- 1Food Web Ecology Group, Limnological Institute, University of Konstanz, Konstanz, Germany
- 2Laboratorio de Limnología, Instituto de Investigaciones en Biodiversidad y Medioambiente (INIBIOMA), CONICET-Universidad Nacional del Comahue, Bariloche, Argentina
- 3Department of Earth, Atmospheric, and Planetary Sciences, Massachusetts Institute of Technology, Cambridge, MA, United States
- 4Biology Department, Woods Hole Oceanographic Institution, Woods Hole, MA, United States
- 5Institut de Ciències del Mar, Consejo Superior de Investigaciones Científicas (CSIC), Barcelona, Spain
- 6School of Mathematics and Science, Institute for Chemistry and Biology of the Marine Environment (ICBM), Carl-von-Ossietzky University of Oldenburg, Oldenburg, Germany
- 7Animal Ecology, Zoological Institute and Museum, University of Greifswald, Greifswald, Germany
In recent decades, there has been a growing recognition that mixotrophy, the ability to utilize both phototrophy and phagotrophy, is more common among plankton than previously assumed. Even though mixotrophs can become highly abundant, especially under nutrient limitation, and significantly alter nutrient cycling and food-web dynamics due to their dual nutritional modes, a comprehensive synthesis from a stoichiometric perspective is still lacking. We conducted a systematic literature review in which we identified over 130 studies that directly relate nutrient ratios to mixotrophic protists at the organism to community scale. By conceptually linking mixotrophy with the concept of ecological stoichiometry, we provide insights into (1) the role of mixotrophic metabolism and nutrient limitation in regulating cellular homeostasis, (2) mixotroph abundance and community scale responses to nutrient limitation, and (3) the specific case of harmful algal bloom forming mixotrophs. On the organism scale, the existing literature points towards a stabilizing effect of mixotrophic metabolism on elemental composition, and the use of grazing as a compensation mechanism under stoichiometric imbalances in the water and prey. At the community scale, mixotrophs were found to increase in abundance relative to strict autotrophs and heterotrophs in nutrient-limited communities, and provide beneficial food for zooplankton grazers by maintaining relatively low and stable stoichiometry. Furthermore, global-scale models and studies of harmful algal blooms reveal the increasing importance of mixotrophs under climate change – highlighting the need for continued research addressing the interactions between mixotrophs and dynamic stoichiometry to understand the impacts of mixotrophs on global nutrient cycles.
Introduction
Planktonic protists play a fundamental role in aquatic ecosystems, particularly in modulating nutrient dynamics. These microorganisms constitute the base of the food web and are crucial in transferring nutrients between trophic levels. Through cellular processes like photosynthesis and nutrient uptake, planktonic protists regulate key biogeochemical processes, including the cycling of carbon (C), nitrogen (N), and phosphorus (P). Phytoplankton contribute approximately 50% of global primary production, fixing around 45-50 gigatons of C annually (Falkowski et al., 1998). Both autotrophic and heterotrophic protists also enhance the efficiency of nutrient recycling in aquatic ecosystems (Sherr and Sherr, 2002). For instance, planktonic protists play a pivotal role in the microbial food web by recycling organic matter and facilitating the transfer of nutrients through microbial grazing (Azam et al., 1983; Worden et al., 2015). Their contribution to nutrient remineralization in surface waters supports the continuous availability of nutrients for other organisms – highlighting their importance in ecosystem and global scale nutrient cycling (Pomeroy et al., 2007).
Traditionally, planktonic protists have been classified as autotrophs or heterotrophs. However, there is increasing evidence of the importance of mixotrophic plankton, which obtain energy and nutrients from both autotrophy and heterotrophy through photosynthesis and phagotrophy, allowing them to adapt to variable environmental conditions by utilizing these two metabolic strategies simultaneously (Flynn and Hansen, 2013; Mitra et al., 2014; Stoecker et al., 2017). Many species previously considered purely autotrophic or heterotrophic are now known to be mixotrophic (Jones, 1997; Stoecker, 1998). Given the functional diversity among these organisms, Mitra et al. (2016) proposed a classification for mixotroph functional groups, distinguishing between constitutive mixotrophs that have an innate photosynthetic ability (i.e., have their own photosystems) and non-constitutive mixotrophs that acquire photosystems from their prey (e.g., via kleptoplasty). Furthermore, previous studies highlight the abundance and ecological importance of mixotrophic protists in aquatic ecosystems, as well as their key role in nutrient cycling and food web dynamics (Flynn et al., 2019). By combining phototrophic and phagotrophic feeding modes, mixotrophs can outcompete strict autotrophic protists in nutrient-poor conditions by grazing on nutrient-rich prey when autotrophy is insufficient for nutrient acquisition (Nygaard and Tobiesen, 1993; Yvon-Durocher et al., 2017; Schenone et al., 2022). Additionally, mixotrophs play a key role in the microbial food web, linking primary producers to higher trophic levels (Zubkov and Tarran, 2008; Hartmann et al., 2012; Schenone et al., 2021).
Despite the growing recognition of their ecological significance, a comprehensive synthesis of the interactions between mixotrophic protists and the relative abundance of nutrients (e.g., C:N:P ratios) is still lacking. The dual nutritional mode of mixotrophs complicates our understanding of how they respond to varying environmental conditions, especially regarding nutrient uptake and cycling (Flynn et al., 2019; Millette et al., 2023). For example, while autotrophic protists are typically constrained by light or inorganic nutrient availability, and heterotrophic protists by prey abundance and nutritional content, mixotrophs may navigate such limitations by utilizing their metabolic plasticity (Flynn and Mitra, 2009; Flynn et al., 2019). However, it remains unclear how they balance these competing demands, and how their nutrient acquisition strategies shift depending on resource ratios.
Ecological stoichiometry provides a powerful framework for understanding nutrient dynamics in ecosystems, particularly in the context of mixotrophy. This framework focuses on the balance of elements (e.g., C:N:P) and the interplay between nutrient ratios and biological processes (Sterner and Elser, 2002; Klausmeier et al., 2004). For mixotrophs, ecological stoichiometry can be especially useful in elucidating how nutrient availability and demand are balanced between their autotrophic and heterotrophic modes of nutrition. Important organismal traits, such as homeostasis (the ability to maintain a stable internal nutrient composition) and efficiency of nutrient acquisition, play critical roles in determining how mixotrophs interact with their environment (Millette et al., 2023). For example, the extent to which an organism’s elemental composition remains stable in response to changing resource supplies has important implications for organism-level responses to nutrient limitation (Sterner and Elser, 2002; Persson et al., 2010), community composition and stability (Yu et al., 2010, 2015), and global C cycling and sequestration (Galbraith and Martiny, 2015). Understanding these stoichiometric principles is therefore key for predicting the role of mixotrophs in nutrient cycling, their interactions with other organisms, and their overall effect on ecosystem function.
Here, we performed a systematic review of the scientific literature to synthesize how ecological stoichiometry can be integrated into the study of mixotrophic protists. We identified, classified, and analyzed papers linking mixotrophs’ cellular elemental ratios with the elemental ratios of their environment, prey, and predators. We focused on key characteristics influencing the role of mixotrophs in nutrient cycles at the organism and community scales – namely their metabolic rates as well as their abundance and trophic links within natural planktonic communities (Figure 1). We analyzed our results in terms of key concepts from the ecological stoichiometry framework, such as homeostasis, nutrient limitation, and trophic transfer efficiency. Furthermore, we discussed our findings in terms of the functional diversity of mixotrophs (Mitra et al., 2016) and their potential to form harmful algal blooms (HABs) (Burkholder et al., 2008; Mitra and Flynn, 2010). With this extensive review, we aim to expand the understanding of mixotrophy in terms of ecological stoichiometry, as well as detect gaps in the literature and identify future research priorities.
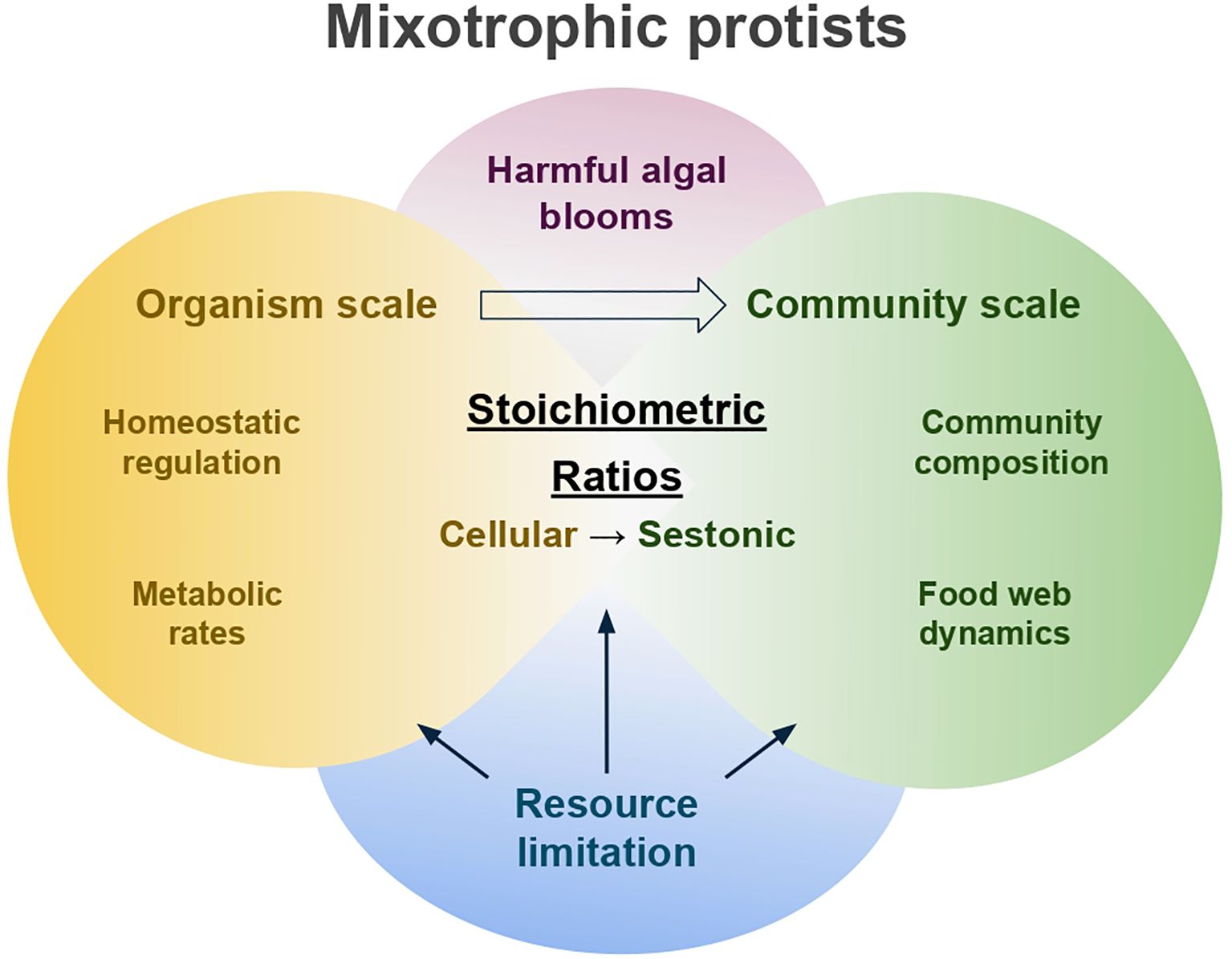
Figure 1. Conceptual framework illustrating the interplay between ecological stoichiometry and mixotrophic protists across organismal and community scales. At the organism scale, mixotrophic protists regulate both autotrophic and heterotrophic metabolic pathways in response to the resource availability, allowing them to maintain greater stoichiometric homeostasis. At the community scale, nutrient limitation and the stoichiometric regulation of mixotrophs shape community composition and food web dynamics, influencing overall seston stoichiometry. This framework is also relevant to harmful algal blooms (HABs) produced by mixotrophs, demonstrating the broader ecological relevance of stoichiometric dynamics.
Materials and methods
We performed a systematic search of the scientific literature published until the 13th of September 2024. The digital database from Web of Science was searched using the terms mixotroph* AND *plankton* OR *protist* OR *ciliate* OR *flagellate* OR *protozo* OR dinophy* OR chrysophy* OR haptophy* OR cryptophy* OR euglen* AND NOT veliger OR chemoauto* OR chemolitho* OR “mixotrophic cultivation”. The term alga* was not included because its results focused on the production of non-phagotrophic taxa (e.g., Chlorella or Chlamydomonas) in “mixotrophic cultures” enriched in dissolved organic C (i.e., defining mixotrophy as the combination of autotrophy and osmotrophy).
