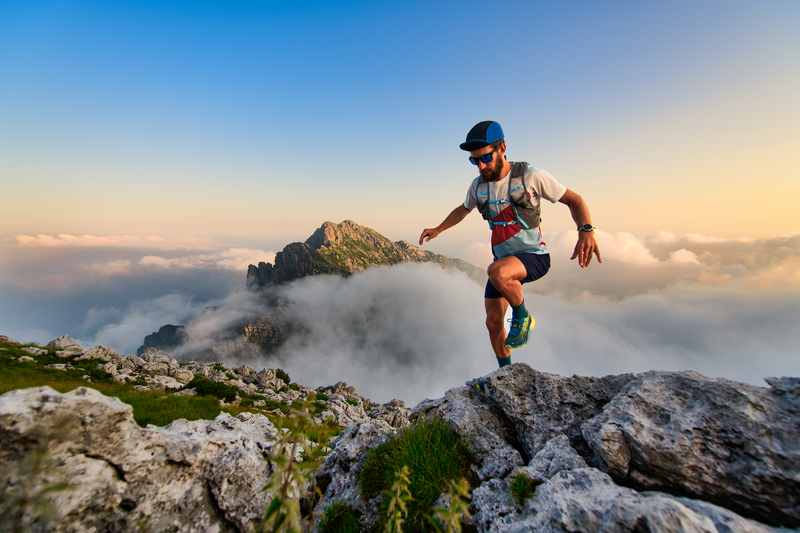
94% of researchers rate our articles as excellent or good
Learn more about the work of our research integrity team to safeguard the quality of each article we publish.
Find out more
REVIEW article
Front. Electron. , 06 February 2025
Sec. Bioelectronics
Volume 6 - 2025 | https://doi.org/10.3389/felec.2025.1503425
Recent advances in manufacturing of flexible and conformable microelectronics have opened opportunities for health monitoring and disease treatment. Other material engineering advances, such as the development of conductive, skin-like hydrogels, liquid metals, electric textiles, and piezoelectric films provide safe and comfortable means of interfacing with the human body. Together, these advances have enabled the design and engineering of bioelectronic devices with integrated multimodal sensing and stimulation capabilities to be worn nearly anywhere on the body. Of particular interest here, the external ear (auricle) offers a unique opportunity to design scalable bioelectronic devices with a high degree of usability and familiarity given the broad use of headphones. This review article discusses recent design and engineering advances in the development of auricular bioelectronic devices capable of physiological and biochemical sensing, cognitive monitoring, targeted neuromodulation, and control for human-computer interactions. Stemming from this scalable foundation, there will be increased growth and competition in research and engineering to advance auricular bioelectronics. This activity will lead to increased adoption of these smart headphone-style devices by patients and consumers for tracking health, treating medical conditions, and enhancing human-computer interactions.
Headphones are an iconic human interface. Current and forthcoming generations of headphones or auricular bioelectronics have capabilities that will fundamentally change how we approach health diagnostics, clinical intervention, and communications. The design of headphones can be traced back to the 1890’s, when Earnest Mercadier developed binaural diaphragms enabling handsfree operation for telephone operators controlling switchboards (Figure 1A) (Mercadier, 2024). A couple decades later Nathaniel Baldwin is credited with inventing the first audio headphones designed to enhance naval communications aboard large and noisy ships. Marking an application transition from their industrial use in communications to personal entertainment use in the audio industry, John Koss designed the first stereo headphones for listening to music in 1958. Since, electrical, mechanical, and biomedical engineering advances have enabled headphone miniaturization, microphone and biometric sensor integration, wireless connectivity, incorporation of digital signal processors (DSP) for active noise cancellation (ANC), audio filtering, amplification, spatial audio encoding, and medical device embodiments for the treatment of health conditions (Figures 1B–D). Today, given their global use in daily communication and digital media consumption, we recognize headphones as being deeply connected to our inner thoughts and emotions, lifestyle, and productivity (Lieberman et al., 2022; Molesworth et al., 2013). As such, headphones and devices intended to be worn on the ear have inspired the design and engineering of modern bioelectronic devices intended for health, medicine, and communications.
Figure 1. Evolution of modern headphones. (A) Shown is the “Bi-Telephone” developed to enable telephone operators hands-free operation of switchboards was invented in 1891 by Ernst Mercadier and is considered to be the first modern headphone (Mercadier). Advances in communications and microelectronics in the 1980–90’s led to the development of wireless headphones. (B) Shows a patent illustration for an embodiment of wireless headphones from the Sony Corporation (Wingate, 1999). Numerous advances in headphone design came about with engineering progress in wireless communication, sensor design, microelectronic packaging, digital signal processing (DSP), and automatic noise cancellation (ANC) in the 2000’s. (C) These advances led to the development of wireless, wearable headphones with integrated microphone arrays, biometric sensors, control electronics, and power management systems as illustrated by the block diagram showing components of an earbud headphone from an Apple, Inc. patent covering headphones with biometric sensing (Qian et al., 2017). (D) Patent illustrations showing an embodiment of Apple, Inc. earbud headphones (AirPods) with biometric sensors in the ear (Qian et al., 2017).
The medical device and personal electronics industries have begun to witness barriers blurred as health agencies, care providers, consumers, and patients, motivated by health monitoring, data analytics, and predictive algorithms have begun to integrate wearables into our daily lives (Haghi et al., 2017; Muzny et al., 2020; Perez-Pozuelo et al., 2020; Channa et al., 2021). Markets suffer from no shortage of low-power consumption, wireless connected, multimodal sensor integrated clinical grade and consumer health wearables measuring heart rate, heart rate variability, respiration rate, sleep and activity patterns, metabolic activity, stress levels, and oxygen levels. Over the past decade, a great race for data-driven, predictive insights afforded by modern machine learning (M/L) and artificial intelligence (AI) methods has attracted additional engineering resources around wearable electronic research and development (Acosta et al., 2022; Nahavandi et al., 2022). Many engineers, scientists, and members of the semiconductor and microelectronics industries have risen to challenges in wearable design, testing, packaging, and manufacturing of a bewildering array of batteries, energy harvesters, microprocessors, MEMS accelerometers, optical, electrical, and acoustic sensors fueling growth in consumer and medical wearables (Iqbal et al., 2021; Mamdiwar et al., 2021; Sreenilayam et al., 2020; Hasan et al., 2021).
Developing wearable electronics that have a size, weight, and power profile sufficient for wearing as headphones has some unique engineering challenges. Physical acoustic constraints combined with the fact that people have ears of different sizes and shapes presents other challenges (Møller, 1992; Møller et al., 1995; Poldy and Borwick, 2001). However, the consumer audio, cochlear implant, and hearing aid industries have solved many of these challenges over the past couple decades (Zeng et al., 2008; Chung, 2004; Edwards, 2007; Sabin et al., 2020). In fact, the FDA has recently cleared several over-the-counter (OTC) hearing aids that are now widely available to patients without the need to be fitted by an audiologist (Sabin et al., 2020; Knoetze et al., 2024; Sheng et al., 2024; F. D. A. Commisioner Office, 2024a). More recently in another innovative step forward, the FDA cleared Apple’s AirPods Pro 2 consumer headphones as an OTC hearing aid software through a de novo Software as a Medical Device (SaMD) regulatory pathway (F. D. A. Commissioner Office, 2024; Apple, 2024).
Other advances in flexible and conformable electronics, as well as polymer materials for skin-device interfaces have enabled the development of sophisticated auricular bioelectronics embodied as headphones capable of sensing biochemical and physiological activity (Figures 1C, D) (Qian et al., 2017; Masè et al., 2020; Röddiger et al., 2022). The fields of neuromodulation and bioelectronic medicine have meanwhile been developing various methods of stimulating auricular branches of cranial and cervical nerves for various outcomes (Kaniusas et al., 2019; Verma et al., 2021; Kim et al., 2022). For example, various vibrotactile and electrical forms of transcutaneous auricular vagus nerve stimulation (taVNS) have been shown to reduce inflammation including neuroinflammation associated with long COVID, reduce stress, improve sleep, decrease depression and anxiety, and enhance learning, cognition, and neurorehabilitation as further discussed below. Collectively these advances have produced a climate where the development of open- and closed-loop auricular bioelectronics will produce a new generation of medical devices, health and performance wearables, and brain-computer interfaces (BCIs) that are as scalable as personal headphones. The goal of this perspective is to highlight the anatomy, physiology, and recent engineering milestones enabling the development of modern auricular bioelectronics.
The structure of the auricle or external ear serves mammals unique physiological roles and is tied closely to our evolution and survival (Webster, 1966; Le Maître et al., 2020). The external ear is a cartilaginous structure that has dense vasculature and sensorimotor innervation to help foster heat dissipation, sound location, and positional awareness. The structure of the external ear has two essential parts, which are the pinna and the external auditory meatus (EAM) or outer ear canal ending at the tympanic membrane where the middle ear begins (Figure 2A). This location provides proximal access to the brain, which is useful for recording brain activity as discussed below. The pinna has many distinct anatomical features, which filter and direct sounds to the EAM as illustrated in Figure 2A, The EAM directs and conducts these sound waves to the middle ear before transmitting them to the inner ear for auditory transduction and processing.
Figure 2. Anatomy of the external ear. (A) The anatomical illustration depicts the anatomy of the human external ear showing prominent structures. (B) The illustrations depict the sensory innervation of the external ear by the lesser occipital nerve (C2; orange), the great auricular nerve (C2, C3; blue), the facial nerve (yellow), the auriculotemporal nerve (ATN) or third branch of the trigeminal nerve (V3; green), and the auricular branch of the vagus nerve (ABVN; purple). The image on the right shows this innervation in the external auditory meatus (EAM) (Jackler, 2019). (C) The images show the lateral (left) and posterior (right) view of a Spalteholz ear with the auricular vasculature including the superior anterior auricular artery (s), middle anterior auricular artery (m), inferior anterior auricular artery (i), and the superficial temporal artery* (Cakmak et al., 2018). (D) The images show three-dimensional reconstructions produced using micro computed tomography (µCT) of auricular vasculature of the external ear (left) and for isolated auricular vascularity (right) (Cakmak et al., 2018). The images in panels (A, B) were reproduced with permission from the illustrator Chris Gralapp (Jackler, 2019). The images in panels (C, D) were modified from reference (Cakmak et al., 2018).
