- 1Division of Endocrinology Diabetes and Metabolism, Baylor College of Medicine, Houston, TX, United States
- 2Center for Translational Research on Inflammatory Disease, Michael E. DeBakey Veterans Affairs (VA) Medical Center, Houston, TX, United States
Fractures associated with Type2 diabetes (T2DM) are major public health concerns in an increasingly obese and aging population. Patients with obesity or T2DM have normal or better than normal bone mineral density but at an increased risk for fractures. Hence it is crucial to understand the pathophysiology and mechanism of how T2DM and obesity result in altered bone physiology leading to increased fracture risk. Although enhanced osteoclast mediated bone resorption has been reported for these patients, the most notable observation among patients with T2DM is the reduction in bone formation from mostly dysfunction in osteoblast differentiation and survival. Studies have shown that obesity and T2DM are associated with increased adipogenesis which is most likely at the expense of reduced osteogenesis and myogenesis considering that adipocytes, osteoblasts, and myoblasts originate from the same progenitor cells. Furthermore, emerging data point to an inter-relationship between bone and metabolic homeostasis suggesting that these physiologic processes could be under the control of common regulatory pathways. Thus, this review aims to explore the complex mechanisms involved in lineage differentiation and their effect on bone pathophysiology in patients with obesity and T2DM along with an examination of potential novel pharmacological targets or a re-evaluation of existing drugs to improve bone homeostasis.
1 Introduction
1.1 Obesity type 2 diabetes and bone
Obesity is associated with increased risk of T2DM (1), Cardiovascular diseases (2) and Cancer (3). The World Health Organization (WHO) defined overweight as a BMI of 25 to 29.9 kg/m2 and obesity as a BMI greater than or equal to 30 kg/m2 (4). According to new world health Atlas 2022, by 2030, 20% of women and 14% of men and over 1 billion people will be living with obesity globally (https://www.worldobesityday.org) and nearly 1 in 4 adults will have severe obesity with prevalence of more than 25% higher in 25 states in US (5). Obesity may lead to T2DM and by 2035, the global prevalence of T2DM is likely to be 592 million (6). The duo (obesity and T2DM) increases as the population ages. Both conditions are associated with normal or better than normal bone mineral density (BMD) but paradoxically increase in the risk for fractures. Obesity is a risk factor for T2DM such that the bone phenotype in the two conditions likely overlap in a major way. Thus, this review aims to examine, the complicated underlying molecular mechanisms involved in the alteration in lineage differentiation and identify pharmacological targets that redirect cell differentiation from the adipogenic to the osteogenic/myogenic pathways.
1.2 Pathophysiology of skeletal fragility in obesity and T2DM
Increase in bone marrow adipose tissue volume has been reported both in diabetes and obesity (7). Earlier studies confirmed an increased risk for hip fracture in both male and female patients with type1 diabetes(T1DM) (8). Osteoporotic fractures especially on the hip, are increased in both T1DM and T2DM, but the risk is 7 fold for those with T1DM compared to 1.38 fold increase in hip fractures of T2DM (9). The increased risk in T1DM is due to lack of anabolic effects of insulin which may contribute to lower peak bone mass while bone mass seems to be preserved in the T2DM (10). Regardless, studies have shown that both T1DM and T2DM is associated with a switch from osteogenesis to adipogenesis, increase in bone marrow adiposity leading to cellular marrow replacement with fat (11). The higher BMD in obesity is believed to be due to skeletal adaptation to accommodate mechanical load and strain (12, 13). However, visceral and total adiposity was not associated with vertebral fractures in men (14). Some studies reported negative correlation between BMD (15, 16). Obesity, is associated with increased secretion of pro-inflammatory factors (as described in Figure 1)) that may be harmful to bone and activation peroxisome proliferator-activated receptor-γ (PPARγ) and CCAAT/enhancer-binding protein alpha (CEBPa), nuclear factor kappa light chain enhancer of activated B cells (NF-Kb) pathway (17, 18). Adipokines produced in the adipocytes have inverse relationship to fat mass (19, 20), variably effects bone mass (21). Cao et al, found reduced serum bone formation marker osteocalcin (OCN) and increased bone resorption markers, serum C-telopeptide of type I collagen (CTx) and Tartrate-resistant acid phosphatase 5b(TRAP5b) in diet-induced obese mice (22). Furthermore, Jain et al.,studies confirmed that visceral adipose tissue (VAT) is negatively associated with bone mineral density (23). On the other hand, in T2D BMD is normal or above normal,likely protective against vertebral fractures (24), but some studies show reduced BMD (25, 26) due to accumulation of advanced glycation end products (AGEs) (27, 28) increased proinflammatory cytokines such as TNF-a, IL-6 (28, 29) high sclerostin levels (30) leading to reduction in bone formation, OCN (31) and (Procollagen I N-terminal propeptide) P1NP levels in T2DM (31, 32) and impairment in osteoblastogenesis (33–35). There is also reduction in bone resorption markers (CTX and TRAP5b) (28) though bone turnover markers are not as predictive of fractures compared to BMD and maybe difficult to interpret,. Mesenchymal stem cells residents in the bone marrow (BMSCs) are endowed with plasticity and can differentiate into the osteogenic, myogenic or adipogenic lineages depending on the predominant transcription factors present. The enhanced potential of skeletal muscle satellite cells or SMSCs for adipogenic differentiation was observed in diabetic rats using a 3-dimensional matrices in vitro model (36) and from in genetically obese Zucker rats (37). Furthermore, myoblasts isolated from Wnt10b (wingless-type mouse mammary tumor virus integration site) null mice showed increased adipogenic potential (38). Jiang et al. found that PRDM16 (Positive Regulatory Domain Motif -16) over expression could partially reverse the effect of mir-499 on adipogenic differentiation of SMSCs and maybe a target for obesity treatment (39). Therefore, there is a need to fully understand the molecular mechanisms behind this shift along with investigations on common regulatory pathways.
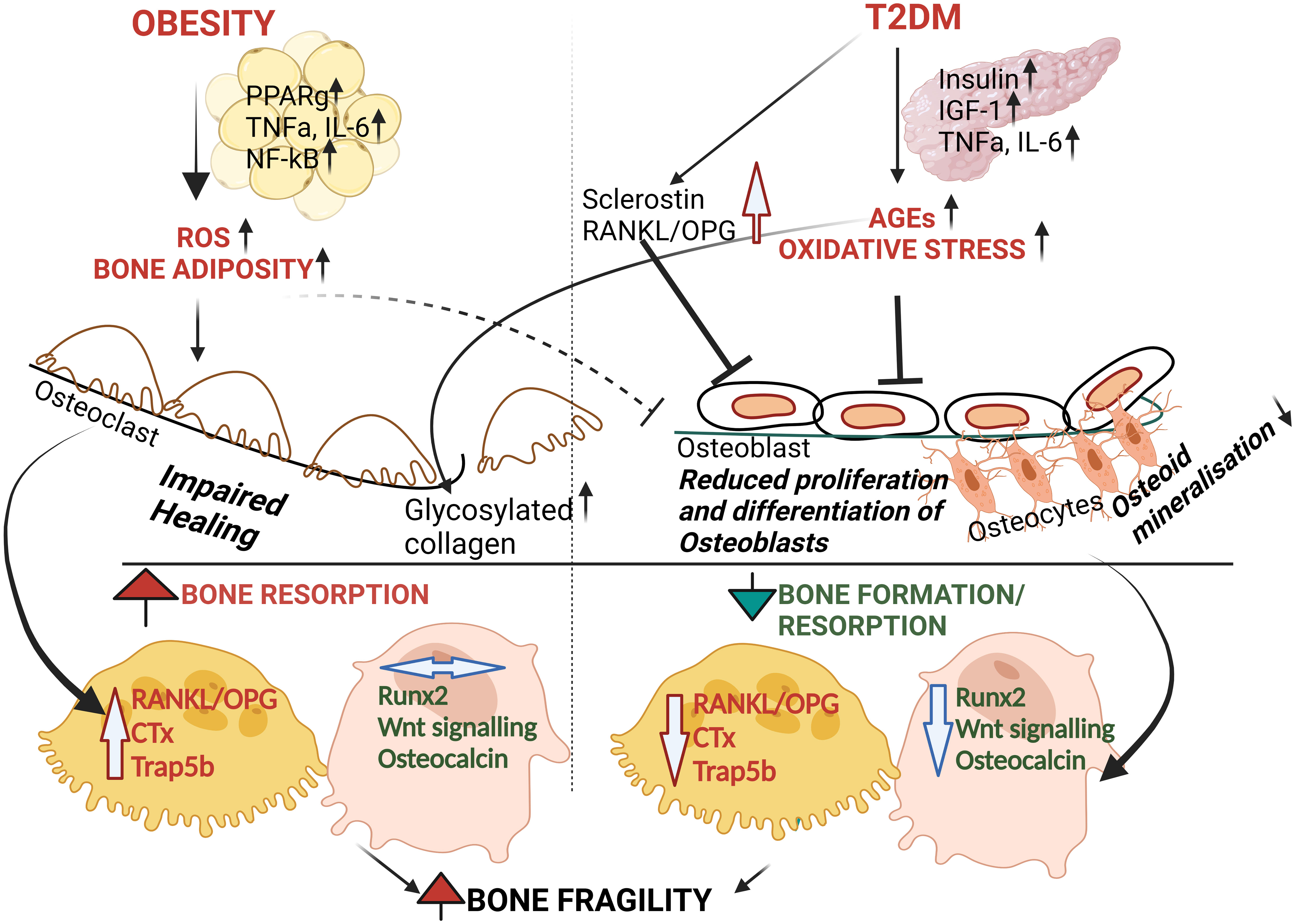
Figure 1 Schematic proposal of pathophysiology of bone fragility in Obesity and T2DM. Even though underlying mechanisms are unclear, both T2DM and Obesity causes oxidative stress and inflammation. T2DM causes the accumulation of AGEs with increased oxidative stress leading to osteoblast dysfunction and reduced bone formation (i.e. reduced Runx2 and OCN) followed by increasing bone fragility. On the other hand, obesity is associated with increased pro-inflammatory markers and pro-resorptive factors such as RANKL, TRAP5b. However, whether obesity by itself has any effect on osteoblastic function is unclear. Altogether, the above contribute to simultaneous effect on bone tissue homeostasis leading to bone fragility15-21.
