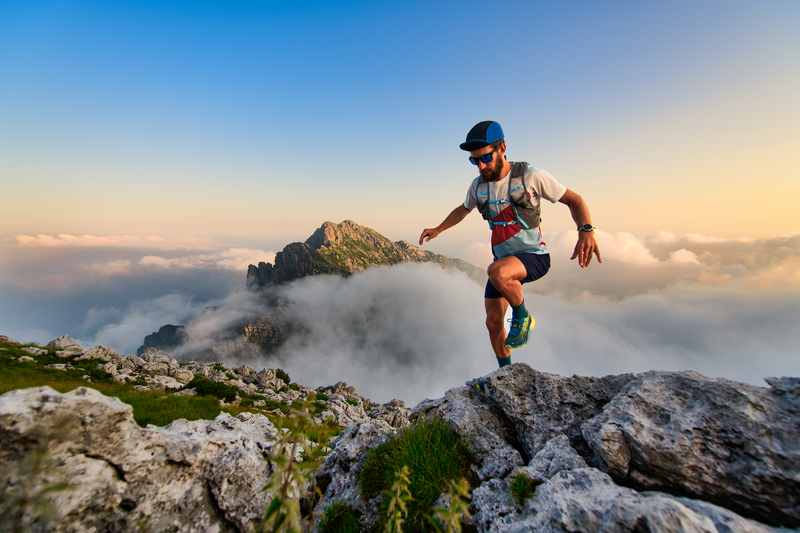
95% of researchers rate our articles as excellent or good
Learn more about the work of our research integrity team to safeguard the quality of each article we publish.
Find out more
MINI REVIEW article
Front. Endocrinol. , 05 August 2022
Sec. Clinical Diabetes
Volume 13 - 2022 | https://doi.org/10.3389/fendo.2022.963282
This article is part of the Research Topic Xenotransplantation for the Therapy of Diabetes: a New Look View all 5 articles
Pancreas (and islet) transplantation is the only curative treatment for type 1 diabetes patients whose β-cell functions have been abolished. However, the lack of donor organs has been the major hurdle to save a large number of patients. Therefore, transplantation of animal organs is expected to be an alternative method to solve the serious shortage of donor organs. More recently, a method to generate organs from pluripotent stem cells inside the body of other species has been developed. This interspecies organ generation using blastocyst complementation (BC) is expected to be the next-generation regenerative medicine. Here, we describe the recent advances and future prospects for these two approaches.
Type 1 diabetes mellitus and diabetes after total pancreatectomy completely deplete insulin secretion. Patients with insulin-dependent diabetes administrate exogenous insulin to control blood glucose levels. However, depletion of a patient’s own insulin secretion makes blood glucose levels extremely unstable, which results not only in the progression of diabetic complications but also in life-threatening and life-long deterioration of quality of life due to severe hypoglycemia. Pancreas transplantation is a great hope for patients suffering from insulin-dependent diabetes because it is the only curative treatment available today. Successful pancreas transplantation can lead to normalization of blood glucose and HbA1c levels which allow patients to be free from daily insulin injections (1). Pancreas transplantation is considered a good option because the glucagon response to hypoglycemia can be restored simultaneously, preventing prolonged hypoglycemia (2). Furthermore, pancreas transplantation may be superior to conventional insulin therapy from the standpoint of health economics (3). Currently, the majority of pancreases as transplant organs rely on donations from brain-dead donors. However, many patients are unable to receive pancreas transplantation due to donor organ shortage. Instead of human-to-human allogeneic transplantation, animal-derived organ transplantation (xenotransplantation) has been expected to solve organ shortage. In January 2022, the University of Maryland School of Medicine announced that they had successfully transplanted a genetically modified pig heart into a patient with end-stage cardiac disease. Unfortunately, the patient passed away about 2 months after the transplantation, but it made a big step toward the clinical application of xenotransplantation.
Xenotransplantation has a long history, with its initial concept of transplanting part of the pancreas or its extract from a heterologous animal into a diabetic patient, first reported more than 100 years ago; in 1894, Williams et al. transplanted minced sheep pancreas subcutaneously into a type 1 diabetic boy with ketoacidosis (4). At that time, xenotransplantation was a reckless challenge because effective immunosuppressive agents and gene editing techniques were not developed yet. In the early days, non-human primate (NHP) organ transplantation was widely studied (5). However, smaller organ size, ethical concerns, the risk of cross-species transmission between humans and NHPs, and the difficulty of reproduction led to a gradual decline. Next, pigs became a candidate organ donor for the similarity in organ size and physiology and for their reproductivity. Despite those advantages, there were remaining difficulties for clinical application, which are immune rejection and pig-to-human infection.