The external ear is innervated by two sensory cervical (C2-3) brachial nerves known as the great auricular nerve (GAN) and the lesser occipital nerve (LON; Figure 2B). It is also innervated by three different sensory cranial nerves (CN), which are the eighth CN nerve or facial nerve (CN VIII), branches of the third branch of the fifth CN nerve (CN V3) via the auriculotemporal nerve (ATN), and auricular branches of the vagus nerve (ABVN) originating from the superior ganglion of the 10th CN nerve or vagus (CN X). These sensory nerves have overlapping distributions throughout the skin of the ear differentially innervating the helix, concha, fossa, tragus, lobule, EAM, and other regions (Figure 2B). They also serve common functions, such as mediating physiological reflexes and gating neurophysiological arousal via the ascending reticular activating system (Magoun, 1952; Urbin et al., 2021). For example, ABVN innervation of the EAM serves the anatomical basis for Arnold’s cough reflex (Ryan et al., 2014; Tekdemir et al., 1998; Gupta et al., 1986). The EAM is also innervated by branches of the ATN and facial nerve (Figure 2B), which reflects their close functional relationship in underlying the mammalian diving reflex (Andersen, 1963; Gooden, 1994; Lin, 1988; Panneton and Gan, 2020) and trigemino-cardiac reflexes (Khurana et al., 1980; Lapi et al., 2016; Schaller, 2004). Stimulation of the ABVN can also trigger anti-inflammatory responses by modulating cytokine activity (Wu et al., 2023; Salama et al., 2020; Seitz et al., 2022; Tynan et al., 2021). Given these and other effects discussed below, methods and devices for stimulation of peripheral nerves of the external ear have broad biomedical utility (see below, Methods and Effects of Auricular Neuromodulation).
The vascular structure of the external ear includes a rich arterial supply, as well as dense venous and lymphatic drainage system. Several types of perivascular sympathetic and parasympathetic nerve fibers regulate the vasomotor activity of the ear (Cakmak et al., 2018). The external ear receives its primary blood supply from the superior anterior auricular artery, a branch of the external carotid artery. This artery is essential for providing blood to most parts of the external ear, including the anterior and inferior aspects (Figures 2C, D). Additionally, the superior auricular artery serves as a connection between the superficial temporal artery and the middle anterior auricular artery, ensuring a robust collateral blood flow to the ear in case of reduced blood supply from one source. The anterior auricular branch of the superficial temporal artery specifically supplies the anterior portion of the external ear. Further vascularization comes from the occipital artery, which contributes blood to the posterior region of the external ear. The EAM receives blood from both the inferior auricular artery and the auricular branches of the maxillary and superficial temporal arteries (Figures 2C, D). Venous drainage closely follows the arterial supply, with the veins of the external ear running alongside the corresponding arteries. This arteriovenous network is believed to play a major role in thermoregulation. Venous flow from the EAM drains into the pterygoid plexus, external jugular vein, and maxillary vein.
Lymphatic drainage of the external ear is achieved through four primary lymphatic vessels (Pan et al., 2011). The anterior region of the ear is drained by lymphatic branches that converge into a single vessel, which then flows into the pre-auricular lymph nodes. Lymphatic vessels from the superior aspect of the helix may travel along the anterior part of the ear, ultimately reaching the infra-auricular lymph nodes. Similarly, vessels originating in the scaphoid fossa, adjacent to the auricular tubercle, form the middle branches that drain the anterior region of the external ear, also converging toward the infra-auricular nodes. Finally, the lobular branches begin as a network of vessels in the auricular lobule. These vessels merge and drain toward the infra-auricular lymph nodes, forming an organized system of lymphatic drainage across the external ear. The unique anatomy and physiology of the external ear, including its rich vasculature, diverse sensory innervation, and proximity to the brain, make it an ideal location for recording physiological, biochemical, and brain activity data, while also providing an accessible site for stimulating nervous system activity as discussed below.
A major challenge for bioelectronic devices arises from material incompatibility between hard charge carriers (i.e., metal electrodes) and the soft, irregular surface of the skin. Conductive hydrogels, polymers, and biomedical adhesives have been developed to possess skin-like mechanical and electrical properties thereby mitigating many issues encountered when interfacing bioelectronic devices with the body (Iqbal et al., 2021; Keplinger et al., 2013; Lim et al., 2021; Yuk et al., 2019; Li et al., 2023). Hydrogels and conductive polymers have proven particularly advantageous in neuroengineering through the development of soft bioelectronics for neural sensing and neuromodulation interfaces (Jeong et al., 2015; Sunwoo et al., 2020; Zhao et al., 2024). Due to their low melting points and other physicochemical properties, liquid metals for the engineering of flexible, stretchable, and wearable electronics have opened new possibilities in bioelectronics (Deng et al., 2024). Liquid metals printed onto or incorporated into different hydrogel and polymer substrates have enabled the development of electric skin, tattooable circuits, neural interfaces, electronic vessels, and soft thermoelectric heaters (Iqbal et al., 2021; Sreenilayam et al., 2020; Deng et al., 2024; Park et al., 2019; Zhao et al., 2023a).
Coating, spinning, or impregnating natural and synthetic fibers with electric inks and conductive polymers has led to the development of electronically active, smart textiles and fabrics for wearable bioelectronics (Baeg and Lee, 2020; Ismar et al., 2020). These innovations in E-textiles have improved the comfort of devices since conducting metal fibers historically used create uncomfortable sensations against the skin despite their superior electrical conductivity. Carbonized nanoparticles and nanocomposites are presenting interesting approaches to the development of wearable electronic textiles. For instance, chemical vapor deposition of graphene monolayers and transfer from copper substrates has been used to manufacture transparent, flexible graphene fibers capable of serving as textile electrodes (Neves et al., 2015; Fang et al., 2020). Thin piezoelectric films and electroactive papers provide methods of fabricating flexible and conformable mechanically active sensors and actuators, as well as means for energy harvesting and power generation (Khan et al., 2016; Yun et al., 2007; Sezer and Koç, 2021). One interesting application is their use in producing piezoelectric textiles, which pose intriguing possibilities for the future of healthcare and power generation (Atalay et al., 2016; Lund et al., 2018). With the materials, fabrication, and engineering knowledge gained over the last decade in wearable microelectronics, nearly any anatomy can be targeted and affixed with sensors and stimulators that seamlessly integrate hardware with the body. The remainder of this perspective will focus on the human external ear as a target for bioelectronic devices and applications in healthcare, medicine, and communications.
The activity of the vagus nerve underlies core aspects of our health including digestion, cardiovascular reflexes, cardiac activity, immune responses, arousal (sleep/wake, consciousness, and fight/flight/freeze), attention, cognition, learning, and memory. Transcutaneous auricular vagus nerve stimulation (taVNS) involves the non-invasive modulation of auricular branches of the vagus nerve (ABVN; Arnold’s nerve or Adleman’s nerve) located under the skin’s surface of the external ear. Using pulsed electrical currents to modulate ABVN fibers located in different locations of the external ear, this approach has gained attention for its safe ability to modulate autonomic nervous system activity, inflammation, neuroplasticity, attention, stress, learning, mood, and sleep, by altering activity of brain nuclei and neurotransmitters known to regulate these processes such as the locus coeruleus and norepinephrine respectively (Verma et al., 2021; Kim et al., 2022; Urbin et al., 2021; Liu C.-H. et al., 2020; Wang et al., 2021; Butt et al., 2020; Sant'Anna et al., 1992; Tyler, 2017; Tan et al., 2023; Bottari et al., 2024; Srinivasan et al., 2023; Ma et al., 2022; Phillips et al., 2021).
Many methods and devices employing taVNS to date however fall short in providing user comfort due to their reliance on inefficient body-electrode coupling approaches. Discomfort during taVNS manifests as an electrical biting sensation, in part due to electromechanical mismatches between the electrode and skin. High current densities produced by metal electrodes with a small surface area of skin coupling, and metal or rubber electrodes clipped onto the ear create mechanical pinching sensations that are distracting and uncomfortable (Figure 3A). This can be further aggravated by wet coupling methods using saline sprays or electrolyte gels where the microfluidic interface (and local impedance) undergoes frequent fluctuations and distortions between the electrode and skin. For enhancing cognition, reducing stress, or promoting sleep it is critical that the user or patient has a comfortable experience, or the off target, distracting and uncomfortable sensations can override intended taVNS outcomes (Miyatsu et al., 2024; Jigo et al., 2024). In other words, stimulating the external ear with electrical currents can both activate and suppress sympathetic activity (stress) depending on many variables including interface comfort and usability.
Figure 3. Electrical methods of auricular neuromodulation. (A) Some transcutaneous auricular vagus nerve stimulation (taVNS) methods and devices utilize metal clip electrodes like the clip (Soterix Medical, Inc.) shown on the left. These clips are used to mechanically couple the skin to a metal electrode using an electrolyte solution or gel. This approach creates a distracting pinching sensation and can produce electrical biting or prickling sensations. The Xen (Neuvana, Inc.) aVNS device shown (middle-left) implements a different skin-electrode coupling approach using a saline-sprayed conductive rubber electrode placed in the left external acoustic meatus. This creates a distracting wet feeling in the ear of users. Due to body motion, fluid flux, absorption, and dehydration this wet coupling method also leads to electromechanical distortions in the fluid coupling layer between the charge carrier and irregular surfaces of the skin. Other taVNS methods like the Nemos device (Cerbomed GmbH) shown middle-right use small, steel ball electrodes that can produce high current densities resulting in discomfort or electrical biting and stinging sensations. The Tinnoff device (SaluStim Group) shown at right features a different type of taVNS clip electrode that can cause distracting sensations as discussed. (B) Images of the BRAIN Buds taVNS electrodes (IST, LLC) shown in the left and middle panels were developed as conductive hydrogel earbud electrodes to produce an easy-to-use, comfortable user-experience. Using conductive hydrogels to couple electrodes to the skin results in more uniform current distributions and enhanced user comfort during transcutaneous electrical stimulation. As shown on the right, BRAIN Buds were designed as a bilateral taVNS system to be used like earbud headphones.
To overcome human factors issues associated with taVNS, we developed methods of using low-impedance, conductive polymers and soft hydrogel earbud electrodes or electrode interfaces inserted into the EAM to achieve external ear stimulation (Figure 3B) (Tyler et al., 2019; Tyler et al., 2022). Earbud electrodes comprised of a hydrogel inserted in this location target the ABVN, facial nerve, and auriculotemporal branches of the trigeminal nerve located just under the skin of the walls of the external acoustic meatus (Figures 2B, 3B). These external ear stimulation approaches, using conductive hydrogel earbud electrodes inserted into the EAM, formed the basis of methods used and devices designed to enhance foreign language learning (Phillips et al., 2021; Pandža et al., 2020) and relieve tinnitus symptoms (Figure 4A) (Tyler Richard et al., 2024). A major reason modern bioelectronic devices use hydrogel coupling methods is that they reduce electromechanical mismatches across skin-electrode interface resulting in stable, uniform current distributions, enhanced user comfort, and improved electrical efficiency (Keplinger et al., 2013; Lim et al., 2021; Yuk et al., 2019; Jia and Rolandi, 2020; Liu K. et al., 2020; Yang and Suo, 2018; Fu et al., 2020).
