- 1Department of Microbiology and Immunology, University of Rochester Medical Center, Rochester, NY, United States
- 2Center for Musculoskeletal Research, University of Rochester Medical Center, Rochester, NY, United States
- 3Department of Orthopedics, University of Rochester Medical Center, Rochester, NY, United States
The gut microbiota regulates multiple facets of host metabolism and immunity through the production of signaling metabolites, such as polyamines which are small organic compounds that are essential to host cell growth and lymphocyte activation. Polyamines are most abundant in the intestinal lumen, where their synthesis by the gut microbiota is influenced by microbiome composition and host diet. Disruption of the host gut microbiome in metabolic syndrome and obesity-related type 2 diabetes (obesity/T2D) results in potential dysregulation of polyamine synthesis. A growing body of evidence suggests that restoration of the dysbiotic gut microbiota and polyamine synthesis is effective in ameliorating metabolic syndrome and strengthening the impaired immune responses of obesity/T2D. In this review, we discuss existing studies on gut microbiome determinants of polyamine synthesis, polyamine production in obesity/T2D, and evidence that demonstrates the potential of polyamines as a nutraceutical in obesity/T2D hosts.
Introduction
More than 90% of diabetes is attributed to obesity and it is therefore referred to as obesity-related type 2 diabetes (obesity/T2D) (1). The majority of individuals with obesity/T2D are also diagnosed with metabolic syndrome, based on diagnostic criteria that includes increased waist circumference (i.e., central obesity), elevated triglycerides, and abnormally high fasting glucose indicative of insulin resistance (2, 3). Based on predictions from 2007, the prevalence of diabetes is expected to reach 366 million worldwide by 2030 (4). However, recent epidemiological findings suggest 537 million individuals were already affected by obesity/T2D globally in 2021 (5), surpassing the original 2007 estimate (4). Parallel increases in obesity and type 2 diabetes are major public health concerns as both are associated with lower health-related quality of life (6, 7) such as adverse post-infection clinical outcomes (8–12). For example, both obesity and diabetes increase the risk for post-operative periprosthetic joint infections (PJIs) (9) and surgical site infections (SSIs) (10). During the COVID-19 pandemic, individuals with obesity/T2D were more likely to be hospitalized (11, 12) with some studies suggesting higher in-hospital mortality rates (13) likely due to increased incidence of cytokine storms preceding septic shock (12). Thus, obesity/T2D is linked to deficits in overall immunity that are associated with severe infections across body sites at higher mortality and morbidity rates compared to non-obese/T2D counterparts. This in part occurs due to chronic low-grade inflammation that is associated with a disturbance in gut microbiome composition and metabolism as compared to a healthy or homeostatic baseline known as gut dysbiosis (14–22).
It is well accepted that long-term exposure to prototypical Western diets characterized by highly processed foods is at the core of obesity/T2D-related metabolic syndrome (23–25) and chronic low-grade inflammation (26). The gut microbiome as the mediator linking diet and obesity has been established in multiple human and animal studies, with diet identified as the primary contributor to changes in gut microbiome diversity and functional capacity associated with obesity and impaired immunity (27–29). Metabolomic studies have identified several groups of gut microbiota metabolites associated with obesity/T2D, including short-chain fatty acids (30–33), triethylamine-N-oxide (34–36), and bile acids (30, 37–39), which regulate host metabolism and immunity. In this review, we focus on polyamines, a group of metabolites increasingly associated with obesity/T2D.
Polyamine metabolites are essential to mammalian health in multiple organs (40) where they regulate cellular metabolism, proliferation, and differentiation (40, 41). Despite extensive work on polyamines in cancer, autoimmune diseases, and aging, the role of polyamines in immunity remains obscure. More importantly, the role of polyamines in obesity/T2D immunity is not well-studied. Herein, we review the contribution of polyamine biosynthesis and function to obesity/T2D, host immunity, and evaluate new work that utilizes polyamines to mitigate metabolic syndrome and complications of obesity/T2D. Understanding the role of polyamines on immunity and metabolic diseases will lead to identification of novel, alternative therapeutics for immunocompromised obese/T2D patients.
Polyamine and obesity-related type 2 diabetes
The gut microbiota is a major contributor to the host polyamine pool
Polyamines are produced primarily from amino acid precursors arginine, ornithine, and methionine (42). Diamine putrescine, triamine spermine, and tetraamine spermidine are the most abundant natural polyamines found in mammals (43). The total polyamine reservoir in mammals is regulated endogenously by host cells (43) and exogenously by gut bacteria (44–47) and diet (48, 49). Putrescine, spermidine, and spermine exist in the micromolar to millimolar range in various foods (48, 49) and across body sites of healthy adults as summarized in Figure 1. The use of radiolabeled polyamines demonstrated that polyamines are readily absorbed and enter the circulation (48), where they are distributed across different organs and metabolic fluids, including the brain (50), kidneys (51), breastmilk (52–54), urine (55–58), and serum/plasma (55, 56, 59–62). Notably, the largest accumulation of polyamines occurs in the intestinal lumen as determined with fecal samples from healthy donors where putrescine is the most abundant followed by spermidine and spermine (63, 64). Fecal levels of polyamines have been shown to correlate with gut microbiota composition in humans (63–65) and rodent models (66, 67). Oral supplementation in rodents with arginine led to increased polyamine concentrations in feces in a dose-dependent manner, which was eliminated when these animals were treated with antibiotics prior to arginine administration (66). This implicates the role of the gut microbiota in amino acid metabolism that results in synthesis of polyamines in mammals. Additionally, both Gram-positive and Gram-negative bacteria isolated from human fecal samples, including Bifidobacterium, Clostridium, Enterococcus, and Lactobacillus, are able to produce polyamines in vitro (68). Of these genera, the abundance of Bifidobacterium animalis is decreased and Lactobacillus reuteri increased in obese individuals (69, 70). Together, these studies demonstrate that the gut microbiota is a major contributor to the total polyamine pool in vivo. Moreover, with the direct link between obesity/T2D and gut dysbiosis (71, 72), there is a compelling and urgent need to study host-microbiota-polyamine interactions and the contribution of polyamines in obesity/T2D.