Despite the high BMD in obese subjects, these individuals are at increased risk of fractures at nonvertebral skeletal sites (i.e. lower extremities and humerus) (40–42). There are several mechanisms proposed to explain the increased skeletal fragility in obese individuals such as low vitamin D with consequent secondary hyperparathyroidism (43, 44), increased levels of proinflammatory cytokine release from the expanded adipose tissue volume and possibly the high levels of leptin and reduced adiponectin though both have variable effects on the skeleton (44) Low vitamin D is easily corrected clinically but the increase in adipose tissue volume and subsequent proinflammatory state requires more effort (45). Likewise, studies (46–52) have shown that BMD is also higher in patients with T2DM compared to nondiabetic subjects but associated with an increased fracture risk affecting any skeletal site (52, 53). Given that obesity is a risk for T2DM, it would be hard to separate out the effect of obesity from diabetes on the bone. Clinical studies (54–57), including from our group (58, 59) demonstrated suppressed bone formation maker OCN, P1NP (48) and bone resorption marker (CTX) in patients with T2DM. Additionally, Vigevano et al. showed that among obese men, those with concurrent T2DM had higher bone density but reduced bone turnover markers (CTx and OCN) (60) and lower bone strength suggesting that if obesity has a negative effect on the bone, T2DM further adds to the skeletal compromise from obesity alone or that diabetes is the driver for the skeletal phenotype in those who have both. In a study of older women, relative to nonobese without diabetes, those with diabetes but nonobese had a 1.9 risk for vertebral or hip fracture and 1.4 for nonvertebral and non-hip fractures. The corresponding numbers for nondiabetic but obese were 1.2 and 1.1, respectively, while they were 1.5 and 1.8, respectively, for those with both diabetes and obesity (61). Meanwhile, given the clinical observation of increased in bone marrow fat in obesity and diabetes, it is likely that MSCs are involved in the pathology of skeletal fragility seen in in patients with obesity, diabetes or both. This hypothesis was supported by a study from Tencerova et al., which showed that an increase in adipocyte differentiation along with accelerated senescence in BMSCs lead to bone fragility in obese men (62). Thus, pathophysiology of brittle bone in both obesity and T2DM may be attributed as due to the mechanisms discussed below.
1.3 Lack of regulation of brown fat synthesis and/or enhancement of adipogenesis
Differentiation of fat and its control is regulated by transcriptional cascade which can affect the physiological functioning of white and brown adipocytes (63). Normally, the conversion of pre-adipocytes to mature lipid containing adipocytes is a multi-step complex process regulated by transcription factors which can be altered by inflammatory signaling pathways of obesity (64). Of all transcription factors, (PPARg and CEBPa) are the key regulators in driving fat cell differentiation (65, 66). Crucially, PPARγ which is the driving factor for adipogenesis needs co-activation by CEBPa to promote myogenesis (67–69) Cohen et al. (70) found that knock out of PRDM16 resulted in obesity and severe insulin resistance mice fed a high-fat diet (70). Several pre-clinical experiments have confirmed the association of PRDM16 with PGC 1α (71) and PPARγ (72) resulting in activation of the myogenic cascade (73) and BAT formation. Recent human studies have shown positive correlations between BAT volume and bone density (74–76). Nevertheless, PPARy remains the novel target because of its dual role in MSC-derived adipogenesis.as well as HSC-derived osteoclastogenesis (67). Studies of Beekman et al. (77) showed that PPARγ inhibitor, GW9962 has no direct impact on bone marrow adipose tissue (BMAT) in C3H/HeJ mice (77) suggesting that BMAT accumulation might be regulated by a different mechanism. In contrast, another study demonstrated upregulation of sphingosine-1-phosphate (S1P) by S1P lyase, mediated PPARg suppression resulting in enhanced bone formation (78). Similarly, Wnt cascade also plays a significant role in the initiation of adipogenesis in obese people (79, 80). Normally, Wnt ligands bind to one of the frizzled family receptors (FZD) and to a co-receptor low-density lipoprotein receptor-related protein (LRP) to activate β-catenin dependent pathway (canonical signaling) and subsequent bone formation (81, 82). Conversely, Wnt signal transduction seems to be redundant in both obesity (83) and T2DM (84). Previous studies showed a close relationship between upregulation of classical Wnt signaling and enhanced myogenesis and/or osteogenesis (85, 86). In humans, subcutaneous injection of Romosozumab which targets sclerostin (an inhibitor of the Wnt pathway), reduced the risk of vertebral and clinical fractures in women with postmenopausal osteoporosis and hence this drug was approved to treat osteoporosis (87). Thus, attractive therapeutic targets using Wnt-targets, acting on obesity associated genes such as secreted frizzled receptor(Srfp1) and Wnt inhibitory factor (WIF-1) acting on classical Wnt-β catenin pathway are undergoing pre-clinical and clinical trials (81).
1.4 Effect of T2DM and obesity on satellite cells and bone senescence
Sarcopenia which is defined as low muscle mass and function is common in the elderly and is associated with increased falls and fractures (88–93). It can accompany obesity in a significant number of older adults for a condition called sarcopenic obesity resulting in frailty (94). Exercise improved muscle strength and physical function in older adults (95–99) and mice (100). For instance, the Lifestyle Interventions and Independence for Elders (LIFE) study (101) in 424 sedentary older persons showed that engaging in moderate-intensity physical activity (combination of aerobic and resistance) intervention reduced the incidence of major mobility disability with an increase in the Short Physical Performance Battery (SPPB) (102). Similarly weight loss from lifestyle intervention by a combination diet and exercise improves physical function, and ameliorates frailty in obese older adults (103–105). In addition, these studies exercise added to diet resulted in amelioration of muscle and bone loss experienced by those who were on diet alone. Since obesity is a risk factor for T2DM, it is expected that a significant number of obese patients with T2DM also have sarcopenic obesity (106). It is likely that skeletal muscle mass and function relies on muscle progenitor cells cascade including satellite cells, interstitial progenitor cells and hence discovery of novel therapeutic targets to improve muscle mass and function are of utmost importance (107). Although the mechanism leading to impairment of muscle dysfunction in obesity remains unclear, the proinflammatory cytokines present in the muscles such TNFα, IL-6 which are elevated in obesity has been found to be reduced by exercise (108).
Verpoorten et al., showed that cluster of differentiation (CD36) deficient mice although protected from diet-induced obesity, developed impaired satellite cell function and muscle regeneration (109). Apart from adipogenic and inflammatory markers, impairment in fatty acid uptake via CD36 can also affect bone integrity (110). Our recent studies showed that in patients with poorly-controlled T2DM had significantly higher circulating osteogenic precursor cells (COPs) compared to well-controlled diabetics. This could mean that COPs are markers of poor metabolic control or the possibility for uncontrolled hyperglycemia results in retardation of differentiation of COPs into mature osteoblasts (59). Studies from our lab also confirmed, that poor glycemic control over 1year is associated with poor bone microarchitecture and strength in men with T2DM (59, 111). On the other hand, alteration in crucial genes of myogenesis can promote development of osteoprogenitor cells. Studies from Hashimoto et al. (112), showed both primary and immortalized progenitor cells derived from muscle of healthy non-dystrophic woman expressed two osteoblastic specific bone proteins, alkaline phosphatase and Runt-related transcription factor 2 (Runx2) (112). Studies in knock-out mice (113) and other aging studies (114) also showed that Runx2 deficiency resulted in impairment in osteoblastogenesis and depletion for satellite cells. Thus, it is likely that satellite cells and its gene machinery, play significant role on mediating the process of bone repair and thus, can be used as strategy in treatment (115). The next section discusses on the targets to minimize/nullify the inflammatory oxidative stress and enhance osteogenesis.
2 Emerging therapeutic treatment in bone loss of obese and T2DM patients
Currently, there are numerous medications and therapeutic options for the treatment of osteoporosis but not for bone fragility in diabetic or obese patients in particular (116–120). Given this unmet need, understanding the pathways involved in bone disease in these patients will potentially lead to future strategies to prevent fractures.
2.1 Novel therapies -targeting bone formation
2.1.1 Role of PRDM16 in adipo-myogenic shift and osteogenesis
The novel therapeutic strategies that suppress bone marrow adipogenesis and bone resorption and enhanced bone formation deserve further research. Human PRDM16 located on chromosome 1p36 with 370kb, a zinc finger containing transcriptional regulator protein (121), was recently reported to interact with PPARg (122), CEBPa (123) and/or Pgc-1a (124) promote browning of fat. Additionally, Prdm16 represses adipogenesis mediated through its association with C-terminal binding proteins (CtBP-1 and -2) suggesting that PPARy can act as bi-directional switch between adipogenesis and myogenesis through its interaction with multiple proteins (125). Apart from Prdm16 and PPARγ, Pgc1a might act as co-activator and play critical role from adipogenic to myogenic shift. This was suggested by studies from Seo et al., showing reduction in obesity among mice fed a high-fat diet through suppression of adipogenesis by upregulation of Prdm16, Pgc1a and uncoupling protein 1 (UCP1) (126). Furthermore, Kaneda et al., found a synergistic association between Prdm16 and Osteogenic Runx2 gene in Mel1/Prdm16-deficient mice (127). They observed that BMP2 stimulated osteoblasts isolated from Mel1/Prdm16+/- mice are highly stained with alizarin due to extensive calcification and enhanced expression of osteogenic markers such as osteopontin (OPN), OCN when compared to control mice (127). Thus, any ligand inducing a confirmational change in PPARg promoting the dissociation of transcriptional repressors and intake of co-activators (Pgc1a) leading to activation of the myogenic cascade (as described in Figure 2) along with promotion of the osteogenic Runx2 gene might be a novel therapeutic targets. The research on these transcriptional activators needs to be investigated. In the next section, we explore the targets involved in myogenesis and osteogenesis and blocking of adipogenesis.