The search returned 1807 papers. The step-wise paper selection and screening followed the guidelines of Preferred Reporting Item for Systematic Reviews and Meta-analysis in Ecology and Evolutionary Biology (O’Dea et al., 2021) (Supplementary Figure S1). First, we accounted only for scientific articles in English. We then read through paper titles and abstracts and excluded those that were not studying mixotrophic protists (e.g., mixotrophic prokaryotes, coral endosymbionts, etc.) or that defined mixotrophy as the combination of autotrophy and osmotrophy (rather than phagotrophy). For the remaining 1641 articles (hereafter ‘all articles’), we performed three classification steps. First, we checked if the studies considered ecological stoichiometry by searching for the terms ‘stoich’ and/or ‘ratio’ within the main text, keywords, and abstract. We only considered the term ‘ratio’ if it was connected to: nutrient(s), light:nutrient, or any ratio between elements, cellular investments, or different dissolved, particulate, or inorganic nutrients (e.g. TN: TP, POC: DOP, POC: PON, DIN: DIP, PSi: POC, Chl:C, FA:C, among others). In the second classification step, we retained primary research articles (i.e., excluded reviews and opinion papers) which considered the terms ‘stoich’ and/or ‘ratio’ within their methods or results sections. Finally, in our third classification step, we analyzed the remaining articles to assess if ratios were used as quantitative response or predictor variables directly related to mixotrophic protist(s). These links were further classified depending on where the ratio was measured (e.g., cellular, sestonic, or media/water C:N:P ratio) and the type of variable (e.g., mixotroph grazing rate or abundance) associated with the mixotroph (Supplementary Figure S2). With this final step, we excluded articles in which ratios were not used as variables or were not related to mixotrophy. Additionally, we extracted information such as the study system (e.g., laboratory culture, field sampling) and whether the article was focused on HAB-forming mixotrophs by searching for the words ‘harmful’, ‘toxic’, and ‘bloom’ in the title, abstract, keywords, and introduction.
From the 1641 articles that passed the initial screening, 32.9% contained the terms ‘stoichiometry’ and/or ‘ratio’ in the main text, 18.6% considered ratios in the methods and/or results sections, and 8.2% of the articles used ratios as response or predictor variables linked to another variable related to mixotrophs (Figure 2). Therefore, our literature search identified 132 papers relating stoichiometric ratios with mixotrophic protists. While our workflow allowed for multiple types of ratios, the vast majority of papers that passed through our classification process considered C:N:P ratios (90%), and thus this was the focus of our discussion. Most of these publications studied mixotroph stoichiometry in laboratory experiments (30%), whereas seston stoichiometry and dissolved nutrient ratios were more commonly assessed in field studies (16% and 25% of the final papers, respectively) (Figure 3). Still, studies on predator and prey stoichiometry associated with mixotrophs were scarce across all study types (<3% each). Articles focused on modeling were also scarce across all categories of ratios measured. To streamline the analysis, we further categorized the 132 papers into those focused on the metabolism and/or composition of individual mixotrophic organisms (organism-scale studies, 55 articles, 42%) and those addressing mixotrophs’ effects on community variables and sestonic ratios (community-scale studies, 70 articles, 53%) (Supplementary Figure S1), with 7 articles (5%) assigned to both categories. At the organism level, the majority of studies examined the influence of dissolved or cellular nutrient ratios on metabolic traits (40%) and the impact of resource manipulations on the cellular composition of mixotrophs (48%). At the community level, the plurality of papers focused on how dissolved nutrient ratios affect community composition and processes (40%), followed by studies considering the impact of spatial-temporal variables (27%) and resource availability (18%) on sestonic ratios. A limited number of studies explored the interactions among mixotrophic metabolism, predator traits, and internal nutrient ratios (6%). Finally, 31 of the final 132 articles (23%) were focused on HAB-forming mixotrophs, from which 9 articles included the effect of nutrient ratios on toxin production. Based on these findings, we organized our discussion into sections addressing the organism and community scales, as well as a separate discussion regarding HAB forming mixotrophs and their toxicity.
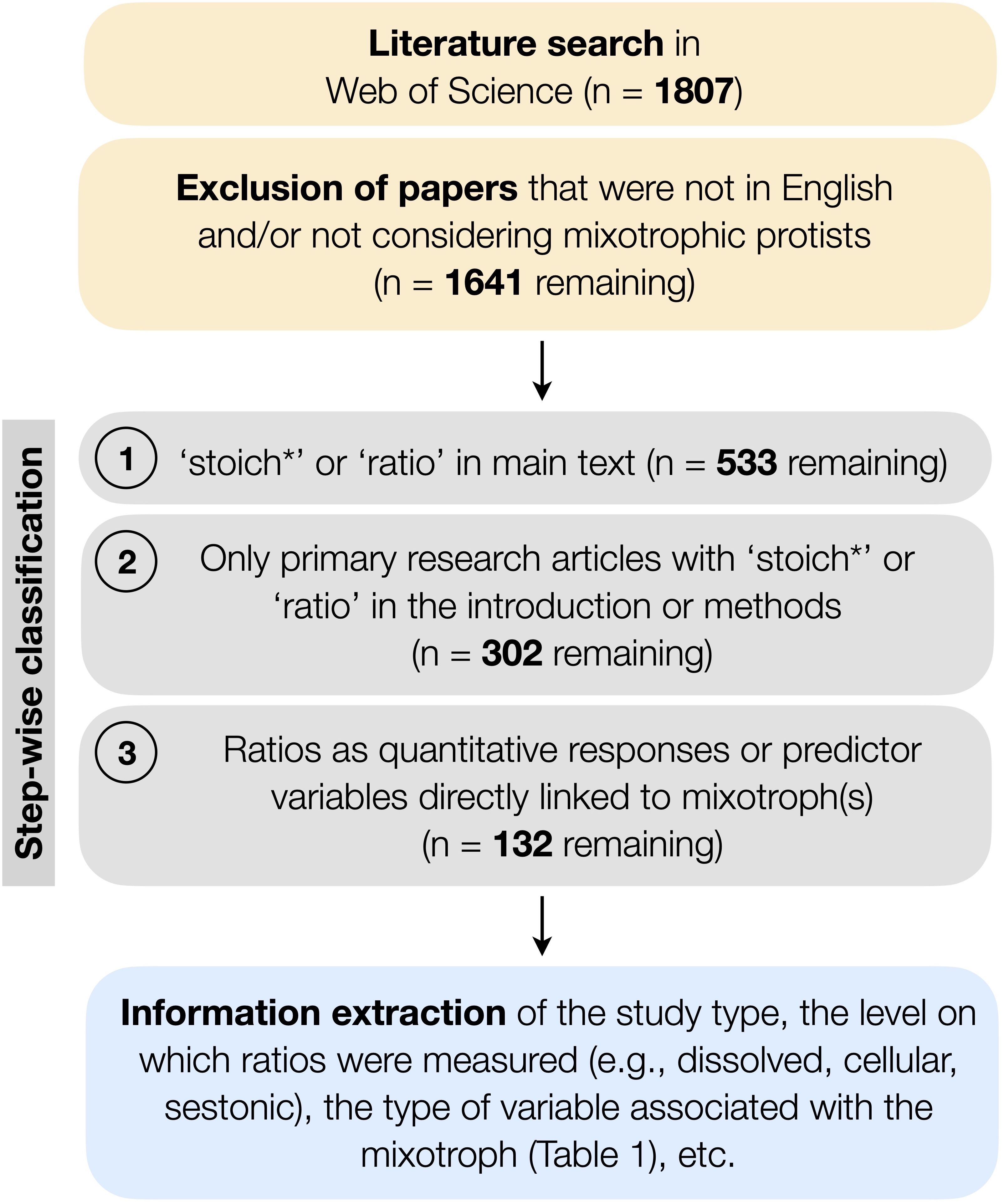
Figure 2. Flow diagram of the paper selection and classification process. The colors of the boxes indicate the workflow step (i.e., orange indicates the literature search and primary exclusion of papers, gray represents the stepwise classification process, and blue denotes the information extraction step). The number of studies at each step is denoted by n. A detailed PRISMA-style flow diagram can be found in the Supplementary Material (Supplementary Figure S1).
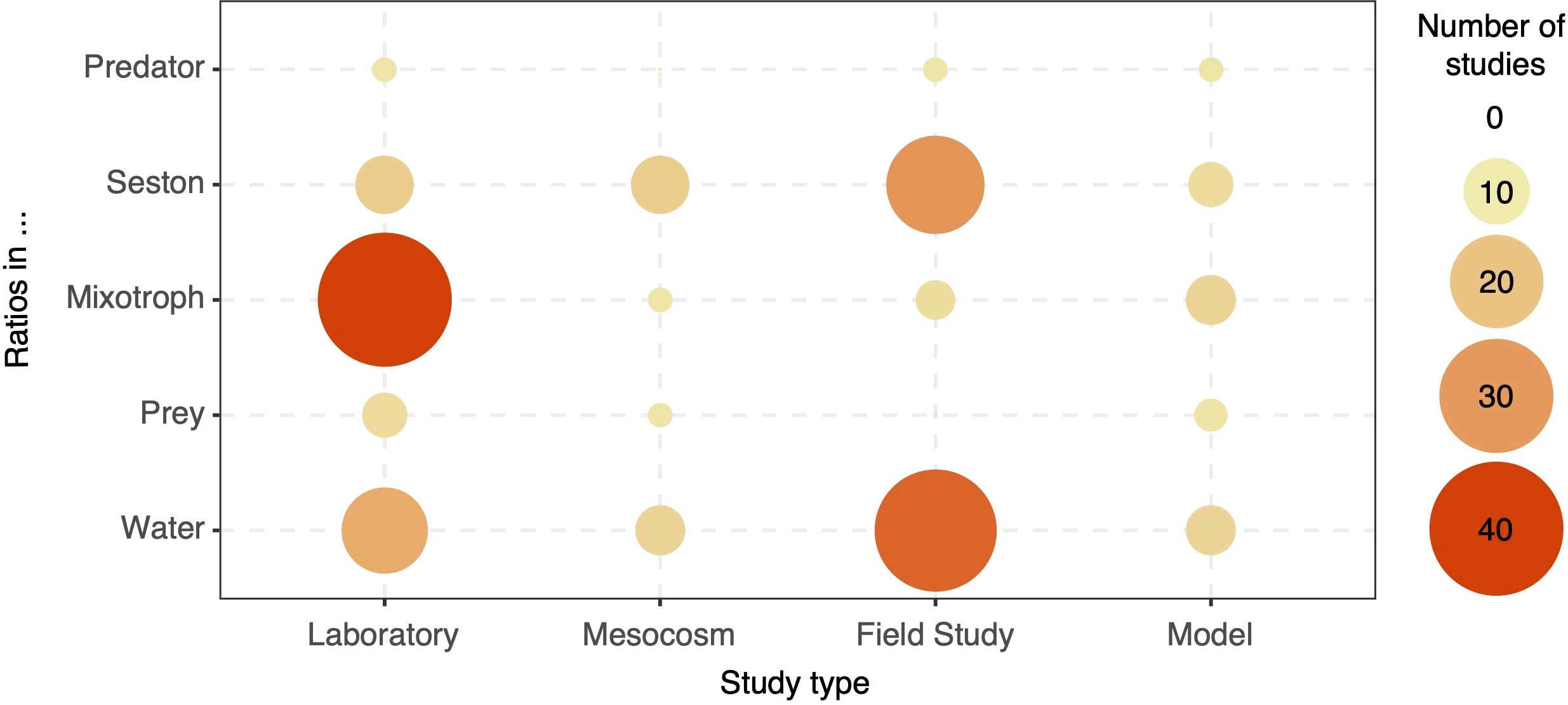
Figure 3. Number of studies conducted in combinations of study type and where the ratios were measured. Circles sizes and colors denote the number of papers. If no circle is present, no study was found for this combination.
