- 1Interdisciplinary Arts and Sciences, University of Washington Bothell, Bothell, WA, United States
- 2Research Collaboratory for Structural Bioinformatics Protein Data Bank, Rutgers, The State University of New Jersey, New Brunswick, NJ, United States
- 3Institute for Quantitative Biomedicine, Rutgers University, New Brunswick, NJ, United States
- 4Cancer Institute of New Jersey, New Brunswick, NJ, United States
Molecular case studies (MCSs) provide educational opportunities to explore biomolecular structure and function using data from public bioinformatics resources. The conceptual basis for the design of MCSs has yet to be fully discussed in the literature, so we present molecular storytelling as a conceptual framework for teaching with case studies. Whether the case study aims to understand the biology of a specific disease and design its treatments or track the evolution of a biosynthetic pathway, vast amounts of structural and functional data, freely available in public bioinformatics resources, can facilitate rich explorations in atomic detail. To help biology and chemistry educators use these resources for instruction, a community of scholars collaborated to create the Molecular CaseNet. This community uses storytelling to explore biomolecular structure and function while teaching biology and chemistry. In this article, we define the structure of an MCS and present an example. Then, we articulate the evolution of a conceptual framework for developing and using MCSs. Finally, we related our framework to the development of technological, pedagogical, and content knowledge (TPCK) for educators in the Molecular CaseNet. The report conceptualizes an interdisciplinary framework for teaching about the molecular world and informs lesson design and education research.
1 Introduction
Structure–function relationships transcend science, technology, engineering, and mathematics (STEM) disciplines and are prioritized as a unifying concept (Yoho et al., 2018). A structure–function lens in biology and chemistry education provides valuable insights into the living world, ranging from organisms to molecules (American Association for the Advancement of Science, 2011; Holme et al., 2015). Whether experimentally determined or computationally modeled, biomolecular structures combine knowledge of physics, chemistry, biology, engineering, and computer science. With access to vast amounts of publicly available data, tools, and resources, we consider how to teach about biomolecular structure and function in biology, chemistry, and interdisciplinary contexts. Since narratives and storytelling are powerful tools in science communication (Dahlstrom, 2014), we use these to engage students in exploring biomolecular structures and examining interactions that facilitate their specific functions.
Fact-based stories about biological molecules engage a broad range of audiences, including students, educators, and the general public, connect them to scientific discovery, and inspire them to learn about the living world (Goodsell et al., 2021). Selected examples of resources that present molecular stories include Molecule of the Month (Goodsell et al., 2015), Proteopedia (Prilusky et al., 2011), and Online Macromolecular Museum (Marcey, 2011). Videos incorporating molecular animations are also sought-after for communicating molecular stories (Barbra and Stark, 2014; Werner, 2022). Recently, undergraduate educators collaborated to develop and use Molecular Case Studies (MCSs) for teaching (Dutta and Dries, 2019). The MCSs are designed to actively engage students in molecular storytelling, an investigative process of biomolecular visualization and bioinformatics exploration, to answer a specific question in the form of molecular stories. Herein, we present the structure and an example of an MCS. We then describe the evolution of a conceptual framework for MCSs. We relate the narrative structure of MCSs to a well-known storytelling framework and present operational definitions of molecular stories and storytelling. Finally, we position the framework for developing and using MCSs at the intersection of technological, pedagogical, and content knowledge (TPCK) to inform their use in interdisciplinary teaching and learning.
2 Motivation and purpose
2.1 Why should we care about molecular structures?
The double helix model of deoxyribonucleic acid (DNA) marked a turning point in our understanding of the world. It provided a structural foundation for understanding DNA’s function as the genetic material for living organisms (Watson and Crick, 1953). In the past seventy years, our knowledge of biomolecular structures has not only helped us understand the molecular basis of life but has also aided the design of molecules with novel properties and interactions to address various challenges in the world. Biomolecular structures have helped us develop specific treatments for many diseases - e.g., sickle cell disease (Oksenberg et al., 2016), COVID-19 (Owen et al., 2021), and cancer (e.g., Mol et al., 2004; Chen et al., 2016). Comparing structures of related biomolecular structures provides a window to witness evolution. For example, the shapes and properties of some viral proteins provide insights into their origins (Mughal et al., 2020); caffeine-producing enzymes derived from different plants can be similar in shape yet catalyze different chemical reactions to produce the same molecule (Huang et al., 2016).
Today, researchers routinely use biomolecular structures to understand the molecular basis of life. Vast amounts of information about biomolecules (e.g., genes, proteins, and their interactions) is freely available from public data resources. For example, GenBank (Benson et al., 2013) provides access to ~250 million gene sequences, UniProt (UniProt Consortium, 2023) has ~250 million amino acid sequences of proteins, and Protein Data Bank (PDB, Berman et al., 2013) provides access to 215 thousand experimentally determined structures of biological macromolecules (such as proteins, nucleic acids, carbohydrates, and a variety of small molecules) including their three dimensional (3D) shapes and interactions with each other. Over a million computed structure models are available for visualization and analysis alongside the experimentally determined structures from RCSB.org (Burley et al., 2023), and another ~200 million are available from Alpha Fold (Callaway, 2022). Various tools are available for exploring data from these resources, while information aggregation platforms allow researchers to integrate relevant details from different data sources to synthesize new knowledge. Millions of users, including researchers and disciplinary experts, use these data regularly. Educators can bring these same tools and platforms to engage students in the process of science and to help them appreciate a molecular view of the world.
2.2 Motivation for teaching about molecular structures
Scientific discourse in most STEM fields, including biology and chemistry, requires that students develop ‘visual literacy,’ a mastery of perceiving, interpreting, and comprehending diverse canonical representations (Schönborn and Anderson, 2006, 2009; Rybarczyk, 2011; LaDue et al., 2015). Teaching and learning about molecular structures are demanding - in addition to being ripe with confusing, counter-intuitive, and complex behavior (Alberts, 1998; Tibell and Rundgren, 2010), the molecules are too small to be directly visible. To be successful, learners must develop a corpus of discipline-specific vocabulary, representations, concepts, and skills to engage with, interpret, and process information about molecular structures from the scientific literature and other data resources (Offerdahl et al., 2017). However, there are many reasons why biomolecular structures are included in teaching and learning biology, chemistry, and related disciplines - we mention a few here.
• Structure and function have been identified as a core concept in undergraduate curricula for over a decade and have informed competency-focused education (American Association for the Advancement of Science, 2011; Yoho et al., 2018).
• Exploring molecular structures provides ample opportunities for collaboration and interdisciplinary learning (e.g., Kohn et al., 2018).
• Vast collections of biomolecular structures (Berman et al., 2003; Callaway, 2022) and tools for exploring them (Olson, 2018) are freely available for teaching and learning. Note: Educators need professional development to keep current with the rapidly growing data resources and continuously evolving visualization and analysis tools.
• Careers in many STEM fields expect familiarity with biomolecular structures and experience in navigating enormous data resources, especially for those related to biology and chemistry, including medicine, public health, and environmental sciences.
• General audiences frequently view familiar images of molecular structures in various media, even outside STEM fields.
• Visualization of biomolecular structures may facilitate comprehension of the relative sizes (of atoms, molecules, cells, tissues, and organisms) and time scales of molecular interactions and functions (Duncan, 2007; Tibell and Rundgren, 2010; Jenkinson, 2018).
We have developed ready-to-use MCSs adaptable to multiple curricular contexts to facilitate molecular structure–function discussions in introductory- and advanced-level courses in biology, chemistry, and related sub-disciplines.
2.3 Molecular CaseNet and the purpose of the article
Various biology and chemistry professional society learning goals (Brownell et al., 2014; Dries et al., 2017; Wilson Sayres et al., 2018; American Chemical Society Committee on Professional Training, 2019; Zeidan et al., 2021) emphasize discussion of biomolecular structure–function relationships. So both biology and chemistry educators value teaching about molecular structures and functions. However, beyond their knowledge of biology and chemistry, educators need to acquire two other types of knowledge to prepare for teaching molecular structures: (a) technological –i.e., the knowledge to use tools and resources to access biomolecular structural data and visualize molecular to relate biomolecular structure and function, and (b) pedagogical –i.e., the knowledge to teach effectively by finding suitable material and engaging contexts that support students learning of molecular structure–function relationships. A community of multidisciplinary postsecondary (community college, undergraduate, and graduate-level) educators collaborated to form Molecular CaseNet and address these challenges. Members of this group focused on developing and using MCSs that explore molecular structure and function at the interface of biology and chemistry (Dutta and Trujillo, 2023; https://molecular-casenet.rcsb.org/).
In the last five years, more than sixty educators have connected with Molecular CaseNet to develop and use ~thirty different MCSs on a variety of topics ranging from genetic disorders, metabolic pathways, infectious diseases, convergent evolution, and more. Nine of these have been peer-reviewed and published as open education resources on the QUBES platform.1 Most of these MCSs have been viewed over a thousand times, downloaded hundreds of times, and adapted by multiple educators to meet various curricular contexts and needs. In addition, a few MCSs are currently in the peer-review process, more than a dozen are being piloted in various colleges and universities in the US and Canada prior to submission for publication, and a few more MCSs are in the process of being developed.
Molecular stories and molecular storytelling have been discussed in the literature (Goodsell et al., 2021) but have not been studied formally in relation to classroom teaching. Herein, we review the literature to summarize previous developments to guide our communication about MCSs. We then present the iterative process of developing a framework for MCSs by educators (members of Molecular CaseNet), testing its usability by piloting them in a variety of curricular settings, identifying challenges, and addressing them. The evolution of the MCS framework was guided by ongoing discussions with the Molecular CaseNet members. The central purpose of this report is to create a conceptual framework for teaching and learning with MCSs.
3 Previous scholarship
3.1 The technological, pedagogical, and content knowledge (TPCK) framework
According to Shulman (1986), knowledge of the subject matter alone is insufficient for teaching effectively; some knowledge of the theories and methods of education is also essential. He suggested that, at the intersection of these two types of knowledge, successful educators can (1) make disciplinary content comprehensible to learners and (2) understand learners’ challenges well enough to help them learn difficult subjects. Shulman originated the idea of combining pedagogical and content knowledge (PCK) for teaching. Later, the conceptualization of how educators must also leverage technology in the classroom extended this idea. The Technological, Pedagogical, and Content Knowledge (TPCK) framework provides a valuable scaffold for the multifaceted challenges and growth opportunities in teaching with technology (Koehler and Mishra, 2009). While discussing each TPCK component is helpful, they are most potent in unison for professional development, education design, and research while considering discipline, classroom, teacher, and student factors (Voogt et al., 2013; Rosenberg and Koehler, 2015).
The motivation for creating MCSs was to facilitate effective combination of three different types of knowledge for teaching (Figure 1):
• Technological, e.g., molecular visualization and bioinformatics
• Pedagogical, e.g., active learning through case studies and storytelling, and
• Content, e.g., biology, chemistry, and related subdisciplines, including structural biology.