What happens when wild-type porcine organs are transplanted into humans? The human immune system recognizes the antigens expressed on the surface of porcine cells and starts attacking immediately after transplantation and results in hyperacute rejection (HAR). It progresses from a few minutes to several tens of minutes, until it gets to the point that the graft loses its function completely. Galactose-a1,3-galactose (α-Gal) was first noticed as the major xenograft antigen in pig-to-human transplantation (6). α-Gal is present in many mammals, including pigs, but not in some primates such as humans and Old-World monkeys. In these primates, natural antibodies against α-Gal exist in blood (7). When anti-α-Gal antibodies bind to the α-Gal antigen expressed on the surface of porcine endothelial cells, which induces the activation of complement proteins, the graft is eliminated (8). To avoid HAR, the gene-encoding α1,3-galactosyltransferase (α1,3GalT, GGTA1) knockout (KO) pigs were required. In 2001, α1,3GalT heterozygous KO pigs were developed at PPL Therapeutics (now Revivicor) and Immerge BioTherapeutics (9, 10) via cloning by somatic cell nuclear transfer. The following year, α1,3GalT was homozygously knocked out, and cloned pigs with the α-Gal antigen completely eliminated were generated (11, 12). To date, two other xenoantigens that were problematic for transplantation, namely, N-glycolylneuraminic acid (Neu5Gc) (13) and β1,4-N-acetylgalactosyltransferase (β4GalNT2, SDa) (14), were defined. Attempts have been made to overcome these new xenoantigens by developing new transgenic pigs (15–17). The triple KO (TKO) pigs, which eliminate α-Gal, Neu5Gc, and the SDa epitopes, exhibited reduced human antibody binding in vitro (18). Thus, TKO pigs became the backbone of any genetically engineered pig.
The second barrier is the presence of porcine endogenous retroviruses (PERVs), which are carried in almost all pigs. PERVs are harmless to pigs; however, potential pathogenicity to humans remains unclear. PERVs have been observed to be transmitted from pig to human and human to human in vitro (19). Since organ transplant recipients are under strong immunosuppression, it is desirable to avoid PERV infection. To this point, the generation of PERV-free pigs has been considered. It was considered impossible to inactivate all of the PERV genes before since PERV is integrated into multiple sites of the porcine genome. The median copy number of PERVs ranged between 46 and 70 copies, although some were found to have more than 100 copies (20). The CRISPR–Cas9 technology (21) enabled us to overcome this. Yang et al. inactivated all copies of the PERV gene from porcine somatic cells using CRISPR–Cas9 (22). They also generated PERV-free pigs from PERV gene-inactivated porcine somatic cells using somatic cell nuclear transfer (23). Xenotransplantation can be free from PERV infection risk by using PERV-free pigs as a donor.
To prevent xenograft rejection, new genetically engineered pigs have been developed based on GalT-KO pigs. While HAR to xenoantigens was humoral-mediated immunity triggered by already existing natural antibodies, cellular immunity is also involved in xenograft rejection. Human NK cells are considered to play an important role in cellular xenograft rejection (24). They are capable of lysing cells with reduced human major histocompatibility complex (MHC) class I expression. High expression of HLA-E, one of the MHC class I molecules, allows porcine cells to evade human NK cytotoxicity (25). Human CD47 expression in porcine cells prevents phagocytosis from human macrophages (26). To regulate complement activation, overexpression of human complement regulatory proteins (CRPs) was attempted (27, 28). Apart from the immune system, coagulation dysregulation is also involved in xenograft rejection. Human coagulation regulatory proteins were expressed to prevent human platelet aggregation in the vessels of the xenografts (29, 30). Finally, porcine organ size was optimized. A rapid increase in graft size has been reported when porcine organs are transplanted into NHPs (31). To solve this problem, pigs suitable for transplantation were generated by knocking out the receptors for growth hormones (32). Revivicor developed genetically engineered pigs with 10 genetic modifications (10-GE pigs). The 10-GE pigs have targeted insertion of six human genes, consisting of two human complement inhibitor genes (hDAF, hCD46), two human anticoagulant genes (hTBM, hEPCR), and two immunomodulatory genes (hCD47, hHO1), as well as KO of three porcine xenoantigens (α-Gal, Neu5Gc, and SDa) and the porcine growth hormone receptor gene. It became possible to generate pigs with multiple gene modifications by gene editing and nuclear transfer, thereby overcoming the various problems that hampered xenotransplantation.
As a result of further development of genetically engineered pigs, many pig-to-NHP transplantations were performed as the final step toward preclinical studies. Among them, a pig-to-baboon heart transplant experiment at the U.S. National Institutes of Health (NIH) reported a long survival time of up to 945 days (33). In 2020, the U.S. Food and Drug Administration (FDA) approved the GalT-KO pigs, the platforms for all transgenic pigs, as a source of human therapeutics including xenotransplantation. In 2022, the University of Alabama reported that a kidney derived from a genetically modified pig was transplanted into a brain-dead decedent (34), which marked the first clinical application of xenotransplantation from a pig to a human. The 10-GE pig kidneys were transplanted into a man who was brain dead from head trauma after bilateral nephrectomy. No HAR and PERV transmission were observed. Although the porcine kidney produced urine within 30 min, serum creatinine did not decrease during the study period. Histological findings on postoperative periods demonstrated endothelial injury with diffuse thrombotic microangiopathy. The decedent’s general condition deteriorated after transplantation, leading to progressive multiple organ failure. Thus, the study was terminated only after 3 days. Further study, including the mechanisms and prevention of microvascular injuries, is required to uncover the etiology of poor renal recovery.
