- 1Departamento de Curriculum e Instrucción, Universidad de Concepción, Concepción, Chile
- 2College of Education, University of Massachusetts Amherst, Amherst, MA, United States
Science educators today still struggle with finding better ways to help students develop strong conceptual understandings as opposed to memorizing isolated facts. Recently there has been increased attention on learning explanatory models as a key to conceptual understanding. Science educators also struggle with how to teach students scientific thinking practices, and sometimes this goal is seen as being in competition with content goals for conceptual understanding. In this study we ask whether whole class discussion can contribute to both of these goals at the same time and whether there are ways that a teacher can support this. We describe the results of a case study of an experienced teacher leading modeling discussions in a series of three middle school life sciences classes. A qualitative microanalysis of the videotaped whole class discussions led to the identification of a variety of modeling processes operating across the lessons at two different time scale levels. These include model competition, in which students compare and evaluate their models, and model evolution, in which the models go through stepwise evaluation and improvement. The latter process involves a smaller time scale pattern of model generation, evaluation, and modification cycles. All of these processes are similar to those found in recent studies of practices of expert scientists. Implications from the case study suggest that: (1) A teacher need not be limited to the two opposing interaction styles of Open Discussion vs. Authoritative lecture. Rather, there are there intermediate discussion styles between these that involve co-construction and cognitive scaffolding; (2) It is possible to start from student-generated models that conflict with the target model in a number of ways, and still arrive at the target model for the lesson through discussion. Processes of model competition and disconfirmation, as well as model evolution, both supported by the teacher's cognitive scaffolding, were central in this accomplishment; (3) In doing so, it is possible for a teacher to foster student modeling practices, as a type of scientific thinking, at the same time that they are teaching science content, by scaffolding the two levels of model construction processes identified.
Introduction
We are encouraged by current educational reform ideas such as emphases on teaching for scientific thinking and teaching for conceptual understanding, as well as the use of small group and whole class discussions that draw out student ideas and thinking. Our particular focus in this article is on how whole class discussions can contribute to the learning of conceptual models in science, as well as fostering modeling practices as a central form of scientific thinking.
However, in our experience in educating preservice and in-service teachers, we have become sensitive to several tensions or dilemmas experienced by them in engaging such reforms, even for those who have accepted the desirability of teaching both scientific thinking practices and models, as a form of disciplinary content goals, in their syllabus. We will distinguish three types of tension (see Figure 1):
Conceptual Dissonance Tension: In trying to utilize open whole class discussion to tap into and start from students' ideas for explaining scientific phenomena, they uncover some useful ideas but also uncover some ideas that are in conflict with their target model for the lesson (Scott et al., 2006, 210).
Lesson Objective Tension: Since discussions for scientific thinking can be time consuming and many are under pressure to cover content, there is a perceived tension or competition between science content goals and scientific thinking goals.
Tension Between Opposing Teaching Approaches: When they try to pursue both of the latter goals, they tend to associate inquiry methods such as open discussion with thinking goals, and there is a tension about not knowing when to use open discussion and when to use a more authoritative approach (e.g., lecture; Scott et al., 2006, p. 606).
In this paper we attempt to identify practices occurring in productive whole class discussions by conducting a case study of an experienced science teacher who appears to have found a method that resolves or reduces these tensions. She appears to use several different modes of discussion and to have ways of cognitively scaffolding student thinking during critical pieces of the discussion. Our goal is to identify new ways of describing the most important classroom interactions and processes that take place in this class sequence.
Doing a qualitative microanalysis of discussions over three consecutive lessons has the potential to develop such a set of descriptive concepts along with developing a multi-level framework of model construction processes, in order to provide a foundation for broader studies. The challenge is to identify the set of model construction processes that allow students to participate in building a complex scientific model (In this article we will use “scientific (thinking) processes” and “scientific practices” as synonyms).
Previous Literature and Theoretical Framework
Important Previous Work on Tensions
In an important precedent for our study, Scott et al. (2006, p. 606) point to a growing interest in studying the role of discussion or discourse in the science classroom. They cite curriculum initiatives based on student inquiry and argumentation, where discussion appears to be central in drawing out student ideas. They conclude that discussion is key for relating new concepts to the student's everyday prior knowledge. On the other hand, they conclude that the teacher is key in guiding and supporting classroom discussion as s/he takes into account students' perspectives but also has the goal of arriving at targeted models for a unit. But they also acknowledge that discussion is still notably absent from science classrooms around the world. We will take these as assumed starting points for the present study.
They also set out to study classroom interactions in small group and whole-class discussions. They conducted a classroom case study of an experienced teacher leading four lessons designed to develop 14–15 years old students' models of heat transfer and temperature change. They observed classes alternating between what they called dialogical (open discussion) and authoritative (teacher centered) passages of interaction. In other words, there is a “turning point” in the flow of discourse as the teacher brings together everyday and scientific views and makes an authoritative case for the scientific view by making a direct juxtaposition of everyday and scientific views. They stated: “We see a tension between authoritative and dialogic approaches as being an inevitable characteristic of meaning making interactions in the science classroom” (Scott et al., 2006, p. 606). We represent this idea in the top half of Figure 1 as a tension between two opposite approaches or teaching styles. An implication is that it may be difficult for a teacher to decide when to use each approach (For reasons of space, we do not present all aspects of their more complex framework, but focus on those relevant here). They see skillful transitions between dialogic and authoritative interactions as being fundamental to support meaningful learning of disciplinary knowledge. However, they point out that in many typical classrooms, dialogic discussion can tend “to fade out altogether” and teachers need strategies in order to prevent this.
Remaining Gaps
However, which teaching approach to use at different times often cannot be mapped out in advance by the teacher, since it depends on the interests and concerns of the students. Finding principles for doing this is one of the objectives of the present paper. Scott et al. (2006, p. 607) indicate that the skillful shifts in teaching approaches resonate with the principles of “productive disciplinary engagement” (Engle and Conant, 2002, p. 400-401), “accountable talk” (Resnick, 1999, p. 40), and “reflective discourse” (van Zee and Minstrell, 1997a, p. 209–210).
We value Scott et al.'s description of the need for both discussion and authoritative input as contrasting approaches to teaching. However, the gap between open discussion and authoritative approaches seems very wide, as represented in Figure 1. In this paper we ask whether there are other productive approaches in between these that may reduce that tension.
The lower half of Figure 1 also represents the other two tensions we identified above. Studies such as Clement (1993) and Minstrell and Kraus (2005) have documented useful ideas that students bring into the science classroom. But it is well known that opening the classroom to students' naive ideas in science can also lead to faulty alternative models. If not dealt with, these can conflict with the target models of a unit. We call this a Conceptual Dissonance Tension.
In addition, since discussions for scientific thinking can be time consuming, and with teachers under pressure to cover content, there can be a tension between pursuing science content goals and scientific thinking goals. We call this a Lesson Objective Tension. We will also ask how these other two tensions in Figure 1 can be dealt with.
Models in the History of Science
In order to discuss prior work on modeling in science classrooms, we will first say how we are using the term “model.” Campbell (1920) and Harré (1961) argued that scientists often think using theoretical explanatory models, such as neutrons, electro-magnetic waves, and fields that are a separate kind of hypothesis from empirical laws, and we will focus on using the term model in this way. Harré proposed that there are four types of knowledge used in science and placed them in a continuum from the more empirical toward the more theoretical: (1) Observations; (2) Empirical law hypotheses (mathematical and verbal descriptions of patterns in the observations); (3) Explanatory models; and (4) Formal principles. Explanatory models then are conjectured theoretical hypotheses providing a picture of a hidden description or process that explains why a pattern in observations occurred. Thus, an explanatory model is not simply a condensed summary of empirical observations but an invention of new theoretical terms and images that are not “implied by” the data. The scientist is free to generate such models via conjecture or analogies or other means, but to survive, the model also needs to be evaluated with respect to a number of criteria, such as empirical testing, simplicity, aesthetic appeal, and consistency with other accepted models.
Machamer et al. (2000) used the term “mechanisms” with a meaning similar to “explanatory models” and described how scientific disciplines are multi-layered (e.g., subatomic, atomic, molecular layers). This can apply to how educators organize a subject to be taught via hierarchical substructures. For example, the concept of blood can be modeled as blood cells and plasma. But one can go further to unpack the structure of blood cells into parts of the cell and their functions, which can be thought of as starting a new cycle of modeling at a lower level, producing nested layers of models.
Kuhn (1970) indicated that the advance of scientific knowledge is the result of a revolutionary process by which an older theory or paradigm is rejected and replaced by an incompatible new one. The process begins when the old paradigm cannot solve new problems and anomalies accumulate producing a crisis in the scientific community that turns to search for new paradigms. Kuhn argues that these paradigms compete until one survives because either most of the researchers are converted to the newer paradigm or are removed by attrition.
On the other hand, Toulmin (1972, p. 202–204) indicated that the advance of scientific knowledge is often the result of an evolutionary process that is comparable to Darwin's natural selection theory. The process involves two steps: selection and innovation. Starting from an existing model, the selection part involves a process of critical evaluation and debate about problems with the model, while the innovation part involves originating new conceptual modifications of the model while until leaving in place the best parts of the model. In this way science can evolve better and better models. This contrast between the views of Kuhn and Toulmin on learning in scientist raises the interesting question of whether scientific practices students engage in classrooms are better thought of as revolutionary or evolutionary.
Nersessian (1995, 2008) and Darden (1991) have conducted important historical studies of the modeling practices of experts in the domains of electro-magnetic theory and genetics, respectively. Clement (1989, 2008a) conducted think aloud studies of problem solving and modeling practices of modern scientists. All three found that experts use a variety of reasoning processes such as analogies, discrepant events, imagistic simulation, and thought experiments. They also found a larger pattern in which expert models can go through a series of successive refinements to produce a chain of better and better models. These are generated by cycles of model Generation, Evaluation and Modification (GEM) processes. Clement (1989, p. 347) used Figure 2 to describe this cyclical process of creative generation of a hidden structure or process to account for the phenomenon, evaluation that can be rationalistic and/or empirical, and modification or rejection (termed a GEM Cycle). As shown in the diagram when the evaluation of a hypothesis is strongly negative, it may be completely disconfirmed. But if it is evaluated only somewhat negatively, it can be improved through modification. Such a cycle can generate a series of successively improved models. We will attempt to use this concise summary of basic central practices in science as one starting point hinting at how one might interpret modeling discussions in classrooms.
