- 1School of Exercise and Health, Shanghai University of Sport, Shanghai, China
- 2School of Exercise and Health, Guangzhou Sport University, Guangzhou, China
- 3College of Sports and Health, Shandong Sport University, Jinan, China
Articular cartilage degeneration has been proved to cause a variety of joint diseases, among which osteoarthritis is the most typical. Osteoarthritis is characterized by articular cartilage degeneration and persistent pain, which affects the quality of life of patients as well as brings a heavy burden to society. The occurrence and development of osteoarthritis is related to the disorder of the subchondral bone microenvironment. Appropriate exercise can improve the subchondral bone microenvironment, thus playing an essential role in preventing and treating osteoarthritis. However, the exact mechanism whereby exercise improves the subchondral bone microenvironment remains unclear. There is biomechanical interaction as well as biochemical crosstalk between bone and cartilage. And the crosstalk between bone and cartilage is the key to bone-cartilage homeostasis maintenance. From the perspective of biomechanical and biochemical crosstalk between bone and cartilage, this paper reviews the effects of exercise-mediated bone-cartilage crosstalk on the subchondral bone microenvironment, aiming to provide a theoretical basis for the prevention and treatment of degenerative bone diseases.
1 Introduction
Osteoarthritis (OA) may be influenced by genetic, environmental, metabolic, and biochemical factors. However, the majority of the time, cartilage wear brought on by aberrant mechanical forces in the joint results in OA (1). OA patients frequently have joint dysfunction due to pain and the deterioration of their joint cartilage, which has a negative impact on their quality of life. Currently, there are both surgical and non-surgical options for treating OA. The main surgical treatments include arthroplasty, and non-surgical treatments include medication and exercise therapy. Exercise therapy is crucial to non-surgical OA treatment since it is efficient, practical, and affordable. It is also adjustable, requires little location or corresponding equipment, and has no side effects when used consistently over time (1). Improving osteoarthritis treatment options is important in relieving symptoms, improving patients’ quality of life, and maximizing social and financial advantages. Currently, numerous studies have confirmed that exercise can prevent and treat osteoarthritis (2–4). A proper amount of aerobic exercise can slow the progression of OA and lessen the patient’s symptoms (5). On the contrary, overtraining can lead to mechanical overloading of weight-bearing joints, which induces degeneration of articular cartilage and eventually OA lesions (6, 7). When considered collectively, the existing research on how exercise affects osteoarthritis suggests that any favorable or unfavorable changes depend on mechanical stress on the subchondral bone transition. This review investigates the role mechanical stress stimulation plays in transmitting biochemical and biomechanical signals between these cells or tissues and considers whether this role might offer the possibility of therapeutic interventions to halt or slow disease progression.
2 The subchondral bone microenvironment in osteoarthritis
2.1 Structural characteristics of the subchondral bone microenvironment in osteoarthritis
Its pathological changes are characterized by focal destruction of articular cartilage within the synovial joint, accompanied by subchondral bone sclerosis and bone redundancy (8). Studies have shown that (9) osteoarthritis is influenced by various factors such as age, genetics, obesity, hormones, and trauma. If these risk factors are not taken seriously, patients may experience joint pain, dysfunction. As the disease progresses, it can potentially cause disability and some psychiatric or psychological difficulties. OA is now the leading cause of pain, limited range of motion, and loss of joint motion in older adults (10), which seriously affects the health of life and quality of life.
Additionally, OA as a widespread debilitating disease will place a significant financial burden on patients and society due to global aging, obesity, and increased joint injury (11). Normal wear and tear, abnormal mechanical loading, injury, and aging are common causes of damage to articular cartilage as well as subchondral bone, synovial tissue, and ligaments, which may alter the molecular composition and organization in the extracellular matrix. Under stimulation, injured chondrocytes produce matrix metalloproteinases (MMP-1, MMP-3, and MMP-13) and ADAMTS (ADAMTS-4 and ADAMTS-5). They lead to a decrease in the levels of proteoglycans, aggregated glycans, and type II collagen in the cartilage matrix by inhibiting the synthesis of critical components of the extracellular matrix, ultimately leading to cartilage degeneration (12). According to studies, the subchondral bone mineral density (BMD), trabecular volume fraction (BV/TV), trabecular number (Tb.N), and trabecular thickness (Tb.Th) of the tibial plateau are all positively connected with the severity of osteoarthritis, which was based on cartilage defects and thinning as well as histological score (13), Kellgren-Lawrenc e (K-L) grading (14), and cartilage defects.
Subchondral bone typically refers to the epiphyseal bone area located immediately below the calcified cartilage. And some scholars contend that this concept should be extended to calcified structures distal to the tidal line of articular cartilage, and calcified cartilage should be classified as subchondral bone (15, 16). Anatomically, subchondral bone structures can be further classified as either subchondral bone plates or subchondral bone trabeculae (17). A distinctive area at the bone interface between the articular cartilage and the long bones of the joint consists of articular cartilage underlain by calcified cartilage located within the subchondral bone plate, which in osteoarthritis has marked progressive destructive changes. Firstly, the subchondral bone plate is a thin lamellar structure (17), similar to the cortical bone in other skeletal areas. Moreover, the subchondral plate is also able to connect the articular cartilage directly to the subchondral bone (18, 19), beneath which is the cancellous bone at the end of the long bones, with internal pores that serve as a direct connection between the articular cartilage and the subchondral trabeculae. The density and distribution of these pores are primarily influenced by the degree of osteochondral bone aging and the magnitude of compression forces transmitted within and between joints via the osteochondral bone (20). These channels are preferentially concentrated in high stress areas within the joint, and as the thickness of the subchondral bone plate changes, the shape and diameter of the channels appear to change accordingly: in areas with thicker subchondral bone plates, the channels are narrower and form a mesh, while areas with thinner subchondral bone plates are wider (20).
Compared to the subchondral bone plate, the subchondral trabeculae contain blood vessels, sensory nerves, and bone marrow with lax porosity and more active metabolic activity (21). The trabeculae of the subchondral bone play a clear role in joints without lesions by acting as essential supports and shock absorbers. At the same time, it can promote the cartilage’s metabolic processes and offer essential nutritional support, all of which play a crucial role (15). The knee joint is distinct from the bones and joints of the extremities in that it is covered in cartilage and subchondral bone. The cartilage of the femoral condyles of the knee joint is rich in blood vessels and nerves, and small trabecular branches enter the calcified cartilage layer. The subchondral bone and the articular cartilage are closely linked. When correctly united, the subchondral bone and articular cartilage create the osteochondral junction. This unique functional unit is capable of preserving and stabilizing the homeostasis of the intra-articular environment (22). In fact, numerous potential functions for components like subchondral bone and calcified cartilage in the development of osteoarthritis exist. The majority of current clinical imaging studies, however, are based on calcified subchondral tissue, and current imaging techniques are unable to distinguish anatomically between subchondral bone and calcified cartilage. Additionally, the absence of distinct anatomical boundaries between the various regions of subchondral bone makes it challenging to conduct in-depth research on the bone’s role.
2.2 Bone remodeling in osteoarthritis
2.2.1 The role of bone remodeling in osteoarthritis
In recent years, as research on osteoarthritis has intensified, people no longer view osteoarthritis as a condition primarily characterized by articular cartilage degeneration and intermittent joint stenosis but rather as a total joint disease affecting articular cartilage, subchondral bone, synovium, ligaments, meniscus, and periarticular muscles. If untreated, subchondral bone lesions that develop early can become osteoarthritis (23). According to animal studies, in early OA, subchondral bone resorption exhibits a transitory increase in subchondral bone resorption and a decrease in bone volume fraction (24, 25). Early in the onset of osteoarthritis, bone remodeling is enhanced due to an accelerated process that delays the completion of mineralization of osteoid, leading to a predominance of bone resorption. However, when the progression of arthritis intensifies, aberrant bone remodeling reduces the subchondral bone’s capacity to conduct stress, coupled with the altered biomechanical structure of the bone, leads to uneven deformation of the cartilage under stress and shear forces, producing cartilage damage such as cracks (23). Thus it can be seen that the dynamic equilibrium of bone remodeling is an essential factor in the development and progression of arthritic disease.
Bone remodeling proceeds closely to the pathological changes of arthritis in a process that includes four stages: activation, resorption, reverse, and formation. The clear boundary between articular cartilage and calcified cartilage is called the tideline, and the subchondral bone deep in the tideline is connected to the articular cartilage by calcified cartilage. In osteoarthritis, there is enhanced calcification of the deeper articular cartilage, leading to an upward shift of the tide line and subsequent thinning of the articular cartilage (26). The highly vascularized synovial membrane secretes synovial fluid, which feeds articular cartilage, while the deeper chondrocytes receive nutritional support from the subchondral bone (22). Subchondral bone sclerosis is another characteristic change in addition to cartilage loss as OA progresses. In the subchondral bone microarchitecture, this is manifested by a decrease in the number of trabeculae, an increase in thickness, a more dispersed arrangement, and an increase in the bone volume fraction (Bone volume/Tissuevolume, BV/TV) (27). Subchondral bone sclerosis is particularly prominent beneath areas of severe cartilage defects in advanced OA. The subchondral bone plate is considerably thickened, and the degree of cartilage defect is favorably connected with the subchondral bone lesion (13, 28, 29).
2.2.2 Important cytokines involved in bone remodeling in arthritis
Endochondral ossification is the process that supports longitudinal bone growth during skeletal maturation. In healthy individuals, this process begins when mesenchymal cells proliferate, differentiate into pre-chondroblasts, and further differentiate into chondroblasts. Chondroblasts embed into the cartilage matrix they secrete and further differentiate into chondrocytes, forming very early bone prototypes. Chondrocytes primarily produce matrix molecules, but they also secrete growth factors, including the receptor activator of nuclear factor (NF)-ĸB-ligand (RANKL) and vascular endothelial growth factor(VEGF), which promote vascular invasion and osteoclast recruitment (30). Periosteum around the prototype is differentiated from mesenchymal cells, and osteoblasts are beginning to form a layer that resembles bone (31). Mammalian bone tissue is formed during embryonic development by two distinct processes. Intramembranous bone formation produces many craniofacial bones directly from mesenchymal coalescence, while endochondral ossification is the primary process of mammalian bone formation, generating bone through the cartilaginous middle. The transformation of cartilage to bone is closely related to chondrocyte, osteoblast, and vascular differentiation.
During the progressive hypertrophy of chondrocytes, cells within the perichondrium differentiate into osteoblasts, and the perichondrium is further vascularized. Vascular invasion into hypertrophic chondrocytes is a critical step. Blood vessels spread osteoblast precursors and osteoclasts into the cartilage matrix, eroding the cartilage matrix while secreting type I collagen to form cancellous bone. Osteoblasts in the periosteum then secrete a highly calcified matrix that forms cortical bone (32). The process of endochondral bone formation is regulated by the interplay of various hormones, growth factors and signaling pathways. For example, parathyroid hormone-related peptide(PTHrP) (33–36), Indian hedgehog (IHH) (37–39), fibroblast growth factor (FGFs) (40–42), bone morphogenetic protein (BMP) (43, 44), WNTs signaling (45), Notch signaling (46, 47), etc. These factors and their associated signaling pathways interact to activate key transcription factors in osteoblast differentiation through various pathways, including SOX9 (48, 49), Runx2 (50, 51), OSX (52, 53), ATF4 (54, 55), etc., ultimately promoting the expression of a series of genes that coordinate and regulate endochondral osteogenesis.