Pig islet transplantation into a human has a longer history than organ transplantation in xenotransplantation (35–37). Transplantation of encapsulated wild-type porcine islets to established brittle type 1 diabetes mellitus was performed without immunosuppression, which resulted in improved HbA1c and reduced unaware hypoglycemic events (37). However, only a slight decrease in insulin doses was observed, and no patient could achieve insulin independence. Aside from encapsulated islet transplantation, there have been attempts to transplant naked porcine islets into NHPs. Xenotransplantation of neonatal porcine islets from α1,3GalT KO piglets into immunosuppressed STZ-induced diabetic rhesus monkeys resulted in reduced complement-mediated rejection (38). Various genetically engineered pigs have been developed to protect pig islets from the host’s immune responses. However, there are still problems regarding effectiveness and long-term survival. Future studies will reveal whether the new genetically engineered pigs, including the 10-GE pigs, will solve these problems.
The other approach to solve organ shortage is by creating a human organ from human pluripotent stem cells (PSCs) in an animal body by using interspecies blastocyst complementation (BC). In this approach, the patient’s own induced PSCs (iPSCs) are injected into the targeted organ-deficient animal’s preimplantation embryos, and then the defective organs are completely replaced by donor cells when the embryo develops to full term. It means that, when the technology is realized for humans, a BC-derived organ is expected to be functional and rejection-free (39) (Figure 1).
Figure 1 Differences between xenotransplantation and animal-developed human organ transplantation by using blastocyst complementation. In xenotransplantation (top), a small percentage of the xenografts have proteins derived from the human transgene, but the majority are of porcine origin. On the other hand, the blastocyst complementation (bottom) generates organs almost completely of human origin in a specific organ-deficient animal. Cartoon pictures (human transgene, pig, and human) were created with BioRender.
The concept of BC was first reported in lymphocyte complementation by using Rag2-KO mouse embryos (40). Chen et al. indicated that B- and T-lymphocyte deficiencies in Rag2 KO were fully covered by donor cells in wild-type mouse embryonic stem cell (mESC)-injected chimeras. They also complemented Rag2 KO blastocysts with mESCs lacking immunoglobulin heavy-chain joining (IGHJ) gene segment, and the chimeras had normal T cells but no B cells. This result demonstrated that the IGHJ gene segment is essential for B-cell development. As described, Chen and colleagues proposed BC as a functional assay to clarify the definitive role of targeted genes in development.
BC was firstly proposed as a technology to generate a whole organ with donor PSCs by Kobayashi etal. (41) (Figure 2). The first targeted organ was the pancreas because of the medical needs for pancreatic islet, and the apancreatic phenotype can be induced by the single-gene KO of Pdx1 (pancreatic and duodenal homeobox1) (42). Not only in an allogeneic setting but also in a rat–mouse interspecies setting, the pancreas was entirely complemented with donor cells in Pdx1−/− mice. Furthermore, these chimeras derived from Pdx1−/− mouse embryos grew to adulthood, maintained normal blood glucose levels, and secreted insulin in response to glucose tolerance tests (GTTs). These results indicated that donor PSCs can compensate for a defective organ niche and form functional organs even in interspecies chimeras. A reciprocal experiment was then performed for islet transplantation by generating rat-sized mouse pancreas in Pdx1−/− rat-derived interspecies chimeras (43). Mouse PSC-derived pancreas in mouse–rat chimera can maintain blood glucose level and respond to high glucose in GTT, albeit with a slightly delayed response compared to wild-type rats. One hundred mouse islets were isolated from Pdx1−/− interspecies chimeric rats and transplanted into the kidney capsule of STZ-induced diabetic mice. Although immunosuppression was stopped 5 days after transplantation, diabetic mice that received mPSC-derived islets exhibited normal blood glucose levels and normal responses to GTTs for over 1 year without continued immunosuppression. Flow cytometric analysis revealed that a substantial proportion of rat-supporting tissues were contaminated with the graft; however, the host immune system specifically eliminated contaminated xenogeneic cells without inducing HAR or damaging mouse insulin-producing cells.
Figure 2 Blastocyst complementation using organ-deficient mice. Blastocyst complementation using Pdx1−/− pancreas-deficient mice. When wild-type pluripotent stem cells (PSCs) are injected into blastocysts derived from a genetically pancreas-deficient mouse, the injected PSCs can complement an empty developmental niche for a pancreas. Whereas the skin, tissues, and organs throughout the body of the chimeric mouse are composed of a mixture of PSCs and host embryos, the pancreas is composed entirely of cells derived from the donor PSCs. The lower right is a photograph of an interspecific chimera. This Pdx1−/− mouse complemented with rat PSCs has a pancreas derived from rat PSCs.
In addition to the pancreas, many other organs have been complemented via BC, such as the kidney (44, 45), thymus (46), blood and vascular endothelial cells (47), lungs and bronchi (48), and gametes (49). BC-derived organ generation can be applicable to pigs (50), which is a great advantage in terms of realization because pigs have similar-sized organs to humans and have multiple pregnancies.
These results demonstrate that most organs or tissues can be generated by interspecies BC, and they are functional and expected not to cause HAR for as long as the contaminated xenogeneic cell portion is relatively small.
