- Eye Center, Renmin Hospital of Wuhan University, Wuhan, Hubei, China
Diabetic microangiopathy is a typical and severe problem in diabetics, including diabetic retinopathy, diabetic nephropathy, diabetic neuropathy, and diabetic cardiomyopathy. Patients with type 2 diabetes and diabetic microvascular complications have significantly elevated levels of Asymmetric dimethylarginine (ADMA), which is an endogenous inhibitor of nitric oxide synthase (NOS). ADMA facilitates the occurrence and progression of microvascular complications in type 2 diabetes through its effects on endothelial cell function, oxidative stress damage, inflammation, and fibrosis. This paper reviews the association between ADMA and microvascular complications of diabetes and elucidates the underlying mechanisms by which ADMA contributes to these complications. It provides a new idea and method for the prevention and treatment of microvascular complications in type 2 diabetes.
1 Introduction
As the global economy progresses and lifestyles change, the prevalence of diabetes is increasing annually in both developed and developing countries (1–3). According to recent statistical research, by 2040, it is anticipated that 642 million people will be diagnosed with diabetes (2). Meanwhile, there has been a significant increase in the incidence of diabetic microvascular complications (3). Diabetic microvascular complications include diabetic retinopathy, diabetic nephropathy, diabetic neuropathy, and diabetic cardiomyopathy. These complications can significantly impact patients’ quality of life and increase their risk of mortality (4, 5). The pathogenesis of diabetic microangiopathy involves several mechanisms, including activation of the protein kinase C (PKC) pathway and accumulation of advanced glycation end products (AGEs), chronic inflammation mediated by the kinin system, oxidative stress injury induced by the NADPH oxidase-reactive oxygen species (NOX-ROS) pathway, and transforming growth factor-beta (TGF-β)-induced fibrosis (6).
Previous studies have suggested that asymmetric dimethylarginine (ADMA), an endogenous inhibitor of nitric oxide synthase, plays an important role in the pathogenesis of diabetic microangiopathy (7). AGEs can enhance ADMA synthesis, leading to endothelial dysfunction (8). Furthermore, ADMA is positively correlated with C-reactive protein (CRP) (9), and can activate macrophages and monocytes to mediate inflammatory responses (10, 11). Additionally, ADMA may promote tissue fibrosis by upregulating TGF-β expression (12). However, the precise mechanisms underlying the effects of ADMA in diabetic microvascular complications remain poorly understood. The aim of this literature review is to provide an overview of the role of ADMA in the pathogenesis of microvascular complications in diabetes and to suggest potential therapeutic strategies and novel therapeutic targets for the prevention and treatment of these complications.
2 Synthesis and metabolism of ADMA
2.1 Synthesis of ADMA
Asymmetric dimethylarginine (ADMA) is generated from post-translational methylation modification of arginine residues in proteins by protein arginine methyltransferases (PRMTs) and is released during proteolysis (13). ADMA is widely present in mammalian bodies, including plasma, tissue fluids, and cytoplasm. There are three known arginine residues, namely NG-monomethyl-L-arginine (L-NMMA), asymmetric NG, NG-dimethyl-L-arginine (ADMA), and symmetric NG, N’G-dimethyl-L-arginine (SDMA) (14, 15). Type I protein arginine methyltransferases (PRMT-I) or type II protein arginine methyltransferase (PRMT-II) can catalyze the formation of L-NMMA, whereas PRMT-I and PRMT-II catalyze the formation of ADMA and SDMA, respectively (16, 17). As endogenous nitric oxide synthase (NOS) inhibitors, L-NMMA and ADMA play an essential role in inhibiting nitric oxide (NO) production (14). However, while SDMA does not significantly affect NOS expression, it still inhibits NO synthesis, most likely due to its ability to competitively inhibit arginine transport (18). Interestingly, the concentration of ADMA in the blood is significantly higher than that of NMMA (15). As a result, over the past few decades, a growing number of studies have focused on the effects of ADMA on vascular diseases, while NMMA and SDMA have received less attention (19, 20) (Figure 1)
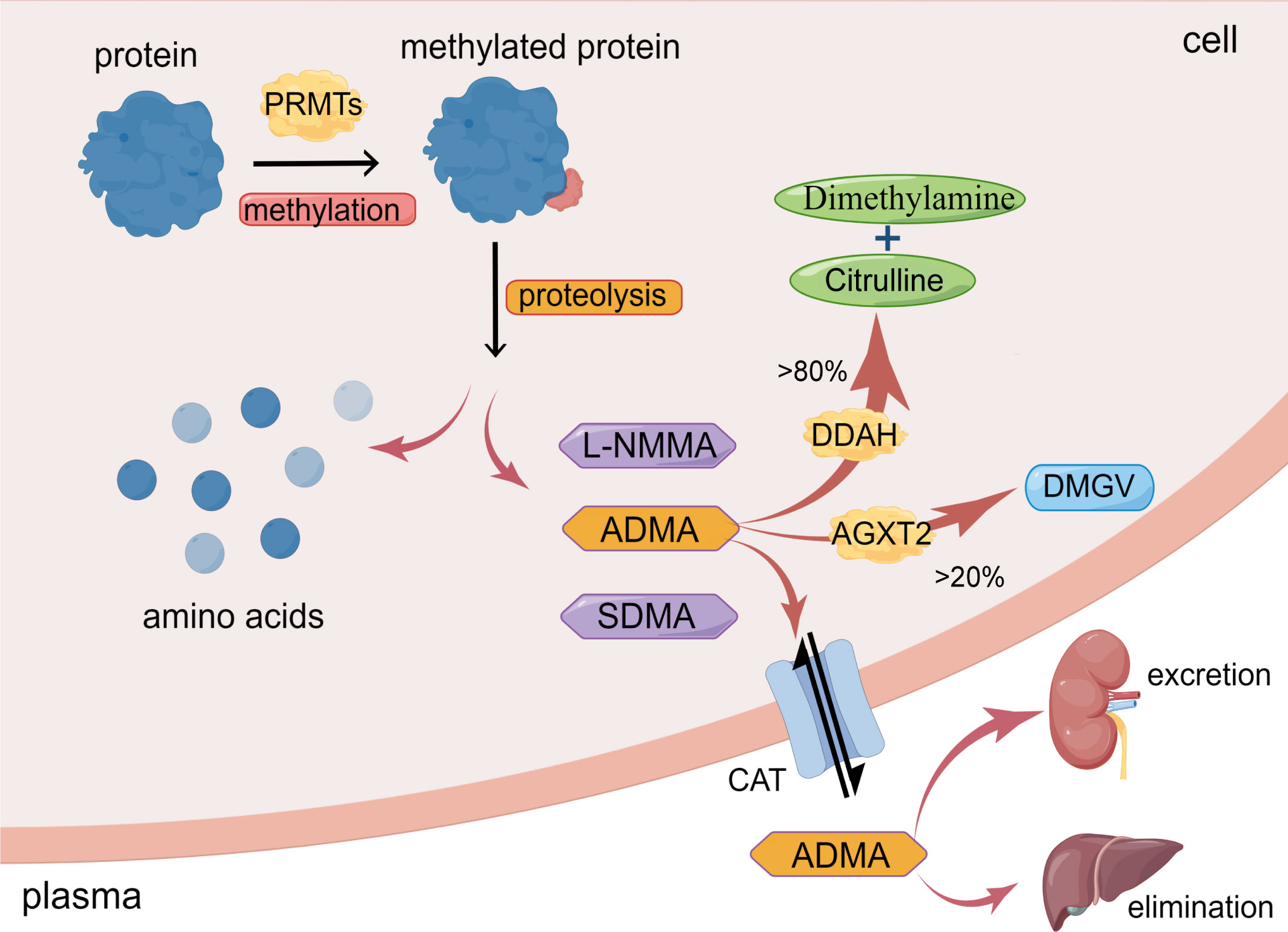
Figure 1 Synthesis and metabolism of ADMA. ADMA is formed by the post-transcriptional methylation of proteins mediated by PRMTs. Amino acids and methylarginines such as ADMA, L-NMMA, and SDMA are subsequently released during protein proteolysis. >80% ADMA is hydrolyzed by DDAH to dimethylamine and citrulline; <20% ADMA is degraded by AGXT2 to DMGV. ADMA can also enter circulation via CAT and excretion by the kidney or elimination by the liver. (The figure was created using Figdraw).
Under normal physiological conditions, healthy adults have an average plasma ADMA level ranging between 0.4-0.6μmol/L (21). However, in pathological conditions such as hypertension and hypercholesterolemia, the plasma level of ADMA in patients can increase up to two times (21). In patients with chronic renal failure, the plasma level of ADMA can increase by almost eight times (22). This increase in ADMA levels in pathological conditions may be due to the increased synthesis and decreased degradation or excretion of ADMA. Physiologically, the intracellular concentration of ADMA is around 3.6 μmol/L, resulting in a mere 10% reduction in the production of nitric oxide (NO). However, if the plasma level of ADMA is increased by 3-9 times, it can significantly impact the intracellular concentration of ADMA, leading to a much greater inhibitory effect on NO synthesis, ranging from 30% to 70% (23, 24).
