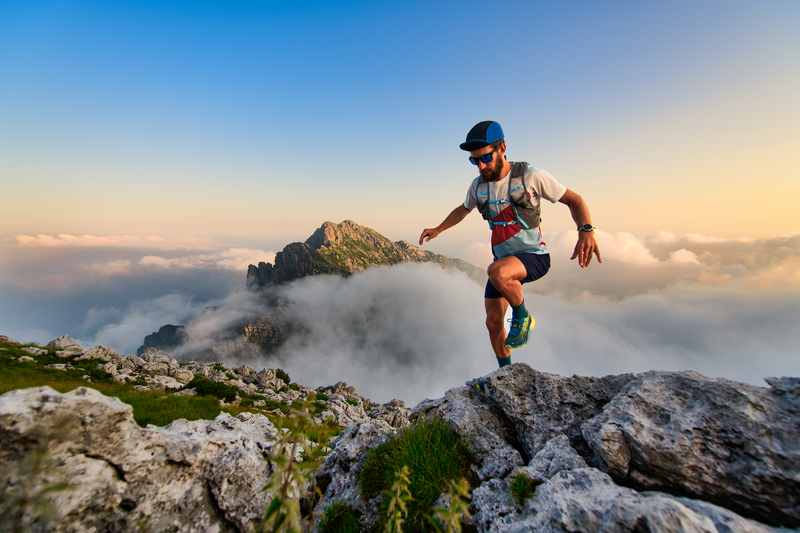
95% of researchers rate our articles as excellent or good
Learn more about the work of our research integrity team to safeguard the quality of each article we publish.
Find out more
ORIGINAL RESEARCH article
Front. Ecol. Evol. , 10 August 2023
Sec. Coevolution
Volume 11 - 2023 | https://doi.org/10.3389/fevo.2023.1079271
This article is part of the Research Topic Holobiont Interactions View all 10 articles
Coral reefs are diverse marine ecosystems that have tremendous ecological and cultural value and support more than 25% of eukaryote marine biodiversity. Increased ocean temperatures and light intensity trigger coral bleaching, the breakdown of the relationship between corals and their photosymbionts, dinoflagellates of the family Symbiodiniaceae. This leaves corals without their primary energy source, thereby leading to starvation and, often, death. Coral bleaching is hypothesized to occur due to an overproduction of reactive oxygen species (ROS) by Symbiodiniaceae, which subsequently accumulate in coral tissues. Bacterial probiotics have been proposed as an approach to mitigate coral bleaching, by reducing ROS levels in the coral holobiont through bacterial antioxidant production. Both corals and Symbiodiniaceae are known to associate with bacteria. However, the Symbiodiniaceae-bacteria relationship, and its impact on Symbiodiniaceae thermal tolerance, remains a poorly studied area. In this study, cultured Symbiodiniaceae of the species Breviolum minutum were treated with antibiotics to reduce their bacterial load. The cultures were subsequently inoculated with bacterial isolates from the genus Roseovarius that were isolated from the same B. minutum culture and showed either high or low ROS-scavenging abilities. The B. minutum cultures were then exposed to experimental heat stress for 16 days, and their health was monitored through measurements of cell density and photochemical efficiency of photosystem II. It was found that B. minutum inoculated with Roseovarius with higher ROS-scavenging abilities showed greater cell growth at elevated temperatures, compared to cultures inoculated with a Roseovarius strain with lower ROS-scavenging abilities. This suggests that Roseovarius may play a role in Symbiodiniaceae fitness at elevated temperatures. Analysis of Symbiodiniaceae-associated bacterial communities through 16S rRNA gene metabarcoding revealed that Roseovarius relative abundance increased in B. minutum cultures following inoculation and with elevated temperature exposure, highlighting the contribution they may have in shielding B. minutum from thermal stress, although other bacterial community changes may have also contributed to these observations. This study begins to unpick the relationship between Symbiodiniaceae and their bacteria and opens the door for the use of Symbiodiniaceae-associated bacteria in coral reef conservation approaches.