Results and discussion
Organism scale
Mixotrophic metabolism promotes stoichiometric homeostasis
Stoichiometric homeostasis is a central tenet of ecological stoichiometry, with significant organism and ecosystem scale effects (Koojiman, 1995; Sterner and Elser, 2002). Mixotrophs derive energy and nutrients from both autotrophy and heterotrophy (Stoecker et al., 2017), giving them significant nutritional flexibility. This flexibility may help maintain a relatively stable elemental composition, with laboratory studies showing interquartiles of cellular N:P ratios from 8.53-31.37 for autotrophic plankton and 12.32-25.40 for mixotrophic plankton (Moorthi et al., 2017). A study of the primarily phototrophic species, Chrysochromulina brevifilum, revealed that cells had a slightly lower C:N ratio when grown as a mixotroph (C:N = 5.8:1) versus autotroph (C:N = 6.4:1) under low light. When grown autotrophically, C:N decreased marginally (6.4:1 to 5.6:1) as irradiance increased (20 to 100 µmol photons m-2 s-1) (Jones et al., 1995). The C:N ratio under mixotrophic growth at 20 µmol photons m-2 s-1 was therefore most similar to autotrophic C:N under sufficient light, indicating that mixotrophy may act as a stabilizing force on stoichiometry when resources are limited. This result, together with studies showing relatively stable cellular ratios in mixotrophs over a range of experimental treatments (Katechakis et al., 2005; González-Olalla et al., 2021; Fischer et al., 2022), supports the hypothesis that mixotroph stoichiometry does not vary substantially with shifts in nutrition or external supply rates, but instead is kept balanced by using multiple metabolic pathways to access resources. Elemental ratios in mixotrophs also appear to be less variable than those of strict autotrophs (Figure 4) (Smalley et al., 2003; Katechakis et al., 2005; Leonardos and Geider, 2005). Two mixotrophic species, Ochromonas tuberculata and Cryptomonas sp., exhibited lower and more stable C:P ratios than an autotrophic phytoplankton, Scenedesmus obliquus, in response to light and P availability – particularly under low P and high light conditions, where the use of phagotrophy resulted in 2-3 times lower C:P ratios (Katechakis et al., 2005). This provides evidence that mixotrophy may have a more significant impact on organism homeostasis under resource limitation or imbalance compared to replete conditions. However, this stabilizing effect may require that mixotrophy is sufficient for the relief of said limitation. Barbaglia et al. (2024) cultured eight strains of Ochromonas and showed that C:N ratios were greater and more variable in low bacteria treatments, which experienced nutrient limitation, than in high prey cultures. Further studies offer mixed support for the potentially stabilizing effects of mixotrophy (Wilken et al., 2014a; Liu et al., 2021). In contrast to the above findings, Wilken et al. (2014a) found substantially greater variability in the cellular C:N ratio of Ochromonas danica when grown mixotrophically (C:N = 19.5 ± 9.9) versus autotrophically (C:N = 13.4 ± 1.2), but no data was available for the stoichiometry of the prey nor for the dissolved nutrient concentrations after inoculation, preventing further analysis of what may have driven the observed differences across treatments. Liu et al. (2021) also provided evidence for weak homeostasis, as the C:N ratio of Lepidodinium sp. in a nutrient-replete temperature manipulation experiment both was higher under mixotrophic (C:N = 6.45 ± 0.275 and 7.30 ± 0.275 at 22 and 31°C respectively) versus autotrophic growth (C:N = 6.22 ± 0.095 and 6.65 ± 0.0314 at 22 and 31°C respectively) and increased with ingestion rate, suggesting that the observed cellular composition under mixotrophic growth may have been impacted by the high C:N ratio of the prey. Despite these caveats, which deserve further consideration, the literature points to mixotrophy as a stabilizing force due to the usage of dual metabolic pathways.
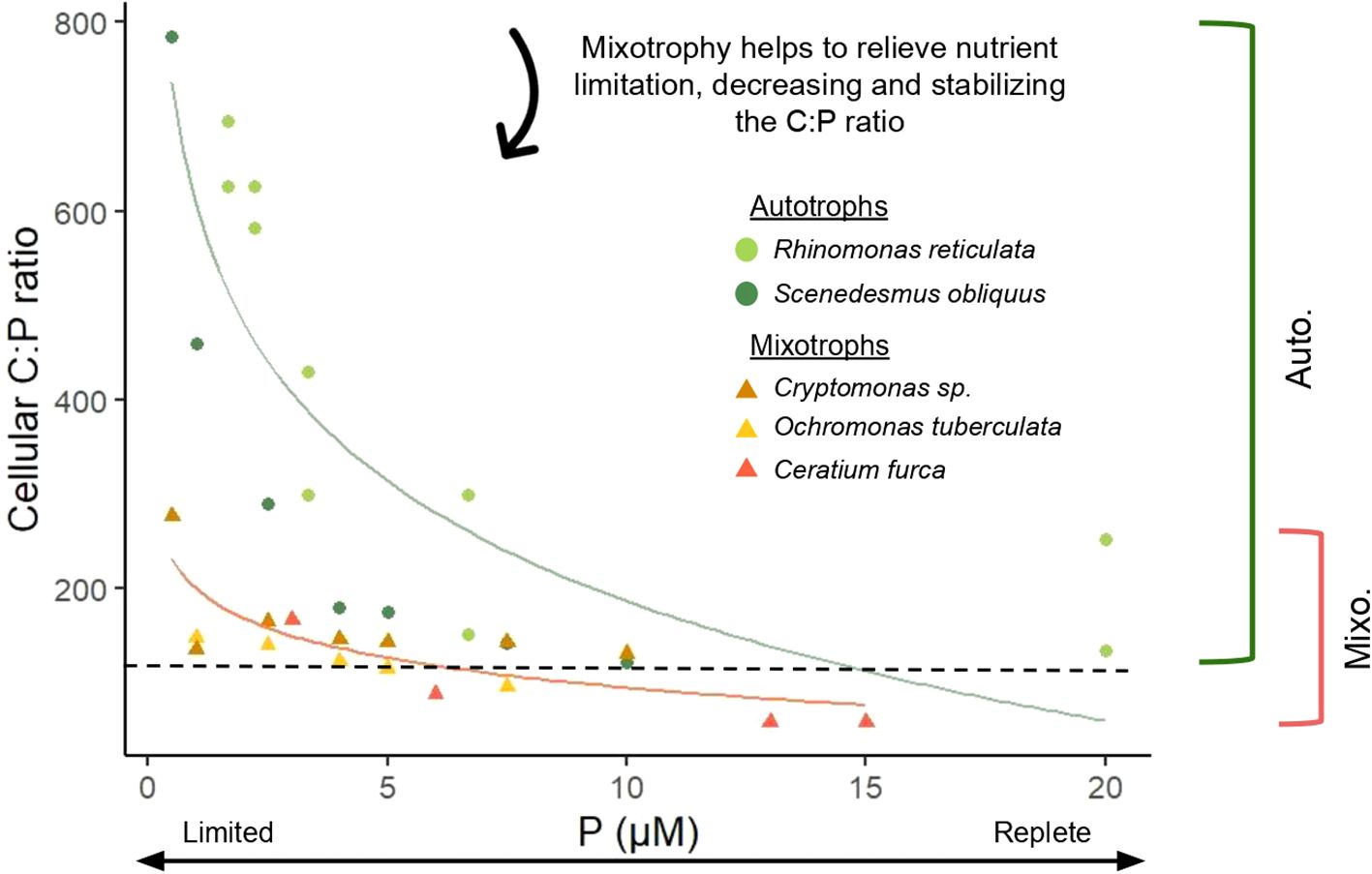
Figure 4. Response of autotrophic and mixotrophic plankton C:P ratios to external P availability (µM P), with mixotrophs exhibiting lower ratios under nutrient limitation and more stable cellular stoichiometry across all treatments (greater homeostatic regulation). Data shown for autotrophic species (circles) Rhinomonas reticulata (Leonardos and Geider, 2005) and Scenedesmus obliquus (Katechakis et al., 2005) and for primarily phototrophic mixotrophs (triangles) Ceratium furca (Smalley et al., 2003), Cryptomonas sp., and Ochromonas tuberculata (Katechakis et al., 2005). Curves were calculated using a logarithmic regression and brackets indicate the range of observed cellular C:P ratios for the plotted autotrophic and mixotrophic protists. The black dashed line represents Redfield C:P ratio.
Because the organismal C:N:P of plankton is a function of cellular macromolecular composition, meaning that investment in cellular pools such as the photosynthetic apparatus are directly related to stoichiometry (Geider and La Roche, 2002; Finkel et al., 2016; Inomura et al., 2020), metabolic rates can help elucidate the mechanisms supporting stoichiometric homeostasis in mixotrophs. In primarily phototrophic organisms, nutrient limited cultures exhibited positive correlations between ingestion rate and both cellular C:N and C:P ratios (Li et al., 2000; Smalley et al., 2003; González-Olalla et al., 2021), and, in eight strains of Ochromonas ranging from facultative heterotroph to autotroph, C:N ratios and investments in phagotrophy were both elevated under low prey concentrations (Barbaglia et al., 2024). Importantly, Smalley et al. (2003) found that the stimulation of phagotrophy in batch cultures of Neoceratium furca lagged several days behind nutrient depletion and increased as cellular composition deviated beyond an optimal range of C:N:P values, resulting in correlation coefficients of 0.439 and 0.480 for ingestion rate vs C:N in N-limited cultures and C:P in P-limited cultures respectively. This offers a potential mechanism for the observed relationships between metabolic rates and cellular ratios, whereby external nutrient availability alters mixotroph stoichiometry, and the resulting deviations in stoichiometry and/or limitation subsequently initiate shifts in the use of phagotrophic and/or photosynthetic nutritional pathways (Smalley et al., 2003, 2012). High C:nutrient ratios in the organism, as observed in the aforementioned studies, are indicative of nutrient limitation (Sterner et al., 1997; Elser et al., 2003b), while increased grazing is a known strategy for supplementing inorganic nutrients (Caron et al., 1993; Carpenter et al., 2018) – allowing the mixotrophic plankton a means by which to counter this limitation and ultimately maintain a relatively stable elemental composition. Furthermore, while changes in metabolic rate may occur in response to internal stoichiometry, mixotroph metabolism can also drive internal ratios. For instance, it was observed that low light levels increased investments in the photosynthetic apparatus of Isochrysis galbana due to photoacclimation, which elevated cellular C:N ratios (from 8.08 to 8.86 at 100 and 25 µmol photons m-2 s-1 respectively) alongside an increase in bacterivory (González-Olalla et al., 2019, 2021). It should also be noted that shifts in C:N and C:P ratios are indicative of growth rate as well as nutrient limitation, with increased allocation to protein and RNA under high growth rates leading to decreased C:N and C:P ratios (Flynn et al., 2010; Inomura et al., 2020). Overall, studies indicate that external resources alter mixotroph physiology, resulting in significant bidirectional impacts between metabolic rates and internal stoichiometry.
It should be noted that, because most papers considering both metabolic rates and cellular ratios focused on inducing phagotrophy in obligate or primarily phototrophic species, data on photosynthetic rates in primarily phagotrophic species, such as Poterioochromonas malhamensis, is minimal (Caron et al., 1990; Sanders et al., 1990). However, we hypothesize that the same theory will hold true for photosynthetic rates, such that, similar to the use of heterotrophy to obtain N and P when organism C:nutrient was high, photosynthesis will be upregulated to provide an additional C source when cellular C:nutrient ratios are low. This is supported by the modeling study of Edwards (2019) which showed that, when phagotrophs are C limited due to feeding on nutrient-enriched bacterioplankton, mixotrophs can outcompete these strict heterotrophs by relieving C limitation via photosynthesis. Further laboratory experiments inducing photosynthesis in mixotrophic plankton could help to test this hypothesis and provide additional insight into how physiology mediates the relationship between external and internal elemental ratios.
Metabolic plasticity benefits mixotrophic protists under nutrient limitation
As evidenced above, external nutrient availability plays a key role in regulating mixotrophic metabolism. Among the papers identified via our workflow, several studies examined how ingestion rates of primarily phototrophic mixotrophs respond to dissolved N:P ratios, illustrating the importance of both absolute and relative nutrient concentrations for modulating phagotrophy. Studies on the dinoflagellates Gyrodinium galatheanum and N. furca found that inorganic nutrient ratios are instrumental in determining feeding rates (Li et al., 2000; Smalley and Coats, 2002; Smalley et al., 2012). Nutrient addition experiments revealed no clear relationship between absolute nutrient concentration and ingestion rate in field samples of N. furca. Instead, these experiments pointed to the importance of inorganic nutrient ratios as an indicator of nutrient limitation, with added N under low dissolved N:P (∼7:1) and added P under high N:P (∼16.5:1-22:1) both decreasing phagotrophy (Smalley et al., 2012). Investigation of feeding in G. galatheanum showed that grazing was not only upregulated in response to nutrient limitation, but also increased as the medium N:P deviated further from the organism’s optimal ratio of 10:1, with ingestion rates increasing by >50% under N-starvation (Li et al., 2000). Laboratory studies of chrysophytes Chrysolepidomonas dendrolepidota and Dinobryon sociale in nutrient replete, reduced N, reduced P, and reduced N+P media both reported increased grazing rates under macro-nutrient limitation, providing evidence that this upregulation of bacterivory was sensitive to the concentration as well as the stoichiometry of available dissolved nutrients (Princiotta et al., 2016; Hamsher et al., 2020). Increases in D. sociale ingestion rate were similar in reduced N (N:P = 5:1), reduced P (N:P = 20:1), and reduced N+P (N:P = 16:1) treatments, all exhibiting grazing rates of ~2-2.25 compared to ~1.4 bacteria cell-1 hr-1 under nutrient replete conditions (Princiotta et al., 2016). These findings suggest that the observed shifts in nutritional balance, meaning the relative contributions of photosynthesis versus phagotrophy, are triggered by dissolved nutrient limitation (i.e., absolute concentrations) in addition to imbalances in N:P availability (i.e., changes in media stoichiometry). While phagotrophy may be upregulated to compensate for imbalances in nutrient availability when external N:P ratios differ greatly from the Redfield or organism-specific optimal ratio, increased grazing can also be a response to imbalances in internal C:N and C:P ratios when inorganic nutrient concentrations are low. This may explain the similar grazing responses observed in C. dendrolepidota and D. sociale under single- and co- nutrient limitation. Therefore, while additional data is necessary to elucidate potential taxon-specific responses, these studies provide further evidence that mixotrophs become more heterotrophic in response to nutrient limitation (Caron et al., 1993; Carpenter et al., 2018), with phagotrophy upregulated in response to both dissolved nutrient concentration and ratios.