Intracellular L-arginine can produce L-citrulline and NO under the catalysis of NOS (25). ADMA competes with the substrate L-arginine for binding to the active site of NOS, leading to a reduction in NO synthesis (26). Nevertheless, this inhibitory effect can be reversed by the exogenous addition of L-arginine (22). This suggests that maintaining an appropriate ratio of L-arginine to ADMA may be crucial for proper NOS function. High levels of ADMA can lead to reduced production of NO, which impairs endothelial function and increases the risk of cardiovascular events such as atherosclerosis and hypertension (27). However, L-arginine therapy has been found to be effective in improving endothelium-mediated vasodilation in individuals with elevated ADMA levels (28). Apart from its role in mediating endothelial dysfunction, ADMA also has the potential to induce oxidative stress by promoting the uncoupling of NOS (29). Elevated ADMA levels inhibit NOS activity and promote NOS uncoupling, resulting in the generation of ROS and peroxynitrite ions (ONOO-) that mediate intracellular oxidative stress and ultimately lead to cell damage (30).
Moreover, recent research suggests that ADMA may have NOS-independent functions in microangiopathy. Increased levels of ADMA promote inflammation and fibrosis in endothelial cells, which could potentially contribute to the onset and advancement of microangiopathy. Research has demonstrated that ADMA activates the nuclear factor kappa B (NF-κB), p38 mitogen-activated protein kinase (p38 MAPK), and extracellular signal-regulated kinase (ERK) pathways in endothelial cells, leading to the secretion of tumor necrosis factor-alpha (TNF-α) and soluble intercellular adhesion molecule-1 (sICAM-1) (31). These molecules mediate the inflammatory response of endothelial cells. ADMA can also induce tissue fibrosis by promoting Epithelial-to-Mesenchymal Transition (EMT) or Endothelial-to-Mesenchymal Transition (EndMT) (12). These effects were found to be unrelated to ADMA’s inhibitory effect on NOS.
2.2 Metabolism of ADMA
The metabolism of ADMA includes three pathways. Firstly, ADMA is mainly hydrolyzed by dimethylarginine dimethylaminohydrolase (DDAH), which specifically metabolizes ADMA and NMMA and does not hydrolyze SDMA (12, 14, 32). Intracellular ADMA is mainly hydrolyzed by DDAH to citrulline and dimethylamine (12, 14). Leiper et al. originally found in 1999 that two isoforms of DDAH exist in mammals, DDAH1 and DDAH2 (33). DDAH1 is predominantly expressed in tissues that express neuronal nitric oxide synthase (nNOS), such as the brain tissue, whereas DDAH2 is mainly expressed in tissues that express endothelial NOS (eNOS) and inducible NOS (iNOS), such as vascular endothelium and immune tissue (34).
Secondly, a minor portion of ADMA is decomposed to α-keto-δ-(NG, NG-dimethylguanidino) valeric acid (DMGV) by alanine-glyoxylate aminotransferase 2 (AGXT2) (12, 35). Despite the limited role of AGXT2 in clearing ADMA, there are still studies showing reduced NO synthesis and increased blood pressure in AGXT2 knockout mice (36).
Thirdly, ADMA exits cells into blood circulation through the cationic amino acid transporter family (CAT) and is excreted primarily through the kidneys or eliminated in the liver (12, 35, 37). Meanwhile, circulating ADMA also could enter cells via CAT to exert its biological effects (12, 35). Under conditions of inflammation, oxidative stress, and hyperglycemia, the activity of DDAH is inhibited (35), resulting in a reduction in the degradation of ADMA. ADMA deposition in the cells eventually leads to cellular dysfunction (Figure 1).
3 ADMA and diabetic microangiopathy
ADMA serves as a biomarker for endothelial cell dysfunction and is involved in a diverse range of pathological processes, including inflammation, angiogenesis, tissue fibrosis, and oxidative stress (12, 20, 38) (Figure 2). Notably, ADMA has been established as a potent and independent prognostic marker of several cardiovascular diseases and chronic kidney disease (27, 39). Meanwhile, ADMA is also closely associated with diabetic microvascular complications. ADMA has been shown to be a more specific predictor of diabetic microvascular complications compared to other markers such as glycated hemoglobin and N-ε-(carboxymethyl)lysine (CML) (40). Higher plasma ADMA concentrations are strongly associated with the development of diabetic microvascular complication (41). Additionally, the duration of diabetes is positively correlated with plasma ADMA concentrations, and hence the longer the duration of diabetes, the higher the risk of microvascular complications (42, 43). Overall, these results indicated that ADMA is an important biomarker for warning of retinal microvascular injury in diabetes mellitus.
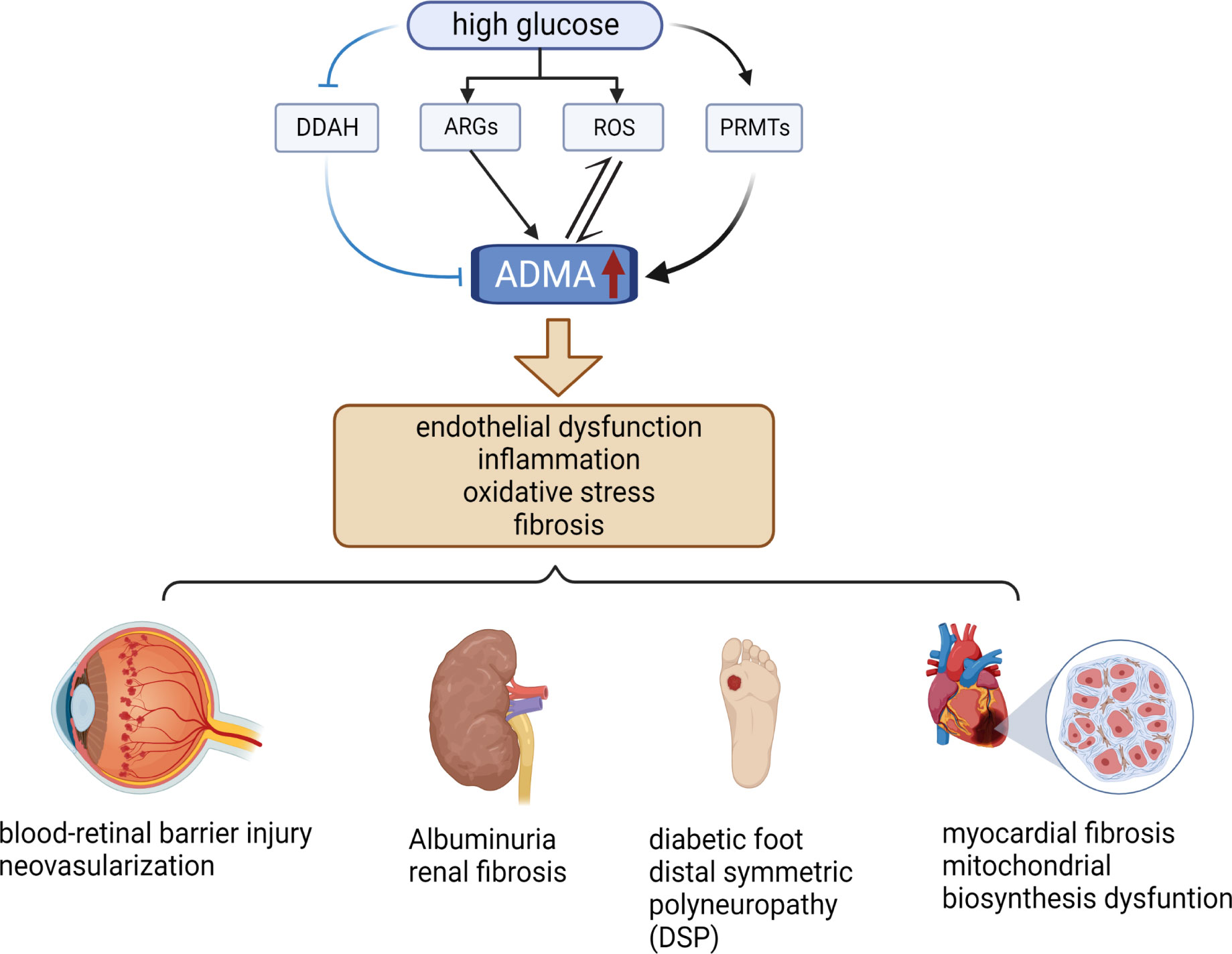
Figure 2 Relationship between ADMA and diabetic microvascular complications. High levels of glucose increase the level of ARGs, ROS, and PRMTs while decreasing the level of DDAH, resulting in elevated levels of ADMA. Elevated levels of ADMA can stimulate even more ROS production and further increase ADMA levels. Elevated ADMA induces endothelial dysfunction, inflammation, oxidative stress, and fibrosis. These pathological changes cause several diabetic microvascular complications, such as DR, DN, DF, DSP, and DCM. (The figure was created with BioRender.com).
3.1 ADMA and diabetic retinopathy
As people’s living standards improve and their dietary habits change, the incidence of diabetes and its associated complications has increased significantly. Among these complications, diabetic retinopathy (DR) is the most prevalent microvascular complication and a leading cause of visual impairment in the working-age population (44, 45). DR can be classified into non-proliferative diabetic retinopathy (NPDR) and proliferative diabetic retinopathy (PDR) based on the severity of retinal lesions. NPDR typically occurs in the early stages of the disease and is primarily characterized by augmented vascular permeability, which leads to microaneurysms, hemorrhage, hard exudates, and cotton wool-like spots (46, 47). PDR typically manifests in the late stages of DR and is characterized by fibrovascular proliferation, resulting in neovascularization, vitreous hemorrhage, and traction retinal detachmentt (46, 47).