Figure 4. Multimodal methods of auricular neuromodulation. (A) The figure illustrates a recent approach combining bilateral, pulsed electrical stimulation of the external ear using hydrogel earbud electrodes inserted into the external acoustic meatus (EAM) presented with notch-filtered auditory stimulation for the treatment of tinnitus (Tyler Richard et al., 2024). (B) The figure illustrates a caloric vestibular stimulation (CVS) system that uses bilateral thermally conductive probes inserted into the EAM. In this embodiment the device uses either hot or cold water circulated through the ear probes to achieve CVS through heating or cooling (Wypych et al., 2019). The images in (A) were adapted from reference (Tyler Richard et al., 2024) and the images in (B) were adapted from reference (Wypych et al., 2019).
Using hydrogel earbud electrodes to achieve comfortable, bimodal, electro-aural stimulation of the external ear during presentation of notch-filtered audio stimuli was recently shown capable of reducing symptoms associated with tinnitus (Figure 4A) (Tyler Richard et al., 2024). Another study recently compared different methods of VNS on language learning. The authors report that taVNS failed to produce an effect on language learning compared to transcutaneous cervical VNS. Interestingly, Miyatsu et al. (2024) implemented a saline sprayed, conductive rubber, earbud electrode that can produce distracting and uncomfortable sensations (Figure 3A). Any distracting electrical sensations produced by the saline-coupled earbud electrodes used in their study might explain why this specific approach to taVNS failed to produce a significant effect on language learning. Further supporting this interpretation, evidence from earlier studies using hydrogel-coupled earbud electrodes to deliver stimulation from the same device indeed led to significant improvements in foreign language learning (Phillips et al., 2021; Tyler et al., 2019). These observations indicate that future taVNS efforts should focus on optimizing electroconductive hydrogels and polymers for comfortably interfacing with the human ear. These efforts can be combined with work to advance neurostimulation algorithms and parameters for continuing to enhance the electrical sensation experiences, ease of use, user comfort, and efficacy of electrical taVNS. This approach should prove valuable considering recent demonstrations that high-frequency (kHz), sub- perceptual taVNS produces significant changes in the functional connectivity of the prefrontal cortex, cingulate cortex, and insula (Mao et al., 2022).
Supported by a growing body of literature, taVNS has immense clinical potential given its ability to provide drug-free, therapeutic approaches to treating some of our most pressing health concerns. Several clinical studies have shown that taVNS is effective for treating insomnia and poor sleep, which is a major contributor to poor health and chronic disease. In a randomized, placebo-controlled study, Zhang et al. (2023) demonstrated that taVNS significantly improved insomnia scores on the Pittsburgh Sleep Quality Index (PSQI) and the Insomnia Severity Index (ISI), as well as by EEG polysomnography compared to sham controls (Zhang et al., 2023). The effects produced by taVNS on PSQI were equivalent to those produced by a cognitive behavior therapy for insomnia (CBT-I) control. Interestingly however, ISI scores demonstrated taVNS produces a more durable effect than CBT-I in follow-up surveys. Another recent randomized, sham-controlled study showed that 8 weeks of taVNS treatment produced significant improvements in insomnia by PSQI scores and that these improvements were maintained for 12 weeks post-treatment compared to controls (Zhang et al., 2024). Several recent functional neuroimaging studies show the effects of taVNS on insomnia are produced by a significant change in the resting state functional connectivity of thalamus, cingulate gyrus, angular gyrus, precuneus, and prefrontal cortex (Zhao et al., 2020; He et al., 2022; Zhao et al., 2023b). With respect to the impacts of poor sleep on daily brain function, another study showed taVNS significantly improves working memory in sleep deprived, stressed human subjects (Zhao R. et al., 2023). Collectively these studies demonstrate that taVNS embodied as auricular bioelectronic devices can transform healthcare by improving the sleep quality in diverse populations suffering from poor sleep quality and efficiency.
Many studies documenting the impacts of taVNS on mental health and quality of life outcomes are also emerging in the literature. Li et al. (2022) demonstrated that 12-week treatment with taVNS produced significant reductions in major depression measured by Hamilton Depression Rating Scores (HAM-D) that were equal to reductions produced by 12-weeks citalopram treatment in a randomized clinical trial designed to compare their effects (Li et al., 2022). An open-label study has demonstrated the proof-of-concept for reducing HAM-D scores while using taVNS to treat peripartum depression (Deligiannidis et al., 2022). Similarly used to treat depression related to life events, a double-blind, randomized, placebo-controlled study recently demonstrated that taVNS produced significant reductions in HAM-D scores while treating post-stroke depression (Liu et al., 2024). Functional neuroimaging studies investigating the effects of taVNS on depression have shown immediate and robust changes in the functional connectivity of several brain circuits following treatment (Sun et al., 2022; Ma et al., 2022; Guo et al., 2024). Several studies have demonstrated that taVNS also produces functional behavioral outcomes that may underlie some aspects of its ability to treat demoralized or depressed moods by restoring proper regulation of psychophysiological arousal. For example, Ferstl et al. (2024) recently conducted a randomized, sham-controlled study showing that taVNS produces significant improvements in invigoration and wanting in subjects suffering from major depression (Ferstl et al., 2024). Another recent randomized, sham-controlled study demonstrated that taVNS produced significant changes in bottom-up neurophysiological arousal leading to significantly improved impulse control during emotional tasks (Camargo et al., 2024). The changes produced by taVNS in these studies are consistent with those expected, which result in improved motivation and mood. Decreased motivation and blunted affect are not only hallmarks of depression, but they are signs and symptoms of over-worked, exhausted, stressed, and over-burdened people struggling with daily life. Interestingly, taVNS has been shown to significantly improve mood recovery following a period of high effort, physical and cognitive exertion (Ferstl et al., 2022). Moreover, another recent human study demonstrated that taVNS significantly improves the invigoration and motivation to work for rewards (Neuser et al., 2020). These studies collectively indicate that electrical taVNS may impact society by improving the motivation, will, and desire of people to work through periods of high stress, depression, and low morale.
In addition to electrical taVNS, other modes of auricular neuromodulation can be intergraded into headphone style devices. Caloric vestibular stimulation (CVS) involves the thermal modulation of the vestibular system by cooling or heating the ear canal (Figure 4B). In a manner similar to electrical taVNS, studies have shown that CVS can modulate brain activity across different cortical regions (Wypych et al., 2019), regulate mood and affect (Preuss et al., 2014), reduce pain evoked potentials (Ferrè et al., 2015), and modulate sensory perception and conscious experience in healthy and brain-damaged patients (Bottini et al., 1995; Bottini et al., 2005; Bottini and Gandola, 2015). A recent study using a wearable, solid-state device embodied as an aluminum, thermal headphone probe to achieve cyclic CVS was effective at reducing both motor and non-motor symptoms in Parkinson’s disease patients following 8 weeks of at home treatment (Wilkinson et al., 2019). Advances in flexible and wearable thermoelectric materials may offer new headphone design opportunities for CVS in therapeutic neuromodulation applications (Wilkinson et al., 2019; Du et al., 2018). Other materials advances in thermally conductive polymers and use of liquid metals for interfacing thermoelectric materials with the skin can improve thermal transfer and insulation to optimize CVS methods (Deng et al., 2024).
Vibrotactile and haptic stimulation of external ear has also shown to have interesting biomedical applications including to mediate human-computer interactions. Targeting vagal nerves innervating the cymba concha, vibrotactile stimulation of the external ear has been shown to modulate arousal (Tan et al., 2024) and reduce cytokine production of TNF, IL-1β and IL-6 to attenuate systemic inflammation in patients with Rheumatoid arthritis (Addorisio et al., 2019). It has been argued the tactile sensitivity of the external ear has been overshadowed by its auditory functions and that haptic stimulation of the ear represents an opportunity for information transfer (Lee et al., 2019). Lee et al. (2019) demonstrated small ear worn haptic stimulation devices could encode environmentally relevant spatiotemporal information by stimulating six different locations on the external ear. In an adaptive embodiment, ear haptics were demonstrated as a human-computer interface to enhance the experience of virtual reality applications for deaf and hard-of-hearing (DHH) individuals (Mirzaei et al., 2020). Haptic stimulation of the ear can convey sound direction in relation to DHH users during a VR experience when a system was not universally designed and intended for hearing enabled persons using spatially encoded audio to simulate sound distance (Mirzaei et al., 2020). The integration of piezoelectric thin films, piezopolymers, and electroactive papers (Khan et al., 2016) into flexible and conformable auricular haptic bioelectronic devices opens fascinating possibilities for medicine and communications. Not only can these materials provide for the design of active stimulation or neuromodulation devices to be worn in the ear, but they can also serve the basis for a wide range of electrophysiological and biochemical sensors to record data for diverse applications in health monitoring and cognitive enhancement.
In some embodiments, auricular bioelectronic devices have sensors that can detect a wide range of physiological, biometric, and chemical signals such as heart rate or brain activity using electroencephalography (EEG), head orientation, and even metabolic markers like lactate. The development of auricular monitoring devices presents challenges like those discussed above for neuromodulation. Integrating advanced sensor materials into devices intended to be worn in or on the ear while ensuring comfort, durability, and accuracy of them in real-world settings can be difficult. The development and use of hydrogels and dry EEG electrode materials represent significant advances over traditional wet electrodes, which require gels and skin preparation. Advances in these materials, flexible electronics, and additive manufacturing has improved the efficiency and comfort of auricular bioelectronic devices making them suitable for continuous everyday use.
Unlike traditional scalp EEG systems, which rely on wet electrodes and conductive gels to reduce impedance, in-ear systems offer a more user-friendly and comfortable experience. For example, in-ear EEG electrodes can be fabricated using viscoelastic materials (memory foam) and silver-coated fabric electrodes (Figure 5A). This approach allows EEG sensors to fit in the EAM while capturing brain signals such as alpha rhythms, visual evoked potentials (VEPs), steady-state visual evoked potentials, and auditory steady-state responses (Goverdovsky et al., 2017). The approach was also useful for conducting polysomnography or recording brain activity during sleep (Goverdovsky et al., 2017). A more custom approach involves the fabrication of individualized EEG earbud electrodes. Joyner et al. (2024) recently used optical scans of individual patient’s ears to create custom EEG earbud electrodes made from a soft silicon material and conductive polymer-coated silver rivets (Figure 5B). These electrodes were used to monitor epileptic activity in validation studies, which demonstrated the earbuds can provide patients with a discrete and comfortable EEG device for continuous monitoring while offering clinicians reliable and accurate data compared to intracranial and scalp recording methods (Joyner et al., 2024). Beyond this type of clinical diagnostic application, there are opportunities to develop auricular bioelectronics for brain-computer interfaces (BCI’s) and human performance monitoring.
Figure 5. Electrophysiological sensing methods for auricular bioelectronics. (A) The images depict an in-ear EEG electrode constructed using viscoelastic foam and Ag impregnated fabric used to record brain activity at rest, during evoked potentials, and during sleep (Goverdovsky et al., 2017). (B) Custom bilateral, auricular EEG electrodes made from a soft silicone, molded from high-resolution optical scans of the external ears of patients. The auricular EEG system was used to monitor epileptic seizure activity demonstrating good performance compared to scalp and intracranial EEG (Joyner et al., 2024). The images in (A) were adopted from reference (Goverdovsky et al., 2017) and the images in (B) were adopted from reference (Joyner et al., 2024).