After the success of interspecies chimera formation in rodents (41, 43, 51), the generation of human PSC-derived interspecies chimeras has been attempted to evaluate its developmental potency and assess the possibility of human organ derivation. However, the contribution of human cells in interspecies chimera was very low in all the studies, and none of them showed significant donor chimerism in neonates or adults (52–56). Donor rat cell chimerism was about 20%–25% in most of the cases of rat–mouse interspecies chimeras (41). Donor human cell chimerism in human–mouse interspecies chimeras was reported to be 0.1%–4.0% (57), while higher results have been reported for human–pig interspecies chimeras (58). Nevertheless, it must be noted that the efficiency of human–animal chimeras is still low. While rats and mice diverged about 20 million years ago, humans diverged from mice and pigs nearly 100 million years ago (59). Such evolutional divergence of developmental molecules and systems might have prevented human cell contribution to host animal development.
Developmental stage mismatch between the host animal embryo and the donor human PSC would be the other reason for the difficulty in forming human–animal chimeras. Mouse ESCs and human ESCs are both derived from the preimplantation epiblast, but human conventional ESCs (and iPSCs) carry characteristics more common to postimplantation epiblast-derived mouse EpiSCs (60, 61). PSCs that resembled to preimplantation epiblast are called “naive” PSCs, and postimplantation epiblast-like PSCs are called “primed” PSCs (62). Mouse EpiSCs do not form chimeras when injected into preimplantation blastocysts, but they do form chimeras when implanted into postimplantation gastrula (63). This indicates that a certain level of developmental stage matching is required for chimera formation. Recently, the establishment of human naive-like PSCs has been reported (56, 64, 65). Although injected human naive PSCs showed poor contribution to animal development (52, 56), they might show difference to the conventional human PSCs when injected into evolutionally more closer species embryos, such as NHPs (66).
The other approach is the prevention of apoptosis in donor human primed PSCs. Our group previously reported that developmental stage-mismatched cells cause apoptosis when injected into preimplantation embryos. By blocking apoptosis in those mismatched donor cells, even the stage-mismatched cells could contribute to chimeras (67). Recently, it was confirmed that apoptosis-resistant human primed PSCs survive in mouse fetus (54, 55). However, only a few numbers of human cells survived in the chimeric embryos, and their contribution to organogenesis has not been reported. To overcome too low chimerism of donor human cells for organ complementation, our group developed the procedure, which allows a gradual increase of donor cell chimerism in later developmental stages by deleting the Igf1 receptor (Igf1r) of host cells in chimeras (68). By using the Igf1r KO animal embryos as a host, the progenies of human PSCs might increase their chimerism in the animal body and then complement the targeted organ or tissues.
In contrast to the successful results within rodents, human organ generation using BC has not been achieved yet. Multiple factors may need to be considered in future studies of generating human–animal chimeras, including the selection of animal species, gene editing of embryos and human PSC, and culture conditions for human PSCs.
This article introduced two concepts as approaches to transplant organ creation: xenotransplantation and interspecies BC. With xenotransplantation of pig-derived organs, many efforts have been made to overcome its major problem with rejection. Hyperacute rejection has been avoided through the use of gene editing techniques, but it is still not certain whether long-term engraftment in the human body is possible. Furthermore, it is unclear whether pig organs will be able to perform the same physiological functions as human organs. Another concern is the adverse effects of strong immunosuppression of the patient to reduce rejection. On the other hand, interspecies chimera-derived human organ transplantation aims to generate organs derived from the patient’s own iPSCs, which are autologous transplants, so the rejection is expected to be none or mild. Thus, there would be no need for immunosuppression. It has been suggested that the differences in the insulin secretion capacity and insulin demands to food intake between humans and pigs may be a problem in pig islet transplantation (69). In this regard, islets made from human PSCs by BC would not have this problem. However, further study is necessary to address the issue of the difficulty of human PSCs to contribute to animal embryos in the early developmental stage. Contaminated host animal cells, such as blood vessels, might cause rejection after transplantation. We previously indicated that those contaminated xenogeneic cells were cleared by the recipient’s immune system in BC-derived pancreatic islet transplantation (43); however, this immune reaction could trigger more intense rejection in orthotropic whole-organ transplantation. To solve this problem, vascular endothelial cell complementation (47, 70) along with targeted organ complementation might be required.
After all, these two types of approaches are not contradictory but rather synergistic; for example, genetically modified animals designed for xenotransplantation could be useful as a host for interspecies chimera-derived human organ as they carried xenogeneic endothelial cells which are less immunogenic to the human immune system. Furthermore, the utilization of PERV-free pigs may provide the ideal host for clinical interspecies organogenesis to prevent PERV transmission from host pig cells. Whichever method is chosen, the future direction of transplantation medicine using animals is to allow for a safe and practical method of growing organs in donor animals as well as saving many patients suffering from irreversible organ failures, including insulin-dependent diabetes mellitus. Just as science has solved many obstacles in the past, we believe that future research developments will lead to the realization of animal-based transplantation medicine.
MK, EM, and HN conceived the study. MK wrote the manuscript. EM, HM, and SH revised the manuscript. All authors contributed to the article and approved the submitted version.
This work was supported by grants from the Centers for Clinical Application Research on Specific Disease/Organ (to HN) of the Research Center Network for Realization of Regenerative Medicine, funded by the Japan Agency for Medical Research and Development (AMED) under Grant Number JP22bm1004002; Grant-in-Aid for Early-Career Scientists (to MK), funded by the Japan Society for the Promotion of Science (JSPS) KAKENHI under Grant Number JP21K16337.