Coral reefs are diverse marine ecosystems with highly beneficial ecological functions, such as providing protection of coastlines from degradation, and housing fish which humans harvest for nutrition and economic growth (Cesar et al., 2003). Reef-building corals associate with photosymbionts, dinoflagellate algae in the family Symbiodiniaceae (LaJeunesse et al., 2018). Nutrient exchange occurs between the two organisms; corals provide Symbiodiniaceae with the compounds they require for photosynthesis such as CO2 (Yellowlees et al., 2008) and in turn, Symbiodiniaceae translocate photosynthate to corals for use in host cellular processes (Fournier, 2013). However, corals are experiencing stress due to climate change-induced summer heatwaves and exposure to high light levels that typically occur during summer heatwaves (Hughes et al., 2017). Such stressors break down the symbiotic relationship between Symbiodiniaceae and corals, causing coral bleaching and starvation (Weis, 2008; Suggett & Smith, 2019). Coral bleaching amounts to widespread habitat destruction for reef-dwelling organisms (Munday, 2004).
One of the mechanisms believed to be responsible for coral bleaching is the excess production of reactive oxygen species (ROS) by the Symbiodiniaceae following thermal and high light-induced damage to the symbionts’ photosystems (Szabó et al., 2020). ROS are products of reactions of photosystems I and II (PSI and PSII) within chloroplasts of Symbiodiniaceae (Mehler, 1951; Richter et al., 1990). Some ROS, such as singlet oxygen from hydrogen peroxide (H2O2), are hypothesized to diffuse from the photosynthetic algae to the coral host, disrupt the mitochondrial membrane (Fournier, 2013), damage DNA (Barzilai and Yamamoto, 2004) and trigger a cellular cascade resulting in the loss of the Symbiodiniaceae (Weis, 2008). Providing exogenous antioxidants to corals has been shown to prevent bleaching under thermal stress, thus confirming a role of ROS underpinning the bleaching response (Lesser et al., 1990; Lesser, 1997).
Several coral bleaching mitigation methods have been proposed that aim to increase thermal tolerance in both corals and Symbiodiniaceae (van Oppen et al., 2015). One approach currently gaining traction is the use of bacteria (i.e., bacterial probiotics and microbiome transplantation) to enhance coral thermal bleaching tolerance (Rosado et al., 2019; Doering et al., 2021; Maire & van Oppen, 2021; Peixoto et al., 2021; Santoro et al., 2021; Dungan et al., 2022; Doering et al., 2023). Both corals and Symbiodiniaceae associate with a diverse community of bacteria (Lawson et al., 2018; van Oppen and Blackall, 2019; Camp et al., 2020; Maire et al., 2021a). Bacteria are also found intracellularly in cultured and in hospite Symbiodiniaceae and on the extracellular surface of cultured Symbiodiniaceae (Maire et al., 2021c). Their contribution to nutrient acquisition or thermal stress tolerance is not well documented (Ritchie, 2012), but studies on other marine microalgae have demonstrated functional roles. For example, Marinobacter in the marine diatom Pseudo-nitzschia multiseries (Amin et al., 2015) aides siderophore production and assists with resolving issues of iron bioavailability and iron acquisition (Amin et al., 2012). Recently, Motone et al. (2020) showed that a bacterium belonging to the genus Muricauda (Flavobacteriia) enhanced heat and light tolerance in Symbiodiniaceae cultures (Durusdinium sp.). Through antibiotic treatment, bacteria from the class Flavobacteriia were entirely depleted prior to experimental stress exposure. When Symbiodiniaceae cultures were exposed to thermal and light stress, photochemical efficiency of photosystem II (PSII) decreased with a concurrent increase in ROS, an effect that was significantly higher in cultures treated with antibiotics. However, this effect was smaller in cultures that were re-inoculated with Muricauda. This restored tolerance was attributed to Muricauda’s production of the carotenoid zeaxanthin, an antioxidant that ultimately promotes Symbiodiniaceae thermal and light resistance by minimizing damage by ROS. Bacteria sourced from Symbiodiniaceae themselves could thus be good candidates for probiotic use, but the functional relationship between Symbiodiniaceae as well as their potential contribution to thermal tolerance is relatively unexplored (Matthews et al., 2020).
Here, we conducted microbiome manipulation using a bacterium belonging to the genus Roseovarius. Roseovarius spp. have previously shown to have traits such as the ability to neutralize ROS molecules (Yoch, 2002; Miller and Belas, 2004; Randle et al., 2020). Breviolum minutum cultures isolated from Exaiptasia diaphana collected at the Great Barrier Reef were treated with antibiotics, inoculated with Roseovarius, and exposed to thermal stress. It was hypothesized that B. minutum inoculated with Roseovarius that had antioxidant abilities would be more thermally tolerant compared to B. minutum inoculated with Roseovarius lacking antioxidant ability, or a no-inoculum control. This study provides the foundation for future research into Symbiodiniaceae-bacteria relationships, and more generally the study of bacterial roles in Symbiodiniaceae heat tolerance.