ADMA, as a risk factor for microvascular injury, has been linked to the development and progression of DR. The association between ADMA and DR was initially established in 2007 by Malecki et al., who reported that increased circulating levels of ADMA were linked to DR and that ADMA was an independent predictor of the disease (48). This finding was later supported by Abhary, S. et al., who also detected significantly elevated serum ADMA levels in patients with severe DR (49). Additionally, Motohiko et al. reported significantly higher levels of ADMA in the serum and aqueous humor of diabetes patients with DR compared to those without diabetes mellitus (50). ADMA is synthesized by PRMT1, and PRMT1 gene polymorphisms have been shown to be associated with an increased incidence of proliferative DR (PDR), further highlighting the critical role of ADMA in PDR (51). Elevated levels of ADMA have been found not only in severe DR but also in prediabetic and diabetic stages, indicating that ADMA may play an essential role in both the development and progression of DR (52). Given the significant correlation between plasma ADMA levels and the development of DR, researchers have investigated the relationship between plasma ADMA levels and retinal imaging. Dag et al. reported that plasma ADMA levels were elevated and choroidal thickness was reduced in patients with PDR compared to the NPDR group and controls, indicating a potential link between ADMA and retinal imaging (53). However, Hernández et al. did not find any correlation between ADMA plasma levels and retinal neurological dysfunction and structural alterations (54). Therefore, further studies are needed to fully elucidate the relationship between ADMA and diabetic retinopathy imaging, as well as to explore its potential correlation with retinal neurological dysfunction and structural changes.
Metabolomics has gained widespread attention from researchers in recent years due to its ability to explore disease pathogenesis and potential therapeutic targets through the qualitative and quantitative analysis of small molecule metabolites in an organism. Researchers have conducted metabolomics studies on DR patients using various biological samples such as serum, plasma, vitreous humor, atrial fluid, and cerebrospinal fluid (55). Jun Ho Yun et al. utilized a targeted metabolomics approach to analyze serum samples from non-DR, NPDR, and PDR patients and identified several differential metabolites (56). They found that total methylarginine (DMA) concentrations were significantly higher in DR patients than in non-DR patients (56). Furthermore, they observed that PDR patients had higher total DMA concentrations compared to NPDR patients (56). The study revealed that the severity of DR was positively correlated with DMA levels, highlighting the possible role of DMA in DR progression. Moreover, in a study by Huiyi Jin et al., the metabolism of aqueous humor from patients with DR was analyzed using a 1H-NMR-based metabolomics approach (57). The researchers found that DMA levels in aqueous humor were significantly higher in patients with DR than in diabetic patients without DR, providing further evidence that DMA may serve as a potential biomarker for DR (57). Total DMA included ADMA and SDMA (56). Compared with SDMA, ADMA plays a more important role in inhibiting the activity of NOS and inducing endothelial cell injury (58). Additionally, researches have shown that the arginine and proline metabolic pathways are also associated with DR patients (25, 55, 59, 60). Since ADMA is generated by the methylation of arginine residues, elevated levels of arginine metabolic pathways may affect the concentrations of ADMA. This increase in ADMA levels can inhibit the activity of NOS, leading to a reduction in NO production, and promote NOS uncoupling, which can produce superoxide anions and lead to oxidative stress injury (55, 61). Therefore, the dysregulation of arginine and proline metabolic pathways may play a role in the pathogenesis of DR by affecting ADMA levels and NO production. Further research is needed to fully understand the relationship between these metabolic pathways and DR, and to determine if they can be targeted for therapeutic intervention in DR patients.
Recent studies have explored the mechanisms of microvascular injury in DR associated with ADMA. ADMA affects NO synthesis, resulting in hemodynamic disorders and ROS production, which causes oxidative stress in endothelial cells (62). Oxidative stress in endothelial cells is a critical contributor to the development of retinal microvascular injury in patients with diabetic retinopathy (DR). Specifically, in a high glucose environment, DDAH expression is down-regulated, while PRMT expression is up-regulated, leading to an increase in the synthesis of asymmetric dimethylarginine (ADMA) (63–65) (Figure 2). Elevated levels of ADMA can further exacerbate oxidative stress-mediated retinal endothelial cell damage by increasing the production of ROS (64). (Figure 2) Additionally, Huang et al. demonstrated that ADMA can damage the blood-retinal barrier by affecting the expression of blood-retinal barrier-specific component connexin 43 (Cx43) from diabetic rats induced by streptozotocin (STZ) (66). The disruption of the blood-retinal barrier is responsible for the formation of microaneurysms, hemorrhage, and hard exudates, which are characteristic of NPDR. Moreover, Du et al. reported that ADMA can induce neovascularization by promoting the proliferation, migration, adhesion, and tube formation of choroid-retinal endothelial cells (RF/6A) (67). Neovascularization is a hallmark of PDR and a critical cause of vitreous hemorrhage, which can lead to severe visual impairment. The studies mentioned above indicate that reducing the level of ADMA may provide protective benefits to the blood-retinal barrier in patients with diabetes and can potentially decrease the formation of neovascularization, thereby delaying the progression of DR. Many studies have shown that DDAH1 is more closely related to the metabolism of ADMA (32, 68, 69). However, the expression of DDAH2 in endothelial cells is higher than that of DDAH1, and DDAH2 co-localizes with endothelial nitric oxide synthase (eNOS) (70, 71). In the oxygen-induced retinopathy mouse model (OIR), both DDAH1 and DDAH2 are expressed in retinal tissues, but DDAH2 expression was significantly higher in retinal than in brain, indicating that DDAH2 has higher specificity in retinal (72). Therefore, increasing the expression of DDAH2 or enhancing its activity may alleviate ADMA-mediated retinal microvascular endothelial cell injury.
3.2 ADMA and diabetic nephropathy
Diabetic nephropathy (DN) is a major complication of diabetes and a leading cause of severe renal function impairment in diabetic patients. It is characterized by the presence of albuminuria and progressive glomerulosclerosis, which can eventually lead to renal failure (73, 74). DN is also a primary cause of the end-stage renal disease (ESRD) (73). The pathogenesis of DN is complex and involves several factors, including hemodynamic changes, oxidative stress, renal fibrosis, and inflammation (75). One critical player in the pathogenesis of DN is ADMA, an inhibitor of NOS. ADMA plays a crucial role not only in regulating hemodynamics, but also in inducing oxidative stress injury, promoting fibrosis, and mediating inflammation (9, 12).
Several studies have demonstrated the close correlation between ADMA and DN. A study by Tanhäuserová et al. found that ADMA is negatively correlated with renal function, such as glomerular filtration rate (GFR), and that the ADMA/GFR ratio is an essential biomarker for predicting the progression of DN (76). In patients with advanced chronic kidney disease (CKD), the higher the ADMA level, the greater the reduction in GFR and the more severe the kidney injury (77). Furthermore, for patients who progressed to ERSD and required hemodialysis treatment, serum levels of ADMA were significantly higher in DN patients than in non-DN patients both before and after dialysis (78). It suggested that ADMA has higher specificity in DN compared to other CKD. Among haemodialysis patients, the level of plasma ADMA is a powerful and independent predictor for both cardiovascular events and overall mortality (79). This highlights the potential of ADMA as a useful biomarker for both the diagnosis and monitoring of DN.
Albuminuria, as a prominent clinical feature of DN, is closely related to ADMA. A prospective study conducted in Brazil revealed that patients with elevated plasma ADMA levels had a higher risk of developing albuminuria in both the hypertension group and the hypertension-diabetes group (80). In addition, the odds ratio of ADMA in the logistic regression analysis was higher in the hypertension-diabetes group than in the hypertension group (80), suggesting that the presence of diabetes exacerbates the risk of ADMA-induced albuminuria. Furthermore, Yilmaz et al. observed a positive correlation between serum level of ADMA and proteinuria and a negatively correlated with endothelial cell function (81). ADMA may promote the progression of albuminuria by damaging renal microvascular endothelial cells. Meanwhile, Kaida et al. have found that proteinuria can induce oxidative stress injury, which further up-regulated the expression of PRMT1 and increase the synthesis of ADMA in renal tubular cells (82). The interaction between ADMA and proteinuria can worsen renal function. Reducing the synthesis or promoting the breakdown of ADMA may slow down the progression of proteinuria. Therefore, ADMA may be a therapeutic target for alleviating albuminuria in patients with DN, and its specific mechanism remains to be further verified.
DDAH is a key enzyme in ADMA degradation. Wetzel et al. have found that overexpression of DDAH1 in mice can reverse ADMA-mediated albuminuria, oxidative stress injury, and inflammatory response, thus improving DN (83). Meanwhile, reduced expression of DDAH, significant accumulation of ADMA in plasma and renal tissue, tubular necrosis, and significantly impaired renal function was found in ischemia-reperfusion-injured mice (84). However, in folate nephropathy and unilateral ureteric obstruction models, renal tissue damage and renal fibrosis were less severe in DDAH1 knockout mice than in controls (85). Whether DDAH inhibition has a protective effect on renal function in DN patients remains to be further verified.