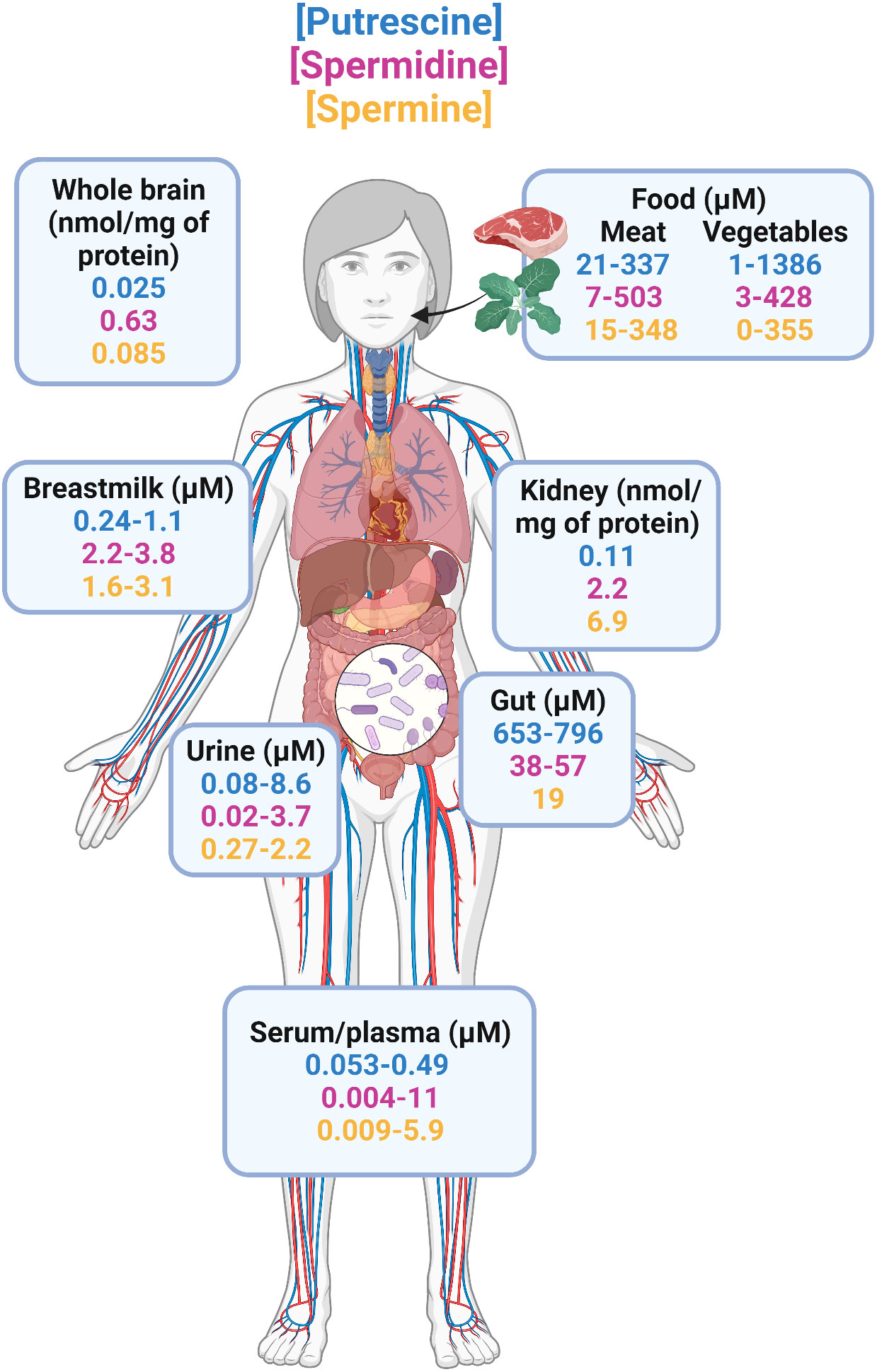
Figure 1 Distribution of natural polyamines in healthy adults. The three most abundant polyamines in humans are putrescine, spermidine, and spermine. Total polyamine is highest in the intestine because diet and gut microbiome are the primary sources of polyamines. From the gastrointestinal tract, polyamines are readily absorbed and enter circulation where they are found in a variety of organs including the brain, kidneys, and in metabolic fluids such as urine, and breastmilk. Studies that reported concentrations in ng/mL or μg/mL were transformed to μM using the molecular weights of putrescine (88.15 g/mol), spermidine (145.25 g/mol), and spermine (202.34 g/mol) for better comparisons.
Polyamine synthesis in obesity/type 2 diabetes
Thus far, there are only a handful of studies that have reported polyamine levels in obese individuals, all of which examined circulating polyamines in serum or plasma (73–75). In a study of 114 overweight/obese adults (BMI=27-40 kg/m2) with and without type 2 diabetes, it was concluded that individuals with obesity/T2D had elevated levels of putrescine as compared to obese, non-diabetic participants (73). This study did not have an additional control group of healthy adults but levels of putrescine (0.076μM), spermidine (0.15μM), and spermine (0.027μM) were in range when compared to previously reported levels in plasma/serum of healthy adults (putrescine = 0.053-0.49μM, spermidine = 0.004-11μM, spermine = 0.009-5.9μM) (55, 56, 59–62). In a second cohort of morbidly obese adults, the same group of authors noted increases in serum putrescine that was associated with failure to ameliorate metabolic syndrome six months after bariatric surgery (74). These results differ from a third study of 102 obese adults that demonstrated there were no differences circulating levels of putrescine, spermine, and spermidine in male vs female obese adults (76). Unfortunately, this study also did not have healthy adult controls but when compared to studies in healthy adults, only putrescine was elevated whereas spermine and spermidine were in the range of previously reported levels (55, 56, 59–62). More recently, spermidine was found to be positively associated with obesity in rural Chinese residents (75). However, these individuals were more likely to decrease their BMI over two years in a follow-up study (75). This suggests that spermidine exert protective effects against weight gain but of unknown mechanism. Large ranges can be seen across these studies illustrated in Figure 1. Variation in these studies likely originates from: i) different methods used to extract and analyze polyamines; e.g., HPLC was used in all obese/T2D studies whereas LCMS is considered to be more accurate and sensitive, ii) only including male participants (59), iii) not considering potential correlation between age and polyamines that has been previously identified (40, 63, 77). Therefore, although the majority of existing studies suggest polyamines are elevated in obesity/T2D, it is difficult to make substantial conclusions without control non-obese, non-diabetic healthy adults because exogenous polyamines have anti-obesity effects in preclinical studies (78–81). Additionally, patients with type 2 diabetes expressed reduced levels of polyamine synthetic enzymes such as ornithine decarboxylase that is necessary for putrescine production (82). Because these studies suggest an elevation in only putrescine when compared to healthy adults of other studies, it is important to identify changes in individual polyamine metabolites in future works. Note that these trends were also concluded from studies primarily in European or rural Chinese residents (59, 73–76). Additional studies are required in countries where obesity/T2D affect over 20% of the population including the United States, Mexico, Russia, and Brazil (83, 84).
Results from animal models of obesity-related diseases are also difficult to interpret due to the use of heterogenous models of obesity/T2D and sampling of tissues. Adipocytes from obese Zucker rats demonstrated a 4-fold increase in concentration of spermine and spermidine that was positively associated with adipose triacylglycerol formation (85). However, two-month-old leptin-deficient obese/T2D mice exhibited 31% less spermidine but 24% higher spermine in whole pancreatic islets (86). Obesity/T2D decreased expression of polyamine synthetic genes Odc (ornithine decarboxylase), Srm (spermidine synthase), and Sms (spermine synthase) that concomitantly resulted in a ~30% reduction in spermidine in the colon (87). These results differed from another study in diet-induced obese/T2D murine model that demonstrated no deficiency in polyamines due to obesity/T2D locally in the gut or systemically in plasma (88). Despite the variations in both humans and animal models of obesity/T2D, polyamines are essential to white adipose tissue homeostasis by stimulating adipocyte lipolysis (81) and the deletion of a spermidine to spermine conversion enzyme, spermidine/spermine N1-acetyltransferase, leads to late-onset obesity and insulin resistance (89–91).
Polyamines regulate host immunity
Polyamines regulate a variety of cellular functions in both innate and adaptive immune cells that are often described to be immunosuppressive (41). At neutral pH, polyamines exist as positively charged molecules that interact with negatively charged macromolecules such as DNA, RNA, and proteins (43). As a result, polyamines are necessary for molecular regulation of growth, autophagy, differentiation, and activation of lymphocytes. Polyamine depletion with enzyme inhibitors induced abnormal differentiation of cytolytic T lymphocytes (92) and caused defects in B-cell production of immunoglobulins (93). Both of these phenotypes were rescued upon exogenous addition of polyamines. T helper cells are the most well-studied when investigating the role of polyamines. Polyamines regulate CD4+ T cell differentiation as demonstrated by spermidine induction of Foxp3 expression to polarize naïve T cells to become regulatory T cells in an autophagy-dependent manner (94). In addition to Tregs, polyamines have been proposed to regulate transcription factors such as Tbx21 (T-Bet), Gata3, and Rorc that mediate CD4+ T cell differentiation into other subsets Th1, Th2, and Th17 epigenetically (95). This suggests that polyamines have significant impacts on adaptive immunity.
The role of polyamines in innate immune cells are less clearly defined. Polyamine synthesis is required for natural killer cell metabolism and effector function including the production of granzyme B and IFN-γ (96). In neutrophils, polyamines regulate effector functions by promoting superoxide and myeloperoxidase production. In healthy human neutrophils, depletion of polyamines treatment led to decreased production of superoxides (, H2O2) and release of myeloperoxidase (97). This is congruent with a second study demonstrating physiological concentrations of spermidine induced superoxide generation in human neutrophils stimulated with chemotactic peptide fMet-Leu-Phe (98). While the effects of polyamines are pro-inflammatory in natural killer cells and neutrophils, polyamines are immunosuppressive in monocytes/macrophages. Spermine inhibited synthesis of pro-inflammatory cytokines (TNF, IL-1, IL-6, MIP-1α, MIP-1β) in LPS-stimulated human peripheral blood mononuclear cells (99) and inhibited the production of nitric oxide in J774 murine macrophages stimulated with LPS or IFN-γ (100). Spermidine promoted hypusination of the eukaryotic translation factor eIF5A that switches macrophage metabolism to oxidative phosphorylation, a phenotype associated with M2-like anti-inflammatory macrophages (95, 101). However, depletion of polyamines in the same cell line induced nitric oxide synthesis when macrophages were stimulated with LPS (102). Thus, the exact role of polyamines in immune cells, particularly innate immune cells, remains unclear. It is particularly interesting to investigate the role of polyamines in innate immune cell dysfunction in obesity/T2D.
