- 1Centre for Applied Synthetic Biology, Concordia University, Montreal, QC, Canada
- 2Department of Chemical Engineering and Applied Chemistry, BioZone – Centre for Applied Bioscience and Bioengineering, University of Toronto, Toronto, ON, Canada
- 3Department of Health Research Methods, Evidence, and Impact (HEI), McMaster University, Hamilton, ON, Canada
- 4School of Biomedical Engineering, McMaster University, Hamilton, ON, Canada
- 5Max Planck Institute for Terrestrial Microbiology, Marburg, Germany
- 6Department of Biology, University of Waterloo, Waterloo, ON, Canada
- 7Ontario Institute for Studies in Education, University of Toronto, Toronto, ON, Canada
- 8Dufferin-Peel Catholic District School Board, Mississauga, ON, Canada
- 9Phyx44 Labs Pvt Ltd, Bengaluru, India
- 10Rapid Novor, Inc, Kitchener, ON, Canada
- 11Department of Biological Sciences, University of Calgary, Calgary, AB, Canada
- 12Stanford University, Stanford, CA, United States
- 13Department of Computer Science, University of British Columbia, Vancouver, BC, Canada
- 14Faculty of Education, University of Ottawa, Ottawa, ON, Canada
- 15Institute of Biomedical Engineering, University of Toronto, Toronto, ON, Canada
- 16Institute for Management & Innovation, University of Toronto, Toronto, ON, Canada
- 17Institute of Parasitology, McGill University, Sainte-Anne-de-Bellevue, QC, Canada
- 18Department of Applied Mathematics, University of Waterloo, Waterloo, ON, Canada
Synthetic biology is a growing field with an increasing number of successful applications. Yet, synthetic biology (SynBio) education initiatives are underreported and disconnected from each other. In this review we survey the literature on SynBio education and stratify this body of work into three categories: classroom activities, course designs, and program-level curricula-planning. For each category, we discuss the methods used to assess students’ experiences and achievement of learning objectives. Throughout, we identify trends and opportunities for further development in SynBio education. We determined that the design of low-cost education kits is a growing opportunity to support student learning at the level of classroom activities. In support of that work, we present a mapping of published education kits onto Bloom’s taxonomy, taking into account increasing accumulation of knowledge through continued experience. We further found that project-based learning is used widely and has proven effective in course designs. To facilitate such activities, we provide a high-level guide for the conversion of a didactic course into a project-based learning course. Further, we note that, currently, programs are delivered primarily at the graduate level, taking inspiration from traditional degree programs while incorporating interdisciplinary training. Finally, we find that design-based research may provide an effective framework for an iterative, mixed-method study design. To support such efforts, we provide a schematic overview of design-based research and its application to a learning progression for interdisciplinary skills. We conclude with a discussion of specific learning concepts that may be useful to SynBio educators and education researchers.
1 Introduction
Physics and chemistry matured into core scientific disciplines throughout the 18th and 19th centuries. Throughout that process, the knowledge passed from one generation to the next became increasingly sophisticated. Innovations in research and technology were driven by a flow of learners acquiring the knowledge and skills needed to address the problems of the times. Since the mid-20th century, biology has matured dramatically and has spurred the development of new biotechnologies beginning to address major problems of our age: e.g., fossil-fuel dependency, antibiotic-resistant pathogens, unsustainable supply chains, and the pollution of ecosystems. In particular, the adoption of engineering principles into biology has given rise to the promising fields of biomedical engineering and synthetic biology (SynBio). Biomedical engineering education is supported by a wealth of pedagogical studies going back 50 years that seek a better understanding of how to train engineers focused on human health (Peura et al., 1975; Potvin et al., 1981; Blanchard and Hale, 1995; Dee et al., 2002; Harris et al., 2002; Allen et al., 2013; Linsenmeier and Saterbak, 2020). In comparison, education in SynBio has received less attention in the pedagogical literature, despite its success across several frontiers of innovation.
Synthetic biologists rely on the rich history of molecular biology (and related fields) to engineer cells and their components (Agapakis, 2014; Cameron et al., 2014; Meng and Ellis, 2020). In 2014, Agapakis identified three main research streams in SynBio: the synthesis of microbial genomes, the production of commodity chemicals and pharmaceuticals by engineered microbes, and the rational design of genetic logic circuits from modular DNA parts (Agapakis, 2014). Commercial products like plant-based burgers from Impossible Foods Inc., the diabetes drug Januvia®, and the biological nitrogen fertilizer PROVEN® are clear demonstrations of SynBio’s utility (Voigt, 2020). These products are the result of inherently interdisciplinary activity. For example, the development of Januvia relied on computational protein design and directed evolution for the engineering of an enzyme that has enantioselective synthesis efficiency beyond what could be achieved by traditional organic synthesis methods (Savile et al., 2010). This new approach to thinking about biology presents challenges to pedagogical norms for training biology students and invites students in other disciplines to translate their seemingly unrelated knowledge (e.g., modelling, machine learning, process engineering, patent law, business) to a biological context. The process of translating biological research to commercial implementation is at the core of many modern SynBio endeavors, shaping SynBio to be inherently interdisciplinary.
Preparing learners for this challenging interdisciplinary environment is difficult. In an earlier study, we surveyed learners participating in Canadian SynBio design teams as part of the iGEM (international Genetically Engineered Machine) competition and identified gaps in the Canadian SynBio training pipeline, most notably the lack of collaborative and interdisciplinary training (Diep et al., 2021). Those findings parallel the conclusion of Agapakis (2014): “[…] collaborations offer engineers the opportunity to imagine new possibilities for how their work might be embedded into the human scale of everyday technology. Through design experiments and speculative prototyping, synthetic biologists can open up new directions for research, new questions, and new hypotheses, bridging the biological, the technological, and the social to communicate and question the potential benefits and risks of a new technology” (Agapakis, 2014). Learners in SynBio must not only master technical material (i.e., establish disciplinary grounding), but must also learn to identify problem areas in which their work would be most impactful and learn to effectively engage with experts grounded in other disciplines.
Interdisciplinary research and teaching is expanding across the globe, accompanied by a growing literature on the effectiveness of interdisciplinary teaching practices and program curricula (Jacob, 2015; You, 2017). Traditional engineering programs (e.g., chemical, mechanical, industrial) have recognized the demand to prepare their graduates to be productive members of interdisciplinary teams. This recognition has led to a growing number of studies and reviews describing effective pedagogical strategies (Jacob, 2015; Van den Beemt et al., 2020). Although SynBio educators can often translate these findings to the biological context, there is a paucity of literature specifically dedicated to education research and pedagogy for the interdisciplinary field of SynBio.
In this review, we survey and synthesize the current literature on SynBio education and stratify this body of work into three categories: (i) classroom activities, (ii) courses, and (iii) programs. This review is organized as follows. After a brief description of our methodology (Section 2), and a high-level description of summary statistics and the distribution of the body of literature we found (Section 3), we describe classroom activities and trends (Section 3.1), and their corresponding education research studies (Section 3.1.1). Next, we describe course designs and trends therein (Section 3.2), stratified by differing course lengths (Sections 3.2.1–3.2.4) and perspective pieces (Section 3.2.5 and 3.2.6), with a discussion of the corresponding education research studies (Section 3.2.7). Afterwards we describe program-level curriculum planning (Section 3.3), factors influencing programs (Section 3.3.1), and the corresponding education research studies (Section 3.3.2). We then proceed to describe learning concepts that may interface with SynBio education (Section 4), before concluding (Section 5).
2 Methods
To identify studies reporting on SynBio education we conducted a preliminary literature search through Google Scholar, PubMed and Web of Science using the search terms: synthetic biology education, synthetic biology pedagogy, synthetic biology curriculum development, and synthetic biology training program. This search was restricted to studies written in English, and published no later than July 2023. From this first set of publications, we then performed a vertical search on Web of Science by analyzing their bibliographies to identify additional relevant studies. We also performed a horizontal search with Web of Science search tools to find publications that had citation lists similar to the previously identified references. We included an additional three publications (Beason-Abmayr and Wilson, 2018; Johnson et al., 2022; Smith et al., 2022) suggested to us during the anonymous review process. Publications that did not report on at least one of the above categories were excluded, resulting in a finalized list of publications to be surveyed. We thus did not survey programs that were not reported on in a publication, systematic inclusion of which would have been challenging given the variability in publicly available information on education strategies.
3 Results and discussion
We surveyed 57 publications on the topic of SynBio education; summary statistics are shown in Figure 1. Most of these publications focused on the effectiveness of classroom activities for teaching specific SynBio concepts or on the effectiveness of course-specific curricula at helping students achieve learning objectives (Figure 1A, Classroom and Course, combined 68%). The majority (53%) were written by authors in the USA; the United Kingdom was the next most significant contributor (19%). This result may reflect the limitation of our search methodology to publications written in English, and that the USA and the UK have directed federal funding towards SynBio research over the past two decades (U.S. Trends in Synthetic Biology Research Funding, 2015; Kitney, 2021). With respect to student educational stage, most publications were focused on undergraduate students (60%), followed by high school students (20%) (Figure 1C). This distribution may reflect three factors: (1) that the main demographic of education research is high school and undergraduate students; (2) the difficulty of teaching SynBio before high school; and (3) that the increasing popularity of SynBio at the undergraduate and high school educational stages is driven by the international Genetically Engineered Machine (iGEM) competition’s primary student demographic (high school and undergraduate students). Finally, according to our survey, the publication record began in 2009 (with a single publication found in 2003), consistent with the fact that the first SynBio research projects were carried out in the early 2000s, laying a foundation for undergraduate and graduate training (Figure 1D). Additionally, the increased rate of publication over time, particularly through the 2010s, may correlate with the growth in iGEM participation during these years (Jainarayanan et al., 2021), as well as with the steady increase in investments made in SynBio-based companies driving demand for practitioners with the relevant expertise (U.S. Trends in Synthetic Biology Research Funding, 2015; Kuiken, 2022).
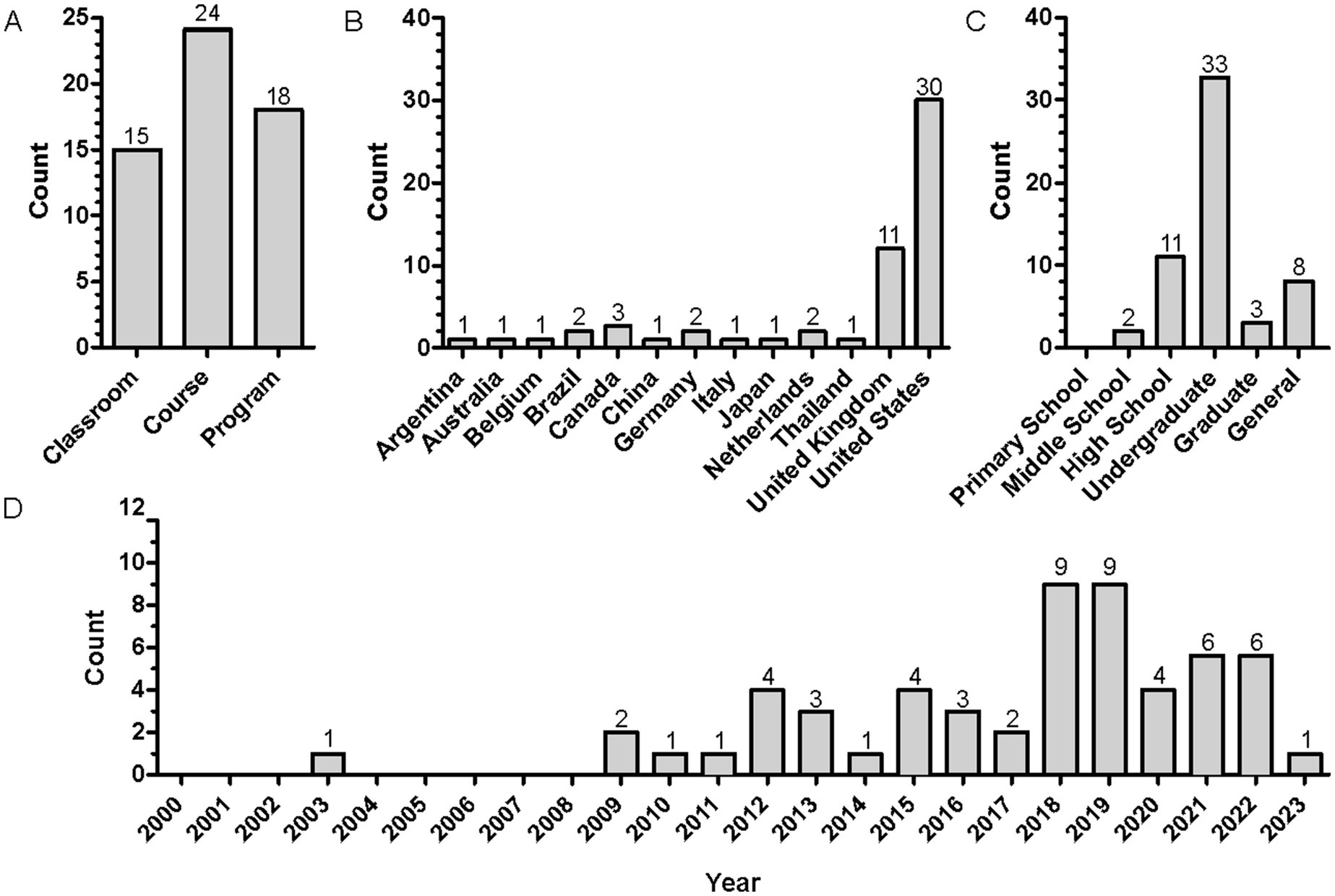
Figure 1. SynBio education papers reviewed. (A) Distribution by level: of classroom activities, courses, and programs. (B) National origin of publications. (C) Target student audience. (D) Distribution over time.