Interstitial fibrosis and glomerulosclerosis are major causes of chronic renal failure in DN patients. ADMA, as a mediator of renal tissue fibrosis, plays an essential role in this process. ADMA has been found to promote the deposition of collagen fibers and damage capillaries of the glomerulus and tubule, thereby aggravating renal fibrosis (12, 86). The underlying mechanism involves ADMA up-regulating hypoxia-inducing factor (HIF) and its downstream target molecule, endothelin-1(ET-1), as well as promoting the expression of Transforming Growth Factor β (TGF-β) (87, 88). This leads to the transdifferentiation of fibroblasts, epithelial cells, or endothelial cells into myofibroblasts, ultimately leading to increased synthesis of extracellular matrix and progression of chronic kidney disease (89–91). Furthermore, Isaivani et al. demonstrated that ADMA can activate the fibrotic signaling pathway through the NOX4/ROS/ERK pathway, leading to increased synthesis of extracellular matrix and accelerating kidney cell fibrosis (92). In rats induced by monocrotaline, DDAH1 knockdown significantly exacerbated oxidative stress, pulmonary vascular remodeling, and lung fibrosis (93). Through the same mechanism, elevated ADMA may mediate oxidative stress and fibrosis of renal tubular epithelial cells and glomerular microvascular endothelial cells, eventually leading to renal failure. Therefore, the reduction of ADMA levels may be a therapeutic strategy for delaying the progression of CKD induced by renal fibrosis.
3.3 ADMA and diabetic neuropathy
Diabetic neuropathy is a common complication of diabetes, seriously affecting patients’ quality of life. The most common type of diabetic neuropathy is distal symmetric polyneuropathy (DSP), which manifests as sensory abnormalities in a stocking-glove distribution in the limbs, including numbness, pain, and weakness (94). Severe DSP can progress to diabetic foot, which may ultimately lead to limb amputation (95). High blood sugar plays a critical role in the development of diabetic neuropathy by inducing oxidative stress and inflammation. However, maintaining glycemic control alone is insufficient to halt the progression of neuropathy (94), suggesting the involvement of other crucial molecules.
As mentioned previously, ADMA is a critical mediator of oxidative stress damage and inflammation, and its role in exacerbating diabetic neuropathy remains controversial. Several studies have found that patients with type 2 diabetes who suffer from peripheral neuropathy exhibit endothelial dysfunction (94–96). ADMA can induce endothelial dysfunction by affecting NO synthesis and promoting oxidative stress. However, the use of antioxidants, such as alpha-lipoic acid (ALA), can improve ADMA-induced endothelial damage and promote the repair of peripheral sensory nerve function (97). Stojanovic et al. found that compared with the control group, plasma ADMA levels were significantly increased in patients with type 2 diabetes mellitus complicated with DSP (98). ADMA can be used as a marker to detect the progression of diabetic neuropathy. Diabetic foot (DF) is an important manifestation of diabetic peripheral neuropathy in patients with diabetes. Hala et al. found that the level of circulating ADMA was significantly higher in DM patients who had DF compared to those without DF, and NO levels were significantly lower in DM patients with DF (99). ADMA may affect the microcirculation of the limbs by affecting the synthesis of NO, resulting in aseptic ulcers in the feet.
However, Kyrillos et al. suggested that the plasma levels of ADMA were not significantly increased in patients with DF compared with the control group (100). Moreover, Halit et al. found no significant difference in ADMA levels between diabetic patients with neuropathy and without neuropathy (101). The differences in these results may be attributed to inadequate sample size or individual differences in the baseline disease of patients. Further clinical controlled studies with larger samples are necessary to confirm the relationship between ADMA and diabetic neuropathy.
3.4 ADMA and diabetic cardiomyopathy
Diabetic cardiomyopathy (DCM) refers to an abnormality in the structure and function of the myocardium that occurs without other traditional cardiovascular risks, such as coronary artery disease, valvular heart disease, hypertension, or hyperlipidemia (102). The early manifestation of DCM includes impaired diastolic function, which may progress to systolic dysfunction and eventually lead to heart failure (103). Although it is commonly believed that DCM is closely associated with diabetic macroangiopathy, studies as early as the 1980s have found evidence of capillary basement membrane thickening and microangioma formation within the myocardium of diabetic patients (104–106). These findings suggest that myocardial microangiopathy also plays a significant role in the development and progression of diabetic cardiomyopathy.
The pathogenesis of DCM involves various factors, including hyperglycemia, insulin resistance, hyperlipidemia, oxidative stress, inflammatory responses, activation of the renin-angiotensin-aldosterone system, and abnormal function of the sympathetic nervous system (107). Studies have shown that abnormal glucose metabolism can lead to oxidative stress, reducing the availability of nitric oxide (NO) and synthesis of vascular endothelial growth factor (VEGF), causing dysfunction of myocardial microvascular endothelial cells, and promoting vasoconstriction, ultimately leading to reduced blood flow to myocardial cells (108, 109). In addition, hyperglycemia increases the plasma level of ET-1 and promotes the endothelial-to-mesenchymal transformation (EndMT) of myocardial microvascular endothelial cells (110). This process can further trigger myocardial fibrosis, which plays a significant role in ventricular remodeling and the development of myocardial systolic and diastolic dysfunction.
In diabetic rat models with DDAH2 overexpression, a decrease in left ventricular end-diastolic pressure and an increase in systolic pressure were observed, along with an improvement in myocardial function (111). In addition, DDAH2 administration to cardiomyocytes cultured in a high-glucose environment resulted in a reduction in ADMA synthesis, an increase in NOS levels, and a decrease in type I collagen fiber, matrix metalloproteinase 2 (MMP2), and tissue inhibitor of metalloproteinase 2 (TIMP2) levels (111). MMP2 and TIMP2 are crucial factors in the synthesis of myocardial extracellular matrix (ECM) and are implicated in the progression of myocardial fibrosis (112). Reducing ADMA levels may alleviate myocardial microvascular injury, inhibit myocardial fibrosis, improve myocardial remodeling, and enhance myocardial function.
In a rat model of diabetic cardiomyopathy, it was observed that ADMA levels were elevated in cardiomyocytes, which interfered with mitochondrial biosynthesis and affected myocardium function (113, 114). The underlying mechanism could be that ADMA up-regulated the expression of coupled protein 2 (UCP2) and inhibited the activity of the peroxisome proliferator-activated receptor-γ-coactivator-1α (PGC1α) promoter, leading to the down-regulation of PGC-1α expression and, thus affecting ATP synthesis (113, 114). However, resveratrol treatment helped to mitigate ADMA accumulation and reverse Adma-mediated PGC-1α expression reduction and acetylation, which ultimately improve myocardial mitochondrial function (115). The findings indicate that managing ADMA levels could be an effective strategy in the treatment of diabetic cardiomyopathy, and resveratrol might have the potential as a therapeutic agent.
4 Discussion
The above findings suggest that ADMA is an important marker of microvascular injury in diabetic patients and is involved in the development and progression of diabetic microvascular complications through multiple pathways. Endothelial dysfunction, inflammation, oxidative stress damage, and fibrosis in endothelial cells are important risk factors for diabetic microvascular complications. Many studies in the past have focused on the effects of ADMA on endothelial cell function through the inhibition of NOS activity and NO production. In recent years, more and more studies have confirmed the important role of ADMA in inducing oxidative stress injury and fibrosis, which brings a new direction for the treatment of diabetic microvascular complications. More studies are still needed in the future to further analyze the mechanistic pathways involved in ADMA, to find key therapeutic targets, and to provide new ideas for the prevention and treatment of diabetic microvascular complications.
ADMA is a by-product of protein arginine methylation and can be significantly increased in various pathological conditions like diabetes, hypertension, coronary heart disease, and chronic kidney disease. This elevation may be caused by an imbalance between ADMA production and degradation. Although ADMA can be partially eliminated through urine excretion, the main pathway for ADMA metabolism is intracellular DDAH degradation, which highlights the importance of PRMTs and DDAH in maintaining normal ADMA levels. Further exploration is required to determine the mechanism by which the expression of these two enzymes can be affected in pathological conditions. Additionally, it has been suggested that the occurrence of PDR could be linked to polymorphisms in the PRMT1 gene (51). However, it is currently unknown whether similar associations exist in other patients with diabetic microvascular complications, as well as whether there is concurrent DDAH gene polymorphism. Further research is required to address these questions.
Elevated ADMA levels have been linked to adverse effects, prompting researchers to explore methods for reducing them. One such approach involves L-arginine, which has been shown to reverse the inhibitory effect of ADMA on NOS (22). However, while L-arginine has been shown to improve endothelial function in individuals with high ADMA levels (28), some studies have produced unexpected results. These studies have found that L-arginine supplementation may not have any positive effects on blood vessels and can even lead to adverse outcomes (23). Moreover, higher L-arginine levels correlate with increased ADMA levels (23). This phenomenon may be attributed to the competitive inhibition of DDAH enzyme activity by higher L-arginine levels, which subsequently reduces ADMA degradation (23). Therefore, the role of arginine in improving endothelial dysfunction remains controversial. Further research is needed to fully understand the potential benefits and adverse effects of L-arginine supplementation for endothelial function and vascular health. Additionally, while studies indicate that various medications like antihypertensive, lipid-lowering, hypoglycemic, and antioxidant drugs can reduce ADMA levels, most of these studies lack placebo controls (116). Currently, no specific drug targets ADMA levels. Therefore, there is a need for further investigation into ADMA-lowering medications, and additional clinical studies are required to determine whether reducing ADMA levels can lead to a significant slowdown of diabetic microvascular complications progression and better prognoses.