We thank Dr. M. Watanabe for critical advice in preparing the manuscript and K. Okada for secretarial support. The authors used BioRender in the creation of the figure.
HN is a cofounder and shareholder in ReproCELL, Megakaryon, and Century Therapeutics.
The remaining authors declare that the research was conducted in the absence of any commercial or financial relationships that could be construed as a potential conflict of interest.
All claims expressed in this article are solely those of the authors and do not necessarily represent those of their affiliated organizations, or those of the publisher, the editors and the reviewers. Any product that may be evaluated in this article, or claim that may be made by its manufacturer, is not guaranteed or endorsed by the publisher.
1. Robertson RP. Seminars in medicine of the Beth Israel hospital, Boston: Pancreatic and islet transplantation for diabetes–cures or curiosities? N Engl J Med (1992) 327:1861–8. doi: 10.1056/NEJM199212243272607
2. Barrou Z, Seaquist ER, Robertson RP. Pancreas transplantation in diabetic humans normalizes hepatic glucose production during hypoglycemia. Diabetes (1994) 43:661–6. doi: 10.2337/diab.43.5.661
3. Douzdjian V, Ferrara D, Silvestri G. Treatment strategies for insulin-dependent diabetics with esrd: A cost-effectiveness decision analysis model. Am J Kidney Dis (1998) 31:794–802. doi: 10.1016/S0272-6386(98)70048-4
4. Pw W. Notes on diabetes treated with extract and by grafts of sheep’s pancreas. BMJ (1894) 2:1303.
5. Deschamps JY, Roux FA, Sat P, Gouin E. History of xenotransplantation. Xenotransplantation (2005) 12:91–109. doi: 10.1111/j.1399-3089.2004.00199.x
6. Good AH, Cooper DK, Malcolm AJ, Ippolito RM, Koren E, Neethling FA, et al. Identification of carbohydrate structures that bind human antiporcine antibodies: Implications for discordant xenografting in humans. Transplant Proc (1992) 24:559–62.
7. Galili U. Interaction of the natural anti-gal antibody with alpha-galactosyl epitopes: A major obstacle for xenotransplantation in humans. Immunol Today (1993) 14:480–2. doi: 10.1016/0167-5699(93)90261-I
8. Yang Y-G, Sykes M. Xenotransplantation: Current status and a perspective on the future. Nat Rev Immunol (2007) 7:519–31. doi: 10.1038/nri2099
9. Dai Y, Vaught TD, Boone J, Chen S-H, Phelps CJ, Ball S, et al. Targeted disruption of the Α1,3-galactosyltransferase gene in cloned pigs. Nat Biotechnol (2002) 20:251–5. doi: 10.1038/nbt0302-251
10. Lai L, Kolber-Simonds D, Park KW, Cheong HT, Greenstein JL, Im GS, et al. Production of alpha-1,3-Galactosyltransferase knockout pigs by nuclear transfer cloning. Science (2002) 295:1089–92. doi: 10.1126/science.1068228
11. Phelps CJ, Koike C, Vaught TD, Boone J, Wells KD, Chen SH, et al. Production of alpha 1,3-Galactosyltransferase-Deficient pigs. Science (2003) 299:411–4. doi: 10.1126/science.1078942
12. Kolber-Simonds D, Lai L, Watt SR, Denaro M, Arn S, Augenstein ML, et al. Production ofα-1,3-Galactosyltransferase null pigs by means of nuclear transfer with fibroblasts bearing loss of heterozygosity mutations. Proc Natl Acad Sci (2004) 101:7335–40. doi: 10.1073/pnas.0307819101
13. Song K-H, Kang Y-J, Jin U-H, Park Y-I, Kim S-M, Seong H-H, et al. Cloning and functional characterization of pig cmp-N-Acetylneuraminic acid hydroxylase for the synthesis of n-glycolylneuraminic acid as the xenoantigenic determinant in pig–human xenotransplantation. Biochem J (2010) 427:179–88. doi: 10.1042/BJ20090835
14. Byrne G, Ahmad-Villiers S, Du Z, Mcgregor C. B4galnt2 and xenotransplantation: A newly appreciated xenogeneic antigen. Xenotransplantation (2018) 25:e12394. doi: 10.1111/xen.12394
15. Lutz AJ, Li P, Estrada JL, Sidner RA, Chihara RK, Downey SM, et al. Double knockout pigs deficient in n-glycolylneuraminic acid and galactose α-1,3-Galactose reduce the humoral barrier to xenotransplantation. Xenotransplantation (2013) 20:27–35. doi: 10.1111/xen.12019
16. Adams AB, Kim SC, Martens GR, Ladowski JM, Estrada JL, Reyes LM, et al. Xenoantigen deletion and chemical immunosuppression can prolong renal xenograft survival. Ann Surgery. (2018) 268:564–73. doi: 10.1097/SLA.0000000000002977
17. Li P, Estrada JL, Burlak C, Montgomery J, Butler JR, Santos RM, et al. Efficient generation of genetically distinct pigs in a single pregnancy using multiplexed single-guide rna and carbohydrate selection. Xenotransplantation (2015) 22:20–31. doi: 10.1111/xen.12131