Below, we separately analyze the surveyed publications after stratifying them into three categories: (i) classroom activities (Section 3.1), (ii) courses (Section 3.2), (iii) programs (Section 3.3). Within each section, we first summarize the literature and compare different approaches educators have taken to achieve particular learning objectives (for classroom activities) or broader learning goals (for courses and programs). We then highlight the overall trends in each category and sketch the current landscape of SynBio education initiatives. To explore where the landscape may grow within each category, we discuss the publications that report research results and methodology (Table 1).
3.1 Classroom activities
We surveyed 15 publications in this category. These describe educational tools and interventions that could be deployed in a short timeframe (hours-to-days) to help students achieve modest learning objectives, such as understanding a specific concept or knowing how to perform a simplified molecular cloning protocol (Table 2). Most activities involved the use of affordable kits and devices that can bring molecular biology into the classroom without the need for expensive laboratory equipment. About half of these kits (7 of 15) provide cell-free, lyophilized (“just add water”) reagents for introductory experiments (Huang et al., 2018; Stark et al., 2018, 2019; Collias et al., 2019; Huang, 2019; Williams et al., 2020; Rybnicky et al., 2022). Here, “cell-free” refers to cell-free synthetic biology, through which researchers use ex vivo preparations (i.e., outside of the cellular context) to recapitulate biological reactions typically performed in cells. To implement cell-free biomolecular activities, researchers have developed mixtures containing cellular lysate (i.e., the “innards” of cells), and added specific key components such as ribosomes, DNA, and enzymatic substrates depending on what kind of biological reactions is intended. These kits enable the experimental study of a range of biological phenomena: from protein expression and biosensing to applications of the genome editing tool called CRISPR-Cas9. Several of the described activities can be completed in just a few hours, allowing students to visually analyze their results the following day. The activities have lower chances of error because the components are prepared ahead of time in the correct quantities. Consequently, educators can confidently integrate this type of activity into a biology unit with minimal cost and preparation.
The design of these kits (including room-temperature storage) allows them to be relatively inexpensive and easy-to-use. However, more advanced audiences, who might benefit from exposure to experimental failure modes and the creative troubleshooting required to correct issues (Collias et al., 2019), may find their scope limited. For example, the application of CRISPR for genome editing requires troubleshooting when the Cas enzyme and guide RNA are suboptimal (Xu and Li, 2020). Additionally, these kits may offer limited modularity. Compared to the flexibility provided by electronic or chemistry hobby sets, these SynBio kits provide fewer opportunities for exploring behaviour beyond the specific procedures described by the manufacturer; users may be able to experiment with varying input concentrations of reagents (Huang et al., 2018), but investigating different promoters, RBSs, or CRISPR systems would require a completely different set of materials.
Of course, these limitations are only relevant to more advanced audiences; most of these kits are designed for junior audiences, or those less acquainted with molecular biology. In contrast, senior undergraduate students would benefit more from laboratory exercises that simulate real-world practice. There appears to be significant untapped potential to use these cell-free systems in conjunction with other low-cost simplified components (e.g., gel electrophoresis set-ups, low-cost plate readers, Arduino-controlled elution columns) to increase the sophistication of the learning objectives associated with these kits (Gamale et al., 2021; Bergua et al., 2022; Diep et al., 2022; Thompson, 2022). Additionally, educators could incorporate digital media tutorials that have more advanced protocols and learning objectives to complement the prescribed kit activities. A repository of YouTube resources on SynBio concepts has been compiled (Dy et al., 2019) that could serve as inspiration for such tutorials, or be used as is for more advanced kits, such as those involving painting logos with pigment-transformed E. coli (Kafai et al., 2017), implementation of CRISPR-Cas12 for diagnostic sensing (Rybnicky et al., 2022), or tuning of genetic circuits in organisms (Magaraci et al., 2016).
3.1.1 Education research studies of classroom-based activities
Using pre-/post-test assessments, Campbell et al. found evidence that their pClone and rClone activity kits were able to significantly, and with large effect, improve students’ knowledge of the relevant material (Campbell et al., 2014; Campbell and Eckdahl, 2018). Also, through a pre-/post-test assessment and a control group, (Williams et al., 2020) found that their Genetic Code Kit benefited students’ learning about transcription and translation. They tested on an undergraduate audience, who may have been exposed to these concepts already. In contrast, the kit may provide a larger benefit to a secondary school audience, for whom transcription and translation might be unfamiliar. While quantitative methodologies such as pre-/post-tests can provide evidence for the effectiveness of an activity, they offer limited insight on how the students are learning, or why the activity is effective. Interviews and observational methodologies can reveal more detailed information (Cohen et al., 2017). For example, in other studies of SynBio classroom activities, students highlighted their sense of agency during project activities, and that activities contextualized their knowledge in real practice; these insights can help educators prioritize new activities that are effective for their students (Kafai et al., 2017; Betten et al., 2018; Walker and Kafai, 2021). For example, in Betten et al.’s (2018) scenario-based activity, interviews revealed that students found the writing process central to their learning.
Ideally, quantitative methods such as pre-/post-tests are paired with qualitative methods such as student interviews. Figure 2 provides a schematic for classifying cell-free expression kits for classroom activities, organized according to audience expectations and complexity of tasks. Ideally, such kits and equipment would be accompanied by “educational spec sheets,” supported by empirical evidence reported in open access publications. These spec sheets could detail the kit’s performance at helping students of different educational stages achieve specific learning objectives. In this manner, a Registry of SynBio Educational Kits could enable educators to easily find characterized SynBio education activities intended for specific audiences.
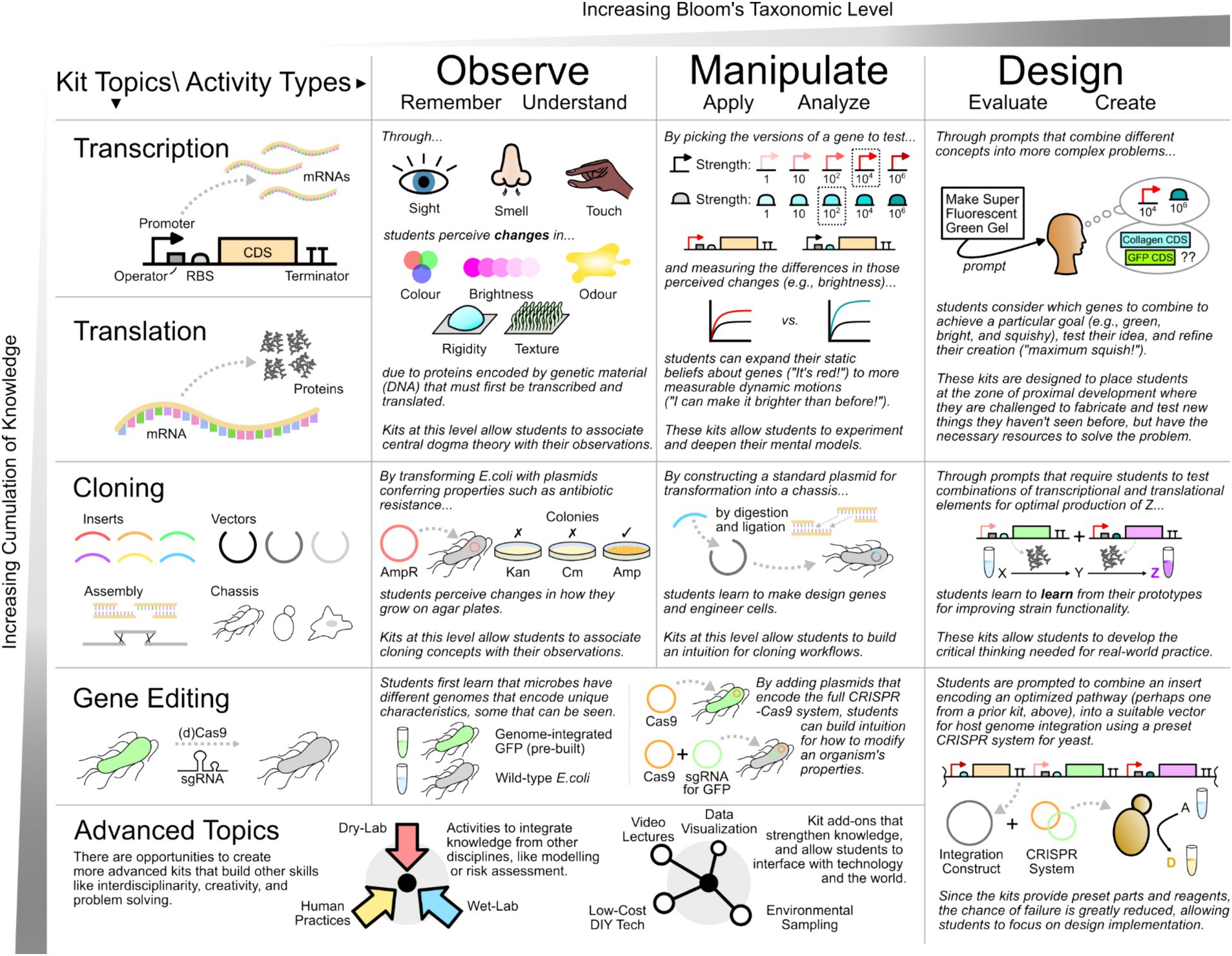
Figure 2. Overview of potential cell-free and cloning education (classroom activity) kits. Kit topics are listed in the far-left column in increasing cumulation of molecular biology knowledge. The activity types correspond to Bloom’s taxonomic learning levels: Observe, where students recall theoretical knowledge and gain a deeper understanding of that knowledge by perceiving changes in some property (i.e., colour of solution) or outcome (e.g., colonies on a plate); Manipulate, where students can make choices about how to change the property or outcome and measure the extent of these changes; and Design, where students learn to make judgements about genetic parts and how to put them together to achieve a more complex property or outcome, thus empowering them with the agency required to practice in the SynBio space. The literature used to synthesize this figure is described in Section 3.1.
3.2 Course design
The 24 publications we reviewed in this category address the delivery of courses that require multiple classroom activities and education exercises to achieve their learning objectives (Table 3). The overarching trend we identified was widespread use of project-based learning (PBL), frequently integrated with activities associated with the iGEM competition. In PBL students work in groups or individually towards an “end product” such as a project proposal or iGEM competition deliverables. PBL has been found to significantly improve students’ academic achievement (compared to teacher-led instruction), to foster participant motivation, to promote strong conceptual understanding, and to facilitate the development of collaboration and goal-setting skills (Kokotsaki et al., 2016; Chen and Yang, 2019; Virtue and Hinnant-Crawford, 2019). In Figure 3 we present a schematic overview of the process of converting a lecture-based course to a project-based learning course, including an overview of stakeholders that may offer useful perspectives for designing the projects. Our discussion of these publications, below, is organized by the length and nature of the courses: week-long, semester-long, year-long, and online. Additionally, we consider perspective pieces that comment both on how the iGEM competition can play an important role in shaping future practitioners in SynBio and on other dimensions of SynBio course design.