In addition to the body of literature addressing the use of phagotrophy to relieve inorganic nutrient limitation, an additional set of experimental studies examined how mixotroph ingestion and growth rates are influenced by the C:N:P ratios of their prey, with consistent results. Under P limitation, high C:P and N:P ratios in protistan prey resulted in increased ingestion in mixotrophic predators (Wickham and Wimmer, 2019; Liu et al., 2022) and increased growth rates in mixotrophic predator O. danica [0.069 h-1 ± 0.004 at high N:P ratios compared to 0.016 h-1 ± 0.001 at balanced ratios (Chrzanowski et al., 2010)]. In their model simulation, Mitra and Flynn (2023) also observed that a high C:P ratio of cyanobacteria prey led to higher ingestion rates by mixotrophs. In N-limited conditions, high prey C:N ratios resulted in increased grazing but a four-fold reduction in growth rates in the mixotroph Ochromonas (Wilken et al., 2014b). These observations are often explained by the assumption that mixotrophs exhibit a strategy of “compensatory feeding” to relieve nutrient limitation, where high prey ingestion compensates for lower stoichiometric nutritional quality to satisfy elemental nutrient requirements (Cruz-Rivera and Hay, 2000; Meunier et al., 2012; Wei et al., 2020). These findings suggest that prey stoichiometry has an impact on mixotroph metabolic rates, with unbalanced C:P or C:N ratios leading to increased ingestion rates in mixotrophic predators to counter nutrient deficiency. Therefore, increased grazing is used to compensate not only for limited inorganic nutrients but also when prey stoichiometry deviates from the optimal ratio for the predator. Overall, these studies show that individual traits of mixotrophs, such as homeostasis and metabolic plasticity, may prove particularly beneficial under nutrient limitation, giving them a competitive advantage over strict autotrophs.
Community scale
Prevalence of mixotrophs under nutrient limitation partially drives sestonic C:N:P
Mixotrophic protists can become highly abundant in response to nutrient limitation, thus affecting and being affected by nutrient ratios in natural aquatic systems (Lagus et al., 2004; De Lima et al., 2019; Leruste et al., 2021). For example, in the Mediterranean Biguglia Lagoon, mixotrophic protists were particularly dominant during nitrogen and phosphorus co-limitation (relative abundance of 60-90%), constituting the highest proportions of the planktonic community under N:P ratios ranging from 65 to 125 in autumn and spring (Leruste et al., 2021). In a nutrient enrichment experiment using a natural planktonic community from the northern Baltic Sea, Lagus et al. (2004) observed that the biomass of the mixotrophic chrysophyte Uroglena increased significantly in treatments with higher N:P ratios (39 ± 2). This result suggests that Uroglena might have been compensating for P limitation by potentially feeding on P-rich bacteria, though phagotrophy was not directly measured. However, not all mixotrophic species in this community exhibited similar responses; for instance, Dinobryon faculiferum did not show a clear positive relationship with the N:P ratio, indicating that responses to nutrient variations may vary based on species-specific traits (Lagus et al., 2004). Furthermore, additional mesocosm and experimental food web studies showed that the prevalence of mixotrophic protists can lead to distinct shifts in sestonic nutrient ratios as a result of their flexible nutrient acquisition strategies (Ptacnik et al., 2004; Faithfull et al., 2011; Carrillo et al., 2017), for example, lowering and stabilizing C:P ratios (Figure 5) (Katechakis and Stibor, 2006; Jäger et al., 2014). Katechakis and Stibor (2006) found that under low dissolved N and P supplies, the mixotroph O. tuberculata achieved a high relative abundance (92%), which led to lower sestonic C:P ratios compared to treatments where strict autotrophs were more abundant. Similarly, using mesocosm experiments, Faithfull et al. (2011) and Jäger et al. (2014) reported that when the community was dominated by mixotrophic flagellates such as Dinobryon (60-90% of relative abundance), sestonic C:P ratios remained lower and less variable compared to communities dominated by the strict autotrophs. These findings align with the expectations detailed at the organismal scale. Conversely, Ptacnik et al. (2004) demonstrated that the presence of the mixotrophic nanoflagellate Chrysochromulina polylepis increased sestonic C:N ratios from 8 to 11 in experimental food webs released from N limitation – shifting the C:N ratio farther from the canonical Redfield value and indicating greater N limitation. On the other hand, field studies identified from our workflow did not show a clear relation between sestonic nutrient ratios and the abundance of mixotrophic protists in the natural community (Lavrentyev et al., 1998; Novotná et al., 2010; González-Olalla et al., 2018). This discrepancy may be explained by natural community stoichiometry being a function of a complex set of abiotic and biotic factors. For example, while the increasing abundance of mixotrophic protists may lower sestonic C:N:P ratios, their increasing abundance is also correlated with nutrient limitation – complicating the observed signal. It should also be noted that while the lack of papers showing predominantly mixotrophic communities in nutrient replete environments is likely related to a decreased competitive advantage of mixotrophs against strict autotrophs and heterotrophs under these scenarios (Flynn and Mitra, 2009; Fischer et al., 2017) it could also be a product of bias in our classification framework, which focused on communities where mixotrophs were abundant. Still, the results shown here highlight the ability of mixotrophs to thrive in classically unfavorable environments (i.e., oligotrophic systems) by compensating for imbalances in their elemental ratios through efficient acquisition of resources.
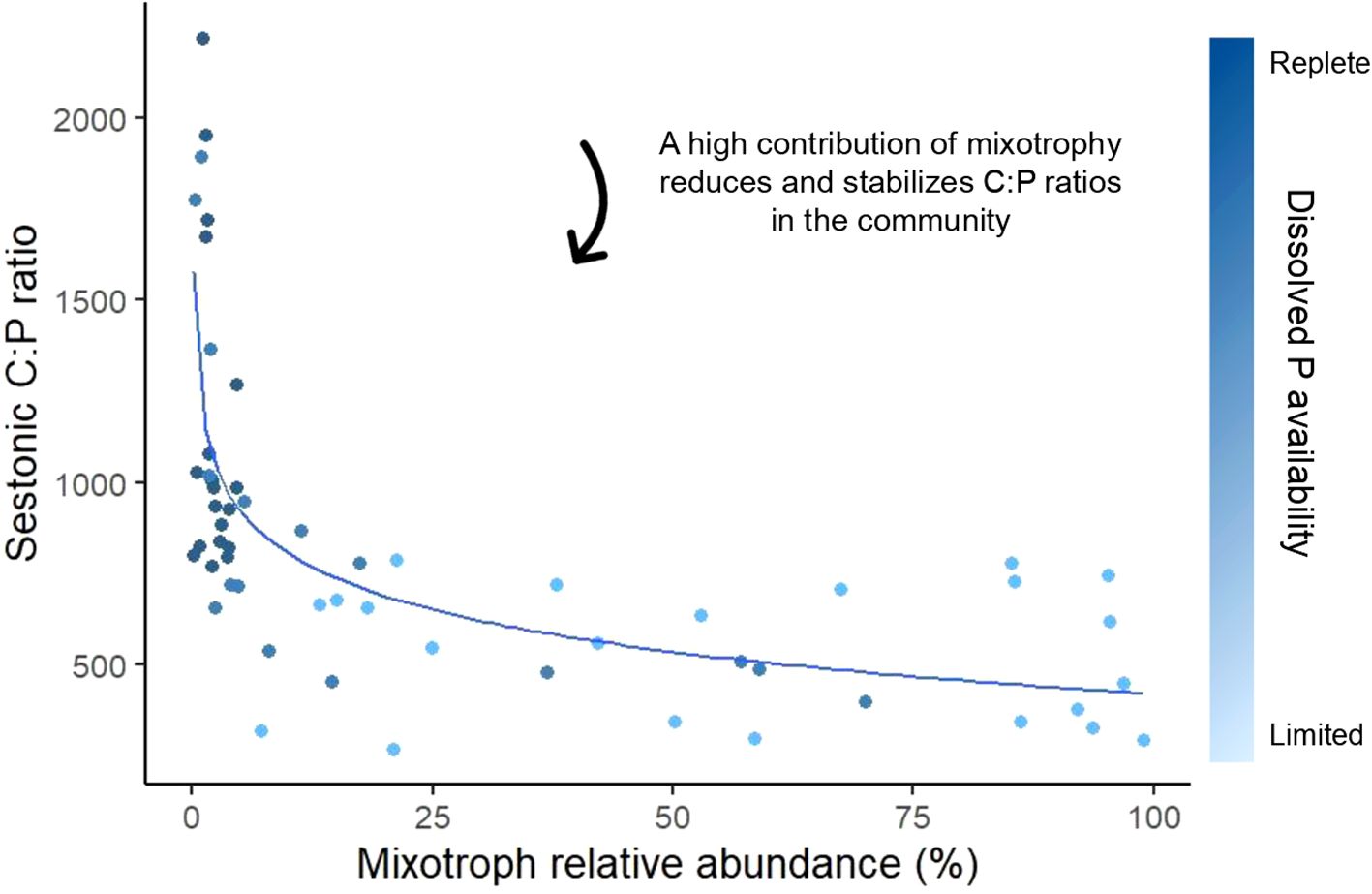
Figure 5. The influence of mixotrophic protists in decreasing and stabilizing sestonic C:P ratios as they became prevalent in the planktonic community. Data points were extracted from the experimental studies in Katechakis and Stibor (2006) and Jäger et al. (2014), which were selected during the last step of our classification framework. The curve was calculated using a logarithmic regression. Nutrient availability was estimated from N and P supply treatments (Katechakis and Stibor, 2006), as well as P initial limitation followed by its subsequent alleviation (Jäger et al., 2014).
Zooplankton grazers may benefit from mixotroph homeostasis
Mixotrophs may also alter plankton community structure and trophic transfer through their role as prey. In particular, mixotrophs’ more stable elemental ratios relative to autotrophic plankton have significant implications for grazer performance, because this plasticity may reduce the nutrient mismatch between consumer nutrient requirements and the elemental composition of their prey – thus providing food of better nutritional quality (Hessen et al., 2013; Golz et al., 2015; Karpowicz et al., 2019; Zhou and Declerck, 2019). Several studies have explored how the relatively stable stoichiometry of mixotrophic prey impacts predator growth and physiology. Overall, these studies show that elemental composition of mixotrophic prey is an important nutritional trait affecting zooplankton, although effects on predator performance are often contrasting. The C:N:P ratios of mixotrophs are generally lower than strict autotrophs [e.g. autotrophic Rhodomonas salina N:P = 18.6 ± 1.34 vs. mixotrophic Strombidium arenicola N:P = 10.3 ± 1.10 (Traboni et al., 2020)] and more stable (Maselli et al., 2022) than those of green algae and autotrophically-grown strains of the same species [at P-limited conditions, autotroph Karlodinium veneficum C:N:P ratio = 110:17 vs. mixotrophic K. veneficum C:N:P ratio = 96:15 (Traboni et al., 2021)]. However, there is substantial variability among mixotrophic species (Vad et al., 2020). Still, experimental studies highlight that feeding on mixotrophic prey improves (Katechakis et al., 2005; Trochine et al., 2019; Vad et al., 2021) or does not change (Vad et al., 2020) zooplankton growth and reproduction [egg production efficiency of Paracartia grani fed mixotrophic K. veneficum = 24.81 ± 1.16 eggs individual-1 day-1 vs. fed autotrophic K. veneficum = 27.13 ± 2.32 eggs individual-1 day-1 (Traboni et al., 2020)] due to more balanced nutrient ratios. Nevertheless, despite their ability to maintain more stable elemental ratios, utilizing mixotrophs as prey can also impair zooplankton grazer performance (Katechakis et al., 2005; Vad et al., 2020; Traboni et al., 2021), even leading to null growth rates (Katechakis et al., 2005). For example, other food quality traits such as poly-unsaturated fatty acid content, edibility, and toxicity, can therefore impact zooplankton growth more strongly than stoichiometry (Weithoff and Wacker, 2007). Scarcity of fatty acids and toxin production in mixotrophs, in particular when used as single prey, were often determined as detrimental for zooplankton grazers, regardless of the stoichiometric ratios (Katechakis et al., 2005; Traboni et al., 2021). Furthermore, prey size has been shown to constrain zooplankton performance, and specifically of copepods (Traboni et al., 2020, 2021). Ultimately, these studies suggest that the relatively low and stable elemental composition of mixotrophs is beneficial for zooplankton grazers, but additional non-stoichiometric food quality traits may have significant negative impacts on the predator response, potentially covering the positive stoichiometric effects.