Author contributions
WJ contributed to conception and design of the review. XG wrote the first draft of the manuscript and draw the figures. WJ and YX contributed to manuscript revision, read, and approved the submitted version.
Funding
The review was funded by Hubei Provincial Science and Technology Planning Project (No.2019CFB489) and supported by The Interdisciplinary Innovative Talents Foundation from Renmin Hospital of Wuhan University(JCRCGW-2022-006).
Acknowledgments
The authors thank Figdraw, developed by home-for-researchers, for helping to create the figure.
Conflict of interest
The authors declare that the research was conducted in the absence of any commercial or financial relationships that could be construed as a potential conflict of interest.
Publisher’s note
All claims expressed in this article are solely those of the authors and do not necessarily represent those of their affiliated organizations, or those of the publisher, the editors and the reviewers. Any product that may be evaluated in this article, or claim that may be made by its manufacturer, is not guaranteed or endorsed by the publisher.
References
1. Lin YK, Gao B, Liu L, Ang L, Mizokami-Stout K, Pop-Busui R, et al. The prevalence of diabetic microvascular complications in China and the USA. Curr Diabetes Rep (2021) 21(6):16. doi: 10.1007/s11892-021-01387-3
2. Ogurtsova K, da Rocha Fernandes JD, Huang Y, Linnenkamp U, Guariguata L, Cho NH, et al. Idf diabetes atlas: global estimates for the prevalence of diabetes for 2015 and 2040. Diabetes Res Clin Pract (2017) 128:40–50. doi: 10.1016/j.diabres.2017.03.024
3. Faselis C, Katsimardou A, Imprialos K, Deligkaris P, Kallistratos M, Dimitriadis K. Microvascular complications of type 2 diabetes mellitus. Curr Vasc Pharmacol (2020) 18(2):117–24. doi: 10.2174/1570161117666190502103733
4. Cole JB, Florez JC. Genetics of diabetes mellitus and diabetes complications. Nat Rev Nephrol (2020) 16(7):377–90. doi: 10.1038/s41581-020-0278-5
5. Saputro SA, Pattanaprateep O, Pattanateepapon A, Karmacharya S, Thakkinstian A. Prognostic models of diabetic microvascular complications: a systematic review and meta-analysis. Syst Rev (2021) 10(1):288. doi: 10.1186/s13643-021-01841-z
6. Park S, Kang HJ, Jeon JH, Kim MJ, Lee IK. Recent advances in the pathogenesis of microvascular complications in diabetes. Arch Pharm Res (2019) 42(3):252–62. doi: 10.1007/s12272-019-01130-3
7. Du MR, Ju GX, Li NS, Jiang JL. Role of asymmetrical dimethylarginine in diabetic microvascular complications. J Cardiovasc Pharmacol (2016) 68(4):322–6. doi: 10.1097/fjc.0000000000000414
8. Ando R, Ueda S, Yamagishi S, Miyazaki H, Kaida Y, Kaifu K, et al. Involvement of advanced glycation end product-induced asymmetric dimethylarginine generation in endothelial dysfunction. Diabetes Vasc Dis Res (2013) 10(5):436–41. doi: 10.1177/1479164113486662
9. Konukoglu D, Firtina S, Serin O. The relationship between plasma asymmetrical dimethyl-L-Arginine and inflammation and adhesion molecule levels in subjects with normal, impaired, and diabetic glucose tolerance. Metabolism (2008) 57(1):110–5. doi: 10.1016/j.metabol.2007.08.013
10. Chen MF, Xie XM, Yang TL, Wang YJ, Zhang XH, Luo BL, et al. Role of asymmetric dimethylarginine in inflammatory reactions by angiotensin ii. J Vasc Res (2007) 44(5):391–402. doi: 10.1159/000103284
11. Zhang GG, Bai YP, Chen MF, Shi RZ, Jiang DJ, Fu QM, et al. Asymmetric dimethylarginine induces tnf-alpha production Via Ros/Nf-kappab dependent pathway in human monocytic cells and the inhibitory effect of reinioside c. Vascul Pharmacol (2008) 48(2-3):115–21. doi: 10.1016/j.vph.2008.01.004
12. Zhao WC, Li G, Huang CY, Jiang JL. Asymmetric dimethylarginine: an crucial regulator in tissue fibrosis. Eur J Pharmacol (2019) 854:54–61. doi: 10.1016/j.ejphar.2019.03.055
13. van der Zwan LP, Scheffer PG, Dekker JM, Stehouwer CD, Heine RJ, Teerlink T. Systemic inflammation is linked to low arginine and high adma plasma levels resulting in an unfavourable nos substrate-to-Inhibitor ratio: the hoorn study. Clin Sci (Lond) (2011) 121(2):71–8. doi: 10.1042/CS20100595
14. Leiper J, Vallance P. Biological significance of endogenous methylarginines that inhibit nitric oxide synthases. Cardiovasc Res (1999) 43(3):542–8. doi: 10.1016/s0008-6363(99)00162-5
15. Jarzebska N, Mangoni AA, Martens-Lobenhoffer J, Bode-Boger SM, Rodionov RN. The second life of methylarginines as cardiovascular targets. Int J Mol Sci (2019) 20(18):1–16 doi: 10.3390/ijms20184592
16. Fulton MD, Brown T, Zheng YG. The biological axis of protein arginine methylation and asymmetric dimethylarginine. Int J Mol Sci (2019) 20(13):1–18. doi: 10.3390/ijms20133322
17. Bulau P, Zakrzewicz D, Kitowska K, Leiper J, Gunther A, Grimminger F, et al. Analysis of methylarginine metabolism in the cardiovascular system identifies the lung as a major source of adma. Am J Physiol Lung Cell Mol Physiol (2007) 292(1):L18–24. doi: 10.1152/ajplung.00076.2006
18. Bode-Boger SM, Scalera F, Kielstein JT, Martens-Lobenhoffer J, Breithardt G, Fobker M, et al. Symmetrical dimethylarginine: a new combined parameter for renal function and extent of coronary artery disease. J Am Soc Nephrol (2006) 17(4):1128–34. doi: 10.1681/ASN.2005101119
19. Ramuschkat M, Appelbaum S, Atzler D, Zeller T, Bauer C, Ojeda FM, et al. Adma, subclinical changes and atrial fibrillation in the general population. Int J Cardiol (2016) 203:640–6. doi: 10.1016/j.ijcard.2015.05.102
20. Dowsett L, Higgins E, Alanazi S, Alshuwayer NA, Leiper FC, Leiper J. Adma: a key player in the relationship between vascular dysfunction and inflammation in atherosclerosis. J Clin Med (2020) 9(9):1–17. doi: 10.3390/jcm9093026
21. Horowitz JD, Heresztyn T. An overview of plasma concentrations of asymmetric dimethylarginine (Adma) in health and disease and in clinical studies: methodological considerations. J Chromatogr B Analyt Technol BioMed Life Sci (2007) 851(1-2):42–50. doi: 10.1016/j.jchromb.2006.09.023
22. Vallance P, Leone A, Calver A, Collier J, Moncada S. Accumulation of an endogenous inhibitor of nitric oxide synthesis in chronic renal failure. Lancet (1992) 339(8793):572–5. doi: 10.1016/0140-6736(92)90865-z
23. Wilcken DE, Sim AS, Wang J, Wang XL. Asymmetric dimethylarginine (Adma) in vascular, renal and hepatic disease and the regulatory role of l-arginine on its metabolism. Mol Genet Metab (2007) 91(4):309–17. doi: 10.1016/j.ymgme.2007.04.017
24. Cardounel AJ, Cui H, Samouilov A, Johnson W, Kearns P, Tsai AL, et al. Evidence for the pathophysiological role of endogenous methylarginines in regulation of endothelial no production and vascular function. J Biol Chem (2007) 282(2):879–87. doi: 10.1074/jbc.M603606200
25. Peters KS, Rivera E, Warden C, Harlow PA, Mitchell SL, Calcutt MW, et al. Plasma arginine and citrulline are elevated in diabetic retinopathy. Am J Ophthalmol (2022) 235:154–62. doi: 10.1016/j.ajo.2021.09.021
26. Brinkmann SJ, Worner EA, Buijs N, Richir M, Cynober L, van Leeuwen PA, et al. The Arginine/Adma ratio is related to the prevention of atherosclerotic plaques in hypercholesterolemic rabbits when giving a combined therapy with atorvastatine and arginine. Int J Mol Sci (2015) 16(6):12230–42. doi: 10.3390/ijms160612230
27. Sibal L, Agarwal SC, Home PD, Boger RH. The role of asymmetric dimethylarginine (Adma) in endothelial dysfunction and cardiovascular disease. Curr Cardiol Rev (2010) 6(2):82–90. doi: 10.2174/157340310791162659
28. Boger GI, Rudolph TK, Maas R, Schwedhelm E, Dumbadze E, Bierend A, et al. Asymmetric dimethylarginine determines the improvement of endothelium-dependent vasodilation by simvastatin: effect of combination with oral l-arginine. J Am Coll Cardiol (2007) 49(23):2274–82. doi: 10.1016/j.jacc.2007.02.051
29. Sydow K, Munzel T. Adma and oxidative stress. Atheroscler Suppl (2003) 4(4):41–51. doi: 10.1016/s1567-5688(03)00033-3
30. Li N, Worthmann H, Deb M, Chen S, Weissenborn K. Nitric oxide (No) and asymmetric dimethylarginine (Adma): their pathophysiological role and involvement in intracerebral hemorrhage. Neurol Res (2011) 33(5):541–8. doi: 10.1179/016164111X13007856084403