Polyamines as a nutraceutical
Polyamines as a nutraceutical for metabolic syndrome in obesity/T2D
Several studies have demonstrated beneficial effects of increased polyamine concentrations in correcting the metabolic complications of obesity/T2D (78–81, 87, 103). The total host polyamine pool can be increased either with diet (Figure 1) or by supplementation with probiotics or prebiotics which increase the abundance of gut bacteria that synthesize polyamines (44–47, 68). Bifidobacterium spp. are often regarded as beneficial bacteria due to their production of short-chain fatty acids, which has a myriad of physiological effects on the host (104). More recently, Bifidobacterium spp. have also been linked to polyamine synthesis in animal models of obesity/T2D and importantly, improved clinical outcomes (67, 87, 88). In a diet-induced model of obesity/T2D, mice fed Bifidobacterium animalis subsp. lactis for 12 weeks rescued polyamine production with a concomitant correction of lipid and glucose metabolism, reduction in metabolic endotoxemia, and strengthening of gut barrier function (87). Similarly, administration of B. lactis with arginine, an amino acid precursor to polyamine synthesis, resulted in increased levels of circulating and colonic levels of polyamines that correlated with reduced inflammation in senescent mice (67). Furthermore, in another diet-induced obese/T2D mouse model, supplementation with oligofructose, a bifidogenic indigestible carbohydrate, led to increases in abundance of B. pseudolongum that was associated with elevation of bacteria-specific polyamine precursor acetyl-ornithine and down-stream levels of spermine and spermidine (88).
Together, these studies demonstrated that restoration of polyamine synthesis by B. lactis and B. pseudolongum ameliorated complications associated with obesity/T2D. Moreover, direct administration of polyamines reduced body weight, adipocyte differentiation, and lipid accumulation in obese mice (78–81). Enhancement of polyamine synthetic enzyme, SAT1, by triethylenetetramine dihydrochloride was also associated with anti-obesity and anti-diabetic effects in mice (103). There is an ongoing need to study the direct effects of polyamines on development of diabetes. In summary, these studies provide evidence that polyamines are integral to regulating metabolic syndrome and illustrate its potential as a nutraceutical for metabolic complications of obesity/T2D (Figure 2).
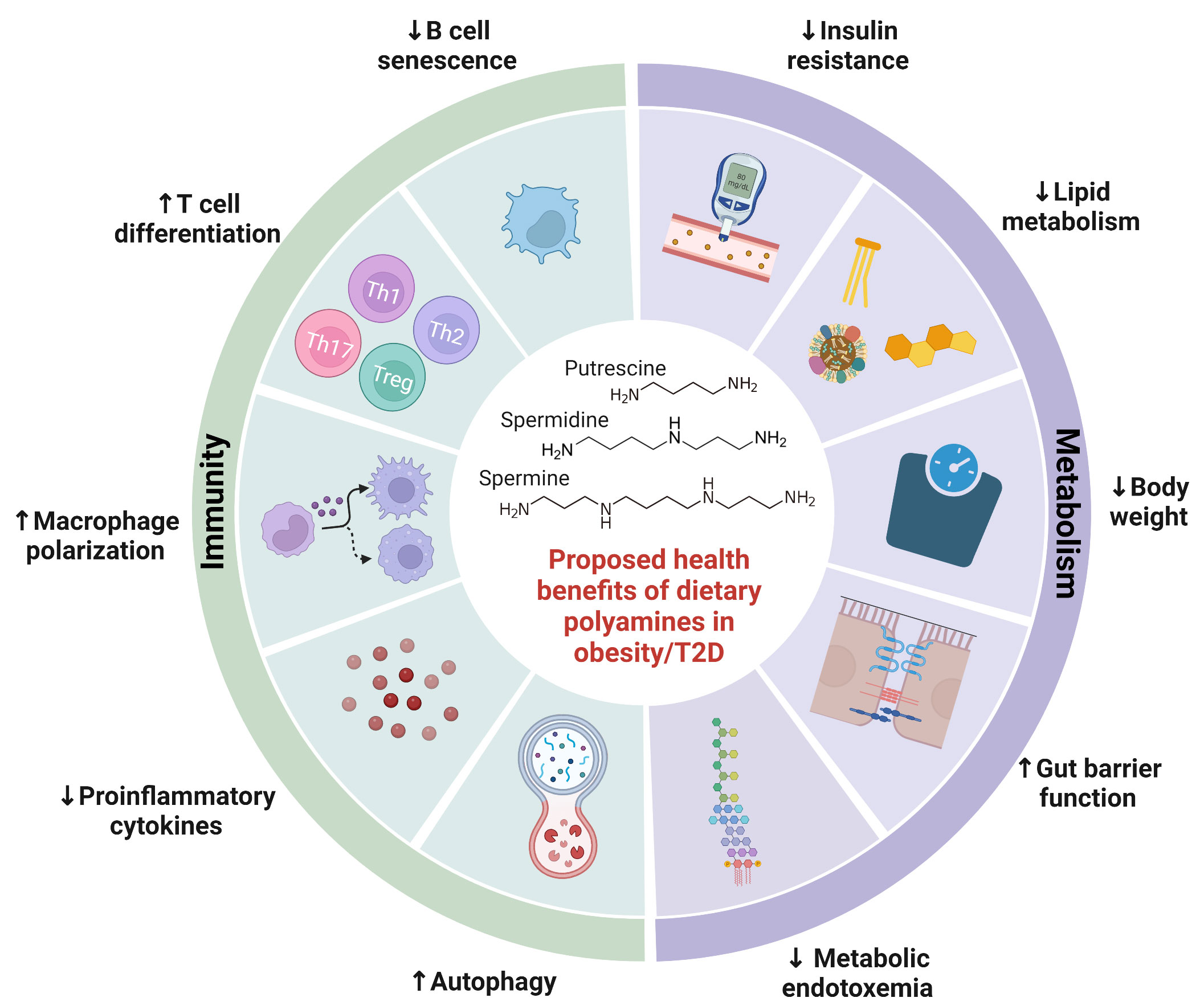
Figure 2 Proposed health benefits of dietary polyamines in obesity-related diseases. Polyamines are essential to human health, with their abundance altered by obesity-related diseases. This figure highlights a summary of potential benefits of polyamines on obesity-related diseases including metabolic syndrome and type 2 diabetes. Polyamines ameliorate metabolic syndrome by reducing weight gain, insulin resistance, lipid metabolism, gut barrier function, and metabolic endotoxemia. Polyamines also regulate both innate and adaptive immunity including proinflammatory cytokine production, autophagy, macrophage polarization, T cell differentiation, and B cell senescence. Further investigation is needed to elucidate polyamine-mediated health benefits in obesity-related diseases.
Polyamines as a nutraceutical to improve immunity against bacterial infections in obesity/T2D
Obesity/T2D is associated with increased risk for adverse clinical outcomes post-infection likely due to deficits in the immune system that have been likened to that of aging (105). It is particularly interesting because aging has been linked to declining levels of polyamines (40, 77), chronic low-grade inflammation (termed “inflammaging”) (106), and higher morbidity and mortality during infections (107). In fact, spermidine levels are depleted in the elderly and polyamine treatment corrects autophagy and B-cell senescence (108, 109). Similar dysregulation of polyamines in obesity/T2D would affect bacteria-host immune interactions that govern infection outcomes. In preclinical studies, obesity/T2D exacerbates inflammation and infection severity in models of osteomyelitis (110, 111), bacteremia (112), and skin infections (113, 114). Although not in the context of infection, administration of polyamines or associated probiotic and prebiotics have alleviated inflammation in a variety of disease models. Treatment with B. lactis and arginine, an amino acid precursor to polyamine synthesis, resulted in increased levels of circulating and colonic levels of polyamines that was associated with reduced inflammatory signaling in serum of mice (67). In a model of T-cell transfer colitis, spermidine potentiated Treg differentiation and ameliorated disease pathology in the gut (94). Injection of spermine protected mice from developing acute footpad inflammation (99), which may be important for mitigating excessive inflammation in diabetic foot ulcers (114). Therefore, polyamines are emerging players in dictating bacterial-host immune interactions in obesity/T2D by regulating inflammation (Figure 2).