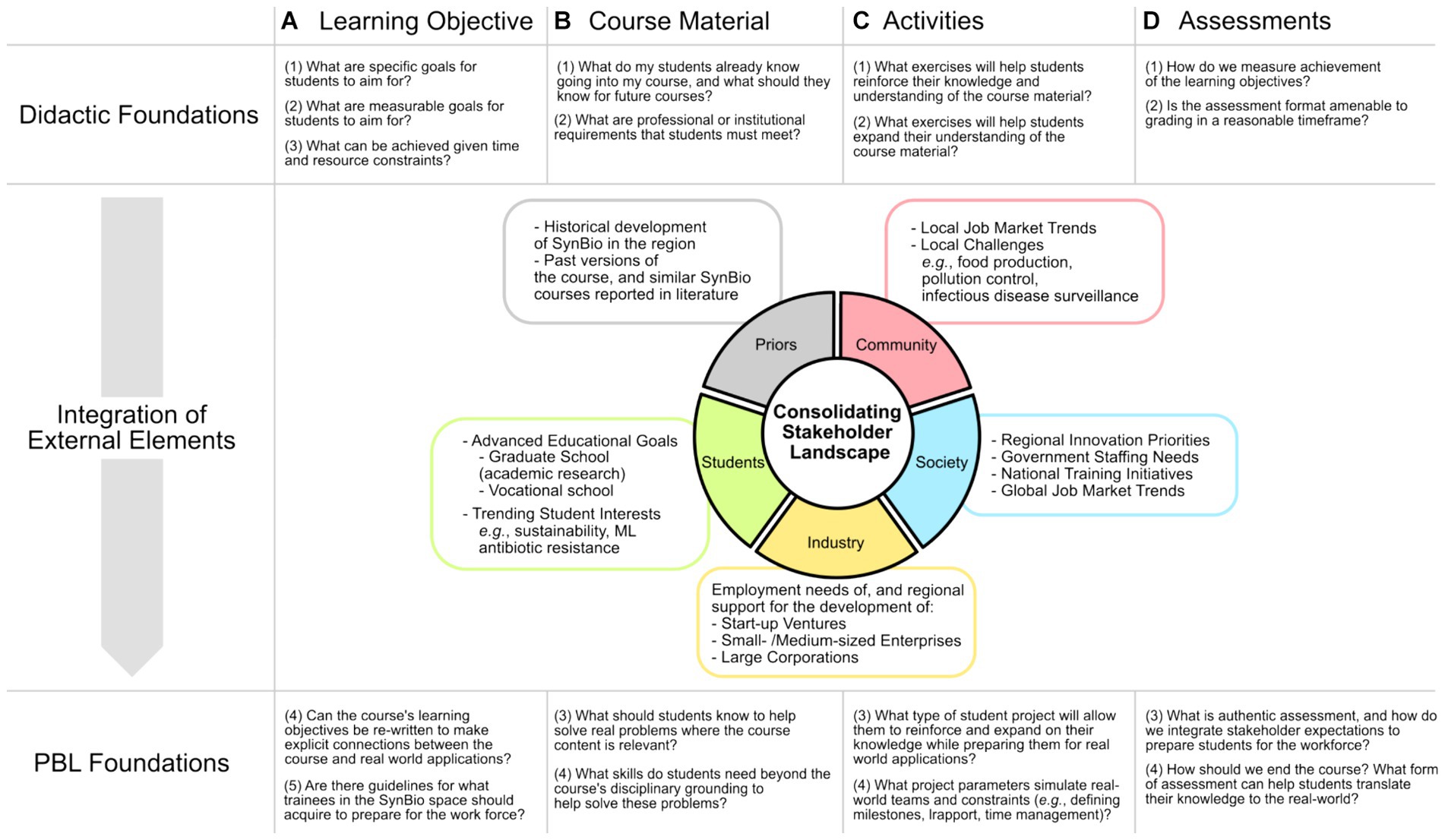
Figure 3. Converting a didactic course to a project-based learning course in the context of SynBio. A course is divided into four parts: Learning Objective, Course Material, Activities, and Assessments. Aspects that the instructor will consider for designing a lecture-focused course (top row) can be informed by engagement with relevant stakeholders (middle row), resulting in questions to be considered when creating a Project-based Learning (PBL) course.
3.2.1 Week-long courses
The week-long courses described in the surveyed publications were summer camps for secondary school students with lecture-style presentations and group projects with mentors. Hendricks et al. (2015) developed a five-day summer camp for high school students, with a focus on bioengineering for global health. They introduced the participants to SynBio, to molecular biology, and to point-of-care diagnostics devices. They invited them to identify a pressing need in global health and then challenged them to design and present a bioengineering solution. The authors found that the camp provided statistically significant improvements in participants’ knowledge of bioengineering. Subsoontorn et al. (2018) developed a five day “hackathon” for high school students that focused on biodigital, SynBio, and biomimicry topics. Each topic was supported by introductory lectures and activities, and ended with an open challenge. In small groups, participants proposed a biotechnology project, considered social implications, and discussed competing or traditional technologies. These works demonstrate how short project-based courses can provide valuable exposure to both the experimental and human practices dimensions of Synbio.
3.2.2 Semester-long courses
Cooper et al. (2012) reported on the Build-a-Genome course (Dymond, 2009) where students learned lab techniques such as PCR, cloning, and sequencing. The course also covered concepts central to SynBio, such as gene synthesis, recombinant DNA technologies, and genetic circuits. Situated learning (Cobb and Bowers, 1999) was deployed by treating the undergraduate students in this course as if they were graduate students or entry-level employees on an R&D team. The participants were provided initial training (eight guided sessions in a “molecular biology boot camp”), briefed on a course project (synthetic yeast genome), and then allowed to work relatively independently while providing updates at regular lab meetings. Students were also encouraged to develop their own “side-projects”; one such side-project was developed into a project for the first John Hopkins iGEM team.
At some institutions, iGEM participation has been integrated into for-credit courses. Such PBL courses were seen as early as 2008 with MIT’s Introduction to Biological Engineering. This PBL course, designed for first-year undergraduates, began with interactive lectures and moved into projects: students designed a biological solution to a problem of their choice (Kuldell and Mitchell, 2015). This approach has expanded to other schools. For example, Schmitt et al. (2021) developed an iGEM-based course focused on large student-led projects: with teams of nearly 20 members, students worked to establish sub-groups and write project proposals. Students also attended traditional lectures about pertinent topics in SynBio and xenobiology. For the remainder of the course, students were tasked with choosing their project, self-delegating experimental work and other tasks, preparing a presentation and online wiki page, then participating in the iGEM jamboree.
iGEM involvement, and large-scale research projects, are not the only ways to bring immersive SynBio education into a course. Smith et al. (2022) exemplify bringing interdisciplinary SynBio methods into a course in their PBL course. Students must both mathematically model, and experimentally construct a CRISPR-based toggle switch throughout the course, and provide a written report on their analyses and experiments. Johnson et al. (2022) report on a SynBio Course-based Undergraduate Research Experience (CURE) that effectively generates a SynBio learning environment through discussion of previous iGEM projects, and design and construction of a biosensor in small groups. Over 15 weeks, students work in groups to present on a number of previous iGEM team projects, familiarize themselves with the Registry of Standard Biological Parts, then work on biosensor projects. For their projects, teams must propose a design, build it using parts from the registry, characterize it, then deposit their work back into the registry for wider usage. This is similar to Beach and Alvarez’s (2015) lab course where students are expected to explore and present on previous iGEM team projects, become familiar with the Registry, design a biosensor, then build one (albeit a different one from their design). These two courses exemplify ways of creating SynBio educational environments with individual and smaller group projects, rather than an iGEM team or a large-scale research project.
3.2.3 Year-long courses
The year-long courses we identified in our survey closely followed the iGEM competition cycle. Steel et al. (2019) described how, through 80 lectures spread over a year, they scaffolded the learning and project lifecycle of a US Air Force Academy iGEM team into a course (in which enrollment was a requirement for participating in their iGEM team). Lee et al. (2020) described an analogous course focused on the engineering of an organism to produce paper-like sheets of nanocellulose. Compared to Steel, Bates and Barnhart’s course, offered to undergraduate-level air force cadets, the course described by Lee, Lux and DeCoste. was delivered to a cohort of professionals from materials science and biology, 82% of whom had more than 5 years of work experience, and 96% of whom had either a master’s or PhD degree. Gill et al. (2022) used the iGEM cycle as a template for their work-integrated learning program alternative, where student teams worked on industry or community-partner defined research projects. These three courses serve as exemplars for creating courses with long-term learning goals and for up-skilling individuals with deeper expertise in related, specialized areas.
3.2.4 Online courses
As highlighted by the COVID-19 pandemic, sometimes in-person project-based courses are not feasible or desirable. We surveyed several examples of online SynBio courses. Anderson et al. (2019) published their Massive Open Online Course (MOOC) “Principles of Synthetic Biology” on the platform edX. This course focused on modeling and simulations, genetic circuits, and a project requiring students to design and model a system (Anderson et al., 2019). Castro de Jesus and Cabral (2022) reported an online PBL course where students worked in groups to design a genetic circuit to address an instructor-provided SynBio challenge. These projects involved students performing literature reviews, justifying their proposed circuit designs and their choices of chassis organism, plasmids, promoters, and cloning strategies. Elsewhere, BioSin was designed to be a course-like organization (akin to a study group or support group) for introducing SynBio to a wider audience: during the first 4 weeks, the group covered introductory molecular biology through peer-to-peer lectures; this was followed by 5 weeks of SynBio-specific topics such as genetic circuits and bioinformatic tools, where small groups broke off to study a topic, then presented to the whole group; and ended with another 5 weeks of discussions and individual student-led topic presentations (Gervásio et al., 2022). These three courses demonstrate an avenue for effectively teaching SynBio online without laboratory access. Perry et al. (2022) demonstrate a project-based distance learning course where automated equipment, or a teaching assistant livestreaming themselves, perform experiments students design; the authors suggest a vision for expanding SynBio education access through the use of interconnected community hubs students may access remotely, akin to a cloud computing network. To assist with the development and design of online SynBio courses, Muth et al. (2021) published a toolbox of digital resources and reviewed their strengths and weaknesses, while Sheets et al. (2023) published a module covering the basics of SynBio. These sources demonstrate that there are many ways to teach (or learn) SynBio concepts before stepping into the lab. We note that synergies between cell-free and cloning kits (as discussed above in Section 3.1) and online courses have not been described in the educational research literature: a clear path for further development.
3.2.5 iGEM-specific perspective pieces
In addition to course designs inspired by the iGEM competition, we found four reports that discussed the pedagogical value of the iGEM experience itself, which can be translated to SynBio course design. Mitchell et al. (2011) performed an extensive survey of all iGEM participants in 2007 and 2008, restricting their analysis to undergraduates. They found that participation in the iGEM competition enabled students to develop lab-related skills and essential research skills such as identifying relevant questions, integrating new data with old data, performing critical literature searches, and communicating scientific ideas. Complementing these findings from a different perspective, Thamamongood et al. (2013) described the experiences of two iGEM teams: the Tokyo Tech and HKUST undergraduate teams. They reported that the competition helped participants develop skills in proposing and managing projects, interdisciplinary teamwork, and leadership. Paralleling these findings, Kelwick et al. (2015) described how the iGEM competition enabled students to develop research skills, communication skills, and project management skills. In addition to technical, research, and soft skills, the overall iGEM experience has been discussed in the context of incentivizing collaboration and human practices for improved product design (Balmer and Bulpin, 2013). These reports give a taste of the impact that iGEM has on new SynBio practitioners, helping them develop technical and interpersonal skills, as well as practical skills in engineering product design.
3.2.6 Other relevant perspective pieces
Beyond these iGEM-specific reports, we surveyed two perspective pieces that focused on interdisciplinarity and misconceptions around SynBio. Using a sequential mixed-methods study design, Song and Wang (2021) found three interrelated factors contributing to interdisciplinary competence at the middle school level: disciplinary grounding, attitudes towards interdisciplinary approaches, and opportunities for interdisciplinary learning. These findings suggest that course design may be enhanced by choosing to emphasize these factors. In complementary work, Verseux et al. (2016) studied how public misconceptions of SynBio may affect interdisciplinary collaboration during a summer school course. They noted that biologists, like many STEM experts, are rarely trained in communicating to non-experts, and that context is important to non-biologist’s reaction to a biotechnology. For example, the use of GMOs in agriculture was met with concerns, whereas GMOs used in manufacturing of medications or materials were seen much more positively.