Models highlight the impact of mixotrophs on global biogeochemistry
Modeling studies can provide insight into the large-scale impacts of mixotrophy. Previous studies have explored how the inclusion of mixotrophs and the manipulation of nutrient stoichiometry in model systems affect trophic transfer efficiency, nutrient dynamics, and global biogeochemical cycles. At the community level, mixotrophic nutrition allows nanoplankton to accumulate higher ratios of C:limiting elements, which is coupled with a shift toward larger size classes (Ward and Follows, 2016; Ho et al., 2020). However, such responses may be sensitive to the functional diversity of mixotrophs (Ghyoot et al., 2017; Mitra, 2024), with varying groups dominating depending on the light and nutrient regime (Leles et al., 2018). Carbon fixation patterns can also differ based on function type, with non-constitutive mixotrophs specifically providing a significant contribution to community dynamics and the C cycle (Mitra, 2024). Furthermore, grazing by mixotrophs can contribute to nutrient remineralisation and thus increase the abundance of primary producers and productivity (up to 17%), indirectly supporting marine copepods (Ghyoot et al., 2017). These ecosystem-scale findings have significant implications for global biogeochemistry, for food web dynamics [increase of up to 31% in trophic transfer efficiency (Ghyoot et al., 2017)] and for organic matter export (Mitra et al., 2014; Ward and Follows, 2016; Ghyoot et al., 2017; Leles et al., 2018), potentially leading to a ~35% increase in C flux to the deep ocean (Ward and Follows, 2016). Therefore, representing the mixotrophic fraction of the microbial community is essential for capturing nutrient dynamics in global biogeochemical models. As existing studies set one or several nutrient ratios as fixed parameters in their simulations, future work considering the dynamic nature of C:N:P ratios is necessary to further understand the role of mixotrophs at the global scale, particularly in the context of ongoing climate change and associated shifts in nutrient availability.
Ecological stoichiometry and toxicity in HAB-forming mixotrophs
Particular attention has been drawn to mixotrophic organisms that are known to trigger HABs (Burkholder et al., 2008). These events of high biomass and potential toxicity are produced by both autotrophic and mixotrophic protists, and occur globally with different frequency and intensity (Mitra and Flynn, 2010; Fu et al., 2012). Similar to other mixotrophs, high abundances of HAB-forming species were associated with nutrient imbalances in their environment [e.g., Myrionecta rubra (Herfort et al., 2012), Pyrodinium bahamense and Ceratium furca (Soler-Figueroa and Otero, 2015), Alexandrium ostenfeldii (Brandenburg et al., 2017), Prorocentrum minimum (Ajani et al., 2018), Noctiluca scintillans (Goes et al., 2020; Sarma et al., 2022), Lingoludinium polyedra (Domingues and Lima, 2023), Alexandrium minutum (Law et al., 2023), and Heterosigma akashiwo (Mardones et al., 2023) blooms]. Thus, the ability of using an organic nutrient source through phagotrophy when inorganic nutrients are limiting, as observed in K. veneficum (Huang et al., 2019; Li et al., 2022), may give a competitive advantage to bloom over purely photosynthetic species (Mulholland et al., 2018). Furthermore, the versatility of mixotrophic protists allows them to thrive in both nutrient-limited and eutrophic environments (Burkholder et al., 2008; Telesh et al., 2021). During nutrient limitation, species like K. veneficum (Huang et al., 2019; Li et al., 2022) can rely on phagotrophy, acquiring organic nutrients and gaining a competitive advantage over strict autotrophs (Mulholland et al., 2018). However, when eutrophication occurs, mixotrophs can rapidly capitalize on the increased availability of inorganic nutrients while still maintaining phagotrophic capabilities (Burkholder et al., 2008; Mitra and Flynn, 2010). This flexibility in nutrient acquisition strategies helps explain why HAB-forming species are associated with both nutrient-limited and nutrient-rich conditions (Flynn and Mitra, 2009; Mitra et al., 2016).
HABs pose a significant ecological and public health concern, primarily due to their potential to produce toxins that can accumulate in marine organisms and disrupt aquatic ecosystems. Among HAB-forming mixotrophs, the shift toward heterotrophic behavior was associated with the production of toxins, which supports assimilation of necessary nutrients through the kill/capture of prey, or as a by-product (Hambright et al., 2014; Blanco et al., 2015; Flood and Burkholder, 2018; Cagle et al., 2021; Vidyarathna et al., 2024). Variations in N and P supplies affected toxicity, despite these variations influencing differently depending on the organism. In nutrient replete conditions, Dinophysis norvegica produced five times more okadaic acid [1.01 ± 0.34 compared to 0.21 ± 0.08 pg cell-1 (Blanco et al., 2015)], while high abundances of Akashiwo sanguinea were shown to boost its allelopathic capacity both under nutrient enrichment and N:P ratio imbalance [~60% of inhibition rate (Yang et al., 2021)]. Prymnesium parvum, one of the most studied species for toxin production, was hypothesized to have evolved toxigenesis to support heterotrophic nutrient acquisition by reducing competition or assimilating necessary nutrients (Hambright et al., 2014; Flood and Burkholder, 2018; Cagle et al., 2021). The unbalanced dissolved N:P conditions that favored toxigenesis in P. parvum were suboptimal for growth and bloom formation (e.g., N:P = 100:1), while conditions that lead to higher growth rates were less favorable for toxigenesis (Hambright et al., 2014; Lundgren et al., 2016). The toxic capacity of these protists may also have serious consequences for grazers: when K. veneficum fed on prey with high N:P ratio (Lin et al., 2017) or Cochlodinium polykrikoides (Mulholland et al., 2009) were offered as prey to Crassostrea virginica oyster larvae and Cyprinodon variegates fish larvae, the mortality of both animals increased substantially, reaching even 100% in the case of the fish. Moreover, large amounts of carbon-rich exopolymer particles produced by Lepidodinium chlorophorum could be toxic for other members of the food web as bivalves (Roux et al., 2022). Such responses to shifts in nutrient limitation are especially important given that climate change and eutrophication are currently driving an increase in HABs (Glibert, 2020). As environmental conditions become more favorable for HAB-forming mixotrophs (Wells et al., 2020), it is important to better understand the mechanisms relating nutrient availability to mixotrophic metabolism and toxin production.
Conclusions
Future research priorities
The existing literature clearly highlights the interplay between mixotrophy and ecological stoichiometry and its significant impacts on both cellular and ecosystem scale processes. However, this mixotroph-stoichiometry link is still an understudied field, with many open scientific questions. Here, we identify several key gaps that require further research to progress our understanding of nutrient cycling within planktonic systems. First, few studies relating mixotrophy and stoichiometry have focused on differences among species functional types. Mixotrophs are often classified as constitutive or non-constitutive, depending on their ability to synthesize their own chloroplasts (Mitra et al., 2016). Yet, while many protozoa supplement their diet through photosynthesis by harboring symbiotic algae or temporarily acquiring chloroplasts from their prey (Stoecker et al., 2009), from the papers identified in our literature review, only six specifically addressed acquired phototrophy via kleptoplasty or symbiosis (Stabell et al., 2002; Flynn and Hansen, 2013; Takagi et al., 2019; Maselli et al., 2020; Wukovits et al., 2021; Mitra, 2024). Given the lack of knowledge on stoichiometric regulation in these organisms and the potential impacts of hosting symbionts or foreign chloroplasts on cell function, further work is necessary to understand the degree of homeostasis and the role of external nutrient ratios in driving internal composition and metabolism in non-constitutive mixotrophs.
Additional research should also explore how cellular elemental ratios may shift in response to climate change-induced global warming. In addition to a predicted increase in mixotrophy due to greater nutrient limitation, mixotrophs are hypothesized to increase their proportion of phagotrophic nutrition relative to photosynthesis at higher temperatures, as respiration becomes necessary to maintain metabolism (Allen et al., 2005; López-Urrutia et al., 2006; Wilken et al., 2013). Yet, while studies show that warming produces the expected impacts on metabolism, leading to increased growth and ingestion rates and lower primary productivity:bacterivory ratios, the results indicate little variation in stoichiometry (Lin et al., 2018; González-Olalla et al., 2019; Liu et al., 2021; Calbet and Saiz, 2022), which disagrees with both the growth rate hypothesis (Elser et al., 2003a; Flynn et al., 2010) and translation compensation hypotheses (Cotner et al., 2006; Toseland et al., 2013). Future studies are required to understand the cellular mechanisms resulting in this observed stability and to explain why these results differ from established hypotheses in the field of ecological stoichiometry. Moreover, the available laboratory studies were conducted under nutrient-replete conditions. Therefore, further research is needed to address the interactions between temperature and resource limitation and/or imbalance on mixotroph stoichiometry and homeostasis. Such work can provide insight into how mixotroph dynamics will change in the coming decades in response to anthropogenic stressors, and with significant potential implications for global carbon export and biogeochemistry.
Final remarks
In this review, we analyzed over 130 scientific articles to explore the relationship between elemental stoichiometry and mixotrophic protists, spanning from organismal to community scales. By examining experimental studies on different mixotrophic species, we illustrated how nutrient imbalances directly influence mixotrophic metabolism and internal homeostasis. The findings help to explain these protists’ ability to alternate between feeding strategies in response to nutrient manipulation, especially by increasing feeding rates on nutrient-rich prey. Moreover, the analyzed mesocosm experiments and field studies revealed a competitive advantage for mixotrophy under nutrient-limited conditions. Modeling studies provided further insight into the intricate food web interactions driven by mixotrophs, supported by common field measurements such as the sestonic ratios. Despite these diverse examples, the studies linking elemental stoichiometry with mixotrophy through quantitative variables represented less than 10% of the reviewed articles. Given the fundamental role of nutrient dynamics in mixotrophs’ ecological functioning, we emphasize the need to expand the application of the ecological stoichiometry framework to deepen our understanding of planktonic food webs and biogeochemical cycles in aquatic systems.
Data availability statement
The original contributions presented in the study are included in the article/Supplementary Material. Further inquiries can be directed to the corresponding author.
Author contributions
LS: Conceptualization, Data curation, Formal analysis, Methodology, Visualization, Writing – original draft, Writing – review & editing. ZSA: Conceptualization, Data curation, Formal analysis, Methodology, Visualization, Writing – original draft, Writing – review & editing. MG-M: Conceptualization, Data curation, Formal analysis, Methodology, Visualization, Writing – original draft, Writing – review & editing. AH: Conceptualization, Data curation, Formal analysis, Methodology, Visualization, Writing – original draft, Writing – review & editing. AR: Conceptualization, Data curation, Formal analysis, Methodology, Visualization, Writing – original draft, Writing – review & editing.
Funding
The author(s) declare that financial support was received for the research, authorship, and/or publication of this article. We thank the AWI for providing access to the guest research facilities of the Biologische Anstalt Helgoland during the Woodstoich Workshop, grant number AWI_BAH_34. LS was supported by the grant “SeeWandel-Climate: Modelling the consequences of climate change and neobiota for Lake Constance” (Grant no. ABH025) within the framework of the Interreg VI programme “Alpenrhein-Bodensee-Hochrhein (Germany/Austria/Switzerland/Liechtenstein)” which funds are provided by the European Regional Development Fund as well as the Swiss cantons. ZSA was supported by the National Science Foundation Graduate Research Fellowship (Grant No. 2141064) and by the Simons Collaboration on Computational Biogeochemical Modeling of Marine Ecosystems/CBIOMES (Grant no. 549931). MG-M was supported by Grant PID2020-118645RB-I00 by MICIU/AEI/10.13039/501100011033 and by Grant (PRE2021-096866) funded by MCIN/AEI/10.13039/501100011033 and by the FSE+. AH was supported by the European Union HORIZON EUROPE program ACTNOW: Advancing understanding of Cumulative Impacts on European marine biodiversity, ecosystem functions and services for human wellbeing (Grant no. 101060072).