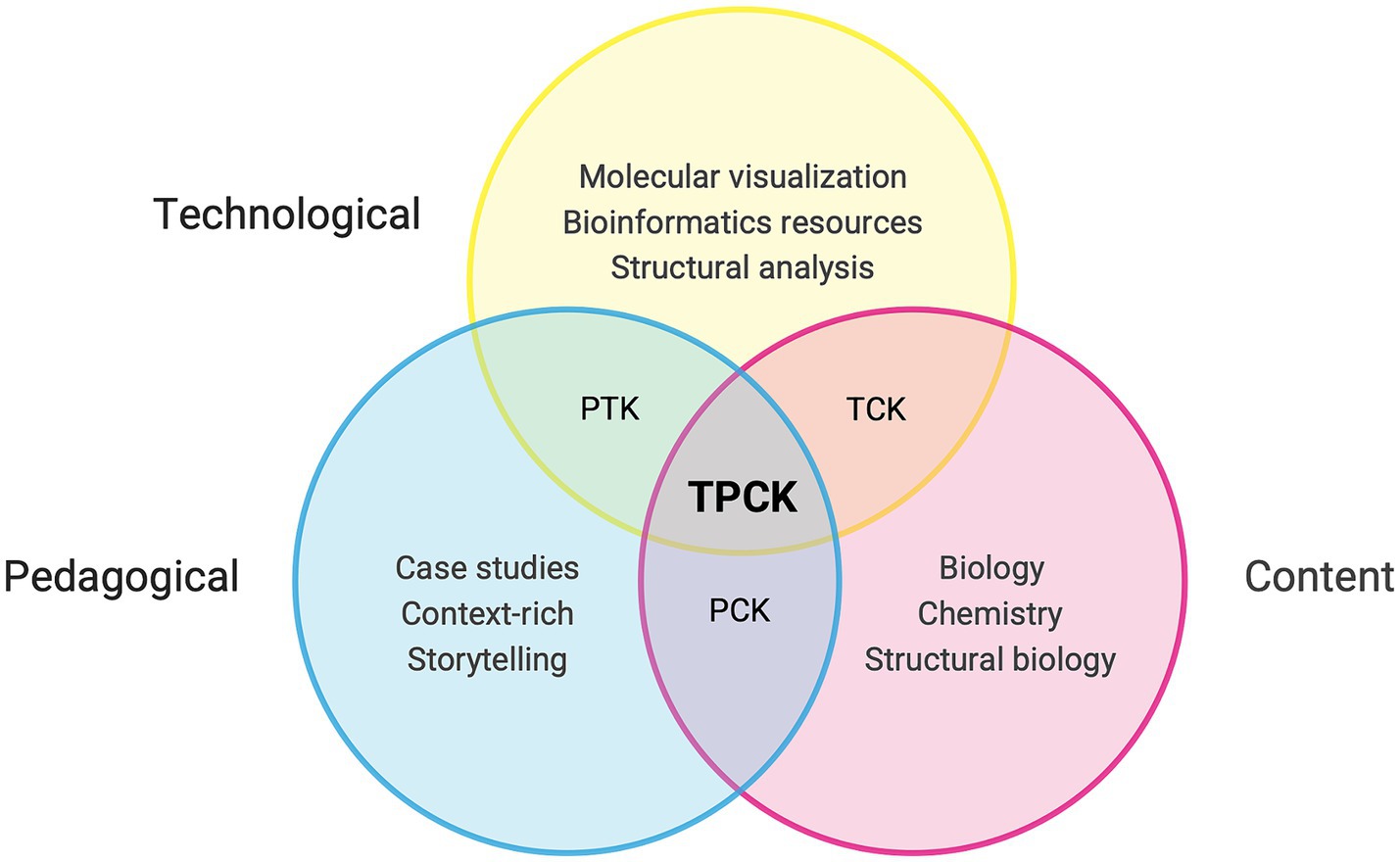
Figure 1. Venn diagram showing the TPCK components relevant to the MCSs. The figure is modified from Koehler and Mishra (2009).
Herein, we present the TPCK components in the context of MCSs and link each to previous literature.
3.2 Separate TPCK components
The technological knowledge (TK) emphasized in MCSs is the mastery of tools for biomolecular visualization and effective use of bioinformatics data resources. The PDB (RCSB.org; Berman et al., 2000, 2003, 2013) provides free access to 3D structural data of biological macromolecules, tools for structure visualization and analysis, and links to various bioinformatics data resources for research and education (Burley et al., 2023). Participating in MCSs not only requires interaction with these data, tools, and resources, but it is also essential to have some understanding of how they relate to each other and how to interpret them.
The pedagogical knowledge (PK) emphasized in MCSs is that of active learning through case studies. Case studies often use stories to provide opportunities to explore real-world scenarios, such as events in news media (Hibbard, 2019), and engage students in context-rich problem-solving (Herried, 1994; Harvard Business Publishing Editors, 2021). A recent review of case studies in chemistry reported that educators used a wide range of teaching methods to deliver instruction (Bernardi and Pazinato, 2022). Students improved conceptual understanding, team-based communication, critical thinking, problem-solving, and decision-making skills. Parallel positive outcomes have been seen in biology (Bonney, 2015) and biochemistry (Kulak et al., 2017). Context-based learning provides similar benefits for student conceptual understanding, motivation, and meta-cognition (Ültay and Çalık, 2012; Dori et al., 2018; Sevian et al., 2018). The MCSs focus on engaging students in actively exploring biomolecular structures to understand the molecular basis of authentic cases and answer questions about them.
Finally, content knowledge (CK) combines comprehension of concepts, vocabulary, and details related to structure and function and learning objectives in biology, chemistry, biochemistry, and bioinformatics listed in Table 1. Some knowledge of structural biology is also necessary to help understand where the 3D structural data (of experimental and computed structure models) come from and how they can facilitate understanding biology and chemistry. MCSs support multidisciplinary teaching and learning. So, educators with complementary disciplinary expertise in biology, chemistry, and related sub-disciplines (biochemistry, microbiology, genetics, etc.) can collaborate while authoring MCSs. Users of MCSs have the benefit of using and adapting published MCSs that may be suitable for their course theme but outside their specific area of expertise. MCSs can also enable educators teaching different disciplinary courses to coordinate their teaching so that students can begin to learn about topics from multidisciplinary perspectives.
3.3 Combined components
Technological pedagogical knowledge (TPK) is the command of teaching the tools used for identifying, visualizing, and analyzing case-related molecular structures and effectively navigating through bioinformatics data resources. In a nationwide survey, educators value the students’ ability to access data and use tools in bioinformatics, and this value increases by degree level (Wilson Sayres et al., 2018). Recently, a framework of themes, goals, and objectives was developed for biochemistry and molecular biology instructors to evaluate visual literacy in students (Dries et al., 2017). Representations or conceptual constructs commonly used in communicating biomolecular shapes and interactions are summarized in Supplementary Table S1A. Discussions amongst Molecular CaseNet members have helped identify and create resources to learn the use of molecular visualization tools and navigation through various bioinformatics resources. To effectively teach with MCSs, educators must master the tools and representations of biomolecules, foresee where learners will likely fall short of learning objectives, and aid their learning as needed.
The technological content knowledge (TCK) is the command of using 3D structural and bioinformatics data, tools, and resources in teaching disciplinary concepts. Molecular structure visualization is gaining popularity in chemistry (Tsaparlis, 1997; Jones, 2013) and biology education (Terrell and Listenberger, 2017). Similarly, the use of bioinformatics tools is growing in biology education (Ditty et al., 2010; Rele et al., 2023). MCSs provide an excellent platform for teaching students how to synthesize information from 3D structural and bioinformatics data with their knowledge of chemistry (e.g., the chemical interactions within and between biomolecules) and biology (e.g., information storage, signal transduction, metabolism), to gain a deeper understanding of the case-related research question(s). Like the other forms of knowledge, educators develop their mastery of TCK by accessing and practicing different technologies that support the disciplinary domain(s) of the courses they teach.
Pedagogical content knowledge (PCK) focuses on teaching biology and chemistry disciplinary content in molecular detail. A variety of discipline-specific experimental methods are used to investigate biomolecules. Method-specific representations are summarized in Supplementary Table S1B. In chemistry education and research, different levels of thought (macroscopic, submicroscopic, and symbolic) have different representations (Johnstone, 1991; Taber, 2013). This triangle of relationships continues to inform the design of instructional materials (e.g., Petillion and McNeil, 2020). In biology education and research, explanations about proteins often describe methods, analogies, contexts, theories, and ‘how’ the mechanism works (Trujillo et al., 2015) and integrate thermodynamics and kinetics when considering protein-folding and dynamics (Jeffery et al., 2018). Biology and chemistry educators strive to help students (a) reason through visual representations (Schönborn and Anderson, 2006, 2009; Offerdahl et al., 2017); (b) relate causal factors in biomolecular phenomena (Schwarz et al., 2020); (c) trace cellular and molecular activities through time and space (Machamer et al., 2000; van Mil et al., 2013, 2016), and (d) apply all of these skills to discuss protein-ligand binding (Trujillo et al., 2015, 2016a,b; Franovic et al., 2023). To effectively teach with MCSs, educators must support students in learning biology and chemistry concepts and the various conceptual and discipline-specific representations of biomolecules (see Supplementary Tables S1A,B).
TPCK is the combination of all of the above components an instructor develops to effectively teach about the biomolecular world in a technology-forward way. Little is known about how educators would develop TPCK for teaching using biomolecular structures and bioinformatics data. This manuscript documents, through the lens of TPCK, the products and process of developing a conceptual framework for MCSs. We describe the products (i.e., MCSs) and the evolution of a conceptual framework for authoring and using MCSs. We then position our conceptual framework for authoring MCSs in the context of TPCK. We will present our findings from observing how authoring and using MCSs impacted the educators’ TPCK in a separate publication.
4 The product: Molecular Case Studies
The MCSs engage students in exploring the molecular basis of authentic case themes and answering questions about them. A central activity in these case studies is the visualization of biomolecular 3D structures relevant to the case theme. Molecular visualization provides a platform for integrating knowledge from different sub-disciplines of biology and chemistry (e.g., biochemistry, genetics, and cell biology) to practice interdisciplinary, team science across STEM disciplines. The MCSs also act as an ideal vehicle for introducing students to a variety of freely available bioinformatics data resources that scientists and researchers use routinely, so that they can learn about the practice of science in a low-stakes environment. Finally, MCSs are effective teaching and learning tools, since they are aligned with the three aspects of learning addressed in the Universal Design for Learning (Orla et al., 2022) - (a) Engagement - i.e., why the case study topic is important; (b) Representation - i.e., what the students will learn from the case; and (c) Action and expression - i.e., how the students will learn and apply this knowledge. Key sections of MCSs aligned with the motivations and molecular story/storytelling components are shown in Figure 2. Herein we present the overall structure of an MCS and the types of learning it can support, followed by an example.
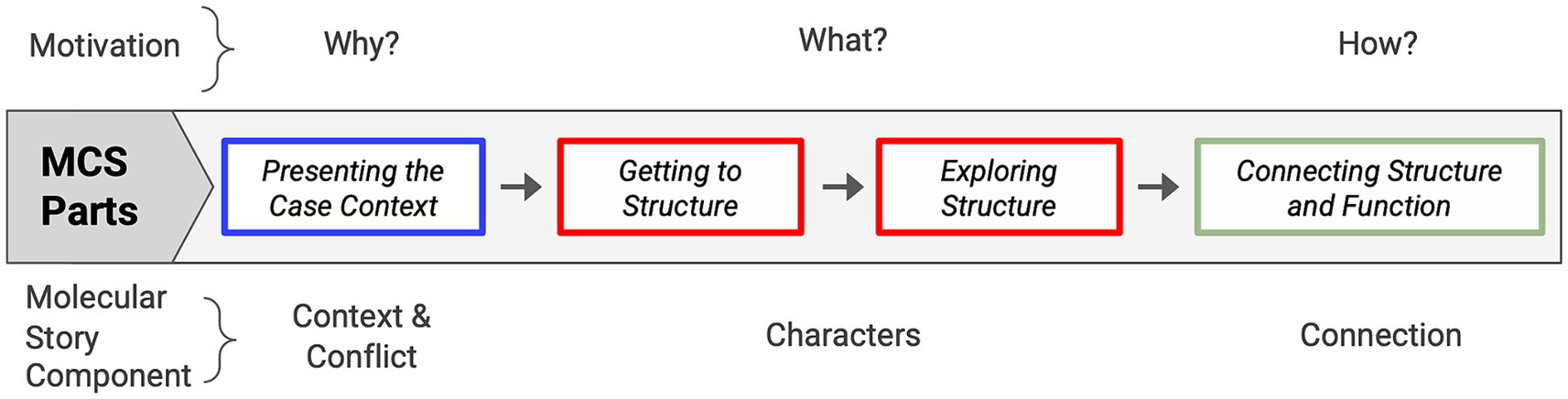
Figure 2. Sections of the molecular case study (MCS) aligned to the motivation for learning explored during phase two (top row) and molecular story component (bottom row).
4.1 Sections of a molecular case study
Each MCS has four main sections (Figure 2) described below:
1 Presenting the case context: The purpose of this section is to engage students in wanting to learn about the case theme. Students usually begin the MCS by watching an engaging video or reading an article or report describing an interesting or relatable observation or event that introduces the case study theme. Resources to introduce students to any essential background knowledge about the topic are included in the case study (so that they may understand the significance of the case context). This sets the stage for taking the first step in scientific curiosity (Jirout, 2020) - asking at least one specific question about the case theme to provide a purpose for the students’ biomolecular explorations.
Skills learned: Students learn to relate to an event or phenomenon they have encountered and ask questions about it, the first step in scientific curiosity.
2 Getting to the structure: The purpose of this section is to determine which biomolecules (proteins and/or their complexes) are relevant to the case study discussions. Students use clues presented in the case context, instructions provided in the case study, and various literature and bioinformatics resources (e.g., described in Sayers et al., 2022) to determine which protein(s) to study. They then search for and identify one or more specific 3D structure(s) to explore.
Skills learned: Students learn to read the scientific literature, gather relevant information, and use it to query the PDB to identify the case study-related biomolecules to explore.