3.2.7 Education research studies of SynBio courses
While the publications described in this section all report detailed course curricula, only some groups investigated the effectiveness of the course design in helping students achieve specified learning objectives. Subsoontorn et al. (2018) surveyed students participating in a week-long event called “Hack BioDesign.” The authors remarked that students were able to learn concepts well enough to “discuss with their teams and staff about the redesigning of novel organisms” (Subsoontorn et al., 2018). During Beach and Alvarez’s (2015) PBL lab course, students were challenged to develop a biosensor using parts from the iGEM Registry and by leveraging previous iGEM team’s projects (Beach and Alvarez, 2015); the authors used a pre-/post-survey to assess whether students were meeting learning objectives (Table 4) through their PBL course, and further supported their study methodology by using students’ weekly progress reports. They found their course significantly improved students’ skills relative to five learning objectives. Moreover, comparing to a control consisting of responses from students who had not participated in their course, but had equivalent backgrounds to their participants, they found evidence that their course fostered a greater appreciation for interdisciplinary contributions, enabled students to imagine more application areas for SynBio, and that a majority of students preferred the project-based format over a traditional lecture format. Highlighting the utility of collecting qualitative feedback, a noteworthy response from a participant unhappy with the project-based format, was that they “got lost when the lab went on for too long” (Beach and Alvarez, 2015); responses such as this provide the course designer with clear options for refinement: e.g. reduce the lab length, or scaffold the lab activity more. Kuldell and Mitchell (2015) also surveyed and interviewed the students in their course. They found that the course helped students better understand content in their other courses, helped them gain skills in reading scientific literature, and helped them work better in groups. Through interviews, they found that central to students’ learning were (i) having mentors providing feedback and occasional guidance, and (ii) the presence of a supportive environment in which mistakes and misconceptions could be addressed constructively (Kuldell and Mitchell, 2015). With extensive surveying and skills assessments, Gill et al. (2022) found evidence that their iGEM-inspired course improved students’ leadership and collaboration skills, among others.
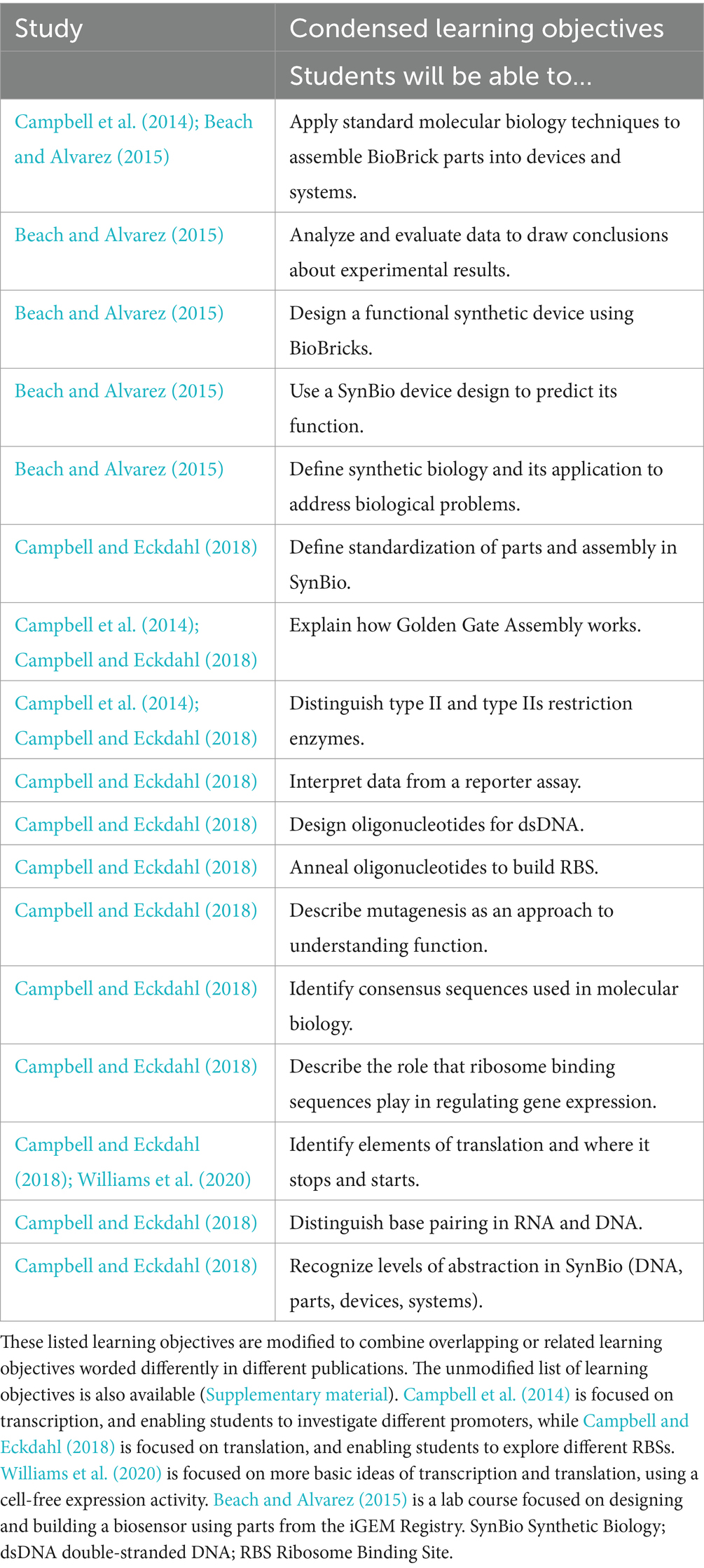
Table 4. Condensed learning objectives from those publications listed in Table 1.
Together, these publications demonstrate how quantitative and qualitative methods can be combined to produce a holistic picture of students’ learning experiences. SynBio education researchers can build on these foundations to investigate more dynamic factors such as the importance of assessing the improvement in effectiveness achieved through, e.g., clearer lecture slides, more realistic projects, or changes in assessment types. An important consideration for such studies is the Hawthorne effect, whereby students’ awareness that they are being assessed in a research context causes them to perform better, thereby undermining assessment of whether improvement in learning can be specifically attributed to the intervention being studied (Cook, 1962). An iterative approach, as presented in Figure 4, may be appropriate; by using the first iteration of the course as a baseline, subsequent improvements (or lack thereof), can better reflect the real effects of interventions (see Section 4.2 for additional discussion).
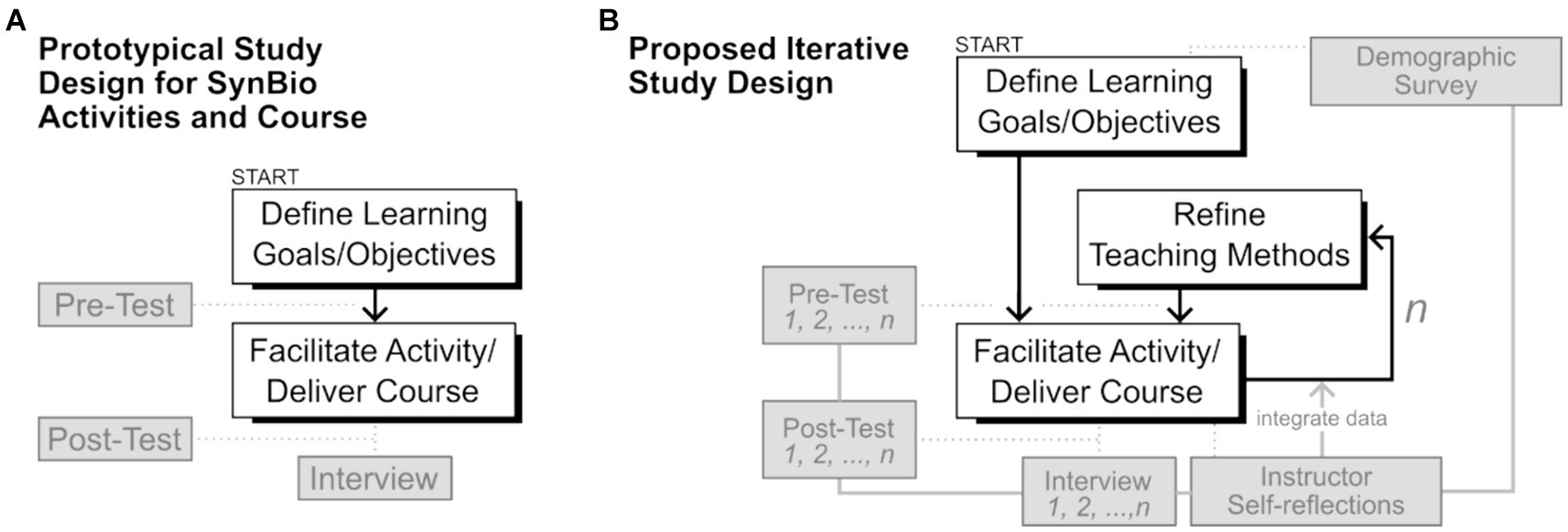
Figure 4. Study design for SynBio education research. (A) Prototypical study design to assess the effectiveness of SynBio activities and courses. A pre-/post-test (i.e., survey) is completed, after which interviews are performed. (B) Integration of an iterative “Refine Teaching Methods” step in which research data collected from one iteration informs the next, for a set number of cycles. Demographic survey data can also be collected prior to the study to assess the generality of the findings. The instructors’ self-reflections are systematically incorporated.
It is important to consider the perspective of instructors throughout and after a course. Indeed, a 2010 teaching workshop consisting of faculty members from 15 different undergraduate institutions (Wolyniak et al., 2010) reported preliminary evidence that instructors may not have the tools or resources to effectively teach SynBio project courses to undergraduates. In a complementary report, Frow and Calvert (2013) published an ethnography of their 6 years as supervisors of the iGEM team at the University of Edinburgh. They provide a nuanced discussion of the issues that arise when students negotiate time constraints and the conflicting objectives of engineering and biological sciences. For example, the standardization of parts in mechanical engineering allows complex systems to be produced with relatively predictable behavior; in contrast, genetic parts and cellular chassis present an element of unpredictability. They found that students often did not discuss standardization in their day-to-day operations, despite iGEM’s focus on standardization in submission of characterized BioBricks. Instead, students often focused on the science behind molecular cloning in attempts to finish constructs rather than the engineering of biological systems toward a specified function. Investigations of instructor experience and perspectives on SynBio education can reveal gaps in resources or challenges in integrating domain expertise. These can highlight opportunities for future work and provide a high-level perspective on the student’s experience.
Another avenue for exploration is how a course’s effectiveness depends on the demographics of the students. We did not find data on student demographics, prior education, or other identity factors in the SynBio education research space; these can be important considerations, especially for online courses intended to be accessible to a wider audience. Additionally, there are few published descriptions of PBL courses where the student audience comprises a “heterogeneous cohort,” meaning the students are enrolled in distinct programs and so might otherwise not interact with one other in an academic setting. Such environments pose unique challenges: the students must demonstrate interdisciplinary skills as well as mastering technical knowledge to complete assignments and projects together. These challenges closely reflect real-world SynBio work environments (Tripp and Shortlidge, 2019; Diep et al., 2021).
3.3 Program-level curriculum planning
We surveyed 18 publications in this category related to the design and delivery of SynBio education programs – multiple courses, offered over extended periods of time, for a broad range of students (Table 5). Hall and Howe (2012) described how SynBio interfaces with a traditional chemical engineering undergraduate program. By drawing inspiration from published descriptions of SynBio courses (RAE, 2009; Cooper et al., 2012; Kuldell and Mitchell, 2015), they described three approaches to incorporation of SynBio: (1) integration of concepts into existing courses, (2) mixed teaching through courses offered from different departments, and (3) dedicated courses that explicitly cover SynBio with a high degree of research-led activity. After noting the merits of each approach, and the potential limitations imposed by an institution’s circumstances, they highlight the advantages of an integrated-mixed approach—where students are exposed to SynBio concepts throughout their program’s courses, but in which students with a keen interest could enroll in a mixed program with deeper immersion through more advanced upper-year courses. In a complementary report, Crowe (2009) discussed the disciplinary differences between biomedical engineering, systems biology, and SynBio, using these differences to highlight how SynBio could enable biomedical engineers to operate at molecular scales to achieve desired physiological effect, in contrast to the cellular and tissue-level focus of many current practitioners in the field.