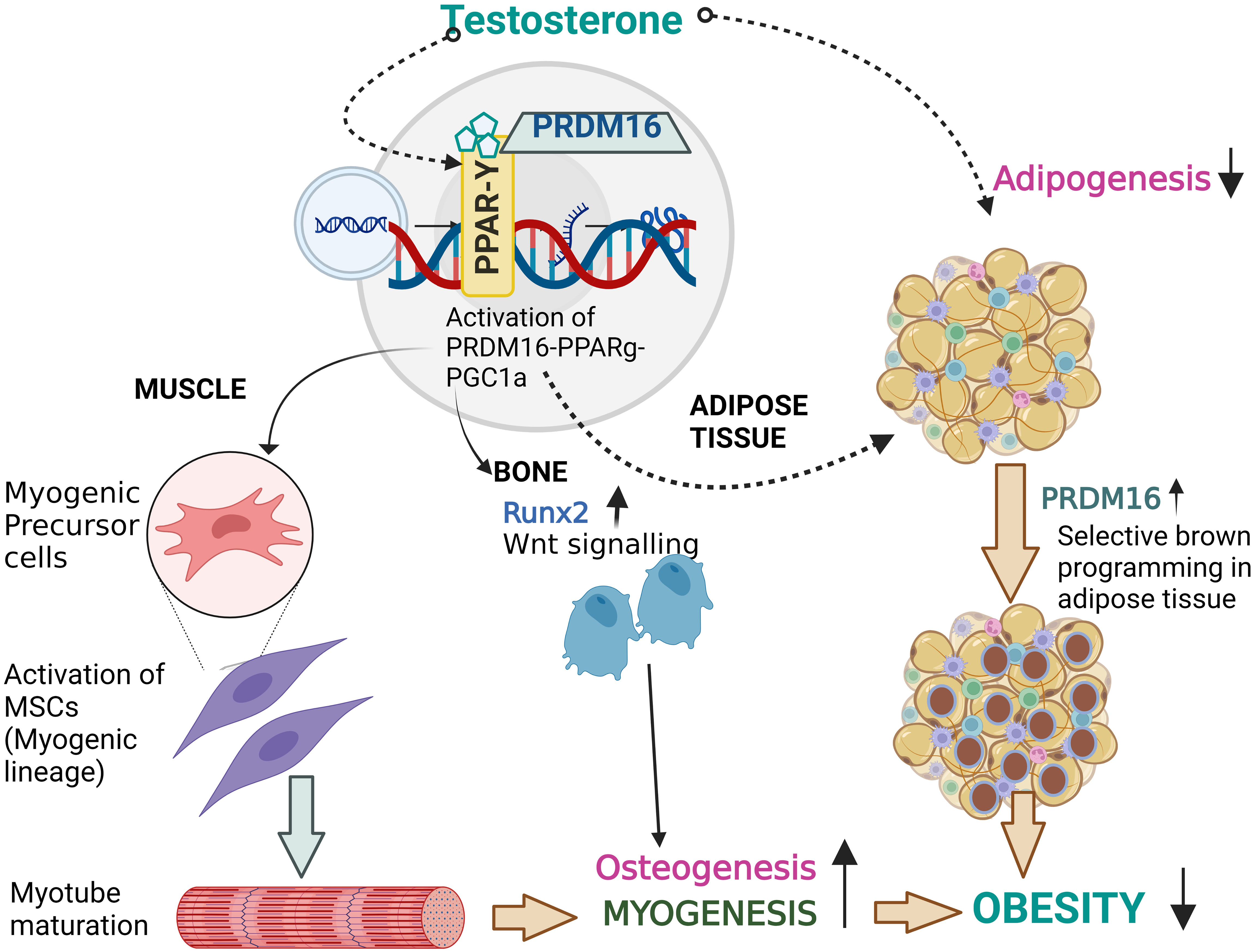
Figure 2 Proposed effect of T therapy in Obesity. Binding of testosterone might initiate the PPARγ-Prdm16-Pgc1a complex with retinoid X receptor (RXR) which not only activates the browning of adipose tissue by re-programming but may also activate the myogenic cascade and runx2 gene involved osteogenesis. Thus, T therapy with its activator Prdm16 might be a novel therapeutic target and research on this transcriptional activator needs to be consider.
2.1.2 Stem cell therapy
Obesity and T2DM enhance the recruitment of adipocyte precursors, resulting in fat deposition in the viscera, muscles, and other organs and bone fragility. Hence, it is critical to develop therapies to prevent adipocyte differentiation. Stem cell therapy remains an attractive candidate for tissue engineering (128). Adipose-derived mesenchymal stem cells (AD-MSCs) can exhibit various phenotypes of ecto and endodermal hematopoietic stem cells (HSCs) and mesodermal adipocytes, myocytes and osteocytes (129). Louwen et al. showed that human ASCs from obese patients had reduced capacity for osteogenic lineage differentiation (130, 131). Furthermore, Lee et al., reported that intra-articular injection of adipose derived AD-MSCs in patients with knee osteoarthritis, resulted in functional improvements for 6 months without major adverse effect (131). Thus, AD-MSC transplantation is feasible and can possibly be used to repair areas where osteoblastogenesis and subsequent endogenous bone formation is necessary (131, 132). This therapeutic potential of AD-MSCs depends on understanding the mechanism of differentiation capacity in the BM. In line with this, are over 1000 clinical trials registered with the Clinicaltrials.gov(http://www.Clinicaltrials.gov) which may demonstrate the clinical applications of AD-MSCs against bone fragility (133).
2.1.3 Si RNA and other inhibitors
Targeted drug delivery strategies with reliable, efficient delivery remain crucial for cell-based therapy. Even though the delivery of siRNA to bone is challenging due to limited drug penetration and poor vascular perfusion, siRNAs (Short interfering RNA) play pivotal role than chemical-based studies (134), Previous studies targeting Shn3 (adaptor protein Schnurri-3) gene silencing by genetically engineered BT-Exo-siShn3 novel MSC-derived exosome as carrier, resulted in osteogenesis along with blocking of Receptor activator of nuclear factor kappa-Β ligand/Dickkopf WNT Signaling Pathway Inhibitor 1 (RANKL/DKK-1), thereby inhibiting osteoclastogenesis in mouse MC3T3-E1 pre-osteoblast cell line (135). Liang et al, developed CH6 aptamer–functionalized lipid nanoparticles (LNPs), specifically targeting both rat and human osteoblasts, was found to promote bone formation (136). Due to high stability and non-immunogenicity aptamers, small single stranded oligonucleotides which can form 3D structure, are used in the ongoing clinical trials for their potential use as novel drug therapy targets for osteoporosis (137). Furthermore, in order to overcome the limitations of direct drug delivery, the combination of nanotechnology with bone target agents can provide more effective therapeutic approach in the near future (138).
2.1.4 Testosterone therapy
Testosterone which is an old drug used for treatment of hypogonadism, has been found in recent years to have beneficial effects in both myogenesis and osteogenesis (139). T is well-known to improve BMD and bone quality in men (140–145). Various studies demonstrated that T therapy increased, levels of OCN (146, 147) and reduced levels of CTx (144, 145) (148) In vitro studies showed that 5α-dihydrotestosterone (a potent agonist of androgen receptor synthesized from T by the enzyme 5α-reductase) treatment of bone forming MC3T3-E1 cells not only enhanced osteoblast differentiation (149) but also downregulated bone resorption promoter RANKL (150) in human osteoblastic cells. Furthermore, testosterone administration increased the width of epiphyseal growth plate of growing rats directly (151, 152). Similarly, Chin et al, showed decreased trabecular bone volume and increased trabecular porosity in orchiectomized (ORX) rats when compared to sham-untreated (SH) group. Conversely, T treatment (7mg/kg) for 8weeks in ORX-TE group prevented these changes and decreased expression of RANKL significantly when compared to SH group (153).
Muscle function contributes in some measure to bone mass and testosterone increases muscle mass and function (154). Preclinical studies suggest a critical role of the adipogenic/myogenic/osteogenic switch on the observed effects of T therapy. Using mouse C3H 10T1/2 pluripotent cells, Singh et al, evaluated the effect of T treatment (0-300 nM) on the myogenic/adipogenic conversion by immunocytochemical staining for MyoD and PPARy (155). They found that T not only promotes commitment of SMSCs into the myogenic lineage but also inhibits adipogenic lineage. Apart from the myogenic machinery, Gao et al., further reported that osteoblast differentiation was activated by T therapy in MC3T3-E1 cells through ERK-1/2, activated Runx2 pathway (156). Changes in body composition and bone density with T therapy from our lab and other investigators support the above findings from in-vitro and animal studies (146, 157, 158). Hence, we hypothesize that the reciprocal effect of T therapy on fat mass, lean mass and bone mass is due to the shift in lineage differentiation from adipogenesis to both myogenesis and osteogenesis. Thus, this concept provided a unifying mechanism for the observed effect of T in hypogonadal men. Roles of other gene machinery such as Prdm16. Pgc1a on the adipogenic/myogenic cascade need to be explored. We hypothesize that T therapy activates the trio cascade PPARγ-Prdm16-Pgc1a leading to initiation of the switch from adipogenesis to myogenesis along with promotion of osteogenesis (Figure 2) responsible for the observed positive effect on fat mass, lean mass and bone mass in hypogonadal men (146, 157, 158). The Endocrine Society has suggested the use of T to maintain or prevent loss of lean mass in men with HIV (159). Given the emergence of a substantial amount of data showing the positive effects of T on body composition and bone, it is possible that obesity may become one of the indications for T therapy.