3 Exploring the structure: The purpose of this section is to visualize and examine the overall shape and detailed interactions of the case study relevant 3D structures (identified in the previous section). Students visualize and analyze these structures using molecular visualization software such as Mol* (Sehnal et al., 2021), and gather details about the molecules and/or case study theme from bioinformatics data resources as needed.
Skills learned: Students learn to analyze and interpret 3D structural data and navigate through public data resources to gather details that are relevant to the information.
4 Connecting structure and function across scales: The purpose of this section is to integrate information from various sources, map them to the 3D structures being explored, to answer the case study question(s). Depending on the case study theme, students may have to relate how interactions at the molecular level can not only impact its function but transcend scales to impact a phenotype or physical observation presented in the case study.
Skills learned: Students learn to use information gathered from various sources to synthesize knowledge and explain the MCS question.
All published MCSs include a video, article, or report that introduces the case study; a worksheet guiding students through the MCS; answer keys for all case study questions, along with resources and reminders for the educator to pause and review or clarify key concepts used in the case study; case-relevant background resources; and teaching notes for implementation. MCSs are presented in a modular format, allowing flexible adoption and adaptation. For example, educators may assign specific sections of an MCS as homework assignments, while others may assign them as in-class activities to meet the needs of the course syllabus. The case study may be adapted to use alternate molecular visualization, bioinformatics, or classroom interaction tools to suit the course setting and resource availability. Additional sections may be added to the MCS to assess learning and connect the MCS to the curricular theme.
4.2 An example: Happy Blue Baby
This case study focuses on understanding the molecular basis of why an otherwise healthy baby girl turned blue soon after birth. The four sections of the case study are described as follows and also summarized in Figure 3:
1 Presenting the case context: The case begins by reading a local newspaper report describing the story of a baby girl born in Toms River, NJ, who turned blue soon after birth. All tests done in the neonatal intensive care unit were unable to diagnose possible reasons for the cyanosis, so the infant was taken to a specialist. The driving question for this case study is “Why did the baby turn blue?”
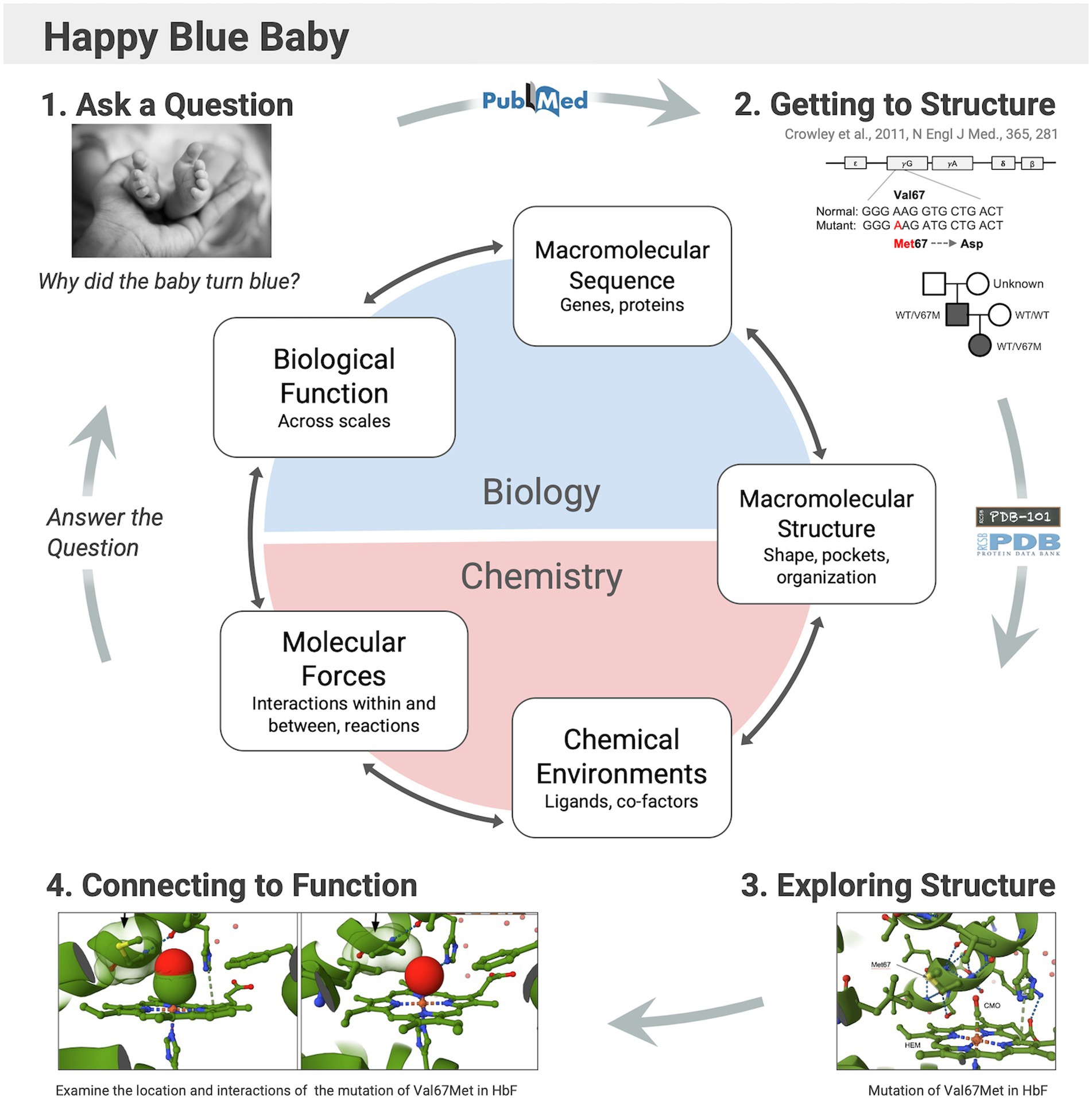
Figure 3. Glimpses of key steps in the MCS “Happy Blue Baby” (numbered 1–4). Note these steps are mapped on the Molecular Case Study cycle construct during phase 1 (modified from Dutta (2020a)).
In a conversation with the specialist, the baby’s grandmother revealed that her son (the baby’s father) had also experienced similar symptoms when he was born. Several tests were done at that time, too, but no conclusive diagnosis was made. Moreover, since the symptoms resolved in the first 6 months of his life, no further investigation was done. This conversation gave the specialist an important clue about the possible molecule to investigate.
At the end of this section, a mini lesson introduces students to fetal and adult hemoglobin. If these ideas have already been introduced in the course where this case study is being implemented, the instructor may decide to skip this mini lesson.
2 Getting to the structure: Following the lead provided by the baby’s grandmother, the specialist ordered some tests that led to the identification of a specific mutation in the fetal hemoglobin. Based on the nature of the mutation, students are asked to develop a hypothesis for why this mutation may lead to the baby turning blue. They are then guided to identify a structure in the Protein Data Bank (PDB) that includes this specific mutation where Valine 67 is changed to Methionine.
3 Exploring the structure: This section focuses on exploring the structure identified in the previous section - visualizing it and examining the mutated amino residue (Methionine 67) in the structure to note that it is located close to the heme group bound to the gamma hemoglobin protein chain in fetal hemoglobin (see Figure 2). However, this observation does not directly answer the question why the baby turned blue. So students are also guided to use specific tools available from RCSB.org to identify native structures of the fetal hemoglobin (without mutations) to compare the interactions of that amino acid and test the hypothesis that they developed about the molecular basis for the baby turning blue.
4 Connecting structure and function across scales: In this section, students compare the structures of the native and mutant proteins. They learn that the specific valine in question lines the pocket where the oxygen would bind the heme group. Since the methionine side chain is larger than the valine, it occupies a larger volume in the pocket and interferes with oxygen binding. The low oxygen binding, in turn, leads to higher proportions of deoxyhemoglobin in the blood, making the baby’s skin blue.
Following its initial publication (Dutta, 2020b), this MCS has been adapted by several educators to meet their specific curricular contexts. In one adaptation, the case was presented as an interrupted case study (Riley and Dutta, 2020), where students had to complete a section of the MCS in order to access instructions and information for the next section. Another adaptation (Procko, 2021) included multiple-choice questions to allow the use of the case study in a large classroom, while another adaptation focused on using the MCS in a large introductory-level virtual biochemistry class (Vardar-Ulu, 2021). Depending on the level and disciplinary focus of the course, instructors may create adaptations that use additional data presented in the case study materials to discuss oxygen binding in native and mutated forms of fetal and adult hemoglobin.
5 The process: developing a conceptual framework for molecular case studies
From initial conception to presenting it as a rich narrative model supporting the exploration of biomolecular structures and telling stories about them, our ideas about the contents, goals, and use of MCS have evolved over time. Herein, we describe some important milestones in the development of MCSs that parallel common steps followed in design-based research (Collins et al., 2004; Trujillo et al., 2016b), e.g., iterations, changes in priorities, and identification of pitfalls. We present this process in three phases, focused on: (1) defining requirements for an MCS; (2) specifying motivations for authoring (and using) MCSs, and (3) improving the MCS narrative, which enables effective molecular storytelling. The MCSs have been implemented in various courses and curricular settings (e.g., large and small classrooms, in-person and virtual classrooms, introductory-level vs. advanced-level courses). Feedback from these implementations helped identify gaps and challenges in the preparation and/or presentation of the MCSs. Discussions between Molecular CaseNet faculty about these challenges helped develop solutions and fueled the evolution of the MCS.
5.1 Phase 1: defining requirements for a molecular case study
When Molecular CaseNet was formed, the steering committee’s first order of business was to figure out the requirements and a format for MCSs enabling learning at the interface of biology and chemistry. Since biomolecular structures form an excellent bridge between biology and chemistry, the steering committee members agreed that exploring at least one biomolecular structure in atomic detail would be required for each MCS. At this stage, the key elements of an MCS included (a) an authentic theme or contexts to engage student interests; (b) a specific question related to the case study theme to motivate molecular structural explorations; and (c) at least one 3D structure of a protein relevant to the MCS, exploring which could help answer the case study question. A conceptual model, the Molecular Case Study Cycle (Dutta, 2020a), was developed to connect these key elements and describe how students could engage with them. An example of the MCS cycle in the context of the Happy Blue Baby case study is shown in Figure 3. The steering committee agreed that each MCS would complete at least one MCS cycle. The distinguishing feature of MCS (compared to other case studies) is the inclusion of an active molecular visualization and analysis and integration of information from public bioinformatics resources to answer the case study question(s).
Educators who joined Molecular CaseNet in the incubator phase of the project collaboratively authored several case studies using the MCS cycle as a guide. These MCSs presented a variety of themes and were written for different disciplinary courses (including biology, chemistry, biochemistry, and genetics). The molecular visualization tool used in these initial case studies was iCn3D (e.g., Wang et al., 2022) since, at that time, this was the only freely available web-based tool that did not need to be downloaded or installed for use. For a few cases, pre-made molecular exhibits from the Online Macromolecular Museum (Marcey, 2011) were also used as the beginning points of molecular explorations. A nationwide team of seventeen undergraduate educators from a variety of undergraduate universities, community colleges, and research institutions piloted these case studies as part of a Faculty Mentoring Network (FMN) hosted on the QUBES online platform (Donovan et al., 2015). The FMN participants provided feedback to the MCS authors so that they could make necessary updates before publishing the MCSs on Molecular CaseNet.
Lessons Learned: Educators who piloted these case studies reflected on their experiences and shared them with other participants during the FMN activities. These discussions indicated that many students, especially in introductory-level biology and chemistry courses, struggled to complete the MCSs since they were unfamiliar with -
a. specific biology and chemistry concepts and vocabulary used in the MCSs, and
b. the interfaces of 3D structural and bioinformatics data resources and tools for interacting with them.
In response to this awareness members of Molecular CaseNet began developing short videos, tutorials, and documentation to help support students and faculty while implementing the MCSs. Many of these resources are available online e.g., (see the Molecular CaseNet YouTube channel, Molecular CaseNet9461, n.d.), and materials continue to be developed, revised, and collected. Additionally, professional development opportunities were created, where practicing experts taught developing faculty interested in joining Molecular CaseNet, how to use various molecular visualization and analysis tools.
5.2 Phase 2: specifying motivations for authoring molecular case studies
After the creation of a few MCSs as proof-of-concept (described above), we set out to recruit Molecular CaseNet members with diverse disciplinary expertise and interests. Our goal was to engage them in authoring MCSs that would (a) be relevant in different disciplinary courses and curricular contexts and (b) address various learning goals that educators in biology, chemistry, and related disciplines set out to cover. To ensure that the MCSs authored by faculty with different disciplinary expertise meet the Molecular CaseNet requirements, we needed clear guidelines for developing and using them.