At the Master’s level, Hallinan et al. (2019) described barriers associated with interdisciplinary fields in SynBio and provided rationale for development of a 1-year Master’s program in SynBio at Newcastle University. They began by highlighting important tools and resources for working in the field, then described the program: through a series of multidisciplinary lectures, computational and experimental assignments, group projects, seminars, and involvement with iGEM, students gained the ability to work with existing technologies and were encouraged to work with, and develop, new technologies in the field. In a complementary report focusing on the doctoral level, Cazimoglu et al. (2019) described the Synthetic Biology Centre for Doctoral Training (SynBioCDT), a collaboration between the Universities of Oxford, Bristol, and Warwick. The program is focused on interdisciplinary training of doctoral students. In the first year, all students learn both experimental and computational skills. The authors found that, as students progressed through their programs, they were able to recognize interdisciplinary links because of their common first-year studies. Importantly, the authors describe integration of student feedback to improve the program (Cazimoglu et al., 2019). Dedicated programs like these can be complemented with additional means of meeting educational goals, such as the establishment of dedicated SynBio centers (as could be outlined in national strategies). For example, the Warwick Integrative Synthetic Biology Centre brings together researchers from different disciplines and incorporates an educational component to connect learners’ studies to the latest advances in research (McCarthy, 2016).
3.3.1 Factors influencing program-level curricula
Competing values, priorities, and community needs can influence the design of a curriculum. Farny (2018) argued the importance of open science, of having new perspectives to propel creativity in the field, and of having diverse teams to drive collaborative discovery and interdisciplinarity. On the other hand, expanding the biotechnology industry relies on commercialization and protecting intellectual property—both of which may run counter to the values of an open, accessible science and which require skills not generally covered by a traditional STEM program. Focusing on these skill gaps, Delebecque and Philp (2019) emphasized the importance of interdisciplinary training at various education stages. Additionally, Edwards and Kelle (2012) argued training should include the ability to assess risks posed by the dual-use nature of SynBio innovations. Expositions like these serve as launch points for institutional leaders to reflect on the values and priorities of a nation’s SynBio community, and how those values make their way into curricula.
National training strategies are developed to gather consensus, to summarize the community’s values, and to suggest how to build training pipelines or how to direct investment. As part of a European Commission programme, Gaisser et al. (2009) surveyed 176 stakeholders within the EU SynBio community, and generated a roadmap. The community priorities reflected in this roadmap were intended to inform discussions by policy-makers, funding agencies, and research organizations on how to strategically invest in, and regulate the development of, this emerging field. In this roadmap, the authors specifically argued for immediate funding to maintain European competitiveness. The United Kingdom (UK) Synthetic Biology Roadmap Coordination Group later provided a clear demonstration of how a national strategy could be drafted through stakeholder feedback. Their national SynBio roadmap in 2012 (UK Synthetic Biology Roadmap Coordination Group, 2012) emphasized the issue of training: “the next generation synthetic biology community needs to [be comprised of] researchers with (a) depth within core disciplines and the ability to work in cross-disciplinary collaboration; and (b) high-level, broad interdisciplinary synthetic biology expertise.” From this roadmap, several UK groups have published work describing the development and outcomes of SynBio programs at the undergraduate and graduate level (Hall and Howe, 2012; McCarthy, 2016; Cazimoglu et al., 2019; Hallinan et al., 2019). Specifically, Kitney (2021) provided a comprehensive review of outcomes from the 2012 UK roadmap, including establishment of research centres, biofoundries, and innovation funds, as well as policy changes. An important step in creating a national training strategy for SynBio, then, is to ground it in the values of the existing community.
Knowledge of the historical development of successful research communities can be used to foster the growth of new communities. Federici et al. (2013) provided a history of SynBio in detail, then delineated how advances in the field could improve Chile’s existing bioindustry. Addressing Latin America more broadly, Nadra et al. (2020) described the pivotal moments that defined that region’s SynBio community of practice, which could be used to ground national training strategies catering to the specific needs of students, academia, and industry. Such comprehensive surveys of regional SynBio stakeholders can build community consensus and identify priorities for national training pipelines.
We did not find other examples of literature that addressed national SynBio strategies supported by government agencies. This gap may highlight community disconnect. Based on the literature, national training strategies can help determine what are the most important values and skills to transmit to students, how to identify and build on the local SynBio community’s successes, and how to guide the development of programs at all post-secondary levels to produce a diverse workforce. National training strategies should be living expositions that change with new demographic and pedagogical data. The resulting training strategies can effectively meet the needs of a broad range of students, promoting a stronger workforce.
3.3.2 Education research studies for program-level curricula
While national training strategies may summarize the perspectives of numerous stakeholders in SynBio education, students are the ones experiencing that education first hand. We found several publications that report results of surveying students at the middle school and high school level. Dawson and Schibeci (2003) surveyed 905 students (15–16 year olds) in Australia to understand their attitudes towards biotechnology. They found that at this early age, these students had already formed opinions about which biotechnologies were more acceptable than others (i.e., bacterial/yeast engineering were mostly acceptable, while human embryo technologies were some of the least acceptable). More recently, Walker (2021) adapted the survey used by Dawson and Schibeci to evaluate American middle school (11–14 year old) students’ perceptions of biotechnology, and noticed similar trends in attitudes towards biotechnologies derived from bacterial/yeast cells versus human-derived cells. These works indicate that understanding the initial attitudes of students should influence how curricula can be designed to appeal to, and introduce biology to, younger students. After discussing high school iGEM teams, Dubé et al. (2017) present the results of surveying the Lethbridge High School iGEM team (24 respondents), finding that iGEM was able to engage students in science, support students learning of science and scientific experimentation, and support the learning goals of the broader Alberta science curriculum. By surveying and interviewing undergraduate students on Canadian iGEM teams, Diep et al. (2021) found the students reported needing additional support, resources, and training from their institutions to reach their SynBio goals. Treating the iGEM experience as a proxy for SynBio education, the findings of Dubé et al. (2017) suggest that teaching SynBio can be consistent with broader STEM curriculum requirements, while the findings of Diep et al. (2021) suggest that students can describe gaps that their current institutions could fill to provide a more successful SynBio education.
4 Bridging learning concepts with SynBio education research
In the previous sections we summarized and identified trends in the SynBio education research literature after stratifying relevant publications into (i) classroom activities, (ii) course design, and (iii) program-level curriculum planning. We considered publications that used quantitative, qualitative, and mixed methodologies to assess students’ experiences and their achievement of learning goals and objectives. In this section, we build on those discussions by gathering three important learning concepts that SynBio educators and education researchers may find applicable to their courses and programs (Figure 5) and highlight a few selected course-based publications.
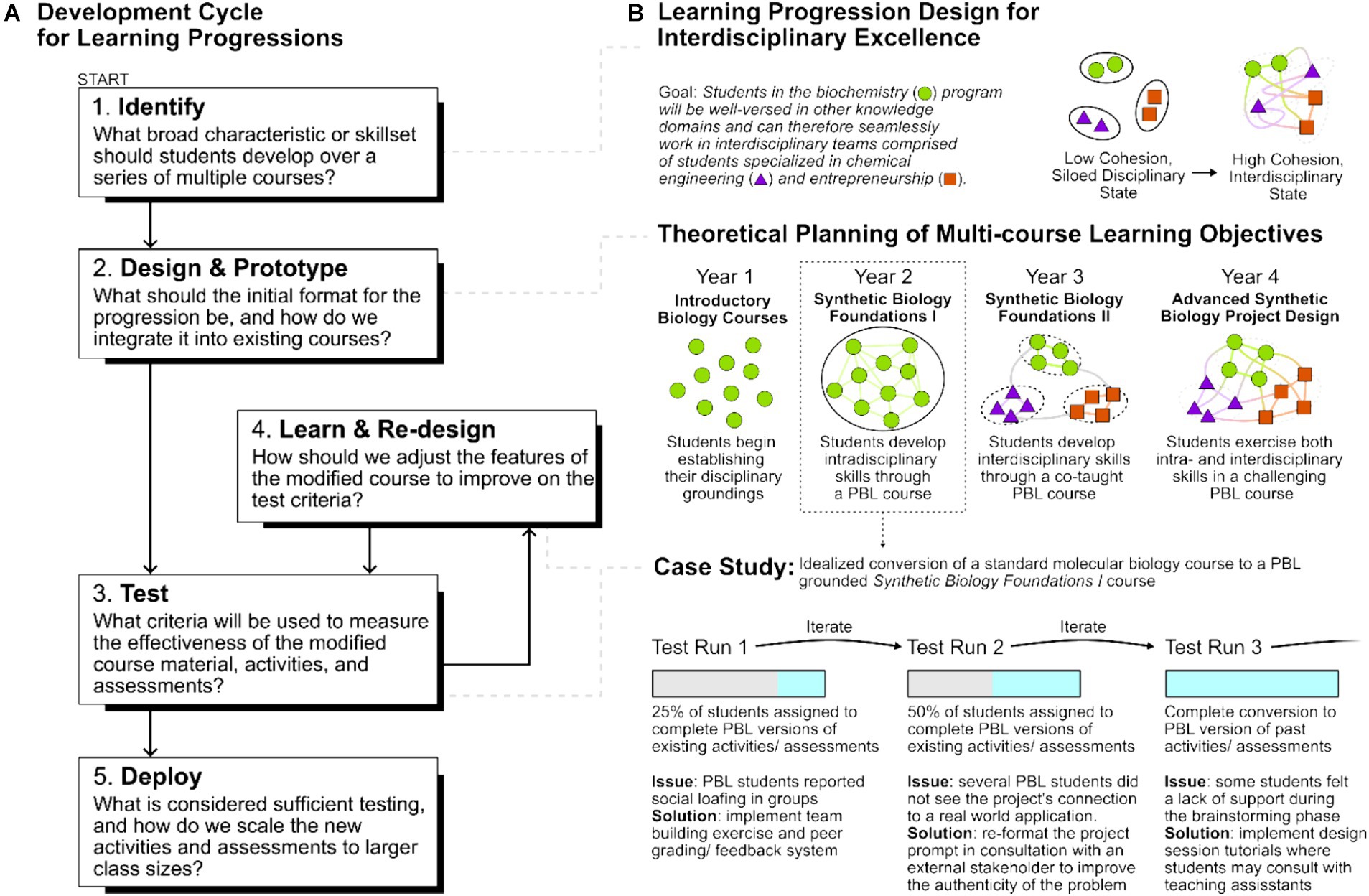
Figure 5. Learning progressions and design-based research. (A) Iterative design and implementation of learning progressions. Each of the five stages is characterized by an overarching question. Stages 3 and 4 are iterated until specific criteria for teaching effectiveness are met. (B) Implementation of a learning progression for interdisciplinary competence, with focus on biochemistry students (green circles). A four-year course series is proposed in which biochemistry students develop disciplinary grounding in Year 1, then build intradisciplinary skills (e.g., conflict management, time management, teamwork, communication) in group projects in Year 2. Throughout Year 3 and 4, these biochemistry students work with students from chemical engineering and business programs who have completed their own disciplinary Year 1 and 2 courses designed for entry into the SynBio space. Case study: Year 2’s “Synthetic Biology Foundations I” course that began as a standard molecular biology course for biochemistry students and was transformed through an iterative design-based research process.
4.1 Learning progressions
Learning progressions are structured course sequences that outline the expected stages of knowledge and skill development in specific subject areas (Scott et al., 2019). These progressions help educators and curriculum designers understand how students typically advance in their understanding and abilities over time (Figure 5A). Learning progressions provide a roadmap for crafting instructional strategies and assessments that are developmentally appropriate and aligned with learners’ needs and that successfully guide them toward more advanced concepts and competencies as they progress through their education. National training strategies and other expositions by stakeholders can highlight what skills students should develop and master in a SynBio program; these insights can align SynBio learning progressions with national priorities.
We can consider interdisciplinary collaboration as an example of a learning progression: in the previous section, we discussed how disciplinary grounding, attitudes toward interdisciplinarity, and opportunities for interdisciplinary collaboration are interrelated factors contributing to interdisciplinary competence (Figure 5B). A learning progression that leverages those factors and PBL could consist of a second-year project-based course offered to students in a particular degree or program (practicing intra-disciplinary collaboration and solidifying disciplinary grounding) followed first by a third-year project-based course taken by students with distinct backgrounds (such as the course described by MacLeod and van der Veen, (2020)), and then by a fourth-year capstone project with teams composed of students from very different backgrounds, in the flavour of an iGEM team (Figure 5).