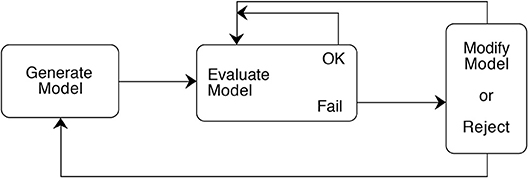
Figure 2. GEM cycle of model generation, evaluation, and modification (adapted with permission from Clement, 1989, p. 347).
Recent Emphases on Models in Education
Authors early on such as Hestenes (1987), White and Frederiksen (2000), and Clement and Steinberg (2002) have emphasized the importance of learning models and modeling as central to the learning of science in schools. In addition there is recent work urging teachers to develop qualitative models during discussions, e.g., Krajcik et al. (2014, p. 163); Louca et al. (2012, p. 1845–1847); Reiser et al. (2012, p. 6–7); Schwarz et al. (2009, p. 640–643), and Windschitl et al. (2012, p. 884–885). They have emphasized that if we want students to learn modeling as a practice, we need to find ways to involve them in generating models. Indeed in the USA, the NGSS (2013) standards now emphasize modeling as a key scientific practice objective for teaching students to do science as well as learning content. That is, it not only calls for students to learn models, but dozens of its performance expectations call on students to develop models.
We will use the term “model based curriculum” to refer to a curriculum that was designed to focus on students making contributions to the construction of explanatory models as a foundation for conceptual understanding. Although they are not yet widely adopted at a national level, there have now been a number of model-based curricula developed at elementary, secondary levels for different subjects, such as: electricity (Capacitor-Aided System for Teaching and Learning Electricity (CASTLE) Curriculum, Steinberg and Wainwright, 1993 and Clement and Steinberg, 2002); mechanics (Preconceptions in Mechanics, Clement, 1993 and Camp et al., 1994); particle theory (Children's Learning in Science (CLIS) Research Group at the University of Leeds in the UK, Driver and Scott, 1996); and life sciences (Energy in the Human Body Curriculum, EHBC, Rea-Ramirez, 1998 and Rea-Ramirez et al., 2004).
These curricula emphasize content learning via model construction and revision processes. They emphasize understanding the dynamic causal mechanisms, not just static structure, of qualitative explanatory models. However, from the teachers' and student's point of view these models are quite complex, and teaching and learning them can involve many conceptual steps. But all these curricula have the following common characteristics: (1) they begin from students' ideas and move toward a conceptual target; (2) instruction was conducted via both small and large group discussions; (3) large significant gains in students' understanding were measured from pre and post-tests; (5) the curricula were field tested in classrooms and revised over multiple years; (6) teachers contributed to the development and testing of the curricula.
Each of these curricula use a teaching sequence that contains up to six steps to support the modeling process but they each use different names for the steps. Clement (2008b) summarized broadly how these curricula use four common steps in supporting students' modeling, namely: Introducing Problems, Building Model Parts, Synthesis (Consolidation), and Application. These are candidates for organizing a high level sequence that may foster modeling processes through classroom interactions. However, these broad steps are still too rough for guiding teachers in moment-to-moment scaffolding of modeling practices. In particular, these four steps do not provide a detailed description of the modeling processes that are taking place within each section particularly within the Building Model Parts section which we consider to be the most challenging.
With respect to discussion leading strategies, van Zee and Minstrell (1997b), Hogan and Pressley (1997), and Chin (2007) have identified important moment-to-moment questioning strategies that teachers use to guide discussions. A few of these are cognitive strategies aimed at specific conceptual processes, but most are broader strategies designed to sustain dialog in general. One important role of discussions is to provide formative assessment feedback to the teacher Minstrell et al. (2011, p. 2–3). Other research groups have described modeling practices that appear to have a large time scale of 2–6 lessons (e.g., generating vs. consolidating ideas) (e.g., Driver and Scott, 1996, p. 99; Windschitl et al., 2012, p. 891 and Brewe, 2008, p. 1158) as well as smaller patterns that appear to occur in smaller 5–20 min segments in classrooms (e.g., using analogies, written records of discussion, and argumentation) (e.g., Hammer, 1995, p. 423–427 and Schwarz et al., 2009, p. 640–643).
Remaining Gaps, Purposes, and Plan for This Paper
While papers in the sections above have highlighted the importance of modeling and of drawing out and having students debate ideas in sustained discussions, they still have not provided a clear description of how students can engage in model construction as a teacher guides a discussion toward a target model, while navigating the tensions described in Figure 1. Some processes have been proposed, but we still lack ways of organizing them into a coherent “big picture” collection of (at least partially) ordered processes and subprocesses.
In the next section we will review some recent work on cognition in expert scientists as an important resource. We will be asking whether those descriptions apply in the classroom or not, and review some of our own group's previous work on this problem. We then formulate more specific research questions and attempt to apply these pieces along with ideas from other researchers to our case study of a series of classroom discussions.
Applying Expert Modeling Practices to Instruction
Clement (1989) and Nersessian (1995, 2008) argued that generation, evaluation and modification cycles are processes that need to take place in students who are learning to comprehend scientific models with conceptual understanding. But neither of these early studies provided guidelines for teachers and curriculum developers about how to guide instruction. In addition, they did not provide descriptions to explain how the processes of model construction and revision take place when multiple subjects are participating in the construction of the same mental model.
Clement (2000) proposed that for complex models too large or too unintuitive to learn all at once that it made sense for students to learn via GEM cycles in a sequence of steps from model Mn to model Mn+1 (see Figure 3). The emergence of successive intermediate mental models is also called a “learning pathway” (Scott, 1992, p. 221). A learning pathway can be envisioned as a chain starting on the left from common misconceptions and possible positive conceptions to build on, and progressing to the right toward a target model for the unit that is usually a simplified version of the expert consensus model. In between are intermediate models that may be model elements or partial approximations. These have the potential to provide a more fine-grained guide to the teacher concerning what pathways of learning can make sense to the student and lead to deeper conceptual understanding.
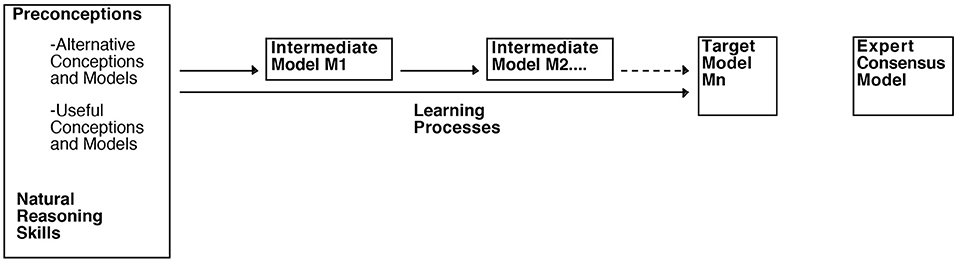
Figure 3. Model evolution sequence with intermediate models (reprinted with permission from Clement, 2000, p. 1042).
Findings From Our Own Group on Modeling Practices in the Classroom
GEM cycles leading to model evolution sequences like those in Figure 3 have been documented by our own group in classroom teaching in chemistry Khan (2008), middle school life sciences Nunez-Oviedo et al. (2002, 2008) and Clement and Steinberg (2002) did so for high school electricity tutoring. They called this “teaching via Model Evolution,” which meant fostering a series of “model criticisms and revisions” to parts of the students' models, often by using dissonance producing techniques and analogies. More recently, Williams and Clement (2015) described GEM cycles in high school physics discussions, and showed how they were supported by other smaller strategies like analogy and discrepant events.
Rea-Ramirez (1998) described the teacher's role as being constantly aware of the students' mental models so as to foster criticism and revision cycles. Thus, an important role of both small and whole class discussion is to allow the teacher to listen in deeply to students' points of view so that s/he is aware of student models. Co-construction is the process that occurs during the cooperative construction of a mental model through which the teacher and the students both contribute ideas to build and evaluate a model. Nunez-Oviedo (2004) developed models to explain the processes within co-construction.
Work on Developing a Multi-Level Modeling Framework
Based on the previous findings (Clement, 2008b) proposed the generation of a larger organizing framework called “Multiple Time Scale Levels of Organization” that includes modeling strategies used by experts as well as the common strategies found in curricula. The framework includes six levels that reflect different time scales, ranging from those strategies operating over months to those operating over seconds. Lower-level strategies are then nested within higher-level strategies as follows:
Level F Curriculum unit integration strategies,
Level E Unit-sized modeling strategies,
Level D Lesson strategies,
Level C Single model element strategies,
Level B Individual cognitive strategies,
Level A Dialogical strategies.
The present study maps most closely to levels D and E in his scheme and seeks to identify detailed structuring and substrategies for scaffolding processes within those.
In addition to GEM cycles producing model evolution, Nunez-Oviedo and Clement (2008) identified different long time scale Macro Processes called “Model Competition.” In Model Competition if different models generated by the students are compared, such as a single tube vs. double tube model of tubes leading down from the throat, the teacher or the students can make positive and/or critical or negative evaluations of each model, encouraging students to eventually confirm or disconfirm a model such as the single tube model.
Clement (2008a, 2017) described modeling processes in experts at different grain size levels. By analyzing data from videotaped protocols of experts thinking aloud about unfamiliar explanation problems he attempted a synthesis in including several levels of modeling practices, the most encompassing and highest of which are shown in Figure 4A. The upper level includes four, large time scale processes. These are supported by processes at the lower level that include several medium scale practices—GEM cycles and assessing competing models.