In vitro experiments have shown that transplanting chondrocytes and subchondral bone fragments into bovine cartilage significantly improves survival compared to transplanting chondrocytes onto bovine cartilage alone (56). After co-culture of chondrocytes with osteoblasts from the subchondral osteosclerotic zone of humans, the gene expression of SOX9, COL2, parathyroid hormone-related peptide, and parathyroid hormone-related peptide receptor (PTH-R) in chondrocytes was significantly reduced. The gene expression of osteoblast-stimulating factor (OSF)-1 was elevated compared to those in osteoblasts derived from areas of subchondral osteosclerosis in humans (57). From the above studies, osteoblasts in different regions of the subchondral bone do enable differential expression of key factors in chondrocytes, more directly illustrating the interactive possibility of bone-cartilage crosstalk. In the osteoblast conditional knockout MMP13 animal model (58), osteoblast remodeling of the surrounding bone matrix is inhibited, resulting in reduced cartilage matrix proteoglycan content, reduced type II collagen, proteoglycan, and MMP13 production by chondrocytes, and increased incidence of cartilage lesions. Osteoblasts’ synthesis of MMP13 has an impact on the homeostasis of cartilage, proving that osteochondral interactions exist.
3 The biomechanical basis of exercise regulating bone-cartilage crosstalk in subchondral bone
3.1 Physiological structure
In the different stages of osteoarthritis development, prolonged inflammation leads to catabolism of bone and cartilage (59); meanwhile, hypoxia due to improper joint loading and pathological changes in the joint vasculature lead to damage bone and cartilage matrix (60, 61). And how osteoarthritis develops and worsens depends on the dynamic balance regulated by all these changes in the subchondral bone microenvironment. This dynamic balance is primarily governed by interactions between bone and cartilage cell types from a cellular and tissue perspective. There is now substantial evidence indicating that the development and progression of arthritis are accompanied by altered survival rates of osteoblasts, osteocytes in bone, as well as chondrocytes in cartilage. Degeneration of cartilage tissue is a hallmark feature of arthritis. Numerous studies have now shown that the entire joint tissue of the body is involved in the process of arthritis. Crosstalk between cartilage and subchondral bone is thought to be the primary characteristic of this process.
Physiological structure from an anatomical perspective, the articular cartilage overlies the subchondral bone and is in close contact. The conventional theory holds that the calcified layer of cartilage immediately above the subchondral plate and the subchondral plate act as an impenetrable barrier, which means it is impossible to achieve bone-cartilage crosstalk in structure. Yet a large body of evidence suggests that these tissues can communicate. Duncan (62) et al. described numerous small holes in the subchondral plate located below the area covered by the meniscus, some of which appear to penetrate the subchondral plate and connect to the bone marrow cavity. Later, to measure the solute transport in calcified cartilage in real time, Jun Pan et al. (63) created an imaging technique based on fluorescence loss due to photobleaching (FLIP) in 2009. They discovered that sodium fluorescein could penetrate from subchondral bone into calcified cartilage. These findings suggest that there may be direct signaling between subchondral bone and articular cartilage, which form functional units with mechanical and biochemical interactions that may play a role in the maintenance and degeneration of the joint (63). This not only confirms the findings of Duncan et al., but also aligns with the 2006 discovery that human cartilage osseointegration is more intricate than previously thought: uncalcified cartilage often penetrates calcified cartilage and reaches into bone and bone marrow interstices (64). The results above are sufficient to indicate that there might be a molecular diffusion channel connecting the two spacers, and this idea has been empirically supported. On the other hand, novel OA therapeutic modalities based on bone-cartilage crosstalk between subchondral bone and cartilage have emerged. Bisphosphonates (BPs) are used as efficient bone-targeting agents to inhibit osteoclast activity, and biomaterials coupled to BPs are also used to carry other active molecules for basic bone-related therapies (65). BPs-modified nanoapatite (NP-BP) enables the targeting of subchondral bone by responding to lower pH in the microenvironment (65), and this system overcomes the deficiency of passive defense against bone resorption by BPs. It can effectively inhibit osteoclastic bone resorption. They showed that the injectable NP-BP system could inhibit abnormal subchondral bone remodeling, abnormal angiogenesis and excessive upward invasion of subchondral bone into calcified cartilage, thereby attenuating cartilage degeneration by inhibiting overactive osteoclast activity in a rat model of osteoarthritis (65). It is believed that the injectable NP-BP system has potential applications in osteoarthritis and other osteoarticular diseases. In addition, targeting crystalline mineral loss and reducing collagen mineralization in subchondral bone may also be potential targets for OA (66). Due to the natural barrier of articular cartilage and low blood circulation in the subchondral bone, there are tremendous difficulties in targeting subchondral bone for treatment options. Although research targeting subchondral bone is limited, the future development of subchondral bone-targeting biomaterials based on surface modification and innovative structures will be a new direction for OA treatment (67).
3.2 The correlation between osteoporosis and osteoarthritis is the embodiment of bone-cartilage crosstalk
Based on the above structural basis, it is evident that a strong linkage exists between osteogenesis and cartilage degeneration during the progression of osteoarthritis. The most typical manifestation is the correlation between osteoporosis and osteoarthritis. Osteoporosis is an age-related systemic metabolic disease with reduced bone mass and bone density as the main pathological changes and is most common in postmenopausal women. Knee OA is also a common and prevalent disease in postmenopausal women, and the prevalence of Knee OA is climbing as the aging of the Chinese population becomes more and more serious (11). OA and osteoporosis (OP) are two different diseases, and multiple factors can influence the development of both. According to existing studies, age, gender, genetics, chronic inflammation, endocrine, and metabolism are considered common risk factors for both diseases, while body mass index (BMI), BMD, and mechanical loading of joints may play different roles in the development of both diseases (68). Although several studies have been conducted on bone density levels in the lumbar spine and hip of patients with osteoarthritis and concluded an association between osteoarthritis and osteoporosis, there is still much controversy about the relationship between the two diseases and their mutual effects. We believe that changes in bone mass during osteoporosis correlate with osteoarthritis as a macroscopic manifestation of cartilage-to-bone crosstalk. Bone mass can laterally reflect the level of bone strength, and BMD tests can show the changes in bone mass more accurately, and there are early studies related to BMD in both OA and OP in pathological states. Nevitt et al. (69) conducted a study on the relationship between hip osteoarthritis and bone density using radiographic imaging. Through linear regression analysis, they discovered that patients with moderate to severe OA had higher bone density in the proximal femur, spine, and extremities compared to those with no or mild OA features. The authors attributed this phenomenon to secondary bone remodeling caused by hip OA in the proximal femur. Jan Dequeker (70) speculated that OA and OP might be linked through bone mass and that patients with OA may delay or hinder the onset of OP because they exhibit higher bone mass. Zhang Y et al. (71, 72) found that high BMD levels delayed the progression of knee OA but did not prevent its occurrence, and the protection of the knee joint by high BMD may be mainly related to the protection of the joint space. Some scholars even discovered that pain in KOA patients might be related to bone loss (73). The aforementioned research is adequate to demonstrate the link between osteoporosis and osteoarthritis.
3.3 Exercise enhances the mechanical crosstalk between bone and cartilage in the subchondral bone microenvironment
Regular exercise is one of the most effective measures to prevent osteoporosis (74), and high-intensity progressive resistance and impact training has the extraordinary ability to improve bone mass and function in older men and women and thus to reduce fracture risk (75–77). Mechanical signaling helps prevent bone loss and reduce the risk of fracture, even if it does not stimulate an increase in bone mass (78). Running is a common form of mechanically loaded exercise, and low-intensity running maintains cartilage homeostasis (79). The skeletal system serves as the mechanical skeleton of the entire body, and increased mechanical loading can treat postmenopausal osteoporosis (11). And the decrease in knee joint loading results in a significant decrease in subchondral bone mass and a reduction in articular cartilage layer thickness in mice, suggesting that appropriate joint loading plays a vital role in maintaining the homeostasis of articular cartilage and subchondral bone. Therefore, we believe that exercise-induced mechanical loading also acts as a catalyst in addition to strengthening the bond of bone-cartilage crosstalk.
We believe that describing the impact of exercise on subchondral bone-cartilage crosstalk in osteoarthritis should consider both the intensity of exercise and the course of osteoarthritis development in a thorough manner. In the study of exercise for osteoarthritis, treadmill running is widely used as an exercise intervention for modeling mice with osteoarthritis. The mechanical overload generated by running is an important factor in the development of osteoarthritis. The different effects of different intensities of treadmill running exercise on osteoarthritis in murine can be understood as a response of the cartilage and subchondral bone microenvironment to mechanical stresses of different loading intensities. In animal experiments, the actual exercise intensity can be controlled by measuring the maximum oxygen uptake to develop the exercise protocol for the experiment (80). Moderate-intensity exercise corresponds to approximately 50% to 70% VO2 max, while treadmill running exercise intensities greater than 70% VO2 max are considered high-intensity exercise (81). Of course, in the case of rats, the modeling of osteoarthritis is not limited to the treadmill running alone. However, it can be combined with other modalities as well. Coyle et al. (79) attempted to create an OA rat model using the anterior cruciate ligament transectionthree weeks after surgery plus treadmill running exercise. The histological Mankin score and anabolic indexes of articular cartilage in the experimental group showed successful OA modeling. Postoperative knee surgery in rats leads to increased articular cartilage wear, and subsequent running exercise aggravates articular cartilage damage, which can advance the OA lesion process, thereby shortening the experimental modeling period or causing lesions in the middle and late stages of OA.
Ni et al. (82) induced OA in rats using high-intensity treadmill running exercise at >70% VO2 max and joint braking simultaneously. The findings revealed that both the high-intensity runner exercise group and the joint braking group had significantly lower levels of extracellular matrix anabolic-related proteins like joint proteoglycan and type II collagen and that the articular cartilage damage in the braking group was more severe than in the high-intensity exercise group. According to Yao Z. et al., strenuous running inhibits PDGF-AA synthesis in the subchondral bone. It leads to the downregulation of PDGF/Akt signaling in articular cartilage, resulting in cartilage degeneration (83). Excessive mechanical stress can induce mitochondrial DNA damage and mitochondrial dysfunction by modulating the p53R2/p53AIP1 protein to activate the mitochondrial apoptotic pathway, which ultimately leads to chondrocyte apoptosis (84). This implies that high-intensity running exercise caused early OA while joint braking exercise caused mid to late-stage OA to form. Compared to high-intensity treadmill running exercises, low-medium-intensity treadmill running exercises are mostly used in experimental animal studies on treating osteoarthritis in murine. Regular exercise training for four weeks alleviates cartilage degeneration in model rats with KOA (85). It has been observed that mild and short-term treadmill walking can safeguard chondrocytes in a rat model by preventing an upsurge in osteocyte mortality (86). Treadmill training over four weeks alleviates subchondral bone loss and remodeling and reprograms the cartilage-subchondral unit (87). The expression of matrix metalloproteinases (MMPs) in articular cartilage depends on the intensity of mechanical stress stimulation. Moderate-intensity exercise inhibits the expression of MMPs, thus reducing apoptosis and extracellular matrix degradation in particular chondrocytes (88). Mechanism studies in exercise treatment of murine osteoarthritis focus mostly on the regulation of key factors and signaling pathways by exercise. Aerobic exercise reduced expression in IL-1β, cystein-3 and MMP-13 and prevented KOA-induced cartilage degeneration in model rats (89). Running machine and wheeled exercise reduced the levels of IL-1β, IL-6 and TNF-α and modulated JNK/NF-κB signaling to prevent inflammation in a model rat with KOA (90). Moderate physical exercise prevents B-type synovial cell dysfunction and delays disease progression in rats with early osteoarthritis (91).In addition, the selection of exercise periods for the treatment for rodent osteoarthritis is also very important.An animal study showed that early intervention with swimming was more effective than delayed intervention in the early stages of cartilage injury when post-traumatic osteoarthritis had already developed (92). Further evidence supporting the role of exercise in regulating bone-cartilage crosstalk in osteoarthritis, involving significant factors and signaling pathways, will be presented subsequently.