As discussed in Diep et al. (2021), learning progressions would ideally be prototyped and tested using iterative mixed-method study designs (Figure 5). Prototyping a course element that contributes to an interdisciplinary aptitude learning progression may involve cross-course collaboration. As an example, consider a project shared across two courses, where students in each course learn different, but related, content, and collaborate on the project. Designing a sufficiently complex project that challenges the students from each course to develop an interdisciplinary response would involve an analysis of each course’s syllabus and rely on collaboration between the instructors. Testing could involve allowing students to opt into either the project shared across courses or a final assessment independent of the other course. Pre-/post-assessments and interviews could be conducted over multiple iterations to determine whether students are developing more interdisciplinary aptitude, how to modify the shared assessment(s), or whether there should be more or less shared content. Figure 5 provides a graphical overview of this process.
As described, cross-course collaboration offers a method to route students from different specializations to collaboration on a project relevant to each of their specializations. The work of MacLeod and van der Veen (2020) is one such example. Bringing second-year undergraduate students from applied mathematics, civil engineering, and industrial & engineering management together to collaborate on a project, MacLeod and van der Veen found that students find the course and project meaningful (albeit with differences across disciplines) and that students found working with partners from disparate disciplines one of the most valuable aspects of the course. Even before students begin collaborating, cross-campus collaboration between a course organizer and a writing centre can prepare students to be stronger communicators. Beason-Abmayr and Wilson (2018) describe a course where students learn to provide better journal presentations through a required writing centre workshop. The authors report that student presentations qualitatively improved and that average scores across the presentation rubric were higher than previous offerings that lacked a writing centre workshop. Examples like these suggest that reaching across the university may provide students with effective and meaningful learning experiences.
4.2 Design-based research
Design-based research (DBR) seeks to improve educational practices (e.g., classroom activities and courses) by integrating research, design, and implementation (Scott et al., 2020). It involves creating and refining instructional strategies, interventions, or educational technologies within real-world educational settings. DBR emphasizes collaboration between researchers and practitioners to address specific educational challenges and enhance learning outcomes. This iterative process mirrors the design-build-test-learn cycle familiar to SynBio practitioners (Figure 4B; Figure 5A). DBR has been used in biology education. For example, Zagallo et al. (2016) reported the three-year development of an activity for molecular and cellular biology majors to practice connecting scientific models with empirical data. By analyzing students’ written work and small-group audio recordings, Zagallo et al. determined strategies that students used to identify and interpret patterns in data. Such results can directly inform subsequent course design. In another report on DBR, Scott et al. (2020) reported the use of two course iterations to guide the development and understanding of their physiology course. From the first offering, the authors learned to broaden their assessments to other physiological systems to ensure students had context to generalize the principle of flux. They refined their learning progression with both written and interview data. These examples illustrate how DBR formalizes measurement of changes in student learning (e.g., through pre-/post-test assessment) and also determines (through qualitative methods) the mechanisms driving those changes leading to practical changes in the course. Indeed, Scott et al. noted “DBR invites the use of mixed methods to understand student learning.” Through DBR, SynBio educators can effectively and precisely improve the practical outcomes of classroom activities and course designs, all while better understanding the student’s experience.
4.3 Concept inventories
Concept inventories are an assessment tool used to measure student understanding of essential concepts within a subject domain. In physics education research, Concept Inventories were first introduced for measuring conceptual understanding of force, motion, and Newtonian physics (Hestenes et al., 1992). More recently, concept inventories were introduced to biology faculty and next steps were discussed, specifically the need to agree on introductory concepts (D’Avanzo, 2008). With evolutionary biology in mind, Furrow and Hsu (2019) surveyed concept inventories for evolution, and described how they may be used. In the same year, Cary et al. (2019) described four biology core concept instruments they developed, inspired by concept inventories. Their instruments are intended to be a method of measuring student understanding of the five core concepts outlined in the Vision and change report (American Association for the Advancement of Science, 2011). Of the publications we surveyed, most of the literature reported on the evaluation of a method or on education research by using a survey as an assessment tool; some performed observational reporting or interviews with their participants (Table 1). Of those that used a survey, only some performed pre- and post-test assessments to estimate the impacts of their activity, course, or program; fewer had follow-up interviews to investigate how or why a particular result was observed (Table 1). Concept inventories could provide a standardized measure of student understanding of core SynBio concepts, although this would require a consensus on what concepts are core to SynBio.
To our knowledge, there is no literature explicitly outlining the development, testing, and refining of concept inventories for SynBio. Here, we propose a 4-step guideline for the process of creating them, using molecular cloning (i.e., DNA assembly, or genetic engineering) at the undergraduate levels as a concrete example. (1) Anchoring. A high-level concept should be defined in a way that explains what the student should know and be capable of doing if they have excellent comprehension of it. This could be linked to learning progressions (Section 4.1). In our example, a student should understand the methods used to manipulate DNA to create genes, or networks of genes, that impart some biological reaction or function. (2) Decomposition. The high-level concept should then be broken down into separate ideas with enough simplification (or abstraction) that one approaches a reasonably reduced form. In this fashion, a fundamental grasp of the concept at its “first principles” can be achieved. In our example, DNA manipulation could be broadly understood as cutting, copying, and pasting of nucleic acids. “Cutting” DNA could involve restriction enzymes and CRISPR-Cas9; all of which could be further reduced to hydrolysis of the phosphodiester backbone of DNA, with specificity imparted through the nature of the enzyme. “Copying” DNA would involve PCR-amplification, which could be further reduced to understanding how double-stranded DNA come together through base-pair matching. “Pasting” DNA would again involve base-pair matching, as well as ligation of the phosphodiester backbone in a condensation reaction. The product of this reductionist approach is a knowledge tree where the starting high-level concept is sub-branched up to a threshold that educators (experts in the field) define. In our example, that threshold is organic chemistry, which we would consider sufficient since the next level of reduction (quantum chemistry and chemical physics) is not required for molecular cloning. (3) Inventorying. The sub-branches can then be converted into tangible learning goals by creating an inventory of assessment tools (i.e., questions, problems, scenarios, tasks) that challenge students’ understanding. For Cutting DNA, a series of knowledge questions about how restriction enzymes work (i.e., DNA recognition, binding, catalysis), and standardized problems (i.e., designing molecular cloning sites, predicting fragment sizes, matching overhangs to their complementary overhangs) could be developed. (4) Iterative Testing. Finally, the educator could perform DBR to understand and improve the effectiveness of their teaching. Other concept inventories include genetic circuits, flux balance analysis, biosensing, and more. Future discussion and research should be directed at defining appropriate concept inventories for synthetic biology where efforts can overlap with development of learning progressions by engaging with relevant stakeholders that employ synthetic biologists.
4.4 Starting points for planning a SynBio course
A number of publications we reviewed provide excellent starting points for designing and planning related SynBio courses. In their publication describing a SynBio device design lab course, Johnson et al. (2022) provide a comprehensive amount of material for planning a similar course. They included faculty training videos and interviews, clear learning objectives, a week-by-week description of what students should be doing, and, in their supplementary material, they included assignment examples, rubrics, and representative student assignment submissions. For an 8-week course balanced between modelling and building a CRISPRi toggle switch, Smith et al. (2022) provide a week-by-week overview of their course, and a GitLab repository1 containing assignments, course schedule, and python notebooks for running code. For a course devoted to modelling in SynBio, Principles of Synthetic Biology is archived on edX2 complete with a course syllabus, schedule, recorded lectures, and problem sets (Anderson et al., 2019).
5 Conclusions and outlook
In our survey of the SynBio education research literature, several noteworthy trends emerged. First, it was evident that cell-free SynBio kits are a popular area of study, with several paths to enhance their educational outcomes by incorporation of low-cost technologies and online courses. Second, in the realm of course-level education research for PBL iGEM-inspired courses, diverse methodologies have been employed. However, there remains room for improvement, particularly regarding the incorporation of iterative DBR, exploration of instructor perspectives, and the consideration of demographic factors. Third, the assessment of program effectiveness has primarily relied on student surveys. While such surveys provide valuable insights, there is an opportunity to broaden the scope of stakeholders (e.g., employment needs of start-ups, SMEs, and corporations; public sector needs of policy-makers) from whom input is solicited. Several valuable learning concepts have yet to be widely adopted by SynBio education researchers. These include the prototyping and testing of learning progressions, the incorporation of DBR principles into course design, and the standardization of concept inventories tailored to the field’s unique requirements. Ultimately, the SynBio community must engage in critical self-examination regarding the definition of SynBio itself, addressing what proficiencies are required of trainees in the field. Over time, the integration of education science methodologies into the field is anticipated to play a pivotal role in advancing the training and preparation of future SynBio practitioners.
Author contributions
JM: Conceptualization, Data curation, Formal analysis, Methodology, Project administration, Visualization, Writing – original draft, Writing – review & editing. PD: Data curation, Formal analysis, Methodology, Visualization, Writing – original draft, Writing – review & editing. FS: Formal analysis, Methodology, Visualization, Writing – original draft. AE: Formal analysis, Methodology, Writing – original draft. CD: Writing – review & editing. VS: Writing – review & editing. AA: Data curation, Formal analysis, Writing – original draft. EB: Data curation, Formal analysis, Writing – original draft. AB: Data curation, Formal analysis, Writing – original draft. SC: Data curation, Formal analysis, Writing – original draft. JD: Data curation, Formal analysis, Writing – original draft. CE: Data curation, Formal analysis, Writing – original draft. TL: Data curation, Formal analysis, Writing – original draft. IM: Data curation, Formal analysis, Writing – original draft. LM: Data curation, Formal analysis, Writing – original draft. DS: Data curation, Formal analysis, Writing – original draft. AS: Data curation, Formal analysis, Writing – original draft. SS: Formal analysis, Writing – original draft, Data curation. BI: Funding acquisition, Supervision, Writing – review & editing.
Funding
The author(s) declare that financial support was received for the research, authorship, and/or publication of this article. BI was supported by Natural Sciences and Engineering Research Council of Canada. JM was supported by NSERC CGS-D throughout the writing of this review.
Conflict of interest
SC was employed by Phyx44 Labs Pvt Ltd. JD was employed by Rapid Novor, Inc.
The remaining authors declare that the research was conducted in the absence of any commercial or financial relationships that could be construed as a potential conflict of interest.
The author(s) declared that they were an editorial board member of Frontiers, at the time of submission. This had no impact on the peer review process and the final decision.
Publisher’s note
All claims expressed in this article are solely those of the authors and do not necessarily represent those of their affiliated organizations, or those of the publisher, the editors and the reviewers. Any product that may be evaluated in this article, or claim that may be made by its manufacturer, is not guaranteed or endorsed by the publisher.
Supplementary material
The Supplementary material for this article can be found online at: https://www.frontiersin.org/articles/10.3389/feduc.2024.1441720/full#supplementary-material
Supplementary TABLe 1 | Additional publications suggested during anonymous review. Plus, un-condensed learning objectives for selected publications, and a short list of graduate programs in SynBio.
Supplementary TABLE 2 | List of publications found and reviewed, plus our early summaries of them. This file also includes the data used for Figure 1.
Footnotes
1. ^https://gitlab.com/wurssb/systems-and-synthetic-biology/
2. ^https://www.edx.org/learn/synthetic-biology/massachusetts-institute-of-technology-principles-of-synthetic-biology?irgwc=1
References
Adames, N. R., Gallegos, J. E., Hunt, S. Y., So, W. K., and Peccoud, J. (2019). Hands-on introduction to synthetic biology for security professionals. Trends Biotechnol. 37, 1143–1146. doi: 10.1016/j.tibtech.2019.06.005
Agapakis, C. M. (2014). Designing synthetic biology. ACS Synth. Biol. 3, 121–128. doi: 10.1021/sb4001068
Allen, R. H., Acharya, S., Jancuk, C., and Shoukas, A. A. (2013). Sharing best practices in teaching biomedical engineering design. Ann. Biomed. Eng. 41, 1869–1879. doi: 10.1007/s10439-013-0781-y
American Association for the Advancement of Science (2011). Vision and change in undergraduate biology education: A call to action. Washington, DC: American Association for the Advancement of Science.