31. Jiang JL, Wang S, Li NS, Zhang XH, Deng HW, Li YJ. The inhibitory effect of simvastatin on the adma-induced inflammatory reaction is mediated by mapk pathways in endothelial cells. Biochem Cell Biol (2007) 85(1):66–77. doi: 10.1139/o06-146
32. Hu X, Atzler D, Xu X, Zhang P, Guo H, Lu Z, et al. Dimethylarginine dimethylaminohydrolase-1 is the critical enzyme for degrading the cardiovascular risk factor asymmetrical dimethylarginine. Arteriosclerosis Thrombosis Vasc Biol (2011) 31(7):1540–6. doi: 10.1161/atvbaha.110.222638
33. Leiper JM, Santa Maria J, Chubb A, MacAllister RJ, Charles IG, Whitley GS, et al. Identification of two human dimethylarginine dimethylaminohydrolases with distinct tissue distributions and homology with microbial arginine deiminases. Biochem J (1999) 343 Pt 1(Pt 1):209–14. doi: 10.1042/bj3430209
34. Tran CTL, Fox MF, Vallance P, Leiper JM. Chromosomal localization, gene structure, and expression pattern of Ddah1: comparison with Ddah2 and implications for evolutionary origins. Genomics (2000) 68(1):101–5. doi: 10.1006/geno.2000.6262
35. Teerlink T. Adma metabolism and clearance. Vasc Med (2005) 10(Suppl 1):S73–81. doi: 10.1191/1358863x05vm597oa
36. Caplin B, Wang Z, Slaviero A, Tomlinson J, Dowsett L, Delahaye M, et al. Alanine-glyoxylate aminotransferase-2 metabolizes endogenous methylarginines, regulates no, and controls blood pressure. Arterioscler Thromb Vasc Biol (2012) 32(12):2892–900. doi: 10.1161/atvbaha.112.254078
37. Nijveldt RJ, Teerlink T, Siroen MP, van der Hoven B, Prins HA, Wiezer MJ, et al. Elevation of asymmetric dimethylarginine (Adma) in patients developing hepatic failure after major hepatectomy. JPEN J Parenter Enteral Nutr (2004) 28(6):382–7. doi: 10.1177/0148607104028006382
38. Böger RH, Bode-Böger SM, Szuba A, Tsao PS, Chan JR, Tangphao O, et al. Asymmetric dimethylarginine (Adma): a novel risk factor for endothelial dysfunction: its role in hypercholesterolemia. Circulation (1998) 98(18):1842–7. doi: 10.1161/01.cir.98.18.1842
39. Liu X, Xu X, Shang R, Chen Y. Asymmetric dimethylarginine (Adma) as an important risk factor for the increased cardiovascular diseases and heart failure in chronic kidney disease. Nitric Oxide (2018) 78:113–20. doi: 10.1016/j.niox.2018.06.004
40. Jing Z, Kuang L, Wang Y, He J, Sun Z, Liu N, et al. Adma: a specific biomarker for pathologic progress in diabetic microvascular complications? biomark Med (2016) 10(4):385–95. doi: 10.2217/bmm-2015-0007
41. Liu J, Li C, Chen W, He K, Ma H, Ma B, et al. Relationship between serum asymmetric dimethylarginine level and microvascular complications in diabetes mellitus: a meta-analysis. BioMed Res Int (2019) 2019:2941861. doi: 10.1155/2019/2941861
42. Zaciragic A, Dervisevic A, Avdagic N, Babic N, Valjevac A, Lepara O, et al. The impact of type 2 diabetes duration on serum asymmetric dimethylarginine and c-reactive protein concentration in Bosnian patients. Endocr Regul (2022) 56(4):271–8. doi: 10.2478/enr-2022-0029
43. Bui HDT, Jing X, Lu R, Chen J, Ngo V, Cui Z, et al. Prevalence of and factors related to microvascular complications in patients with type 2 diabetes mellitus in tianjin, China: a cross-sectional study. Ann Transl Med (2019) 7(14):325. doi: 10.21037/atm.2019.06.08
44. Ansari P, Tabasumma N, Snigdha NN, Siam NH, Panduru RVNRS, Azam S, et al. Diabetic retinopathy: an overview on mechanisms, pathophysiology and pharmacotherapy. Diabetology (2022) 3(1):159–75. doi: 10.3390/diabetology3010011
45. Porta M, Bandello F. Diabetic retinopathya clinical update. Diabetologia (2002) 45(12):1617–34. doi: 10.1007/s00125-002-0990-7
46. Fong DS, Aiello L, Gardner TW, King GL, Blankenship G, Cavallerano JD, et al. Retinopathy in diabetes. Diabetes Care (2004) 27(Suppl 1):S84–7. doi: 10.2337/diacare.27.2007.s84
47. Cheung N, Mitchell P, Wong TY. Diabetic retinopathy. Lancet (2010) 376(9735):124–36. doi: 10.1016/S0140-6736(09)62124-3
48. Malecki MT, Undas A, Cyganek K, Mirkiewicz-Sieradzka B, Wolkow P, Osmenda G, et al. Plasma asymmetric dimethylarginine (Adma) is associated with retinopathy in type 2 diabetes. Diabetes Care (2007) 30(11):2899–901. doi: 10.2337/dc07-1138
49. Abhary S, Kasmeridis N, Burdon KP, Kuot A, Whiting MJ, Yew WP, et al. Diabetic retinopathy is associated with elevated serum asymmetric and symmetric dimethylarginines. Diabetes Care (2009) 32(11):2084–6. doi: 10.2337/dc09-0816
50. Sugai M, Ohta A, Ogata Y, Nakanishi M, Ueno S, Kawata T, et al. Asymmetric dimethylarginine (Adma) in the aqueous humor of diabetic patients. Endocr J (2007) 54(2):303–9. doi: 10.1507/endocrj.k06-140
51. Iwasaki H, Shichiri M. Protein arginine n-methyltransferase 1 gene polymorphism is associated with proliferative diabetic retinopathy in a Japanese population. Acta Diabetol (2022) 59(3):319–27. doi: 10.1007/s00592-021-01808-5
52. Wieczor R, Wieczor AM, Kulwas A, Rosc D. Adma (Asymmetric dimethylarginine) and angiogenic potential in patients with type 2 diabetes and prediabetes. Exp Biol Med (Maywood) (2021) 246(2):153–62. doi: 10.1177/1535370220959738
53. Dag U, Caglayan M, Alakus MF, Oncul H. The relationship between reduced choroidal thickness due to high plasma asymmetrical dimethylarginine level and increased severity of diabetic retinopathy. Arq Bras Oftalmol (2023) 86(1):27–32. doi: 10.5935/0004-2749.20230007
54. Hernandez C, Porta M, Bandello F, Grauslund J, Harding SP, Aldington SJ, et al. The usefulness of serum biomarkers in the early stages of diabetic retinopathy: results of the eurocondor clinical trial. J Clin Med (2020) 9(4):1–10. doi: 10.3390/jcm9041233
55. Jian Q, Wu Y, Zhang F. Metabolomics in diabetic retinopathy: from potential biomarkers to molecular basis of oxidative stress. Cells (2022) 11(19):1–19. doi: 10.3390/cells11193005
56. Yun JH, Kim JM, Jeon HJ, Oh T, Choi HJ, Kim BJ. Metabolomics profiles associated with diabetic retinopathy in type 2 diabetes patients. PloS One (2020) 15(10):e0241365. doi: 10.1371/journal.pone.0241365
57. Jin H, Zhu B, Liu X, Jin J, Zou H. Metabolic characterization of diabetic retinopathy: an (1)H-Nmr-Based metabolomic approach using human aqueous humor. J Pharm BioMed Anal (2019) 174:414–21. doi: 10.1016/j.jpba.2019.06.013
58. Oliva-Damaso E, Oliva-Damaso N, Rodriguez-Esparragon F, Payan J, Baamonde-Laborda E, Gonzalez-Cabrera F, et al. Asymmetric (Adma) and symmetric (Sdma) dimethylarginines in chronic kidney disease: a clinical approach. Int J Mol Sci (2019) 20(15):1–15 doi: 10.3390/ijms20153668
59. Sumarriva K, Uppal K, Ma C, Herren DJ, Wang Y, Chocron IM, et al. Arginine and carnitine metabolites are altered in diabetic retinopathy. Invest Ophthalmol Vis Sci (2019) 60(8):3119–26. doi: 10.1167/iovs.19-27321
60. Liew G, Lei Z, Tan G, Joachim N, Ho IV, Wong TY, et al. Metabolomics of diabetic retinopathy. Curr Diabetes Rep (2017) 17(11):102. doi: 10.1007/s11892-017-0939-3
61. Narayanan SP, Rojas M, Suwanpradid J, Toque HA, Caldwell RW, Caldwell RB. Arginase in retinopathy. Prog Retin Eye Res (2013) 36:260–80. doi: 10.1016/j.preteyeres.2013.06.002
62. Simo-Servat O, Simo R, Hernandez C. Circulating biomarkers of diabetic retinopathy: an overview based on physiopathology. J Diabetes Res (2016) 2016:5263798. doi: 10.1155/2016/5263798
63. Jiang DJ, Jiang JL, Tan GS, Du YH, Xu KP, Li YJ. Protective effects of daviditin a against endothelial damage induced by lysophosphatidylcholine. Naunyn Schmiedebergs Arch Pharmacol (2003) 367(6):600–6. doi: 10.1007/s00210-003-0756-x
64. Chen Y, Xu X, Sheng M, Zhang X, Gu Q, Zheng Z. Prmt-1 and ddahs-induced adma upregulation is involved in ros- and ras-mediated diabetic retinopathy. Exp Eye Res (2009) 89(6):1028–34. doi: 10.1016/j.exer.2009.09.004
65. Lin KY, Ito A, Asagami T, Tsao PS, Adimoolam S, Kimoto M, et al. Impaired nitric oxide synthase pathway in diabetes mellitus: role of asymmetric dimethylarginine and dimethylarginine dimethylaminohydrolase. Circulation (2002) 106(8):987–92. doi: 10.1161/01.cir.0000027109.14149.67
