10.1007/s00429-016-1193-1
51. Ossewaarde L, van Wingen GA, Rijpkema M, Bäckström T, Hermans EJ, Fernández G. Menstrual cycle-related changes in amygdala morphology are associated with changes in stress sensitivity. Hum Brain Mapp (2013) 34(5):1187–93. doi: 10.1002/hbm.21502
52. Hertel J, König J, Homuth G, van der Auwera S, Wittfeld K, Pietzner M, et al. Evidence for stress-like alterations in the HPA-axis in women taking oral contraceptives. Sci Rep (2017) 7(1):14111. doi: 10.1038/s41598-017-13927-7
53. Pletzer B. Sex hormones and gender role relate to gray matter volumes in sexually dimorphic brain areas. Front Neurosci (2019) 13:592. doi: 10.3389/fnins.2019.00592
54. Taylor CM, Pritschet L, Olsen RK, Layher E, Santander T, Grafton ST, et al. Progesterone shapes medial temporal lobe volume across the human menstrual cycle. NeuroImage (2020) 220:117125. doi: 10.1016/j.neuroimage.2020.117125
55. Lisofsky N, Riediger M, Gallinat J, Lindenberger U, Kuhn S. Hormonal contraceptive use is associated with neural and affective changes in healthy young women. Neuroimage (2016) 134:597–606. doi: 10.1016/j.neuroimage.2016.04.042
56. Chen KX, Worley S, Foster H, Edasery D, Roknsharifi S, Ifrah C, et al. Oral contraceptive use is associated with smaller hypothalamic and pituitary gland volumes in healthy women: A structural MRI study. PloS One (2021) 16(4):e0249482. doi: 10.1371/journal.pone.0249482
57. Petersen N, Touroutoglou A, Andreano JM, Cahill L. Oral contraceptive pill use is associated with localized decreases in cortical thickness: Oral Contraceptives Morphometry. Hum Brain Mapp (2015) 36(7):2644–54. doi: 10.1002/hbm.22797
58. Petersen N, Kearley NW, Ghahremani DG, Pochon JB, Fry ME, Rapkin AJ, et al. Effects of oral contraceptive pills on mood and magnetic resonance imaging measures of prefrontal cortical thickness. Mol Psychiatry (2021) 26:917–26. doi: 10.1038/s41380-020-00990-2
59. Rehbein E, Hornung J, Sundström Poromaa I, Derntl B. Shaping of the female human brain by sex hormones – a review. Neuroendocrinology (2020) 111(3):183–206. doi: 10.1159/000507083
60. Brønnick MK, Økland I, Graugaard C, Brønnick KK. The effects of hormonal contraceptives on the brain: A systematic review of neuroimaging studies. Front Psychol (2020) 11:556577. doi: 10.3389/fpsyg.2020.556577
61. Heller C, Kimmig ACS, Kubicki MR, Derntl B, Kikinis Z. Imaging the human brain on oral contraceptives: A review of structural imaging methods and implications for future research goals. Front Neuroendocrinol (2022) 67:101031. doi: 10.1016/j.yfrne.2022.101031
62. Oinonen KA, Mazmanian D. To what extent do oral contraceptives influence mood and affect? J Affect Disord (2002) 70(3):229–40. doi: 10.1016/S0165-0327(01)00356-1
63. DeSoto MC, Geary DC, Hoard MK, Sheldon MS, Cooper L. Estrogen fluctuations, oral contraceptives and borderline personality. Psychoneuroendocrinology (2003) 28(6):751–66. doi: 10.1016/S0306-4530(02)00068-9
64. Stidham Hall K, White KO, Rickert VI, Reame N, Westhoff C. Influence of depressed mood and psychological stress symptoms on perceived oral contraceptive side effects and discontinuation in young minority women. Contraception (2012) 86(5):518–25. doi: 10.1016/j.contraception.2012.04.010
65. Stenbæk DS, Budtz-Jørgensen E, Pinborg A, Jensen PS, Frokjaer VG. Neuroticism modulates mood responses to pharmacological sex hormone manipulation in healthy women. Psychoneuroendocrinology (2019) 99:251–6. doi: 10.1016/j.psyneuen.2018.10.016
66. Lindh I, Blohm F, Andersson-Ellstrom A, Milsom I. Contraceptive use and pregnancy outcome in three generations of Swedish female teenagers from the same urban population. Contraception (2009) 80(2):163—169. doi: 10.1016/j.contraception.2009.01.019
67. Pletzer B, Lang C, Derntl B, Griksiene R. Weak associations between personality and contraceptive choice. Front Neurosci (2022) 16:898487. doi: 10.3389/fnins.2022.898487
68. Piekarski DJ, Johnson CM, Boivin JR, Thomas AW, Lin WC, Delevich K, et al. Does puberty mark a transition in sensitive periods for plasticity in the associative neocortex? Brain Res (2017) 1654:123–44. doi: 10.1016/j.brainres.2016.08.042
69. Juraska JM, Willing J. Pubertal onset as a critical transition for neural development and cognition. Brain Res (2017) 1654(Pt B):87–94. doi: 10.1016/j.brainres.2016.04.012
70. Martínez-García M, Paternina-Die M, Barba-Müller E, Martín De Blas D, Beumala L, Cortizo R, et al. Do pregnancy-induced brain changes reverse? The brain of a mother six years after parturition. Brain Sci (2021) 11(2):168. doi: 10.3390/brainsci11020168