More recently, polyamines have been directly shown to reduce osteomyelitis severity in a murine model of obesity/T2D (88). Supplementation with oligofructose, a bifidogenic prebiotic, decreased Staphylococcus aureus burden in infected bone and tissue in obese/T2D mice. Oligofructose dampened systemic inflammatory signaling that normally exacerbates infections in obesity/T2D (111, 114, 115), consistent with prior studies (99, 116). The authors determined a 6-log fold-change in B. pseudolongum in the gut microbiota of obese/T2D mice due to oligofructose treatment. This compositional change was associated with elevated polyamines in the cecum and plasma of obese/T2D mice. Remarkably, direct oral administration of spermine and spermidine led to a reduction in osteomyelitis severity similar to oligofructose. These results suggest that polyamines promote beneficial effects during infections in obesity/T2D and is an unexplored area that warrants further investigation.
Conclusion and perspectives
In this review, we propose that polyamines metabolites have the potential to ameliorate metabolic syndrome and the complications of obesity/T2D. Increasing evidence suggests that polyamines contribute to regulation of metabolic health and immunity in obesity/T2D. However, preclinical and clinical studies that examine polyamines in obesity/T2D are inconsistent due to heterogenous methods and tissue sampling. With their pleiotropic effects on transcription and translation in both eukaryotes and prokaryotes, the mechanisms by which polyamines affect human health remain unknown. In addition to the cautionary points we raise with current human data, future preclinical studies are needed to fully define the role of polyamines in obesity-associated metabolic disorders. First, obesity/T2D occurs through different mechanisms in the established diet-induced, transgenic leptin-deficient, and leptin receptor-deficient animal obesity models (117). The distinct gut microbiota and drug responses in these models affect microbiome compositional and metabolomic studies of obese/T2D animals and lean/controls (117–119). Second, the frequent use of male animals which gain weight more consistently in diet-induced murine models ignores the effect of sex on complications of obesity/T2D (120). Third, animal studies that implement probiotic, prebiotic, or post-biotics like polyamines often treat animals in parallel during the development of obesity/T2D. However, individuals with obesity/T2D often seek medical care after they have developed obesity/T2D and the complications of metabolic syndrome. Finally, previous literature has only analyzed polyamines in plasma of obese/T2D individuals, which does not consider the contributions of the gut microbiota as the primary driver of polyamine production. Further studies are needed to investigate polyamine levels in fecal samples to better understand polyamine production in obese/T2D individuals. Therefore, it is difficult to translate the results of animal experiments to personalized nutrition and precision medicine in humans.
With polyamines found as ubiquitous metabolites among eukaryotes and prokaryotes, their role in infectious disease is more complex than other microbiota-derived metabolites. Polyamines have been shown to be critical for survival and virulence of human bacterial pathogens (121, 122). While some pathogens produce polyamines, others rely on the extracellular polyamine pool regulated by host cells and the gut microbiota through uptake via transporter systems (121, 122) where polyamines have been shown negative effects on infectious bacteria. For example, spermine directly inhibits the growth of pathogenic Escherichia coli, Salmonella enterica serovar Typhimurium, and Staphylococcus aureus, while increasing susceptibility to β-lactam antibiotics in Pseudomonas aeruginosa (123). Because polyamines regulate survival and proliferation of bacteria and mammalian cells, this suggests that the host and bacteria pathogens compete for the extracellular polyamines pool during infections. For example, the polyamine transport operon potABCD in Streptococcus pneumoniae is required for resistance to neutrophil killing in vivo (124).This further implies a need to investigate the intracellular levels of polyamines in both mammalian cells and bacterial pathogens.
In conclusion, a growing body of evidence suggests that dysregulation of polyamines is associated with obesity/T2D and the related complications of immune deficits and metabolic disorders. The obesity/T2D epidemic is a public health concern and calls for alternative therapeutics. Probiotics, prebiotics, and post-biotics serve as mediators of metabolic syndrome and immunity in obesity/T2D. Prebiotics like oligofructose and probiotics like Bifidobacterium spp. that is associated polyamine production can be therapeutic alternatives to treating metabolic syndrome and strengthening of immunity, but relies on modulation of the gut microbiome. Therefore, direct application of the post-biotic, polyamines, to obese/T2D patients is a more attractive target. However, the biology of polyamines is heavily understudied outside the context cancer and remains to be investigated. Further research is needed to elucidate the mechanisms of polyamine regulation that contribute to diminished gut health, chronic inflammation in obesity, and development of diabetes, which will aid in the use of polyamines as a diagnostic tool for these complications.
Author contributions
TB conducted the literature review and prepared the manuscript. TB, SG, GM, and EB contributed to writing and manuscript preparation. All authors contributed to the article and approved the submitted version.
Funding
This work was supported by NIH NIDCR T90-DE021985 (TB); University of Rochester Sproull Fellowship (EB); NIH NIAID R21 AI69736 and University of Rochester Research Award (GM); NIH NIAMS R01 AR078414, DoD W81XWH-19-10808, and NIH NIMH R01 MH125103 (SG).
Conflict of interest
The authors declare that the research was conducted in the absence of any commercial or financial relationships that could be construed as a potential conflict of interest.
Publisher’s note
All claims expressed in this article are solely those of the authors and do not necessarily represent those of their affiliated organizations, or those of the publisher, the editors and the reviewers. Any product that may be evaluated in this article, or claim that may be made by its manufacturer, is not guaranteed or endorsed by the publisher.
References
1. Bramante CT, Lee CJ, Gudzune KA. Treatment of obesity in patients with diabetes. Diabetes Spectr Publ Am Diabetes Assoc (2017) 30:237–43. doi: 10.2337/ds17-0030
2. Eckel RH, Alberti K, Grundy SM, Zimmet PZ. The metabolic syndrome. Lancet (2010) 375:181–3. doi: 10.1016/s0140-6736(09)61794-3
3. Zimmet P, Magliano D, Matsuzawa Y, Alberti G, Shaw J. The metabolic syndrome: A global public health problem and a new definition. J Atheroscler Thromb (2005) 12:295–300. doi: 10.5551/jat.12.295
4. Hossain P, Kawar B, Nahas ME. Obesity and diabetes in the developing world — a growing challenge. N Engl J Med (2007) 356:213–5. doi: 10.1056/nejmp068177
5. Federation ID. IDF diabetes atlas. In: International diabetes federation, 10th edn (2021). Available at: https://www.diabetesatlas.org.