66. Huang CY, Zhou T, Li G, Li MY, Xiong XM, Wu MT, et al. Asymmetric dimethylarginine aggravates blood-retinal barrier breakdown of diabetic retinopathy via inhibition of intercellular communication in retinal pericytes. Amino Acids (2019) 51(10-12):1515–26. doi: 10.1007/s00726-019-02788-1
67. Du MR, Yan L, Li NS, Wang YJ, Zhou T, Jiang JL. Asymmetric dimethylarginine contributes to retinal neovascularization of diabetic retinopathy through Ephrinb2 pathway. Vascul Pharmacol (2018) 108:46–56. doi: 10.1016/j.vph.2018.05.004
68. Hulin JA, Gubareva EA, Jarzebska N, Rodionov RN, Mangoni AA, Tommasi S. Inhibition of dimethylarginine dimethylaminohydrolase (Ddah) enzymes as an emerging therapeutic strategy to target angiogenesis and vasculogenic mimicry in cancer. Front Oncol (2019) 9:1455. doi: 10.3389/fonc.2019.01455
69. Zhang P, Xu X, Hu X, Wang H, Fassett J, Huo Y, et al. Ddah1 deficiency attenuates endothelial cell cycle progression and angiogenesis. PloS One (2013) 8(11):e79444. doi: 10.1371/journal.pone.0079444
70. Wang D, Gill PS, Chabrashvili T, Onozato ML, Raggio J, Mendonca M, et al. Isoform-specific regulation by N(G),N(G)-dimethylarginine dimethylaminohydrolase of rat serum asymmetric dimethylarginine and vascular endothelium-derived relaxing Factor/No. Circ Res (2007) 101(6):627–35. doi: 10.1161/CIRCRESAHA.107.158915
71. Palm F, Onozato ML, Luo Z, Wilcox CS. Dimethylarginine dimethylaminohydrolase (Ddah): expression, regulation, and function in the cardiovascular and renal systems. Am J Physiol Heart Circ Physiol (2007) 293(6):H3227–45. doi: 10.1152/ajpheart.00998.2007
72. Lange C, Mowat F, Sayed H, Mehad M, Duluc L, Piper S, et al. Dimethylarginine dimethylaminohydrolase-2 deficiency promotes vascular regeneration and attenuates pathological angiogenesis. Exp Eye Res (2016) 147:148–55. doi: 10.1016/j.exer.2016.05.007
73. Zhang C, Zhuang S. The role of protein arginine methyltransferases in kidney diseases. Clin Sci (Lond) (2020) 134(15):2037–51. doi: 10.1042/CS20200680
74. Gross JL, de Azevedo MJ, Silveiro SP, Canani LH, Caramori ML, Zelmanovitz T. Diabetic nephropathy: diagnosis, prevention, and treatment. Diabetes Care (2005) 28(1):164–76. doi: 10.2337/diacare.28.1.164
75. Wolf G. New insights into the pathophysiology of diabetic nephropathy: from haemodynamics to molecular pathology. Eur J Clin Invest (2004) 34(12):785–96. doi: 10.1111/j.1365-2362.2004.01429.x
76. Tanhauserova V, Tomandl J, Pacal L, Kleparnik M, Maluskova D, Bartakova V, et al. Adma, sdma and l-Arginine/Adma ratio but not ddah genetic polymorphisms are reliable predictors of diabetic nephropathy progression as identified by competing risk analysis. Kidney Blood Press Res (2012) 36(1):200–8. doi: 10.1159/000343409
77. Eiselt J, Rajdl D, Racek J, Vostry M, Rulcova K, Wirth J. Asymmetric dimethylarginine and progression of chronic kidney disease: a one-year follow-up study. Kidney Blood Press Res (2014) 39(1):50–7. doi: 10.1159/000355776
78. Avci E, Cakir E, Cevher SC, Yaman H, Agilli M, Bilgi C. Determination of oxidative stress and cellular inflammation in patients with diabetic nephropathy and non-diabetic nephropathy being administered hemodialysis treatment due to chronic renal failure. Ren Fail (2014) 36(5):767–73. doi: 10.3109/0886022X.2014.890841
79. Zoccali C, Bode-Böger SM, Mallamaci F, Benedetto FA, Tripepi G, Malatino LS, et al. Plasma concentration of asymmetrical dimethylarginine and mortality in patients with end-stage renal disease: a prospective study. Lancet (2001) 358(9299):2113–7. doi: 10.1016/s0140-6736(01)07217-8
80. Triches CB, Quinto M, Mayer S, Batista M, Zanella MT. Relation of asymmetrical dimethylarginine levels with renal outcomes in hypertensive patients with and without type 2 diabetes mellitus. J Diabetes Complications (2018) 32(3):316–20. doi: 10.1016/j.jdiacomp.2017.12.006
81. Yilmaz MI, Sonmez A, Saglam M, Qureshi AR, Carrero JJ, Caglar K, et al. Adma levels correlate with proteinuria, secondary amyloidosis, and endothelial dysfunction. J Am Soc Nephrol (2008) 19(2):388–95. doi: 10.1681/ASN.2007040461
82. Kaida Y, Ueda S, Yamagishi S, Nakayama Y, Ando R, Iwatani R, et al. Proteinuria elevates asymmetric dimethylarginine levels via protein arginine methyltransferase-1 overexpression in a rat model of nephrotic syndrome. Life Sci (2012) 91(9-10):301–5. doi: 10.1016/j.lfs.2012.06.015
83. Wetzel MD, Gao T, Stanley K, Cooper TK, Morris SM Jr., Awad AS. Enhancing kidney ddah-1 expression by adenovirus delivery reduces adma and ameliorates diabetic nephropathy. Am J Physiol Renal Physiol (2020) 318(2):F509–f17. doi: 10.1152/ajprenal.00518.2019
84. Nakayama Y, Ueda S, Yamagishi S, Obara N, Taguchi K, Ando R, et al. Asymmetric dimethylarginine accumulates in the kidney during Ischemia/Reperfusion injury. Kidney Int (2014) 85(3):570–8. doi: 10.1038/ki.2013.398
85. Tomlinson JA, Caplin B, Boruc O, Bruce-Cobbold C, Cutillas P, Dormann D, et al. Reduced renal methylarginine metabolism protects against progressive kidney damage. J Am Soc Nephrol (2015) 26(12):3045–59. doi: 10.1681/ASN.2014030280
86. Ueda S, Yamagishi S, Matsumoto Y, Kaida Y, Fujimi-Hayashida A, Koike K, et al. Involvement of asymmetric dimethylarginine (Adma) in glomerular capillary loss and sclerosis in a rat model of chronic kidney disease (Ckd). Life Sci (2009) 84(23-24):853–6. doi: 10.1016/j.lfs.2009.03.018
87. Wang L, Zhang D, Zheng J, Feng Y, Zhang Y, Liu W. Actin cytoskeleton-dependent pathways for adma-induced nf-kappab activation and tgf-beta high expression in human renal glomerular endothelial cells. Acta Biochim Biophys Sin (Shanghai) (2012) 44(11):918–23. doi: 10.1093/abbs/gms077
88. Liu M, Ning X, Li R, Yang Z, Yang X, Sun S, et al. Signalling pathways involved in hypoxia-induced renal fibrosis. J Cell Mol Med (2017) 21(7):1248–59. doi: 10.1111/jcmm.13060
89. Gonzalez DM, Medici D. Signaling mechanisms of the epithelial-mesenchymal transition. Sci Signal (2014) 7(344):re8. doi: 10.1126/scisignal.2005189
90. Zeisberg EM, Potenta SE, Sugimoto H, Zeisberg M, Kalluri R. Fibroblasts in kidney fibrosis emerge Via endothelial-to-Mesenchymal transition. J Am Soc Nephrol (2008) 19(12):2282–7. doi: 10.1681/ASN.2008050513
91. Mihout F, Shweke N, Bige N, Jouanneau C, Dussaule JC, Ronco P, et al. Asymmetric dimethylarginine (Adma) induces chronic kidney disease through a mechanism involving collagen and tgf-Beta1 synthesis. J Pathol (2011) 223(1):37–45. doi: 10.1002/path.2769
92. Jayachandran I, Sundararajan S, Venkatesan S, Paadukaana S, Balasubramanyam M, Mohan V, et al. Asymmetric dimethylarginine (Adma) accelerates renal cell fibrosis under high glucose condition through Nox4/Ros/Erk signaling pathway. Sci Rep (2020) 10(1):16005. doi: 10.1038/s41598-020-72943-2
93. Wang D, Li H, Weir EK, Xu Y, Xu D, Chen Y. Dimethylarginine dimethylaminohydrolase 1 deficiency aggravates monocrotaline-induced pulmonary oxidative stress, pulmonary arterial hypertension and right heart failure in rats. Int J Cardiol (2019) 295:14–20. doi: 10.1016/j.ijcard.2019.07.078
94. Callaghan BC, Cheng HT, Stables CL, Smith AL, Feldman EL. Diabetic neuropathy: clinical manifestations and current treatments. Lancet Neurol (2012) 11(6):521–34. doi: 10.1016/S1474-4422(12)70065-0
95. Gordois A, Scuffham P, Shearer A, Oglesby A, Tobian JA. The health care costs of diabetic peripheral neuropathy in the U.S. Diabetes Care (2003) 26(6):1790–5. doi: 10.2337/diacare.26.6.1790
96. Shi Y, Vanhoutte PM. Macro- and microvascular endothelial dysfunction in diabetes. J Diabetes (2017) 9(5):434–49. doi: 10.1111/1753-0407.12521
97. Sztanek F, Lőrincz H, Molnár Á, Szentpéteri A, Zöld E, Seres I, et al. The effect of A-lipoic acid treatment on plasma asymmetric dimethylarginine, a biomarker of endothelial dysfunction in diabetic neuropathy. Arch Med Sci (2020). Available at: https://www.archivesofmedicalscience.com/The-effect-of-lipoic-acid-treatment-on-plasma-asymmetric-dimethylarginine-a-biomarker,119785,0,2.html.