71. Carmona S, Martínez-García M, Paternina-Die M, Barba-Müller E, Wierenga LM, Alemán-Gómez Y, et al. Pregnancy and adolescence entail similar neuroanatomical adaptations: A comparative analysis of cerebral morphometric changes. Hum Brain Mapp (2019) 40(7):2143–52. doi: 10.1002/hbm.24513
72. Hoekzema E, Barba-Muller E, Pozzobon C, Picado M, Lucco F, Garcia-Garcia D, et al. Pregnancy leads to long-lasting changes in human brain structure. Nat Neurosci (2017) 20(2):287–96. doi: 10.1038/nn.4458
73. Kantarci K, Tosakulwong N, Lesnick TG, Zuk SM, Lowe VJ, Fields JA, et al. Brain structure and cognition 3 years after the end of an early menopausal hormone therapy trial. Neurology (2018) 90(16):e1404–12. doi: 10.1212/WNL.0000000000005325
74. Daniel JM, Witty CF, Rodgers SP. Long-term consequences of estrogens administered in midlife on female cognitive aging. Hormones Behavior (2015) 74:77–85. doi: 10.1016/j.yhbeh.2015.04.012
75. Chan MF, Dowsett M, Folkerd E, Wareham N, Luben R, Welch A, et al. Past oral contraceptive and hormone therapy use and endogenous hormone concentrations in postmenopausal women. Menopause (2008) 15(2):332–9. doi: 10.1097/gme.0b013e31806458d9
76. Pletzer B, Harris T, Hidalgo-Lopez E. Previous contraceptive treatment relates to grey matter volumes in the hippocampus and basal ganglia. Sci Rep (2019) 9. doi: 10.1038/s41598-019-47446-4
77. Schelbaum E, Loughlin L, Jett S, Zhang C, Jang G, Malviya N, et al. Association of reproductive history with brain MRI biomarkers of dementia risk in midlife. Neurology (2021) 97(23):e2328–39. doi: 10.1212/WNL.0000000000012941
78. Egan KR, Gleason CE. Longer duration of hormonal contraceptive use predicts better cognitive outcomes later in life. J Womens Health (Larchmt) (2012) 21(12):1259–66. doi: 10.1089/jwh.2012.3522
79. Noachtar IA, Hidalgo-Lopez E, Pletzer B. Duration of oral contraceptive use relates to cognitive performance and brain activation in current and past users. Front Endocrinol (2022) 13:885617. doi: 10.3389/fendo.2022.885617
80. Brouillard A, Davignon LM, Fortin J, Marin MF. A year through the COVID-19 pandemic: deleterious impact of hormonal contraception on psychological distress in women. Front Psychiatry (2022) 13:13. doi: 10.3389/fpsyt.2022.835857
81. Cheslack-Postava K, Keyes KM, Lowe SR, Koenen KC. Oral contraceptive use and psychiatric disorders in a nationally representative sample of women. Arch Womens Ment Health (2015) 18(1):103–11. doi: 10.1007/s00737-014-0453-4
82. Pletzer B, Kronbichler M, Kerschbaum H. Differential effects of androgenic and anti-androgenic progestins on fusiform and frontal gray matter volume and face recognition performance. Brain Res (2015) 1596:108–15. doi: 10.1016/j.brainres.2014.11.025
83. Pletzer B, Noachtar I, Hidalgo-Lopez E. Hormonal contraception & face processing: Examining face gender, androgenicity & treatment duration. Psychoneuroendocrinology (2023) 154:106292. doi: 10.1016/j.psyneuen.2023.106292
84. Hampson E. Oral contraceptives in the central nervous system: Basic pharmacology, methodological considerations, and current state of the field. Front Neuroendocrinol (2023) 68:101040. doi: 10.1016/j.yfrne.2022.101040
85. Lacasse JM, Gomez-Perales E, Brake WG. Modeling hormonal contraception in female rats: A framework for studies in behavioral neurobiology. Front Neuroendocrinol (2022) 67:101020. doi: 10.1016/j.yfrne.2022.101020
86. Sitruk-Ware R. Reprint of Pharmacological profile of progestins. Maturitas (2008) 61(1):151–7. doi: 10.1016/j.maturitas.2008.11.011
87. Beltz AM, Loviska AM, Kelly DP, Nielson MG. The link between masculinity and spatial skills is moderated by the estrogenic and progestational activity of oral contraceptives. Front Behav Neurosci (2022) 15:777911. doi: 10.3389/fnbeh.2021.777911
88. Griksiene R, Monciunskaite R, Arnatkeviciute A, Ruksenas O. Does the use of hormonal contraceptives affect the mental rotation performance? Hormones Behav (2018) 100:29–38. doi: 10.1016/j.yhbeh.2018.03.004
89. Griksiene R, Ruksenas O. Effects of hormonal contraceptives on mental rotation and verbal fluency. Psychoneuroendocrinology (2011) 36(8):1239–48. doi: 10.1016/j.psyneuen.2011.03.001
90. Gurvich C, Warren AM, Worsley R, Hudaib AR, Thomas N, Kulkarni J. Effects of oral contraceptive androgenicity on visuospatial and social-emotional cognition: A prospective observational trial. Brain Sci (2020) 10(4):194. doi: 10.3390/brainsci10040194