Anderson, D. A., Jones, R. D., Arkin, A. P., and Weiss, R. (2019). Principles of synthetic biology: a MOOC for an emerging field. Synth. Biol. 4. doi: 10.1093/synbio/ysz010
Balmer, A. S., and Bulpin, K. J. (2013). Left to their own devices: post-ELSI, ethical equipment and the international genetically engineered machine (iGEM) competition. BioSocieties 8, 311–335. doi: 10.1057/biosoc.2013.13
Beach, D. L., and Alvarez, C. J. (2015). Biotechnology by design: an introductory level, project-based, synthetic biology laboratory program for undergraduate students. J. Microbiol. Biol. Educ. 16, 237–246. doi: 10.1128/jmbe.v16i2.971
Beason-Abmayr, B., and Wilson, J. S. (2018). ‘Building a partnership with a campus communication center’. J. Microbiol. Biol. Educ. 19. doi: 10.1128/jmbe.v19i1.1495
Bergua, J. F., Álvarez-Diduk, R., Idili, A., Parolo, C., Maymó, M., Hu, L., et al. (2022). Low-cost, user-friendly, all-integrated smartphone-based microplate reader for optical-based biological and chemical analyses. Anal. Chem. 94, 1271–1285. doi: 10.1021/acs.analchem.1c04491
Betten, A. W., Rerimassie, V., Broerse, J. E. W., Stemerding, D., and Kupper, F. (2018). Constructing future scenarios as a tool to foster responsible research and innovation among future synthetic biologists. Life Sci. Soc. Policy 14:21. doi: 10.1186/s40504-018-0082-1
Blanchard, S. M., and Hale, S. A. (1995) ‘Using the world wide web to teach biological engineering’, in proceedings Frontiers in education 1995 25th annual conference. Engineering education for the 21st century. Proceedings Frontiers in Education 1995 25th Annual Conference. Engineering Education for the 21st Century, vol. 2, 4c5.9-4c514. doi: 10.1109/FIE.1995.483226
Blount, B. A., and Ellis, T. (2018). The synthetic genome summer course. Synth. Biol. 3:ysy020. doi: 10.1093/synbio/ysy020
Cameron, D. E., Bashor, C. J., and Collins, J. J. (2014). A brief history of synthetic biology. Nat. Rev. Microbiol. 12, 381–390. doi: 10.1038/nrmicro3239
Campbell, A. M., and Eckdahl, T. T. (2018). rClone red facilitates bacterial gene expression research by undergraduates in the teaching laboratory. Synth. Biol. 3. doi: 10.1093/synbio/ysy013
Campbell, A. M., Eckdahl, T., Cronk, B., Andresen, C., Frederick, P., Huckuntod, S., et al. (2014). ‘pClone: synthetic biology tool makes promoter research accessible to beginning biology students’. CBE—Life Sci. Educ. 13, 285–296. doi: 10.1187/cbe.13-09-0189
Cary, T. L., Wienhold, C. J., and Branchaw, J. (2019). ‘A biology Core concept instrument (BCCI) to teach and assess student conceptual understanding’. CBE—Life Sci. Educ. 18:ar46. doi: 10.1187/cbe.18-09-0192
Castro de Jesus, L., and Cabral, L. M. (2022). Synthetic biology as a tool to online teaching undergraduate level molecular biology. Biochem. Mol. Biol. Educ. 50, 122–123. doi: 10.1002/bmb.21590
Cazimoglu, I., Darlington, A. P. S., Grigonyte, A., Hoskin, C. E. G., Liu, J., Oppenheimer, R., et al. (2019). Developing a graduate training program in synthetic biology: SynBioCDT. Synth. Biol. 4. doi: 10.1093/synbio/ysz006
Chen, C.-H., and Yang, Y.-C. (2019). Revisiting the effects of project-based learning on students’ academic achievement: a meta-analysis investigating moderators. Educ. Res. Rev. 26, 71–81. doi: 10.1016/j.edurev.2018.11.001
Cobb, P., and Bowers, J. (1999). Cognitive and situated learning perspectives in theory and practice. Educ. Res. 28, 4–15. doi: 10.3102/0013189X028002004
Cohen, L., Manion, L., and Morrison, K. (2017). Research methods in education. 8th Edn. London: Routledge.
Collias, D., Marshall, R., Collins, S. P., Beisel, C. L., and Noireaux, V. (2019). An educational module to explore CRISPR technologies with a cell-free transcription-translation system. Synth. Biol. 4. doi: 10.1093/synbio/ysz005
Cooper, E. M., Müller, H., Chandrasegaran, S., Bader, J. S., and Boeke, J. D. (2012). The build-a-genome course. Methods Mol. Biol. 852, 273–283. doi: 10.1007/978-1-61779-564-0_20
Crowe, J. A. (2009). “The role of biomedical engineers in systems / synthetic biology” in 4th European Conference of the International Federation for Medical and Biological Engineering. eds. J. V. Sloten, P. Verdonck, M. Nyssen, and J. Haueisen (Berlin, Heidelberg: Springer Berlin Heidelberg (IFMBE Proceedings)), 2714–2717.
D’Avanzo, C. (2008). Biology concept inventories: overview, status, and next steps. Bioscience 58, 1079–1085. doi: 10.1641/B581111
Dawson, V., and Schibeci, R. (2003). Western Australian high school students’ attitudes towards biotechnology processes. J. Biol. Educ. 38, 7–12. doi: 10.1080/00219266.2003.9655889
Dee, K. C., Nauman, E. A., Livesay, G. A., and Rice, J. (2002). Research report: learning styles of biomedical engineering students. Ann. Biomed. Eng. 30, 1100–1106. doi: 10.1114/1.1512677
Delebecque, C. J., and Philp, J. (2019). Education and training for industrial biotechnology and engineering biology. Eng. Biol. 3, 6–11. doi: 10.1049/enb.2018.0001
Diep, P., Boucinha, A., Kell, B., Yeung, B. A., Chen, X., Tsyplenkov, D., et al. (2021). Advancing undergraduate synthetic biology education: insights from a Canadian iGEM student perspective. Can. J. Microbiol. 67, 749–770. doi: 10.1139/cjm-2020-0549
Diep, P., Cadavid, J. L., Yakunin, A. F., McGuigan, A. P., and Mahadevan, R. (2022). REVOLVER: a low-cost automated protein purifier based on parallel preparative gravity column workflows. HardwareX 11:e00291. doi: 10.1016/j.ohx.2022.e00291
Dubé, S., Orr, D., Dempsey, B., and Wieden, H. J. (2017). A synthetic biology approach to integrative high school STEM training. Nat. Biotechnol. 35, 591–595. doi: 10.1038/nbt.3896
Dy, A. J., Aurand, E. R., and Friedman, D. C. (2019). YouTube resources for synthetic biology education. Synth. Biol. 4. doi: 10.1093/synbio/ysz022
Dymond, J. S. (2009). Teaching synthetic biology, bioinformatics and engineering to undergraduates: the interdisciplinary build-a-genome course. Genetics. 181, 13–21. doi: 10.1534/genetics.108.096784
Edwards, B., and Kelle, A. (2012). A life scientist, an engineer and a social scientist walk into a lab: challenges of dual-use engagement and education in synthetic biology. Med. Confl. Surviv. 28, 5–18. doi: 10.1080/13623699.2012.658659
Farny, N. G. (2018). A vision for teaching the values of synthetic biology. Trends Biotechnol. 36, 1097–1100. doi: 10.1016/j.tibtech.2018.07.019
Federici, F., Rudge, T. J., Pollak, B., Haseloff, J., and Gutiérrez, R. A. (2013). Synthetic biology: opportunities for Chilean bioindustry and education. Biol. Res. 46, 383–393. doi: 10.4067/S0716-97602013000400010
Frow, E., and Calvert, J. (2013). “Can simple biological systems be built from standardized interchangeable parts?” Negotiating biology and engineering in a synthetic biology competition. Eng. Stud. 5, 42–58. doi: 10.1080/19378629.2013.764881
Furrow, R. E., and Hsu, J. L. (2019). Concept inventories as a resource for teaching evolution. Evol.: Educ. Outreach 12:2. doi: 10.1186/s12052-018-0092-8
Gaisser, S., Reiss, T., Lunkes, A., Müller, K. M., and Bernauer, H. (2009). Making the most of synthetic biology. EMBO Rep. 10, S5–S8. doi: 10.1038/embor.2009.118
Gamale, J. N. L., Acut, D. P., Niere, K. M. F. P., Silagan, G. S. S., Curaraton, E. P., Latonio, G. C., et al. (2021). Development of a do-it-yourself (D.I.Y.) gel electrophoresis apparatus for Grade-12 STEM general biology students. J. Phys. Conf. Ser. 1835:012033. doi: 10.1088/1742-6596/1835/1/012033
Gervásio, J., Sampaio, Y., Muniz, P., Yamada, C., Liza, F., Kafai, F., et al. (2022). BioSin UFMG Club: engaging a multilevel and multidisciplinary community in synthetic biology. Biochem. Mol. Biol. Educ. 50, 249–253. doi: 10.1002/bmb.21609
Gill, H., Ahsan, M., Khalil, Y., Feng, V., Pearce, J., Sharma, T., et al. (2022). The BioExperience research and entrepreneurship challenge: an iGEM-inspired applied research program for BIOSTEM talent and skills development. Front. Bioeng. Biotechnol. 10. doi: 10.3389/fbioe.2022.1046723
Hall, G. M., and Howe, J. (2012). The impact of synthetic biology in chemical engineering—educational issues. Educ. Chem. Eng. 7, e51–e55. doi: 10.1016/j.ece.2012.02.002
Hallinan, J. S., Wipat, A., Kitney, R., Woods, S., Taylor, K., and Goñi-Moreno, A. (2019). Future-proofing synthetic biology: educating the next generation. Eng. Biol. 3, 25–31. doi: 10.1049/enb.2019.0001
Harris, T. R., Bransford, J. D., and Brophy, S. P. (2002). Roles for learning sciences and learning technologies in biomedical engineering education: a review of recent advances. Annu. Rev. Biomed. Eng. 4, 29–48. doi: 10.1146/annurev.bioeng.4.091701.125502
Hendricks, D., Pick, L., and Taylor, A. (2015) ‘Bioengineering global health: design and implementation of a summer day camp for high school students’, in 2015 ASEE Annual Conference and Exposition Proceedings. 2015 ASEE Annual Conference and Exposition, Seattle, Washington: ASEE Conferences, 26.283.1–26.283.19. doi: 10.18260/p.23622
Hestenes, D., Wells, M., and Swackhamer, G. (1992). Force concept inventory. Phys. Teach. 30, 141–158. doi: 10.1119/1.2343497
Huang, A. (2019) Accessible and easy-to-use educational tools to teach molecular and synthetic biology using freeze-dried, cell-free technology. Thesis. Massachusetts Institute of Technology. Available at: https://dspace.mit.edu/handle/1721.1/123213 (Accessed November 2, 2021).
Huang, A., Nguyen, P. Q., Stark, J. C., Takahashi, M. K., Donghia, N., Ferrante, T., et al. (2018). BioBits™ explorer: a modular synthetic biology education kit. Sci. Adv. 4:eaat5105. doi: 10.1126/sciadv.aat5105
Jacob, W. J. (2015). Interdisciplinary trends in higher education. Palgrave Commun. 1:15001. doi: 10.1057/palcomms.2015.1
Jainarayanan, A. K., Galanis, A., Sreejith, A., Suresh, S., Nakara, A. M., Kundlatsch, G. E., et al. (2021). iGEM comes of age: trends in its research output. Nat. Biotechnol. 39, 1599–1601. doi: 10.1038/s41587-021-01152-7
Johnson, K. C., Sabel, J. L., Cole, J., Pruett, C. L., Plymale, R., and Reyna, N. S. (2022). From genetics to biotechnology: synthetic biology as a flexible course-embedded research experience. Biochem. Mol. Biol. Educ. 50, 580–591. doi: 10.1002/bmb.21662
Kafai, Y., Telhan, O., Hogan, K., Lui, D., Anderson, E., Walker, J. T., et al. (2017). ‘Growing designs with biomakerlab in high school classrooms’, in Proceedings of the 2017 Conference on Interaction Design and Children. New York, NY, USA: Association for Computing Machinery (IDC ‘17), 503–508. doi: 10.1145/3078072.3084316
Kelwick, R., Bowater, L., Yeoman, K. H., and Bowater, R. P. (2015). Promoting microbiology education through the iGEM synthetic biology competition. FEMS Microbiol. Lett. 362. doi: 10.1093/femsle/fnv129
Kitney, R. I. (2021). Building the UK’s industrial base in engineering biology. Eng. Biol. 5, 98–106. doi: 10.1049/enb2.12016
Kokotsaki, D., Menzies, V., and Wiggins, A. (2016). Project-based learning: a review of the literature. Improv. Sch. 19, 267–277. doi: 10.1177/1365480216659733
Kuiken, T. (2022) Synthetic/engineering biology: Issues for congress. Congressional Research Service.