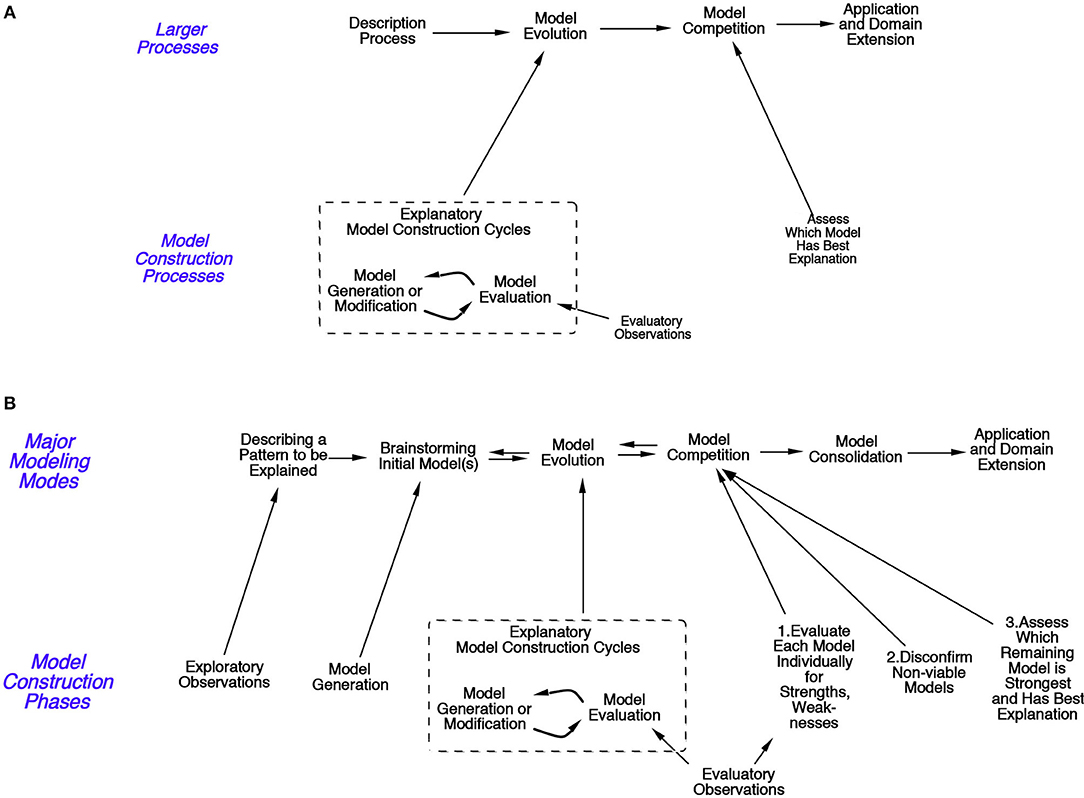
Figure 4. (A) Initial modeling practices framework from expert study showing two nested levels of processes. (B) Modeling practices framework for classrooms showing two nested levels of processes (Phases in lower level of (B) are shown here only for the first four Modes in the upper level. See transcript analysis for others).
In this study, we will use this Expert Framework as a departure point for thinking about cognitive processes involved in producing classroom model construction of the kind shown in Figure 3, by asking whether any of its elements can be seen in classroom whole class discussions. It may suggest initial hypotheses or concepts for what large scale and medium size scientific knowledge construction processes are taking place in the classroom. We will then reject, modify expand or add to elements of the initial framework where needed. Our final framework is shown in Figure 4B as an advanced organizer and will be discussed in the results section.
Questions Motivating This Study
Thus, many researchers have worked on pieces of this problem and the challenge for the present case study is to build from these studies to describe a coherent framework of multi-level processes involved in the teaching and learning of explanatory models along with modeling practices. If a viable framework is found, it should be able to describe repeated patterns of processes occurring over multiple lessons.
General Long Term Background Questions
The following three items are not in themselves specific research questions but they describe some general long-term motivations for our work (derived from the three tensions in Figure 1).
Opposing Teaching Approaches Question
Are Teachers limited to two opposing choices for discussion: Open Discussion vs. Authoritative? Or are there intermediate modes between these?
Conceptual Dissonance Question
Given the topic of this study, we are assuming the teacher has a goal of conceptual understanding for a concept in the form of a target model, and assuming student discussions for active learning are desirable. Can a class start from student-generated models that may conflict with the target model in a number of ways, and arrive at the target model through discussion? How? Won't students' faulty models interfere?
Lesson Objective Tension Question
Can a teacher guide or scaffold discussions to foster model construction (reasoning) practices, as a type of scientific thinking, at the same time that they are learning science content? How? (see Figure 1).
Specific Research Questions
Assuming we find a class with some or all of the characteristics listed above, we can ask more specific research questions for a case study of how such discussions evolve. Scott et al. (2006) focused on finding a sequence of sociocultural discourse patterns. To complement this, we ask, for a teacher experienced in fostering the learning of conceptual models in biology, what are the major cognitive processes involved in constructing a scientific target model within the social context of classroom discussions?
RQ1. Is there a pattern of large model construction Modes that occurs over a large time scale of 1–5 lessons?
RQ2. Is there a pattern of smaller model construction phases or processes that occurs over a medium sized time scale of 5–20 min cycles within lessons?
RQ3. If present, how are these patterns connected?
These questions are about: (1) generating new descriptions and hypotheses for teaching and learning processes; (2) providing existence demonstrations for several newly described types of discussion modes and phases. For this reason a descriptive case study is the method of choice.
Methods
Context
We conducted a video case study microanalysis of whole class discussions in a series of three classes taught by an experienced science teacher. The broader topic of the unit was “how the glucose goes to the cells through the blood stream.” During the lesson sequence, the 24 7th grade students examined the processes and structures that allow glucose absorption in the small intestine. The teacher had nearly 20 years of teaching experience, very good content domain and classroom management skills, and conducted her teaching by taking into account students' contributions. Students were organized into six groups or tables of four students. The teaching episodes took place almost at the end of the school year and consisted of three 45 min lessons that were videotaped and transcribed verbatim. We chose to look at a teacher who had strong content goals for the students learning particular models of the digestive system and also had goals for their doing scientific thinking.
The lessons were part of a model-based curriculum whose goal is to teach middle school students the function of major body systems by starting from their own conceptions. Examples of topics they had studied in previous chapters were: cellular respiration (Chapter V); the circulatory system (Chapter VI); and the respiratory system (Chapter VII). The teacher sometimes asked students to work individually or in pairs and write down or draw their ideas in the curriculum workbook or on their small group's shared whiteboard. At other times, students were asked to share, compare, draw, and discuss their ideas within their small group until reaching consensus. In whole class discussions the students shared and evaluated their ideas, often by displaying drawings they had made on their white boards, and these discussions are what we focus on here.
Data Analysis
Transcripts were analyzed to find large scale and medium scale reasoning patterns that were occurring in this three-lesson sequence by conducting a micro analysis of the teacher and students exchanges, including questions, answers, and drawings. To develop viable constructs for the processes taking place, we employed a construct development cycle (Miles and Huberman, 1994, p. 308) leading to the progressive refinement (Engle et al., 2007, p. 240) of hypotheses about modeling processes. This consisted of: (a) segmenting the transcript into meaningful teacher and student statements or turns, (b) making observations from a cluster of statements, (c) utilizing the framework in Figure 4A, where possible, to form a tentative classification of the process behind the statement (or if that failed, generating a tentative new construct), (d) examining the data to look for more confirming or disconfirming instances of the process, (e) criticizing and modifying or extending the hypothesized category to be consistent with, or differentiated from, other instances, and adding it to the framework; (f) returning to the data in (c) again to apply the modified construct, and so on. Because of the difficulty involved in studying a relatively unexplored area of large and medium sized complex processes with high inference coding, coding was done jointly by the two authors. Triangulation from (1) both analysts having to reach agreement and (2) from checks on the ability to use the same constructs across all three lessons and subtopics served to improve and support viability and validity.
Results: Case Study Findings
In Modeling Processes Identified we will first summarize the process modes and phases found at two levels that provide our main answers speaking to research questions 1 and 2. The section Transcript Analysis below presents detailed findings in the form of a transcript analysis according to the modes and phases identified. The section Findings by Research Question collects these together to give more general findings that speak to each of our three research questions.
Modeling Processes Identified
Our video transcript analysis yielded evidence for two levels of large and medium sized modeling processes, with the large processes operating over a longer time scale and the medium sized processes nested within the larger ones. The two levels are termed Major Modeling Modes and medium sized Model Construction Phases. Each level can be cyclical.
Table 1 shows six Major Modeling Modes at larger time scales that were identified. Four of these modes are similar to those from studies in expert reasoning shown in Figure 4A. Two other modes were found that were not observed in expert protocols yet but appear to be important steps in classroom modeling (see Figure 4B).
Table 2 shows six smaller Model Construction Phases or processes identified in the transcript analysis that can take place within some of the larger Modes above (especially within Model Evolution Mode).
For example within the Model Evolution Mode in Table 1, we will see the teacher and students engaging in the model Generation, Evaluation, and Modification phases in Table 2, as they try to improve or repair a model they have generated, and as it evolves toward the teacher's target model. While we have presented the two levels of processes in the tables above as an advanced organizer, each table is the culmination of a long process of transcript analysis, evaluation, and revision to arrive at stable categories that fit the protocol.
Transcript Analysis
In this section we use the above categories in presenting our case study microanalysis of a three-lesson sequence. We display the fit between the processes and the transcript episodes, showing how a small number of processes can be seen to underlie a relatively long and complex transcript sequence of statements by the teacher and students. Readers who wish to see a summary of the analysis as another advanced organizer can preview Figure 6. We are not displaying the teaching here as either a perfect example or a bad example; rather our purpose is to develop constructs to describe the processes she attempts to foster. Later we will discuss what we consider to be positive and negative elements of the discussion.
We first divided the transcript into 21 “topics,” numbered in the second column of Table 3. The third column contains a narrative of the dialogue with teacher and student quotations; the rather long transcript over three lessons is condensed for reasons of space. The fourth column and fifth columns show the Major Modeling Modes and the Model Construction Phases, respectively. The topics of the three lessons are grouped into six major parts.
Transcript Part 1—Pattern to Be Explained, and Brainstorming Initial Models Modes
In the first lesson, the teacher introduced the topic to the class and then asked the students, “How does the glucose go into the blood at the small intestine and then to the cell?” (Topic 1). We consider this question to be an example of fulfilling the Identifying a Pattern To Be Explained Mode because the students do not have a model of how the glucose is able to get into the blood through the intestinal wall (In other cases a series of exploratory observations might lead to a pattern of observations to be explained).
The teacher then asked the students to draw out their ideas individually for about 7 min and then asked the students to share their drawings in small group and come up with a team model (Topic 2). The teacher then called on the groups to share their ideas in whole class (Topic 3). During the students' presentations in this period the teacher did not evaluate drawings or encourage the students to do so. Through their presentations, students explained processes and used concepts such as villi and absorption but none of the six groups had a working model of the transfer of glucose to the blood at the small intestine. In other words, the students' ideas were still far from the target model of the lesson.