3.4 The role of exercise therapy in arthritis rehabilitation
As a non-pharmacological therapy, exercise therapy is currently employed extensively in treating osteoarthritis. One of the prerequisites for preserving the stability of the skeletal system is mechanical stress stimulation, which is produced on the skeleton during movement of the body by gravity, ground reaction forces, and skeletal muscle contraction (93). Scientific exercise interventions have been shown to improve proprioception, increase periarticular muscle strength, and restore the biomechanical balance of periarticular tissues (94). Exercise therapy is an efficient way to treat knee pain and dysfunction in KOA patients, and it is a treatment that is both safe without side effects and easily accepted by patients (95). In recent years, various human studies have investigated the efficacy of different exercise interventions on osteoarthritis, such as plyometric training, aquatic exercise, aerobic exercise, neuromuscular motor control training, balance training, proprioceptive training, and traditional exercise. And the mechanism of exercise for osteoarthritis is discussed from the biomechanical perspective (12, 94, 96, 97).
As a common form of mechanically loaded exercise, and the intensity of the exercise plays a crucial role in the development of osteoarthritis, treadmill running is often used for experimental animal modeling. The growth and maintenance of knee ligaments, bones, and cartilage are all encouraged by adequate exercise intensity (98), which also improves muscle strength around the joint to lessen the strain on the joint suitably. However, vigorous platform running may put a more mechanical strain on the knee, which could lead to articular cartilage degradation (7). Low-intensity mechanical stress inhibits the expression of inflammatory genes in articular cartilage and decreases joint pain, according to related ex vivo investigations (99). On the other hand, excessive mechanical stress is known to encourage the development of inflammatory factors in articular cartilage and can damage the cartilage matrix. Therefore, moderate exercise can effectively relieve joint pain by enhancing the biomechanical relationship around the joints, controlling the expression of inflammatory factors in articular cartilage, and significantly impacting the treatment and prevention of osteoarthritis (Figure 1).
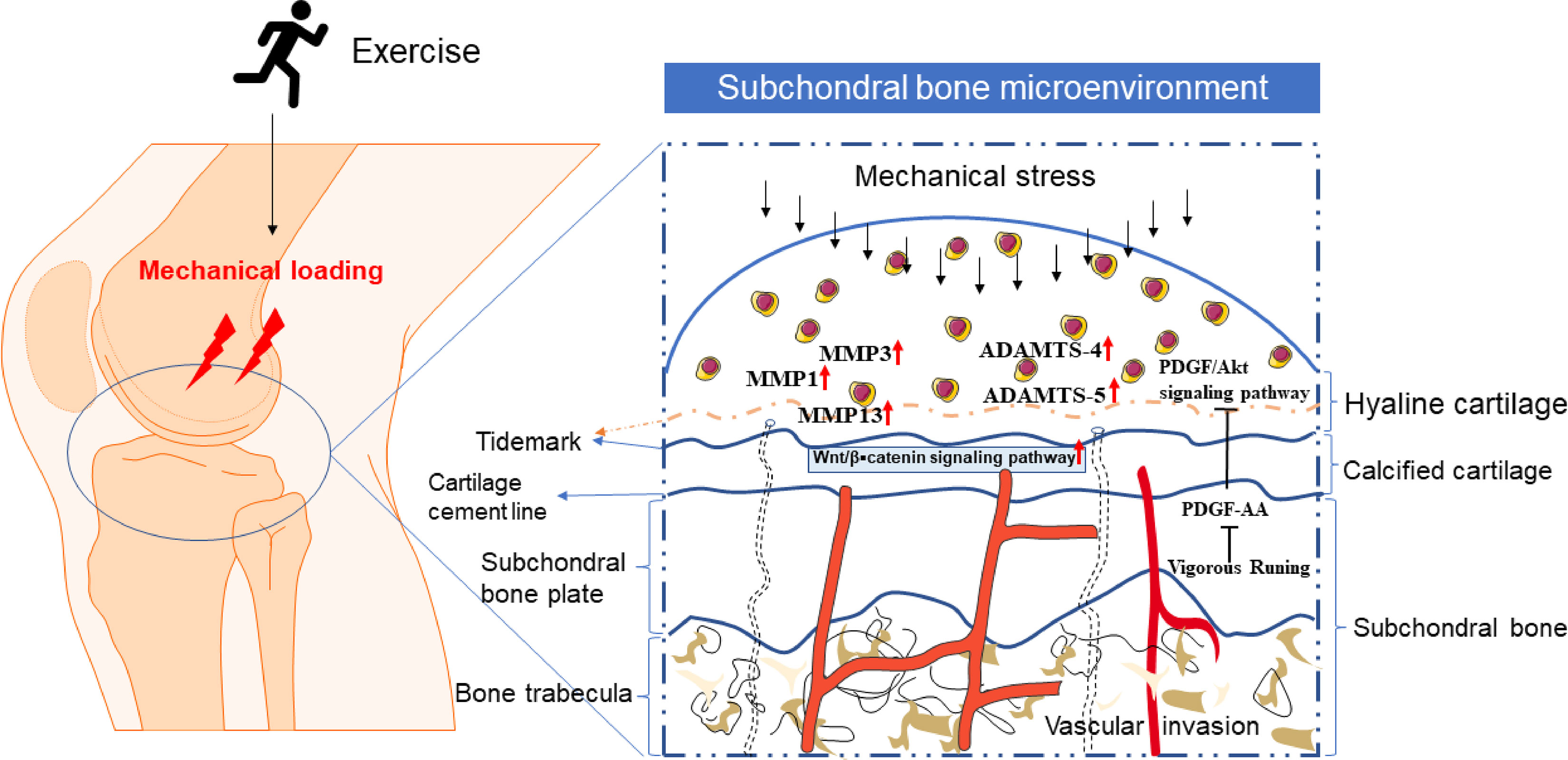
Figure 1 The microenvironment of cartilage and subchondral bone under exercise or mechanical stress. Mechanical loading caused by exercise or other factors can penetrate the scope of articular cartilage. Proper exercise will not harm normal articular cartilage. However, when the lesion of normal cartilage or arthritis aggravates, the tidal line under hyaline cartilage moves upward (the tidal line represented by the dotted line), the calcification of articular cartilage increases, and the articular cartilage degenerates. The pathway of bone-cartilage crosstalk in subchondral bone, such as vascular invasion and holes that can pass through the subchondral plate into the bone marrow, may be the basis and ways for exercise to regulate bone-cartilage crosstalk.
4 A molecular mechanism for the exercise regulation of bone-cartilage crosstalk
The observations we have summarized above indicate the likelihood of direct signaling transmission between subchondral bone and articular cartilage, suggesting that cartilage and bone form a functional unit mechanically and biochemically, which may play an essential role in intra-articular homeostasis and disease. A number of cytokines and growth factors may have a role in the high-frequency alteration of subchondral bone during the course of arthritis. According to the review by Lajeunesse in 2004 (100), these cytokines and growth factors can penetrate the cartilage that covers the surface of subchondral bone and control chondrocyte biology, thus establishing a positive feedback loop between cartilage and subchondral bone.
4.1 Signaling pathways involved in the exercise-regulated bone-cartilage crosstalk
Many studies have shown the presence of abnormal subchondral bone remodeling in the progression of osteoarthritis (101, 102). This remodeling process is dominated by chondrocytes, osteoblasts, osteocytes, and mesenchymal stem cells, and bone remodeling in the course of OA includes bone formation and bone resorption. It is now generally accepted that subchondral bone exhibits bone resorption in the early stages of OA and excessive bone sclerosis in the late stages of OA. A large number of changes occur in the behavior of cartilage and bone cells, which leads to changes in the expression of many molecules that may have both an autocrine role in their producing tissues and may contribute to altering the dialogue between bone and cartilage, as proteins and other larger molecules are able to be transported between these tissues. In the case of chondrocytes (103),osteoblasts and osteocytes (104, 105) both have good mechanosensing capabilities, especially in the overload state. Mechanical stress stimulation plays an important role in cartilage-bone crosstalk Mechanical stress stimulation is able to facilitate information exchange between tissues or cells to some extent. Many signaling pathways, including Wnt/β–catenin, RANK/RANKL/OPG, and ROS involved in both chondrogenesis and osteogenesis, are also sensitive to mechanical stress stimulation (Figure 2).
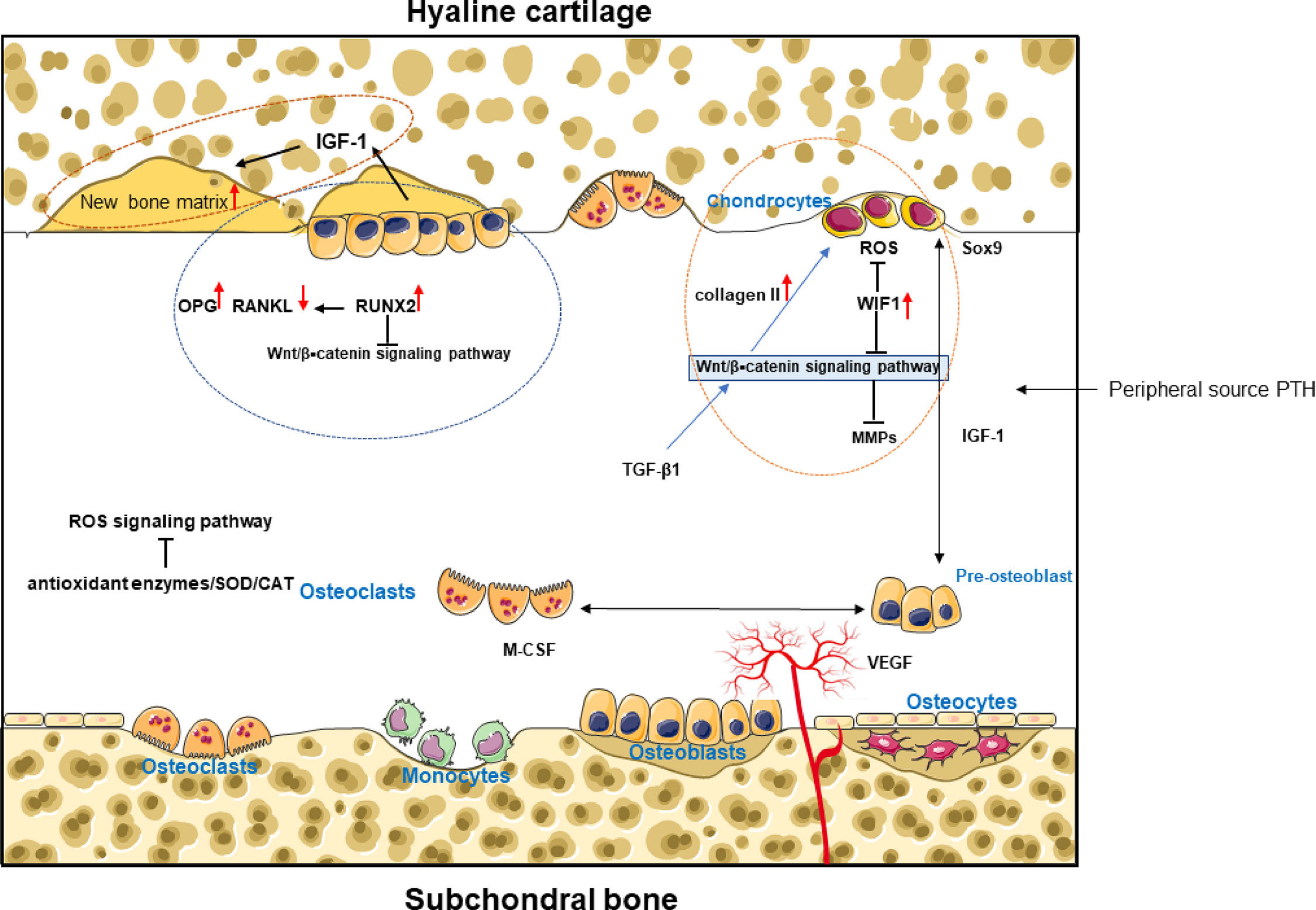
Figure 2 Cytokines and growth factors regulate chondrocyte biology and establish a positive feedback loop via biochemical crosstalk between cartilage and subchondral bone. In the cartilage and subchondral bone microenvironment, osteoblast-dominated bone formation and osteoclast-dominated bone resorption affect cartilage formation or cartilage degeneration. (Figure 2) mainly shows the main signal pathways and cytokines of bone-cartilage crosstalk in the subchondral bone under exercise intervention.