Documenting the steps for authoring MCSs was jump-started in 2020 during the COVID-19 lockdowns when many faculty had to find activities to support student learning outside the laboratory. A sub-group of faculty piloting MCSs engaged their senior-level undergraduate students in authoring MCSs as a partial course-based undergraduate research experience (CURE; Riley et al., 2021). These faculty collaborated to create a detailed guide for their students authoring MCSs. This guide was modified for broader use by all MCS authors. To ensure that faculty (both authors and users) would find an MCSs relevant to their courses and curricula, various professional society learning goals in biology, chemistry, bioinformatics, and molecular visualization (see Table 1), were woven into the guidelines. Listing these learning goals can (a) help authors stay on topic, (b) guide other educators in selecting MCS that works best for their curriculum, and (c) help Molecular CaseNet reviewers evaluate the MCSs for publication.
Our experiences described in the previous phase emphasized the value of piloting the MCSs at least once prior to submission to Molecular CaseNet for review and publication as an open educational resource. Given that MCSs may cover a variety of disciplinary topics the process for reviewing these case studies also needed to be clearly defined. In fact, the review process is divided into two parts - a part that applies to all MCSs and a discipline specific part that is unique to each case study and related to its case topic and or emphasis. A group of educators who had participated in authoring and using MCSs, worked together to document each step in the Molecular CaseNet pipeline for authoring, piloting, and reviewing MCSs for publication (Pettit et al., 2023).
Lessons Learned: As part of the Faculty Mentoring Network (hosted on the QUBES platform), diverse educators nationwide implemented the MCSs in various curricular contexts in spring 2021 and 2022. These educators reported their observations from implementing these case studies. Based on these reports, it became clear that many students and some of the developing educators (who have limited experience in molecular structure–function discussions) faced three types of challenges:
a. Relating different types of data: Many students (and even some developing faculty) had gaps in their understanding of the relationship between different types of bioinformatics data (e.g., protein and nucleic acid sequences, variations, mutations, functions), biomolecular structural data, and their biological/chemical implications.
b. Identifying relevant data: Many students needed to recognize and use the specific attributes of the case-related biomolecules to reliably identify their structures and specific details about them from bioinformatics data resources for structure–function discussions.
c. Connecting structure–function discussions to the case context: Many students needed more support in organizing and relating the structure–function discussions in the MCS with the case context and using biology/chemistry knowledge to answer the case study’s research question.
The first challenge is independent of the specific case study topic. Educators participated in post-FMN brainstorming sessions to suggest ways to overcome them. An outcome of these discussions motivated a group of the Molecular CaseNet faculty to create a collection of curricular materials called Box of Lessons, which introduces students and developing faculty to the data, tools, and resources available from RCSB.org and how to relate them to our knowledge of various types of biological macromolecule (Johnson et al., 2022). Following piloting, these lessons were published on the PDB-101 educational portal.2
The latter two challenges noted in these pilot implementations of the MCSs are case study specific. To address these, we have attempted to re-envision the narrative structure of the MCSs to improve the flow of activities in the MCS and facilitate improved teaching and learning.
5.3 Phase 3: improving the molecular case study narrative
To improve the MCS narrative, we needed to better understand the case-specific challenge points identified in the implementations described in section 5.2. We decided to map the narrative structure of MCSs to a popular storytelling framework. However, before we describe this mapping, we provide operational definitions for two terms used in the context of these case studies and then explain their relevance to MCSs:
• Molecular stories: They describe how in specific biological processes (contexts), key molecules (characters) related to the MCS topic, interact with other molecules (partner proteins, nucleic acids, ions, substrates, inhibitors, regulators etc.) to mediate one or more functions (changes and conflicts), and offer new insights or understanding (connections) about the topic and/or theme.
• Molecular storytelling: This is the investigative process or journey that the student takes in the MCS to identify, organize, and analyze crucial elements of the molecular story and relate them meaningfully to help complete the MCS and answer the question(s).
5.3.1 Molecular stories
A molecular story describes how specific molecules (characters) in specific biological processes (contexts) interact to mediate one or more functions (changes and conflicts) to offer new insights (connections) about the topic. The significance of each of these elements is described herein:
• Characters: The primary characters or biomolecules that play vital roles in the molecular story are driven by physical and chemical laws, facilitating biological processes on the cellular stage. In introductory-level courses, these characters may be shown in simplified representations, such as circles or boxes, while in an advanced-level discussion, the molecules may be shown in a variety of representations: - e.g., as a polymer sequence, chemical formula, or in 3D atomic detail as ribbons, molecular surfaces, or a combination of them. In addition to particulars of the primary character’s properties, shapes, interactions, and functions, the story may also discuss properties of partner proteins (i.e., secondary characters) that form a complex assembly with the primary character, especially when discussing their roles in tissue, organ, or organism-level functions.
• Context: Molecular characters in the story are in a situated biological and chemical system. Characteristics of the context need to be noted, understood, and considered throughout the story. Biological contexts may specify the type of cell and location in the cell, while chemical contexts may specify the nature of the environment where the molecular story takes place (e.g., in the nucleus, on the surface of the cell, in the blood plasma). In introductory-level discussions, the context may focus on only one or a few aspects of the molecular characters’ environment. In contrast, accounting of the overall environment and its interactions with the molecular character of interest, may provide a more accurate description and be suitable for advanced-level discussions. Understanding the context in detail may also include accounting for complexities and ambiguities due to experimental method-specific assumptions and limitations in the available data.
• Conflict: Learning about the conflict or challenge in the molecular characters helps explore their role in the story. A simple story may describe the normal functioning of a molecule and how its shape and interactions change under specific conditions. In a conflict (or disease situation), such as the presence of a mutation or binding to an inhibitor, the molecule’s interactions may change, leading to altered functions. Depending on the level of discussion (introductory or advanced), the detail in which the conflict or change-related interactions are described may vary - ranging from descriptive to quantitative.
• Connection: The final component of a molecular story connects or ties together the characters, context, and conflict. It presents rationales for how structural features of biomolecular characters facilitate its function(s). While an introductory-level discussion may be limited to visualizing biomolecular structures to explain their function(s), advanced-level dialogues connect specific interactions in molecular structures to chemical and biological measures of its functions - e.g., binding constants or the rate of reactions.
Example: In the Happy Blue Baby case study, the central character of the molecular story was the fetal hemoglobin, the context discussed in the story was the presence of a specific amino acid mutation, the conflict was the location of the mutated amino acid’s side chain near the oxygen binding pocket near the heme group, and the connection to function is the interference of the larger side chain of the mutated residue with oxygen binding.
5.3.2 Positioning molecular stories within molecular case studies
The molecular story only focuses on the biomolecules, their properties, interactions, and functions. MCSs, on the other hand, present an authentic and interesting story, motivating students to engage with the molecular story. Note that the MCS is also presented as a narrative with characters, contexts, conflicts or challenges, and connections. In introductory-level MCSs, the relationship between the molecular story and MCS components may be explicit, while in advanced-level implementations, students may have to explore the literature and various bioinformatics resources to figure out these relationships. Either way, the main role of these MCS components is to provide motivations for engaging with the topic of the molecular story. The significance of each of these is described herein:
• Characters that engage interest: The MCS characters may be an individual or a group of humans, animals, or plants facing a specific circumstance worthy of discussion. They may be introduced through a newspaper article, video, audio recording, or image as they present facts, experiences, and observations about the case study topic. The narrative of these characters makes the case study topic relatable, can engage students’ interest, and motivate them to learn more. Additional characters may also play essential roles in the case study discussion (e.g., educators, peer group members, or mentors) by providing complementary information about the topic or guiding students to resources to learn more about the case study topic.
• Context that provides real-world relevance: The MCS context presents authentic and observable conditions of the MCS character(s) that students can relate to. Exploring MCS contexts in molecular detail can help students appreciate why learning about this topic is important. Context also helps provide boundaries for meaningful discussions and effective information searches.
• Conflict that presents the case study problem(s): Conflict in the MCS refers to an observable challenge faced by the case study character(s). This challenge usually is the foundation of the research question driving the case study. Curiosity is a human instinct that can motivate students to learn more about the case study topic in molecular detail to answer the question.
• Connections that help design solutions: The crux of MCS is using knowledge about the molecule’s structure and function to help answer the research question. The MCS’s connection transcends scales and multiple disciplinary discussions to connect molecular functions to cellular, tissue, or organism-level outcomes. This connection may be limited to explaining the molecular basis for a macroscopic observation in an introductory molecular case study. However, at the most advanced-level, this connection may make way for designing new features or molecules with specific functions of interest, facilitating novel solutions to the conflict in the case study.
Example: In the Happy Blue Baby case study, the baby, her grandmother, her father, and the specialist (a doctor who diagnosed her condition) are all important characters in the MCS. The context and conflict is the baby’s cyanosis (baby turning blue after birth), which is diagnosed by connecting the information shared between the characters, test results, and follow-up research to uncover the molecular story about the fetal hemoglobin mutation that interferes with oxygen binding.
In summary, each MCS has at least one molecular story included in it. While both MCSs and molecular stories include four components - characters, contexts, change or conflicts, and connections, they play different roles. The relationships between these components in introductory- and advanced-level discussions of MCSs are summarized in Table 2.
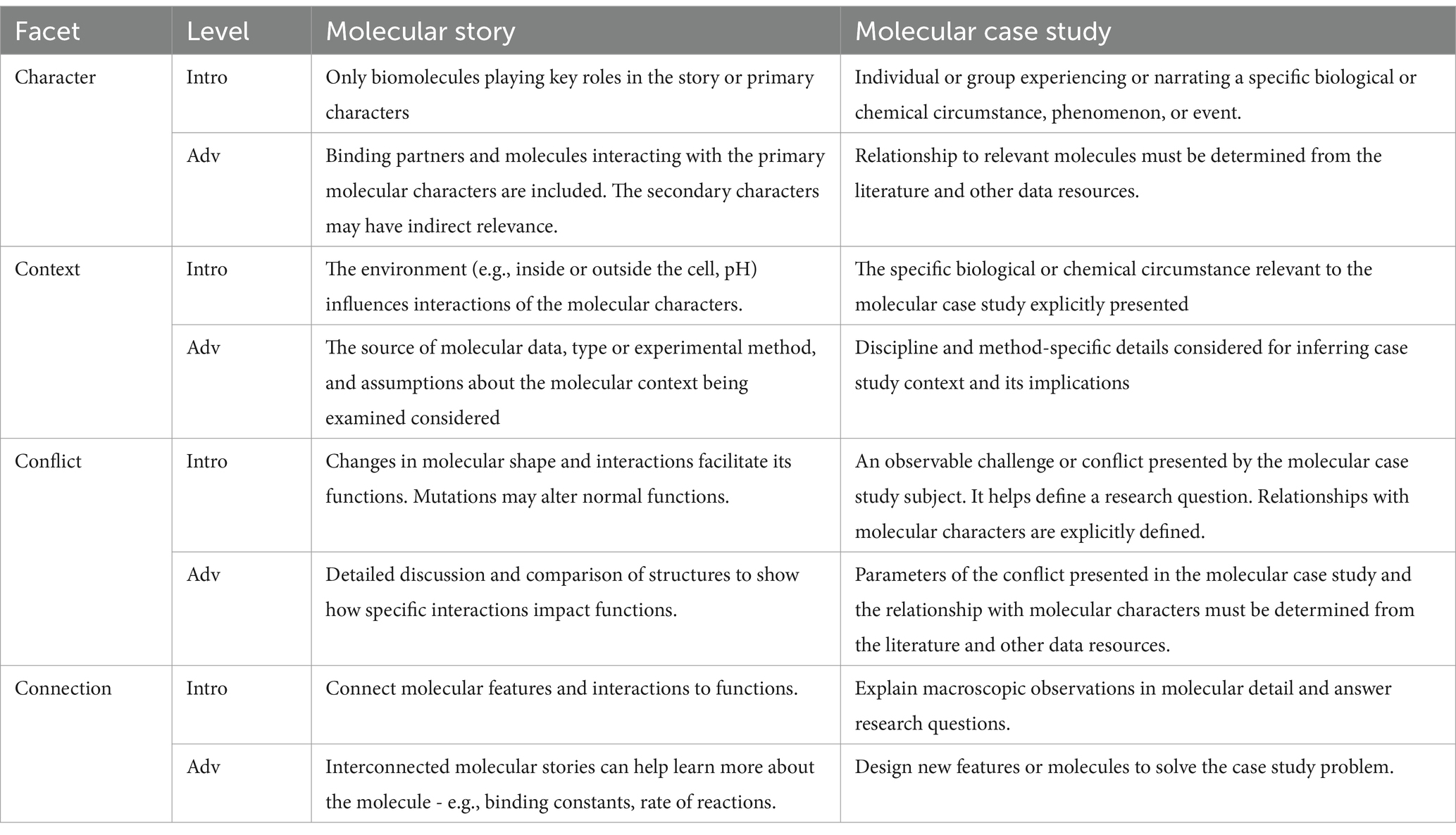
Table 2. Relating the key components of molecular stories and molecular case studies to each other at introductory (Intro) and advanced (Adv) levels.