In Topics 2–3, we classified the activities as belonging to a mode called Brainstorming Initial Models that primarily involves the process of Model Generation. It is worth noting, that the students did not build the initial model in one step. Instead, the teacher asked the students to thinking individually, then to share their ideas at their small group, and finally each team presented their ideas in whole class.
Part 2—Evolution and Consolidation Modes
The next section is one of the most important but also challenging part of the lessons because it is where the teacher scaffolds the students' modeling processes as she moves toward the target model. The interaction pattern of the discussion shifts markedly here from an open discussion (brainstorming) pattern wherein ideas are not evaluated, to a pattern of Model Evolution wherein the teacher fosters model evaluation or modification, usually implicitly by hinting that models could be improved and then modified by the students.
The teacher focused on a small segment of the small intestine to foster student models and their evolution toward the target model (see Table 4; Students had also studied the idea previously that cells had semi-permeable membranes, and one student brings this up).
Beginning in Table 4 the teacher conducted a different large scale modeling process that we call Model Evolution Mode” (see Figures 4A,B) through which students' ideas evolve from “villi are like hands to grab nutrients” to villi “look like fingers”; villi “grab little bits of glucose like fingers”; to villi “are absorbing this glucose”; “maybe it is a kind of filter…”; to “villi's cells have semipermeable membrane that might act sort of a filter”; to “when it is bumpy there is like more space to absorb glucose?” to “villi increase the surface area to increase the amount of nutrients to be absorbed.” The evolution of these ideas was the result of four cycles of medium sized (medium time scale) processes shown on the column located on the far right side of the table: Model Generation, Model Evaluation, and Model Modification (We use “Medium Sized” because in future publications, we plan to also discuss even smaller reasoning processes). Referring back to Tables 1, 2, the reader can see that we so far have encountered the first three modes in Table 1 and the first four phases in Table 2.
Part 3—New Pattern to be Explained, Brainstorming, and Competition Modes
The teacher then went further and asked the students to conjecture and discuss in their teams about “where to locate the capillaries to make villi an efficient absorbing machine” (Topic 9 in Table 5) and then to draw their ideas on an overhead (Topic 10). We consider this a new modeling question at a different micro level of detail or grain size, and it starts a new pass through the Major Modeling Modes. The students disagreed about where to locate the capillaries (see models A, B, C in Figure 5A).
Here the teacher challenged the students to generate ideas by conducting new episodes of the Pattern to be Explained Mode and the Brainstorm Initial Model Mode at a new level of detail. However, none of the three models were close to her target model shown in Figure 5B. As result, the teacher scaffolded the students in evaluating their ideas. With three alternative models in play, she enters a Model Competition Mode.
The teacher asked the students “what is the least efficient, Model A, B, or C?” and the discussion proceeded as shown in Table 5, Topic 11. The teacher and the students collaborated in evaluating ideas, with the teacher guiding the discussion with questions. The class concluded that Model C was the best model (Topic 13). In Table 5 we show the class entering a Model “Competition” Mode where several alternative models are evaluated until one emerges as preferable (When working with teachers we call this a Model “Comparison” Mode instead to emphasize that the goal is a joint decision rather than to find a “winner”). This contrasts with the earlier Model Evolution Mode that worked with a single model and tried to improve it. As shown in the table, the Competition Mode was fostered by smaller processes of model Evaluation, Disconfirmation, and Confirmation.
Phases involved in the model competition mode
Figure 4B shows a picture of the modeling practices framework we are assembling. It shows two nested levels of processes allowing us to summarize steps in the Model Competition Mode as:
1. Evaluate each model individually for strengths, weaknesses
2. Disconfirm non-viable model
3. Assess which remaining model is strongest and has best explanation
In this case the last step 3 was not needed because there was only one model remaining.
How the Interaction Is Different From Recitation
Since there are many interactions in the transcript with turns of the form TST or TSST…(where T, teacher; S, student), one might ask whether the teacher is just doing recitation (called IRE by some, but see Nassaji and Wells (2000, p. 393) for different views) in these classes. We can define recitation as the teacher:
• asking for recall of prior “School Knowledge,” including “School Science knowledge,”
• getting a student response, and then,
• providing an immediate judgment on the correctness of the response.
There are some instances of recitation in the full transcript, especially where the teacher is trying to review what happened in the previous class, but most of the interactions are not recitation. The most obvious example of that is during Brainstorming Mode when the teacher is eliciting student models without evaluation. In addition, in many other sections, the teacher engages in TSTTST…exchanges where:
• instead of recall, the teacher is most often asking for students to reason in order to generate, evaluate, or modify a model;
• instead of an immediate judgment on correctness, she often asks them to participate in evaluating a proposed model. Even when she does indicate whether a model is correct, she is usually not just doing that, but giving a reason for her evaluation. For a positive evaluation she may state a reason or question that could add to or enhance the model. For a negative evaluation she gives a reason that explains important constraints on the modeling.
This has the atmosphere of the teacher helping the students reason to construct a visual model together rather than a “quiz game” of reciting memorized words. The repeated evaluations and modifications of student drawings add to this atmosphere of construction that appears to be very different from recitation. So in the transcript we appear to have a whole spectrum of interaction styles between the poles of brainstorming on one end and recitation and lecture on the other end. The teacher was able to adaptively change her interaction style as she moved through the different Modeling Modes.
We should also caution that the processes we describe here are not all necessarily conscious or articulated strategies for the teacher. With her years of experience, she is somehow able to intuitively scaffold student thinking in ways that led to the process patterns identified here, without her knowing or using terms like “Evolution Mode” or “Model Modification Phase.” Rather, those are constructs we have formulated to describe the patterns of reasoning that emerged from the discussions.
Part 4—Model Evolution Mode
In the next segment in Table 6, the teacher attempts to take the remaining Model C in Figure 5A and have students modify it to be closer to the target model by returning to Model Evolution Mode.
We infer that the teacher scaffolded the students in improving their shared Model C in Figure 5A toward the target model by returning to Model Evolution Mode. The student models evolved from “villi having dead ends” to “villi having loops” (Topic 15) and to “villi having loops and red and blue colors” (Topic 16). The red color indicated blood moving away from the heart. We also view the Evolution Mode as utilizing subprocesses—i.e., as being the result of three cycles of smaller scale Model Evaluation and Model Modification processes. This is shown in Figure 4B by the upward arrow pointing to Model Evolution.
Classroom dialogue diagram
The classroom dialogue diagram in Figure 6 was created to depict a summary picture of events in Topics 9 to 16 above. It shows the student and teacher contributions to co-constructing an explanatory model by starting from the students' ideas.
Williams and Clement (2015) describe such classroom dialogue diagrams as follows:
The diagrams are (abridged) horizontal versions of the transcript with student statements [above] and teacher statements [below], with time running from left to right. The horizontal strip across the middle of the diagram contains short written phrases to describe the evolving explanatory model. These phrases represent our hypotheses for teacher's conception of what a student's addition to the model was at a given point in the discussion…It was assumed that the teacher was aiming to foster model construction based on their view of the students' model at that time, and how it differed from the target model… arrows that point from both teacher and student statements toward the explanatory model descriptions in the center strip indicate their shared contributions to the changes or additions in the models (p. 13).
The transcript in this diagram is highly abbreviated. The symbol AX indicates Model A was disconfirmed. The figure highlights three aspects of our analysis of model construction processes:
(1) The arrows in the diagram provide a picture of how statements from both the teacher and the students contribute to the model co-construction process taking place from left to right in row 3.
(2) It illustrates discussion modes of Model Competition and Model Evolution that are intermediate between purely open discussion and pure lecture.
(3) The bottom two rows depict processes that we hypothesize are simultaneously occuring in parallel during instruction. It shows the large scale modes (5) operating over longer time scales than the smaller scale phases (6), and each smaller scale phase contributing as a subprocess to the large scale mode above it.
The overall pattern that set up the conditions for these intermediate discussion modes was:
• After a Pattern to be Explained has been identified, the teacher elicits model ideas proposed by the students. These will naturally include flaws and gaps from the scientific target point of view;
• The teacher assesses the proposed students' ideas on the fly to determine their distance from the scientific model. In other words, the teacher needs to determine what the students' ideas are that need to be discarded and what ideas they can build on and modify to reach the target;
• The teacher scaffolds the students in examining and discarding those students' ideas that are farthest from the scientific model. We described this as a Competition Mode that was fostered by evaluation processes—that disconfirmed Models A and B;
• The teacher scaffolds the students in improving or repairing the most promising ideas until they are close to the scientific model. The transcript provides evidence that the teacher worked in a Model Evolution Mode that was comprised of two GEM modeling cycles to repair Model C until it was very close to the target model.
• We believe that the sequence in Topics 9–16 above is not atypical: the Model Competition mode ended with one surviving model, but that model still needed improvement via the Model Evolution mode.
Part 5—Consolidation Mode
Due to space limitiations, we do not include later phases of the instruction in the classroom dialogue diagram. But we will describe them here. In what we call Model Consolidation Mode, the teacher then showed the students four transparencies (see Table 7) that contained the scientific version of the target model, with connections from the vessels in each villus to a common artery and vein. The teacher also asked the students to go back to their initial drawing and asked them to compare it with what they had learned and change in case it was necessary (Topic 18).
In the first segment, the teacher showed the students the scientific model as an authority by using transparencies and then asked the students to compare what she was showing them with their ideas. We call this process “Consolidating the Scientific Model” (Topic 17). In the second segment, the teacher asked the students to compare the scientific model with their initial drawings and asked them to modify them if necessary to explain exactly how sucrose transfer occurs. We call this process “Explaining the Original Pattern” (Topic 18). During this time we infer that there were also smaller, Model Construction Phases occurring, in particular, Model Evaluation and Model Modification. We note that as the students get nearer to the target model, the teacher has become more proactive in evaluating their models, and suggesting directions for their modification. But she does not tell them exactly how to draw the modifications.
Part 6—Application Mode
The teacher then asked the students to apply their new understanding to an entirely different subject, the respiratory system in what we call Model Application and/or Domain Extension Mode. She asked them “if lungs are another site of exchange, what they might have?” (see Table 8).