3 Conclusion
Obesity and T2DM are increasing at an alarming rate worldwide. Despite the normal or better than normal BMD, both appear to be associated with increased fracture risk, most especially with T2DM. Though it is difficult to separate the skeletal effects of one from the other, there seems to be more data supporting the negative skeletal effects of T2DM than that of obesity, however, this is a complicated issue that needs further investigation. To date, there is no drug approved specifically to treat skeletal fragility in these patients. Since BMD cannot alone predict the risk of bone fragility, this review explores potential new methods or agents to promote the adipo-myogenic/osteogenic lineage shift which may include but not limited to targeting Prdm16, stem cell therapy, si-RNA inhibitors and repurposing of an old drug, testosterone in the general population of patients with obesity, T2DM or both. With further drug development, it is possible to prevent skeletal fragility and promote overall health in these patients.
Author contributions
SB and RA-V, conceptualization, resources and analysis, writing, reviewing, and editing. The figures in this manuscript were created with biorender software. All authors contributed to the article and approved the submitted version.
Funding
This work was supported by the US Department of Veterans Affairs Clinical Sciences Research and Development Merit Review Award 101CX001665 and National Institutes of Health R01HD093047 to RA-V.
Conflict of interest
The authors declare that the research was conducted in the absence of any commercial or financial relationships that could be construed as a potential conflict of interest.
Publisher’s note
All claims expressed in this article are solely those of the authors and do not necessarily represent those of their affiliated organizations, or those of the publisher, the editors and the reviewers. Any product that may be evaluated in this article, or claim that may be made by its manufacturer, is not guaranteed or endorsed by the publisher.
Author disclaimer
The contents do not represent the views of the U.S. Department of Veterans Affairs or the United States Government.
References
1. Maggio CA, Pi-Sunyer FX. Obesity and type 2 diabetes. Endocrinol Metab Clin North Am (2003) 32(4):805–22, viii. doi: 10.1016/S0889-8529(03)00071-9
2. Hariharan R, Odjidja EN, Scott D, Shivappa N, Hebert JR, Hodge A, et al. The dietary inflammatory index, obesity, type 2 diabetes, and cardiovascular risk factors and diseases. Obes Rev (2022) 23(1):e13349. doi: 10.1111/obr.13349
3. Avgerinos KI, Spyrou N, Mantzoros CS, Dalamaga M. Obesity and cancer risk: Emerging biological mechanisms and perspectives. Metabolism (2019) 92:121–35. doi: 10.1016/j.metabol.2018.11.001
4. Schetz M, De Jong A, Deane AM, Druml W, Hemelaar P, Pelosi P, et al. Obesity in the critically ill: a narrative review. Intensive Care Med (2019) 45(6):757–69. doi: 10.1007/s00134-019-05594-1
5. Ward ZJ, Bleich SN, Cradock AL, Barrett JL, Giles CM, Flax C, et al. Projected U.S. State-Level Prevalence of Adult Obesity and Severe Obesity. N Engl J Med (2019) 381(25):2440–50. doi: 10.1007/s00223-022-00993-x
6. Forouhi NG, Wareham NJ. Epidemiology of diabetes. Med (Abingdon) (2014) 42(12):698–702. doi: 10.1016/j.mpmed.2014.09.007
7. Walsh JS, Vilaca T. Obesity, Type 2 Diabetes and Bone in Adults. Calcif Tissue Int (2017) 100(5):528–35. doi: 10.1007/s00223-016-0229-0
8. Miao J, Brismar K, Nyren O, Ugarph-Morawski A, Ye W. Elevated hip fracture risk in type 1 diabetic patients: a population-based cohort study in Sweden. Diabetes Care (2005) 28(12):2850–5. doi: 10.2337/diacare.28.12.2850
9. Vestergaard P. Discrepancies in bone mineral density and fracture risk in patients with type 1 and type 2 diabetes–a meta-analysis. Osteoporos Int (2007) 18(4):427–44. doi: 10.1007/s00198-006-0253-4
10. Hofbauer LC, Busse B, Eastell R, Ferrari S, Frost M, Muller R, et al. Bone fragility in diabetes: novel concepts and clinical implications. Lancet Diabetes Endocrinol (2022) 10(3):207–20. doi: 10.1016/S2213-8587(21)00347-8
11. Sebo ZL, Rendina-Ruedy E, Ables GP, Lindskog DM, Rodeheffer MS, Fazeli PK, et al. Bone Marrow Adiposity: Basic and Clinical Implications. Endocr Rev (2019) 40(5):1187–206. doi: 10.1210/er.2018-00138
12. Aguirre LE, Colleluori G, Dorin R, Robbins D, Chen R, Jiang B, et al. Hypogonadal Men with Higher Body Mass Index have Higher Bone Density and Better Bone Quality but Reduced Muscle Density. CalcifTissue Int (2017) 101(6):602–11. doi: 10.1007/s00223-017-0316-x
13. Iwaniec UT, Turner RT. Influence of body weight on bone mass, architecture and turnover. J Endocrinol (2016) 230(3):R115–30. doi: 10.1530/JOE-16-0089
14. Hind K, Pearce M, Birrell F. Total and Visceral Adiposity Are Associated With Prevalent Vertebral Fracture in Women but Not Men at Age 62 Years: The Newcastle Thousand Families Study. J Bone Miner Res (2017) 32(5):1109–15. doi: 10.1002/jbmr.3085
15. Bhupathiraju SN, Dawson-Hughes B, Hannan MT, Lichtenstein AH, Tucker KL. Centrally located body fat is associated with lower bone mineral density in older Puerto Rican adults. Am J Clin Nutr (2011) 94(4):1063–70. doi: 10.3945/ajcn.111.016030
16. Zhao LJ, Liu YJ, Liu PY, Hamilton J, Recker RR, Deng HW. Relationship of obesity with osteoporosis. JClinEndocrinolMetab (2007) 92(5):1640–6. doi: 10.1210/jc.2006-0572
17. Ramirez-Moreno E, Arias-Rico J, Jimenez-Sanchez RC, Estrada-Luna D, Jimenez-Osorio AS, Zafra-Rojas QY, et al. Role of Bioactive Compounds in Obesity: Metabolic Mechanism Focused on Inflammation. Foods (2022) 11(9):1232. doi: 10.3390/foods11091232
18. Gkastaris K, Goulis DG, Potoupnis M, Anastasilakis AD, Kapetanos G. Obesity, osteoporosis and bone metabolism. J Musculoskelet Neuronal Interact (2020) 20(3):372–81.