5.3.3 Storytelling in molecular case studies for teaching and learning
Case studies are designed to be active learning experiences. Molecular storytelling is an active learning process where students take on an investigative journey to identify, organize, and analyze crucial elements of the molecular story and meaningfully relate them to complete the molecular case study. Students begin by learning about the MCS context, characters, and conflict. Their goal is to identify and explore the structure and functions of key molecular players (i.e., narrate a molecular story) and connect the MCS components to answer the case study question(s). Just as in any case study, where students are assigned to a specific point of view (Herried, 2019), students participating in MCSs also take on particular roles or perspectives - e.g., that of a person living with a specific disease or their friend, caregiver, or doctor. The student’s journey to answer a research question in molecular detail can be equated to the Hero’s Journey, a popular framework for narrative storytelling.
Campbell’s (1949) book titled The Hero with a Thousand Faces detailed the recurring patterns in stories about mythological heroes told across cultures. He claimed that virtually every Hero’s Journey or story from across the globe follows a single unifying pattern, a monomyth. Although Campbell’s thesis has faced criticism, it has since been repurposed from mythology to provide a roadmap for producing compelling stories by Vogler (1992), a Hollywood film producer and writer. This structure can be seen in cinematic epics like Star Wars, Harry Potter, and Lord of the Rings and in science communication through the power of stories (Olson, 2015). We noted the similarity between the stages of the student’s active learning journey in the MCS (molecular storytelling) and that described in the Hero’s Journey:
Hero’s Journey:
Heroes are (1) introduced in the Ordinary World, where they (2) receive the call to adventure. They (3) are reluctant at first or refuse the call, but (4) are encouraged by a mentor to (5) cross the first threshold and enter the Special World, where they (6) encounter tests, allies, and enemies. They (7) approach the inmost cave, crossing a second threshold where they (8) endure the supreme ordeal. They (9) take possession of their reward and are (10) pursued on the road back to the Ordinary World. They cross the third threshold, (11) experience a Resurrection, and are transformed by the experience. They (12) return with the elixir, a boon or treasure, to benefit the Ordinary World. [Emphasis modified] (Vogler, 1992, pg. 30).
Molecular Storytelling Journey (a parallel description of Hero’s Journey for MCSs):
Students are (1) introduced to a case theme in the Macroscopic World, where they (2) receive the call to answer a research question. They (3) are reluctant at first or need to learn new concepts and tools, but (4) are encouraged by a teacher to (5) cross the threshold and enter the Molecular World, where they (6) encounter many molecular structures and bioinformatics data. They (7) approach the case-related molecular structures, where they (8) endure a detailed analysis. They (9) take possession of their knowledge of structure–function relationships and (10) are driven to bring back answers to the research questions in the Macroscopic World. They cross the next threshold, (11) experience a new understanding of the case study, and are transformed by their knowledge. They (12) return with their structure–function insights to benefit the Macroscopic World.
We mapped the MCS cycle (Dutta, 2020a) onto the Hero’s Journey steps that Vogler (1992) articulated to identify parallels in the student’s Molecular Storytelling Journey. The mapping is shown in Figure 4 and listed in Table 3, along with the pedagogical relevance of the main steps. The ordinary world of the hero parallels the macroscopic world of the student’s case study theme. The student’s journey begins with an introduction to the case (in the blue portion, Figure 4), setting the stage for asking the research question (steps 1 and 2). Students learn (or review) disciplinary concepts and relevant technology (steps 3 and 4) before embarking on their journey. They then enter the molecular world to search for, identify, retrieve, visualize, and analyze relevant molecular structures and information from public data resources to relate them to structure and function (steps 5–9, red portion). Finally, they connect the molecular and macroscopic worlds by synthesizing new knowledge and applying it to answer the case study’s question (steps 10–12, green portion of cycle).
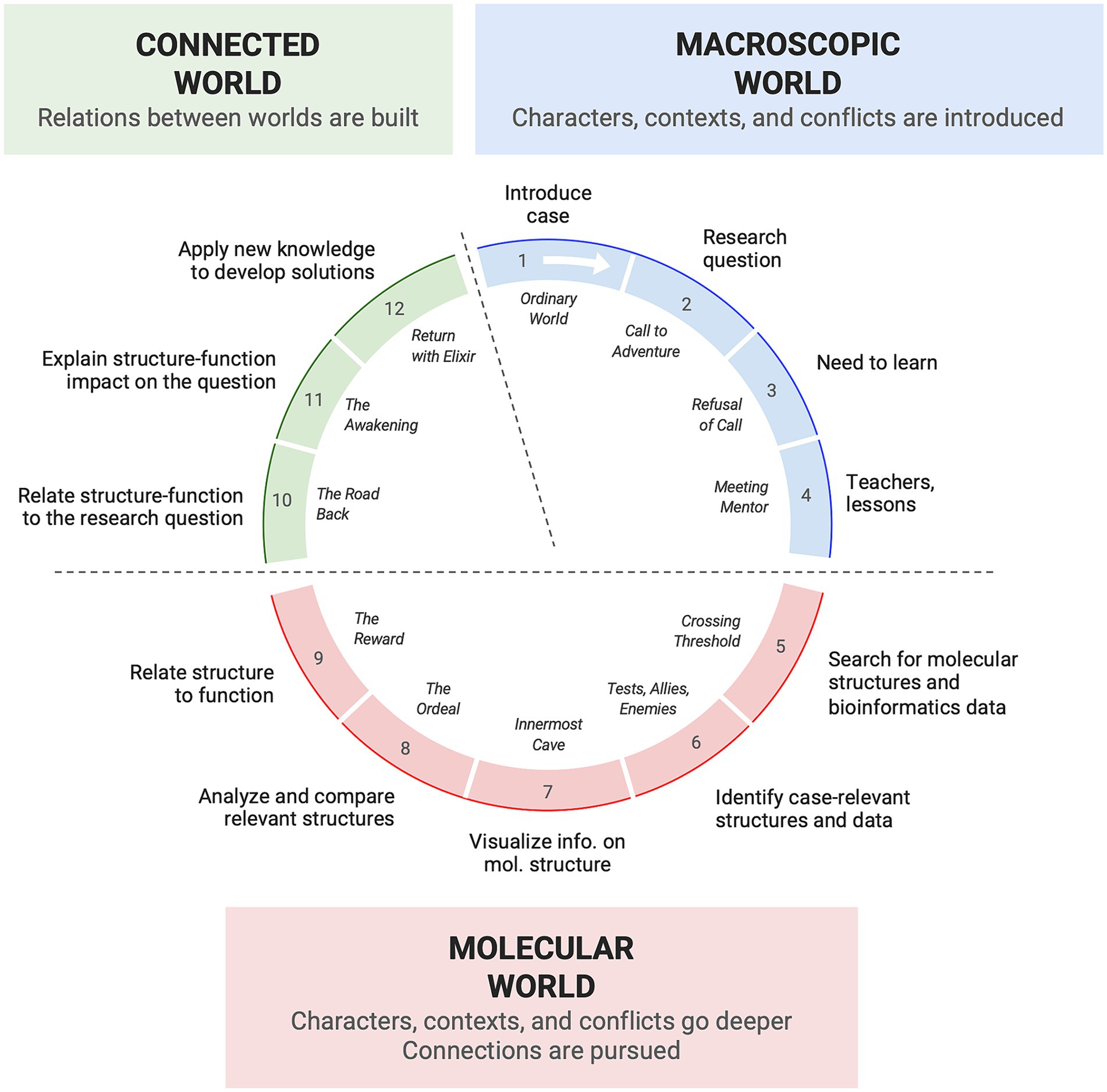
Figure 4. Mapping the Hero’s Journey to steps in the student’s Molecular Storytelling Journey. A Hero’s Journey model that combines key elements of molecular stories, case studies, and storytelling. The model begins at the top center and shows a clockwise sequence of steps the students take (outer circle). The corresponding steps of the Hero’s Journey are in italics in the inner circle. A horizontal dashed line separates the macroscopic and molecular worlds, while a vertical line separates the journey beginning from its conclusion. The macroscopic, microscopic, and integrated worlds are marked in blue, red, and green.
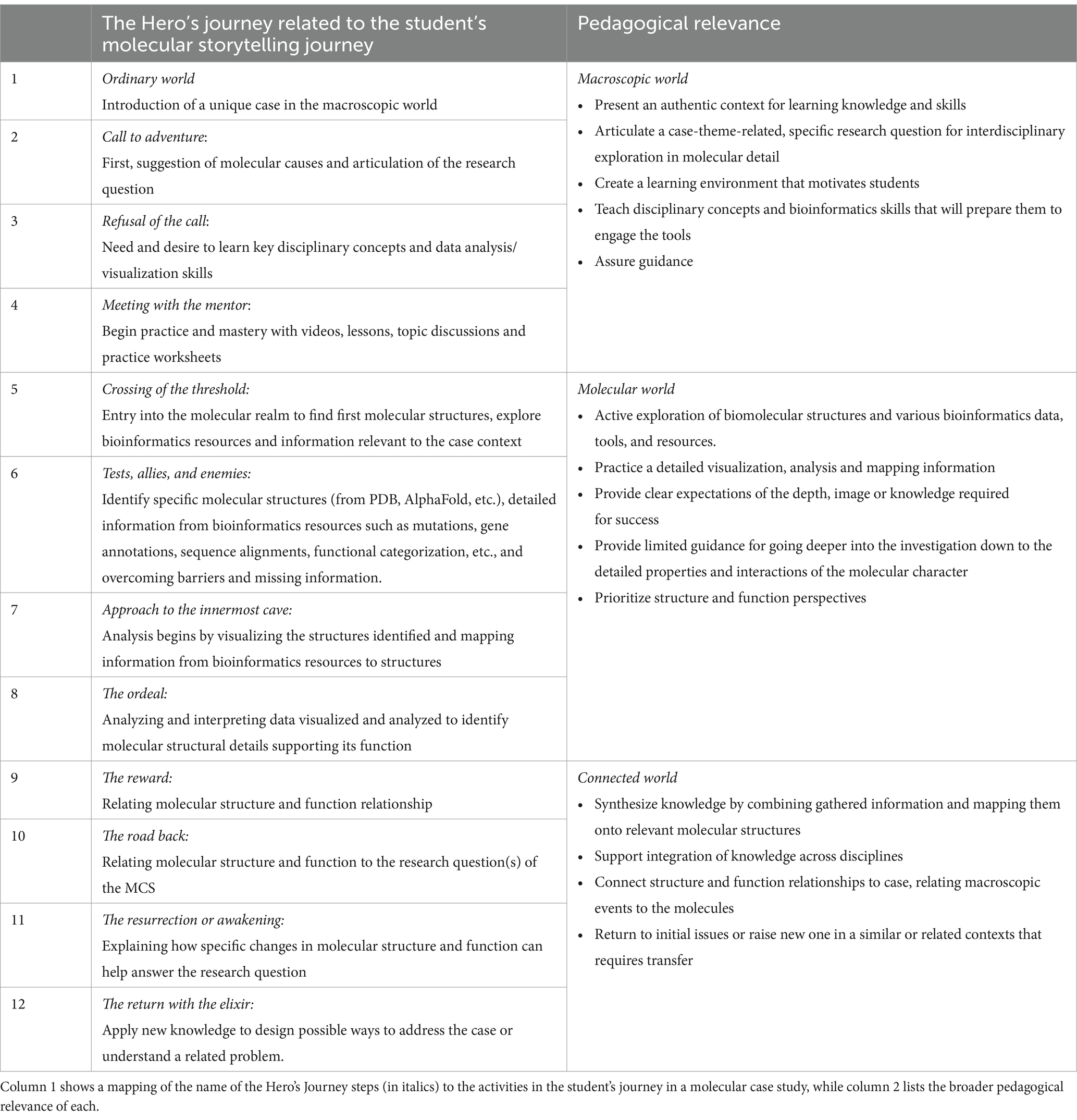
Table 3. Relating the steps of the student’s molecular storytelling journey to the pedagogical relevance.