EEG metrics provide insights into how focused or mentally engaged a person may be, making them invaluable for applications in cognitive performance and mental health. By tracking these metrics, auricular bioelectronics can offer real-time feedback on mental states, enhancing both medical and consumer applications. To reach the scalable potential of these approaches however, highly personalized auricular bioelectronics as described above will need to be developed. Another innovative approach towards developing individualized, in-ear bioelectronics was recently demonstrated using spiral shaped, electrothermal actuating electrodes (SpiralE) that conform to the EAM structure of users (Figure 6A) (Wang et al., 2023). Wang et al. (2023) engineered a flexible electrode using double-layer shape memory polymers embedded in an electrothermal actuation layer with an EEG detection top layer comprised of Au wires insulated in polyimide (Wang et al., 2023). This design enabled in-ear EEG electrodes to comfortably conform to the shape of the ear of individual users (Figure 6A), while being worn and used for high fidelity recordings in visual and auditory brain-computer interfaces (BCI’s) (Wang et al., 2023). These studies have shown that the quality of the signal for EEG obtained through auricular approaches is sufficient to monitor many brain states in a manner equivalent to conventional scalp EEG. In addition, by monitoring electrical impedance and heart rate and respiration from one integrated device can improve the reliability of recordings within and across recording sessions. In addition to EEG monitoring, these devices have incorporated various other sensors, including accelerometers for tracking head movements and orientation, as well as biochemical sensors for detecting metabolic markers like lactate during physical activity.
Figure 6. Flexible auricular bioelectronics for electrophysiological and biochemical sensing. (A) The images on the left show a spiralized, electrothermally conforming EEG electrode (SpiralE) designed to fit in the external auditory meatus (EAM) of users (Wang et al., 2023). The SpiralE conforms to the shape of individual user’s EAM for a comfortable and electrically efficient fit. The images in the middle show the SpiralE in a baseline state (top) and adapted state (bottom). The images on the right show SpiralE inserted into the EAM of a user in its conformed state. The SpiralE auricular EEG electrode was demonstrated to be effective for recording brain activity patterns useful in auditory and visual brain-computer interface embodiments (Wang et al., 2023). (B) The images on the left depict an approach to recording brain activity using EEG sensors and recording biochemical signals from sweat glands in the ear using flexible, multimodal sensing, auricular electrodes (Xu et al., 2023). The image in the middle shows a photograph of the flexible, multi-electrode array with EEG sensors and electrochemical sensors designed to detect lactate. The photographs on the right show the multi-electrode array mounted onto an earbud chassis to create an auricular sensor capable of sensing brain activity and lactate. This approach was demonstrated useful for recording brain activity and lactate in human subjects during exercise (Xu et al., 2023). The images in (A) were adopted from reference (Wang et al., 2023) and the images in (B) were adopted from reference (Xu et al., 2023).
Optical sensors can also be integrated into headphones to record heart rate, SpO2 (blood oxygen saturation), VO2max and other physiological metrics. These optical sensors use photoplethysmography (PPG) technology to measure blood flow non-invasively, allowing for continuous monitoring of cardiovascular health in auricular bioelectronic devices (Patterson et al., 2009; Leboeuf et al., 2014; Passler et al., 2019). Piezoelectric and MEMS-based sensors capable of detecting heart rate through pressure fluctuations in the ear have also been shown useful for cardiac monitoring (Park et al., 2015; Cui et al., 2022). Advances in thermoforming techniques allow for the creation of custom and generic earpieces that can house multiple sensors while maintaining comfort and stability. Flexible and stretchable materials are used to integrate multiple sensors into a compact design, suitable for long-term wear. Xu et al. (2023) recently developed integrated electrochemical, chronoamperometry sensors with flexible Ag electrodes, which were 3D-printed, coated with a layer of PVA hydrogel, bonded to a flexible PCB, and mounted into an earphone assembly to simultaneously record lactate from sweat and EEG from the ear during exercise (Figure 6B) (Xu et al., 2023). This multimodal sensing approach including biochemical measures from sweat may be useful in monitoring other variables beyond lactate during EEG, HR, and head position. For instance, obtaining measures of stress hormones or drug metabolites continuously to gain insights related to human performance using auricular bioelectronic devices may be particularly useful.
Another exciting area of development in auricular bioelectronics is the integration of spatial audio with sensor data, such as EEG and accelerometry. Spatial audio enhances the auditory experience by simulating how sound moves in the user’s environment. With the help of accelerometers and gyroscopes, which track head movements in real-time, spatial audio can adjust sound orientation based on the user’s head position, maintaining a consistent and immersive auditory experience. This data, combined with EEG monitoring of cognitive load, attention, and engagement can optimize the auditory experience for various activities, such as work, studying, relaxation, focus, or gaming. Wireless in-ear EEG systems, with multi-channel, multimodal recording capabilities will continue to expand the potential for BCIs and neurotechnology. As the capabilities of wearable auricular devices continue to evolve, the integration of both stimulation and sensing functions within a closed-loop system opens new possibilities for real-time neuromodulation, personalized health interventions, and advanced brain-computer interfaces.
Closed-loop, auricular bioelectronics possess transformative potential for neuromodulation by integrating real-time sensing and stimulation capabilities. One prominent application would be for the treatment of atrial fibrillation or arrhythmias, where in-ear sensors can monitor for abnormal heart rhythms while taVNS provides corrective feedback. The sensing of arrhythmic events using PPG or piezoelectric-based heart rate sensors embedded in the ear canal could trigger taVNS to regulate parasympathetic activity and restore normal cardiac rhythms (Kharbanda et al., 2022; Murray et al., 2016; Stavrakis et al., 2020). Similarly, stimulation of vagal or trigeminal fibers may hold potential for treating some forms of sleep apnea and sleep-disordered breathing (Ratneswaran et al., 2023; Chowdhury et al., 2017). In this embodiment, auricular bioelectronics can be engineered to sense abnormal breathing patterns to trigger responsive stimulation of trigeminal and ABVN fibers in the EAM to reduce airway resistance and restore proper respiration during sleep.
There are other promising avenues for closed-loop auricular bioelectronics, such as for the treatment of neuromuscular disorders like Restless Leg Syndrome (RLS) which benefits from taVNS treatments (Hartley et al., 2023a; Hartley et al., 2023b; Merkl et al., 2007). Such an embodiment may include recordings of muscle activity using wireless electromyography (EMG) sensors placed on the legs to detect RLS episodes for triggering taVNS to mitigate symptoms and discomfort. In a similar fashion, EMG sensors placed in auricular bioelectronic devices could detect abnormal activity associated with nocturnal bruxism and responsively trigger taVNS stimulation protocols, which can reduce bruxism severity (Polini and Budai, 2022). Methods for cognitive enhancement and attention regulation with closed-loop auricular bioelectronics are also ripe for development. In-ear EEG sensors capable of monitoring neural activity related to attention and cognitive engagement can be paired with taVNS to enhance vigilance or sustained attention (Tyler, 2017). Cognitive enhancement with closed-loop taVNS has potential applications for treating attention, memory, and learning disorders, as well as for enhancing human performance in high-stakes environments, such as in military, first responder, or aerospace operations, where maintaining optimal cognitive function and decision-making processes under stress is critical (McKinley et al., 2011; Musson et al., 2004; Dhami et al., 2015; Picano et al., 2006).
In human performance enhancement or health applications, closed-loop systems can help regulate stress responses by continuously monitoring physiological and biochemical stress markers. Integrated electrochemical or optical sensors in auricular devices can detect cortisol or other stress hormones, while heart rate variability sensors can report sympathetic tone. When elevated stress is detected, auricular bioelectronic devices can deliver taVNS protocols to reduce sympathetic activity and promote parasympathetic activation thereby dampening stress and improving overall wellbeing. Additionally, the integration of these sensors and stimulation methods with BCIs creates opportunities for enhanced human-computer interactions like accelerating human learning. As auricular bioelectronics advance, they will enable seamless, scalable devices that integrate into our daily lives just like personal headphones do today. The next generation of headphones embodied as auricular bioelectronics will provide for the global delivery of several new medical therapies, human-computer interfaces, and communication technologies.
Auricular bioelectronics are poised to revolutionize healthcare and human performance by providing a versatile, wearable, non-invasive platform for real-time monitoring and neuromodulation. These devices, integrating advanced sensors and stimulation capabilities, hold potential for treating a wide range of conditions, from arrhythmias and sleep apnea to inflammation and cognitive disorders. As material science and flexible electronics continue to advance, auricular bioelectronics will become increasingly dynamic, comfortable, effective, and personalized. With scalable designs akin to personal headphones, these devices are likely to play a significant role in BCIs, offering yet unrealized opportunities for improving health, cognition, and human-machine interactions.
One of the most promising future applications of auricular bioelectronics is to enable the scalable delivery of on-demand, digital immunotherapies to treat acute and chronic inflammation. It is well established that chronic inflammation is a number one cause of disease and death, as well as a major driver of health disparity. By modulating the cholinergic anti-inflammatory pathway, it has been shown that taVNS can reduce acute and chronic inflammation in several conditions (Wu et al., 2023; Salama et al., 2020; Liu et al., 2020a; van Beekum et al., 2022; Kaniusas et al., 2020; Mastitskaya et al., 2021). Embodied as a set of headphones taVNS may provide the ability to replace many over the counter and prescription drugs like non-steroidal anti-inflammatory drugs, steroidal compounds, antihistamines, and others used one to treat inflammation. The integration of these approaches with closed-loop monitoring of cytokines and other inflammatory biomarkers using wearable bioelectronic methods will enable responsive taVNS for real-time, personalized, digital immunotherapeutic devices, which may be capable of disrupting the medical device, pharmaceutical, and consumer health industries (Figure 7).
Figure 7. Auricular and wearable bioelectronics for personalized, digital immunotherapies. The figure shows a closed-loop, reactive transcutaneous auricular vagus nerve stimulation (taVNS) device worn as headphones. The taVNS device is shown integrated into a system comprised of: (1) a wearable sensor containing microneedles for monitoring cytokines from the interstitial fluid (ISF) using electrochemical and photoelectric methods; and (2) a networked layer for computation of a predictive Inflammation Index or score from periodic cytokine sampling to reflect an individual’s state of inflammation. Decisions made in the network layer will serve to (3) trigger taVNS protocols to (4) reduce inflammation via the cholinergic anti-inflammatory pathway regulated by the vagus nerve. This is an exemplar of future auricular bioelectronic devices that can be used to treat acute and chronic inflammation causing many health problems and concerns.