19. Vega GL, Grundy SM. Metabolic risk susceptibility in men is partially related to adiponectin/leptin ratio. J Obes (2013) 2013:409679. doi: 10.1155/2013/409679
20. Gavrila A, Chan JL, Yiannakouris N, Kontogianni M, Miller LC, Orlova C, et al. Serum adiponectin levels are inversely associated with overall and central fat distribution but are not directly regulated by acute fasting or leptin administration in humans: cross-sectional and interventional studies. J Clin Endocrinol Metab (2003) 88(10):4823–31. doi: 10.1210/jc.2003-030214
21. Lenchik L, Register TC, Hsu FC, Lohman K, Nicklas BJ, Freedman BI, et al. Adiponectin as a novel determinant of bone mineral density and visceral fat. Bone (2003) 33(4):646–51. doi: 10.1016/S8756-3282(03)00237-0
22. Cao JJ, Sun L, Gao H. Diet-induced obesity alters bone remodeling leading to decreased femoral trabecular bone mass in mice. Ann N Y Acad Sci (2010) 1192:292–7. doi: 10.1111/j.1749-6632.2009.05252.x
23. Jain RK, Vokes T. Visceral Adipose Tissue is Negatively Associated With Bone Mineral Density in NHANES 2011-2018. J Endocr Soc (2023) 7(4):bvad008. doi: 10.1210/jendso/bvad008
24. Dytfeld J, Michalak M. Type 2 diabetes and risk of low-energy fractures in postmenopausal women: meta-analysis of observational studies. Aging Clin Exp Res (2017) 29(2):301–9. doi: 10.1007/s40520-016-0562-1
25. Faienza MF, Pontrelli P, Brunetti G. Type 2 diabetes and bone fragility in children and adults. World J Diabetes (2022) 13(11):900–11. doi: 10.4239/wjd.v13.i11.900
26. Kurra S, Siris E. Diabetes and bone health: the relationship between diabetes and osteoporosis-associated fractures. Diabetes Metab Res Rev (2011) 27(5):430–5. doi: 10.1002/dmrr.1197
27. Cavati G, Pirrotta F, Merlotti D, Ceccarelli E, Calabrese M, Gennari L, et al. Role of Advanced Glycation End-Products and Oxidative Stress in Type-2-Diabetes-Induced Bone Fragility and Implications on Fracture Risk Stratification. Antioxidants (Basel) (2023) 12(4):928. doi: 10.3390/antiox12040928
28. Mirza S, Hossain M, Mathews C, Martinez P, Pino P, Gay JL, et al. Type 2-diabetes is associated with elevated levels of TNF-alpha, IL-6 and adiponectin and low levels of leptin in a population of Mexican Americans: a cross-sectional study. Cytokine (2012) 57(1):136–42. doi: 10.1016/j.cyto.2011.09.029
29. Sanguineti R, Puddu A, Mach F, Montecucco F, Viviani GL. Advanced glycation end products play adverse proinflammatory activities in osteoporosis. Mediators Inflammation (2014) 2014:975872. doi: 10.1155/2014/975872
30. Sylvawani M, Setyohadi B, Purnamasari D, Abdullah M, Kurniawan MR. Comparison of insulin-like growth factor-1 and sclerostin levels between premenopausal women with and without diabetes mellitus. J Taibah Univ Med Sci (2021) 16(5):719–23. doi: 10.1016/j.jtumed.2021.05.007
31. An Y, Liu S, Wang W, Dong H, Zhao W, Ke J, et al. Low serum levels of bone turnover markers are associated with the presence and severity of diabetic retinopathy in patients with type 2 diabetes mellitus. J Diabetes (2021) 13(2):111–23. doi: 10.1111/1753-0407.13089
32. Hou Y, Hou X, Nie Q, Xia Q, Hu R, Yang X, et al. Association of Bone Turnover Markers with Type 2 Diabetes Mellitus and Microvascular Complications: A Matched Case-Control Study. Diabetes Metab Syndr Obes (2023) 16:1177–92. doi: 10.2147/DMSO.S400285
33. Sanches CP, Vianna AGD, Barreto FC. The impact of type 2 diabetes on bone metabolism. Diabetol Metab Syndr (2017) 9:85. doi: 10.1186/s13098-017-0278-1
34. Wu X, Zhang Y, Xing Y, Zhao B, Zhou C, Wen Y, et al. High-fat and high-glucose microenvironment decreases Runx2 and TAZ expression and inhibits bone regeneration in the mouse. J Orthop Surg Res (2019) 14(1):55. doi: 10.1186/s13018-019-1084-2
35. Fowlkes JL, Bunn RC, Liu L, Wahl EC, Coleman HN, Cockrell GE, et al. Runt-related transcription factor 2 (RUNX2) and RUNX2-related osteogenic genes are down-regulated throughout osteogenesis in type 1 diabetes mellitus. Endocrinology (2008) 149(4):1697–704. doi: 10.1210/en.2007-1408
36. Acosta FM, Jia UA, Stojkova K, Pacelli S, Brey EM, Rathbone C. Divergent effects of myogenic differentiation and diabetes on the capacity for muscle precursor cell adipogenic differentiation in a fibrin matrix. Biochem Biophys Res Commun (2020) 526(1):21–8. doi: 10.1016/j.bbrc.2020.03.025
37. Scarda A, Franzin C, Milan G, Sanna M, Dal Pra C, Pagano C, et al. Increased adipogenic conversion of muscle satellite cells in obese Zucker rats. Int J Obes (Lond) (2010) 34(8):1319–27. doi: 10.1038/ijo.2010.47
38. Vertino AM, Taylor-Jones JM, Longo KA, Bearden ED, Lane TF, McGehee RE Jr., et al. Wnt10b deficiency promotes coexpression of myogenic and adipogenic programs in myoblasts. MolBiolCell (2005) 16(4):2039–48. doi: 10.1091/mbc.e04-08-0720
39. Jiang J, Li P, Ling H, Xu Z, Yi B, Zhu S. MiR-499/PRDM16 axis modulates the adipogenic differentiation of mouse skeletal muscle satellite cells. Hum Cell (2018) 31(4):282–91. doi: 10.1007/s13577-018-0210-5
40. Compston JE, Watts NB, Chapurlat R, Cooper C, Boonen S, Greenspan S, et al. Obesity is not protective against fracture in postmenopausal women: GLOW. AmJMed (2011) 124(11):1043–50. doi: 10.1016/j.amjmed.2011.06.013
41. Prieto-Alhambra D, Premaor MO, Fina AF, Hermosilla E, Martinez-Laguna D, Carbonell-Abella C, et al. The association between fracture and obesity is site-dependent: a population-based study in postmenopausal women. JBone MinerRes (2012) 27(2):294–300. doi: 10.1002/jbmr.1466
42. Armstrong ME, Spencer EA, Cairns BJ, Banks E, Pirie K, Green J, et al. Body mass index and physical activity in relation to the incidence of hip fracture in postmenopausal women. J Bone Miner Res (2011) 26(6):1330–8. doi: 10.1002/jbmr.315
43. Cipriani C, Pepe J, Colangelo L, Minisola S. Vitamin D and Secondary Hyperparathyroid States. Front Horm Res (2018) 50:138–48. doi: 10.1159/000486077
44. Vilaca T, Evans A, Gossiel F, Paggiosi M, Eastell R, Walsh JS. Fat, adipokines, bone structure and bone regulatory factors associations in obesity. Eur J Endocrinol (2022) 187(6):743–50. doi: 10.1530/EJE-22-0530
45. Karampela I, Sakelliou A, Vallianou N, Christodoulatos GS, Magkos F, Dalamaga M. Vitamin D and Obesity: Current Evidence and Controversies. Curr Obes Rep (2021) 10(2):162–80. doi: 10.1007/s13679-021-00433-1
46. Oei L, Zillikens MC, Dehghan A, Buitendijk GH, Castano-Betancourt MC, Estrada K, et al. High bone mineral density and fracture risk in type 2 diabetes as skeletal complications of inadequate glucose control: the Rotterdam Study. Diabetes Care (2013) 36(6):1619–28. doi: 10.2337/dc12-1188
47. Ma L, Oei L, Jiang L, Estrada K, Chen H, Wang Z, et al. Association between bone mineral density and type 2 diabetes mellitus: a meta-analysis of observational studies. Eur J Epidemiol (2012) 27(5):319–32. doi: 10.1007/s10654-012-9674-x
48. Liu B, Liu J, Pan J, Zhao C, Wang Z, Zhang Q. The association of diabetes status and bone mineral density among US adults: evidence from NHANES 2005-2018. BMC Endocr Disord (2023) 23(1):27. doi: 10.1186/s12902-023-01266-w
49. Tonks KT, White CP, Center JR, Samocha-Bonet D, Greenfield JR. Bone Turnover Is Suppressed in Insulin Resistance, Independent of Adiposity. J Clin Endocrinol Metab (2017) 102(4):1112–21. doi: 10.1210/jc.2016-3282
50. Lee RH, Sloane R, Pieper C, Lyles KW, Adler RA, Van Houtven C, et al. Glycemic Control and Insulin Treatment Alter Fracture Risk in Older Men With Type 2 Diabetes Mellitus. J Bone Miner Res (2019) 34(11):2045–51. doi: 10.1002/jbmr.3826
51. Vestergaard P. Diabetes and bone fracture: risk factors for old and young. Diabetologia (2014) 57(10):2007–8. doi: 10.1007/s00125-014-3338-1
52. Schwartz AV. Epidemiology of fractures in type 2 diabetes. Bone (2016) 82:2–8. doi: 10.1016/j.bone.2015.05.032
53. Bonds DE, Larson JC, Schwartz AV, Strotmeyer ES, Robbins J, Rodriguez BL, et al. Risk of fracture in women with type 2 diabetes: the Women's Health Initiative Observational Study. JClinEndocrinolMetab (2006) 91(9):3404–10. doi: 10.1210/jc.2006-0614
54. Purnamasari D, Puspitasari MD, Setiyohadi B, Nugroho P, Isbagio H. Low bone turnover in premenopausal women with type 2 diabetes mellitus as an early process of diabetes-associated bone alterations: a cross-sectional study. BMC Endocr Disord (2017) 17(1):72. doi: 10.1186/s12902-017-0224-0
55. Zeng H, Ge J, Xu W, Ma H, Chen L, Xia M, et al. Type 2 Diabetes Is Causally Associated With Reduced Serum Osteocalcin: A Genomewide Association and Mendelian Randomization Study. J Bone Miner Res (2021) 36(9):1694–707. doi: 10.1002/jbmr.4330
56. Wang Q, Zhang B, Xu Y, Xu H, Zhang N. The Relationship between Serum Osteocalcin Concentration and Glucose Metabolism in Patients with Type 2 Diabetes Mellitus. Int J Endocrinol (2013) 2013:842598. doi: 10.1155/2013/842598
57. Manavalan JS, Cremers S, Dempster DW, Zhou H, Dworakowski E, Kode A, et al. Circulating osteogenic precursor cells in type 2 diabetes mellitus. JClinEndocrinolMetab (2012) 97(9):3240–50. doi: 10.1210/jc.2012-1546