The student’s journey framework (Figure 4) allows MCS authors and users to visually map molecular storytelling in a purposeful way. It recognizes points where problems may arise, helping educators proactively prepare to support student learning. For example, the challenges identified during initial implementations of MCSs in introductory-level courses, i.e., gaps in understanding concepts, vocabulary, and tool interfaces (section 5.1, Lessons Learned, a and b) and data relationships (section 5.2, Lessons Learned, a) can be addressed in steps 3 and 4 before the student attempts to enter the molecular realm. The horizontal dashed line in Figure 4 separates the macroscopic world (top) from the molecular world (bottom). Two case-specific challenges, i.e., identification of case-relevant data and connecting molecular structure–function to macroscopic observations (Section 5.2, Lessons Learned, b and c) mapped around the intersection points of the horizontal line and the student’s journey circle (Figure 4) at steps 5 and 10. Depending on the course level and prior experience of students participating in the MCS, these steps may be suitably scaffolded by the educator (using notes and resources included in the MCS).
A conceptual framework for developing and using MCSs: The overarching goal of MCSs is to engage students in using MCSs to relate macroscopic observations of real-world situations to molecular explanations. We began by identifying key elements of an MCS and connecting them in the MCS cycle (Section 5.1). Following iterations of creation, implementation, and identification of challenges (sections 5.1–5.3), we have developed a conceptual framework that relates key aspects of the MCS authoring process (Figure 2) and the MCS cycle (Dutta, 2020a,b) in the student’s Molecular Storytelling Journey (Figure 4). The students’ journey presents a conceptual framework that can guide MCS authors and users to successfully implement the MCSs. Three key elements of this framework include:
a. An engaging case: The first section of an MCS (Figure 1) and steps 1–4, the blue section of the students’ journey (Figure 4), help set the stage. If students can relate to the MCS characters, context, and conflict presented here, they may be engaged in the molecular explorations.
b. The molecular story: The second and third sections of an MCS (Figure 1), and steps 5–9, the red section (Figure 4), are situated in the molecular realm and provide opportunities to learn how to access data from public data resources, select case-relevant molecules, visualize their structures, examine interactions, and explain their functions. In doing so, students learn more about the molecular characters, context, conflict, and the connections between them. With practice, students can gain confidence in using these tools and resources for projects beyond the MCS.
c. Connecting worlds: The last section of an MCS, and steps 10–12, the green section of the student’s journey (Figure 4), focus on building connections between the macroscopic and molecular worlds. Here students have the opportunity to understand the importance of structure–function discussions in addressing real-world challenges.
Observing Molecular CaseNet faculty as they engaged in authoring, implementing, publishing, and using MCSs allowed us to develop a foundation for examining how by developing MCSs (and using them to engage students in molecular storytelling), educators can explore and teach about biomolecular structural and bioinformatics data to further interdisciplinary teaching, learning, and scholarship.
6 Situating the MCS conceptual framework within TPCK
To teach effectively, educators need to fully integrate their TPCK. As we progressed through iterative phases of authoring, implementing, and revising MCSs (described in the above sections), the representation, field testing, and redesign of the educators’ TPCK needs were also addressed (Trujillo et al., 2016b). Herein we review the various phases in developing the conceptual framework for authoring and using MCSs with a TPCK lens.
In phase 1, educators used the MCS cycle to guide both the authoring and implementation of MCSs. When authoring an MCS, they identified a molecule relevant to a lesson in their course (CK), created a hook (interesting story related to the topic) for the case study (PK), and included molecular visualization tools to explore the structures (TK). We supported the educators as they authored these molecule-centered case studies (PCK). However, educators who implemented the MCSs lacked some of the foundational concepts in structural biology and case topic-related disciplinary knowledge (CK). They also had limited awareness of bioinformatics data resources and their disciplinary implications in the MCSs (TCK). For some educators, these challenges reduced their confidence in effectively implementing MCSs in their classrooms (TPCK). Following initial implementations, the Molecular CaseNet members collectively addressed gaps by developing instructions and video tutorials for using some of the technological tools (TK, TPK), short concept-focused lessons (CK, PCK), and other materials to support the diverse disciplinary backgrounds of our participants (CK). Additionally, professional development workshops were hosted with experts to support general and case-specific integration of visualization tools and bioinformatics resources (TK, TPK, TCK, TPCK).
In phase 2, once the basic structure and process for authoring MCSs were in place, we focused on expanding our MCS collection. We set out to develop case studies that could be used in diverse disciplinary courses and cover a variety of professional society learning goals (CK, PCK, TPCK). In this phase, many educators set up collaborations to author MCSs (TPCK through collaborations) - they found other educators with complementary and multidisciplinary expertise (CK, PCK) or those with experience in using a variety of molecular visualization and bioinformatics tools (TK, TCK). We also saw the beginnings of sharing knowledge through peer partnerships and mentorship for newer members of the CaseNet community. A small group of Molecular CaseNet educators collaboratively developed detailed instructions for the complete publication pipeline (including authoring, piloting, revising, submitting, and reviewing MCSs, TPCK). Implementation of MCSs in this phase identified challenges that students were facing in understanding the relationships between different types of data (CK), how to access them (TK), and use them in the context of the case study (TPCK). In response, the Box of Lessons, a collection of curricular materials, was collaboratively developed by CaseNet members, piloted, and published (All TPCK).
In phase 3, our recent focus has been to improve the narrative structure of MCSs (PCK, TPK). We defined key story elements (characters, context, conflicts, and connections) at the level of the molecular story (CK, PCK) and also for MCS (All TPCK). By mapping the investigative journey in the MCS to the Hero’s Journey, we developed a road map for guiding student discussions across scales, i.e., in the macroscopic and molecular worlds (TPCK). As new MCSs are authored using the student’s journey framework and implemented in classrooms, we will monitor if all the challenge points identified in previous sections have been addressed using this framework.
7 Discussion
7.1 Summary
The goal of this project is to engage students in actively visualizing and analyzing biomolecular structures, exploring bioinformatics data resources, and participating in structure–function discussions about authentic topics at the interface of biology and chemistry. We chose case studies as the pedagogical approach for attaining this goal and developed molecular case studies (MCSs). We began by defining the requirements for MCSs, and through a process of collaborative creation, testing, and refinement, we developed a conceptual framework for authoring and using MCSs. In addition to describing the key components, we present operational definitions for molecular stories and storytelling in the context of MCSs. This report describes the products (MCSs) of this effort and the process for developing the conceptual framework.
As we collaborated in authoring, implementing, and revising the MCSs, we noted that the participating post-secondary educators teaching various disciplines (biology, chemistry, biochemistry, microbiology, etc.) had different levels of knowledge, experience, and confidence in teaching with and about biomolecular structure and function. We noted that through our discussions identifying and addressing challenges in developing and using MCSs, it was important to pay attention to the TPCK (Figure 1, Koehler and Mishra, 2009) of the people (faculty) involved. By creating the MCS products and process for developing and using them, we have constructed a framework for assessing how participating in Molecular CaseNet activities can shift educators’ TPCK.
7.2 Contributions
The main contributions described in this report are organized into three categories: products, processes, and people.
7.2.1 Products
The scientific literature is full of papers describing stories of molecular structures and their functions. Many molecular stories have also been told for teaching and learning (Marcey, 2011; Prilusky et al., 2011; Goodsell et al., 2015). So neither molecular storytelling nor the incorporation of molecular visualizations in education is an original idea. However, the operational definitions connecting molecular stories and storytelling in the context of MCSs are novel. Educators can use the conceptual framework described here to author and use MCSs to advance interdisciplinary teaching and learning. Moreover, MCSs present low-stakes, guided opportunities to explore public data resources that experts routinely use. Thus, in addition to providing students with a molecular understanding of the topic and introducing them to new technologies, data, and tools to use them, MCSs also motivate students to practice the scientific process of identifying relevant data and integrating multidisciplinary information to derive new knowledge.
7.2.2 Processes
Most textbooks and lessons exploring molecular structures introduce students to structure–function discussions about a limited set of well-studied molecules such as lysozyme, hemoglobin, and chymotrypsin. MCSs present opportunities to examine a broad range of topics in molecular detail and gain new insights and knowledge about the course theme. Additionally, these open education resources are modular and adaptable, enabling educators to customize MCSs to meet their specific curricular needs.
Besides MCSs, a number of supporting resources, such as short tutorials for using tools, videos to learn key concepts, and practice worksheets, were developed as a part of Molecular CaseNet activities. This was facilitated by discussions as part of the online faculty mentoring networks hosted on the QUBES platform.
7.2.3 People
The work described here primarily involves post-secondary educators authoring and using MCSs. Currently, more than a dozen MCSs are in various stages of completion. Immediate benefits to participating faculty include access to the collection of MCSs on diverse topics. In addition, connections to a community of peers using and developing MCSs have allowed participating faculty options to independently or collaboratively author new MCSs focused on a topic of interest. As part of the Molecular CaseNet activities, participating educators have availed and also presented a number of professional development opportunities. For example, some educators have established collaborations across disciplines, geography, and expertise on projects beyond authoring MCSs. Others have engaged their students in developing MCSs (e.g., Riley et al., 2021) or materials for the Box of Lessons. Through these efforts, faculty have produced publications and developed resources that facilitate their career growth and further interdisciplinary teaching, learning, and scholarship.
For students, the MCSs position them as the protagonists of their learning, engaging in real-world examples, using authentic data resources, learning key concepts and skills in biology, chemistry, and related sciences, and doing what researchers would do as they practice science. Based on previous studies, we anticipate that students exploring case studies build their representational competency and molecular explanations, an area known to be difficult (Tibell and Rundgren, 2010). The MCS narratives activate Johnstone’s triangle by guiding students to integrate their understanding of the macroscopic and atomic events through domain-appropriate representations (see Supplementary materials; Petillion and McNeil, 2020). Additionally, the purposeful engagement to use data, molecular visualizations, and bioinformatics practices in specific contexts aligns closely with the parts molecular life scientists and students include in their explanations (Trujillo et al., 2015, 2016a; Jeffery et al., 2018). The ultimate goal of Molecular CaseNet is to improve learners’ understanding of the molecular world.
7.3 Considerations
The modular structure of MCSs offers flexibility but places the responsibility on the implementing faculty to invest some time in preparing for and adapting it for desired learning goals and alignment with their specific course and curriculum. This report proposes a conceptual framework for developing and using MCSs derived from the previous literature and through an iterative model development process (Trujillo et al., 2016b). While the molecular story and storytelling components seem to be logically organized and complementary within the MCSs, their impact on implementation and student learning is yet to be validated empirically. We hypothesize that chemistry and biology educators using or authoring MCSs in the classroom will produce evidence of integrated TPCK as their knowledge related to teaching biomolecular structural and bioinformatics data increases. A report that includes data to test our hypothesis and validate a model for TPCK development through molecular storytelling is forthcoming.
In most published cases, the student’s experiences in molecular storytelling map well to the Hero’s Journey. However, we encountered a few case studies where the transitions between the macroscopic and molecular worlds did not answer the research question asked. For example, this may happen when the structure of the protein of interest has not yet been determined. The student taking the molecular storytelling journey can respond to this situation by looking for highly similar structures of homologous proteins and exploring them to try and answer the research question. These events closely matched another type of story structure, a plot-point narrative, with multiple rising actions and resolutions along the journey. We would argue that changing research questions or data sources due to a lack of reliable information is an authentic science practice compatible with MCSs, and a wonderful way to encourage students to discuss the nature of research and the process that scientists use to push the frontiers of knowledge.
8 Conclusion and future outlook
The molecular stories contribute an interdisciplinary perspective of the biomolecular world that aids educators in teaching structure–function relationships through engaging case studies. Placing educators and students as heroes and storytellers allows them to build connections and approach biomolecules as a journey of discovery. Doing so provides opportunities to bring online biomolecular data and platforms into the classroom.
Engagement with technology is essential but often not the central priority in many current interdisciplinary learning environments. While the specific tools and technology (e.g., molecular visualization tool) may evolve, the emphasis here is on practicing to take on the journey. The Molecular Storytelling Journey depicts active learning with a purpose. The student’s journey crosses boundaries between macroscopic and molecular worlds to seek a deep understanding of molecular structure–function relationships and their observable phenotypes. As students return from their molecular world adventure, they bring back new insights to share - both as the students and as a new molecular storyteller.
The conceptual framework presented gives us a way to test the impact that molecular storytelling and MCSs have on TPCK. Each of the anticipated TPCK changes could be measured through interviews, surveys, the produced lesson designs, their practices in the classrooms, and the success of their students. As a learning theory that could aid interpretation, social constructivism (Amineh and Asl, 2015) holds that knowledge develops through social activities like collaborations and shared language development. It will help explain the process of TPCK development to understand how Molecular CaseNet collaborations impacted faculty. Well-developed TPCK will also support student growth in engaging and exploring the invisible living world.