In this part of the lesson, the teacher asked the students to transfer part of their understanding of villi as a site of exchange to alveoli at the lungs. We hypothesize that a Model Application activity may not only be a shortcut as a starting point for building the new alveoli model, but also may be another way of exercising and consolidating the model of the villi that they have just learned. However, the teacher was running out of time at this point and was actually only able to introduce the topic of gas transfer to the students in this brief segment, but it did serve to exercise the model they had just learned. In other cases where the application is not as big a leap as going from intestines to lungs (e.g., application to transfer of other substances besides glucose via the villi), the Application Mode may serve to simply extend the domain of application of the model they have just learned.
Findings by Research Question
Figure 4B summarizes the modeling processes identified at two major levels in our case study. It is somewhat surprising that processes derived from studies of sophisticated experts working on physics problems (in Figure 4A) could have parallels with some of the learning processes in a 7th grade life sciences classroom (in Figure 4B). This adds authenticity to the idea that the students were contributing to some real scientific reasoning practices.
Research Question (1): Is There a Pattern of Large Model Construction Strategies That Occurs Over a Large Time Scale of 1–5 Lessons?
We found six large modes of model-based teaching within these lessons, called Major Modeling Modes, described in Table 1 of the Results section which is our main answer to this question. The top level of Figure 4B also summarizes the Major Modeling Modes identified in the case study. Describing a Pattern to be Explained Mode is the starting point for modeling there.
It was of interest to us to see the different styles of interaction occurring in each of the subsequent modes. The Brainstorming Initial Models Mode was characterized by divergent open discussion with the teacher preventing evaluation of the models, in contrast to ensuing modes. Model Competition Mode served to evaluate and disconfirm the least viable student models and the Model Evolution Mode served to repair the most promising models in a sequence of progressive refinements. Model Competition and Model Evolution Modes were characterized by the teacher's efforts to foster student contributions to those evaluation and repair processes as well as adding some herself. We call this interaction style teacher-student co-construction. As illustrated in Figure 6, it yielded many ideas and inferences that were student-generated as well as some that were teacher-generated. These two modes were the most lengthily ones in these discussions.
Near the right side of Figure 4B, Model Consolidation Mode was characterized by a very different mini-lecture style that was the most convergent style, followed by final model modifications in student drawings. By convergent (as opposed to divergent) we mean that the number of models and the distance of the models under discussion from the target model was getting smaller. The Application and/or Domain Extension Modes were a mixture of co-construction and mini-lecture styles. Thus, a spectrum of styles was seen within these large scale modes, from student-generated ideas in open discussion, to teacher-generated ideas in mini-lectures, with a co-construction style of shared idea generation in between. These styles appeared to fall on a spectrum running from divergent to convergent. This resonates with others who have identified the need for both divergent and convergent discussions (see Scott et al., 2006 and Windschitl et al., 2012). The balancing of divergent and convergent thinking is also a hallmark of model construction work for scientists Clement (2008a). (See Lehesvuori et al., 2013 for contrasting case studies of two teachers who each moved in opposite directions between convergent and divergent styles).
The Mode sequence was cyclical, with the first round passing through the first three modes in the upper level of Figure 4B plus Model Consolidation, and the second round restarting with a new Pattern to be Explained in Topic 9 in Table 5 and passing through all six modes. This reflects the view that that scientific models are nested (Machamer et al., 2000). The double arrows in the upper level of Figure 4B indicate that Model Evolution and Model Competition might occur in a different order, or even alternate, depending on when new models occur to students. So the upper level is intended to portray a loosely ordered sequence.
Research Question (2) Is There a Pattern of Smaller Model Construction Phases or Processes That Occurs Over a Medium Sized Time Scale of 5–20 min Cycles Within Lessons?
We found such a pattern that occurred repeatedly in this case study shown as Model Construction Phases in the lower level of Figure 4B and defined in Table 2. The three most frequent processes were Model Generation, Model Evaluation, and Model Modification (GEM), and these participated in another smaller GEM cycle within Model Evolution, although they could also occur as individual processes. We refer to repeated GEM cycles as a model evolution process capable of producing a sequence of more and more adequate models (Figure 3).
In addition, as shown in Figure 4B, Evaluatory Observation was proposed as a subprocess that could implement an Evaluation Phase, and Exploratory Observation was identified as a subprocess that could lead initially to a Pattern to be Explained, although the latter process did not appear in this particular case study.
Research Question (3) If Present How Are the Above Patterns Connected?
Another pattern in the transcript analysis is that the smaller time scale Model Construction Phases are nested within the larger Major Modeling Mode processes; the small phases are subprocesses that contribute to the purpose of the larger process, as shown in Figures 4B, 6. For example we have found that individual Generation, Evaluation, and Modification processes are utilized within both the Model Evolution Mode and the Model Competition Mode. The Evolution mode utilizes all three GEM processes, while the Competition Mode was seen to utilize mostly the Model Evaluation process applied to several different models, leading to some models being disconfirmed while others being confirmed (see Table 2). In contrast, the Model Evolution mode is the focus on a single partially correct model that is modified by the GEM cycle pattern (hence the name Model Evolution mode).
Rows 5 and 6 of Figure 6 show the two levels of processes operating in parallel, with the large scale modes (row 5) operating over longer time scales than the smaller scale phases (row 6), and each smaller scale phase contributing as a subprocess to the large scale mode above it. For example, if a teacher had evolution toward the target model as a goal during Model Evolution Mode, they could keep that goal in mind as they fostered repeated subprocesses of model Evaluation and Modification.
Discussion
Connections to Previous Literature
Our general objective in this study was to describe a coherent framework of multi-level processes involved in the teaching and learning of explanatory models. The sequence of six Major Modes in the top row of Figure 4B shares several individual processes with those described by other researchers such as: Driver and Scott (1996, p. 99); Minstrell et al. (2011, p. 4); Driver and Scott (1996, p. 613); Windschitl et al. (2012, p. 887) and Campbell et al. (2012). The article by Driver and Scott (1996, p. 99) was particularly pioneering in anticipating several of the major modes described here at an early date. We have attempted to add clarity to options within the Identifying a Pattern to be Explained Mode, as well as to separating two levels of processes and especially adding new modes that we call Evolution and Competition Modes. Based on studies of the history of science reviewed earlier, we consider Model Evolution and Competition to be central and essential to active engagement in modeling. We will describe these additions to theory in more detail, moving from left to right in Figure 4B in what follows.
Describing a Pattern to be Explained is the first Mode there. Although identifying a major question for modeling in the unit is a mode that others such as Windschitl et al. (2012) have identified, a new feature to us was to include the possibility that its departure point was sometimes an already learned model rather than a pattern in observations. In this case students who had a model of glucose somehow going to the bloodstream from the small intestine were asked to open up the deeper level question of how that transfer takes place– as a pattern to be explained. The explanation was provided by generating a new model at that deeper level.
In this study we focused on the “big picture” of all six modes, but the interested reader can find other case studies focused on individual modes of Model Competition in Nunez-Oviedo and Clement (2008) and Model Evolution in Nunez-Oviedo et al. (2008). These two modes have a very rough but interesting analogy to, respectively, the revolutionary (Kuhnian) and evolutionary views of science discussed earlier. The analogy is weak here because none of the models in the present case study are persistent enough to act like a resilient mini-paradigm, but the analogy would be closer for a persistent student model such as impetus-like ideas in physics (Even there, the analogy is controversial, but we think still interesting; see Smith et al., 1994, Clement, 2013, and Lattery, 2016).
The bottom row of Figure 4B shows the structure of the smaller model construction processes being used within several of the modes above them as the teacher scaffolds student thinking. These findings complement those of authors such as Hestenes (1987, p. 443); Minstrell and Kraus (2005, p. 480; 484; p. 489-491); Schwarz et al. (2009, p. 635); and Windschitl et al. (2012, p. 887) who have described students generating models and discussing them, accompanied by multiple revisions and teacher scaffolding. Here we have attempted to dissect the concept of “scaffolding” further to describe in some detail the nature of the cognitive processes being scaffolded for model development. And a distinctive feature that we have not seen discussed by other groups is the idea of different time scale levels of connected strategies for scaffolding these processes Clement (2008a,b); and Williams and Clement (2015).
We also observed that the Model Generation phase occurred less often than the model Evaluation and model Modification phases in the case study. We explain this by saying that once the teacher supports the students in generating an explanatory model that contains several elements, the teacher repeatedly guides the students in evaluating and modifying each one of the elements of the explanatory model until it gets close to the target model.
Regarding Our General Long Term Background Questions
On the first page of this article, we listed three tensions that we believe teachers face when drawing out students' ideas in whole class discussions, summarized in Figure 1. These were related to three long-term questions that challenge us.
1. Opposing Approaches Tension Are Teachers limited to two opposing choices for discussion represented in Figure 1: Open Discussion vs. Authoritative Lecture [described by Scott et al. (2006) as the tension between dialogic and authoritative discourse]. Or are there intermediate modes between these?
We did find that the teacher used open discussions (in Generation of Initial Models Mode) near the beginning of the sequence and some authoritative discussions (in Model Consolidation Mode) near the end. However, we observed that this teacher conducted other two discussions modes with interaction styles that do not fit this dichotomy. In particular, as shown in Figure 7, Model Competition and Model Evolution modes involve scaffolding on the part of the teacher that is somewhere in between these open and authoritative approaches. We have described these two newly identified modes as the core of a teaching approach that we call “guided co-construction.” As a result, we believe that the present approach may involve a longer delay of closure than in Scott et al.'s case. But the intermediate approach there is not just a simple blend mixing open student discussion and teacher lecturing. As shown in the box between these in Figure 7, and under Competition and Evolution in Figure 4B, we have tried to unpack an impressive set of nested processes that the teacher is supporting through a particular kind of scaffolding we call cognitive scaffolding.