58. Colleluori G, Aguirre L, Dorin R, Robbins D, Blevins D, Barnouin Y, et al. Hypogonadal men with type 2 diabetes mellitus have smaller bone size and lower bone turnover. Bone (2017) 99:14–9. doi: 10.1016/j.bone.2017.03.039
59. Ballato E, Deepika F, Prado M, Russo V, Fuenmayor V, Bathina S, et al. Circulating osteogenic progenitors and osteoclast precursors are associated with long-term glycemic control, sex steroids, and visceral adipose tissue in men with type 2 diabetes mellitus. Front Endocrinol (Lausanne) (2022) 13:936159. doi: 10.3389/fendo.2022.936159
60. Vigevano F, Gregori G, Colleluori G, Chen R, Autemrongsawat V, Napoli N, et al. In Men With Obesity, T2DM Is Associated With Poor Trabecular Microarchitecture and Bone Strength and Low Bone Turnover. J Clin Endocrinol Metab (2021) 106(5):1362–76. doi: 10.1210/clinem/dgab061
61. Adami G, Gatti D, Rossini M, Orsolini G, Pollastri F, Bertoldo E, et al. Risk of fragility fractures in obesity and diabetes: a retrospective analysis on a nation-wide cohort. Osteoporos Int (2020) 31(11):2113–22. doi: 10.1007/s00198-020-05519-5
62. Tencerova M, Frost M, Figeac F, Nielsen TK, Ali D, Lauterlein JL, et al. Obesity-Associated Hypermetabolism and Accelerated Senescence of Bone Marrow Stromal Stem Cells Suggest a Potential Mechanism for Bone Fragility. Cell Rep (2019) 27(7):2050–62 e6. doi: 10.1016/j.celrep.2019.04.066
63. Mota de Sa P, Richard AJ, Hang H, Stephens JM. Transcriptional Regulation of Adipogenesis. Compr Physiol (2017) 7(2):635–74. doi: 10.1002/cphy.c160022
64. Sarjeant K, Stephens JM. Adipogenesis. Cold Spring Harb Perspect Biol (2012) 4(9):a008417. doi: 10.1101/cshperspect.a008417
65. Cereijo R, Giralt M, Villarroya F. Thermogenic brown and beige/brite adipogenesis in humans. Ann Med (2015) 47(2):169–77. doi: 10.3109/07853890.2014.952328
66. Madsen MS, Siersbaek R, Boergesen M, Nielsen R, Mandrup S. Peroxisome proliferator-activated receptor gamma and C/EBPalpha synergistically activate key metabolic adipocyte genes by assisted loading. Mol Cell Biol (2014) 34(6):939–54. doi: 10.1128/MCB.01344-13
67. Barak Y, Nelson MC, Ong ES, Jones YZ, Ruiz-Lozano P, Chien KR, et al. PPAR gamma is required for placental, cardiac, and adipose tissue development. Mol Cell (1999) 4(4):585–95. doi: 10.1016/S1097-2765(00)80209-9
68. Dammone G, Karaz S, Lukjanenko L, Winkler C, Sizzano F, Jacot G, et al. PPARgamma Controls Ectopic Adipogenesis and Cross-Talks with Myogenesis During Skeletal Muscle Regeneration. Int J Mol Sci (2018) 19(7):2044. doi: 10.3390/ijms19072044
69. Sun W, He T, Qin C, Qiu K, Zhang X, Luo Y, et al. A potential regulatory network underlying distinct fate commitment of myogenic and adipogenic cells in skeletal muscle. Sci Rep (2017) 7:44133. doi: 10.1038/srep44133
70. Cohen P, Levy JD, Zhang Y, Frontini A, Kolodin DP, Svensson KJ, et al. Ablation of PRDM16 and beige adipose causes metabolic dysfunction and a subcutaneous to visceral fat switch. Cell (2014) 156(1-2):304–16. doi: 10.1016/j.cell.2013.12.021
71. Seale P, Kajimura S, Yang W, Chin S, Rohas LM, Uldry M, et al. Transcriptional control of brown fat determination by PRDM16. Cell Metab (2007) 6(1):38–54. doi: 10.1016/j.cmet.2007.06.001
72. Chen S, Bastarrachea RA, Shen JS, Laviada-Nagel A, Rodriguez-Ayala E, Nava-Gonzalez EJ, et al. Ectopic BAT mUCP-1 overexpression in SKM by delivering a BMP7/PRDM16/PGC-1a gene cocktail or single PRMD16 using non-viral UTMD gene therapy. Gene Ther (2018) 25(7):497–509. doi: 10.1038/s41434-018-0036-5
73. Seale P, Bjork B, Yang W, Kajimura S, Chin S, Kuang S, et al. PRDM16 controls a brown fat/skeletal muscle switch. Nature (2008) 454(7207):961–7. doi: 10.1038/nature07182
74. Bredella MA, Gill CM, Rosen CJ, Klibanski A, Torriani M. Positive effects of brown adipose tissue on femoral bone structure. Bone (2014) 58:55–8. doi: 10.1016/j.bone.2013.10.007
75. Lee P, Brychta RJ, Collins MT, Linderman J, Smith S, Herscovitch P, et al. Cold-activated brown adipose tissue is an independent predictor of higher bone mineral density in women. Osteoporos Int (2013) 24(4):1513–8. doi: 10.1007/s00198-012-2110-y
76. Ponrartana S, Aggabao PC, Hu HH, Aldrovandi GM, Wren TA, Gilsanz V. Brown adipose tissue and its relationship to bone structure in pediatric patients. J Clin Endocrinol Metab (2012) 97(8):2693–8. doi: 10.1210/jc.2012-1589
77. Beekman KM, Veldhuis-Vlug AG, van der Veen A, den Heijer M, Maas M, Kerckhofs G, et al. The effect of PPARgamma inhibition on bone marrow adipose tissue and bone in C3H/HeJ mice. Am J Physiol Endocrinol Metab (2019) 316(1):E96–E105. doi: 10.1152/ajpendo.00265.2018
78. Weske S, Vaidya M, Reese A, von Wnuck Lipinski K, Keul P, Bayer JK, et al. Targeting sphingosine-1-phosphate lyase as an anabolic therapy for bone loss. Nat Med (2018) 24(5):667–78. doi: 10.1038/s41591-018-0005-y
79. Prestwich TC, Macdougald OA. Wnt/beta-catenin signaling in adipogenesis and metabolism. Curr Opin Cell Biol (2007) 19(6):612–7. doi: 10.1016/j.ceb.2007.09.014
80. Colaianni G, Brunetti G, Faienza MF, Colucci S, Grano M. Osteoporosis and obesity: Role of Wnt pathway in human and murine models. World J Orthop (2014) 5(3):242–6. doi: 10.5312/wjo.v5.i3.242
81. Nie X, Wei X, Ma H, Fan L, Chen WD. The complex role of Wnt ligands in type 2 diabetes mellitus and related complications. J Cell Mol Med (2021) 25(14):6479–95. doi: 10.1111/jcmm.16663
82. Martinez-Gil N, Ugartondo N, Grinberg D, Balcells S. Wnt Pathway Extracellular Components and Their Essential Roles in Bone Homeostasis. Genes (Basel) (2022) 13(1):138. doi: 10.3390/genes13010138
83. Benzler J, Andrews ZB, Pracht C, Stohr S, Shepherd PR, Grattan DR, et al. Hypothalamic WNT signalling is impaired during obesity and reinstated by leptin treatment in male mice. Endocrinology (2013) 154(12):4737–45. doi: 10.1210/en.2013-1746
84. Gassel LC, Schneider S, Banke IJ, Braun KF, Volkering C, Zeeb L, et al. Dysregulation of Wnt signaling in bone of type 2 diabetes mellitus and diabetic Charcot arthropathy. BMC Musculoskelet Disord (2022) 23(1):365. doi: 10.1186/s12891-022-05314-9
85. Galli C, Passeri G, Macaluso GM. Osteocytes and WNT: the mechanical control of bone formation. JDentRes (2010) 89(4):331–43. doi: 10.1177/0022034510363963
86. Kang S, Bennett CN, Gerin I, Rapp LA, Hankenson KD, Macdougald OA. Wnt signaling stimulates osteoblastogenesis of mesenchymal precursors by suppressing CCAAT/enhancer-binding protein alpha and peroxisome proliferator-activated receptor gamma. J Biol Chem (2007) 282(19):14515–24. doi: 10.1074/jbc.M700030200
87. Cosman F, Crittenden DB, Grauer A. Romosozumab Treatment in Postmenopausal Osteoporosis. N Engl J Med (2017) 376(4):396–7. doi: 10.1056/NEJMc1615367
88. Scott D, Seibel M, Cumming R, Naganathan V, Blyth F, Le Couteur DG, et al. Sarcopenic Obesity and Its Temporal Associations With Changes in Bone Mineral Density, Incident Falls, and Fractures in Older Men: The Concord Health and Ageing in Men Project. J Bone Miner Res (2017) 32(3):575–83. doi: 10.1002/jbmr.3016
89. Yeung SSY, Reijnierse EM, Pham VK, Trappenburg MC, Lim WK, Meskers CGM, et al. Sarcopenia and its association with falls and fractures in older adults: A systematic review and meta-analysis. J Cachexia Sarcopenia Muscle (2019) 10(3):485–500. doi: 10.1002/jcsm.12411
90. Verschueren S, Gielen E, O'Neill TW, Pye SR, Adams JE, Ward KA, et al. Sarcopenia and its relationship with bone mineral density in middle-aged and elderly European men. OsteoporosInt (2012) 24(1):87–98. doi: 10.1007/s00198-012-2057-z
91. Turcotte AF, Jean S, Morin SN, Mac-Way F, Gagnon C. Relationships between Obesity and Incidence of Fractures in a Middle-Aged Population: A Study from the CARTaGENE Cohort. JBMR Plus (2023) 7(5):e10730. doi: 10.1002/jbm4.10730
92. Turcotte AF, O'Connor S, Morin SN, Gibbs JC, Willie BM, Jean S, et al. Association between obesity and risk of fracture, bone mineral density and bone quality in adults: A systematic review and meta-analysis. PloS One (2021) 16(6):e0252487. doi: 10.1371/journal.pone.0252487
93. Johansson H, Kanis JA, Oden A, McCloskey E, Chapurlat RD, Christiansen C, et al. A meta-analysis of the association of fracture risk and body mass index in women. J Bone Miner Res (2014) 29(1):223–33. doi: 10.1002/jbmr.2017
94. Batsis JA, Mackenzie TA, Lopez-Jimenez F, Bartels SJ. Sarcopenia, sarcopenic obesity, and functional impairments in older adults: National Health and Nutrition Examination Surveys 1999-2004. NutrRes (2015) 35(12):1031–9. doi: 10.1016/j.nutres.2015.09.003
95. Marzetti E, Calvani R, Tosato M, Cesari M, Di Bari M, Cherubini A, et al. Physical activity and exercise as countermeasures to physical frailty and sarcopenia. Aging Clin Exp Res (2017) 29(1):35–42. doi: 10.1007/s40520-016-0705-4
96. Landi F, Marzetti E, Martone AM, Bernabei R, Onder G. Exercise as a remedy for sarcopenia. Curr Opin Clin Nutr Metab Care (2014) 17(1):25–31. doi: 10.1097/MCO.0000000000000018
97. Phu S, Boersma D, Duque G. Exercise and Sarcopenia. J Clin Densitom (2015) 18(4):488–92. doi: 10.1016/j.jocd.2015.04.011
98. Montero-Fernandez N, Serra-Rexach JA. Role of exercise on sarcopenia in the elderly. Eur J Phys Rehabil Med (2013) 49(1):131–43.