91. Wharton W, Hirshman E, Merritt P, Doyle L, Paris S, Gleason C. Oral contraceptives and androgenicity: influences on visuospatial task performance in younger individuals. Exp Clin Psychopharmacol (2008) 16(2):156–64. doi: 10.1037/1064-1297.16.2.156
92. Menting-Henry S, Hidalgo-Lopez E, Aichhorn M, Kronbichler M, Kerschbaum H, Pletzer B. Oral contraceptives modulate the relationship between resting brain activity, amygdala connectivity and emotion recognition – A resting state fMRI study. Front Behav Neurosci (2022) 16:775796. doi: 10.3389/fnbeh.2022.775796
93. Backhausen LL, Herting MM, Tamnes CK, Vetter NC. Best practices in structural neuroimaging of neurodevelopmental disorders. Neuropsychol Rev (2022) 32(2):400–18. doi: 10.1007/s11065-021-09496-2
94. Bethlehem RAI, Seidlitz J, White SR, Vogel JW, Anderson KM, Adamson C, et al. Brain charts for the human lifespan. Nature (2022) 604(7906):525–33. doi: 10.1038/s41586-022-04554-y
95. Ashburner J. Computational anatomy with the SPM software. Magnetic Resonance Imag (2009) 27(8):1163–74. doi: 10.1016/j.mri.2009.01.006
96. Beltz AM. Hormonal contraceptive influences on cognition and psychopathology: Past methods, present inferences, and future directions. Front Neuroendocrinol (2022) 67:101037. doi: 10.1016/j.yfrne.2022.101037
97. Bernal A, Paolieri D. The influence of estradiol and progesterone on neurocognition during three phases of the menstrual cycle: Modulating factors. Behav Brain Res (2022) 417:113593. doi: 10.1016/j.bbr.2021.113593
98. Catenaccio E, Mu W, Lipton ML. Estrogen- and progesterone-mediated structural neuroplasticity in women: evidence from neuroimaging. Brain Struct Funct (2016) 221(8):3845–67. doi: 10.1007/s00429-016-1197-x
99. Dubol M, Epperson CN, Sacher J, Pletzer B, Derntl B, Lanzenberger R, et al. Neuroimaging the menstrual cycle: A multimodal systematic review. Front Neuroendocrinol (2021) 60:100878. doi: 10.1016/j.yfrne.2020.100878
100. Gamsakhurdashvili D, Antov MI, Stockhorst U. Facial emotion recognition and emotional memory from the ovarian-hormone perspective: A systematic review. Front Psychol (2021) 12:641250. doi: 10.3389/fpsyg.2021.641250
101. Green SA, Graham BM. Symptom fluctuation over the menstrual cycle in anxiety disorders, PTSD, and OCD: a systematic review. Arch Womens Ment Health (2022) 25:71–85. doi: 10.1007/s00737-021-01187-4
102. Peyrot C, Brouillard A, Morand-Beaulieu S, Marin MF. A review on how stress modulates fear conditioning: Let’s not forget the role of sex and sex hormones. Behav Res Ther (2020) 129:103615. doi: 10.1016/j.brat.2020.103615
103. Arain M, Haque M, Johal L, Mathur P, Nel W, Rais A, et al. Maturation of the adolescent brain. Neuropsychiatr Dis Treat (2013) 9:449–61. doi: 10.2147/NDT.S39776
104. Casey BJ, Getz S, Galvan A. The adolescent brain. Dev Rev (2008) 28(1):62–77. doi: 10.1016/j.dr.2007.08.003
105. Grieger JA, Norman RJ. Menstrual cycle length and patterns in a global cohort of women using a mobile phone app: retrospective cohort study. J Med Internet Res (2020) 22(6):e17109. doi: 10.2196/17109
106. Baggio T, Grecucci A, Meconi F, Messina I. Anxious brains: A combined data fusion machine learning approach to predict trait anxiety from morphometric features. Sensors (2023) 23(2):610. doi: 10.3390/s23020610
107. Buggio L, Barbara G, Facchin F, Ghezzi L, Dridi D, Vercellini P. The influence of hormonal contraception on depression and female sexuality: a narrative review of the literature. Gynecological Endocrinol (2022) 38(3):193–201. doi: 10.1080/09513590.2021.2016693
108. Del Casale A, Ferracuti S, Barbetti AS, Bargagna P, Zega P, Iannuccelli A, et al. Grey matter volume reductions of the left hippocampus and amygdala in PTSD: A coordinate-based meta-analysis of magnetic resonance imaging studies. Neuropsychobiology (2022) 81(4):257–64. doi: 10.1159/000522003
109. Ibrahim HM, Kulikova A, Ly H, Rush AJ, Sherwood Brown E. Anterior cingulate cortex in individuals with depressive symptoms: A structural MRI study. Psychiatry Research: Neuroimaging (2022) 319:111420. doi: 10.1016/j.pscychresns.2021.111420
110. Rim JI, Ojeda JC, Svob C, Kayser J, Drews E, Kim Y, et al. Current understanding of religion, spirituality, and their neurobiological correlates. Harv Rev Psychiatry (2019) 27(5):303–16. doi: 10.1097/HRP.0000000000000232
111. Skovlund CW, Mørch LS, Kessing LV, Lidegaard Ø. Association of hormonal contraception with depression. JAMA Psychiatry (2016) 73(11):1154. doi: 10.1001/jamapsychiatry.2016.2387
112. Beck AT, Steer RA, Brown GK. Manual for the Beck Depression Inventory-II. San Antonio, TX: Psychological Corporation (1996).