Acknowledgments
We are grateful to the Woodstoich 5 organizers and participants for their support and great company before and during the event at the AWI - Biologische Anstalt Helgoland. We thank Cédric Meunier and Cecilia Laspoumaderes for giving precious feedback to early versions of this paper, as well as Esteban Balseiro and Beatriz Modenutti for the feedback in the project proposal, and the external reviewers who returned their reviews within the requested 24-h turnaround period.
Conflict of interest
The authors declare that the research was conducted in the absence of any commercial or financial relationships that could be construed as a potential conflict of interest.
Generative AI statement
The authors declare that no Generative AI was used in the creation of this manuscript.
Publisher’s note
All claims expressed in this article are solely those of the authors and do not necessarily represent those of their affiliated organizations, or those of the publisher, the editors and the reviewers. Any product that may be evaluated in this article, or claim that may be made by its manufacturer, is not guaranteed or endorsed by the publisher.
Supplementary material
The Supplementary Material for this article can be found online at: https://www.frontiersin.org/articles/10.3389/fevo.2024.1505037/full#supplementary-material
References
Ajani P. A., Larsson M. E., Woodcock S., Rubio A., Farrell H., Brett S., et al. (2018). Bloom drivers of the potentially harmful dinoflagellate Prorocentrum minimum (Pavillard) Schiller in a south eastern temperate Australian estuary. Estuarine Coast. Shelf Sci. 215, 161–171. doi: 10.1016/j.ecss.2018.09.029
Allen A. P., Gillooly J. F., Brown J. H. (2005). Linking the global carbon cycle to individual metabolism. Funct. Ecol. 19, 202–213. doi: 10.1111/j.1365-2435.2005.00952.x
Azam F., Fenchel T., Field J., Gray J., Meyer-Reil L., Thingstad F. (1983). The ecological role of water-column microbes in the sea. Mar. Ecol. Prog. Ser. 10, 257–263. doi: 10.3354/meps010257
Barbaglia G. S., Paight C., Honig M., Johnson M. D., Marczak R., Lepori-Bui M., et al. (2024). Environment-dependent metabolic investments in the mixotrophic chrysophyte Ochromonas. J. Phycology 60, 170–184. doi: 10.1111/jpy.13418
Blanco E., Karlsson C., Pallon J., Yasumoto T., Granéli E. (2015). Cellular nutrient content measured with the nuclear microprobe and toxins produced by Dinophysis norvegica (Dinophyceae) from the Trondheim fjord (Norway). Aquat. Microb. Ecol. 75, 259–269. doi: 10.3354/ame01762
Brandenburg K. M., Domis L. N. D. S., Wohlrab S., Krock B., John U., Van Scheppingen Y., et al. (2017). Combined physical, chemical and biological factors shape Alexandrium ostenfeldii blooms in The Netherlands. Harmful Algae 63, 146–153. doi: 10.1016/j.hal.2017.02.004
Burkholder J. M., Glibert P. M., Skelton H. M. (2008). Mixotrophy, a major mode of nutrition for harmful algal species in eutrophic waters. Harmful Algae 8, 77–93. doi: 10.1016/j.hal.2008.08.010
Cagle S. E., Roelke D. L., Muhl R. W. (2021). Allelopathy and micropredation paradigms reconcile with system stoichiometry. Ecosphere 12, e03372. doi: 10.1002/ecs2.3372
Calbet A., Saiz E. (2022). Thermal acclimation and adaptation in marine protozooplankton and mixoplankton. Front. Microbiol. 13. doi: 10.3389/fmicb.2022.832810
Caron D. A., Porter K. G., Sanders R. W. (1990). Carbon, nitrogen, and phosphorus budgets for the mixotrophic phytoflagellate Poterioochromonas malhamensis (Chrysophyceae) during bacterial ingestion. Limnology Oceanography 35, 433–443. doi: 10.4319/lo.1990.35.2.0433
Caron D. A., Sanders R. W., Lim E. L., Marrasé C., Amaral L. A., Whitney S., et al. (1993). Light-dependent phagotrophy in the freshwater mixotrophic chrysophyte Dinobryon cylindricum. Microbial Ecol. 25, 93–111. doi: 10.1007/BF00182132
Carpenter K. J., Bose M., Polerecky L., Lie A. A. Y., Heidelberg K. B., Caron D. A. (2018). Single-cell view of carbon and nitrogen acquisition in the mixotrophic alga Prymnesium parvum (Haptophyta) inferred from stable isotope tracers and nanoSIMS. Front. Mar. Sci. 5. doi: 10.3389/fmars.2018.00157
Carrillo P., Medina-Sánchez J. M., Villar-Argaiz M., Bullejos F. J., Durán C., Bastidas-Navarro M., et al. (2017). Vulnerability of mixotrophic algae to nutrient pulses and UVR in an oligotrophic Southern and Northern Hemisphere lake. Sci. Rep. 7, 6333. doi: 10.1038/s41598-017-06279-9
Chrzanowski T. H., Lukomski N. C., Grover J. P. (2010). Element stoichiometry of a mixotrophic protist grown under varying resource conditions. J. Eukaryotic Microbiol. 57, 322–327. doi: 10.1111/j.1550-7408.2010.00487.x
Cotner J. B., Makino W., Biddanda B. A. (2006). Temperature affects stoichiometry and biochemical composition of Escherichia coli. Microb. Ecol. 52, 26–33. doi: 10.1007/s00248-006-9040-1
Cruz-Rivera E., Hay M. E. (2000). Can quantity replace quality? food choice, compensatory feeding, and fitness of marine mesograzers. Ecology 81, 201–219. doi: 10.1890/0012-9658(2000)081[0201:CQRQFC]2.0.CO;2
De Lima D. T., Moser G. A. O., Piedras F. R., Cunha L. C. D., Tenenbaum D. R., Tenório M. M. B., et al. (2019). Abiotic changes driving microphytoplankton functional diversity in admiralty bay, king george island (Antarctica). Front. Mar. Sci. 6. doi: 10.3389/fmars.2019.00638
Domingues R. B., Lima M. J. (2023). Unusual red tide of the dinoflagellate Lingulodinium polyedra during an upwelling event off the Algarve coast (SW Iberia). Regional Stud. Mar. Sci. 63, 102998. doi: 10.1016/j.rsma.2023.102998
Edwards K. F. (2019). Mixotrophy in nanoflagellates across environmental gradients in the ocean. Proc. Natl. Acad. Sci. United States America 116, 6211–6220. doi: 10.1073/pnas.1814860116
Elser J. J., Acharya K., Kyle M., Cotner J., Makino W., Markow T., et al. (2003a). Growth rate-stoichiometry couplings in diverse biota. Ecol. Lett. 6, 936–943. doi: 10.1046/j.1461-0248.2003.00518.x
Elser J. J., Kyle M., Makino W., Yoshida T., Urabe J. (2003b). Ecological stoichiometry in the microbial food web: a test of the light:nutrient hypothesis. Aquat. Microb. Ecol. 31, 49–65. doi: 10.3354/ame031049
Faithfull C. L., Wenzel A., Vrede T., Bergström A.-K. (2011). Testing the light: nutrient hypothesis in an oligotrophic boreal lake. Ecosphere 2, art123. doi: 10.1890/ES11-00223.1
Falkowski P. G., Barber R. T., Smetacek V. (1998). Biogeochemical controls and feedbacks on ocean primary production. Science 281, 200–206. doi: 10.1126/science.281.5374.200
Finkel Z. V., Follows M. J., Liefer J. D., Brown C. M., Benner I., Irwin A. J. (2016). Phylogenetic diversity in the macromolecular composition of microalgae. PloS One 11, 1–16. doi: 10.1371/journal.pone.0155977
Fischer R., Giebel H., Hillebrand H., Ptacnik R. (2017). Importance of mixotrophic bacterivory can be predicted by light and loss rates. Oikos 126, 713–722. doi: 10.1111/oik.03539
Fischer R., Kitzwögerer J., Ptacnik R. (2022). Light-dependent niche differentiation in two mixotrophic bacterivores. Environ. Microbiol. Rep. 14, 530–537. doi: 10.1111/1758-2229.13071
Flood S. L., Burkholder J. M. (2018). Imbalanced nutrient regimes increase Prymnesium parvum resilience to herbicide exposure. Harmful Algae 75, 57–74. doi: 10.1016/j.hal.2018.04.006
Flynn K. J., Hansen P. J. (2013). Cutting the canopy to defeat the “Selfish gene”; conflicting selection pressures for the integration of phototrophy in mixotrophic protists. Protist 164, 811–823. doi: 10.1016/j.protis.2013.09.002
Flynn K. J., Mitra A. (2009). Building the “perfect beast”: modelling mixotrophic plankton. J. Plankton Res. 31, 965–992. doi: 10.1093/plankt/fbp044
Flynn K. J., Mitra A., Anestis K., Anschütz A. A., Calbet A., Ferreira G. D., et al. (2019). Mixotrophic protists and a new paradigm for marine ecology: where does plankton research go now? J. Plankton Res. 41, 375–391. doi: 10.1093/plankt/fbz026
Flynn K. J., Raven J. A., Rees T. A. V., Finkel Z., Quigg A., Beardall J. (2010). Is the growth rate hypothesis applicable to microalgae? J. Phycology 46, 1–12. doi: 10.1111/j.1529-8817.2009.00756.x
Fu F., Tatters A., Hutchins D. (2012). Global change and the future of harmful algal blooms in the ocean. Mar. Ecol. Prog. Ser. 470, 207–233. doi: 10.3354/meps10047
Galbraith E. D., Martiny A. C. (2015). A simple nutrient-dependence mechanism for predicting the stoichiometry of marine ecosystems. Proc. Natl. Acad. Sci. U.S.A 112, 8199–8204. doi: 10.1073/pnas.1423917112
Geider R., La Roche J. (2002). Redfield revisited: variability of C:N:P in marine microalgae and its biochemical basis. Eur. J. Phycology 37, 1–17. doi: 10.1017/S0967026201003456
Ghyoot C., Lancelot C., Flynn K. J., Mitra A., Gypens N. (2017). Introducing mixotrophy into a biogeochemical model describing an eutrophied coastal ecosystem: The Southern North Sea. Prog. Oceanography 157, 1–11. doi: 10.1016/j.pocean.2017.08.002
Glibert P. M. (2020). Harmful algae at the complex nexus of eutrophication and climate change. Harmful Algae 91, 101583. doi: 10.1016/j.hal.2019.03.001
Goes J. I., Tian H., Gomes H. D. R., Anderson O. R., Al-Hashmi K., deRada S., et al. (2020). Ecosystem state change in the Arabian Sea fuelled by the recent loss of snow over the Himalayan-Tibetan Plateau region. Sci. Rep. 10, 7422. doi: 10.1038/s41598-020-64360-2
Golz A.-L., Burian A., Winder M. (2015). Stoichiometric regulation in micro- and mesozooplankton. J. Plankton Res. 37, 293–305. doi: 10.1093/plankt/fbu109
González-Olalla J. M., Medina-Sánchez J. M., Carrillo P. (2019). Mixotrophic trade-off under warming and UVR in a marine and a freshwater alga. J. Phycology 55, 1028–1040. doi: 10.1111/jpy.12865
González-Olalla J. M., Medina-Sánchez J. M., Lozano I. L., Villar-Argaiz M., Carrillo P. (2018). Climate-driven shifts in algal-bacterial interaction of high-mountain lakes in two years spanning a decade. Sci. Rep. 8, 10278. doi: 10.1038/s41598-018-28543-2
González-Olalla J. M., Medina-Sánchez J. M., Norici A., Carrillo P. (2021). Regulation of phagotrophy by prey, low nutrients, and low light in the mixotrophic haptophyte isochrysis galbana. Microb. Ecol. 82, 981–993. doi: 10.1007/s00248-021-01723-w
Hambright K. D., Easton J. D., Zamor R. M., Beyer J., Easton A. C., Allison B. (2014). Regulation of growth and toxicity of a mixotrophic microbe: implications for understanding range expansion in Prymnesium parvum. Freshw. Sci. 33, 745–754. doi: 10.1086/677198
Hamsher S. E., Ellis K., Holen D., Sanders R. W. (2020). Effects of light, dissolved nutrients and prey on ingestion and growth of a newly identified mixotrophic alga, Chrysolepidomonas dendrolepidota (Chrysophyceae). Hydrobiologia 847, 2923–2932. doi: 10.1007/s10750-020-04293-z
Hartmann M., Grob C., Tarran G. A., Martin A. P., Burkill P. H., Scanlan D. J., et al. (2012). Mixotrophic basis of Atlantic oligotrophic ecosystems. Proc. Natl. Acad. Sci. 109, 5756–5760. doi: 10.1073/pnas.1118179109