The Wnt/β–catenin signaling pathway is a more typical representative. β-catenin is a multifunctional protein in the cytoplasm and a key molecule in the Wnt signaling pathway that regulates gene transcription (106). The Wnt/β-catenin signaling pathway is a key regulator of bone, cartilage, joint development, and homeostasis. It plays an important role in many biological processes, including the cohesion and differentiation of mesenchymal cells, maintenance of the phenotype of mature articular cartilage, and maturation of hypertrophic tissue during endochondral osteogenesis (107). This is the basis for the Wnt/β-catenin signaling pathway that maintains the cartilage and subchondral bone endoskeletal environment homeostasis.Most importantly, it plays an essential role in regulating the function of osteoblasts, osteoclasts, and osteocytes and influences skeletal development and homeostasis. Abnormal Wnt signaling can lead to diseases such as skeletal defects (108). Upregulation of Wnt inhibitory factor 1 (WIF1) would promote OA chondrocyte proliferation, inhibit apoptosis by eliminating ROS production, and reduce MMP secretion by blocking the Wnt/β-linked protein signaling pathway (109). Adequate mechanical loading promotes bone formation and increases SB thickness and trabecular volume fraction by activating Wnt/β-linked protein signaling. Related studies have shown that upregulation of β-linked protein and Wnt-3a was found in both the injury exercise-induced OA model group and the exercise-induced OA model group (110). Thus, β-linked protein and Wnt-3a may be involved in the pathogenesis Page 7of exercise-induced OA through the abnormal activation of the Wnt/β-linked protein pathway by frequent excessive stress during exercise.
The OPG/RANKL/RANK signaling pathway is an important signaling channel for the interaction between osteoblasts and osteoclasts. Among them, RANKL and OPG are considered to be key molecules in regulating bone reconstruction. Both factors are produced by osteoblasts/stromal cells as well as human chondrocytes, whereas the RANK receptor is only expressed in human osteoarthritic chondrocytes (111). Compared to normal cartilage, the RANKL/OPG ratio is increased in both human osteoarthritic cartilage and in mandibular osteoarthritic synovial cartilage from osteoarthritic rats (111, 112). Overall, the RANKL/OPG ratio increases and then decreases in OA (113), consistent with the pathological changes in OA where early bone resorption predominates and late bone formation predominates.The RANK/RANKL/OPG signaling pathway regulates subchondral bone remodeling through the Wnt/β-linked protein signaling pathway. On the one hand, the OPG/RANKL signaling pathway is involved in the secretion of β-linked proteins by chondrocytes (114). However, in osteoblasts,OPG expression is regulated by the Wnt/β-linked protein signaling pathway (115). On the other hand, the knockdown of Runx2, a key regulator of bone formation, induces high expression of RANKL and suppresses OPG expression. In contrast, overexpression of Runx2 inhibits the function of the typical Wnt/β-linked protein signaling pathway by depleting β-linked protein, resulting in reduced bone volume and volume (116). However, due to Runx2 overexpression, β-linked protein activation reverses the high bone resorption in mouse subchondral bone, which is closely associated with RANKL/OPG signaling (116).
Inflammatory factors are closely related to stress itself;inflammatory factor expression induces chondrocyte stress, and prolonged stress induces inflammatory factor secretion. During stress, a large amount of active ROS is produced, eventually leading to chondrocyte damage if the excess ROS is not cleared in time (117). Exercise itself is also a kind of stress, as oxygen consumption increases during exercise, and a large amount of ROS is produced to meet the body’s oxygen demand, at which time the activity of antioxidant enzymes is also enhanced to improve the body’s tolerance to oxidative stress and remove excess ROS. Generally, moderate aerobic exercise can significantly increase the activity of the antioxidant enzymes such as superoxide dismutase (SOD). Kaczor et al. (118) found that long-term low-intensity exercise increased adaptive cellular responses, slowed cellular damage caused by ROS, and inhibited apoptosis. We suggest that the Wnt/β-linked protein signaling pathway and OPG/RANKL/RANK signaling pathway are highly activated when articular cartilage is damaged. In contrast, appropriate exercise or mechanical stress stimulation inhibits the activation of these pathways and gradually stabilizes them by reducing inflammatory factors and chondrocyte stress.Low to moderate-intensity aerobic exercise can be an excellent way to intervene in damaged cartilage.
4.2 Cytokines involved in the exercise regulation of bone-cartilage crosstalk
IGF is one of the important growth factors that regulate bone formation. In 2005, Koch (119) et al.found that IGF can up-regulate the expression of early osteogenic genes in human bone marrow mesenchymal stem cells (hBMSCs), including type I collagen, alkaline phosphatase, and Runx2, and thus to promote the osteogenesis of hBMSCs. In osteoarthritis, osteoblasts of the subchondral bone can produce large amounts of different types of IGF-1, while production of IGF-1 binding protein is reduced compared to normal subchondral bone (120), so large amounts of free IGF-1 promote bone reconstruction and lead to the development of osteosclerosis, which simultaneously exacerbates cartilage matrix degradation. Studies have shown that 12 weeks of combined anaerobic and aerobic exercise improves IGF-1 levels as well as insulin resistance in older women and that exercise can affect IGF-1 levels (121). Matheny et al. (122) found increased locomotor performance, up-regulated IGF-1 mRNA expression in muscle, and increased muscle weight in the hind legs of adult liver IGF-1 conditional knockout mice after 16 weeks of ladder climbing endurance training. Currently, although there are fewer studies on exercise regulation of IGF-1 expression in relation to osteoarthritis, it is not difficult to make the hypothesis that exercise can be involved in regulating bone-cartilage crosstalk in subchondral bone through IGF-1 based on its important interaction in osteogenesis and osteoarthritis, and its sensitivity to exercise performance.
Transforming growth factor β1 (TGF-β1) is a cytokine that plays an important role in the induction of chondrogenesis (123). TGF-β1 expression is significantly higher in healthy cartilage than in OA cartilage (124). In the progression of chondrocyte phenotypic degeneration including senescence and dedifferentiation, downregulation of TGF-β1 indirectly induces disorders of chondrocyte metabolism (125). TGF-β1 promotes chondrocyte proliferation through β-linked protein signaling and maintains the chondrocyte phenotype by enhancing collagen II synthesis (125, 126), suggesting that TGF-β acts as a mediator for cartilage and subchondral bone (127). Zhen et al. (128) studied the rat OA model with anterior cruciate ligament ostomy. They found that TGF-β in subchondral bone activated responsively to altered mechanical loading. Increased TGF-β in subchondral bone increased the number of mesenchymal stem cells, osteoprogenitor cells, and osteoblasts, leading to abnormal bone reconstruction as well as angiogenesis. It is important evidence for bone-cartilage crosstalk under mechanical stress stimulation conditions. In addition, R. K. Zhang (129) et al. also conducted a related study on the response of TGF-β1 signaling pathway to mechanical overload in osteoblast and chondrocyte co-cultured cells, and the results showed that mechanical stress might be a trigger for TGF-β1 upregulation in osteoblasts.
Mechanical stress intervention in osteoclasts inhibits chondrocyte proliferation and can induce apoptosis in the osteoclast/chondrocyte co-culture system (129). Transgenic expression of active TGF-β1 in osteoblasts is sufficient to induce osteoarthritis. In contrast, direct inhibition of TGF-β1 activity in subchondral bone attenuates degeneration of articular cartilage (123), suggesting that exercise, or exercise-induced mechanical stress, plays an important role in the cartilage-osteoblast-broken bone-dominated subchondral bone transition and bone homeostasis. This further confirms the role of bone-cartilage crosstalk catalysis by mechanical stress in the subchondral bone microenvironment. Of course, this catalytic effect cannot be achieved without the participation of various cytokines, and here we only describe IGF and TGF-β1 in detail. Other factors playing messenger roles are Sox9, PTH, M-CSF, VEGF, etc. Among them, macrophage colony-stimulating factor (M-CSF), although indispensable in the proliferation and differentiation of osteoblasts as well as in the fusion of their cellular precursors, also regulates the resorptive activity of mature osteoblasts, and its dissemination in the cytoplasm simultaneously inhibits the apoptosis of mature osteoblasts (130), but it is not associated with a strong sensitivity to mechanical loading.
Sox9 plays a key role in the differentiation of mesenchymal cells into chondrocytes (131), and Sox9 can be found in abundance at the site of chondrogenesis, as well as when mesenchymal cells are concentrated prior to differentiation into chondrocytes. Invitro studies conducted on cultured chondroprogenitor cells have demonstrated that biomechanical stimulation significantly promotes the differentiation of chondroprogenitor cells and the growth of the extracellular matrix. Moreover, the Sox9 gene has been found to play a crucial role in this process, which suggests its importance in stress transmission and response (132). At present, there are many kinds of exercise interventions in animal studies related to exercise-mediated Sox9 treatment of osteoarthritis, for example, treadmill running, Combined therapies with exercise, ozone, and mesenchymal stem cells (133), vibration exercise with different frequencies (134).