113. Beck AT, Steer RA, Brown GK. Inventaire de dépression de Beck: BDI-II. Paris: les Éditions du Centre de psychologie appliquée (1998).
115. Schnurr P, Vielhauer M, Weathers F, Findler M. The Brief Trauma Questionnaire. White River Junction, VT: National Center for PTSD (1999).
116. McCrae RR, Costa PT. Brief versions of the NEO-PI-3. J Individ differences. (2007) 28(3):116–28. doi: 10.1027/1614-0001.28.3.116
117. Plante TG, Boccaccini MT. The santa clara strength of religious faith questionnaire. Pastoral Psychol (1997) 45(5):375–87. doi: 10.1007/BF02230993
118. Gauthier J, Bouchard S. Adaptation canadienne-française de la forme révisée du State–Trait Anxiety Inventory de Spielberger. Can J Behav Sci / Rev Can Des Sci du comportement. (1993) 25(4):559–78. doi: 10.1037/h0078881
119. Spielberger CD, Gorsuch RW, Lushene RE. State Trait Anxiety Inventory. Palo Alto: Consulting Psychologists Press (1970).
120. Xu L, Spink DC. Analysis of steroidal estrogens as pyridine-3-sulfonyl derivatives by liquid chromatography electrospray tandem mass spectrometry. Analytical Biochem (2008) 375(1):105–14. doi: 10.1016/j.ab.2007.11.028
121. Nave G, Nadler A, Dubois D, Zava D, Camerer C, Plassmann H. Single-dose testosterone administration increases men’s preference for status goods. Nat Commun (2018) 9(1):2433. doi: 10.1038/s41467-018-04923-0
122. Bedford SA, Park MTM, Devenyi GA, Tullo S, Germann J, Patel R, et al. Large-scale analyses of the relationship between sex, age and intelligence quotient heterogeneity and cortical morphometry in autism spectrum disorder. Mol Psychiatry (2020) 25(3):614–28. doi: 10.1038/s41380-019-0420-6
123. Sherif T, Rioux P, Rousseau ME, Kassis N, Beck N, Adalat R, et al. CBRAIN: a web-based, distributed computing platform for collaborative neuroimaging research. Front Neuroinform (2014) 8:54/abstract. doi: 10.3389/fninf.2014.00054/abstract
124. Destrieux C, Fischl B, Dale A, Halgren E. Automatic parcellation of human cortical gyri and sulci using standard anatomical nomenclature. NeuroImage (2010) 53(1):1–15. doi: 10.1016/j.neuroimage.2010.06.010
125. Destrieux C, Terrier LM, Andersson F, Love SA, Cottier JP, Duvernoy H, et al. A practical guide for the identification of major sulcogyral structures of the human cortex. Brain Struct Funct (2017) 222(4):2001–15. doi: 10.1007/s00429-016-1320-z
126. Vogt BA, Berger GR, Derbyshire SWG. Structural and functional dichotomy of human midcingulate cortex. Eur J Neurosci (2003) 18(11):3134–44. doi: 10.1111/j.1460-9568.2003.03034.x
127. Billot B, Bocchetta M, Todd E, Dalca AV, Rohrer JD, Iglesias JE. Automated segmentation of the hypothalamus and associated subunits in brain MRI. NeuroImage (2020) 223:117287. doi: 10.1016/j.neuroimage.2020.117287
128. Marek S, Tervo-Clemmens B, Calabro FJ, Montez DF, Kay BP, Hatoum AS, et al. Reproducible brain-wide association studies require thousands of individuals. Nature (2022) 603(7902):654–60. doi: 10.1038/s41586-022-04492-9
130. Botvinik-Nezer R, Wager TD. Reproducibility in neuroimaging analysis: challenges and solutions. Biol Psychiatry: Cogn Neurosci Neuroimaging (2022) 8(8):780–8. doi: 10.1016/j.bpsc.2022.12.006
131. Jünger J, Motta-Mena NV, Cardenas R, Bailey D, Rosenfield KA, Schild C, et al. Do women’s preferences for masculine voices shift across the ovulatory cycle? Hormones Behav (2018) 106:122–34. doi: 10.1016/j.yhbeh.2018.10.008
132. Herbers J, Miller R, Walther A, Schindler L, Schmidt K, Gao W, et al. How to deal with non-detectable and outlying values in biomarker research: Best practices and recommendations for univariate imputation approaches. Compr Psychoneuroendocrinol (2021) 7:100052. doi: 10.1016/j.cpnec.2021.100052
133. Delignette-Muller ML, Dutang C. fitdistrplus: an R package for fitting distributions. J Stat Soft (2015) 64(4):1–34. doi: 10.18637/jss.v064.i04
135. Canales RA, Wilson AM, Pearce-Walker JI, Verhougstraete MP, Reynolds KA. Methods for handling left-censored data in quantitative microbial risk assessment. Appl Environ Microbiol (2018) 84(20):e01203–18. Schaffner DW. doi: 10.1128/AEM.01203-18
136. Field A. Discovering Statistics Using IBM SPSS Statistics. 4th ed. London, England: SAGE Publications (2013).
137. Tabachnick BG, Fidell LS. Using Multivariate Statistics. 6th ed. Boston: Person Education (2012).
138. Blaine BE. Winsorizing. In: The SAGE Encyclopedia of Educational Research, Measurement, and Evaluation. Thousand Oaks, California: SAGE Publications, Inc. (2018). p. 1817–8.