While auricular bioelectronics hold great promise, several pitfalls and limitations must be addressed for these technologies to achieve their full potential. First, there are challenges associated with overcoming the technological barriers to developing reliable, long-lasting, and comfortable wearable devices. Ensuring the accuracy, reliability, durability of sensors, particularly in real-world environments with variable conditions, is essential for making closed-loop systems robust and effective. Optimizing energy efficiency will require attention since microelectronics require advanced power management systems to enable continuous operation. Several barriers to adoption might be related to the cost and complexity of engineering devices, which may limit access to those who can afford them unless manufacturing and production costs can be minimized. The lack of public understanding of potential risks, benefits, and functions of auricular bioelectronics could cause hesitancy among consumers, patients, and healthcare providers.
From a societal and ethical perspective, data privacy and security will always pose concerns and challenges. Auricular bioelectronics, which continuously collect sensitive biometric and neural data, could pose privacy risks if not properly protected and safeguarded. Another ethical consideration as alluded above is the availability and accessibility of this technology, which could exacerbate existing healthcare disparities if not distributed equitably. Despite these challenges, the next era of technological developments, including continued advances in flexible electronics, packaging, improved sensor algorithms, and improved energy harvesting methods, will drive innovation forward in the development of auricular bioelectronics. It will be critical to form collaborations between medical, research, semiconductor, and regulatory organizations to establish standards and guidelines to ensure auricular bioelectronics are safe, effective, and accessible to a broad range of users. These collaborations will ultimately pave the way for the integration of auricular bioelectronics into everyday healthcare and cognitive performance enhancement.
WT: Writing–original draft, Writing–review and editing.
The author(s) declare that financial support was received for the research, authorship, and/or publication of this article. The development of BRAIN Buds by IST, LLC was funded based on research sponsored by Air Force Research Laboratory under agreement number FA8650-18-2-5402. The U.S. Government is authorized to reproduce and distribute reprints for Government purposes notwithstanding any copyright notation thereon. The views and conclusions contained herein are those of the authors and should not be interpreted as necessarily representing the official policies or endorsements, either expressed or implied, of Air Force Research Laboratory (AFRL) or the U.S. Government.
WJT is a co-founder of IST, LLC and inventor of patents covering neuromodulation methods and devices, which are described in this manuscript.
The author(s) declare that no Generative AI was used in the creation of this manuscript.
All claims expressed in this article are solely those of the authors and do not necessarily represent those of their affiliated organizations, or those of the publisher, the editors and the reviewers. Any product that may be evaluated in this article, or claim that may be made by its manufacturer, is not guaranteed or endorsed by the publisher.
Acosta, J. N., Falcone, G. J., Rajpurkar, P., and Topol, E. J. (2022). Multimodal biomedical AI. Nat. Med. 28 (9), 1773–1784. doi:10.1038/s41591-022-01981-2
Addorisio, M. E., Imperato, G. H., de Vos, A. F., Forti, S., Goldstein, R. S., Pavlov, V. A., et al. (2019). Investigational treatment of rheumatoid arthritis with a vibrotactile device applied to the external ear. Bioelectron. Med. 5, 4. doi:10.1186/s42234-019-0020-4
Andersen, H. T. (1963). The reflex nature of the physiological adjustments to diving and their afferent pathway. Acta Physiol. Scand. 58, 263–273. doi:10.1111/j.1748-1716.1963.tb02648.x
Apple, I. (2024). “Apple introduces groundbreaking health features,” in Apple newsroom. Available at: https://www.apple.com/newsroom/2024/09/apple-introduces-groundbreaking-health-features/.
Atalay, A., Atalay, O., Husain, M. D., Fernando, A., and Potluri, P. (2016). Piezofilm yarn sensor-integrated knitted fabric for healthcare applications. J. Industrial Text. 47 (4), 505–521. doi:10.1177/1528083716652834
Baeg, K.-J., and Lee, J. (2020). Flexible electronic systems on plastic substrates and textiles for smart wearable technologies. Adv. Mater. Technol. 5 (7), 2000071. doi:10.1002/admt.202000071
Bottari, S. A., Lamb, D. G., Porges, E. C., Murphy, A. J., Tran, A. B., Ferri, R., et al. (2024). Preliminary evidence of transcutaneous vagus nerve stimulation effects on sleep in veterans with post-traumatic stress disorder. J. Sleep Res. 33 (1), e13891. doi:10.1111/jsr.13891
Bottini, G., and Gandola, M. (2015). Beyond the non-specific attentional effect of caloric vestibular stimulation: evidence from healthy subjects and patients. Multisens. Res. 28 (5-6), 591–612. doi:10.1163/22134808-00002504
Bottini, G., Paulesu, E., Gandola, M., Loffredo, S., Scarpa, P., Sterzi, R., et al. (2005). Left caloric vestibular stimulation ameliorates right hemianesthesia. Neurology 65 (8), 1278–1283. doi:10.1212/01.wnl.0000182398.14088.e8
Bottini, G., Paulesu, E., Sterzi, R., Warburton, E., Wise, R. J. S., Vallar, G., et al. (1995). Modulation of conscious experience by peripheral sensory stimuli. Nature 376 (6543), 778–781. doi:10.1038/376778a0
Butt, M. F., Albusoda, A., Farmer, A. D., and Aziz, Q. (2020). The anatomical basis for transcutaneous auricular vagus nerve stimulation. J. Anat. 236 (4), 588–611. doi:10.1111/joa.13122
Cakmak, Y. O., Cotofana, S., Jäger, C., Morawski, M., Sora, M. C., Werner, M., et al. (2018). Peri-arterial autonomic innervation of the human ear. Sci. Rep. 8 (1), 11469. doi:10.1038/s41598-018-29839-z
Camargo, L., Pacheco-Barrios, K., Gianlorenço, A. C., Menacho, M., Choi, H., Song, J. J., et al. (2024). Evidence of bottom-up homeostatic modulation induced taVNS during emotional and Go/No-Go tasks. Exp. Brain Res. 242 (9), 2069–2081. doi:10.1007/s00221-024-06876-x
Channa, A., Popescu, N., Skibinska, J., and Burget, R. (2021). The rise of wearable devices during the COVID-19 pandemic: a systematic review. Sensors 21 (17), 5787. doi:10.3390/s21175787
Chowdhury, T., Bindu, B., Singh, G. P., and Schaller, B. (2017). Sleep disorders: is the trigemino-cardiac reflex a missing link? Front. Neurology, Mini Rev. 8, 63. doi:10.3389/fneur.2017.00063
Chung, K. (2004). Challenges and recent developments in hearing aids: Part I. Speech understanding in noise, microphone technologies and noise reduction algorithms. Trends Amplif. 8 (3), 83–124. doi:10.1177/108471380400800302
Cui, J., Li, Y., Yang, Y., Shi, P., Wang, B., Wang, S., et al. (2022). Design and optimization of MEMS heart sound sensor based on bionic structure. Sensors Actuators A Phys. 333, 113188. doi:10.1016/j.sna.2021.113188
Deligiannidis, K. M., Robakis, T., Homitsky, S. C., Ibroci, E., King, B., Jacob, S., et al. (2022). Effect of transcutaneous auricular vagus nerve stimulation on major depressive disorder with peripartum onset: a multicenter, open-label, controlled proof-of-concept clinical trial (DELOS-1). J. Affect. Disord. 316, 34–41. doi:10.1016/j.jad.2022.07.068
Deng, Y., Bu, F., Wang, Y., Chee, P. S., Liu, X., and Guan, C. (2024). Stretchable liquid metal based biomedical devices. npj Flex. Electron. 8 (1), 12. doi:10.1038/s41528-024-00298-z
Dhami, M. K., Mandel, D. R., Mellers, B. A., and Tetlock, P. E. (2015). Improving intelligence analysis with decision science. Perspect. Psychol. Sci. 10 (6), 753–757. doi:10.1177/1745691615598511
Du, Y., Xu, J., Paul, B., and Eklund, P. (2018). Flexible thermoelectric materials and devices. Appl. Mater. Today 12, 366–388. doi:10.1016/j.apmt.2018.07.004
Edwards, B. (2007). The future of hearing aid technology. Trends Amplif. 11 (1), 31–45. doi:10.1177/1084713806298004
Fang, B., Chang, D., Xu, Z., and Gao, C. (2020). A review on graphene fibers: expectations, advances, and prospects. Adv. Mater. 32 (5), 1902664. doi:10.1002/adma.201902664
F. D. A. Commisioner Office (2024a). FDA finalizes historic rule enabling access to over-the-counter hearing aids for millions of Americans. FDA. Available at: https://web.archive.org/web/20221028042729/https:/www.fda.gov/news-events/press-announcements/fda-finalizes-historic-rule-enabling-access-over-counter-hearing-aids-millions-americans.
F. D. A. Commissioner Office (2024b). FDA authorizes first over-the-counter hearing aid software. FDA. Available at: https://www.fda.gov/news-events/press-announcements/fda-authorizes-first-over-counter-hearing-aid-software.