Parathyroid hormone (PTH), the most important regulator of calcium and phosphorus metabolism in humans, increases reactively when serum calcium levels decrease. And its overproduction leads to the development of bone resorption, while low, intermittent doses act to promote increased bone mass (135). After stimulating the skeleton with dynamic loading, exercise results in a systemic rise in parathyroid hormone (PTH) (136). Both clinical and animal studies have found a transient release of PTH in nature by following a single exercise session (137–140). In addition to PTH released by the parathyroid glands, exercise causes local expression of PTH-related peptide (PTHrP) and PTH/PTHrP type 1 receptor (PPR) (141, 142), while exercise significantly increases PTHrP production by osteoblasts, this then causes PPR to get activated in an autocrine or paracrine way. Recent animal experiments have shown that the synthetic parathyroid hormone PTH (1–34) improves the structure of cartilage surfaces in OA and contributes to subchondral bone reconstruction (143). PTH signaling has a unique role in bone adaptation during exercise, which is mediated through the activation of PTH-related peptide type 1 receptor (PPR) along the osteoblast spectrum (144). In 2014, Yan et al. (145) found that PTH (1–34)-treated cartilage had increased type II collagen expression, decreased SOST expression, an increased OPG/RANKL ratio, and an increased amount of subchondral trabecular bone compared to controls. It was shown that PTH (1–34) has a role in preventing OA cartilage destruction and delaying subchondral trabecular bone degeneration. PTH and its associated receptors are sensitive to exercise load, and the involvement of PTH in the bone-cartilage crosstalk of subchondral bone has been well-documented in related studies. It is likely that PTH is a potentially critical factor in the exercise-promoted bone-cartilage crosstalk of subchondral bone. However, the specific mechanism by which exercise modulates the subchondral bone-cartilage crosstalk via PTH needs to be investigated in more depth.
5 Summary
The review summarizes the feasibility of exercise to promote bone-cartilage crosstalk in subchondral bone and the related molecular mechanisms. Excessive mechanical stimulation triggers cartilage lesions,and moderate exercise promotes cartilage repair.This suggests differences in the effects of different intensities of exercise on the bone-cartilage crosstalk of subchondral bone.Bone-cartilage crosstalk is the basis for maintaining subchondral bone transition and bone homeostasis. Exercise promotes the effect of bone-cartilage crosstalk mainly by regulating mechanosensitive cytokines and signaling pathways such as Wnt/β⁃catenin, RANK/RANKL/OPG, and ROS signaling pathways. However, many questions still need to be answered in more scientific and comprehensive related studies. For example, do relevant animal and in vitro studies provide valuable information on specific exercise protocols for exercise prevention and treatment of osteoarthritis in humans? Indeed, with the advancement of high-resolution tools such as MRI, we will be able to explain the positive modulatory effects produced by exercise more visually. At the same time, the creation of new responsive biomaterials targeting subchondral bone opens up more possibilities for the treatment of osteoarthritis patients. Thus, in the future, the combination of exercise and nascent therapeutic modalities is a new potential way whereby exercise prevents and treats osteoarthritis.
Author contributions
SZ: conceptualization, formal analysis, resources, writing-original draft, writing-review and editing. TL: writing-original draft, writing-review and editing. YF: data curation, formal analysis, and editing. KZ: data curation, writing-review and editing. JZ: writing-review and editing. XW: writing-review and editing.YY: funding acquisition, supervision, writing-original draft, writing-review and editing. LZ: funding acquisition, supervision, writing-review and editing, project administration. All authors contributed to the article and approved the submitted version.
Funding
This research was funded by the National Natural Science Foundation of China, grant number 81901430; Natural Science Foundation of Guangdong Province, grant number 2022A1515010379;Innovation Project (2021KTSCX055) from Department of Education of Guangdong Province, the Shanghai Frontiers Science Research Base of Exercise and Metabolic Health, and the Shanghai Key Laboratory for Human Athletic Ability Development and Support (Shanghai University of Sport) grant number 11DZ2261100.
Conflict of interest
The authors declare that the research was conducted in the absence of any commercial or financial relationships that could be construed as a potential conflict of interest.
Publisher’s note
All claims expressed in this article are solely those of the authors and do not necessarily represent those of their affiliated organizations, or those of the publisher, the editors and the reviewers. Any product that may be evaluated in this article, or claim that may be made by its manufacturer, is not guaranteed or endorsed by the publisher.
References
1. Peeler J, Ripat J. The effect of low-load exercise on joint pain, function, and activities of daily living in patients with knee osteoarthritis. Knee (2018) 25(1):135–45. doi: 10.1016/j.knee.2017.12.003
2. Aguiar GC, Do Nascimento MR, De Miranda AS, Rocha NP, Teixeira AL, Scalzo PL. Effects of an exercise therapy protocol on inflammatory markers, perception of pain, and physical performance in individuals with knee osteoarthritis. Rheumatol Int (2015) 35(3):525–31. doi: 10.1007/s00296-014-3148-2
3. Holsgaard-Larsen A, Clausen B, Sondergaard J, Christensen R, Andriacchi TP, Roos EM. The effect of instruction in analgesic use compared with neuromuscular exercise on knee-joint load in patients with knee osteoarthritis: a randomized, single-blind, controlled trial. Osteoarthritis Cartilage (2017) 25(4):470–80. doi: 10.1016/j.joca.2016.10.022
4. Fransen M, McConnell S, Harmer AR, van der Esch M, Simic M, Bennell KL. Exercise for osteoarthritis of the knee: a cochrane systematic review. Br J Sports Med (2015) 49(24):1554–7. doi: 10.1136/bjsports-2015-095424
5. Cheung C, Wyman JF, Bronas U, McCarthy T, Rudser K, Mathiason MA. Managing knee osteoarthritis with yoga or aerobic/strengthening exercise programs in older adults: a pilot randomized controlled trial. Rheumatol Int (2017) 37(3):389–98. doi: 10.1007/s00296-016-3620-2
6. Eckstein F, Hudelmaier M, Putz R. The effects of exercise on human articular cartilage. J Anat (2006) 208(4):491–512. doi: 10.1111/j.1469-7580.2006.00546.x
7. Sekiya I, Tang T, Hayashi M, Morito T, Ju YJ, Mochizuki T, et al. Periodic knee injections of BMP-7 delay cartilage degeneration induced by excessive running in rats. J Orthop Res (2009) 27(8):1088–92. doi: 10.1002/jor.20840
8. Pereira D, Peleteiro B, Araújo J, Branco J, Santos RA, Ramos E. The effect of osteoarthritis definition on prevalence and incidence estimates: a systematic review. Osteoarthritis Cartilage (2011) 19(11):1270–85. doi: 10.1016/j.joca.2011.08.009
9. Abramoff B, Caldera FE. Osteoarthritis: pathology, diagnosis, and treatment options. Med Clinics North America (2020) 104(2):293–311. doi: 10.1016/j.mcna.2019.10.007
10. Axford J, Butt A, Heron C, Hammond J, Morgan J, Alavi A, et al. Prevalence of anxiety and depression in osteoarthritis: use of the hospital anxiety and depression scale as a screening tool. Clin Rheumatol (2010) 29(11):1277–83. doi: 10.1007/s10067-010-1547-7
11. Hunter DJ, Bierma-Zeinstra S. Osteoarthritis. Lancet (2019) 393(10182):1745–59. doi: 10.1016/S0140-6736(19)30417-9
12. Zeng CY, Zhang ZR, Tang ZM, Hua FZ. Benefits and mechanisms of exercise training for knee osteoarthritis. Front Physiol (2021) 12:794062. doi: 10.3389/fphys.2021.794062
13. Finnila MAJ, Thevenot J, Aho OM, Tiitu V, Rautiainen J, Kauppinen S, et al. Association between subchondral bone structure and osteoarthritis histopathological grade. J Orthop Res (2017) 35(4):785–92. doi: 10.1002/jor.23312
14. Wada M, Maezawa Y, Baba H, Shimada S, Sasaki S, Nose Y. Relationships among bone mineral densities, static alignment and dynamic load in patients with medial compartment knee osteoarthritis. Rheumatol (Oxford) (2001) 40(5):499–505. doi: 10.1093/rheumatology/40.5.499
15. Castaneda S, Roman-Blas JA, Largo R, Herrero-Beaumont G. Subchondral bone as a key target for osteoarthritis treatment. Biochem Pharmacol (2012) 83(3):315–23. doi: 10.1016/j.bcp.2011.09.018
16. Zhou X, Cao H, Yuan Y, Wu W. Biochemical signals mediate the crosstalk between cartilage and bone in osteoarthritis. BioMed Res Int (2020) 2020:5720360. doi: 10.1155/2020/5720360
17. Burr DB. Anatomy and physiology of the mineralized tissues: role in the pathogenesis of osteoarthrosis. Osteoarthritis Cartilage (2004) 12 Suppl A:S20–30. doi: 10.1016/j.joca.2003.09.016
18. Goldring MB, Goldring SR. Articular cartilage and subchondral bone in the pathogenesis of osteoarthritis. Ann N Y Acad Sci (2010) 1192:230–7. doi: 10.1111/j.1749-6632.2009.05240.x
19. Milz S, Putz R. Quantitative morphology of the subchondral plate of the tibial plateau. J Anat (1994) 185(Pt 1):103–10.