Kuldell, N., and Mitchell, R. (2015) ‘MIT’s introduction to biological engineering: a longitudinal study of a freshman inquiry-based class’, in Inquiry-Based Learning for Science, Technology, Engineering, and Math (Stem) Programs: A Conceptual and Practical Resource for Educators. Emerald Group Publishing Limited (Innovations in Higher Education Teaching and Learning), 4, doi: 10.1108/S2055-364120150000004006
Lee, M. S., Lux, M. W., and DeCoste, J. B. (2020). BEAMS: a workforce development program to bridge the gap between biologists and material scientists. Synth. Biol. 5:ysaa009. doi: 10.1093/synbio/ysaa009
Linsenmeier, R. A., and Saterbak, A. (2020). Fifty years of biomedical engineering undergraduate education. Ann. Biomed. Eng. 48, 1590–1615. doi: 10.1007/s10439-020-02494-0
MacLeod, M., and van der Veen, J. T. (2020). Scaffolding interdisciplinary project-based learning: a case study. Eur. J. Eng. Educ. 45, 363–377. doi: 10.1080/03043797.2019.1646210
Magaraci, M. S., Bermudez, J. G., Yogish, D., Pak, D. H., Mollov, V., Tycko, J., et al. (2016). Toolbox for exploring modular gene regulation in synthetic biology training. ACS Synth. Biol. 5, 781–785. doi: 10.1021/acssynbio.6b00057
McCarthy, J. (2016). WISB: Warwick integrative synthetic biology centre. Biochem. Soc. Trans. 44, 678–680. doi: 10.1042/BST20160011
Meng, F., and Ellis, T. (2020). The second decade of synthetic biology: 2010–2020. Nat. Commun. 11:5174. doi: 10.1038/s41467-020-19092-2
Mitchell, R., Dori, Y. J., and Kuldell, N. H. (2011). Experiential engineering through iGEM—an undergraduate summer competition in synthetic biology. J. Sci. Educ. Technol. 20, 156–160. doi: 10.1007/s10956-010-9242-7
Muth, L. T., Jenkins Sánchez, L. R., Claus, S., Salvador Lopez, J. M., and van Bogaert, I. (2021). A toolbox for digitally enhanced teaching in synthetic biology. FEMS Microbiol. Lett. 368. doi: 10.1093/femsle/fnab115
Nadra, A. D., Rodríguez, P. E., Grunberg, R., Olalde, L. G., and Sánchez, I. E. (2020). Developing synthetic biology in Argentina: the Latin American TECNOx community as an alternative way for growth of the field. Crit. Rev. Biotechnol. 40, 357–364. doi: 10.1080/07388551.2020.1712322
Perry, E., Weber, J., Pataranutaporn, P., Volf, V., Gonzalez, L. M., Nejad, S., et al. (2022). How to grow (almost) anything: a hybrid distance learning model for global laboratory-based synthetic biology education. Nat. Biotechnol. 40, 1874–1879. doi: 10.1038/s41587-022-01601-x
Peura, R. A., Boyd, J. R., Shahnarian, A., Driscoll, W. G., and Wheeler, H. B. (1975). Organization and function of a hospital biomedical engineering internship program. IEEE Trans. Biomed. Eng. BME-22, 134–140. doi: 10.1109/TBME.1975.324433
Porter, S. E. G., Barber, A. E., Colella, O. K., and Roach, T. D. (2018). Using biological organisms as chemical sensors: the MicRoboCop project. J. Chem. Educ. 95, 1392–1397. doi: 10.1021/acs.jchemed.8b00008
Potvin, A. R., Long, F. M., Webster, J. G., and Jendrucko, R. J. (1981). Biomedical engineering education: enrollment, courses, degrees, and employment. IEEE Trans. Biomed. Eng. BME-28, 22–28. doi: 10.1109/TBME.1981.324841
RAE (2009). Synthetic biology: Scope, applications and implications. London, UK: The Royal Academy of Engineering, 64.
Rybnicky, G. A., Dixon, R. A., Kuhn, R. M., Karim, A. S., and Jewett, M. C. (2022). Development of a freeze-dried CRISPR-Cas12 sensor for detecting Wolbachia in the secondary science classroom. ACS Synth. Biol. 11, 835–842. doi: 10.1021/acssynbio.1c00503
Savile, C. K., Janey, J. M., Mundorff, E. C., Moore, J. C., Tam, S., Jarvis, W. R., et al. (2010). Biocatalytic asymmetric synthesis of chiral amines from ketones applied to Sitagliptin manufacture. Science 329, 305–309. doi: 10.1126/science.1188934
Schmitt, F.-J., Frielingsdorf, S., Friedrich, T., and Budisa, N. (2021). Courses based on iGEM/BIOMOD competitions are the ideal format for research-based learning of Xenobiology. Chembiochem 22, 818–825. doi: 10.1002/cbic.202000614
Scott, E. E., Wenderoth, M. P., and Doherty, J. H. (2019). ‘Learning progressions: an empirically grounded, learner-centered framework to guide biology instruction’. CBE—Life Sci. Educ. 18:es5. doi: 10.1187/cbe.19-03-0059
Scott, E. E., Wenderoth, M. P., and Doherty, J. H. (2020). ‘Design-based research: a methodology to extend and enrich biology education research’. CBE—Life Sci. Educ. 19:es11. doi: 10.1187/cbe.19-11-0245
Sheets, M. B., Atkinson, J. T., Styczynski, M. P., and Aurand, E. R.EBRC Education & Engagement Working Group (2023). Introduction to engineering biology: a conceptual framework for teaching synthetic biology. ACS Synth. Biol. 12, 1574–1578. doi: 10.1021/acssynbio.3c00194
Smith, R. W., Garcia-Morales, L., Martins dos Santos, V. A. P., and Saccenti, E. (2022). Research-driven education: an introductory course to systems and synthetic biology. Front. Syst. Biol. 2. doi: 10.3389/fsysb.2022.981800
Song, G., and Wang, Z. (2021). Factors influencing middle school students’ interdisciplinary competence in science education. J. Res. Sci. Teach. 58, 1041–1072. doi: 10.1002/tea.21692
Stark, J. C., Huang, A., Hsu, K. J., Dubner, R. S., Forbrook, J., Marshalla, S., et al. (2019). BioBits health: classroom activities exploring engineering, biology, and human health with fluorescent readouts. ACS Synth. Biol. 8, 1001–1009. doi: 10.1021/acssynbio.8b00381
Stark, J. C., Huang, A., Nguyen, P. Q., Dubner, R. S., Hsu, K. J., Ferrante, T. C., et al. (2018). BioBits™ bright: a fluorescent synthetic biology education kit. Sci. Adv. 4:eaat5107. doi: 10.1126/sciadv.aat5107
Steel, J. J., Bates, K. L., and Barnhart, M. D. (2019). Investing in our nation’s future military leaders’ synthetic biology knowledge to understand and recognize threats and applications. Synth. Biol. 4. doi: 10.1093/synbio/ysz024
Subsoontorn, P., Ounjai, P., Ngarmkajornwiwat, P., Sakulkueakulsuk, B., Pensupha, N., Surareungchai, W., et al. (2018). ‘Hack biodesign: an integrative STEAM education platform for biology, engineering, and design’, in 2018 IEEE International Conference on Teaching, Assessment, and Learning for Engineering (TALE). 2018 IEEE International Conference on Teaching, Assessment, and Learning for Engineering (TALE), 1016–1021. doi: 10.1109/TALE.2018.8615404
Thamamongood, T., Lim, N. Z. L., Ho, T. Y. H., Ayukawa, S., Kiga, D., and Chow, K. L. (2013). Cultivation of synthetic biology with the iGEM competition. J. Adv. Comput. Intell. Intell. Inform. 17, 161–166. doi: 10.20965/jaciii.2013.p0161
Thompson, J. E. (2022). Low-cost microplate reader with 3D printed parts for under 500 USD. Sensors 22. doi: 10.3390/s22093242
Tripp, B., and Shortlidge, E. E. (2019). A framework to guide undergraduate education in interdisciplinary science. CBE—Life Sci. Educ. 18:es3. doi: 10.1187/cbe.18-11-0226
U.S. Trends in Synthetic Biology Research Funding (2015). The Synthetic Biology Project, with the Wilson Centre.
UK Synthetic Biology Roadmap Coordination Group. (2012). ‘A synthetic biology roadmap for the UK’. Technology Strategy Board. Available at: https://www.ifm.eng.cam.ac.uk/uploads/Roadmapping/Synthetic_Biology_Roadmap_-_TSB.pdf (Accessed August 9, 2022).
Van den Beemt, A., MacLeod, M., Van der Veen, J., Van de Ven, A., van Baalen, S., Klaassen, R., et al. (2020). Interdisciplinary engineering education: a review of vision, teaching, and support. J. Eng. Educ. 109, 508–555. doi: 10.1002/jee.20347
Verseux, C., Acevedo-Rocha, C. G., Chizzolini, F., and Rothschild, L. J. (2016). Misconceptions of synthetic biology: lessons from an interdisciplinary summer school. NanoEthics 10, 327–336. doi: 10.1007/s11569-016-0264-3
Virtue, E. E., and Hinnant-Crawford, B. N. (2019). “We’re doing things that are meaningful”: student perspectives of project-based learning across the disciplines. Interdiscip. J. Probl.-Based Learn. 13. doi: 10.7771/1541-5015.1809
Voigt, C. A. (2020). Synthetic biology 2020–2030: six commercially-available products that are changing our world. Nat. Commun. 11:6379. doi: 10.1038/s41467-020-20122-2
Walker, J. T. (2021). Middle school student knowledge of and attitudes toward synthetic biology. J. Sci. Educ. Technol. 30, 791–802. doi: 10.1007/s10956-021-09919-y
Walker, J. T., and Kafai, Y. B. (2021). The biodesign studio: constructions and reflections of high school youth on making with living media. Br. J. Educ. Technol. 52, 1116–1129. doi: 10.1111/bjet.13081
Williams, L. C., Gregorio, N. E., So, B., Kao, W. Y., Kiste, A. L., Patel, P. A., et al. (2020). The genetic code kit: an open-source cell-free platform for biochemical and biotechnology education. Front. Bioeng. Biotechnol. 8:941. doi: 10.3389/fbioe.2020.00941
Wolyniak, M. J., Alvarez, C. J., Chandrasekaran, V., Grana, T. M., Holgado, A., Jones, C. J., et al. (2010). Building better scientists through cross-disciplinary collaboration in synthetic biology: a report from the genome consortium for active teaching workshop 2010. CBE—Life Sci. Educ. 9, 399–404. doi: 10.1187/cbe.10-07-0097
Xu, Y., and Li, Z. (2020). CRISPR-Cas systems: overview, innovations and applications in human disease research and gene therapy. Comput. Struct. Biotechnol. J. 18, 2401–2415. doi: 10.1016/j.csbj.2020.08.031
You, H. S. (2017). Why teach science with an interdisciplinary approach: history, trends, and conceptual frameworks. J. Educ. Learn. 6:66. doi: 10.5539/jel.v6n4p66
Keywords: synthetic biology, education, pedagogy, curriculum, education kits, project-based learning, design-based research
Citation: Menard J, Diep P, Sheikh F, Escobar A, Dykstra CB, Sajtovich VA, Ahmadi A, Bodyreva E, Boucinha A, Chandrasekharan S, Duan J, Emond C, Lu T, McLean I, Morse L, Serra D, Stancescu A, Suresh S and Ingalls BP (2024) Synthetic biology education and pedagogy: a review of evolving practices in a growing discipline. Front. Educ. 9:1441720. doi: 10.3389/feduc.2024.1441720
Edited by:
Natalie Gilks Farny, Worcester Polytechnic Institute, United StatesReviewed by:
Joshua T. Atkinson, Princeton University, United StatesIngmar Riedel-Kruse, University of Arizona, Molecular and Cellular Biology (and courtesy BME, Applied Math, Physics), United States
Copyright © 2024 Menard, Diep, Sheikh, Escobar, Dykstra, Sajtovich, Ahmadi, Bodyreva, Boucinha, Chandrasekharan, Duan, Emond, Lu, McLean, Morse, Serra, Stancescu, Suresh and Ingalls. This is an open-access article distributed under the terms of the Creative Commons Attribution License (CC BY). The use, distribution or reproduction in other forums is permitted, provided the original author(s) and the copyright owner(s) are credited and that the original publication in this journal is cited, in accordance with accepted academic practice. No use, distribution or reproduction is permitted which does not comply with these terms.
*Correspondence: Jyler Menard, anlsZXIubWVuYXJkQG1haWwuY29uY29yZGlhLmNh; Patrick Diep, cGF0cmlja19kaWVwQG91dGxvb2suY29t; Brian P. Ingalls, YmluZ2FsbHNAdXdhdGVybG9vLmNh