98. Stojanovic I, Djordjevic G, Pavlovic R, Djordjevic V, Pavlovic D, Cvetkovic T, et al. The importance of l-arginine metabolism modulation in diabetic patients with distal symmetric polyneuropathy. J Neurol Sci (2013) 324(1-2):40–4. doi: 10.1016/j.jns.2012.09.026
99. El-Mesallamy HO, Hamdy NM, Ezzat OA, Reda AM. Levels of soluble advanced glycation end product-receptors and other soluble serum markers as indicators of diabetic neuropathy in the foot. J Investig Med (2011) 59(8):1233–8. doi: 10.2130/JIM.0b013e318231db64
100. Kyrillos F, El-Nahas M, Amer T, Albayoumy A, Abdulaziz M. Plasma levels of asymmetric di methyl arginine in diabetic patients with neuropathic foot ulceration. Zagazig Univ Med J (2015) 21(2):161–7 doi: 10.21608/zumj.2015.4509
101. Yasar H, Senol MG, Kendirli T, Onem Y, Ozdag F, Saracoglu M. Serum asymmetric dimethylarginine levels in diabetic patients with neuropathy. Diabetes Res Clin Pract (2011) 92(2):223–7. doi: 10.1016/j.diabres.2011.01.015
102. Jia G, Hill MA, Sowers JR. Diabetic cardiomyopathy: an update of mechanisms contributing to this clinical entity. Circ Res (2018) 122(4):624–38. doi: 10.1161/CIRCRESAHA.117.311586
103. Boudina S, Abel ED. Diabetic cardiomyopathy, causes and effects. Rev Endocr Metab Disord (2010) 11(1):31–9. doi: 10.1007/s11154-010-9131-7
104. Fischer VW, Barner HB, Leskiw ML. Capillary basal laminar thichness in diabetic human myocardium. Diabetes (1979) 28(8):713–9. doi: 10.2337/diab.28.8.713
105. Factor SM, Okun EM, Minase T. Capillary microaneurysms in the human diabetic heart. N Engl J Med (1980) 302(7):384–8. doi: 10.1056/nejm198002143020706
106. Cavusoglu E, Ruwende C, Chopra V, Poludasu S, Yanamadala S, Frishman WH, et al. Relation of baseline plasma adma levels to cardiovascular morbidity and mortality at two years in men with diabetes mellitus referred for coronary angiography. Atherosclerosis (2010) 210(1):226–31. doi: 10.1016/j.atherosclerosis.2009.10.034
107. Tan Y, Zhang Z, Zheng C, Wintergerst KA, Keller BB, Cai L. Mechanisms of diabetic cardiomyopathy and potential therapeutic strategies: preclinical and clinical evidence. Nat Rev Cardiol (2020) 17(9):585–607. doi: 10.1038/s41569-020-0339-2
108. Adameova A, Dhalla NS. Role of microangiopathy in diabetic cardiomyopathy. Heart Fail Rev (2014) 19(1):25–33. doi: 10.1007/s10741-013-9378-7
109. Chou E, Suzuma I, Way KJ, Opland D, Clermont AC, Naruse K, et al. Decreased cardiac expression of vascular endothelial growth factor and its receptors in insulin-resistant and diabetic states: a possible explanation for impaired collateral formation in cardiac tissue. Circulation (2002) 105(3):373–9. doi: 10.1161/hc0302.102143
110. Widyantoro B, Emoto N, Nakayama K, Anggrahini DW, Adiarto S, Iwasa N, et al. Endothelial cell-derived endothelin-1 promotes cardiac fibrosis in diabetic hearts through stimulation of endothelial-to-Mesenchymal transition. Circulation (2010) 121(22):2407–18. doi: 10.1161/CIRCULATIONAHA.110.938217
111. Zhu ZD, Ye JM, Fu XM, Wang XC, Ye JY, Wu XR, et al. Ddah2 alleviates myocardial fibrosis in diabetic cardiomyopathy through activation of the Ddah/Adma/Nos/No pathway in rats. Int J Mol Med (2019) 43(2):749–60. doi: 10.3892/ijmm.2018.4034
112. Mishra PK, Givvimani S, Chavali V, Tyagi SC. Cardiac matrix: a clue for future therapy. Biochim Biophys Acta (2013) 1832(12):2271–6. doi: 10.1016/j.bbadis.2013.09.004
113. Xiong Y, Hai C-X, Fang W-J, Lei Y-P, Li X-M, Zhou X-K. Endogenous asymmetric dimethylarginine accumulation contributes to the suppression of myocardial mitochondrial biogenesis in type 2 diabetic rats. Nutr Metab (2020) 17(1):1–17 doi: 10.1186/s12986-020-00486-4
114. Xiong Y, He Y-L, Li X-M, Nie F, Zhou X-K. Endogenous asymmetric dimethylarginine accumulation precipitates the cardiac and mitochondrial dysfunctions in type 1 diabetic rats. Eur J Pharmacol (2021) 902:1–12. doi: 10.1016/j.ejphar.2021.174081
115. Fang WJ, Li XM, Zhou XK, Xiong Y. Resveratrol improves diabetic cardiomyopathy by preventing asymmetric dimethylarginine-caused peroxisome proliferator-activated receptor-gamma coactivator-1alpha acetylation. Eur J Pharmacol (2022) 936:175342. doi: 10.1016/j.ejphar.2022.175342
Keywords: diabetes mellitus, asymmetric dimethylarginine, microvascular complications, diabetic retinopathy, diabetic nephropathy, diabetic neuropathy, diabetic cardiomyopathy
Citation: Guo X, Xing Y and Jin W (2023) Role of ADMA in the pathogenesis of microvascular complications in type 2 diabetes mellitus. Front. Endocrinol. 14:1183586. doi: 10.3389/fendo.2023.1183586
Received: 10 March 2023; Accepted: 11 April 2023;
Published: 21 April 2023.
Edited by:
Yanshan Dai, Bristol Myers Squibb, United StatesReviewed by:
Hongzhi Chen, Central South University, ChinaQingbo Chen, University of Massachusetts Medical School, United States
Copyright © 2023 Guo, Xing and Jin. This is an open-access article distributed under the terms of the Creative Commons Attribution License (CC BY). The use, distribution or reproduction in other forums is permitted, provided the original author(s) and the copyright owner(s) are credited and that the original publication in this journal is cited, in accordance with accepted academic practice. No use, distribution or reproduction is permitted which does not comply with these terms.
*Correspondence: Yiqiao Xing, yiqiao_xing57@whu.edu.cn; Wei Jin, rm001566@whu.edu.cn