20. Muratovic D, Findlay DM, Cicuttini FM, Wluka AE, Lee YR, Edwards S, et al. Bone marrow lesions in knee osteoarthritis: regional differences in tibial subchondral bone microstructure and their association with cartilage degeneration. Osteoarthritis Cartilage (2019) 27(11):1653–62. doi: 10.1016/j.joca.2019.07.004
21. Pountos I, Giannoudis PV. Modulation of cartilage's response to injury: can chondrocyte apoptosis be reversed? Injury (2017) 48(12):2657–69. doi: 10.1016/j.injury.2017.11.032
22. Suri S, Walsh DA. Osteochondral alterations in osteoarthritis. Bone (2012) 51(2):204–11. doi: 10.1016/j.bone.2011.10.010
23. Burr DB, Gallant MA. Bone remodelling in osteoarthritis. Nat Rev Rheumatol (2012) 8(11):665–73. doi: 10.1038/nrrheum.2012.130
24. Hayami T, Pickarski M, Zhuo Y, Wesolowski GA, Rodan GA, Duong LT. Characterization of articular cartilage and subchondral bone changes in the rat anterior cruciate ligament transection and meniscectomized models of osteoarthritis. Bone (2006) 38(2):234–43. doi: 10.1016/j.bone.2005.08.007
25. Zhu J, Zhu Y, Xiao W, Hu Y, Li Y. Instability and excessive mechanical loading mediate subchondral bone changes to induce osteoarthritis. Ann Transl Med (2020) 8(6):350. doi: 10.21037/atm.2020.02.103
26. Bonde HV, Talman ML, Kofoed H. The area of the tidemark in osteoarthritis–a three-dimensional stereological study in 21 patients. APMIS (2005) 113(5):349–52. doi: 10.1111/j.1600-0463.2005.apm_113506.x
27. Kamibayashi L, Wyss UP, Cooke TD, Zee B. Trabecular microstructure in the medial condyle of the proximal tibia of patients with knee osteoarthritis. Bone (1995) 17(1):27–35. doi: 10.1016/8756-3282(95)00137-3
28. Jia H, Ma X, Wei Y, Tong W, Tower RJ, Chandra A, et al. Loading-induced reduction in sclerostin as a mechanism of subchondral bone plate sclerosis in mouse knee joints during late-stage osteoarthritis. Arthritis Rheumatol (2018) 70(2):230–41. doi: 10.1002/art.40351
29. Han X, Cui J, Xie K, Jiang X, He Z, Du J, et al. Association between knee alignment, osteoarthritis disease severity, and subchondral trabecular bone microarchitecture in patients with knee osteoarthritis: a cross-sectional study. Arthritis Res Ther (2020) 22(1):203. doi: 10.1186/s13075-020-02274-0
30. Henriksen K, Karsdal M, Delaisse JM, Engsig MT. RANKL and vascular endothelial growth factor (VEGF) induce osteoclast chemotaxis through an ERK1/2-dependent mechanism. J Biol Chem (2003) 278(49):48745–53. doi: 10.1074/jbc.M309193200
31. Hermey DC, Popoff SN, Marks SC Jr. Reduced bone resorption in toothless (osteopetrotic) rats–an abnormality of osteoblasts related to their inability to activate osteoclast activity in vitro. Connect Tissue Res (1996) 35(1-4):273–8. doi: 10.3109/03008209609029201
32. Wuelling M, Vortkamp A. Chondrocyte proliferation and differentiation. Endocrine Dev (2011) 21:1–11. doi: 10.1159/000328081
33. Lee K, Deeds JD, Segre GV. Expression of parathyroid hormone-related peptide and its receptor messenger ribonucleic acids during fetal development of rats. Endocrinology (1995) 136(2):453–63. doi: 10.1210/endo.136.2.7835276
34. Karaplis AC, Luz A, Glowacki J, Bronson RT, Tybulewicz VL, Kronenberg HM, et al. Lethal skeletal dysplasia from targeted disruption of the parathyroid hormone-related peptide gene. Genes Dev (1994) 8(3):277–89. doi: 10.1101/gad.8.3.277
35. Schipani E, Kruse K, Jüppner H. A constitutively active mutant PTH-PTHrP receptor in Jansen-type metaphyseal chondrodysplasia. Sci (New York NY) (1995) 268(5207):98–100. doi: 10.1126/science.7701349
36. Schipani E, Lanske B, Hunzelman J, Luz A, Kovacs CS, Lee K, et al. Targeted expression of constitutively active receptors for parathyroid hormone and parathyroid hormone-related peptide delays endochondral bone formation and rescues mice that lack parathyroid hormone-related peptide. Proc Natl Acad Sci USA (1997) 94(25):13689–94. doi: 10.1073/pnas.94.25.13689
37. Østerlund T, Kogerman P. Hedgehog signalling: how to get from smo to ci and gli. Trends Cell Biol (2006) 16(4):176–80. doi: 10.1016/j.tcb.2006.02.004
38. Karp SJ, Schipani E, St-Jacques B, Hunzelman J, Kronenberg H, McMahon AP. Indian Hedgehog coordinates endochondral bone growth and morphogenesis via parathyroid hormone related-protein-dependent and -independent pathways. Dev (Cambridge England) (2000) 127(3):543–8. doi: 10.1242/dev.127.3.543
39. St-Jacques B, Hammerschmidt M, McMahon AP. Indian Hedgehog signaling regulates proliferation and differentiation of chondrocytes and is essential for bone formation. Genes Dev (1999) 13(16):2072–86. doi: 10.1101/gad.13.16.2072
40. Deng C, Wynshaw-Boris A, Zhou F, Kuo A, Leder P. Fibroblast growth factor receptor 3 is a negative regulator of bone growth. Cell (1996) 84(6):911–21. doi: 10.1016/S0092-8674(00)81069-7
41. Eswarakumar VP, Schlessinger J. Skeletal overgrowth is mediated by deficiency in a specific isoform of fibroblast growth factor receptor 3. Proc Natl Acad Sci USA (2007) 104(10):3937–42. doi: 10.1073/pnas.0700012104
42. Priore R, Dailey L, Basilico C. Downregulation of akt activity contributes to the growth arrest induced by FGF in chondrocytes. J Cell Physiol (2006) 207(3):800–8. doi: 10.1002/jcp.20620
43. Naski MC, Colvin JS, Coffin JD, Ornitz DM. Repression of hedgehog signaling and BMP4 expression in growth plate cartilage by fibroblast growth factor receptor 3. Dev (Cambridge England) (1998) 125(24):4977–88. doi: 10.1242/dev.125.24.4977
44. Retting KN, Song B, Yoon BS, Lyons KM. BMP canonical smad signaling through Smad1 and Smad5 is required for endochondral bone formation. Dev (Cambridge England) (2009) 136(7):1093–104. doi: 10.1242/dev.029926
45. Boyden LM, Mao J, Belsky J, Mitzner L, Farhi A, Mitnick MA, et al. High bone density due to a mutation in LDL-receptor-related protein 5. New Engl J Med (2002) 346(20):1513–21. doi: 10.1056/NEJMoa013444
46. Ellies DL, Viviano B, McCarthy J, Rey JP, Itasaki N, Saunders S, et al. Bone density ligand, sclerostin, directly interacts with LRP5 but not LRP5G171V to modulate wnt activity. J Bone mineral Res Off J Am Soc Bone Mineral Res (2006) 21(11):1738–49. doi: 10.1359/jbmr.060810
47. Gong Y, Slee RB, Fukai N, Rawadi G, Roman-Roman S, Reginato AM, et al. LDL receptor-related protein 5 (LRP5) affects bone accrual and eye development. Cell (2001) 107(4):513–23. doi: 10.1016/S0092-8674(01)00571-2
48. Bi W, Deng JM, Zhang Z, Behringer RR, de Crombrugghe B. Sox9 is required for cartilage formation. Nat Genet (1999) 22(1):85–9. doi: 10.1038/8792
49. Akiyama H, Chaboissier MC, Martin JF, Schedl A, de Crombrugghe B. The transcription factor Sox9 has essential roles in successive steps of the chondrocyte differentiation pathway and is required for expression of Sox5 and Sox6. Genes Dev (2002) 16(21):2813–28. doi: 10.1101/gad.1017802
50. Komori T, Yagi H, Nomura S, Yamaguchi A, Sasaki K, Deguchi K, et al. Targeted disruption of Cbfa1 results in a complete lack of bone formation owing to maturational arrest of osteoblasts. Cell (1997) 89(5):755–64. doi: 10.1016/S0092-8674(00)80258-5
51. Lee B, Thirunavukkarasu K, Zhou L, Pastore L, Baldini A, Hecht J, et al. Missense mutations abolishing DNA binding of the osteoblast-specific transcription factor OSF2/CBFA1 in cleidocranial dysplasia. Nat Genet (1997) 16(3):307–10. doi: 10.1038/ng0797-307
52. Nakashima K, Zhou X, Kunkel G, Zhang Z, Deng JM, Behringer RR, et al. The novel zinc finger-containing transcription factor osterix is required for osteoblast differentiation and bone formation. Cell (2002) 108(1):17–29. doi: 10.1016/S0092-8674(01)00622-5
53. Zhou X, Zhang Z, Feng JQ, Dusevich VM, Sinha K, Zhang H, et al. Multiple functions of osterix are required for bone growth and homeostasis in postnatal mice. Proc Natl Acad Sci USA (2010) 107(29):12919–24. doi: 10.1073/pnas.0912855107
54. Yang X, Matsuda K, Bialek P, Jacquot S, Masuoka HC, Schinke T, et al. ATF4 is a substrate of RSK2 and an essential regulator of osteoblast biology; implication for coffin-lowry syndrome. Cell (2004) 117(3):387–98. doi: 10.1016/S0092-8674(04)00344-7
55. Yu VW, Ambartsoumian G, Verlinden L, Moir JM, Prud'homme J, Gauthier C, et al. FIAT represses ATF4-mediated transcription to regulate bone mass in transgenic mice. J Cell Biol (2005) 169(4):591–601. doi: 10.1083/jcb.200412139
56. Amin AK, Huntley JS, Simpson AH, Hall AC. Chondrocyte survival in articular cartilage: the influence of subchondral bone in a bovine model. J Bone Joint Surg Br (2009) 91(5):691–9. doi: 10.1302/0301-620X.91B5.21544
57. Sanchez C, Deberg MA, Piccardi N, Msika P, Reginster JY, Henrotin YE. Subchondral bone osteoblasts induce phenotypic changes in human osteoarthritic chondrocytes. Osteoarthritis Cartilage (2005) 13(11):988–97. doi: 10.1016/j.joca.2005.07.012
58. Mazur CM, Woo JJ, Yee CS, Fields AJ, Acevedo C, Bailey KN, et al. Osteocyte dysfunction promotes osteoarthritis through MMP13-dependent suppression of subchondral bone homeostasis. Bone Res (2019) 7:34. doi: 10.1038/s41413-019-0070-y
59. Liu-Bryan R, Terkeltaub R. Emerging regulators of the inflammatory process in osteoarthritis. Nat Rev Rheumatol (2015) 11(1):35–44. doi: 10.1038/nrrheum.2014.162
60. Verborgt O, Gibson GJ, Schaffler MB. Loss of osteocyte integrity in association with microdamage and bone remodeling after fatigue in vivo. J Bone Miner Res (2000) 15(1):60–7. doi: 10.1359/jbmr.2000.15.1.60
61. Hussain SM, Dawson C, Wang Y, Tonkin AM, Chou L, Wluka AE, et al. Vascular pathology and osteoarthritis: a systematic review. J Rheumatol (2020) 47(5):748–60. doi: 10.3899/jrheum.181236
62. Duncan H, Jundt J, Riddle JM, Pitchford W, Christopherson T. The tibial subchondral plate. a scanning electron microscopic study. J Bone Joint Surg Am (1987) 69(8):1212–20. doi: 10.2106/00004623-198769080-00015
63. Pan J, Zhou X, Li W, Novotny JE, Doty SB, Wang L. In situ measurement of transport between subchondral bone and articular cartilage. J Orthop Res (2009) 27(10):1347–52. doi: 10.1002/jor.20883
64. Lyons TJ, McClure SF, Stoddart RW, McClure J. The normal human chondro-osseous junctional region: evidence for contact of uncalcified cartilage with subchondral bone and marrow spaces. BMC Musculoskelet Disord (2006) 7:52. doi: 10.1186/1471-2474-7-52
65. Geng Z, Wang XG, Yu YM, Ji LL, Wang J, Liu CS. Attenuating osteoarthritis by a high efficient anti-bone resorption injectable pH-responsive bisphosphonate-conjugated nano-apatite system. Chem Eng J (2021) 420:12674. doi: 10.1016/j.cej.2020.127674
66. Zhai M, Lu Y, Fu J, Zhu Y, Zhao Y, Shang L, et al. Fourier Transform infrared spectroscopy research on subchondral bone in osteoarthritis. Spectrochim Acta A Mol Biomol Spectrosc (2019) 218:243–7. doi: 10.1016/j.saa.2019.04.020
67. Li J, Zhang H, Han Y, Hu Y, Geng Z, Su J. Targeted and responsive biomaterials in osteoarthritis. Theranostics (2023) 13(3):931–54. doi: 10.7150/thno.78639
68. Bultink IE, Lems WF. Osteoarthritis and osteoporosis: what is the overlap? Curr Rheumatol Rep (2013) 15(5):328. doi: 10.1007/s11926-013-0328-0
69. Nevitt MC, Lane NE, Scott JC, Hochberg MC, Pressman AR, Genant HK, et al. Radiographic osteoarthritis of the hip and bone mineral density. the study of osteoporotic fractures research group. Arthritis Rheum (1995) 38(7):907–16. doi: 10.1002/art.1780380706
70. Dequeker J, Boonen S, Aerssens J, Westhovens R. Inverse relationship osteoarthritis-osteoporosis: what is the evidence? what are the consequences? Br J Rheumatol (1996) 35(9):813–8. doi: 10.1093/rheumatology/35.9.813
71. Tokgoz MA, Atik OS, Esendagli G, Ogut B, Bozkurt HH. Is it possible that the pathogenesis of osteoarthritis could start with subchondral trabecular bone loss like osteoporosis? Eklem Hastalik Cerrahisi (2018) 29(3):152–8. doi: 10.5606/ehc.2018.007
72. Zhang Y, Hannan MT, Chaisson CE, McAlindon TE, Evans SR, Aliabadi P, et al. Bone mineral density and risk of incident and progressive radiographic knee osteoarthritis in women: the framingham study. J Rheumatol (2000) 27(4):1032–7.