Ferrè, E. R., Haggard, P., Bottini, G., and Iannetti, G. D. (2015). Caloric vestibular stimulation modulates nociceptive evoked potentials. Exp. Brain Res. 233 (12), 3393–3401. doi:10.1007/s00221-015-4412-8
Ferstl, M., Kühnel, A., Klaus, J., Lin, W. M., and Kroemer, N. B. (2024). Non-invasive vagus nerve stimulation conditions increased invigoration and wanting in depression. Compr. Psychiatry 132, 152488. doi:10.1016/j.comppsych.2024.152488
Ferstl, M., Teckentrup, V., Lin, W. M., Kräutlein, F., Kühnel, A., Klaus, J., et al. (2022). Non-invasive vagus nerve stimulation boosts mood recovery after effort exertion. Psychol. Med. 52 (14), 3029–3039. doi:10.1017/s0033291720005073
Fu, Y., Zhao, J., Dong, Y., and Wang, X. (2020). Dry electrodes for human bioelectrical signal monitoring. Sensors 20 (13), 3651. doi:10.3390/s20133651
Gooden, B. A. (1994). Mechanism of the human diving response. Integr. Physiol. Behav. Sci. 29 (1), 6–16. doi:10.1007/bf02691277
Goverdovsky, V., von Rosenberg, W., Nakamura, T., Looney, D., Sharp, D. J., Papavassiliou, C., et al. (2017). Hearables: multimodal physiological in-ear sensing. Sci. Rep. 7 (1), 6948. doi:10.1038/s41598-017-06925-2
Guo, Z. P., Liao, D., Chen, L., Wang, C., Qu, M., Lv, X. Y., et al. (2024). Transcutaneous auricular vagus nerve stimulation modulating the brain topological architecture of functional network in major depressive disorder: an fMRI study. Brain Sci. 14 (9), 945. doi:10.3390/brainsci14090945
Gupta, D., Verma, S., and Vishwakarma, S. K. (1986). Anatomic basis of Arnold's ear-cough reflex. Surg. Radiol. Anat. 8 (4), 217–220. doi:10.1007/bf02425070
Haghi, M., Thurow, K., and Stoll, R. (2017). Wearable devices in medical internet of things: scientific research and commercially available devices. Healthc. Inf. Res. 23 (1), 4–15. doi:10.4258/hir.2017.23.1.4
Hartley, S., Bao, G., Russo, A., Zagdoun, M., Chevallier, S., Lofaso, F., et al. (2023a). Self-administered non-invasive vagus nerve stimulation therapy for severe pharmacoresistant restless legs syndrome: outcomes at 6 months. J. Sleep. Res. 33, e14066. doi:10.1111/jsr.14066
Hartley, S., Bao, G., Zagdoun, M., Chevallier, S., Lofaso, F., Leotard, A., et al. (2023b). Noninvasive vagus nerve stimulation: a new therapeutic approach for pharmacoresistant restless legs syndrome. Neuromodulation J. Int. Neuromodulation Soc. 26 (3), 629–637. doi:10.1016/j.neurom.2022.10.046
Hasan, M. N., Sahlan, S., Osman, K., and Mohamed Ali, M. S. (2021). Energy harvesters for wearable electronics and biomedical devices. Adv. Mater. Technol. 6 (3), 2000771. doi:10.1002/admt.202000771
He, J.-K., Jia, B. H., Wang, Y., Li, S. Y., Zhao, B., Zhou, Z. G., et al. (2022). Transcutaneous auricular vagus nerve stimulation modulates the prefrontal cortex in chronic insomnia patients: fMRI study in the first session. Front. Neurology 13, 827749. doi:10.3389/fneur.2022.827749
Iqbal, S. M. A., Mahgoub, I., Du, E., Leavitt, M. A., and Asghar, W. (2021). Advances in healthcare wearable devices. npj Flex. Electron. 5 (1), 9. doi:10.1038/s41528-021-00107-x
Ismar, E., Kurşun Bahadir, S., Kalaoglu, F., and Koncar, V. (2020). Futuristic clothes: electronic textiles and wearable technologies. Glob. Challenges 4 (7), 1900092. doi:10.1002/gch2.201900092
Jackler, R. K. (2019). Ear surgery illustrated: a comprehensive atlas of otologic microsurgical techniques. 1st edition ed. Thieme, 1297.
Jeong, J.-W., Shin, G., Park, S. I., Yu, Ki J., Xu, L., and Rogers, J. A. (2015). Soft materials in neuroengineering for hard problems in neuroscience. Neuron 86 (1), 175–186. doi:10.1016/j.neuron.2014.12.035
Jia, M., and Rolandi, M. (2020). Soft and ion-conducting materials in bioelectronics: from conducting polymers to hydrogels. Adv. Healthc. Mater. 9 (5), 1901372. doi:10.1002/adhm.201901372
Jigo, M., Carmel, J. B., Wang, Q., and Rodenkirch, C. (2024). Transcutaneous cervical vagus nerve stimulation improves sensory performance in humans: a randomized controlled crossover pilot study. Sci. Rep. 14 (1), 3975. doi:10.1038/s41598-024-54026-8
Joyner, M., Hsu, S. H., Martin, S., Dwyer, J., Chen, D. F., Sameni, R., et al. (2024). Using a standalone ear-EEG device for focal-onset seizure detection. Bioelectron. Med. 10 (1), 4. doi:10.1186/s42234-023-00135-0
Kaniusas, E., Kampusch, S., Tittgemeyer, M., Panetsos, F., Gines, R. F., Papa, M., et al. (2019). Current directions in the auricular vagus nerve stimulation I – a physiological perspective. Front. Neurosci. 13, 854. doi:10.3389/fnins.2019.00854
Kaniusas, E., Szeles, J. C., Kampusch, S., Alfageme-Lopez, N., Yucuma-Conde, D., Li, X., et al. (2020). Non-invasive auricular vagus nerve stimulation as a potential treatment for covid19-originated acute respiratory distress syndrome. Front. Physiology, Hypothesis Theory 11, 890. doi:10.3389/fphys.2020.00890
Keplinger, C., Sun, J.-Y., Foo, C. C., Rothemund, P., Whitesides, G. M., and Suo, Z. (2013). Stretchable, transparent, ionic conductors. Science 341 (6149), 984–987. doi:10.1126/science.1240228
Khan, A., Abas, Z., Soo Kim, H., and Oh, I.-K. (2016). Piezoelectric thin films: an integrated review of transducers and energy harvesting. Smart Mater. Struct. 25 (5), 053002. doi:10.1088/0964-1726/25/5/053002
Kharbanda, R. K., van der Does, W. F. B., van Staveren, L. N., Taverne, Y. J. H. J., Bogers, A. J. J. C., and de Groot, N. M. S. (2022). Vagus nerve stimulation and atrial fibrillation: revealing the paradox. Neuromodulation 25 (3), 356–365. doi:10.1016/j.neurom.2022.01.008
Khurana, R. K., Watabiki, S., Hebel, J. R., Toro, R., and Nelson, E. (1980). Cold face test in the assessment of trigeminal-brainstem-vagal function in humans. Ann. Neurol. 7 (2), 144–149. doi:10.1002/ana.410070209
Kim, A. Y., Marduy, A., de Melo, P. S., Gianlorenco, A. C., Kim, C. K., Choi, H., et al. (2022). Safety of transcutaneous auricular vagus nerve stimulation (taVNS): a systematic review and meta-analysis. Sci. Rep. 12 (1), 22055. doi:10.1038/s41598-022-25864-1
Knoetze, M., Manchaiah, V., Oosthuizen, I., Beukes, E., and Swanepoel, D. W. (2024). Perspectives on hearing aid cost and uptake for prescription and over-the-counter hearing aid users. Am. J. Audiology 33 (3), 942–952. doi:10.1044/2024_AJA-23-00116
Lapi, D., Scuri, R., and Colantuoni, A. (2016). Trigeminal cardiac reflex and cerebral blood flow regulation. Front. Neurosci. 10, 470. doi:10.3389/fnins.2016.00470
Leboeuf, S. F., Aumer, M. E., Kraus, W. E., Johnson, J. L., and Duscha, B. (2014). Earbud-based sensor for the assessment of energy expenditure, HR, and VO2max. Med. Sci. Sports Exerc 46 (5), 1046–1052. doi:10.1249/mss.0000000000000183
Lee, M., Je, S., Lee, W., Ashbrook, D., and Bianchi, A. (2019). ActivEarring: spatiotemporal haptic cues on the ears. IEEE Trans. Haptics 12 (4), 554–562. doi:10.1109/toh.2019.2925799
Le Maître, A., Grunstra, N. D. S., Pfaff, C., and Mitteroecker, P. (2020). Evolution of the mammalian ear: an evolvability hypothesis. Evol. Biol. 47 (3), 187–192. doi:10.1007/s11692-020-09502-0
Li, S., Rong, P., Wang, Y., Jin, G., Hou, X., Li, S., et al. (2022). Comparative effectiveness of transcutaneous auricular vagus nerve stimulation vs citalopram for major depressive disorder: a randomized trial. Neuromodulation Technol. A. T. Neural Interface 25 (3), 450–460. doi:10.1016/j.neurom.2021.10.021
Li, Y., Li, N., Liu, W., Prominski, A., Kang, S., Dai, Y., et al. (2023). Achieving tissue-level softness on stretchable electronics through a generalizable soft interlayer design. Nat. Commun. 14 (1), 4488. doi:10.1038/s41467-023-40191-3
Lieberman, A., Schroeder, J., and Amir, O. (2022). A voice inside my head: the psychological and behavioral consequences of auditory technologies. Organ. Behav. Hum. Decis. Process. 170, 104133. doi:10.1016/j.obhdp.2022.104133
Lim, C., Hong, Y. J., Jung, J., Shin, Y., Sunwoo, S. H., Baik, S., et al. (2021). Tissue-like skin-device interface for wearable bioelectronics by using ultrasoft, mass-permeable, and low-impedance hydrogels. Sci. Adv. 7 (19), eabd3716. doi:10.1126/sciadv.abd3716
Lin, Y. C. (1988). Applied physiology of diving. Sports Med. 5 (1), 41–56. doi:10.2165/00007256-198805010-00004
Liu, C., Tang, H., Liu, C., Ma, J., Liu, G., Niu, L., et al. (2024). Transcutaneous auricular vagus nerve stimulation for post-stroke depression: a double-blind, randomized, placebo-controlled trial. J. Affect Disord. 354, 82–88. doi:10.1016/j.jad.2024.03.005
Liu, C.-H., Yang, M. H., Zhang, G. Z., Wang, X. X., Li, B., Li, M., et al. (2020a). Neural networks and the anti-inflammatory effect of transcutaneous auricular vagus nerve stimulation in depression. J. Neuroinflammation 17 (1), 54. doi:10.1186/s12974-020-01732-5
Liu, K., Wei, S., Song, L., Liu, H., and Wang, T. (2020b). Conductive hydrogels—a novel material: recent advances and future perspectives. J. Agric. Food Chem. 68 (28), 7269–7280. doi:10.1021/acs.jafc.0c00642
Lund, A., Rundqvist, K., Nilsson, E., Yu, L., Hagström, B., and Müller, C. (2018). Energy harvesting textiles for a rainy day: woven piezoelectrics based on melt-spun PVDF microfibres with a conducting core. npj Flex. Electron. 2 (1), 9. doi:10.1038/s41528-018-0022-4
Ma, Y., Wang, Z., He, J., Sun, J., Guo, C., Du, Z., et al. (2022). Transcutaneous auricular vagus nerve immediate stimulation treatment for treatment-resistant depression: a functional magnetic resonance imaging study. Front. Neurology 13, 931838. doi:10.3389/fneur.2022.931838
Magoun, H. W. (1952). An ascending reticular activating system in the brain stem. A.M.A. Archives Neurology and Psychiatry 67 (2), 145–154. doi:10.1001/archneurpsyc.1952.02320140013002
Mamdiwar, S. D., R, A., Shakruwala, Z., Chadha, U., Srinivasan, K., and Chang, C.-Y. (2021). Recent advances on IoT-assisted wearable sensor systems for healthcare monitoring. Biosensors 11 (10), 372. doi:10.3390/bios11100372
Mao, Y., Chen, C., Falahpour, M., MacNiven, K. H., Heit, G., Sharma, V., et al. (2022). Effects of sub-threshold transcutaneous auricular vagus nerve stimulation on cingulate cortex and insula resting-state functional connectivity. Front. Hum. Neurosci. Orig. Res. 16, 862443. doi:10.3389/fnhum.2022.862443
Masè, M., Micarelli, A., and Strapazzon, G. (2020). Hearables: new perspectives and pitfalls of in-ear devices for physiological monitoring. A scoping review. Front. Physiology, Syst. Rev. 11, 568886. doi:10.3389/fphys.2020.568886
Mastitskaya, S., Thompson, N., and Holder, D. (2021). Selective vagus nerve stimulation as a therapeutic approach for the treatment of ards: a rationale for neuro-immunomodulation in COVID-19 disease. Front. Neurosci. Mini Rev. 15, 667036. doi:10.3389/fnins.2021.667036
McKinley, R. A., McIntire, L. K., and Funke, M. A. (2011). Operator selection for unmanned aerial systems: comparing video game players and pilots. Aviat. Space, Environ. Med. 82 (6), 635–642. doi:10.3357/ASEM.2958.2011
Mercadier, E. J. P. (2024). Bi-Telephone. Pat. US454138A, 1891-06-16, 1891. Available at: https://patents.google.com/patent/US454138A/en.