99. Keogh J, Henwood T, Climstein M. Exercise to reduce effects of sarcopenia. Aust Nurs J (2012) 19(9):39–40.
100. Styner M, Pagnotti GM, McGrath C, Wu X, Sen B, Uzer G, et al. Exercise Decreases Marrow Adipose Tissue Through ss-Oxidation in Obese Running Mice. J Bone Miner Res (2017) 32(8):1692–702. doi: 10.1002/jbmr.3159
101. Pahor M, Guralnik JM, Ambrosius WT, Blair S, Bonds DE, Church TS, et al. Effect of structured physical activity on prevention of major mobility disability in older adults: the LIFE study randomized clinical trial. JAMA (2014) 311(23):2387–96. doi: 10.1001/jama.2014.5616
102. Custodero C, Agosti P, Anton SD, Manini TM, Lozupone M, Panza F, et al. Effect of Physical Activity Intervention on Gait Speed by Frailty Condition: A Randomized Clinical Trial. J Am Med Dir Assoc (2023) 24(4):489–96. doi: 10.1016/j.jamda.2023.01.023
103. Villareal DT, Aguirre L, Gurney AB, Waters DL, Sinacore DR, Colombo E, et al. Aerobic or Resistance Exercise, or Both, in Dieting Obese Older Adults. N Engl J Med (2017) 376(20):1943–55. doi: 10.1056/NEJMoa1616338
104. Villareal DT, Chode S, Parimi N, Sinacore DR, Hilton T, Armamento-Villareal R, et al. Weight loss, exercise, or both and physical function in obese older adults. NEnglJMed (2011) 364(13):1218–29. doi: 10.1056/NEJMoa1008234
105. Batsis JA, Villareal DT. Sarcopenic obesity in older adults: aetiology, epidemiology and treatment strategies. Nat Rev Endocrinol (2018) 14(9):513–37. doi: 10.1038/s41574-018-0062-9
106. Wang M, Tan Y, Shi Y, Wang X, Liao Z, Wei P. Diabetes and Sarcopenic Obesity: Pathogenesis, Diagnosis, and Treatments. Front Endocrinol (Lausanne) (2020) 11:568. doi: 10.3389/fendo.2020.00568
107. Yoshimoto Y, Oishi Y. Mechanisms of skeletal muscle-tendon development and regeneration/healing as potential therapeutic targets. Pharmacol Ther (2023) 243:108357. doi: 10.1016/j.pharmthera.2023.108357
108. Lambert CP, Wright NR, Finck BN, Villareal DT. Exercise but not diet-induced weight loss decreases skeletal muscle inflammatory gene expression in frail obese elderly persons. JApplPhysiol (2008) 105(2):473–8. doi: 10.1152/japplphysiol.00006.2008
109. Verpoorten S, Sfyri P, Scully D, Mitchell R, Tzimou A, Mougios V, et al. Loss of CD36 protects against diet-induced obesity but results in impaired muscle stem cell function, delayed muscle regeneration and hepatic steatosis. Acta Physiol (Oxf) (2020) 228(3):e13395. doi: 10.1111/apha.13395
110. Kevorkova O, Martineau C, Martin-Falstrault L, Sanchez-Dardon J, Brissette L, Moreau R. Low-bone-mass phenotype of deficient mice for the cluster of differentiation 36 (CD36). PloS One (2013) 8(10):e77701. doi: 10.1371/journal.pone.0077701
111. Ballato E, Deepika FNU, Russo V, Fleires-Gutierrez A, Colleluori G, Fuenmayor V, et al. One-Year Mean A1c of > 7% is Associated with Poor Bone Microarchitecture and Strength in Men with Type 2 Diabetes Mellitus. Calcif Tissue Int (2022) 11(3):267–78. doi: 10.1007/s00223-022-00993-x
112. Hashimoto N, Kiyono T, Wada MR, Umeda R, Goto Y, Nonaka I, et al. Osteogenic properties of human myogenic progenitor cells. Mech Dev (2008) 125(3-4):257–69. doi: 10.1016/j.mod.2007.11.004
113. Qin X, Jiang Q, Komori H, Sakane C, Fukuyama R, Matsuo Y, et al. Runt-related transcription factor-2 (Runx2) is required for bone matrix protein gene expression in committed osteoblasts in mice. J Bone Miner Res (2021) 36(10):2081–95. doi: 10.1002/jbmr.4386
114. Moerman EJ, Teng K, Lipschitz DA, Lecka-Czernik B. Aging activates adipogenic and suppresses osteogenic programs in mesenchymal marrow stroma/stem cells: the role of PPAR-gamma2 transcription factor and TGF-beta/BMP signaling pathways. Aging Cell (2004) 3(6):379–89. doi: 10.1111/j.1474-9728.2004.00127.x
115. Xu R, Zeng Q, Xia C, Chen J, Wang P, Zhao S, et al. Fractions of Shen-Sui-Tong-Zhi Formula Enhance Osteogenesis Via Activation of beta-Catenin Signaling in Growth Plate Chondrocytes. Front Pharmacol (2021) 12:711004. doi: 10.3389/fphar.2021.711004
116. Murphy MM, Lawson JA, Mathew SJ, Hutcheson DA, Kardon G. Satellite cells, connective tissue fibroblasts and their interactions are crucial for muscle regeneration. Development (2011) 138(17):3625–37. doi: 10.1242/dev.064162
117. Sambasivan R, Yao R, Kissenpfennig A, Van Wittenberghe L, Paldi A, Gayraud-Morel B, et al. Pax7-expressing satellite cells are indispensable for adult skeletal muscle regeneration. Development (2011) 138(17):3647–56. doi: 10.1242/dev.067587
118. Kim MJ, Kim WS, Byun JE, Choi JH, Yoon SR, Choi I, et al. Inhibition of Osteoclastogenesis by Thioredoxin-Interacting Protein-Derived Peptide (TN13). J Clin Med (2019) 8(4):431. doi: 10.3390/jcm8040431
119. Luo J, Yang Z, Ma Y, Yue Z, Lin H, Qu G, et al. LGR4 is a receptor for RANKL and negatively regulates osteoclast differentiation and bone resorption. Nat Med (2016) 22(5):539–46. doi: 10.1038/nm.4076
120. Nagaoka M, Maeda T, Moriwaki S, Nomura A, Kato Y, Niida S, et al. Petunidin, a B-ring 5'-O-Methylated Derivative of Delphinidin, Stimulates Osteoblastogenesis and Reduces sRANKL-Induced Bone Loss. Int J Mol Sci (2019) 20(11):2795. doi: 10.3390/ijms20112795
121. Mochizuki N, Shimizu S, Nagasawa T, Tanaka H, Taniwaki M, Yokota J, et al. A novel gene, MEL1, mapped to 1p36.3 is highly homologous to the MDS1/EVI1 gene and is transcriptionally activated in t(1;3)(p36;q21)-positive leukemia cells. Blood (2000) 96(9):3209–14.