18. Estrada JL, Martens G, Li P, Adams A, Newell KA, Ford ML, et al. Evaluation of human and non-human primate antibody binding to pig cells lacking Ggta1/Cmah/β4galnt2 genes. Xenotransplantation (2015) 22:194–202. doi: 10.1111/xen.12161
19. Denner J, Specke V, Thiesen U, Karlas A, Kurth R. Genetic alterations of the long terminal repeat of an ecotropic porcine endogenous retrovirus during passage in human cells. Virology (2003) 314:125–33. doi: 10.1016/S0042-6822(03)00428-8
20. Fiebig U, Fischer K, Bähr A, Runge C, Schnieke A, Wolf E, et al. Porcine endogenous retroviruses: Quantification of the copy number in cell lines, pig breeds, and organs. Xenotransplantation (2018) 25:e12445. doi: 10.1111/xen.12445
21. Jinek M, Chylinski K, Fonfara I, Hauer M, Doudna JA, Charpentier E. A programmable dual-Rna-Guided dna endonuclease in adaptive bacterial immunity. Science (2012) 337:816–21. doi: 10.1126/science.1225829
22. Yang L, Güell M, Niu D, George H, Lesha E, Grishin D, et al. Genome-wide inactivation of porcine endogenous retroviruses (Pervs). Science (2015) 350:1101–4. doi: 10.1126/science.aad1191
23. Niu D, Wei H-J, Lin L, George H, Wang T, Lee I-H, et al. Inactivation of porcine endogenous retrovirus in pigs using crispr-Cas9. Science (2017) 357:1303–7. doi: 10.1126/science.aan4187
24. Seebach JD, Waneck GL. Natural killer cells in xenotransplantation. Xenotransplantation (1997) 4(4):201–11. doi: 10.1111/j.1399-3089.1997.tb00184.x
25. Lilienfeld BG, Crew MD, Forte P, Baumann BC, Seebach JD. Transgenic expression of hla-e single chain trimer protects porcine endothelial cells against human natural killer cell-mediated cytotoxicity. Xenotransplantation (2007) 14:126–34. doi: 10.1111/j.1399-3089.2007.00378.x
26. Ide K, Wang H, Tahara H, Liu J, Wang X, Asahara T, et al. Role for Cd47-Sirpα signaling in xenograft rejection by macrophages. Proc Natl Acad Sci (2007) 104:5062–6. doi: 10.1073/pnas.0609661104
27. Burdorf L, Stoddard T, Zhang T, Rybak E, Riner A, Avon C, et al. Expression of human Cd46 modulates inflammation associated with galtko lung xenograft injury. Am J Transplant (2014) 14:1084–95. doi: 10.1111/ajt.12673
28. Liu F, Liu J, Yuan Z, Qing Y, Li H, Xu K, et al. Generation of gtko diannan miniature pig expressing human complementary regulator proteins Hcd55 and Hcd59 via T2a peptide-based bicistronic vectors and scnt. Mol Biotechnol (2018) 60:550–62. doi: 10.1007/s12033-018-0091-6
29. Iwase H, Ekser B, Hara H, Phelps C, Ayares D, Cooper DKC, et al. Regulation of human platelet aggregation by genetically modified pig endothelial cells and thrombin inhibition. Xenotransplantation (2014) 21:72–83. doi: 10.1111/xen.12073
30. Bae J-S, Yang L, Rezaie AR. Receptors of the protein c activation and activated protein c signaling pathways are colocalized in lipid rafts of endothelial cells. Proc Natl Acad Sci (2007) 104:2867–72. doi: 10.1073/pnas.0611493104
31. Iwase H, Ball S, Adams K, Eyestone W, Walters A, Cooper DKC. Growth hormone receptor knockout: Relevance to xenotransplantation. Xenotransplantation (2021) 28:E12652. doi: 10.1111/xen.12652
32. Hinrichs A, Riedel EO, Klymiuk N, Blutke A, Kemter E, Längin M, et al. Growth hormone receptor knockout to reduce the size of donor pigs for preclinical xenotransplantation studies. Xenotransplantation (2021) 28:e12664. doi: 10.1111/xen.12664
33. Mohiuddin MM, Singh AK, Corcoran PC, Thomas Iii ML, Clark T, Lewis BG, et al. Chimeric 2c10r4 anti-Cd40 antibody therapy is critical for long-term survival of Gtko.Hcd46.Htbm pig-To-Primate cardiac xenograft. Nat Commun (2016) 7:11138. doi: 10.1038/ncomms11138
34. Porrett PM, Orandi BJ, Kumar V, Houp J, Anderson D, Cozette Killian A, et al. First clinical-grade porcine kidney xenotransplant using a human decedent model. Am J Transplant (2022) 22:1037–53. doi: 10.1111/ajt.16930
35. Groth CG, Tibell A, Tollemar J, Bolinder J, Östman J, Möller E, et al. Transplantation of porcine fetal pancreas to diabetic patients. Lancet (1994) 344(8394):1402–4. doi: 10.1016/S0140-6736(94)90570-3
36. Matsumoto S, Tan P, Baker J, Durbin K, Tomiya M, Abalovich K, et al. Clinical porcine islet xenotransplantation under comprehensive regulation. Transplantat Proc (2014) 46(6):1992–5. doi: 10.1016/j.transproceed.2014.06.008