6. Rubin RR, Peyrot M. Quality of life and diabetes. Diabetes Metab Res Rev (1999) 15:205–18. doi: 10.1002/(sici)1520-7560(199905/06)15:3<205::aid-dmrr29>3.0.co;2-o
7. Kolotkin RL, Meter K, Williams GR. Quality of life and obesity. Obes Rev (2001) 2:219–29. doi: 10.1046/j.1467-789x.2001.00040.x
8. Huttunen R, Syrjänen J. Obesity and the risk and outcome of infection. Int J Obes (2013) 37:333–40. doi: 10.1038/ijo.2012.62
9. Dowsey MM, Choong PFM. Obese diabetic patients are at substantial risk for deep infection after primary TKA. Clin Orthop Relat R (2009) 467:1577–81. doi: 10.1007/s11999-008-0551-6
10. Olsen MA, Lock-Buckley P, Hopkins D, Polish LB, Sundt TM, Fraser VJ. The risk factors for deep and superficial chest surgical-site infections after coronary artery bypass graft surgery are different. J Thorac Cardiovasc Surg (2002) 124:136–45. doi: 10.1067/mtc.2002.122306
11. Bello-Chavolla OY, Bahena-López JP, Antonio-Villa NE, Vargas-Vázquez A, González-Díaz A, Márquez-Salinas A, et al. Predicting mortality due to SARS-CoV-2: A mechanistic score relating obesity and diabetes to COVID-19 outcomes in Mexico. J Clin Endocrinol Metab (2020) 105:dgaa346. doi: 10.1210/clinem/dgaa346
12. Holly JMP, Biernacka K, Maskell N, Perks CM. Obesity, diabetes and COVID-19: An infectious disease spreading from the East collides with the consequences of an unhealthy Western lifestyle. Front Endocrinol (2020) 11:582870. doi: 10.3389/fendo.2020.582870
13. Nikniaz Z, Somi MH, Dinevari MF, Taghizadieh A, Mokhtari L. Diabesity associates with poor COVID-19 outcomes among hospitalized patients. J Obes Metab Syndrome (2021) 30:149–54. doi: 10.7570/jomes20121
14. Mortensen OH, Nielsen AR, Erikstrup C, Plomgaard P, Fischer CP, Krogh-Madsen R, et al. Calprotectin — a novel marker of obesity. PloS One (2009) (Brussels:International Diabetes Federation) 4:e7419. doi: 10.1371/journal.pone.0007419
15. Nijhuis J, Rensen SS, Slaats Y, Dielen FMH, Buurman WA, Greve JWM. Neutrophil activation in morbid obesity, chronic activation of acute inflammation. Obesity (2009) 17:2014–8. doi: 10.1038/oby.2009.113
16. Li Q, Wang Q, Xu W, Ma Y, Wang Q, Eatman D, et al. C-reactive protein causes adult-onset obesity through chronic inflammatory mechanism. Front Cell Dev Biol (2020) 8:18. doi: 10.3389/fcell.2020.00018
17. Cani PD, Possemiers S, de WTV, Guiot Y, Everard A, Rottier O, et al. Changes in gut microbiota control inflammation in obese mice through a mechanism involving GLP-2-driven improvement of gut permeability. Gut (2009) 58:1091–103. doi: 10.1136/gut.2008.165886
18. Cani PD, Bibiloni R, Knauf C, Waget A, Neyrinck AM, Delzenne NM, et al. Changes in gut microbiota control metabolic endotoxemia-induced inflammation in high-fat diet-induced obesity and diabetes in mice. Diabetes (2008) 57:1470–81. doi: 10.2337/db07-1403
19. Delzenne NM, Neyrinck AM, Bäckhed F, Cani PD. Targeting gut microbiota in obesity: Effects of prebiotics and probiotics. Nat Rev Endocrinol (2011) 7:639–46. doi: 10.1038/nrendo.2011.126
20. Pereira SS, Alvarez-Leite JI. Low-grade inflammation, obesity, and diabetes. Curr Obes Rep (2014) 3:422–31. doi: 10.1007/s13679-014-0124-9
21. Kim K-A, Gu W, Lee I-A, Joh E-H, Kim D-H. High fat diet-induced gut microbiota exacerbates inflammation and obesity in mice via the TLR4 signaling pathway. PloS One (2012) 7:e47713. doi: 10.1371/journal.pone.0047713
22. Cox AJ, West NP, Cripps AW. Obesity, inflammation, and the gut microbiota. Lancet Diabetes Endocrinol (2015) 3:207–15. doi: 10.1016/s2213-8587(14)70134-2
23. Pagliai G, Dinu M, Madarena MP, Bonaccio M, Iacoviello L, Sofi F. Consumption of ultra-processed foods and health status: A systematic review and meta-analysis. Brit J Nutr (2021) 125:308–18. doi: 10.1017/s0007114520002688
24. Chen X, Zhang Z, Yang H, Qiu P, Wang H, Wang F, et al. Consumption of ultra-processed foods and health outcomes: A systematic review of epidemiological studies. Nutr J (2020) 19:86. doi: 10.1186/s12937-020-00604-1
25. Elizabeth L, Machado P, Zinöcker M, Baker P, Lawrence M. Ultra-processed foods and health outcomes: A narrative review. Nutrients (2020) 12:1955. doi: 10.3390/nu12071955
26. Snelson M, Tan SM, Clarke RE, de PC, Thallas-Bonke V, Nguyen T-V, et al. Processed foods drive intestinal barrier permeability and microvascular diseases. Sci Adv (2021) 7:eabe4841. doi: 10.1126/sciadv.abe4841
27. Newman TM, Shively CA, Register TC, Appt SE, Yadav H, Colwell RR, et al. Diet, obesity, and the gut microbiome as determinants modulating metabolic outcomes in a non-human primate model. Microbiome (2021) 9:100. doi: 10.1186/s40168-021-01069-y
28. Thingholm LB, Rühlemann MC, Koch M, Fuqua B, Laucke G, Boehm R, et al. Obese individuals with and without type 2 diabetes show different gut microbial functional capacity and composition. Cell Host Microbe (2019) 26:252–264.e10. doi: 10.1016/j.chom.2019.07.004
29. Turnbaugh PJ, Ridaura VK, Faith JJ, Rey FE, Knight R, Gordon JI. The effect of diet on the human gut microbiome: A metagenomic analysis in humanized gnotobiotic mice. Sci Transl Med (2009) 1:6ra14. doi: 10.1126/scitranslmed.3000322
30. Zhao L, Lou H, Peng Y, Chen S, Fan L, Li X. Elevated levels of circulating short-chain fatty acids and bile acids in type 2 diabetes are linked to gut barrier disruption and disordered gut microbiota. Diabetes Res Clin Pr (2020) 169:108418. doi: 10.1016/j.diabres.2020.108418
31. Schwiertz A, Taras D, Schäfer K, Beijer S, Bos NA, Donus C, et al. Microbiota and SCFA in lean and overweight healthy subjects. Obes Silver Spring Md (2009) 18:190–5. doi: 10.1038/oby.2009.167
32. de la Cuesta-Zuluaga J, Mueller NT, Álvarez-Quintero R, Velásquez-Mejía EP, Sierra JA, Corrales-Agudelo V, et al. Higher fecal short-chain fatty acid levels are associated with gut microbiome dysbiosis, obesity, hypertension and cardiometabolic disease risk factors. Nutrients (2018) 11:51. doi: 10.3390/nu11010051
33. Yamamura R, Nakamura K, Ukawa S, Okada E, Nakagawa T, Imae A, et al. Fecal short-chain fatty acids and obesity in a community-based Japanese population: The DOSANCO health study. Obes Res Clin Pract (2021) 15:345–50. doi: 10.1016/j.orcp.2021.06.003
34. Barrea L, Annunziata G, Muscogiuri G, Somma CD, Laudisio D, Maisto M, et al. Trimethylamine-n-oxide (TMAO) as novel potential biomarker of early predictors of metabolic syndrome. Nutrients (2018) 10:1971. doi: 10.3390/nu10121971
35. Schugar RC, Shih DM, Warrier M, Helsley RN, Burrows A, Ferguson D, et al. The TMAO-producing enzyme flavin-containing monooxygenase 3 regulates obesity and the beiging of white adipose tissue. Cell Rep (2017) 19:2451–61. doi: 10.1016/j.celrep.2017.05.077