122. Hondares E, Rosell M, Diaz-Delfin J, Olmos Y, Monsalve M, Iglesias R, et al. Peroxisome proliferator-activated receptor alpha (PPARalpha) induces PPARgamma coactivator 1alpha (PGC-1alpha) gene expression and contributes to thermogenic activation of brown fat: involvement of PRDM16. J Biol Chem (2011) 286(50):43112–22. doi: 10.1074/jbc.M111.252775
123. He L, Tang M, Xiao T, Liu H, Liu W, Li G, et al. Obesity-Associated miR-199a/214 Cluster Inhibits Adipose Browning via PRDM16-PGC-1alpha Transcriptional Network. Diabetes (2018) 67(12):2585–600. doi: 10.2337/db18-0626
124. Pradhan RN, Zachara M, Deplancke B. A systems perspective on brown adipogenesis and metabolic activation. Obes Rev (2017) 18 Suppl 1:65–81. doi: 10.1111/obr.12512
125. Jiang N, Yang M, Han Y, Zhao H, Sun L. PRDM16 Regulating Adipocyte Transformation and Thermogenesis: A Promising Therapeutic Target for Obesity and Diabetes. Front Pharmacol (2022) 13:870250. doi: 10.3389/fphar.2022.870250
126. Seo YJ, Kim KJ, Choi J, Koh EJ, Lee BY. Spirulina maxima Extract Reduces Obesity through Suppression of Adipogenesis and Activation of Browning in 3T3-L1 Cells and High-Fat Diet-Induced Obese Mice. Nutrients (2018) 10(6):712. doi: 10.3390/nu10060712
127. Kaneda-Nakashima K, Igawa K, Suwanruengsri M, Naoyuki F, Ichikawa T, Funamoto T, et al. Role of Mel1/Prdm16 in bone differentiation and morphology. Exp Cell Res (2022) 410(2):112969. doi: 10.1016/j.yexcr.2021.112969
128. Minteer D, Marra KG, Rubin JP. Adipose-derived mesenchymal stem cells: biology and potential applications. Adv Biochem Eng Biotechnol (2013) 129:59–71. doi: 10.1007/10_2012_146
129. Song Y, Du H, Dai C, Zhang L, Li S, Hunter DJ, et al. Human adipose-derived mesenchymal stem cells for osteoarthritis: a pilot study with long-term follow-up and repeated injections. Regener Med (2018) 13(3):295–307. doi: 10.2217/rme-2017-0152
130. Louwen F, Ritter A, Kreis NN, Yuan J. Insight into the development of obesity: functional alterations of adipose-derived mesenchymal stem cells. Obes Rev (2018) 19(7):888–904. doi: 10.1111/obr.12679
131. Lee WS, Kim HJ, Kim KI, Kim GB, Jin W. Intra-Articular Injection of Autologous Adipose Tissue-Derived Mesenchymal Stem Cells for the Treatment of Knee Osteoarthritis: A Phase IIb, Randomized, Placebo-Controlled Clinical Trial. Stem Cells Transl Med (2019) 8(6):504–11. doi: 10.1002/sctm.18-0122
132. Ye X, Zhang P, Xue S, Xu Y, Tan J, Liu G. Adipose-derived stem cells alleviate osteoporosis by enhancing osteogenesis and inhibiting adipogenesis in a rabbit model. Cytotherapy (2014) 16(12):1643–55. doi: 10.1016/j.jcyt.2014.07.009
133. Arjmand B, Sarvari M, Alavi-Moghadam S, Payab M, Goodarzi P, Gilany K, et al. Prospect of Stem Cell Therapy and Regenerative Medicine in Osteoporosis. Front Endocrinol (Lausanne) (2020) 11:430. doi: 10.3389/fendo.2020.00430
134. Wang Y, Grainger DW. Developing siRNA therapies to address osteoporosis. Ther Delivery (2013) 4(10):1239–46. doi: 10.4155/tde.13.85
135. Cui Y, Guo Y, Kong L, Shi J, Liu P, Li R, et al. A bone-targeted engineered exosome platform delivering siRNA to treat osteoporosis. Bioact Mater (2022) 10:207–21. doi: 10.1016/j.bioactmat.2021.09.015
136. Liang C, Guo B, Wu H, Shao N, Li D, Liu J, et al. Aptamer-functionalized lipid nanoparticles targeting osteoblasts as a novel RNA interference-based bone anabolic strategy. Nat Med (2015) 21(3):288–94. doi: 10.1038/nm.3791
137. He F, Wen N, Xiao D, Yan J, Xiong H, Cai S, et al. Aptamer-Based Targeted Drug Delivery Systems: Current Potential and Challenges. Curr Med Chem (2020) 27(13):2189–219. doi: 10.2174/0929867325666181008142831
138. Chen Y, Wu X, Li J, Jiang Y, Xu K, Su J. Bone-Targeted Nanoparticle Drug Delivery System: An Emerging Strategy for Bone-Related Disease. Front Pharmacol (2022) 13:909408. doi: 10.3389/fphar.2022.909408
139. Mohamad NV, Soelaiman IN, Chin KY. A concise review of testosterone and bone health. Clin Interv Aging (2016) 11:1317–24. doi: 10.2147/CIA.S115472
140. Behre HM, Kliesch S, Leifke E, Link TM, Nieschlag E. Long-term effect of testosterone therapy on bone mineral density in hypogonadal men. JClinEndocrinolMetab (1997) 82(8):2386–90. doi: 10.1210/jcem.82.8.4163
141. Benito M, Vasilic B, Wehrli FW, Bunker B, Wald M, Gomberg B, et al. Effect of testosterone replacement on trabecular architecture in hypogonadal men. JBone MinerRes (2005) 20(10):1785–91. doi: 10.1359/JBMR.050606
142. Snyder PJ, Peachey H, Hannoush P, Berlin JA, Loh L, Holmes JH, et al. Effect of testosterone treatment on bone mineral density in men over 65 years of age. JClinEndocrinolMetab (1999) 84(6):1966–72. doi: 10.1210/jc.84.6.1966
143. Snyder PJ, Kopperdahl DL, Stephens-Shields AJ, Ellenberg SS, Cauley JA, Ensrud KE, et al. Effect of Testosterone Treatment on Volumetric Bone Density and Strength in Older Men With Low Testosterone: A Controlled Clinical Trial. JAMA InternMed (2017) 177(4):471–9. doi: 10.1001/jamainternmed.2016.9539
144. Ng Tang Fui M, Hoermann R, Bracken K, Handelsman DJ, Inder WJ, Stuckey BGA, et al. Effect of Testosterone Treatment on Bone Microarchitecture and Bone Mineral Density in Men: A 2-Year RCT. J Clin Endocrinol Metab (2021) 106(8):e3143–e58. doi: 10.1210/clinem/dgab149
145. Ng Tang Fui M, Hoermann R, Nolan B, Clarke M, Zajac JD, Grossmann M. Effect of testosterone treatment on bone remodelling markers and mineral density in obese dieting men in a randomized clinical trial. Sci Rep (2018) 8(1):9099. doi: 10.1038/s41598-018-27481-3
146. Colleluori G, Aguirre L, Napoli N, Qualls C, Villareal DT, Armamento-Villareal R. Testosterone Therapy Effects on Bone Mass and Turnover in Hypogonadal Men with Type 2 Diabetes. J Clin Endocrinol Metab (2021) 106(8):e3058–e68. doi: 10.1210/clinem/dgab181
147. Ghanim H, Dhindsa S, Green K, Abuaysheh S, Batra M, Makdissi A, et al. Increase in Osteocalcin Following Testosterone Therapy in Men With Type 2 Diabetes and Subnormal Free Testosterone. J Endocr Soc (2019) 3(8):1617–30. doi: 10.1210/js.2018-00426
148. Groti Antonic K. Impact of testosterone therapy on bone turnover markers in obese males with type 2 diabetes and functional hypogonadism. Aging male (2022) 25(1):269–77. doi: 10.1080/13685538.2022.2134338
149. Thu HE, Mohamed IN, Hussain Z, Shuid AN. Dihydrotestosterone, a robust promoter of osteoblastic proliferation and differentiation: understanding of time-mannered and dose-dependent control of bone forming cells. Iran J Basic Med Sci (2017) 20(8):894–904. doi: 10.22038/IJBMS.2017.9111
150. Huber DM, Bendixen AC, Pathrose P, Srivastava S, Dienger KM, Shevde NK, et al. Androgens suppress osteoclast formation induced by RANKL and macrophage-colony stimulating factor. Endocrinology (2001) 142(9):3800–8. doi: 10.1210/endo.142.9.8402
151. Phillip M, Maor G, Assa S, Silbergeld A, Segev Y. Testosterone stimulates growth of tibial epiphyseal growth plate and insulin-like growth factor-1 receptor abundance in hypophysectomized and castrated rats. Endocrine (2001) 16(1):1–6. doi: 10.1385/ENDO:16:1:01
152. Ren SG, Malozowski S, Sanchez P, Sweet DE, Loriaux DL, Cassorla F. Direct administration of testosterone increases rat tibial epiphyseal growth plate width. Acta Endocrinol (Copenh) (1989) 121(3):401–5. doi: 10.1530/acta.0.1210401
153. Chin KY, Ima-Nirwana S. The effects of orchidectomy and supraphysiological testosterone administration on trabecular bone structure and gene expression in rats. Aging male (2015) 18(1):60–6. doi: 10.3109/13685538.2014.954995
154. Sattler F, Bhasin S, He J, Chou CP, Castaneda-Sceppa C, Yarasheski K, et al. Testosterone threshold levels and lean tissue mass targets needed to enhance skeletal muscle strength and function: the HORMA trial. J Gerontol A Biol Sci Med Sci (2011) 66(1):122–9. doi: 10.1093/gerona/glq183
155. Singh R, Artaza JN, Taylor WE, Gonzalez-Cadavid NF, Bhasin S. Androgens stimulate myogenic differentiation and inhibit adipogenesis in C3H 10T1/2 pluripotent cells through an androgen receptor-mediated pathway. Endocrinology (2003) 144(11):5081–8. doi: 10.1210/en.2003-0741
156. Gao K, Wang X, Liu Q, Chen W, Wang G, Zhang D, et al. Evaluation of osteoblast differentiation and function when cultured on mesoporous bioactive glass adsorbed with testosterone. J Cell Biochem (2018) 119(7):5222–32. doi: 10.1002/jcb.26566
157. Deepika F, Ballato E, Colleluori G, Aguirre L, Chen R, Qualls C, et al. Baseline Testosterone Predicts Body Composition and Metabolic Response to Testosterone Therapy. Front Endocrinol (Lausanne) (2022) 13:915309. doi: 10.3389/fendo.2022.915309
158. Aguirre LE, Colleluori G, Robbins D, Dorin R, Shah VO, Chen R, et al. Bone and body composition response to testosterone therapy vary according to polymorphisms in the CYP19A1 gene. Endocrine (2019) 65(3):692–706. doi: 10.1007/s12020-019-02008-6
Keywords: obesity, diabetes, adipogenesis, myogenesis, osteogenesis
Citation: Bathina S and Armamento-Villareal R (2023) The complex pathophysiology of bone fragility in obesity and type 2 diabetes mellitus: therapeutic targets to promote osteogenesis. Front. Endocrinol. 14:1168687. doi: 10.3389/fendo.2023.1168687
Received: 17 February 2023; Accepted: 05 July 2023;
Published: 20 July 2023.
Edited by:
Manuel Naves-Diaz, Hospital Universitario Central de Asturias (ISPA), SpainReviewed by:
Maya Styner, University of North Carolina at Chapel Hill, United StatesCopyright © 2023 Bathina and Armamento-Villareal. This is an open-access article distributed under the terms of the Creative Commons Attribution License (CC BY). The use, distribution or reproduction in other forums is permitted, provided the original author(s) and the copyright owner(s) are credited and that the original publication in this journal is cited, in accordance with accepted academic practice. No use, distribution or reproduction is permitted which does not comply with these terms.
*Correspondence: Siresha Bathina, c2lyZXNoYS5iYXRoaW5hQGJjbS5lZHU=