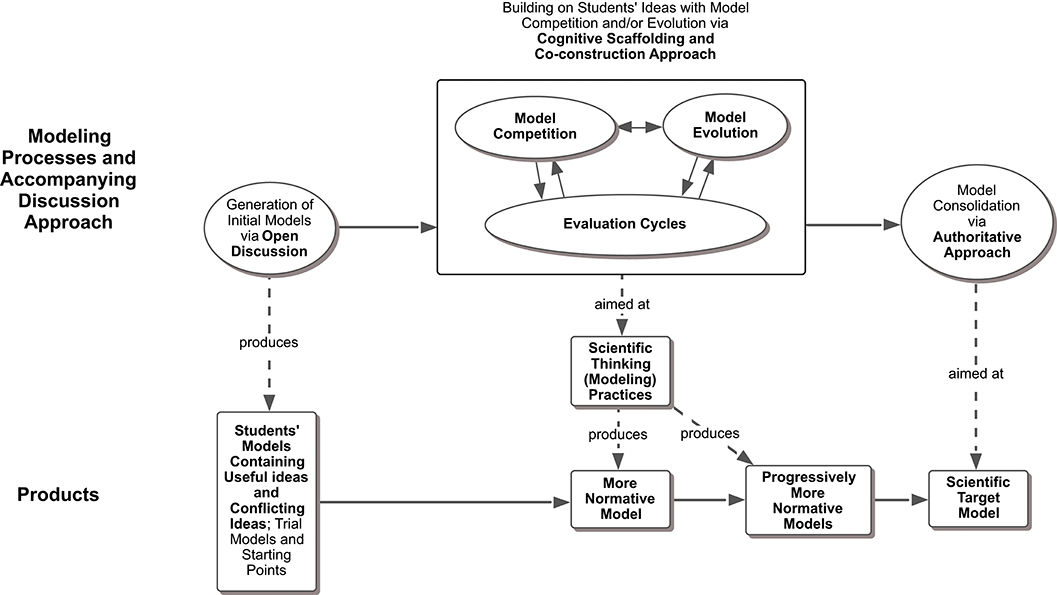
Figure 7. Cognitive scaffolding of student modeling helps resolve tensions shown in Figure 1.
In order to first say what we do not mean by cognitive scaffolding, researchers such as van Zee and Minstrell (1997b) and Williams and Clement (2015) have described less content specific and less cognitive forms of teacher support for participation in discussions such as paraphrasing student statements for clarity, using wait time, probing for clarification, and providing norms for respectful discussion. These are important general strategies for keeping any discussion going and fostering participation. In contrast to those moves we can define cognitive scaffolding as including moves that foster, guide, or support students' content-specific reasoning or idea formation about the topic of the lesson. This kind of scaffolded reasoning can take place for example through specific teacher questioning that involves students in doing model evaluation and modification. The goal is to provide just enough support to keep students in a zone where they are able to participate in the reasoning processes (here model construction). This is a broad definition of “scaffolding,” because it does not include the idea of withdrawing support gradually, which is sometimes included, but not as relevant in this study over such a short time period. Scott (1998, 68–72) theorized that effective scaffolding involves a feedback loop that contains three steps (1) analyze the learner's situation; (2) assist the learner by using pedagogical means; (3) monitor the learner's progress. The present paper focuses on unpacking step (2).
Even within the Evolution and Competition Modes, cognitive scaffolding can vary from strong to weak. Some teacher statements classified as scaffolding model evaluation in Table 4 for example are quite subtle, sometimes merely repeating a student's words in what van Zee and Minstrell (1997b) called a “reflective toss,” and leaving most of the evaluation and modification process to be done by the student (e.g., Topics 5 to 8). Whereas in topic 15 in Table 6, much more, but not all, of the reasoning in the evaluation and modification phases is done by or strongly hinted at by the teacher. Williams and Clement (2019) found that successful teachers can differ strongly as to the ratio of teacher initiated to student initiated modeling moves (e.g., model generation, modification, or evaluation). Thus we can identify a spectrum of approaches from open inquiry, to scaffolded inquiry with various degrees of cognitive scaffolding, to recitation, to lecture. This spectrum of approaches along with the analysis of purposes and substrategies for each may help mitigate the dilemma of having only two opposite approaches and not knowing when to switch, as depicted in Figures 1, 7.
2. Conceptual Dissonance Tension Can a class elicit student-generated models that may conflict with the target model in a number of ways, and arrive at the target model through discussion? How? Won't students' faulty models interfere? (see Figure 1).
Once the teacher opens up the classroom to student generated models and ideas, it is true that a divergent variety of models can emerge. This is uncomfortable for many teachers. In this case study the students generated at least three models that conflicted with the scientific target model. However, two of them were disconfirmed with reasoned plausibility arguments, some drawn from the student and some from the teacher. Then evaluation and revision cycles evolved the third model until it was close to the scientific target concept. As shown at the bottom of Figure 7, such a sequence of progressively more normative models can bridge the gap between initial ideas and a scientific target model. Importantly this should allow the student to use some elements of their prior knowledge as meaningful building blocks, while disconfirming other elements as not relevant. We hypothesize that this grounding in prior knowledge may have advantages for imageability, meaningfulness, and memorability (Ausubel, 1968). In summary, the teacher did not consider these students' incorrect models as interfering with their learning. Instead, she engaged students in reasoning about why some were less viable and used others as a stepping stone to build toward the target. This is quite different than just juxtaposing them with the scientific target model in a lecture.
3. Lesson Objective Tension Can a class foster model construction (reasoning) practices, as a type of scientific thinking, at the same time that they are learning science content? How? (see Figure 1).
In the USA, despite calls from NGSS to integrate the teaching of disciplinary core ideas, practices, and cross-cutting concepts, many teachers still think about content goals separately from scientific thinking goals. The teacher in this study, like many teachers, also had a goal of engaging students in scientific thinking practices. However, given time pressures, such a goal is often seen as in conflict with content goals and may be neglected. But as shown in Figure 7, in these lessons the teacher appeared to foster scientific modeling practices as a means to arrive at the target model, a content goal. By starting from expert modeling practices in this study we have seen how this teacher scaffolded basic science practices while simultaneously guiding students toward the target model. This gave the students experience with the ideas that models can be invented, can compete, can be disconfirmed, can be evaluated and modified by asking challenging questions, can be confirmed, and can be transferred to new contexts. Here, the method of learning content was scientific thinking.
Limitations
Figure 4B is a simplified representation of sequences (horizontal arrows) and subprocesses (diagonal arrows) that we have found. However, there are certainly variations and exceptions to the sequence, as is partly indicated by the double horizontal arrows there. As we saw in Topics 1–8, the teacher may not complete every mode in a sequence before starting a new sequence.
We would describe many of the teacher's actions during the competition and evolution modes as “moderately strong scaffolding.” There are certainly other approaches that could have been taken here, some with stronger directness and some with less. Which produce more content learning and which produce more learning of scientific thinking are important issues that need further research.
This paper's focus is on the qualitative objective of unpacking and sorting out connections between two nested levels of processes occurring in classroom discussions. It does not consider the measurement of gains in comprehension. One study of very similar types of scaffolded discussions in high school physics, which fostered GEM cycles, did measure significant gain differences over controls in comprehension with a large effect size (Williams and Clement, 2015). But it did not identify connections to higher level Mode processes as we have done in this study.
We are also interested in finer-grained levels of processes below those shown in Figure 4B, but that is a large topic on its own for another paper. For example, the figure shows “Evaluatory Observations” as a process contributing to model evaluation, but there are other subsidiary processes for model evaluation such as thought experiments and coherence criteria (see Williams and Clement, 2015).
We should note that we did not find evidence of deep seated, persistent alternative conceptions in this case study. Units dealing with such conceptions will need to use multiple methods and revisit them over a longer time period, sometimes much longer (see Clement and Steinberg, 2002; Kalman and Lattery, 2018, 1; Lattery, 2016; Minstrell and Kraus, 2005).
Instructional Implications
Positive Features of the Classroom Discussions
There were several positive features of the classroom dialog diagram analyzed:
(1) This was an advanced topic for 12 year olds, but students participated in a co-construction process, where they contributed ideas along with the teacher. Perhaps the most radical feature was that almost all of the initial model generation ideas came from the students before receiving instruction on them. Both the teacher and the students contributed to modifying ideas (see also Williams and Clement, 2019). Most model evaluations were initiated by teacher questions, with students contributing to the reasoning especially in the first two thirds of the transcript. The student contributions are key for their experiencing scientific modeling practices while learning about a scientific subject, and a source of any benefits from active learning.
(2) Evidence for student engagement in these lessons was provided by the large number of on topic contributions, the variety of models generated by the students and the inventiveness some of them display. Students' ideas such as placing the capillaries with respect to villi into three different positions (outside of villi, on top of villi, and inside of villi), illustrate the variety. In addition, some were quite inventive, e.g., that “villi looked like hands that grab things,” that “villi were like filters,” and transferring the concept of semi-permeable membrane from cellular exchange in other parts of the body to the villi. There is wide agreement on the importance of engagement (see Resnick, 1999; Engle and Conant, 2002; van Zee and Minstrell, 1997a).
(3) We also see this teacher as doing “responsive teaching.” The teacher skillfully navigated the class through all of the Modes shown in Figure 4B, as scientific practices, by appropriately using individual, small group, and whole class work. The teacher appeared to order the large scale modes of teaching depending on the topic, and the spontaneous models generated by students. For example, when students generated a wider variety of models, she fostered model comparisons (Competition). In addition she appeared to scaffold the lower level model construction phases in Figure 4B repeatedly. Since these are shorter processes, they require faster teacher decisions depending on what the students said.
Negative Features of the Classroom Discussions
There were also some negative features in our view: (1) many of the student answers to the teacher's questions were short. This meant that their opportunities for expression were not as great as they could have been. No doubt the teacher had a tradeoff with time on her mind. (2) In the last class the teacher was definitely running out of time. This meant that near the end she did not foster as many student contributions as she might have done; (3) the last section in Model Application and/or Domain Extension Mode was consequently quite short, and although students made a connection to the new topic of gas transfer in the lungs, we assume that this was too brief a segment for most to develop deep conceptual understanding of that area.
Time is unfortunately scarce in today's classrooms. Model based learning can take longer than lecture-based approaches. Resulting increases in conceptual understanding should save time later, but teachers under real institutional pressure to cover wide content may need to “pick their fights” in choosing which content areas they think are most valuable for significant student modeling practices. Driver and Scott (1996, P. 624) suggested prioritizing interactive ways of teaching when detecting strong differences or gaps between students' initial ideas and the scientific model, in which case stepwise model construction should be even more important for understanding. One tactic we have observed at the college level is to do modeling activities in small and large group in class but then assign readings and problem solving for the Consolidation and Application Modes. More innovation and research work is needed here.
General Instructional Implications for Teacher Education
In addition to being a lens for lesson microanalysis, we can consider whether the process patterns we have identified suggest a set of model development strategies for teachers in teacher education courses. There are many teachers who do not conduct the kind and level of responsive teaching we saw in the present protocol. For those who want to learn to incorporate students' ideas, once student generated models have been admitted into classroom discussions, the teacher can be unsure of how to deal with the divergent variety of student ideas, and there is a need to have some strategies/guidelines for thinking about how to scaffold further modeling. We hypothesize that these teachers might learn to use the six Major Modeling Modes and their nested Model Construction (GEM) Processes in Figure 4B as large and medium scale strategies for scaffolding modeling. Although the items in that figure were described as student processes that were fostered in the classroom, each process can also be seen to identify a corresponding teaching strategy of scaffolding that particular process.