Looking ahead, we intend to increase the number of CaseNet program participants, MCSs, and supporting educational resources to successfully implement the MCSs. We invite interested educators to connect with us and join our group. The eventual goal is to assess the impact of MCSs on student learning of the disciplinary concepts and processes of science. It is important to understand how the MCSs are being effectively developed and implemented in various curricular settings.
Data availability statement
The original contributions presented in the study are included in the article Supplementary material, further inquiries can be directed to the corresponding author.
Author contributions
CT: Writing – review & editing, Writing – original draft, Visualization, Methodology, Investigation, Funding acquisition, Conceptualization. SD: Writing – review & editing, Writing – original draft, Visualization, Supervision, Project administration, Investigation, Funding acquisition, Conceptualization.
Funding
The author(s) declare that financial support was received for the research, authorship, and/or publication of this article. This material is based on work supported by the NSF under grant numbers DBI 1827011 and 2018884. Any opinions, findings, conclusions, or recommendations expressed in this publication are those of the authors and do not necessarily reflect the views of the National Science Foundation. Author Dutta works for RCSB PDB, which is funded by the National Science Foundation (DBI-1832184), the US Department of Energy (DE-SC0019749), and the National Cancer Institute, National Institute of Allergy and Infectious Diseases, and National Institute of General Medical Sciences of the National Institutes of Health under grant R01GM133198.
Acknowledgments
We want to thank all of the participants in the Molecular CaseNet for their many contributions to our ongoing conversations and for developing, publishing, and teaching molecular cases. We also thank them for their tireless efforts to improve the teaching and learning of biomolecular structure and function in various disciplinary contexts. This report is a direct product of several years of insightful discussions.
Conflict of interest
The authors declare that the research was conducted in the absence of any commercial or financial relationships that could be construed as a potential conflict of interest.
Publisher’s note
All claims expressed in this article are solely those of the authors and do not necessarily represent those of their affiliated organizations, or those of the publisher, the editors and the reviewers. Any product that may be evaluated in this article, or claim that may be made by its manufacturer, is not guaranteed or endorsed by the publisher.
Supplementary material
The Supplementary material for this article can be found online at: https://www.frontiersin.org/articles/10.3389/feduc.2024.1379515/full#supplementary-material
Footnotes
References
Alberts, B. (1998). The cell as a collection of protein machines: preparing the next generation of molecular biologists. Cell 92, 291–294. doi: 10.1016/S0092-8674(00)80922-8
American Association for the Advancement of Science (2011) in Vision and change in undergraduate biology education: A call to action. eds. C. A. Brewer and D. Smith (Washington, DC: American Association for the Advancement of Science).
American Chemical Society Committee on Professional Training. (2019) Macromolecular, Supramolecular, and Nanoscale (MSN) Systems in the Curriculum. Available at: https:///Users/sdutta/Downloads/macromolecular-supramolecular-nanoscale-supplement-3.pdf
Amineh, R. J., and Asl, H. D. (2015). Review of constructivism and social constructivism. J. Soc. Sci. Lit. Lang. 1, 9–16
Barbra, N. C., and Stark, L. A. (2014). Engaging with molecular form to understand function. CBE Life Sci. Educ. 13, 21–24. doi: 10.1187/cbe.13-12-0247
Benson, D. A., Cavanaugh, M., Clark, K., Karsch-Mizrachi, I., Lipman, D. J., Ostell, J., et al. (2013). GenBank. Nucleic Acids Res. 41, D36–D42. doi: 10.1093/nar/gks1195
Berman, H., Henrick, K., and Nakamura, H. (2003). Announcing the worldwide protein data Bank. Nat. Struct. Mol. Biol. 10:980. doi: 10.1038/nsb1203-980
Berman, H. M., Kleywegt, G. J., Nakamura, H., and Markley, J. L. (2013). The future of the protein data Bank. Biopolymers 99, 218–222. doi: 10.1002/bip.22132
Berman, H. M., Westbrook, J., Feng, Z., Gilliland, G., Bhat, T. N., Weissig, H., et al. (2000). The Protein Data Bank. Nucleic Acids Res. 28, 235–242. doi: 10.1093/nar/28.1.235
Bernardi, F. M., and Pazinato, M. S. (2022). The case study method in chemistry teaching: a systematic review. J. Chem. Educ. 99, 1211–1219. doi: 10.1021/acs.jchemed.1c00733
Bonney, K. M. (2015). Case study teaching method improves student performance and perceptions of learning gains. J. Microbiol. Biol. Educ. 16, 21–28. doi: 10.1128/jmbe.v16i1.846
Brownell, S. E., Freeman, S., Wenderoth, M. P., and Crowe, A. J. (2014). BioCore guide: a tool for interpreting the core concepts of vision and change for biology majors. CBE Life Sci. Educ. 13, 200–211. doi: 10.1187/cbe.13-12-0233
Burley, S. K., Bhikadiya, C., Bi, C., Bittrich, S., Chao, H., Chen, L., et al. (2023). RCSB protein data Bank (RCSB.org): delivery of experimentally-determined PDB structures alongside one million computed structure models of proteins from artificial intelligence/machine learning. Nucleic Acids Res. 51, D488–D508. doi: 10.1093/nar/gkac1077
Callaway, E. (2022). The entire protein universe’: AI predicts shape of nearly every known protein. Nature 608, 15–16. doi: 10.1038/d41586-022-02083-2
Campbell, J. (1949). The Hero with a thousand faces. 3rd Edn. Novato, California: New World Library, Joseph Campbell Foundation.
Chen, P., Lee, N. V., Hu, W., Xu, M., Ferre, R. A., Lam, H., et al. (2016). Spectrum and degree of CDK drug interactions predicts clinical performance. Mol. Cancer Ther. 15, 2273–2281. doi: 10.1158/1535-7163.MCT-16-0300
Collins, A., Joseph, D., and Bielaczyc, K. (2004). Design Research: Theoretical and Methodological Issues Journal of the Learning Sciences, 13, 15–42.
Dahlstrom, M. F. (2014). Using narratives and storytelling to communicate science with nonexpert audiences. Proc. Natl. Acad. Sci. 111, 13614–13620. doi: 10.1073/pnas.1320645111
Ditty, J. L., Kvaal, C. A., Goodner, B., et al. (2010). Incorporating genomics and bioinformatics across the life sciences curriculum. PLoS Biol. 8:e1000448. doi: 10.1371/journal.pbio.1000448
Donovan, S., Eaton, C. D., Gower, S. T., Jenkins, K. P., LaMar, M. D., Poli, D., et al. (2015). QUBES: a community focused on supporting teaching and learning in quantitative biology. Lett. Biomath. 2, 46–55. doi: 10.30707/LiB2.1Donovan
Dori, Y. J., Avargil, S., Kohen, Z., and Saar, L. (2018). Context-based learning and metacognitive prompts for enhancing scientific text comprehension. Int. J. Sci. Educ. 40, 1198–1220. doi: 10.1080/09500693.2018.1470351
Dries, D. R., Dean, D. M., Listenberger, L. L., Novak, W. R., Franzen, M. A., and Craig, P. A. (2017). An expanded framework for biomolecular visualization in the classroom: learning goals and competencies. Biochem. Mol. Biol. Educ. 45, 69–75. doi: 10.1002/bmb.20991
Duncan, R. G. (2007). The role of domain-specific knowledge in generative reasoning about complicated multileveled phenomena. Cogn. Instruct 25, 271–336. doi: 10.1080/07370000701632355
Dutta, S. (2020a). The molecular case study cycle : CCCE Newsletter American Chemical Society division of chemical education committee on computers in chemical education. Available at: https://confchem.ccce.divched.org/2020CCCENLP2.
Dutta, S. (2020b). Happy Blue Baby. Molecular CaseNet faculty mentoring network spring 2020, QUBES educational resources. doi: 10.25334/2R67-W933
Dutta, S., and Dries, D. (2019). Virtual exploration of biomolecular structure and function. Biochem. Educ. Theory Pract. 1337, 21–41. doi: 10.1021/bk-2019-1337.ch00
Dutta, S., and Trujillo, C. (2023). Molecular CaseNet - developing and using molecular case studies at the interface of biology and chemistry (RCN-UBE introduction). RCN-UBE Community, QUBES Educational Resources. doi: 10.25334/VKQN-0X96
Franovic, C. G. C., Noyes, K., Stoltzfus, J. R., Schwarz, C. V., Long, T. M., and Cooper, M. M. (2023). Undergraduate chemistry and biology students’ use of causal mechanistic reasoning to explain and predict preferential protein-ligand binding activity. J. Chem. Educ. 100, 1716–1727. doi: 10.1021/acs.jchemed.2c00737
Goodsell, D. S., Dutta, S., Voigt, M., and Zardecki, C. (2021). Molecular storytelling for online structural biology outreach and education. Struct. Dyn. 8:020401. doi: 10.1063/4.0000077
Goodsell, D. S., Dutta, S., Zardecki, C., Voigt, M., Berman, H. M., and Burley, S. K. (2015). The RCSB PDB “molecule of the month”: inspiring a molecular view of biology. PLoS Biol. 13:e1002140. doi: 10.1371/journal.pbio.1002140
Harvard Business Publishing Editors (2021). The heart of the case method, part 2: Students as stakeholders in their own learning success : Harvard Business Publishing Available at: https://hbsp.harvard.edu/inspiring-minds/the-heart-of-the-case-method-centennial-part-2.
Herried, C. F. (1994). Case studies in science--a novel method of science education. J. Coll. Sci. Teach. 23, 221–229
Herried, C. F. (2019). The chef returns: a recipe for writing great case studies. J. Coll. Sci. Teach. 48, 38–42. doi: 10.2505/4/jcst19_048_03_38
Hibbard, L. (2019). Case studies for general chemistry: teaching with a newsworthy story. J. Chem. Educ. 96, 2528–2531. doi: 10.1021/acs.jchemed.9b00420
Holme, T., Luxford, C., and Murphy, K. (2015). Updating the general chemistry anchoring concepts content map. J. Chem. Educ. 92, 1115–1116. doi: 10.1021/ed500712k
Huang, R., O’Donnell, A. J., Barboline, J. J., and Barkman, T. J. (2016). Convergent evolution of caffeine in plants by co-option of exapted ancestral enzymes. Proc. Natl. Acad. Sci. 113, 10613–10618. doi: 10.1073/pnas.1602575113
Jeffery, K. A., Pelaez, N., and Anderson, T. R. (2018). How four scientists integrate thermodynamic and kinetic theory, context, analogies, and methods in protein-folding and dynamics research: implications for biochemistry instruction. CBE Life Sci. Educ. 17:ar13. doi: 10.1187/cbe.17-02-0030
Jenkinson, J. (2018). Molecular biology meets the learning sciences: visualizations in education and outreach. J. Mol. Biol. 430, 4013–4027. doi: 10.1016/j.jmb.2018.08.020
Jirout, J. J. (2020). Supporting earlyscientific thinking through curiosity. Front. Psychol. 11:1717. doi: 10.3389/fpsyg.2020.01717
Johnson, K. A., Pettit, A. S., Vemu, S., and Dutta, S. (2022). A “Box of Lessons” for exploring biomolecular structure and function. 2022 Biology and Mathematics Educators (BIOME) Institute, QUBES Educational Resources. doi: 10.25334/K480-QR38
Johnstone, A. H. (1991). Why is science difficult to learn? Things are seldom what they seem. J. Comp. Assist. Learn. 7, 75–83. doi: 10.1111/j.1365-2729.1991.tb00230.x
Jones, L. J. (2013). How multimedia-based learning and molecular visualization change the landscape of chemical education research. J. Chem. Educ. 90, 1571–1576. doi: 10.1021/ed4001206
Koehler, M. J., and Mishra, P. (2009). What is technological pedagogical content knowledge? Contemp. Issues Technol. Teach. Educ. 9, 60–70
Kohn, K. P., Underwood, S. M., and Cooper, M. M. (2018). Connecting structure-property and structure-function relationships across the disciplines of chemistry and biology: exploring student perceptions. CBE Life Sci. Educ. 17:ar33. doi: 10.1187/cbe.18-01-0004
Kulak, V., Newton, G., and Sharma, R. (2017). Does the use of case-based learning impact the retention of key concepts in undergraduate biochemistry? Int. J. High. Educ. 6, 110–120. doi: 10.5430/ijhe.v6n2p110
LaDue, N. D., Libarkin, J. C., and Thomas, S. R. (2015). Visual representations on high school biology, chemistry, earth science, and physics assessments. J. Sci. Educ. Technol. 24, 818–834. doi: 10.1007/s10956-015-9566-4
Machamer, P., Darden, L., and Craver, C. F. (2000). Thinking about mechanisms. Philos. Sci. 67, 1–25. doi: 10.1086/392759
Marcey, D. (2011). The Online Macromolecular Museum Exhibits. Available at: http://earth.callutheran.edu/Academic_Programs/Departments/BioDev/omm/exhibits.htm. (Accessed January 1, 2024).