Herfort L., Peterson T. D., Prahl F. G., McCue L. A., Needoba J. A., Crump B. C., et al. (2012). Red waters of myrionecta rubra are biogeochemical hotspots for the columbia river estuary with impacts on primary/secondary productions and nutrient cycles. Estuaries Coasts 35, 878–891. doi: 10.1007/s12237-012-9485-z
Hessen D. O., Elser J. J., Sterner R. W., Urabe J. (2013). Ecological stoichiometry: An elementary approach using basic principles. Limnology Oceanography 58, 2219–2236. doi: 10.4319/lo.2013.58.6.2219
Ho P.-C., Chang C.-W., Shiah F.-K., Wang P.-L., Hsieh C., Andersen K. H. (2020). Body size, light intensity, and nutrient supply determine plankton stoichiometry in mixotrophic plankton food webs. Am. Nat. 195, E100–E111. doi: 10.1086/707394
Huang H.-L., Shao Q.-W., Zhu X.-J., Luo J., Meng R., Zhou C.-X., et al. (2019). Distribution of Karlodinium veneficum in the coastal region of Xiangshan Bay in the East China Sea, as detected by a real-time quantitative PCR assay of ribosomal ITS sequence. Harmful Algae 81, 65–76. doi: 10.1016/j.hal.2018.12.001
Inomura K., Omta A. W., Talmy D., Bragg J., Deutsch C., Follows M. J. (2020). A mechanistic model of macromolecular allocation, elemental stoichiometry, and growth rate in phytoplankton. Front. Microbiol. 11. doi: 10.3389/fmicb.2020.00086
Jäger C. G., Vrede T., Persson L., Jansson M. (2014). Interactions between metazoans, autotrophs, mixotrophs and bacterioplankton in nutrient-depleted high DOC environments: a long-term experiment. Freshw. Biol. 59, 1596–1607. doi: 10.1111/fwb.12366
Jones H. (1997). A classification of mixotrophic protists based on their behaviour. Freshw. Biol. 37, 35–43. doi: 10.1046/j.1365-2427.1997.00138.x
Jones H. L. J., Durjun P., Leadbeater B. S. C., Green J. C. (1995). The relationship between photoacclimation and phagotrophy with respect to chlorophyll a, carbon and nitrogen content, and cell size of Chrysochromulina brevifilum (Prymnesiophyceae). Phycologia 34, 128–134. doi: 10.2216/i0031-8884-34-2-128.1
Karpowicz M., Feniova I., Gladyshev M. I., Ejsmont-Karabin J., Górniak A., Zieliński P., et al. (2019). The stoichiometric ratios (C:N:P) in a pelagic food web under experimental conditions. Limnologica 77, 125690. doi: 10.1016/j.limno.2019.125690
Katechakis A., Haseneder T., Kling R., Stibor H. (2005). Mixotrophic versus photoautotrophic specialist algae as food for zooplankton: The light: nutrient hypothesis might not hold for mixotrophs. Limnology Oceanography 50, 1290–1299. doi: 10.4319/lo.2005.50.4.1290
Katechakis A., Stibor H. (2006). The mixotroph Ochromonas tuberculata may invade and suppress specialist phago- and phototroph plankton communities depending on nutrient conditions. Oecologia 148, 692–701. doi: 10.1007/s00442-006-0413-4
Klausmeier C. A., Litchman E., Daufresne T., Levin S. A. (2004). Optimal nitrogen-to-phosphorus stoichiometry of phytoplankton. Nature 429, 171–174. doi: 10.1038/nature02454
Koojiman S. A. L. M. (1995). The stoichiometry of animal energetics. J. Theor. Biol. 177, 139–149. doi: 10.1006/jtbi.1995.0232
Lagus A., Suomela J., Weithoff G., Heikkola K., Helminen S., Sipura J. (2004). Species-specific differences in phytoplankton responses to N and P enrichments and the N:P ratio in the Archipelago Sea, northern Baltic Sea. J. Plankton Res. 26, 779–798. doi: 10.1093/plankt/fbh070
Lavrentyev P., Bootsma H., Johengen T., Cavaletto J., Gardner W. (1998). Microbial plankton response to resource limitation:insights from the community structure and seston stoichiometry in Florida Bay, USA. Mar. Ecol. Prog. Ser. 165, 45–57. doi: 10.3354/meps165045
Law I. K., Hii K. S., Lau W. L. S., Leaw C. P., Lim P. T. (2023). Coastal micro-phytoplankton community changes during the toxigenic Alexandrium minutum blooms in a semi-enclosed tropical coastal lagoon (Malaysia, South China Sea). Regional Stud. Mar. Sci. 57, 102733. doi: 10.1016/j.rsma.2022.102733
Leles S. G., Polimene L., Bruggeman J., Blackford J., Ciavatta S., Mitra A., et al. (2018). Modelling mixotrophic functional diversity and implications for ecosystem function. J. Plankton Res. 40, 627–642. doi: 10.1093/plankt/fby044
Leonardos N., Geider R. J. (2005). Elemental and biochemical composition of rhinomonas reticulata (Cryptophyta) in relation to light and nitrate-to-phosphate supply ratios. J. Phycology 41, 567–576. doi: 10.1111/j.1529-8817.2005.00082.x
Leruste A., Garrido M., Malet N., Bec B., De Wit R., Cecchi P., et al. (2021). Impact of nutrient availability on the trophic strategies of the planktonic protist communities in a disturbed Mediterranean coastal lagoon. Hydrobiologia 848, 1101–1119. doi: 10.1007/s10750-021-04517-w
Li A., Stoecker D. K., Coats D. W. (2000). Mixotrophy in Gyrodinium galatheanum (Dinophyceae): grazing responses to light intensity and inorganic nutrients. J. Phycology 36, 33–45. doi: 10.1046/j.1529-8817.2000.98076.x
Li M., Chen Y., Zhang F., Song Y., Glibert P. M., Stoecker D. K. (2022). A three-dimensional mixotrophic model of Karlodinium veneficum blooms for a eutrophic estuary. Harmful Algae 113, 102203. doi: 10.1016/j.hal.2022.102203
Lin C., Accoroni S., Glibert P. (2017). Karlodinium veneficum feeding responses and effects on larvae of the eastern oyster Crassostrea virginica under variable nitrogen:phosphorus stoichiometry. Aquat. Microb. Ecol. 79, 101–114. doi: 10.3354/ame01823
Lin C.-H., Flynn K. J., Mitra A., Glibert P. M. (2018). Simulating effects of variable stoichiometry and temperature on mixotrophy in the harmful dinoflagellate karlodinium veneficum. Front. Mar. Sci. 5. doi: 10.3389/fmars.2018.00320
Liu K., Ng H. Y.-T., Gao Z., Liu H. (2022). Selective feeding of a mixotrophic dinoflagellate (Lepidodinium sp.) in response to experimental warming and inorganic nutrient imbalance. Front. Microbiol. 13. doi: 10.3389/fmicb.2022.805306
Liu K., Ng H., Zhang S., Liu H. (2021). Effects of temperature on a mixotrophic dinoflagellate (Lepidodinium sp.) under different nutritional strategies. Mar. Ecol. Prog. Ser. 678, 37–49. doi: 10.3354/meps13865
López-Urrutia Á., San Martin E., Harris R. P., Irigoien X. (2006). Scaling the metabolic balance of the oceans. Proc. Natl. Acad. Sci. 103, 8739–8744. doi: 10.1073/pnas.0601137103
Lundgren V. M., Glibert P. M., Granéli E., Vidyarathna N. K., Fiori E., Ou L., et al. (2016). Metabolic and physiological changes in Prymnesium parvum when grown under, and grazing on prey of, variable nitrogen:phosphorus stoichiometry. Harmful Algae 55, 1–12. doi: 10.1016/j.hal.2016.01.002
Mardones J. I., Paredes-Mella J., Flores-Leñero A., Yarimizu K., Godoy M., Artal O., et al. (2023). Extreme harmful algal blooms, climate change, and potential risk of eutrophication in Patagonian fjords: Insights from an exceptional Heterosigma akashiwo fish-killing event. Prog. Oceanography 210, 102921. doi: 10.1016/j.pocean.2022.102921
Maselli M., Altenburger A., Stoecker D. K., Hansen P. J. (2020). Ecophysiological traits of mixotrophic Strombidium spp. J. Plankton Res. 42, 485–496. doi: 10.1093/plankt/fbaa041
Maselli M., Van De Waal D. B., Hansen P. J. (2022). Impacts of inorganic nutrients on the physiology of a mixoplanktonic ciliate and its cryptophyte prey. Oecologia 199, 41–52. doi: 10.1007/s00442-022-05162-3
Meunier C. L., Hantzsche F. M., Cunha-Dupont A.Ö., Haafke J., Oppermann B., Malzahn A. M., et al. (2012). Intraspecific selectivity, compensatory feeding and flexible homeostasis in the phagotrophic flagellate Oxyrrhis marina: three ways to handle food quality fluctuations. Hydrobiologia 680, 53–62. doi: 10.1007/s10750-011-0900-4
Millette N. C., Gast R. J., Luo J. Y., Moeller H. V., Stamieszkin K., Andersen K. H., et al. (2023). Mixoplankton and mixotrophy: future research priorities. J. Plankton Res. 45, 576–596. doi: 10.1093/plankt/fbad020
Mitra A. (2024). Importance of dynamics of acquired phototrophy amongst mixoplankton; a unique example of essential nutrient transmission in community ecology. Community Ecol. doi: 10.1007/s42974-024-00202-9
Mitra A., Flynn K. J. (2010). Modelling mixotrophy in harmful algal blooms: More or less the sum of the parts? J. Mar. Syst. 83, 158–169. doi: 10.1016/j.jmarsys.2010.04.006
Mitra A., Flynn K. J. (2023). Low rates of bacterivory enhances phototrophy and competitive advantage for mixoplankton growing in oligotrophic waters. Sci. Rep. 13, 6900. doi: 10.1038/s41598-023-33962-x
Mitra A., Flynn K. J., Burkholder J. M., Berge T., Calbet A., Raven J. A., et al. (2014). The role of mixotrophic protists in the biological carbon pump. Biogeosciences 11, 995–1005. doi: 10.5194/bg-11-995-2014
Mitra A., Flynn K. J., Tillmann U., Raven J. A., Caron D., Stoecker D. K., et al. (2016). Defining planktonic protist functional groups on mechanisms for energy and nutrient acquisition: incorporation of diverse mixotrophic strategies. Protist 167, 106–120. doi: 10.1016/j.protis.2016.01.003
Moorthi S., Ptacnik R., Sanders R., Fischer R., Busch M., Hillebrand H. (2017). The functional role of planktonic mixotrophs in altering seston stoichiometry. Aquat. Microb. Ecol. 79, 235–245. doi: 10.3354/ame01832
Mulholland M. R., Morse R. E., Boneillo G. E., Bernhardt P. W., Filippino K. C., Procise L. A., et al. (2009). Understanding causes and impacts of the dinoflagellate, cochlodinium polykrikoides, blooms in the chesapeake bay. Estuaries Coasts 32, 734–747. doi: 10.1007/s12237-009-9169-5
Mulholland M. R., Morse R., Egerton T., Bernhardt P. W., Filippino K. C. (2018). Blooms of dinoflagellate mixotrophs in a lower chesapeake bay tributary: carbon and nitrogen uptake over diurnal, seasonal, and interannual timescales. Estuaries Coasts 41, 1744–1765. doi: 10.1007/s12237-018-0388-5
Novotná J., Nedbalová L., Kopáček J., Vrba J. (2010). Cell-specific extracellular phosphatase activity of dinoflagellate populations in acidified mountain lakes: phosphatases of dinophyte species. J. Phycology 46, 635–644. doi: 10.1111/j.1529-8817.2010.00858.x
Nygaard K., Tobiesen A. (1993). Bacterivory in algae: A survival strategy during nutrient limitation. Limnology Oceanography 38, 273–279. doi: 10.4319/lo.1993.38.2.0273
O’Dea R. E., Lagisz M., Jennions M. D., Koricheva J., Noble D. W. A., Parker T. H., et al. (2021). Preferred reporting items for systematic reviews and meta-analyses in ecology and evolutionary biology: a PRISMA extension. Biol. Rev. 96, 1695–1722. doi: 10.1111/brv.12721
Persson J., Fink P., Goto A., Hood J. M., Jonas J., Kato S. (2010). To be or not to be what you eat: regulation of stoichiometric homeostasis among autotrophs and heterotrophs. Oikos 119, 741–751. doi: 10.1111/j.1600-0706.2009.18545.x
Pomeroy J. W., Gray D. M., Brown T., Hedstrom N. R., Quinton W. L., Granger R. J., et al. (2007). The cold regions hydrological model: a platform for basing process representation and model structure on physical evidence. Hydrological Processes 21, 2650–2667. doi: 10.1002/hyp.6787