The larger time scale Major Modeling Modes there could provide an organization for design at a unit level as a “modeling sequence pattern.” As we saw, this modes sequence can be repeated in a cycle for each major piece of a model in a unit. That would allow students to gain experience with modeling practices across different contexts. At the lesson design level, projecting from the framework in Figure 4B and the examples in the case study we can speculate that the different modeling modes may benefit from different styles of discussion leading. The Generating Initial Model(s) Mode would appear to benefit from an open style. The teacher used individual, small group, and whole class discussion formats for this (with the teacher mainly restricted to drawing out, rather than evaluating, ideas). The Competition Mode required more scaffolding, with the teacher clarifying the differences between models and prompting students to evaluate the different models. The teacher used both small group and whole class discussion for this. Model Evolution Mode required perhaps the most scaffolding and the most skill on the part of the teacher because the teacher will need to creatively figure out how to evolve certain models toward the target model through questioning. This may best be done in whole class discussion. Thus, it should be possible to use the framework for unit and lesson design, and as a guide to using different teaching styles at different times. The distinction between the Brainstorming Mode and subsequent Model Evolution or Model Competition Modes is important, because teachers using this approach could then learn first to withhold judgements and hints and the providing of correct answers in Brainstorming Mode. Only after practicing that would they build on it to add the more difficult skills of scaffolding Model Evolution or Competition.
However, the strategy sequence in Figure 4B is nothing like a full algorithm; a teacher who bravely opens the floor to discussion can receive many student ideas with varying degrees of distance from the target model, and they will have to make decisions about which to take up and in what order. This is part of the art of responsive discussion leading and it is a skill that takes a long time to learn. We believe it would need to be learned slowly, ideally with a support group in an in-service course. Scaffolding strategies would need to be simplified and introduced one major piece or level at a time (see Price et al., 2017; Krajcik and Merrit (2012, 11–12); Williams and Clement, 2015; and Stephens and Clement, 2010).
Near the end of the sequence, in Consolidation Mode, the teacher consolidated and confirmed the target model for the class using mostly a mini-lecture style. But why, one may ask, make the effort to elicit and work with students' ideas if the teacher is going to present and confirm the target model at the end anyway? Isn't this inefficient? Certainly one important reason to do so is to pursue scientific thinking goals in addition to content goals. But even for content goals, the potential of the present method for fostering deeper conceptual understanding would seem to lie in eliciting students' ideas and engaging them in thinking, allowing them to build on their connections to prior knowledge, to talk about and evaluate how various models function dynamically and experience cognitive dissonance with some of them, to build difficult models more slowly with understanding, and to see why certain models are better than others. On the other hand, lectures can sometimes be very inefficient, either if the concepts are presented too quickly because the teacher does not have feedback from student discussion, or if the students do not discuss and make sense of the given information, or if they do not engage in active learning with the ideas.
We can speculate that the strategies in Figure 4B may apply to fields outside of science education. For example, historians generate and revise models. And the general strategies for designing a scientific model should not be far removed from those for designing systems in engineering, although some of the criteria for evaluation may be different, and the mode sequence would start from a problem to be solved, rather than a pattern to be explained.
Conclusion
We began this article by reviewing previous work identifying many individual processes involved in scientific modeling in classrooms, with a focus on whole class discussions. However, this work still lacked an overall coherent framework for how these processes fit together. Imposing the constraints of accounting for each episode in the microanalysis of a case study in a real classroom allowed us to identify a coherent set of modeling practices at two nested levels, summarized in abbreviated form by the scheme shown in Figure 4B. Most of those processes are similar to those found in recent studies of the modeling practices of expert scientists. Each process in the Framework can also be viewed as designating a strategy for scaffolding modeling.
Figure 6 shows the two levels of processes operating in parallel in a classroom interaction style we call teacher-student co-construction. Figure 7 indicates how the framework strategies have the potential to remove or reduce the tensions described in Figure 1- tensions felt, we believe, by any teacher beginning to open up their classrooms to real modeling discussions:
• A teacher need not be limited to the two opposing interaction styles of Open Discussion vs. Authoritative lecture. Rather, there are there intermediate discussion styles between these that involve co-construction and cognitive scaffolding.
• It is possible to start from student-generated models that conflict with the target model in a number of ways, and still arrive at a target model in a discussion. Processes of model competition and disconfirmation, as well as model evolution, both supported by the teacher's cognitive scaffolding, were central in this accomplishment in the case study.
• In doing so, it is possible for a teacher to foster student modeling practices, as a type of scientific thinking, at the same time that they are teaching science content.
This study is intended as a starting point for developing a more adequate picture of the modeling practices and scaffolding strategies involved in discussions for learning science. We look forward to evaluating and modifying elements of the theory as more studies are completed by ourselves and others.
Data Availability
The datasets generated for this study are available on request to the corresponding author.
Ethics Statement
Human Subjects Statement: This study was carried out in accordance with the recommendations of the Human Subjects Guidelines, Human Research Protection Office, University of Massachusetts. The protocol was approved by the University of Massachusetts, Amherst, Institutional Review Board. All subjects gave written informed consent in accordance with the Declaration of Helsinki.
Author Contributions
MN-O and JC contributed to the conception, design of the study and analyzed the data. MN-O organized the database and wrote the first draft of the manuscript. JC wrote sections of the manuscript. All authors contributed to manuscript revision, read, and approved the submitted version.
Funding
This material is based upon work supported by the U.S. National Science Foundation under Grants DRL-1503456, JC PI. Any opinions, findings, conclusions or recommendations expressed in this paper are those of the authors and do not necessarily reflect the views of the National Science Foundation.
Conflict of Interest Statement
The authors declare that the research was conducted in the absence of any commercial or financial relationships that could be construed as a potential conflict of interest.
References
Ausubel, D. (1968). Educational Psychology: A Cognitive View. New York, NY: Holt, Rinehart and Winston.
Brewe, E. (2008). Modeling theory applied: modeling Instruction in introductory physics. Am. J. Phys. 76, 1155–1160. doi: 10.1119/1.2983148
Camp, C. W., Clement, J., Brown, D., Gonzalez, K., Kudukey, J., Minstrell, J., et al. (1994). Preconceptions in Mechanics: Lessons Dealing With Conceptual Difficulties, 2nd Edn. College Park, MD: American Association of Physics Teachers.
Campbell, N. R. (1920). Physics. The Elements. Cambridge: Cambridge University Press; Republished in 1957 as The foundation of science. New York, NY: Dover.
Campbell, T., Oh, P. S., and Neilson, D. (2012). Discursive modes and their pedagogical functions in Model-Based Inquiry (MBI) classrooms. Int. J. Sci. Educ. 34, 2393–2419. doi: 10.1080/09500693.2012.704552
Chin, C. (2007). Teacher questioning in science classrooms: approaches that stimulate productive thinking. J. Res. Sci. Teach. 44, 815–843. doi: 10.1002/tea.20171
Clement, J. (1989). “Learning via model construction and criticism. Protocol evidence on sources of creativity in science,” in Handbook of Creativity: Assessment, Theory And Research, eds G. Glover, R. Ronning, and C. Reynolds (New York, NY: Plenum), 341–381. doi: 10.1007/978-1-4757-5356-1_20
Clement, J. (1993). Using bridging analogies and anchoring intuitions to deal with students' preconceptions in physics. J. Res. Sci. Teach. 30, 1241–1257. doi: 10.1002/tea.3660301007
Clement, J. (2000). Model based learning as a key research area for science education. Int. J. Sci. Educ. 22, 1041–1053. doi: 10.1080/095006900416901
Clement, J. (2008a). Creative Model Construction in Scientists and Students. The Role of Imagery, Analogy, and Mental Simulation. (Netherlands: Springer). doi: 10.1007/978-1-4020-6712-9
Clement, J. (2008b). “Six levels of organization for curriculum design and teaching,” in Model Based Learning and Instruction in Science, eds J. Clement and M. A. Rea-Ramirez (Dordrecht: Springer), 260–277. doi: 10.1007/978-1-4020-6494-4_14
Clement, J. (2013). “Roles for explanatory models and analogies in conceptual change,” in International Handbook of Research on Conceptual Change, ed S. Vosniadou (New York, NY: Routledge), 412–446. doi: 10.4324/9780203154472.ch22
Clement, J. (2017). Four levels of scientific modeling practices in expert learning. Paper presented at the National Association for Research in Science Teaching (NARST), San Antonio, TX. Available online at: https://cadrek12.org/resources/four-levels-scientific-modeling-practices-expert-learning (accessesd June 27, 2017).
Clement, J., and Steinberg, M. S. (2002). Step-wise evolution of mental models of electric c ircuits: a learning-aloud case study. J. Learn. Sci. 11, 389–452. doi: 10.1207/S15327809JLS1104_1
Darden, L. (1991). Theory Change in Science. Strategies From Mendelian Genetics. Oxford: Oxford University Press.
Driver, R., and Scott, P. H. (1996). “Curriculum development as research: a constructivist approach to science curriculum development and teaching,” in Improving Teaching and Learning in Science and Mathematics, eds F. David Treagust, R. Duit and J. Barry Fraser (New York, NY: Teachers College Press, 94–108.
Engle, R. A., and Conant, F. R. (2002). Guiding principles for fostering productive disciplinary engagement: explaining an emergent argument in a community of learners classroom. Cogn. Instr. 20, 399–483. doi: 10.1207/S1532690XCI2004_1
Engle, R. A., Conant, F. R., and Green, D. W. (2007). “Progessive refinement of hypotheses in video-supported research,” in Video Research in the Learning Sciences, eds R. Goldman, R. Pea, B. Barron and Sharon J. Derry (Mahwah, NJ: Erlbaum), 240–243.
Hammer, D. (1995). Students inquiry in a physics class discussion. Cogn. Instr. 13, 401–430. doi: 10.1207/s1532690xci1303_3
Hestenes, D. (1987). Toward a modeling theory of physics instruction. Am. J. Phys. 55, 440–454. doi: 10.1119/1.15129
Hogan, K., and Pressley, M. (eds.). (1997). Scaffolding Student Learning: Instructional Approaches and Issues. Cambridge, MA: Brookline Books.