139. Barnes J, Ridgway GR, Bartlett J, Henley SMD, Lehmann M, Hobbs N, et al. Head size, age and gender adjustment in MRI studies: a necessary nuisance? NeuroImage (2010) 53(4):1244–55. doi: 10.1016/j.neuroimage.2010.06.025
140. Goto M, Abe O, Hagiwara A, Fujita S, Kamagata K, Hori M, et al. Advantages of using both voxel- and surface-based morphometry in cortical morphology analysis: A review of various applications. MRMS (2022) 21(1):41–57. doi: 10.2463/mrms.rev.2021-0096
141. VanderWeele TJ. Principles of confounder selection. Eur J Epidemiol (2019) 34(3):211–9. doi: 10.1007/s10654-019-00494-6
142. Benjamini Y, Hochberg Y. Controlling the false discovery rate: A practical and powerful approach to multiple testing. J R Stat Society: Ser B (Methodological) (1995) 57(1):289–300. doi: 10.1111/j.2517-6161.1995.tb02031.x
143. Liening SH, Stanton SJ, Saini EK, Schultheiss OC. Salivary testosterone, cortisol, and progesterone: Two-week stability, interhormone correlations, and effects of time of day, menstrual cycle, and oral contraceptive use on steroid hormone levels. Physiol Behavior. (2010) 99(1):8–16. doi: 10.1016/j.physbeh.2009.10.001
144. Bao A, Liu R, Van Someren E, Hofman M, Cao Y, Zhou J. Diurnal rhythm of free estradiol during the menstrual cycle. Eur J Endocrinol (2003) 148(2):227–32. doi: 10.1530/eje.0.1480227
145. Van Anders SM, Goldey KL, Bell SN. Measurement of testosterone in human sexuality research: methodological considerations. Arch Sex Behav (2014) 43(2):231–50. doi: 10.1007/s10508-013-0123-z
146. Brun CC, Leporé N, Luders E, Chou YY, Madsen SK, Toga AW, et al. Sex differences in brain structure in auditory and cingulate regions. NeuroReport (2009) 20(10):930–5. doi: 10.1097/WNR.0b013e32832c5e65
147. DeCasien AR, Guma E, Liu S, Raznahan A. Sex differences in the human brain: a roadmap for more careful analysis and interpretation of a biological reality. Biol Sex Differ (2022) 13(1):43. doi: 10.1186/s13293-022-00448-w
148. Liu S, Seidlitz J, Blumenthal JD, Clasen LS, Raznahan A. Integrative structural, functional, and transcriptomic analyses of sex-biased brain organization in humans. Proc Natl Acad Sci USA (2020) 117(31):18788–98. doi: 10.1073/pnas.1919091117
149. Ruigrok ANV, Salimi-Khorshidi G, Lai MC, Baron-Cohen S, Lombardo MV, Tait RJ, et al. A meta-analysis of sex differences in human brain structure. Neurosci Biobehav Rev (2014) 39:34–50. doi: 10.1016/j.neubiorev.2013.12.004
150. Sisk CL, Zehr JL. Pubertal hormones organize the adolescent brain and behavior. Front Neuroendocrinol (2005) 26(3–4):163–74. doi: 10.1016/j.yfrne.2005.10.003
151. Ehrlich E, Gibson T, Mark T. Trends in prescriptions for oral contraceptives among US teenagers. Truven Health Analytics (2011).
152. Hales CM, Kit BK, Gu Q, Ogden CL. Trends in prescription medication use among children and adolescents—United States, 1999-2014. JAMA (2018) 319(19):2009. doi: 10.1001/jama.2018.5690
153. Statistique Canada. Oral contraceptive use among women aged 15 to 49: Results from the Canadian Health Measures Survey, Catalogue no. 82-003-X. Health Reports (2015) 26(10):21–8. Available at https://www150.statcan.gc.ca/n1/en/pub/82-003-x/2015010/article/14222-eng.pdf.