Merkl, A., Brakemeier, E. L., Danker-Hopfe, H., and Bajbouj, M. (2007). Vagus nerve stimulation improves restless legs syndrome associated with major depression: a case report. J. Clin. Psychiatry 68 (4), 635–636. doi:10.4088/jcp.v68n0423c
Mirzaei, M., Kan, P., and Kaufmann, H. (2020). EarVR: using ear haptics in virtual reality for deaf and hard-of-hearing people. IEEE Trans. Vis. Comput. Graph 26 (5), 2084–2093. doi:10.1109/tvcg.2020.2973441
Miyatsu, T., Oviedo, V., Reynaga, J., Karuzis, V. P., Martinez, D., O’Rourke, P., et al. (2024). Transcutaneous cervical vagus nerve stimulation enhances second-language vocabulary acquisition while simultaneously mitigating fatigue and promoting focus. Sci. Rep. 14 (1), 17177. doi:10.1038/s41598-024-68015-4
Molesworth, B. R. C., Burgess, M., and Kwon, D. (2013). The use of noise cancelling headphones to improve concurrent task performance in a noisy environment. Appl. Acoust. 74 (1), 110–115. doi:10.1016/j.apacoust.2012.06.015
Møller, H. (1992). Fundamentals of binaural technology. Appl. Acoust. 36 (3), 171–218. doi:10.1016/0003-682X(92)90046-U
Møller, H., Jensen, C. B., Hammershøi, D., and Sørensen, M. F. (1995). Design criteria for headphones. J. Audio Eng. Soc. 43 (4), 218–232. Available at: https://www.aes.org/e-lib/browse.cfm?elib=10274.
Murray, A. R., Atkinson, L., Mahadi, M. K., Deuchars, S. A., and Deuchars, J. (2016). The strange case of the ear and the heart: the auricular vagus nerve and its influence on cardiac control. Aut. Neurosci. 199, 48–53. doi:10.1016/j.autneu.2016.06.004
Musson, D. M., Sandal, G. M., and Helmreich, R. L. (2004). Personality characteristics and trait clusters in final stage astronaut selection. Aviat. Space, Environ. Med. 75 (4), 342–349. Available at: https://www.ingentaconnect.com/content/asma/asem/2004/00000075/00000004/art00007.
Muzny, M., Henriksen, A., Giordanengo, A., Muzik, J., Grøttland, A., Blixgård, H., et al. (2020). Wearable sensors with possibilities for data exchange: analyzing status and needs of different actors in mobile health monitoring systems. Int. J. Med. Inf. 133, 104017. doi:10.1016/j.ijmedinf.2019.104017
Nahavandi, D., Alizadehsani, R., Khosravi, A., and Acharya, U. R. (2022). Application of artificial intelligence in wearable devices: opportunities and challenges. Comput. Methods Programs Biomed. 213, 106541. doi:10.1016/j.cmpb.2021.106541
Neuser, M. P., Teckentrup, V., Kühnel, A., Hallschmid, M., Walter, M., and Kroemer, N. B. (2020). Vagus nerve stimulation boosts the drive to work for rewards. Nat. Commun. 11 (1), 3555. doi:10.1038/s41467-020-17344-9
Neves, A. I. S., Bointon, T. H., Melo, L. V., Russo, S., de Schrijver, I., Craciun, M. F., et al. (2015). Transparent conductive graphene textile fibers. Sci. Rep. 5 (1), 9866. doi:10.1038/srep09866
Pan, W.-R., le Roux, C. M., Levy, S. M., and Briggs, C. A. (2011). Lymphatic drainage of the external ear. Head and Neck 33 (1), 60–64. doi:10.1002/hed.21395
Pandža, N. B., Phillips, I., Karuzis, V. P., O'Rourke, P., and Kuchinsky, S. E. (2020). Neurostimulation and pupillometry: new directions for learning and research in applied linguistics. Annu. Rev. Appl. Linguistics 40, 56–77. doi:10.1017/S0267190520000069
Panneton, W. M., and Gan, Q. (2020). The mammalian diving response: inroads to its neural control. Front. Neurosci. 14, 524. doi:10.3389/fnins.2020.00524
Park, J.-E., Kang, H. S., Baek, J., Park, T. H., Oh, S., Lee, H., et al. (2019). Rewritable, printable conducting liquid metal hydrogel. ACS Nano 13 (8), 9122–9130. doi:10.1021/acsnano.9b03405
Park, J.-H., Jang, D.-G., Park, J. W., and Youm, S.-K. (2015). Wearable sensing of in-ear pressure for heart rate monitoring with a piezoelectric sensor. Sensors 15 (9), 23402–23417. doi:10.3390/s150923402
Passler, S., Müller, N., and Senner, V. (2019). In-ear pulse rate measurement: a valid alternative to heart rate derived from electrocardiography? Sensors 19 (17), 3641. doi:10.3390/s19173641
Patterson, J. A. C., McIlwraith, D. C., and Yang, G. Z. (2009). “A flexible, low noise reflective PPG sensor platform for ear-worn heart rate monitoring,” in 2009 sixth international workshop on wearable and implantable body sensor networks, 286–291. doi:10.1109/BSN.2009.16
Perez-Pozuelo, I., Zhai, B., Palotti, J., Mall, R., Aupetit, M., Garcia-Gomez, J. M., et al. (2020). The future of sleep health: a data-driven revolution in sleep science and medicine. npj Digit. Med. 3 (1), 42. doi:10.1038/s41746-020-0244-4
Phillips, I., Calloway, R. C., Karuzis, V. P., Pandža, N. B., O'Rourke, P., and Kuchinsky, S. E. (2021). Transcutaneous auricular vagus nerve stimulation strengthens semantic representations of foreign language tone words during initial stages of learning. J. Cognitive Neurosci. 34 (1), 127–152. doi:10.1162/jocn_a_01783
Picano, J. J., Williams, T. J., and Roland, R. R. (2006). “Assessment and selection of high-risk operational personnel,” in Military psychology: clinical and operational applications, 353–370.
Poldy, C. A. (2001). “Headphones,” in Loudspeaker and headphone handbook. Editor J. Borwick 3rd ed. (Woburn MA: Focal Press), 585–692.
Polini, F., and Budai, R. (2022). Multimodal transcutaneous auricular vagus nerve stimulation: an option in the treatment of sleep bruxism in a “polyvagal” context. Cranio 42, 779–787. doi:10.1080/08869634.2022.2055866
Preuss, N., Hasler, G., and Mast, F. W. (2014). Caloric vestibular stimulation modulates affective control and mood. Brain Stimul. 7 (1), 133–140. doi:10.1016/j.brs.2013.09.003
Qian, P., Siahaan, E., Grinker, S. C., and LeBlanc, J. J. (2017). Earbuds with biometric sensing. Pat. US20170078780A1 Pat. Appl. US14856344, 2017-03-16. Available at: https://patents.google.com/patent/US20170078780A1/en.
Ratneswaran, D., Cheng, M., Nasser, E., Madula, R., Pengo, M., Hope, K., et al. (2023). Domiciliary transcutaneous electrical stimulation in patients with obstructive sleep apnoea and limited adherence to continuous positive airway pressure therapy: a single-centre, open-label, randomised, controlled phase III trial. eClinicalMedicine 62, 102112. doi:10.1016/j.eclinm.2023.102112
Röddiger, T., Clarke, C., Breitling, P., Schneegans, T., Zhao, H., Gellersen, H., et al. (2022). Sensing with earables: a systematic literature review and taxonomy of phenomena. Proc. ACM Interact. Mob. Wearable Ubiquitous Technol. 6 (3), 1–57. doi:10.1145/3550314
Ryan, N. M., Gibson, P. G., and Birring, S. S. (2014). Arnold's nerve cough reflex: evidence for chronic cough as a sensory vagal neuropathy. J. Thorac. Dis. 6 (Suppl. 7), S748–S752. doi:10.3978/j.issn.2072-1439.2014.04.22
Sabin, A. T., Van Tasell, D. J., Rabinowitz, B., and Dhar, S. (2020). Validation of a self-fitting method for over-the-counter hearing aids. Trends Hear. 24, 2331216519900589. doi:10.1177/2331216519900589
Salama, M., Akan, A., and Mueller, M. R. (2020). Transcutaneous stimulation of auricular branch of the vagus nerve attenuates the acute inflammatory response after lung lobectomy. World J. Surg. 44 (9), 3167–3174. doi:10.1007/s00268-020-05543-w
Sant'Anna, F. M., Resende, R. C. L., Sant’Anna, L. B., Couceiro, S. L. M., Pinto, R. B. S., Sant’Anna, M. B., et al. (1992). Auricular vagus nerve stimulation: a new option to treat inflammation in COVID-19? Rev. Assoc. Med. Bras. 69 (6), e20230345. doi:10.1590/1806-9282.20230345
Schaller, B. (2004). Trigeminocardiac reflex. A clinical phenomenon or a new physiological entity? J. Neurol. 251 (6), 658–665. doi:10.1007/s00415-004-0458-4
Seitz, T., Szeles, J. C., Kitzberger, R., Holbik, J., Grieb, A., Wolf, H., et al. (2022). Percutaneous auricular vagus nerve stimulation reduces inflammation in critical covid-19 patients. Front. Physiology, Orig. Res. 13, 897257. doi:10.3389/fphys.2022.897257
Sezer, N., and Koç, M. (2021). A comprehensive review on the state-of-the-art of piezoelectric energy harvesting. Nano Energy 80, 105567. doi:10.1016/j.nanoen.2020.105567
Sheng, T., Pasquesi, L., Gilligan, J., Chen, X.-J., and Swaminathan, J. (2024). Subjective benefits from wearing self-fitting over-the-counter hearing aids in the real world. Front. Neurosci. Orig. Res. 18, 1373729. doi:10.3389/fnins.2024.1373729
Sreenilayam, S. P., Ahad, I. U., Nicolosi, V., Acinas Garzon, V., and Brabazon, D. (2020). Advanced materials of printed wearables for physiological parameter monitoring. Mater. Today 32, 147–177. doi:10.1016/j.mattod.2019.08.005
Srinivasan, V., Abathsagayam, K., Suganthirababu, P., Alagesan, J., Vishnuram, S., and Vasanthi, R. K. (2023). Effect of vagus nerve stimulation (taVNS) on anxiety and sleep disturbances among elderly health care workers in the post COVID-19 pandemic, 78, 1149, 1156. doi:10.3233/wor-231362
Stavrakis, S., Stoner, J. A., Humphrey, M. B., Morris, L., Filiberti, A., Reynolds, J. C., et al. (2020). TREAT af (transcutaneous electrical vagus nerve stimulation to suppress atrial fibrillation): a randomized clinical trial. JACC Clin. Electrophysiol. 6 (3), 282–291. doi:10.1016/j.jacep.2019.11.008
Sun, J., Ma, Y., Du, Z., Wang, Z., Guo, C., Luo, Y., et al. (2022). Immediate modulation of transcutaneous auricular vagus nerve stimulation in patients with treatment-resistant depression: a resting-state functional magnetic resonance imaging study. Front. Psychiatry 13, 923783. doi:10.3389/fpsyt.2022.923783
Sunwoo, S.-H., Han, S. I., Joo, H., Cha, G. D., Kim, D., Choi, S. H., et al. (2020). Advances in soft bioelectronics for brain research and clinical neuroengineering. Matter 3 (6), 1923–1947. doi:10.1016/j.matt.2020.10.020
Tan, C., Qiao, M., Ma, Y., Luo, Y., Fang, J., and Yang, Y. (2023). The efficacy and safety of transcutaneous auricular vagus nerve stimulation in the treatment of depressive disorder: a systematic review and meta-analysis of randomized controlled trials. J. Affect. Disord. 337, 37–49. doi:10.1016/j.jad.2023.05.048
Tan, G., Adams, J., Donovan, K., Demarest, P., Willie, J. T., Brunner, P., et al. (2024). Does vibrotactile stimulation of the auricular vagus nerve enhance working memory? A behavioral and physiological investigation. Brain Stimul. Basic, Transl. Clin. Res. Neuromodulation 17 (2), 460–468. doi:10.1016/j.brs.2024.04.002
Tekdemir, I., Aslan, A., and Elhan, A. (1998). A clinico-anatomic study of the auricular branch of the vagus nerve and Arnold's ear-cough reflex. Surg. Radiol. Anat. 20 (4), 253–257. doi:10.1007/s00276-998-0253-5
Tyler, W. J. (2017). Multimodal neural interfaces for augmenting human cognition. Cham: Springer International Publishing, 389–407. in Augmented Cognition. Enhancing Cognition and Behavior in Complex Human Environments.