36. Dehghan P, Farhangi MA, Nikniaz L, Nikniaz Z, Asghari-Jafarabadi M. Gut microbiota-derived metabolite trimethylamine n-oxide (TMAO) potentially increases the risk of obesity in adults: An exploratory systematic review and dose-response meta- analysis. Obes Rev (2020) 21:e12993. doi: 10.1111/obr.12993
37. Chávez-Talavera O, Tailleux A, Lefebvre P, Staels B. Bile acid control of metabolism and inflammation in obesity, type 2 diabetes, dyslipidemia, and nonalcoholic fatty liver disease. Gastroenterology (2017) 152:1679–1694.e3. doi: 10.1053/j.gastro.2017.01.055
38. Li T, Francl JM, Boehme S, Ochoa A, Zhang Y, Klaassen CD, et al. Glucose and insulin induction of bile acid synthesis mechanisms and implication in diabetes and obesity*. J Biol Chem (2012) 287:1861–73. doi: 10.1074/jbc.m111.305789
39. Haeusler RA, Camastra S, Nannipieri M, Astiarraga B, Castro-Perez J, Xie D, et al. Increased bile acid synthesis and impaired bile acid transport in human obesity. J Clin Endocrinol Metab (2016) 101:1935–44. doi: 10.1210/jc.2015-2583
40. Madeo F, Eisenberg T, Pietrocola F, Kroemer G. Spermidine in health and disease. Science (2018) 359:eaan2788. doi: 10.1126/science.aan2788
41. Proietti E, Rossini S, Grohmann U, Mondanelli G. Polyamines and kynurenines at the intersection of immune modulation. Trends Immunol (2020) 41:1037–50. doi: 10.1016/j.it.2020.09.007
42. Sagar NA, Tarafdar S, Agarwal S, Tarafdar A, Sharma S. Polyamines: Functions, metabolism, and role in human disease management. Med Sci (2021) 9:44. doi: 10.3390/medsci9020044
43. Pegg AE. Mammalian polyamine metabolism and function. IUBMB Life (2009) 61:880–94. doi: 10.1002/iub.230
44. Kitada Y, Muramatsu K, Toju H, Kibe R, Benno Y, Kurihara S, et al. Bioactive polyamine production by a novel hybrid system comprising multiple indigenous gut bacterial strategies. Sci Adv (2018) 4:eaat0062. doi: 10.1126/sciadv.aat0062
45. Nakamura A, Ooga T, Matsumoto M. Intestinal luminal putrescine is produced by collective biosynthetic pathways of the commensal microbiome. Gut Microbes (2018) 10:1–13. doi: 10.1080/19490976.2018.1494466
46. Matsumoto M, Ooga T, Kibe R, Aiba Y, Koga Y, Benno Y. Colonic absorption of low-Molecular-Weight metabolites influenced by the intestinal microbiome: A pilot study. PloS One (2017) 12:e0169207. doi: 10.1371/journal.pone.0169207
47. Tofalo R, Cocchi S, Suzzi G. Polyamines and gut microbiota. Front Nutr (2019) 6:16. doi: 10.3389/fnut.2019.00016
48. Bardócz S, Grant G, Brown DS, Ralph A, Pusztai A. Polyamines in food–implications for growth and health. J Nutr Biochem (1993) 4:66–71. doi: 10.1016/0955-2863(93)90001-d
49. Cipolla BG, Havouis R, Moulinoux JP. Polyamine contents in current foods: a basis for polyamine reduced diet and a study of its long term observance and tolerance in prostate carcinoma patients. Amino Acids (2007) 33:203–12. doi: 10.1007/s00726-007-0524-1
50. Morrison LD, Becker L, Ang LC, Kish SJ. Polyamines in human brain: Regional distribution and influence of aging. J Neurochem (1995) 65:636–42. doi: 10.1046/j.1471-4159.1995.65020636.x
51. Dunzendorfer U, Russell DH. Altered polyamine profiles in prostatic hyperplasia and in kidney tumors. Cancer Res (1978) 38:2321–4.
52. Buts J-P, Keyser ND, Raedemaeker LD, Collette E, Sokal EM. Polyamine profiles in human milk, infant artificial formulas, and semi-elemental diets. J Pediatr Gastr Nutr (1995) 21:44–9. doi: 10.1097/00005176-199507000-00007
53. Ali MA, Strandvik B, Sabel K -G., Kilander CP, Strömberg R, Yngve A. Polyamine levels in breast milk are associated with mothers’ dietary intake and are higher in preterm than full-term human milk and formulas. J Hum Nutr Diet (2014) 27:459–67. doi: 10.1111/jhn.12156
54. Ali MA, Strandvik B, Palme-Kilander C, Yngve A. Lower polyamine levels in breast milk of obese mothers compared to mothers with normal body weight. J Hum Nutr Diet (2013) 26:164–70. doi: 10.1111/jhn.12097
55. Liu R, Li Q, Ma R, Lin X, Xu H, Bi K. Determination of polyamine metabolome in plasma and urine by ultrahigh performance liquid chromatography–tandem mass spectrometry method: Application to identify potential markers for human hepatic cancer. Anal Chim Acta (2013) 791:36–45. doi: 10.1016/j.aca.2013.06.044
56. Lu C-Y, Su H-H, Chen Y-L, Tseng W-L. Micro extraction to quantitate spermidine and spermine in human urine and blood by matrix-assisted laser desorption ionization time-of-flight mass spectrometry. J Chromatogr A (2014) 1326:1–6. doi: 10.1016/j.chroma.2013.12.037
57. Maráková K, Piešťanský J, Zelinková Z, Mikuš P. Simultaneous determination of twelve biogenic amines in human urine as potential biomarkers of inflammatory bowel diseases by capillary electrophoresis – tandem mass spectrometry. J Pharmaceut BioMed (2020) 186:113294. doi: 10.1016/j.jpba.2020.113294
58. Vargas AJ, Ashbeck EL, Thomson CA, Gerner EW, Thompson PA. Dietary polyamine intake and polyamines measured in urine. Nutr Cancer (2014) 66:1144–53. doi: 10.1080/01635581.2014.949801
59. Bartos D, Campbell RA, Bartos F, Grettie DP. Direct determination of polyamines in human serum by radioimmunoassay. Cancer Res (1975) 35:2056–60.
60. Sakata K, Kashiwagi K, Sharmin S, Ueda S, Irie Y, Murotani N, et al. Increase in putrescine, amine oxidase, and acrolein in plasma of renal failure patients. Biochem Bioph Res Co (2003) 305:143–9. doi: 10.1016/s0006-291x(03)00716-2
61. Löser C, Fölsch UR, Paprotny C, Creutzfeldt W. Polyamines in colorectal cancer. evaluation of polyamine concentrations in the colon tissue, serum, and urine of 50 patients with colorectal cancer. Cancer (1990) 65:958–66. doi: 10.1002/1097-0142(19900215)65:4<958::aid-cncr2820650423>3.0.co;2-z
62. Byun JA, Lee SH, Jung BH, Choi MH, Moon MH, Chung BC. Analysis of polyamines as carbamoyl derivatives in urine and serum by liquid chromatography–tandem mass spectrometry. BioMed Chromatogr (2008) 22:73–80. doi: 10.1002/bmc.898
63. Matsumoto M, Benno Y. The relationship between microbiota and polyamine concentration in the human intestine: A pilot study. Microbiol Immunol (2007) 51:25–35. doi: 10.1111/j.1348-0421.2007.tb03887.x
64. Matsumoto M, Benno Y. Consumption of bifidobacterium lactis LKM512 yogurt reduces gut mutagenicity by increasing gut polyamine contents in healthy adult subjects. Mutat Res Fundam Mol Mech Mutagen (2004) 568:147–53. doi: 10.1016/j.mrfmmm.2004.07.016
65. Matsumoto M, Kakizoe K, Benno Y. Comparison of fecal microbiota and polyamine concentration in adult patients with intractable atopic dermatitis and healthy adults. Microbiol Immunol (2007) 51:37–46. doi: 10.1111/j.1348-0421.2007.tb03888.x