73. Zhang Y, McAlindon TE, Hannan MT, Chaisson CE, Klein R, Wilson PW, et al. Estrogen replacement therapy and worsening of radiographic knee osteoarthritis: the framingham study. Arthritis Rheum (1998) 41(10):1867–73. doi: 10.1002/1529-0131(199810)41:10<1867::AID-ART20>3.0.CO;2-W
74. Weaver CM, Gordon CM, Janz KF, Kalkwarf HJ, Lappe JM, Lewis R, et al. The national osteoporosis foundation's position statement on peak bone mass development and lifestyle factors: a systematic review and implementation recommendations. Osteoporos Int (2016) 27(4):1281–386. doi: 10.1007/s00198-015-3440-3
75. Harding AT, Weeks BK, Lambert C, Watson SL, Weis LJ, Beck BR. A comparison of bone-targeted exercise strategies to reduce fracture risk in middle-aged and older men with osteopenia and osteoporosis: LIFTMOR-m semi-randomized controlled trial. J Bone Miner Res (2020) 35(8):1404–14. doi: 10.1002/jbmr.4008
76. Lambert C, Beck BR, Harding AT, Watson SL, Weeks BK. Regional changes in indices of bone strength of upper and lower limbs in response to high-intensity impact loading or high-intensity resistance training. Bone (2020) 132:115192. doi: 10.1016/j.bone.2019.115192
77. Watson SL, Weeks BK, Weis LJ, Harding AT, Horan SA, Beck BR. High-intensity resistance and impact training improves bone mineral density and physical function in postmenopausal women with osteopenia and osteoporosis: the LIFTMOR randomized controlled trial. J Bone Miner Res (2018) 33(2):211–20. doi: 10.1002/jbmr.3284
78. Ozcivici E, Luu YK, Adler B, Qin YX, Rubin J, Judex S, et al. Mechanical signals as anabolic agents in bone. Nat Rev Rheumatol (2010) 6(1):50–9. doi: 10.1038/nrrheum.2009.239
79. Huiskes R, Ruimerman R, van Lenthe GH, Janssen JD. Effects of mechanical forces on maintenance and adaptation of form in trabecular bone. Nature (2000) 405(6787):704–6. doi: 10.1038/35015116
80. Rodrigues B, Figueroa DM, Mostarda CT, Heeren MV, Irigoyen MC, De Angelis K. Maximal exercise test is a useful method for physical capacity and oxygen consumption determination in streptozotocin-diabetic rats. Cardiovasc Diabetol (2007) 6:38. doi: 10.1186/1475-2840-6-38
81. Lancha AH Jr., Recco MB, Abdalla DS, Curi R. Effect of aspartate, asparagine, and carnitine supplementation in the diet on metabolism of skeletal muscle during a moderate exercise. Physiol Behav (1995) 57(2):367–71. doi: 10.1016/0031-9384(94)00243-X
82. Ni GX, Zhou YZ, Chen W, Xu L, Li Z, Liu SY, et al. Different responses of articular cartilage to strenuous running and joint immobilization. Connect Tissue Res (2016) 57(2):143–51. doi: 10.3109/03008207.2015.1117457
83. Yao Z, Chen P, Wang S, Deng G, Hu Y, Lin Q, et al. Reduced PDGF-AA in subchondral bone leads to articular cartilage degeneration after strenuous running. J Cell Physiol (2019) 234(10):17946–58. doi: 10.1002/jcp.28427
84. Kawakita K, Nishiyama T, Fujishiro T, Hayashi S, Kanzaki N, Hashimoto S, et al. Akt phosphorylation in human chondrocytes is regulated by p53R2 in response to mechanical stress. Osteoarthritis Cartilage (2012) 20(12):1603–9. doi: 10.1016/j.joca.2012.08.022
85. Fallah Mohammadi M, Hajizadeh Moghaddam A, Mirkarimpur H. The effects of a moderate exercise program on knee osteoarthritis in male wistar rats. Iran J Basic Med Sci (2013) 16(5):683–8.
86. Iijima H, Aoyama T, Ito A, Yamaguchi S, Nagai M, Tajino J, et al. Effects of short-term gentle treadmill walking on subchondral bone in a rat model of instability-induced osteoarthritis. Osteoarthritis Cartilage (2015) 23(9):1563–74. doi: 10.1016/j.joca.2015.04.015
87. Hao X, Wang S, Zhang J, Xu T. Effects of body weight-supported treadmill training on cartilage-subchondral bone unit in the rat model of posttraumatic osteoarthritis. J Orthop Res (2021) 39(6):1227–35. doi: 10.1002/jor.24791
88. Zhou X, Cao H, Wang M, Zou J, Wu W. Moderate-intensity treadmill running relieves motion-induced post-traumatic osteoarthritis mice by up-regulating the expression of lncRNA H19. BioMed Eng Online (2021) 20(1):111. doi: 10.1186/s12938-021-00949-6
89. Assis L, Milares LP, Almeida T, Tim C, Magri A, Fernandes KR, et al. Aerobic exercise training and low-level laser therapy modulate inflammatory response and degenerative process in an experimental model of knee osteoarthritis in rats. Osteoarthritis cartilage / OARS Osteoarthritis Res Soc (2016) 24(1):169–77. doi: 10.1016/j.joca.2015.07.020
90. Chen L, Lou Y, Pan Z, Cao X, Zhang L, Zhu C, et al. Treadmill and wheel exercise protect against JNK/NF-kappaB induced inflammation in experimental models of knee osteoarthritis. Biochem Biophys Res Commun (2020) 523(1):117–22. doi: 10.1016/j.bbrc.2019.12.014
91. Castrogiovanni P, Di Rosa M, Ravalli S, Castorina A, Guglielmino C, Imbesi R, et al. Moderate physical activity as a prevention method for knee osteoarthritis and the role of synoviocytes as biological key. Int J Mol Sci (2019) 20(3):511. doi: 10.3390/ijms20030511
92. Hsieh YL, Yang CC. Early intervention of swimming exercises attenuate articular cartilage destruction in a rat model of anterior cruciate ligament and meniscus knee injuries. Life Sci (2018) 212:267–74. doi: 10.1016/j.lfs.2018.10.013
93. Carmeliet P, Jain RK. Molecular mechanisms and clinical applications of angiogenesis. Nature (2011) 473(7347):298–307. doi: 10.1038/nature10144
94. Barker KL, Newman M, Stallard N, Leal J, Minns Lowe C, Javaid MK, et al. Exercise or manual physiotherapy compared with a single session of physiotherapy for osteoporotic vertebral fracture: three-arm PROVE RCT. Health Technol Assess (Winchester England) (2019) 23(44):1–318. doi: 10.3310/hta23440
95. Jing H, Liao L, Su X, Shuai Y, Zhang X, Deng Z, et al. Declining histone acetyltransferase GCN5 represses BMSC-mediated angiogenesis during osteoporosis. FASEB J (2017) 31(10):4422–33. doi: 10.1096/fj.201700118R
96. Patschan D, Sugiarto N, Henze E, Mossner R, Mohr J, Muller GA, et al. Early endothelial progenitor cells and vascular stiffness in psoriasis and psoriatic arthritis. Eur J Med Res (2018) 23(1):56. doi: 10.1186/s40001-018-0352-7
97. Bartholdy C, Juhl C, Christensen R, Lund H, Zhang W, Henriksen M. The role of muscle strengthening in exercise therapy for knee osteoarthritis: a systematic review and meta-regression analysis of randomized trials. Semin Arthritis rheumatism (2017) 47(1):9–21. doi: 10.1016/j.semarthrit.2017.03.007
98. Abbasi J. Can exercise prevent knee osteoarthritis? Jama (2017) 318(22):2169–71. doi: 10.1001/jama.2017.16144
99. Deschner J, Hofman CR, Piesco NP, Agarwal S. Signal transduction by mechanical strain in chondrocytes. Curr Opin Clin Nutr Metab Care (2003) 6(3):289–93. doi: 10.1097/01.mco.0000068964.34812.2b
100. Rim YA, Nam Y, Ju JH. The role of chondrocyte hypertrophy and senescence in osteoarthritis initiation and progression. Int J Mol Sci (2020) 21(7):2358. doi: 10.3390/ijms21072358
101. Fang H, Huang L, Welch I, Norley C, Holdsworth DW, Beier F, et al. Early changes of articular cartilage and subchondral bone in the DMM mouse model of osteoarthritis. Sci Rep (2018) 8(1):2855. doi: 10.1038/s41598-018-21184-5
102. Lin C, Shao Y, Zeng C, Zhao C, Fang H, Wang L, et al. Blocking PI3K/AKT signaling inhibits bone sclerosis in subchondral bone and attenuates post-traumatic osteoarthritis. J Cell Physiol (2018) 233(8):6135–47. doi: 10.1002/jcp.26460
103. Sanchez-Adams J, Leddy HA, McNulty AL, O'Conor CJ, Guilak F. The mechanobiology of articular cartilage: bearing the burden of osteoarthritis. Curr Rheumatol Rep (2014) 16(10):451. doi: 10.1007/s11926-014-0451-6
104. Burger EH, Klein-Nulend J. Mechanotransduction in bone–role of the lacuno-canalicular network. FASEB J (1999) 13 Suppl:S101–12.