37. Matsumoto S, Abalovich A, Wechsler C, Wynyard S, Elliott RB. Clinical Benefit of Islet Xenotransplantation for the Treatment of Type 1 Diabetes. EBioMedicine (2016) 12:255–62. doi: 10.1016/j.ebiom.2016.08.034
38. Thompson P, Badell IR, Lowe M, Cano J, Song M, Leopardi F, et al. Islet xenotransplantation using gal-deficient neonatal donors improves engraftment and function. Am J Of Transplant (2011) 11:2593–602. doi: 10.1111/j.1600-6143.2011.03720.x
39. Elisseeff J, Badylak SF, Boeke JD. Immune and genome engineering as the future of transplantable tissue. New Engl J Of Med (2021) 385:2451–62. doi: 10.1056/NEJMra1913421
40. Chen J, Lansford R, Stewart V, Young F, Alt FW. Rag-2-Deficient blastocyst complementation: An assay of gene function in lymphocyte development. Proc Natl Acad Sci U.S.A. (1993) 90:4528–32. doi: 10.1073/pnas.90.10.4528
41. Kobayashi T, Yamaguchi T, Hamanaka S, Kato-Itoh M, Yamazaki Y, Ibata M, et al. Generation of rat pancreas in mouse by interspecific blastocyst injection of pluripotent stem cells. Cell (2010) 142:787–99. doi: 10.1016/j.cell.2010.07.039
42. Offield MF, Jetton TL, Labosky PA, Ray M, Stein RW, Magnuson MA, et al. Pdx-1 is required for pancreatic outgrowth and differentiation of the rostral duodenum. Development (1996) 122:983–95. doi: 10.1242/dev.122.3.983
43. Yamaguchi T, Sato H, Kato-Itoh M, Goto T, Hara H, Sanbo M, et al. Interspecies organogenesis generates autologous functional islets. Nature (2017) 542:191–6. doi: 10.1038/nature21070
44. Usui J, Kobayashi T, Yamaguchi T, Knisely AS, Nishinakamura R, Nakauchi H. Generation of kidney from pluripotent stem cells via blastocyst complementation. Am J Pathol (2012) 180:2417–26. doi: 10.1016/j.ajpath.2012.03.007
45. Goto T, Hara H, Sanbo M, Masaki H, Sato H, Yamaguchi T, et al. Generation of pluripotent stem cell-derived mouse kidneys in Sall1-targeted anephric rats. Nat Commun (2019) 10:451. doi: 10.1038/s41467-019-08394-9
46. Isotani A, Hatayama H, Kaseda K, Ikawa M, Okabe M. Formation of a thymus from rat Es cells in xenogeneic nude Mouse↔Rat Es chimeras. Genes Cells (2011) 16:397–405. doi: 10.1111/j.1365-2443.2011.01495.x
47. Hamanaka S, Umino A, Sato H, Hayama T, Yanagida A, Mizuno N, et al. Generation of vascular endothelial cells and hematopoietic cells by blastocyst complementation. Stem Cell Rep (2018) 11:988–97. doi: 10.1016/j.stemcr.2018.08.015
48. Mori M, Furuhashi K, Danielsson JA, Hirata Y, Kakiuchi M, Lin CS, et al. Generation of functional lungs via conditional blastocyst complementation using pluripotent stem cells. Nat Med (2019) 25:1691–8. doi: 10.1038/s41591-019-0635-8
49. Kobayashi T, Goto T, Oikawa M, Sanbo M, Yoshida F, Terada R, et al. Blastocyst complementation using Prdm14-deficient rats enables efficient germline transmission and generation of functional mouse spermatids in rats. Nat Commun (2021) 12:1328. doi: 10.1038/s41467-021-21557-x
50. Matsunari H, Nagashima H, Watanabe M, Umeyama K, Nakano K, Nagaya M, et al. Blastocyst complementation generates exogenic pancreas In vivo in apancreatic cloned pigs. Proc Natl Acad Sci (2013) 110:4557–62. doi: 10.1073/pnas.1222902110
51. Honda A, Choijookhuu N, Izu H, Kawano Y, Inokuchi M, Honsho K, et al. Flexible adaptation of Male germ cells from female ipscs of endangered tokudaia osimensis. Sci Adv (2017) 3:e1602179. doi: 10.1126/sciadv.1602179
52. Wu J, Platero-Luengo A, Sakurai M, Sugawara A, Gil MA, Yamauchi T, et al. Interspecies chimerism with mammalian pluripotent stem cells. Cell (2017) 168:473–486.E15. doi: 10.1016/j.cell.2016.12.036
53. Zheng C, Hu Y, Sakurai M, Pinzon-Arteaga CA, Li J, Wei Y, et al. Cell competition constitutes a barrier for interspecies chimerism. Nature (2021) 592:272–6. doi: 10.1038/s41586-021-03273-0
54. Huang K, Zhu Y, Ma Y, Zhao B, Fan N, Li Y, et al. Bmi1 enables interspecies chimerism with human pluripotent stem cells. Nat Commun (2018) 9:4649. doi: 10.1038/s41467-018-07098-w
55. Wang X, Li T, Cui T, Yu D, Liu C, Jiang L, et al. Human embryonic stem cells contribute to embryonic and extraembryonic lineages in mouse embryos upon inhibition of apoptosis. Cell Res (2018) 28:126–9. doi: 10.1038/cr.2017.138
56. Theunissen TW, Friedli M, He Y, Planet E, O'neil RC, Markoulaki S, et al. Molecular criteria for defining the naive human pluripotent state. Cell Stem Cell (2016) 19:502–15. doi: 10.1016/j.stem.2016.06.011