66. Matsumoto M, Kurihara S, Kibe R, Ashida H, Benno Y. Longevity in mice is promoted by probiotic-induced suppression of colonic senescence dependent on upregulation of gut bacterial polyamine production. PloS One (2011) 6:e23652. doi: 10.1371/journal.pone.0023652
67. Kibe R, Kurihara S, Sakai Y, Suzuki H, Ooga T, Sawaki E, et al. Upregulation of colonic luminal polyamines produced by intestinal microbiota delays senescence in mice. Sci Rep-uk (2014) 4:4548. doi: 10.1038/srep04548
68. Pugin B, Barcik W, Westermann P, Heider A, Wawrzyniak M, Hellings P, et al. A wide diversity of bacteria from the human gut produces and degrades biogenic amines. Microb Ecol Health D (2017) 28:1353881. doi: 10.1080/16512235.2017.1353881
69. Million M, Maraninchi M, Henry M, Armougom F, Richet H, Carrieri P, et al. Obesity-associated gut microbiota is enriched in lactobacillus reuteri and depleted in bifidobacterium animalis and methanobrevibacter smithii. Int J Obes (2012) 36:817–25. doi: 10.1038/ijo.2011.153
70. Million M, Angelakis E, Maraninchi M, Henry M, Giorgi R, Valero R, et al. Correlation between body mass index and gut concentrations of lactobacillus reuteri, bifidobacterium animalis, methanobrevibacter smithii and escherichia coli. Int J Obes (2013) 37:1460–6. doi: 10.1038/ijo.2013.20
71. Turnbaugh PJ, Bäckhed F, Fulton L, Gordon JI. Diet-induced obesity is linked to marked but reversible alterations in the mouse distal gut microbiome. Cell Host Microbe (2008) 3:213–23. doi: 10.1016/j.chom.2008.02.015
72. Ley RE, Turnbaugh PJ, Klein S, Gordon JI. Microbial ecology: human gut microbes associated with obesity. Nature (2006) 444:1022–3. doi: 10.1038/4441022a
73. Fernandez-Garcia JC, Delpino-Rius A, Samarra I, Castellano-Castillo D, Muñoz-Garach A, Bernal-Lopez MR, et al. Type 2 diabetes is associated with a different pattern of serum polyamines: A case–control study from the PREDIMED-plus trial. J Clin Med (2019) 8:71. doi: 10.3390/jcm8010071
74. Ocaña-Wilhelmi L, Cardona F, Garrido-Sanchez L, Fernandez-Garcia D, Tinahones FJ, Ramos-Molina B. Change in serum polyamine metabolome pattern after bariatric surgery in obese patients with metabolic syndrome. Surg Obes Relat Dis (2020) 16:306–11. doi: 10.1016/j.soard.2019.10.024
75. Gao H, Zhang Q, Xu J, Yuan W, Li R, Guo H, et al. Elevation of serum spermidine in obese patients: Results from a cross-sectional and follow-up study. Nutrients (2022) 14:2613. doi: 10.3390/nu14132613
76. Magnes C, Fauland A, Gander E, Narath S, Ratzer M, Eisenberg T, et al. Polyamines in biological samples: Rapid and robust quantification by solid-phase extraction online-coupled to liquid chromatography–tandem mass spectrometry. J Chromatogr A (2014) 1331:44–51. doi: 10.1016/j.chroma.2013.12.061
77. Minois N, Carmona-Gutierrez D, Madeo F. Polyamines in aging and disease. Aging Albany Ny (2011) 3:716–32. doi: 10.18632/aging.100361
78. Nakatani S, Horimoto Y, Nakabayashi N, Karasawa M, Wada M, Kobata K. Spermine suppresses adipocyte differentiation and exerts anti-obesity effects In vitro and In vivo. Int J Mol Sci (2022) 23:11818. doi: 10.3390/ijms231911818
79. Sadasivan SK, Vasamsetti B, Singh J, Marikunte VV, Oommen AM, Jagannath MR, et al. Exogenous administration of spermine improves glucose utilization and decreases bodyweight in mice. Eur J Pharmacol (2014) 729:94–9. doi: 10.1016/j.ejphar.2014.01.073
80. Wang D, Yin J, Zhou Z, Tao Y, Jia Y, Jie H, et al. Oral spermidine targets brown fat and skeletal muscle to mitigate diet-induced obesity and metabolic disorders. Mol Nutr Food Res (2021) 65:2100315. doi: 10.1002/mnfr.202100315
81. Monelli E, Villacampa P, Zabala-Letona A, Martinez-Romero A, Llena J, Beiroa D, et al. Angiocrine polyamine production regulates adiposity. Nat Metab (2022) 4:327–43. doi: 10.1038/s42255-022-00544-6
82. Çelik VK, Kapancık S, Kaçan T, Kaçan SB, Kapancık S, Kılıçgün H. Serum levels of polyamine synthesis enzymes increase in diabetic patients with breast cancer. Endocr Connect (2017) 6:574–9. doi: 10.1530/ec-17-0137
83. Blüher M. Obesity: Global epidemiology and pathogenesis. Nat Rev Endocrinol (2019) 15:288–98. doi: 10.1038/s41574-019-0176-8
84. Khan MAB, Hashim MJ, King JK, Govender RD, Mustafa H, Kaabi JA. Epidemiology of type 2 diabetes – global burden of disease and forecasted trends. J Epidemiol Global Heal (2019) 10:107–11. doi: 10.2991/jegh.k.191028.001
85. Jamdar SC, Cao WF, Samaniego E. Relationship between adipose polyamine concentrations and triacylglycerol synthetic enzymes in lean and obese zucker rats. Enzym Protein (1996) 49:222–30. doi: 10.1159/000468632
86. Sjöholm Å, Arkhammar P, Berggren P-O, Andersson A. Polyamines in pancreatic islets of obese-hyperglycemic (ob/ob) mice of different ages. Am J Physiol-cell Ph (2001) 280:C317–23. doi: 10.1152/ajpcell.2001.280.2.c317
87. Ma L, Zheng A, Ni L, Wu L, Hu L, Zhao Y, et al. Bifidobacterium animalis subsp. lactis lkm512 attenuates obesity-associated inflammation and insulin resistance through the modification of gut microbiota in high-fat diet-induced obese mice. Mol Nutr Food Res (2022) 66:2100639. doi: 10.1002/mnfr.202100639
88. Bui TI, Gill AL, Mooney RA, Gill SR. Modulation of gut microbiota metabolism in obesity-related type 2 diabetes reduces osteomyelitis severity. Microbiol Spectr (2022), 8 e00170–22. doi: 10.1128/spectrum.00170-22
89. Yuan F, Zhang L, Cao Y, Gao W, Zhao C, Fang Y, et al. Spermidine/spermine N1-acetyltransferase-mediated polyamine catabolism regulates beige adipocyte biogenesis. Metabolis (2018) 85:298–304. doi: 10.1016/j.metabol.2018.04.007
90. Niiranen K, Keinänen TA, Pirinen E, Heikkinen S, Tusa M, Fatrai S, et al. Mice with targeted disruption of spermidine/spermine N1-acetyltransferase gene maintain nearly normal tissue polyamine homeostasis but show signs of insulin resistance upon aging. J Cell Mol Med (2006) 10:815–27. doi: 10.2755/jcmm010.004.02
91. Pirinen E, Kuulasmaa T, Pietilä M, Heikkinen S, Tusa M, Itkonen P, et al. Enhanced polyamine catabolism alters homeostatic control of white adipose tissue mass, energy expenditure, and glucose metabolism▿. Mol Cell Biol (2007) 27:4953–67. doi: 10.1128/mcb.02034-06
92. Bowlin TL, McKown BJ, Sunkara PS. Increased ornithine decarboxylase activity and polyamine biosynthesis are required for optimal cytolytic T lymphocyte induction. Cell Immunol (1987) 105:110–7. doi: 10.1016/0008-8749(87)90060-8
93. Pasquali JL, Mamont PS, Weryha A, Knapp AM, Blervaque A, Siat M. Immunosuppressive effects of (2R,5R)-6-heptyne-2,5-diamine an inhibitor of polyamine synthesis: I. effects on mitogen-induced immunoglobulin production in human cultured lymphocytes. Clin Exp Immunol (1988) 72:141–4.