Kalman, C. S., and Lattery, M. J. (2018). Three active learning strategies to address mixed student epistemologies and promote conceptual change. Front. ICT 5:19. doi: 10.3389/fict.2018.00019
Khan, S. (2008). “Co-construction and model evolution in chemistry,” in Model Based Learning and Instruction in Science, eds J. Clement and M. A. Rea-Ramirez (Springer), 68–87. doi: 10.1007/978-1-4020-6494-4_4
Krajcik, J., Codere, S., Dahsah, C., Bayer, R., and Mun, K. (2014). Planning instruction to meet the intent of the next generation science standards. J. Sci. Teacher Educ. 25:157–175. doi: 10.1007/s10972-014-9383-2
Krajcik, J., and Merrit, J. (2012). Engaging students in scientific practices: what does constructing and revising models look like in the science classroom. Sci. Child. 49, 10–13. Available online at: https://www.jstor.org/stable/43747325
Kuhn, T. S. (1970). “Foundations of the Unity of Science,” in The Structure of Scientific Revolutions, 2nd Edn, eds O. Neurath, R. Carnap and C. Morris, Vol. I and II (Chicago, IL: The University of Chicago Press, 18–20; 49–50; 101–102.
Lattery, M. J. (2016). “Exploratory studies of Model-Based Reasoning,” in Deep Learning in Introductory Physics; Science & Engineering Education Sources, ed S. Calvin Kalman (Charlotte, NC: Information AGE Publishing, Inc.).
Lehesvuori, S., Viiri, J., Rasku-Puttonen, H., Moate, J., and Helaakoski, J. (2013). Visualizing communication structures in science classrooms: tracing cumulativity in teacher-led whole class discussions. J. Res. Sci. Teach. 50, 912–939. doi: 10.1002/tea.21100
Louca, L. T., Zacharia, Z. C., and Tzialli, D. (2012). Identification, Interpretation—evaluation, response: an alternative framework for analyzing teacher discourse in science. Int. J. Sci. Educ. 34, 1823–1856. doi: 10.1080/09500693.2012.671971
Machamer, P., Darden, L., and Craver, C. F. (2000). Thinking about mechanisms. Philos. Sci. 67, 1–25. doi: 10.1086/392759
Miles, M. B., and Huberman, M. A. (1994). Qualitative Data Analysis. An Expanded Sourcebook. Thousand Oaks, CA: Sage.
Minstrell, J., Anderson, R., and Li, M. (2011). “Building on learner thinking: A framework for assessment in instruction,” in Successful K-12 STEM Education: Identifying Effective Approaches in Science, Technology, Engineering, and Mathematics, ed Committee on Highly Successful Schools or Programs in K-12 StEM Education; National Research Council (Washington, DC: National Academies Press), 17.
Minstrell, J., and Kraus, P. (2005). “Guided inquiry in the science classroom,” in How Students Learn: History, Mathematics, and Science in the Classroom, edited by M. Suzanne Donovan and D. John Bransford (Washington, DC: The National Academies Press, 475–513.
Nassaji, H., and Wells, G. (2000). What's the use of 'Triadic Dialogue'?: An investigation of teacher-student interaction. Appl. Linguist. 21, 376–406. doi: 10.1093/applin/21.3.376
Nersessian, N. J. (1995). Should physicists preach what they practice? Constructive modeling in doing and learning physics. Sci. Educ. 4, 203–226.
Nersessian, N. J. (2008). Creating Scientific Concepts. Cambridge MA: MIT Press. doi: 10.7551/mitpress/7967.001.0001
NGSS Lead States. (2013). Next Generation Science Standards: For States, by States. Washington, DC: The National Academies Press.
Nunez-Oviedo, M. C. (2004). Teacher-Student Co-construction Processes in Biology: Strategies for Developing Mental Models in Large Group Discussions. Amherst: Ed.D., TECS, Massachusetts (Doctoral Dissertations Available online at: https://scholarworks.umass.edu/dissertations/AAI3118319).
Nunez-Oviedo, M. C., and Clement, J. (2008). “A competition strategy and other modes for developing mental models in large group discussion,” in Model Based Learning and Instruction in Science, edited by J. Clement and M. A. Rea-Ramirez (Dordrecht: Springer), 127–148. doi: 10.1007/978-1-4020-6494-4_7
Nunez-Oviedo, M. C., Clement, J., and Rea-Ramirez, M. A. (2008). “Developing complex mental models in Biology through model evolution,” in Model Based Learning and Instruction in Science, edited by J. Clement and M. A. Rea-Ramirez (Dordrecht: Springer), 182–202. doi: 10.1007/978-1-4020-6494-4_10
Nunez-Oviedo, M. C., Rea-Ramirez, M. A., Clement, J., and ane Else, M. (2002). “Teacher-student co-construction in middle school life science,” in Proceedings of the AETS Conference. (Charlotte, NC). Available online at: https://files.eric.ed.gov/fulltext/ED465602.pdf
Price, N., Lynn Stephens, A., Clement, J., and Nunez-Oviedo, M. C. (2017). Using imagery support strategies to develop powerful imagistic models. Sci. Scope 41, 40–49. doi: 10.2505/4/ss17_041_04_40
Rea-Ramirez, M. A. (1998). Models of conceptual understanding in human respiration and strategies for instruction (Doctoral Dissertations). Ed. D., School of Education, University of Massachusetts, Amherst, MA, United States. Available online at: https://scholarworks.umass.edu/dissertations/AAI9909208
Rea-Ramirez, M. A., Nunez-Oviedo, M. C., Clement, J., and Jane Else, M. (2004). Energy in the Human Body Curriculum. Amherst: National Science Foundation; University of Massachusetts. Available online at: http://www.cesd.umass.edu/energyinthehumanbody/
Reiser, B. J., Berland, L. K., and Kenyon, L. (2012). Engaging students in the scientific practices of explanation and argumentation. Understanding a framework for K-12 science education. Sci. Child. 35, 8–13. Available online at: https://www.jstor.org/stable/43747348
Schwarz, C. V., Reiser, B. J., Davis, E. A., Kenyon, L., Acher, A., Fortus, D., et al. (2009). Developing a learning progression for scientific modeling: making scientific modeling accessible and meaningful for learners. J. Res. Sci. Teach. 46, 632–654. doi: 10.1002/tea.20311
Scott, P. H. (1992). “Pathways in learning science: a case study of the development of one student's ideas relating to the structure of matter,” in Research in Physics Learning: Theoretical Issues and Empirical Studies. Proceedings of an International Workshop held at the University of Bremen, eds R. Duit, F. Goldberg, and H. Niederer (Kiel: IPN/Institute for Science Education, 203–224.
Scott, P. H. (1998). Teacher talk and meaning making in science classrooms: a Vygotskian analysis and review. Stud. Sci. Educ. 32, 45–80. doi: 10.1080/03057269808560127
Scott, P. H., Mortimer, E. F., and Aguiar, O. G. (2006). The tension between authoritative and dialogic discourse: a fundamental characteristic of meaning making interactions in high school science lessons. Sci. Educ. 90, 605–631. doi: 10.1002/sce.20131
Smith, J. P. I. I. I., diSessa, A. A., and Roschelle, J. (1994). Misconceptions reconceived: a constructivist analysis of knowledge in transition. J. Learn. Sci. 3, 115–163. doi: 10.1207/s15327809jls0302_1
Steinberg, M. S., and Wainwright, C. L. (1993). Using models to teach electricity -the CASTLE project. Phys. Teach. 31, 353–357. doi: 10.1119/1.2343798
Stephens, A. L., and Clement, J. (2010). Documenting the use of expert scientific reasoning processes by high school physic students. Phys. Rev. Spec. Phys. Educ. Res. 6, 1–15. doi: 10.1103/PhysRevSTPER.6.020122
Toulmin, S. (1972). Human Understanding. The Collective Use and Evolution of Concepts, Vol. I. London: Oxford University Press.
van Zee, E. H., and Minstrell, J. (1997a). Reflective discourse: developing shared understanding in a physics classroom. Int. J. Sci. Educ. 19, 209–228. doi: 10.1080/0950069970190206
van Zee, E. H., and Minstrell, J. (1997b). Using questioning to guide student thinking. J. Learn. Sci. 6, 227–269. doi: 10.1207/s15327809jls0602_3
White, B. Y., and Frederiksen, J. R. (2000). “Metacognitive facilitation: An approach to making scientific inquiry accessible to all,” in Teaching in the Inquiry-Based Science Classroom, eds J. Minstrell and E. van Zee (Washington, DC: American Association for the Advancement of Science), 20–21.
Williams, G., and Clement, J. (2015). Identifying multiple levels of discussion-based teaching strategies for constructing scientific models. Int. J. Sci. Educ. 37, 82–107. doi: 10.1080/09500693.2014.966257
Williams, G., and Clement, J. (2019). “Co-constructing models through whole class discussions in high school physics,” in Physics Teaching and Learning: Challenging the Paradigm, eds D. Sunal, J. Shemwell, J. Harrell, and C. Sunal (Charlotte, NC: Information Age Publishing), 102–103.
Keywords: science learning, science teaching, classroom discussion, scaffolding, scientific thinking, scientific practices, modeling
Citation: Nunez-Oviedo MC and Clement JJ (2019) Large Scale Scientific Modeling Practices That Can Organize Science Instruction at the Unit and Lesson Levels. Front. Educ. 4:68. doi: 10.3389/feduc.2019.00068
Received: 14 February 2019; Accepted: 26 June 2019;
Published: 23 July 2019.
Edited by:
Mark Lattery, University of Wisconsin–Oshkosh, United StatesReviewed by:
Allen Leung, Hong Kong Baptist University, Hong KongDina Tsybulsky, Technion Israel Institute of Technology, Israel
Copyright © 2019 Nunez-Oviedo and Clement. This is an open-access article distributed under the terms of the Creative Commons Attribution License (CC BY). The use, distribution or reproduction in other forums is permitted, provided the original author(s) and the copyright owner(s) are credited and that the original publication in this journal is cited, in accordance with accepted academic practice. No use, distribution or reproduction is permitted which does not comply with these terms.
*Correspondence: Maria Cecilia Nunez-Oviedo, bWFybnVuZXpAdWRlYy5jbA==