57. Zhang B, Li H, Hu Z, Jiang H, Stablewski AB, Marzullo BJ, et al. Generation of mouse–human chimeric embryos. Nat Protoc (2021) 16:3954–80. doi: 10.1038/s41596-021-00565-7
58. Das S, Koyano-Nakagawa N, Gafni O, Maeng G, Singh BN, Rasmussen T, et al. Generation of human endothelium in pig embryos deficient in Etv2. Nat Biotechnol (2020) 38:297–302. doi: 10.1038/s41587-019-0373-y
59. Suchy F, Nakauchi H. Interspecies chimeras. Curr Opin Genet Dev (2018) 52:36–41. doi: 10.1016/j.gde.2018.05.007
60. Brons IG, Smithers LE, Trotter MW, Rugg-Gunn P, Sun B, Chuva De Sousa Lopes SM, et al. Derivation of pluripotent epiblast stem cells from mammalian embryos. Nature (2007) 448:191–5. doi: 10.1038/nature05950
61. Tesar PJ, Chenoweth JG, Brook FA, Davies TJ, Evans EP, Mack DL, et al. New cell lines from mouse epiblast share defining features with human embryonic stem cells. Nature (2007) 448:196–9. doi: 10.1038/nature05972
62. Nichols J, Smith A. Naive and primed pluripotent states.. Cell Stem Cell (2009) 4(6):487–92. doi: 10.1016/j.stem.2009.05.015
63. Huang Y, Osorno R, Tsakiridis A, Wilson V. In vivo differentiation potential of epiblast stem cells revealed by chimeric embryo formation. Cell Rep (2012) 2:1571–8. doi: 10.1016/j.celrep.2012.10.022
64. Takashima Y, Guo G, Loos R, Nichols J, Ficz G, Krueger F, et al. Resetting transcription factor control circuitry toward ground-state pluripotency in human. Cell (2014) 158:1254–69. doi: 10.1016/j.cell.2014.08.029
65. Guo G, Von Meyenn F, Rostovskaya M, Clarke J, Dietmann S, Baker D, et al. Epigenetic resetting of human pluripotency. Development (2017) 144:2748–63. doi: 10.1242/dev.146811
66. Tan T, Wu J, Si C, Dai S, Zhang Y, Sun N, et al. Chimeric contribution of human extended pluripotent stem cells to monkey embryos ex vivo. Cell (2021) 184:2020–2032.E14. doi: 10.1016/j.cell.2021.03.020
67. Masaki H, Kato-Itoh M, Takahashi Y, Umino A, Sato H, Ito K, et al. Inhibition of apoptosis overcomes stage-related compatibility barriers to chimera formation in mouse embryos. Cell Stem Cell (2016) 19:587–92. doi: 10.1016/j.stem.2016.10.013
68. Nishimura T, Suchy FP, Bhadury J, Igarashi KJ, Charlesworth CT, Nakauchi H. Generation of functional organs using a cell-competitive niche in intra- and inter-species rodent chimeras. Cell Stem Cell (2021) 28:141–149.e3. doi: 10.1016/j.stem.2020.11.019
69. Graham ML, Bellin MD, Papas KK, Hering BJ, Schuurman H-J. Species incompatibilities in the pig-to-Macaque islet xenotransplant model affect transplant outcome: A comparison with allotransplantation. Xenotransplantation (2011) 18:328–42. doi: 10.1111/j.1399-3089.2011.00676.x
Keywords: xenotransplantation, blastocyst complementation, pluripotent stem cells, chimera, type 1 diabetes, pancreas, islet
Citation: Kano M, Mizutani E, Homma S, Masaki H and Nakauchi H (2022) Xenotransplantation and interspecies organogenesis: current status and issues. Front. Endocrinol. 13:963282. doi: 10.3389/fendo.2022.963282
Received: 07 June 2022; Accepted: 06 July 2022;
Published: 05 August 2022.
Edited by:
Kazuhiko Yamada, Columbia University Irving Medical Center, United StatesReviewed by:
Munemasa Mori, Columbia University Irving Medical Center, United StatesCopyright © 2022 Kano, Mizutani, Homma, Masaki and Nakauchi. This is an open-access article distributed under the terms of the Creative Commons Attribution License (CC BY). The use, distribution or reproduction in other forums is permitted, provided the original author(s) and the copyright owner(s) are credited and that the original publication in this journal is cited, in accordance with accepted academic practice. No use, distribution or reproduction is permitted which does not comply with these terms.
*Correspondence: Hiromitsu Nakauchi, bmFrYXVjaGlAc3RhbmZvcmQuZWR1; Hideki Masaki, bWFzYWtpaC5zY3RAdG1kLmFjLmpw
Disclaimer: All claims expressed in this article are solely those of the authors and do not necessarily represent those of their affiliated organizations, or those of the publisher, the editors and the reviewers. Any product that may be evaluated in this article or claim that may be made by its manufacturer is not guaranteed or endorsed by the publisher.
Research integrity at Frontiers
Learn more about the work of our research integrity team to safeguard the quality of each article we publish.