94. Carriche GM, Almeida L, Stüve P, Velasquez L, Dhillon-LaBrooy A, Roy U, et al. Regulating T-cell differentiation through the polyamine spermidine. J Allergy Clin Immun (2021) 147:335–348.e11. doi: 10.1016/j.jaci.2020.04.037
95. Puleston DJ, Baixauli F, Sanin DE, Edwards-Hicks J, Villa M, Kabat AM, et al. Polyamine metabolism is a central determinant of helper T cell lineage fidelity. Cell (2021) 184:4186–4202.e20. doi: 10.1016/j.cell.2021.06.007
96. O’Brien KL, Assmann N, O’Connor E, Keane C, Walls J, Choi C, et al. De novo polyamine synthesis supports metabolic and functional responses in activated murine NK cells. Eur J Immunol (2021) 51:91–102. doi: 10.1002/eji.202048784
97. Mühling J, Engel J, Halabi M, Müller M, Fuchs M, Krüll M, et al. Nitric oxide and polyamine pathway-dependent modulation of neutrophil free amino- and α-keto acid profiles or host defense capability. Amino Acids (2006) 31:11. doi: 10.1007/s00726-006-0273-6
98. Guarnieri C, Georgountzos A, Caldarera I, Flamigni F, Ligabue A. Polyamines stimulate superoxide production in human neutrophils activated by n-fMet-Leu-Phe but not by phorbol myristate acetate. Biochim Et Biophys Acta Bba - Mol Cell Res (1987) 930:135–9. doi: 10.1016/0167-4889(87)90024-3
99. Zhang M, Caragine T, Wang H, Cohen PS, Botchkina G, Soda K, et al. Spermine inhibits proinflammatory cytokine synthesis in human mononuclear cells: A counterregulatory mechanism that restrains the immune response. J Exp Med (1997) 185:1759–68. doi: 10.1084/jem.185.10.1759
100. Szabó C, Southan GJ, Wood E, Thiemermann C, Vane JR. Inhibition by spermine of the induction of nitric oxide synthase in J774.2 macrophages: requirement of a serum factor. Brit J Pharmacol (1994) 112:355–6. doi: 10.1111/j.1476-5381.1994.tb13078.x
101. Latour YL, Gobert AP, Wilson KT. The role of polyamines in the regulation of macrophage polarization and function. Amino Acids (2020) 52:151–60. doi: 10.1007/s00726-019-02719-0
102. Baydoun AR, Morgan DML. Inhibition of ornithine decarboxylase potentiates nitric oxide production in LPS-activated J774 cells. Brit J Pharmacol (1998) 125:1511–6. doi: 10.1038/sj.bjp.0702231
103. Castoldi F, Hyvönen MT, Durand S, Aprahamian F, Sauvat A, Malik SA, et al. Chemical activation of SAT1 corrects diet-induced metabolic syndrome. Cell Death Differ (2020) 27:2904–20. doi: 10.1038/s41418-020-0550-z
104. Rivière A, Selak M, Lantin D, Leroy F, Vuyst LD. Bifidobacteria and butyrate-producing colon bacteria: Importance and strategies for their stimulation in the human gut. Front Microbiol (2016) 7:979. doi: 10.3389/fmicb.2016.00979
105. Ahima RS. Connecting obesity, aging and diabetes. Nat Med (2009) 15:996–7. doi: 10.1038/nm0909-996
106. Franceschi C, Garagnani P, Vitale G, Capri M, Salvioli S. Inflammaging and ‘Garb-aging.’. Trends Endocrinol Metab (2017) 28:199–212. doi: 10.1016/j.tem.2016.09.005
107. Kline KA, Bowdish DM. Infection in an aging population. Curr Opin Microbiol (2016) 29:63–7. doi: 10.1016/j.mib.2015.11.003
108. Zhang H, Alsaleh G, Feltham J, Sun Y, Napolitano G, Riffelmacher T, et al. Polyamines control eIF5A hypusination, TFEB translation, and autophagy to reverse b cell senescence. Mol Cell (2019) 76:110–125.e9. doi: 10.1016/j.molcel.2019.08.005
109. Zhang H, Simon AK. Polyamines reverse immune senescence via the translational control of autophagy. Autophagy (2020) 16:181–2. doi: 10.1080/15548627.2019.1687967
110. Farnsworth CW, Schott EM, Jensen SE, Zukoski J, Benvie AM, Refaai MA, et al. (ClfA) by staphylococcus aureus in the obese, type 2 diabetic host mediates increased virulence. Infect Immun (2017) 85:e01005–16. doi: 10.1128/iai.01005-16
111. Farnsworth CW, Schott EM, Benvie AM, Zukoski J, Kates SL, Schwarz EM, et al. Obesity/type 2 diabetes increases inflammation, periosteal reactive bone formation, and osteolysis during staphylococcus aureus implant-associated bone infection. J Orthop Res (2018) 36:1614–23. doi: 10.1002/jor.23831
112. Nielsen TB, Pantapalangkoor P, Yan J, Luna BM, Dekitani K, Bruhn K, et al. Diabetes exacerbates infection via hyperinflammation by signaling through TLR4 and RAGE. Mbio (2017) 8:e00818–17. doi: 10.1128/mbio.00818-17
113. Zhang L, Guerrero-Juarez CF, Chen SX, Zhang X, Yin M, Li F, et al. Diet-induced obesity promotes infection by impairment of the innate antimicrobial defense function of dermal adipocyte progenitors. Sci Transl Med (2021) 13. doi: 10.1126/scitranslmed.abb5280
114. Farnsworth CW, Schott EM, Benvie A, Kates SL, Schwarz EM, Gill SR, et al. Exacerbated staphylococcus aureus foot infections in Obese/Diabetic mice are associated with impaired germinal center reactions, ig class switching, and humoral immunity. J Immunol (2018) 201:560–72. doi: 10.4049/jimmunol.1800253
115. Schott EM, Farnsworth CW, Grier A, Lillis JA, Soniwala S, Dadourian GH, et al. Targeting the gut microbiome to treat the osteoarthritis of obesity. JCI Insight (2018) 3:e95997. doi: 10.1172/jci.insight.95997
116. Paul S, Kang SC. Natural polyamine inhibits mouse skin inflammation and macrophage activation. Inflammation Res (2013) 62:681–8. doi: 10.1007/s00011-013-0620-5
117. Suriano F, Vieira-Silva S, Falony G, Roumain M, Paquot A, Pelicaen R, et al. Novel insights into the genetically obese (ob/ob) and diabetic (db/db) mice: two sides of the same coin. Microbiome (2021) 9:147. doi: 10.1186/s40168-021-01097-8
118. Wang J-H, Shin NR, Lim S-K, Im U, Song E-J, Nam Y-D, et al. Diet control more intensively disturbs gut microbiota than genetic background in wild type and ob/ob mice. Front Microbiol (2019) 10:1292. doi: 10.3389/fmicb.2019.01292
119. Young AA, Gedulin BR, Bhavsar S, Bodkin N, Jodka C, Hansen B, et al. Glucose-lowering and insulin-sensitizing actions of exendin-4: Studies in obese diabetic (ob/ob, db/db) mice, diabetic fatty zucker rats, and diabetic rhesus monkeys (Macaca mulatta). Diabetes (1999) 48:1026–34. doi: 10.2337/diabetes.48.5.1026
120. Surwit RS, Kuhn CM, Cochrane C, McCubbin JA, Feinglos MN. Diet-induced type II diabetes in C57BL/6J mice. Diabetes (1988) 37:1163–7. doi: 10.2337/diab.37.9.1163
121. Shah P, Swiatlo E. A multifaceted role for polyamines in bacterial pathogens. Mol Microbiol (2008) 68:4–16. doi: 10.1111/j.1365-2958.2008.06126.x
122. Martino MLD, Campilongo R, Casalino M, Micheli G, Colonna B, Prosseda G. Polyamines: Emerging players in bacteria–host interactions. Int J Med Microbiol (2013) 303:484–91. doi: 10.1016/j.ijmm.2013.06.008
123. Kwon D-H, Lu C-D. Polyamine effects on antibiotic susceptibility in bacteria. Antimicrob Agents Ch (2007) 51:2070–7. doi: 10.1128/aac.01472-06
Keywords: polyamine, obesity, type 2 diabetes, metabolic syndrome, nutraceutical
Citation: Bui TI, Britt EA, Muthukrishnan G and Gill SR (2023) Probiotic induced synthesis of microbiota polyamine as a nutraceutical for metabolic syndrome and obesity-related type 2 diabetes. Front. Endocrinol. 13:1094258. doi: 10.3389/fendo.2022.1094258
Received: 09 November 2022; Accepted: 16 December 2022;
Published: 13 January 2023.
Edited by:
Mozhdeh Sojoodi, Massachusetts General Hospital and Harvard Medical School, United StatesReviewed by:
Hannah Xiaoyan Hui, The Chinese University of Hong Kong, ChinaAfsaneh Goudarzi, Shahid Beheshti University of Medical Sciences, Iran
Copyright © 2023 Bui, Britt, Muthukrishnan and Gill. This is an open-access article distributed under the terms of the Creative Commons Attribution License (CC BY). The use, distribution or reproduction in other forums is permitted, provided the original author(s) and the copyright owner(s) are credited and that the original publication in this journal is cited, in accordance with accepted academic practice. No use, distribution or reproduction is permitted which does not comply with these terms.
*Correspondence: Steven R. Gill, c3RldmVuX2dpbGxAdXJtYy5yb2NoZXN0ZXIuZWR1