Princiotta S. D., Smith B. T., Sanders R. W. (2016). Temperature-dependent phagotrophy and phototrophy in a mixotrophic chrysophyte. J. Phycology 52, 432–440. doi: 10.1111/jpy.12405
Ptacnik R., Sommer U., Hansen T., Martens V. (2004). Effects of microzooplankton and mixotrophy in an experimental planktonic food web. Limnology Oceanography 49, 1435–1445. doi: 10.4319/lo.2004.49.4_part_2.1435
Roux P., Siano R., Souchu P., Collin K., Schmitt A., Manach S., et al. (2022). Spatio-temporal dynamics and biogeochemical properties of green seawater discolorations caused by the marine dinoflagellate Lepidodinium chlorophorum along southern Brittany coast. Estuarine Coast. Shelf Sci. 275, 107950. doi: 10.1016/j.ecss.2022.107950
Sanders R. W., Porter K. G., Caron D. A. (1990). Relationship between phototrophy and phagotrophy in the mixotrophic chrysophyte Poterioochromonas malhamensis. Microb. Ecol. 19, 97–109. doi: 10.1007/BF02015056
Sarma N. S., Baliarsingh S. K., Pandi S. R., Lotliker A. A., Samanta A. (2022). Noctiluca blooms intensify when northwesterly winds complement northeasterlies in the northern Arabian Sea: Possible implications. Oceanologia 64, 717–734. doi: 10.1016/j.oceano.2022.06.004
Schenone L., Balseiro E., Modenutti B. (2022). Light dependence in the phototrophy–phagotrophy balance of constitutive and non-constitutive mixotrophic protists. Oecologia 200, 295–306. doi: 10.1007/s00442-022-05226-4
Schenone L., Modenutti B., Martyniuk N., Bastidas Navarro M., Laspoumaderes C., Balseiro E. (2021). Modelling key variables for understanding the effects of grazing and nutrient recycling by zooplankton on the freshwater microbial loop. Freshw. Biol. 66, 2322–2337. doi: 10.1111/fwb.13835
Sherr E. B., Sherr B. F. (2002). Significance of predation by protists in aquatic microbial food webs. Antonie van Leeuwenhoek 81, 293–308. doi: 10.1023/A:1020591307260
Smalley G. W., Coats D. W. (2002). Ecology of the red-tide dinoflagellate ceratium furca: distribution, mixotrophy, and grazing impact on ciliate populations of chesapeake bay. J. Eukaryotic Microbiol. 49, 63–73. doi: 10.1111/j.1550-7408.2002.tb00343.x
Smalley G. W., Coats D. W., Stoecker D. K. (2003). Feeding in the mixotrophic dinoflagellate Ceratium furca is influenced by intracellular nutrient concentrations. Mar. Ecol. Prog. Ser. 262, 137–151. doi: 10.3354/meps262137
Smalley G. W., Coats D. W., Stoecker D. K. (2012). Influence of inorganic nutrients, irradiance, and time of day on food uptake by the mixotrophic dinoflagellate Neoceratium furca. Aquat. Microbial Ecol. 68, 29–41. doi: 10.3354/ame01599
Soler-Figueroa B. M., Otero E. (2015). The influence of rain regimes and nutrient loading on the abundance of two dinoflagellate species in a tropical bioluminescent bay, bahía fosforescente, la parguera, Puerto Rico. Estuaries Coasts 38, 84–92. doi: 10.1007/s12237-014-9827-0
Stabell T., Andersen T., Klaveness D. (2002). Ecological significance of endosymbionts in a mixotrophic ciliate–an experimental test of a simple model of growth coordination between host and symbiont. J. Plankton Res. 24, 889–899. doi: 10.1093/plankt/24.9.889
Sterner R. W., Elser J. J. (2002). Ecological Stoichiometry: The Biology Of Elements From Molecules To The Biosphere. (William Street Princeton, New Jersey: Princeton University Press).
Sterner R. W., Elser J. J., Fee E. J., Guildford S. J., Chrzanowski T. H. (1997). The light: nutrient ratio in lakes: the balance of energy and materials affects ecosystem structure and process. Am. Nat. 150, 663–684. doi: 10.1086/286088
Stoecker D. K. (1998). Conceptual models of mixotrophy in planktonic protists and some ecological and evolutionary implications. Eur. J. Protistology 34, 281–290. doi: 10.1016/S0932-4739(98)80055-2
Stoecker D. K., Hansen P. J., Caron D. A., Mitra A. (2017). Mixotrophy in the marine plankton. Annu. Rev. Mar. Sci. 9, 311–335. doi: 10.1146/annurev-marine-010816-060617
Stoecker D., Johnson M., deVargas C., Not F. (2009). Acquired phototrophy in aquatic protists. Aquat. Microb. Ecol. 57, 279–310. doi: 10.3354/ame01340
Takagi H., Kimoto K., Fujiki T., Saito H., Schmidt C., Kucera M., et al. (2019). Characterizing photosymbiosis in modern planktonic foraminifera. Biogeosciences 16, 3377–3396. doi: 10.5194/bg-16-3377-2019
Telesh I., Schubert H., Skarlato S. (2021). Abiotic stability promotes dinoflagellate blooms in marine coastal ecosystems. Estuarine Coast. Shelf Sci. 251, 107239. doi: 10.1016/j.ecss.2021.107239
Toseland A., Daines S. J., Clark J. R., Kirkham A., Strauss J., Uhlig C., et al. (2013). The impact of temperature on marine phytoplankton resource allocation and metabolism. Nat. Climate Change 3, 979–984. doi: 10.1038/nclimate1989
Traboni C., Calbet A., Saiz E. (2020). Effects of prey trophic mode on the gross-growth efficiency of marine copepods: the case of mixoplankton. Sci. Rep. 10, 12259. doi: 10.1038/s41598-020-69174-w
Traboni C., Calbet A., Saiz E. (2021). Mixotrophy upgrades food quality for marine calanoid copepods. Limnology Oceanography 66, 4125–4139. doi: 10.1002/lno.11948
Trochine C., Díaz Villanueva V., Balseiro E., Modenutti B. (2019). Nutritional stress by means of high C:N ratios in the diet and starvation affects nitrogen isotope ratios and trophic fractionation of omnivorous copepods. Oecologia 190, 547–557. doi: 10.1007/s00442-019-04438-5
Vad C. F., Schneider C., Fischer R., Kainz M. J., Ptacnik R. (2021). From adverse to beneficial: Contrasting dietary effects of freshwater mixotrophs on zooplankton. Freshw. Biol. 66, 2272–2282. doi: 10.1111/fwb.13832
Vad C. F., Schneider C., Lukić D., Horváth Z., Kainz M. J., Stibor H., et al. (2020). Grazing resistance and poor food quality of a widespread mixotroph impair zooplankton secondary production. Oecologia 193, 489–502. doi: 10.1007/s00442-020-04677-x
Vidyarathna N. K., Smith L. E., Miller K. R., Coyne K. J., Cohen J. H., Warner M. E. (2024). Short-term and long-term exposure to combined elevated temperature and CO2 leads to differential growth, toxicity, and fatty acid profiles in the harmful dinoflagellate Karlodinium veneficum. Front. Mar. Sci. 11. doi: 10.3389/fmars.2024.1305495
Ward B. A., Follows M. J. (2016). Marine mixotrophy increases trophic transfer efficiency, mean organism size, and vertical carbon flux. Proc. Natl. Acad. Sci. U.S.A 113, 2958–2963. doi: 10.1073/pnas.1517118113
Wei C., Wang H., Ma M., Hu Q., Gong Y. (2020). Factors affecting the mixotrophic flagellate poterioochromonas malhamensis grazing on chlorella cells. J. Eukaryotic Microbiol. 67, 190–202. doi: 10.1111/jeu.12770
Weithoff G., Wacker A. (2007). The mode of nutrition of mixotrophic flagellates determines the food quality for their consumers. Funct. Ecol. 21, 1092–1098. doi: 10.1111/j.1365-2435.2007.01333.x
Wells M. L., Karlson B., Wulff A., Kudela R., Trick C., Asnaghi V., et al. (2020). Future HAB science: Directions and challenges in a changing climate. Harmful Algae 91, 101632. doi: 10.1016/j.hal.2019.101632
Wickham S. A., Wimmer R. (2019). Does Mixotrophy in ciliates compensate for poor-quality prey? Experiments with heterotrophic–mixotrophic species pairs. J. Plankton Res. 41, 583–593. doi: 10.1093/plankt/fbz052
Wilken S., Huisman J., Naus-Wiezer S., Van Donk E. (2013). Mixotrophic organisms become more heterotrophic with rising temperature. Ecol. Lett. 16, 225–233. doi: 10.1111/ele.12033
Wilken S., Schuurmans J. M., Matthijs H. C. P. (2014a). Do mixotrophs grow as photoheterotrophs? Photophysiological acclimation of the chrysophyte Ochromonas danica after feeding. New Phytol. 204, 882–889. doi: 10.1111/nph.12975
Wilken S., Verspagen J. M. H., Naus-Wiezer S., Van Donk E., Huisman J. (2014b). Biological control of toxic cyanobacteria by mixotrophic predators: an experimental test of intraguild predation theory. Ecol. Appl. 24, 1235–1249. doi: 10.1890/13-0218.1
Worden A. Z., Follows M. J., Giovannoni S. J., Wilken S., Zimmerman A. E., Keeling P. J. (2015). Rethinking the marine carbon cycle: Factoring in the multifarious lifestyles of microbes. Science 347, 1257594. doi: 10.1126/science.1257594
Wukovits J., Enge A., Bukenberger P., Wanek W., Watzka M., Heinz P. (2021). Phytodetrital quality (C:N ratio) and temperature changes affect C and N cycling of the intertidal mixotrophic foraminifer Haynesina germanica. Aquat. Biol. 30, 119–132. doi: 10.3354/ab00746
Yang Y., Huang B., Tang Y., Xu N. (2021). Allelopathic effects of mixotrophic dinoflagellate Akashiwo sanGuinea on co-occurring phytoplankton: the significance of nutritional ecology. J. Ocean. Limnol 39, 903–917. doi: 10.1007/s00343-020-0132-4
Yu Q., Chen Q., Elser J. J., He N., Wu H., Zhang G., et al. (2010). Linking stoichiometric homoeostasis with ecosystem structure, functioning and stability. Ecol. Lett. 13, 1390–1399. doi: 10.1111/j.1461-0248.2010.01532.x
Yu Q., Wilcox K., Pierre K. L., Knapp A. K., Han X., Smith M. D. (2015). Stoichiometric homeostasis predicts plant species dominance, temporal stability, and responses to global change. Ecology 96, 2328–2335. doi: 10.1890/14-1897.1
Yvon-Durocher G., Schaum C. E., Trimmer M. (2017). The temperature dependence of phytoplankton stoichiometry: Investigating the roles of species sorting and local adaptation. Front. Microbiol. 8. doi: 10.3389/fmicb.2017.02003
Zhou L., Declerck S. A. J. (2019). Herbivore consumers face different challenges along opposite sides of the stoichiometric knife-edge. Ecol. Lett. 22, 2018–2027. doi: 10.1111/ele.13386
Keywords: mixotrophy, protist, stoichiometry, nutrient limitation, C:N:P ratios, homeostasis, food web dynamics, harmful algal blooms
Citation: Schenone L, Aarons ZS, García-Martínez M, Happe A and Redoglio A (2024) Mixotrophic protists and ecological stoichiometry: connecting homeostasis and nutrient limitation from organisms to communities. Front. Ecol. Evol. 12:1505037. doi: 10.3389/fevo.2024.1505037
Received: 02 October 2024; Accepted: 04 November 2024;
Published: 25 November 2024.
Edited by:
Cedric Leo Meunier, Alfred Wegener Institute Helmholtz Centre for Polar and Marine Research (AWI), GermanyReviewed by:
James Cotner, University of Minnesota Twin Cities, United StatesElena Litchman, Michigan State University, United States
Jana Isanta-Navarro, University of Copenhagen, Denmark
Copyright © 2024 Schenone, Aarons, García-Martínez, Happe and Redoglio. This is an open-access article distributed under the terms of the Creative Commons Attribution License (CC BY). The use, distribution or reproduction in other forums is permitted, provided the original author(s) and the copyright owner(s) are credited and that the original publication in this journal is cited, in accordance with accepted academic practice. No use, distribution or reproduction is permitted which does not comply with these terms.
*Correspondence: Luca Schenone, bHVjYXNjaGVub25lQGNvbWFodWUtY29uaWNldC5nb2IuYXI=