154. Blakemore SJ, Burnett S, Dahl RE. The role of puberty in the developing adolescent brain. Hum Brain Mapp (2010) 31(6):926–33. doi: 10.1002/hbm.21052
155. McCormick CM, Mathews IZ. Adolescent development, hypothalamic-pituitary-adrenal function, and programming of adult learning and memory. Prog Neuropsychopharmacol Biol Psychiatry (2010) 34(5):756–65. doi: 10.1016/j.pnpbp.2009.09.019
156. Neufang S, Specht K, Hausmann M, Gunturkun O, Herpertz-Dahlmann B, Fink GR, et al. Sex differences and the impact of steroid hormones on the developing human brain. Cereb Cortex (2009) 19(2):464–73. doi: 10.1093/cercor/bhn100
157. Stefanick ML, Schiebinger L. Analysing how sex and gender interact. Lancet (2020) 396(10262):1553–4. doi: 10.1016/S0140-6736(20)32346-1
158. Etkin A, Egner T, Kalisch R. Emotional processing in anterior cingulate and medial prefrontal cortex. Trends Cogn Sci (2011) 15(2):85–93. doi: 10.1016/j.tics.2010.11.004
159. Shang J, Fu Y, Ren Z, Zhang T, Du M, Gong Q, et al. The common traits of the ACC and PFC in anxiety disorders in the DSM-5: meta-analysis of voxel-based morphometry studies. PloS One (2014) 9(3):e93432. doi: 10.1371/journal.pone.0093432
160. Giuliani NR, Drabant EM, Gross JJ. Anterior cingulate cortex volume and emotion regulation: Is bigger better? Biol Psychol (2011) 86(3):379–82. doi: 10.1016/j.biopsycho.2010.11.010
161. Grabe HJ, Wittfeld K, Hegenscheid K, Hosten N, Lotze M, Janowitz D, et al. Alexithymia and brain gray matter volumes in a general population sample: Alexithymia and Gray Matter. Hum Brain Mapp (2014) 35(12):5932–45. doi: 10.1002/hbm.22595
162. Nouchi R, Takeuchi H, Taki Y, Sekiguchi A, Kotozaki Y, Nakagawa S, et al. Neuroanatomical bases of effortful control: evidence from a large sample of young healthy adults using voxel-based morphometry. Sci Rep (2016) 6(1):31231. doi: 10.1038/srep31231
163. Wei L, Guo N, Baeken C, Bi M, Wang X, Qiu J, et al. Grey matter volumes in the executive attention system predict individual differences in effortful control in young adults. Brain Topogr (2019) 32(1):111–7. doi: 10.1007/s10548-018-0676-1
164. Apetroaia A, Hill C, Creswell C. Parental responsibility beliefs: associations with parental anxiety and behaviours in the context of childhood anxiety disorders. J Affect Disord (2015) 188:127–33. doi: 10.1016/j.jad.2015.08.059
165. Eek F, Axmon A. Gender inequality at home is associated with poorer health for women. Scand J Public Health (2015) 43(2):176–82. doi: 10.1177/1403494814562598
166. McLean CP, Anderson ER. Brave men and timid women? A review of the gender differences in fear and anxiety. Clin Psychol Review (2009) 29(6):496–505. doi: 10.1016/j.cpr.2009.05.003
167. Raznahan A, Lee Y, Stidd R, Long R, Greenstein D, Clasen L, et al. Longitudinally mapping the influence of sex and androgen signaling on the dynamics of human cortical maturation in adolescence. Proc Natl Acad Sci USA (2010) 107(39):16988–93. doi: 10.1073/pnas.1006025107
168. Yang G, Bozek J, Han M, Gao J. Constructing and evaluating a cortical surface atlas and analyzing cortical sex differences in young Chinese adults. Hum Brain Mapp. (2020) 41(9):2495–513. doi: 10.1002/hbm.24960
169. Eliot L, Ahmed A, Khan H, Patel J. Dump the “dimorphism”: Comprehensive synthesis of human brain studies reveals few male-female differences beyond size. Neurosci Biobehav Rev (2021) 125:667–97. doi: 10.1016/j.neubiorev.2021.02.026
170. Ritchie SJ, Cox SR, Shen X, Lombardo MV, Reus LM, Alloza C, et al. Sex differences in the adult human brain: evidence from 5216 UK biobank participants. Cereb Cortex (2018) 28(8):2959–75. doi: 10.1093/cercor/bhy109
171. Sowell ER, Peterson BS, Kan E, Woods RP, Yoshii J, Bansal R, et al. Sex differences in cortical thickness mapped in 176 healthy individuals between 7 and 87 years of age. Cereb Cortex (2007) 17(7):1550–60. doi: 10.1093/cercor/bhl066
172. Milad MR, Quinn BT, Pitman RK, Orr SP, Fischl B, Rauch SL. Thickness of ventromedial prefrontal cortex in humans is correlated with extinction memory. Proc Natl Acad Sci USA (2005) 102(30):10706–11. doi: 10.1073/pnas.0502441102
173. Winkelmann T, Grimm O, Pohlack ST, Nees F, Cacciaglia R, Dinu-Biringer R, et al. Brain morphology correlates of interindividual differences in conditioned fear acquisition and extinction learning. Brain Struct Funct (2016) 221(4):1927–37. doi: 10.1007/s00429-015-1013-z
174. Cha J, Greenberg T, Carlson JM, DeDora DJ, Hajcak G, Mujica-Parodi LR. Circuit-wide structural and functional measures predict ventromedial prefrontal cortex fear generalization: implications for generalized anxiety disorder. J Neurosci (2014) 34(11):4043–53. doi: 10.1523/JNEUROSCI.3372-13.2014