Tyler, W. J., Law, W., Jeffery, D., and Piersiak, R. (2022). Methods and apparatuses for transdermal stimulation of the outer ear. U. S. A. Pat. 11, 534–608.
Tyler, W. J., Wyckoff, S., Hearn, T., and Hool, N. (2019). The safety and efficacy of transdermal auricular vagal nerve stimulation earbud electrodes for modulating autonomic arousal, attention, sensory gating, and cortical brain plasticity in humans. bioRxiv, 732529. doi:10.1101/732529
Tyler Richard, S., Varghese, L., Furman Adam, C., Snell, K., Ji, H., and Rabinowitz William, M. (2024). An exploratory study of bimodal electro-aural stimulation through the ear canals for tinnitus. Am. J. Audiology, 1–10. doi:10.1044/2024_AJA-23-00144
Tynan, A., Brines, M., and Chavan, S. S. (2021). Control of inflammation using non-invasive neuromodulation: past, present and promise. Int. Immunol. 34 (2), 119–128. doi:10.1093/intimm/dxab073
Urbin, M. A., Lafe, C. W., Simpson, T. W., Wittenberg, G. F., Chandrasekaran, B., and Weber, D. J. (2021). Electrical stimulation of the external ear acutely activates noradrenergic mechanisms in humans. Brain Stimul. 14 (4), 990–1001. doi:10.1016/j.brs.2021.06.002
van Beekum, C. J., von Websky, M. W., Willis, M. A., Panknin, C., Coenen, M., Fimmers, R., et al. (2022). Transcutaneous vagal nerve simulation to reduce a systemic inflammatory response syndrome and the associated intestinal failure: study protocol of a prospective, two-armed, sham-controlled, double-blinded trial in healthy subjects (the NeuroSIRS-Study). Int. J. Colorectal Dis. 37 (1), 259–270. doi:10.1007/s00384-021-04034-1
Verma, N., Mudge, J. D., Kasole, M., Chen, R. C., Blanz, S. L., Trevathan, J. K., et al. (2021). Auricular vagus neuromodulation—a systematic review on quality of evidence and clinical effects. Front. Neurosci. Syst. Rev. 15, 664740. doi:10.3389/fnins.2021.664740
Wang, Y., Li, S. Y., Wang, D., Wu, M. Z., He, J. K., Zhang, J. L., et al. (2021). Transcutaneous auricular vagus nerve stimulation: from concept to application. Neurosci. Bull. 37 (6), 853–862. doi:10.1007/s12264-020-00619-y
Wang, Z., Shi, N., Zhang, Y., Zheng, N., Li, H., Jiao, Y., et al. (2023). Conformal in-ear bioelectronics for visual and auditory brain-computer interfaces. Nat. Commun. 14 (1), 4213. doi:10.1038/s41467-023-39814-6
Webster, D. B. (1966). Ear structure and function in modern mammals. Am. Zoologist 6 (3), 451–466. doi:10.1093/icb/6.3.451
Wilkinson, D., Podlewska, A., Banducci, S. E., Pellat-Higgins, T., Slade, M., Bodani, M., et al. (2019). Caloric vestibular stimulation for the management of motor and non-motor symptoms in Parkinson's disease. Park. Relat. Disord. 65, 261–266. doi:10.1016/j.parkreldis.2019.05.031
Wingate, R. C. (1999). Wireless headphones for entertainment and telephonic communication. Pat. US6006115A Pat. Appl. US08950833. Available at: https://patents.google.com/patent/US6006115A/en.
Wu, Z., Zhang, X., Cai, T., Li, Y., Guo, X., Zhao, X., et al. (2023). Transcutaneous auricular vagus nerve stimulation reduces cytokine production in sepsis: an open double-blind, sham-controlled, pilot study. Brain Stimul. 16 (2), 507–514. doi:10.1016/j.brs.2023.02.008
Wypych, A., Serafin, Z., Marzec, M., Osiński, S., Sielski, Ł., Kaźmierczak, H., et al. (2019). Grey matter activation by caloric stimulation in patients with unilateral peripheral vestibular hypofunction. Neuroradiology 61 (5), 585–593. doi:10.1007/s00234-019-02194-0
Xu, Y., De la Paz, E., Paul, A., Mahato, K., Sempionatto, J. R., Tostado, N., et al. (2023). In-ear integrated sensor array for the continuous monitoring of brain activity and of lactate in sweat. Nat. Biomed. Eng. 7 (10), 1307–1320. doi:10.1038/s41551-023-01095-1
Yang, C., and Suo, Z. (2018). Hydrogel ionotronics. Nat. Rev. Mater. 3 (6), 125–142. doi:10.1038/s41578-018-0018-7
Yuk, H., Lu, B., and Zhao, X. (2019). Hydrogel bioelectronics. Chem. Soc. Rev. 48 (6), 1642–1667. doi:10.1039/C8CS00595H
Yun, S., Kim, J., and Song, C. (2007). Performance of Electro-active paper actuators with thickness variation. Sensors Actuators A Phys. 133 (1), 225–230. doi:10.1016/j.sna.2006.03.007
Zeng, F. G., Rebscher, S., Harrison, W., Sun, X., and Feng, H. (2008). Cochlear implants: system design, integration, and evaluation, IEEE Rev. Biomed. Eng., 1, pp. 115–142. doi:10.1109/rbme.2008.2008250
Zhang, L., Jin, Y., Zhang, Q., Liu, H., Chen, C., Song, L., et al. (2023). Transcutaneous vagus nerve stimulation for insomnia in people living in places or cities with high altitudes: a randomized controlled trial. Brain Sci. 13 (7), 985. doi:10.3390/brainsci13070985
Zhang, S., Zhao, Y., Qin, Z., Han, Y., He, J., Zhao, B., et al. (2024). Transcutaneous auricular vagus nerve stimulation for chronic insomnia disorder: a randomized clinical trial. JAMA Netw. Open 7 (12), e2451217. doi:10.1001/jamanetworkopen.2024.51217
Zhao, B., Bai, Z., Lv, H., Yan, Z., Du, Y., Guo, X., et al. (2023a). Self-healing liquid metal magnetic hydrogels for smart feedback sensors and high-performance electromagnetic shielding. Nano-Micro Lett. 15 (1), 79. doi:10.1007/s40820-023-01043-3
Zhao, B., Bi, Y., Chen, Y., Zhang, J., Zhang, S., Zhang, D., et al. (2023b). Altered functional connectivity of the thalamus in patients with insomnia disorder after transcutaneous auricular vagus nerve stimulation therapy. Front. Neurology 14, 1164869. doi:10.3389/fneur.2023.1164869
Zhao, B., Bi, Y., Li, L., Zhang, J., Hong, Y., Zhang, L., et al. (2020). The instant spontaneous neuronal activity modulation of transcutaneous auricular vagus nerve stimulation on patients with primary insomnia. Front. Neurosci. 14, 205. doi:10.3389/fnins.2020.00205
Zhao, C., Park, J., Root, S. E., and Bao, Z. (2024). Skin-inspired soft bioelectronic materials, devices and systems. Nat. Rev. Bioeng. 2, 671–690. doi:10.1038/s44222-024-00194-1
Keywords: bioelectronics, flexible electronics, human interface, neuromodulation, sensors
Citation: Tyler WJ (2025) Auricular bioelectronic devices for health, medicine, and human-computer interfaces. Front. Electron. 6:1503425. doi: 10.3389/felec.2025.1503425
Received: 03 October 2024; Accepted: 17 January 2025;
Published: 06 February 2025.
Edited by:
Jhonathan Prieto Rojas, King Fahd University of Petroleum and Minerals, Saudi ArabiaReviewed by:
Mary Donahue, Linköping University, SwedenCopyright © 2025 Tyler. This is an open-access article distributed under the terms of the Creative Commons Attribution License (CC BY). The use, distribution or reproduction in other forums is permitted, provided the original author(s) and the copyright owner(s) are credited and that the original publication in this journal is cited, in accordance with accepted academic practice. No use, distribution or reproduction is permitted which does not comply with these terms.
*Correspondence: William J. Tyler, d2pwdEB1YWIuZWR1
Disclaimer: All claims expressed in this article are solely those of the authors and do not necessarily represent those of their affiliated organizations, or those of the publisher, the editors and the reviewers. Any product that may be evaluated in this article or claim that may be made by its manufacturer is not guaranteed or endorsed by the publisher.
Research integrity at Frontiers
Learn more about the work of our research integrity team to safeguard the quality of each article we publish.