Mol, C. D., Dougan, D. R., Schneider, T. R., Skene, R. J., Kraus, M. L., Scheibe, D. N., et al. (2004). Structural basis for the autoinhibition and STI-571 inhibition of c-kit tyrosine kinase. J. Biol. Chem. 279, 31655–31663. doi: 10.1074/jbc.M403319200
Molecular CaseNet9461. (n.d.) Molecular CaseNet Home [YouTube Channel]. YouTube. Available at: https://www.youtube.com/@molecularcasenet9461
Mughal, F., Nasir, A., and Caetano-Anollés, G. (2020). The origin and evolution of viruses inferred from fold family structure. Arch. Virol. 165, 2177–2191. doi: 10.1007/s00705-020-04724-1
Offerdahl, E. G., Arneson, J. B., and Byrne, N. (2017). Lighten the load: scaffolding visual literacy in biochemistry and molecular biology. CBE Life Sci. Educ. 16:es1. doi: 10.1187/cbe.16-06-0193
Oksenberg, D., Dufu, K., Patel, M. P., Chuang, C., Li, Z., Xu, Q., et al. (2016). GBT440 increases haemoglobin oxygen affinity, reduces sickling and prolongs RBC half-life in a murine model of sickle cell disease. Br. J. Haematol. 175, 141–153. doi: 10.1111/bjh.14214
Olson, R. (2015). Houston, we have a narrative: Why science needs story. Chicago: University of Chicago Press.
Olson, A. J. (2018). Perspectives on structural molecular biology visualization: from past to present. J. Mol. Biol. 430, 3997–4012. doi: 10.1016/j.jmb.2018.07.009
Orla, K., Buckley, K., Lieberman, L., and Arndt, K. (2022). Universal Design for Learning - a framework for inclusion in outdoor learning. J. Outdoor Environ. Educ. 25, 75–89. doi: 10.1007/s42322-022-00096-z
Owen, D. R., Allerton, C. M. N., Anderson, A. S., Aschenbrenner, L., Avery, M., Berritt, S., et al. (2021). An oral SARS-CoV-2 Mpro inhibitor clinical candidate for the treatment of COVID-19. Science 374, 1586–1593. doi: 10.1126/science.abl4784
Petillion, R. J., and McNeil, W. S. (2020). Johnstone’s triangle as a pedagogical framework for flipped-class instructional videos in introductory chemistry. J. Chem. Educ. 97, 1536–1542. doi: 10.1021/acs.jchemed.9b01105
Pettit, A. S., Pollock, E., Procko, K., Riley, K., Vardar-Ulu, D., and Dutta, S. (2023). The Molecular CaseNet pipeline for authoring, piloting, and evaluating molecular case studies. 2023 BIOME Institute, QUBES Educational Resources. doi: 10.25334/9G7Y-8E04
Prilusky, J., Hodis, E., Canner, D., Decatur, W. A., Oberholser, K., Martz, E., et al. (2011). Proteopedia: a status report on the collaborative, 3D web-encyclopedia of proteins and other biomolecules. J. Struct. Biol. 175, 244–252. doi: 10.1016/j.jsb.2011.04.011
Procko, K. P. (2021). Happy Blue Baby: MCQs for large classes. Molecular CaseNet Faculty Mentoring Network spring 2021, QUBES Educational Resources. doi: 10.25334/8ETN-K437
Rele, C. P., Sandlin, K. M., Leung, W., and Reed, L. K. (2023). Manual annotation of Drosophila genes: a genomics education partnership protocol. F1000Res 11:1579. doi: 10.12688/f1000research.126839.3
Riley, K., and Dutta, S. (2020). The Happy Blue Baby Hemoglobin. Molecular CaseNet Faculty Mentoring Network Spring 2020, QUBES Educational Resources. doi: 10.25334/N5JP-1P96
Riley, K. J., Vardar-Ulu, D., Pollock, E., and Dutta, S. (2021). Students authoring molecular case studies as a partial course-based undergraduate research experience (CURE) for lab instruction. Biochem. Mol. Biol. Educ. 49, 853–855. doi: 10.1002/bmb.21578
Rosenberg, J. M., and Koehler, M. J. (2015). Context and technological pedagogical content knowledge (TPACK): a systematic review. J. Res. Technol. Educ. 47, 186–210. doi: 10.1080/15391523.2015.1052663
Rybarczyk, B. (2011). Visual literacy in biology: a comparison of visual representations in textbooks and journal articles. J. Coll. Sci. Teach. 41:106
Sayers, E. W., Bolton, E. E., Brister, J. R., Canese, K., Chan, J., Comeau, D. C., et al. (2022). Database resources of the National Center for Biotechnology Information. Nucleic Acids Res. 50, D20–D26. doi: 10.1093/nar/gkab1112
Schönborn, K. J., and Anderson, T. R. (2006). The importance of visual literacy in the education of biochemists. Biochem. Mol. Biol. Educ. 34, 94–102. doi: 10.1002/bmb.2006.49403402094
Schönborn, K. J., and Anderson, T. R. (2009). A model of factors determining students’ ability to interpret external representations in biochemistry. Int. J. Sci. Educ. 31, 193–232. doi: 10.1080/09500690701670535
Schwarz, C., Cooper, M., Long, T., Trujillo, C., Noyes, K., de Lima, J, et al. (2020). Mechanistic explanations across undergraduate chemistry and biology courses. In M. Gresalfi and I. S. Horn (Eds.), The Interdisciplinarity of the Learning Sciences, 14th International Conference of the Learning Sciences (ICLS) 2020, 1 (625–628)
Sehnal, D., Bittrich, S., Deshpande, M., Svobodová, R., Berka, K., Bazgier, V., et al. (2021). Mol* viewer: modern web app for 3D visualization and analysis of large biomolecular structures. Nucleic Acids Res. 49, W431–W437. doi: 10.1093/nar/gkab314
Sevian, H., Dori, Y. J., and Parchmann, I. (2018). How does STEM context-based learning work: what we know and what we still do not know. Int. J. Sci. Educ. 40, 1095–1107. doi: 10.1080/09500693.2018.1470346
Shulman, L. S. (1986). Those who understand: knowledge growth in teaching. Educ. Researcher 15, 4–14. doi: 10.2307/1175860
Taber, K. S. (2013). Revisiting the chemistry triplet: drawing upon the nature of chemical knowledge and the psychology of learning to inform chemistry education. Chem. Educ. Res. Pract. 14, 156–168. doi: 10.1039/C3RP00012E
Terrell, C. R., and Listenberger, L. L. (2017). Using molecular visualization to explore protein structure and function and enhance student facility with computational tools. Biochem. Mol. Biol. Educ. 45, 318–328. doi: 10.1002/bmb.21040
Tibell, L. A., and Rundgren, C. J. (2010). Educational challenges of molecular life science: characteristics and implications for education and research. CBE Life Sci. Educ. 9, 25–33. doi: 10.1187/cbe.08-09-0055
Trujillo, C. M., Anderson, T. R., and Pelaez, N. J. (2015). A model of how different biology experts explain molecular and cellular mechanisms. CBE Life Sci. Educ. 14:14:ar20. doi: 10.1187/cbe.14-12-0229
Trujillo, C. M., Anderson, T. R., and Pelaez, N. J. (2016a). Exploring the MACH model’s potential as a metacognitive tool to help undergraduate students monitor their explanations of biological mechanisms. CBE Life Sci. Educ. 15:ar12. doi: 10.1187/cbe.15-03-0051
Trujillo, C. M., Anderson, T. R., and Pelaez, N. J. (2016b). An instructional design process based on expert knowledge for teaching students how mechanisms are explained. Adv. Physiol. Educ. 40, 265–273. doi: 10.1152/advan.00077.2015
Tsaparlis, G. (1997). Atomic and molecular structure in chemical education – a critical analysis from various perspectives of science education. J. Chem. Educ. 74, 922–925. doi: 10.1021/ed074p922
Ültay, N., and Çalık, M. (2012). A thematic review of studies into the effectiveness of context-based chemistry curricula. J. Sci. Educ. Technol. 21, 686–701. doi: 10.1007/s10956-011-9357-5
UniProt Consortium (2023). UniProt: the universal protein knowledgebase in 2023. Nucleic Acids Res. 6, D523–D531. doi: 10.1093/nar/gkac1052
van Mil, M. H. W., Boerwinkel, D. J., and Waarlo, A. J. (2013). Modelling molecular mechanisms: a framework of scientific reasoning to construct molecular-level explanations for cellular behaviour. Sci. Educ. 22, 93–118. doi: 10.1007/s11191-011-9379-7
van Mil, M. H. W., Postma, P. A., Boerwinkel, D. J., Klaassen, K., and Waarlo, A. J. (2016). Molecular mechanistic reasoning: toward bridging the gap between the molecular and cellular levels in life science education. Sci. Educ. 100, 517–585. doi: 10.1002/sce.21215
Vardar-Ulu, D. (2021). Happy Blue Baby. Molecular CaseNet Faculty Mentoring Network Spring 2021, QUBES Educational Resources, doi: 10.25334/0T61-9383
Vogler, C. (1992). The Writer’s journey: Mythic structure for writers. Michael Wiese Productions. 3rd, 1st Edn.
Voogt, J., Fisser, P., Pareja Roblin, N., Tondeur, J., and van Braak, J. (2013). Technological pedagogical content knowledge– a review of the literature. J. Comput. Assist. Learn. 29, 109–121. doi: 10.1111/j.1365-2729.2012.00487.x
Wang, J., Youkharibache, P., Marchler-Bauer, A., Lanczycki, C., Zhang, D., Lu, S., et al. (2022). iCn3D: from web-based 3D viewer to structural analysis tool in batch mode. Front. Mol. Biosci. 9:831740. doi: 10.3389/fmolb.2022.831740
Watson, J., and Crick, F. (1953). Molecular structure of nucleic acids: a structure for deoxyribose nucleic acid. Nature 171, 737–738. doi: 10.1038/171737a0
Werner, E. (2022). Strategies for the production of molecular animations. Front. Bioinform. 2:793914. doi: 10.3389/fbinf.2022.793914
Wilson Sayres, M. A., Hauser, C., Sierk, M., Robic, S., Rosenwald, A. G., Smith, T. M., et al. (2018). Bioinformatics core competencies for undergraduate life sciences education. PLoS One 13:e019687:e0196878. doi: 10.1371/journal.pone.0196878
Yoho, R., Urban-Lurain, M., Merrill, J., and Haudek, K. (2018). Structure and function relationships in the educational expectations of professional societies across the STEM disciplines. J. Coll. Sci. Teach. 47, 24–31. doi: 10.2505/4/jcst18_047_06_24
Zeidan, Q., Loertscher, J., Wolfson, A. J., Tansey, J. T., Offerdahl, E. G., Kennelly, P. J., et al. (2021). Development of a certification exam to assess undergraduate students’ proficiency in biochemistry and molecular biology core concepts. CBE life. Sci. Educ. 20:es6. doi: 10.1187/cbe.19-12-0265
Keywords: biology education, case studies, technological pedagogical and content knowledge (TPCK), molecular structure and function, molecular visualization, bioinformatics education, conceptual modeling
Citation: Trujillo CM and Dutta S (2024) Molecular storytelling: a conceptual framework for teaching and learning with molecular case studies. Front. Educ. 9:1379515. doi: 10.3389/feduc.2024.1379515
Edited by:
Carlos C. Goller, North Carolina State University, United StatesReviewed by:
Pilar Gema Rodríguez Ortega, University of Cordoba, SpainMichaela Gazdik Stofer, North Carolina State University, United States
Copyright © 2024 Trujillo and Dutta. This is an open-access article distributed under the terms of the Creative Commons Attribution License (CC BY). The use, distribution or reproduction in other forums is permitted, provided the original author(s) and the copyright owner(s) are credited and that the original publication in this journal is cited, in accordance with accepted academic practice. No use, distribution or reproduction is permitted which does not comply with these terms.
*Correspondence: Caleb M. Trujillo, Y2FsZWJ0cnVAdXcuZWR1