175. Jeong H, Lee YJ, Kim N, Jeon S, Jun JY, Yoo SY, et al. Increased medial prefrontal cortical thickness and resilience to traumatic experiences in North Korean refugees. Sci Rep (2021) 11(1):14910. doi: 10.1038/s41598-021-94452-6
176. Hoexter MQ, Diniz JB, Lopes AC, Batistuzzo MC, Shavitt RG, Dougherty DD, et al. Orbitofrontal thickness as a measure for treatment response prediction in obsessive-compulsive disorder. Depress Anxiety (2015) 32(12):900–8. doi: 10.1002/da.22380
177. Albaugh MD, Hudziak James J, Orr C, Spechler PA, Chaarani B, Mackey S, et al. Amygdalar reactivity is associated with prefrontal cortical thickness in a large population-based sample of adolescents. PloS One (2019) 14(5):e0216152. doi: 10.1371/journal.pone.0216152
178. Giustino TF, Maren S. The role of the medial prefrontal cortex in the conditioning and extinction of fear. Front Behav Neurosci (2015) 9:298/abstract. doi: 10.3389/fnbeh.2015.00298/abstract
179. Raeder F, Heidemann F, Schedlowski M, Margraf J, Zlomuzica A. No pills, more skills: The adverse effect of hormonal contraceptive use on exposure therapy benefit. J Psychiatr Res (2019) 119:95–101. doi: 10.1016/j.jpsychires.2019.09.016
180. Elliott ML, Knodt AR, Ireland D, Morris ML, Poulton R, Ramrakha S, et al. What is the test-retest reliability of common task-functional MRI measures? New empirical evidence and a meta-analysis. Psychol Sci (2020) 31(7):792–806. doi: 10.1177/0956797620916786
181. Ehlers MR, Nold J, Kuhn M, Klingelhöfer-Jens M, Lonsdorf TB. Revisiting potential associations between brain morphology, fear acquisition and extinction through new data and a literature review. Sci Rep (2020) 10(1):19894. doi: 10.1038/s41598-020-76683-1
182. Kharabian Masouleh S, Eickhoff SB, Hoffstaedter F, Genon S, Alzheimer’s Disease Neuroimaging Initiative. Empirical examination of the replicability of associations between brain structure and psychological variables. eLife (2019) 8:e43464. doi: 10.7554/eLife.43464.001
183. Craig AD. How do you feel — now? The anterior insula and human awareness. Nat Rev Neurosci (2009) 10(1):59–70. doi: 10.1038/nrn2555
184. Gasquoine PG. Contributions of the insula to cognition and emotion. Neuropsychol Rev (2014) 24(2):77–87. doi: 10.1007/s11065-014-9246-9
185. Uddin LQ, Nomi JS, Hébert-Seropian B, Ghaziri J, Boucher O. Structure and function of the human insula. J Clin Neurophysiol (2017) 34(4):300–6. doi: 10.1097/WNP.0000000000000377
186. Shankman SA, Gorka SM, Nelson BD, Fitzgerald DA, Phan KL, O’Daly O. Anterior insula responds to temporally unpredictable aversiveness: an fMRI study. NeuroReport (2014) 25(8):596–600. doi: 10.1097/WNR.0000000000000144
187. Manzler CA, Radoman M, Khorrami KJ, Gorka SM. Association between startle reactivity to uncertain threats and structural brain volume. Psychophysiology (2022) 59(10):e14074. doi: 10.1111/psyp.14074
188. Anderl C, Li G, Chen FS. Oral contraceptive use in adolescence predicts lasting vulnerability to depression in adulthood. J Child Psychol Psychiatr (2020) 61(2):148–56. doi: 10.1111/jcpp.13115
189. Anderl C, Wit AE, Giltay EJ, Oldehinkel AJ, Chen FS. Association between adolescent oral contraceptive use and future major depressive disorder: a prospective cohort study. Child Psychol Psychiatry (2022) 63(3):333–41. doi: 10.1111/jcpp.13476
190. de Wit AE, Booij SH, Giltay EJ, Joffe H, Schoevers RA, Oldehinkel AJ. Association of use of oral contraceptives with depressive symptoms among adolescents and young women. JAMA Psychiatry (2020) 77(1):52. doi: 10.1001/jamapsychiatry.2019.2838
191. Cahill L. How does hormonal contraception affect the developing human adolescent brain? Curr Opin Behav Sci (2018) 23:131–5. doi: 10.1016/j.cobeha.2018.06.015
192. Taylor CM, Pritschet L, Jacobs EG. The scientific body of knowledge – whose body does it serve? A spotlight on oral contraceptives and women’s health factors in neuroimaging. Front Neuroendocrinol (2021) 60:100874. doi: 10.1016/j.yfrne.2020.100874
193. Baroncini M, Jissendi P, Catteau-Jonard S, Dewailly D, Pruvo JP, Francke JP, et al. Sex steroid hormones-related structural plasticity in the human hypothalamus. NeuroImage (2010) 6:428–33. doi: 10.1016/j.neuroimage.2009.11.074
194. Barth C, Villringer A, Sacher J. Sex hormones affect neurotransmitters and shape the adult female brain during hormonal transition periods. Front Neurosci (2015) 9(37):1–20. doi: 10.3389/fnins.2015.00037
195. Barth C, Steele CJ, Mueller K, Rekkas VP, Arélin K, Pampel A, et al. In-vivo dynamics of the human hippocampus across the menstrual cycle. Sci Rep (2016) 6(1):32833. doi: 10.1038/srep32833
196. Arslan RC, Blake K, Botzet LJ, Bürkner PC, DeBruine L, Fiers T, et al. Not within spitting distance: Salivary immunoassays of estradiol have subpar validity for predicting cycle phase. Psychoneuroendocrinology (2023) 149:105994. doi: 10.1016/j.psyneuen.2022.105994