105. Bonewald LF. Mechanosensation and transduction in osteocytes. Bonekey Osteovision (2006) 3(10):7–15. doi: 10.1138/20060233
106. Nusse R. Wnt signaling in disease and in development. Cell Res (2005) 15(1):28–32. doi: 10.1038/sj.cr.7290260
107. Yuan X, Liu H, Huang H, Liu H, Li L, Yang J, et al. The key role of canonical wnt/beta-catenin signaling in cartilage chondrocytes. Curr Drug Targets (2016) 17(4):475–84. doi: 10.2174/1389450116666150825112623
108. Ma B, Landman EB, Miclea RL, Wit JM, Robanus-Maandag EC, Post JN, et al. WNT signaling and cartilage: of mice and men. Calcif Tissue Int (2013) 92(5):399–411. doi: 10.1007/s00223-012-9675-5
109. Zhu Z, Bai X, Wang H, Li X, Sun G, Zhang P. A study on the mechanism of wnt inhibitory factor 1 in osteoarthritis. Arch Med Sci (2020) 16(4):898–906. doi: 10.5114/aoms.2020.95667
110. Liu SS, Zhou P, Zhang Y. Abnormal expression of key genes and proteins in the canonical wnt/beta-catenin pathway of articular cartilage in a rat model of exercise-induced osteoarthritis. Mol Med Rep (2016) 13(3):1999–2006. doi: 10.3892/mmr.2016.4798
111. Kwan Tat S, Amiable N, Pelletier JP, Boileau C, Lajeunesse D, Duval N, et al. Modulation of OPG, RANK and RANKL by human chondrocytes and their implication during osteoarthritis. Rheumatology (2009) 48(12):1482–90. doi: 10.1093/rheumatology/kep300
112. Jiao K, Niu LN, Wang MQ, Dai J, Yu SB, Liu XD, et al. Subchondral bone loss following orthodontically induced cartilage degradation in the mandibular condyles of rats. Bone (2011) 48(2):362–71. doi: 10.1016/j.bone.2010.09.010
113. Upton AR, Holding CA, Dharmapatni AA, Haynes DR. The expression of RANKL and OPG in the various grades of osteoarthritic cartilage. Rheumatol Int (2012) 32(2):535–40. doi: 10.1007/s00296-010-1733-6
114. Wang B, Jin H, Shu B, Mira RR, Chen D. Chondrocytes-specific expression of osteoprotegerin modulates osteoclast formation in metaphyseal bone. Sci Rep (2015) 5:13667. doi: 10.1038/srep13667
115. Boyce BF, Xing L, Chen D. Osteoprotegerin, the bone protector, is a surprising target for beta-catenin signaling. Cell Metab (2005) 2(6):344–5. doi: 10.1016/j.cmet.2005.11.011
116. Haxaire C, Hay E, Geoffroy V. Runx2 controls bone resorption through the down-regulation of the wnt pathway in osteoblasts. Am J Pathol (2016) 186(6):1598–609. doi: 10.1016/j.ajpath.2016.01.016
117. Nesi RT, Barroso MV, Souza Muniz V, de Arantes AC, Martins MA, Brito Gitirana L, et al. Pharmacological modulation of reactive oxygen species (ROS) improves the airway hyperresponsiveness by shifting the Th1 response in allergic inflammation induced by ovalbumin. Free Radic Res (2017) 51(7-8):708–22. doi: 10.1080/10715762.2017.1364377
118. Kaczor JJ, Hall JE, Payne E, Tarnopolsky MA. Low intensity training decreases markers of oxidative stress in skeletal muscle of mdx mice. Free Radic Biol Med (2007) 43(1):145–54. doi: 10.1016/j.freeradbiomed.2007.04.003
119. Koch H, Jadlowiec JA, Campbell PG. Insulin-like growth factor-I induces early osteoblast gene expression in human mesenchymal stem cells. Stem Cells Dev (2005) 14(6):621–31. doi: 10.1089/scd.2005.14.621
120. Massicotte F, Fernandes JC, Martel-Pelletier J, Pelletier JP, Lajeunesse D. Modulation of insulin-like growth factor 1 levels in human osteoarthritic subchondral bone osteoblasts. Bone (2006) 38(3):333–41. doi: 10.1016/j.bone.2005.09.007
121. Angulo J, El Assar M, Alvarez-Bustos A, Rodriguez-Manas L. Physical activity and exercise: strategies to manage frailty. Redox Biol (2020) 35:101513. doi: 10.1016/j.redox.2020.101513
122. Matheny RW, Merritt E, Zannikos SV, Farrar RP, Adamo ML. Serum IGF-i-deficiency does not prevent compensatory skeletal muscle hypertrophy in resistance exercise. Exp Biol Med (Maywood) (2009) 234(2):164–70. doi: 10.3181/0808-RM-251
123. Crane JL, Cao X. Bone marrow mesenchymal stem cells and TGF-beta signaling in bone remodeling. J Clin Invest (2014) 124(2):466–72. doi: 10.1172/JCI70050
124. Hase H, Kanno Y, Kojima H, Sakurai D, Kobata T. Coculture of osteoclast precursors with rheumatoid synovial fibroblasts induces osteoclastogenesis via transforming growth factor beta-mediated down-regulation of osteoprotegerin. Arthritis Rheum (2008) 58(11):3356–65. doi: 10.1002/art.23971
125. Ashraf S, Cha BH, Kim JS, Ahn J, Han I, Park H, et al. Regulation of senescence associated signaling mechanisms in chondrocytes for cartilage tissue regeneration. Osteoarthritis Cartilage (2016) 24(2):196–205. doi: 10.1016/j.joca.2015.07.008
126. Li TF, Chen D, Wu Q, Chen M, Sheu TJ, Schwarz EM, et al. Transforming growth factor-beta stimulates cyclin D1 expression through activation of beta-catenin signaling in chondrocytes. J Biol Chem (2006) 281(30):21296–304. doi: 10.1074/jbc.M600514200
127. Yuan XL, Meng HY, Wang YC, Peng J, Guo QY, Wang AY, et al. Bone-cartilage interface crosstalk in osteoarthritis: potential pathways and future therapeutic strategies. Osteoarthritis Cartilage (2014) 22(8):1077–89. doi: 10.1016/j.joca.2014.05.023
128. Zhen G, Wen C, Jia X, Li Y, Crane JL, Mears SC, et al. Inhibition of TGF-beta signaling in mesenchymal stem cells of subchondral bone attenuates osteoarthritis. Nat Med (2013) 19(6):704–12. doi: 10.1038/nm.3143
129. Zhang RK, Li GW, Zeng C, Lin CX, Huang LS, Huang GX, et al. Mechanical stress contributes to osteoarthritis development through the activation of transforming growth factor beta 1 (TGF-β1). Bone Joint Res (2018) 7(11):587–94. doi: 10.1302/2046-3758.711.BJR-2018-0057.R1
130. Hodge JM, Kirkland MA, Nicholson GC. Multiple roles of m-CSF in human osteoclastogenesis. J Cell Biochem (2007) 102(3):759–68. doi: 10.1002/jcb.21331
131. Rabie AB, She TT, Hagg U. Functional appliance therapy accelerates and enhances condylar growth. Am J Orthod Dentofacial Orthop (2003) 123(1):40–8. doi: 10.1067/mod.2003.45
132. Juhasz T, Matta C, Somogyi C, Katona E, Takacs R, Soha RF, et al. Mechanical loading stimulates chondrogenesis via the PKA/CREB-Sox9 and PP2A pathways in chicken micromass cultures. Cell Signal (2014) 26(3):468–82. doi: 10.1016/j.cellsig.2013.12.001
133. Asadi S, Farzanegi P, Azarbayjani MA. Combined therapies with exercise, ozone and mesenchymal stem cells improve the expression of HIF1 and SOX9 in the cartilage tissue of rats with knee osteoarthritis. Physiol Int (2020) 107(2):231–42. doi: 10.1556/2060.2020.00024
134. Wang ZB, Wang L, Liu QQ, Yang YH, Liu P, Li SL, et al. Repair impact of vibration exercise with different frequencies on articular cartilage of rats with early knee osteoarthritis and its JNK/NF-kappaB, SOX9 mechanisms. Zhongguo Ying Yong Sheng Li Xue Za Zhi (2022) 38(1):41–6. doi: 10.12047/j.cjap.6204.2022.008
135. Lombardi G, Di Somma C, Rubino M, Faggiano A, Vuolo L, Guerra E, et al. The roles of parathyroid hormone in bone remodeling: prospects for novel therapeutics. J Endocrinol Invest (2011) 34(7 Suppl):18–22.
136. Maimoun L, Sultan C. Effect of physical activity on calcium homeostasis and calciotropic hormones: a review. Calcif Tissue Int (2009) 85(4):277–86. doi: 10.1007/s00223-009-9277-z
137. Maimoun L, Simar D, Caillaud C, Coste O, Barbotte E, Peruchon E, et al. Response of calciotropic hormones and bone turnover to brisk walking according to age and fitness level. J Sci Med sport / Sports Med Aust (2009) 12(4):463–7. doi: 10.1016/j.jsams.2008.05.004
138. Maimoun L, Simar D, Malatesta D, Caillaud C, Peruchon E, Couret I, et al. Response of bone metabolism related hormones to a single session of strenuous exercise in active elderly subjects. Br J Sports Med (2005) 39(8):497–502. doi: 10.1136/bjsm.2004.013151
139. Nishiyama S, Tomoeda S, Ohta T, Higuchi A, Matsuda I. Differences in basal and postexercise osteocalcin levels in athletic and nonathletic humans. Calcif Tissue Int (1988) 43(3):150–4. doi: 10.1007/BF02571312
140. Rong H, Berg U, Torring O, Sundberg CJ, Granberg B, Bucht E. Effect of acute endurance and strength exercise on circulating calcium-regulating hormones and bone markers in young healthy males. Scand J Med Sci Sports (1997) 7(3):152–9. doi: 10.1111/j.1600-0838.1997.tb00132.x
141. Gardinier JD, Rostami N, Juliano L, Zhang C. Bone adaptation in response to treadmill exercise in young and adult mice. Bone Rep (2018) 8:29–37. doi: 10.1016/j.bonr.2018.01.003
142. Maycas M, Ardura JA, de Castro LF, Bravo B, Gortazar AR, Esbrit P. Role of the parathyroid hormone type 1 receptor (PTH1R) as a mechanosensor in osteocyte survival. J Bone Miner Res (2015) 30(7):1231–44. doi: 10.1002/jbmr.2439
143. Orth P, Cucchiarini M, Zurakowski D, Menger MD, Kohn DM, Madry H. Parathyroid hormone [1-34] improves articular cartilage surface architecture and integration and subchondral bone reconstitution in osteochondral defects in vivo. Osteoarthritis Cartilage (2013) 21(4):614–24. doi: 10.1016/j.joca.2013.01.008
144. Gardinier JD, Daly-Seiler C, Rostami N, Kundal S, Zhang C. Loss of the PTH/PTHrP receptor along the osteoblast lineage limits the anabolic response to exercise. PloS One (2019) 14(1):e0211076. doi: 10.1371/journal.pone.0211076
145. Yan JY, Tian FM, Wang WY, Cheng Y, Song HP, Zhang YZ, et al. Parathyroid hormone (1-34) prevents cartilage degradation and preserves subchondral bone micro-architecture in guinea pigs with spontaneous osteoarthritis. Osteoarthritis Cartilage (2014) 22(11):1869–77. doi: 10.1016/j.joca.2014.07.013
Keywords: bone, exercise, bone-cartilage crosstalk, osteoarthritis, mechanical stress
Citation: Zhang S, Li T, Feng Y, Zhang K, Zou J, Weng X, Yuan Y and Zhang L (2023) Exercise improves subchondral bone microenvironment through regulating bone-cartilage crosstalk. Front. Endocrinol. 14:1159393. doi: 10.3389/fendo.2023.1159393
Received: 05 February 2023; Accepted: 04 May 2023;
Published: 23 May 2023.
Edited by:
An Qin, Shanghai Jiao Tong University, ChinaReviewed by:
Zhen Geng, Shanghai University, ChinaDan Zhang, Shandong University of Traditional Chinese Medicine, China
Qiguan Jin, Yangzhou University, China
Jun Zhang, Shanghai Normal University, China
Copyright © 2023 Zhang, Li, Feng, Zhang, Zou, Weng, Yuan and Zhang. This is an open-access article distributed under the terms of the Creative Commons Attribution License (CC BY). The use, distribution or reproduction in other forums is permitted, provided the original author(s) and the copyright owner(s) are credited and that the original publication in this journal is cited, in accordance with accepted academic practice. No use, distribution or reproduction is permitted which does not comply with these terms.
*Correspondence: Yu Yuan, eXVhbnl1bWFpbEAxMjYuY29t; Lan Zhang, emhhbmdsYW5fc2R0eUAxNjMuY29t
†These authors have contributed equally to this work