197. Schultheiss OC, Dlugash G, Mehta PH. Hormone measurement in social neuroendocrinology. In: Schultheiss OC, Mehta PH, editors. Routledge International Handbook of Social Neuroendocrinology, 1st ed, vol. 2018 . Abingdon, Oxon ; New York: NY : Routledge (2019). p. 26–40. doi: 10.4324/9781315200439-3
198. Stanczyk FZ, Clarke NJ. Advantages and challenges of mass spectrometry assays for steroid hormones. J Steroid Biochem Mol Biol (2010) 121(3–5):491–5. doi: 10.1016/j.jsbmb.2010.05.001
199. Taylor AE, Keevil B, Huhtaniemi IT. Mass spectrometry and immunoassay: how to measure steroid hormones today and tomorrow. Eur J Endocrinol (2015) 173(2):D1–12. doi: 10.1530/EJE-15-0338
200. Gavrilova N, Lindau ST. Salivary sex hormone measurement in a national, population-based study of older adults. Journals Gerontol Ser B: psychol Sci Soc Sci (2009) 64B(Supplement 1):i94–105. doi: 10.1093/geronb/gbn028
201. Worthman CM, Stallings JF, Hofman LF. Sensitive salivary estradiol assay for monitoring ovarian function. Clin Chem (1990) 36(10):1769–73. doi: 10.1093/clinchem/36.10.1769
202. Woolley CS, McEwen BS. Roles of estradiol and progesterone in regulation of hippocampal dendritic spine density during the estrous cycle in the rat. J Comp Neurol (1993) 336(2):293–306. doi: 10.1002/cne.903360210
203. Escande A, Pillon A, Servant N, Cravedi JP, Larrea F, Muhn P, et al. Evaluation of ligand selectivity using reporter cell lines stably expressing estrogen receptor alpha or beta. Biochem Pharmacol (2006) 71(10):1459–69. doi: 10.1016/j.bcp.2006.02.002
204. Jeyakumar M, Carlson KE, Gunther JR, Katzenellenbogen JA. Exploration of dimensions of estrogen potency. J Biol Chem (2011) 286(15):12971–82. doi: 10.1074/jbc.M110.205112
205. Maioli S, Leander K, Nilsson P, Nalvarte I. Estrogen receptors and the aging brain. Essays Biochem (2021) 65(6):913–25. doi: 10.1042/EBC20200162
206. Montague D, Weickert CS, Tomaskovic-Crook E, Rothmond DA, Kleinman JE, Rubinow DR. Oestrogen receptor α Localisation in the prefrontal cortex of three mammalian species. J Neuroendocrinol (2008) 20(7):893–903. doi: 10.1111/j.1365-2826.2008.01743.x
207. Khan MM, Dhandapani KM, Brann DW. Estrogen regulation of spine density and excitatory synapses in rat prefrontal and somatosensory cerebral cortex. Steroids (2013) 78(6):614–23. doi: 10.1016/j.steroids.2012.12.005
208. Wallace M, Luine V, Arellanos A, Frankfurt M. Ovariectomized rats show decreased recognition memory and spine density in the hippocampus and prefrontal cortex. Brain Res (2006) 1126(1):176–82. doi: 10.1016/j.brainres.2006.07.064
209. Graham BM, Scott E. Effects of systemic estradiol on fear extinction in female rats are dependent on interactions between dose, estrous phase, and endogenous estradiol levels. Hormones Behavior (2018) 97:67–74. doi: 10.1016/j.yhbeh.2017.10.009
210. Bayer J, Gläscher J, Finsterbusch J, Schulte LH, Sommer T. Linear and inverted U-shaped dose-response functions describe estrogen effects on hippocampal activity in young women. Nat Commun (2018) 9(1):1220. doi: 10.1038/s41467-018-03679-x
211. Golobof A, Kiley J. The current status of oral contraceptives: progress and recent innovations. Semin Reprod Med (2016) 34(03):145–51. doi: 10.1055/s-0036-1572546
212. Casto KV, Jordan T, Petersen N. Hormone-based models for comparing menstrual cycle and hormonal contraceptive effects on human resting-state functional connectivity. Front Neuroendocrinol (2022) 67:101036. doi: 10.1016/j.yfrne.2022.101036
Keywords: sex hormones, oral contraceptives, structural MRI, fear circuitry, gray matter volume, cortical thickness
Citation: Brouillard A, Davignon L-M, Turcotte A-M and Marin M-F (2023) Morphologic alterations of the fear circuitry: the role of sex hormones and oral contraceptives. Front. Endocrinol. 14:1228504. doi: 10.3389/fendo.2023.1228504
Received: 24 May 2023; Accepted: 27 September 2023;
Published: 07 November 2023.
Edited by:
Caroline Gurvich, Monash University, AustraliaReviewed by:
Joanna Spencer-Segal, University of Michigan, United StatesDamian Gabriel Zuloaga, University at Albany, United States
Copyright © 2023 Brouillard, Davignon, Turcotte and Marin. This is an open-access article distributed under the terms of the Creative Commons Attribution License (CC BY). The use, distribution or reproduction in other forums is permitted, provided the original author(s) and the copyright owner(s) are credited and that the original publication in this journal is cited, in accordance with accepted academic practice. No use, distribution or reproduction is permitted which does not comply with these terms.
*Correspondence: Marie-France Marin, bWFyaW4ubWFyaWUtZnJhbmNlQHVxYW0uY2E=