Breviolum minutum MMSF01 were obtained from Exaiptasia diaphana (Aiptasia) from the central Great Barrier Reef (Tortorelli et al., 2020). Cultures were maintained in 15 mL Daigo’s IMK medium (1X concentration), prepared with filtered red sea salt water (fRSSW, 34 ppt) in sterile 50 mL polypropylene culture flasks where media was changed fortnightly. These flasks were kept in a 12 h light:12 h dark incubator (50–60 μmol photons m−2s−1 of photosynthetically active radiation) at 26°C.
The method detailed by Costa et al. (2019) was adapted to minimize bacterial community diversity and bacterial load in B. minutum cultures. First, 10 mL of Symbiodiniaceae culture were mixed with 18.7 μL of 0.1% Triton X-100 solution, and gently shaken for 30 sec to remove any loosely associated bacteria. This solution was then centrifuged for 5 min at 5000 × g and subsequently suspended in 10 mL of an antibiotic cocktail solution containing rifampicin, nalidixic acid, carbenicillin, nystatin and erythromycin (Table S1) for two days, then centrifuged and resuspended in IMK 1X for five days and this process was repeated three times in August 2020. During this time, flasks were maintained for three weeks in the same light and temperature conditions as described above. Following this antibiotic treatment, 50 uL Symbiodiniaceae culture were transferred to 1% agar plates that contained IMK (1X concentration), ampicillin, streptomycin, and gentamicin to further remove bacterial communities. Plates were maintained for one month in the same light and temperature conditions as described above, after which one colony was transferred to a new IMK plate supplemented with antibiotics and grown for one month. A single Symbiodiniaceae colony was then picked from the antibiotic agar plates and transferred to sterile 50 mL polypropylene culture flasks that contained 5 mL Daigo’s IMK medium (0.5X concentration, prepared with fRSSW). This volume was then increased to 15 mL after two weeks and these cultures were then maintained (media changed fortnightly) for a year until the temperature stress experiment. Medium was diluted to 0.5X to increase reliance of the Symbiodiniaceae on their bacteria rather than receiving all their nutrients from the media itself. The resulting culture from this antibiotic treatment was named MMSF01-Ax.
Twenty-three bacterial strains belonging to four genera of interest were selected based on potential beneficial functions reported in the literature (Labrenzia, Muricauda, Marinobacter and Roseovarius); these strains were previously isolated from B. minutum (Maire et al., 2021c) and were revived from glycerol stocks kept at -80°C as part of the culture collection at the Marine Microbial Symbiont Facility (University of Melbourne, VIC, Australia). These bacterial isolates were streaked onto marine agar (Difco™ Marine Agar 2216) or Reasoner’s 2A (Oxoid R2A Agar CM0906B) agar plates, grown in an incubator at 26°C, and subcultured by re-streaking for use when required.
The 23 bacterial isolates mentioned earlier were assessed for their antioxidant capacity with a qualitative 2,2-diphenyl-1-picrylhydrazyl (DPPH) assay. DPPH is a free radical that appears purple when in solution, however antioxidant compounds present in solution cause a purple to yellow color change (Floegel et al., 2011). This qualitative assay was completed by streaking bacterial isolates onto marine agar or Reasoner’s 2A agar plates which were then grown in a 26°C incubator for six days. Sterile Whatman #4 filter papers were placed on top of the streak plates with bacterial colonies, and the plates with filters were placed back into the incubator for approximately six hours to ensure bacteria were absorbed by the filter paper. In a fume hood, filter papers were removed using sterile forceps, and left to dry with the ‘bacterial side’ up for ~30 min, before 1 mL of 0.2 mM DPPH (Sigma-Aldrich) solution in methanol was applied with a pipette as per Dungan et al. (2021). Bacterial isolates were assessed for the formation of a white-yellow halo around the bacterial colonies following DPPH application. If a halo appeared within 1 min the isolate was deemed strongly positive. If a halo appeared between 1 and 3 min it was weakly positive, and negative readings occurred when no halos formed.
To count the number of bacteria present in B. minutum cultures, staining with SYBR green dye which binds to nucleic acids was carried out using a modified method by Lawson et al. (2020). SYBR green dye 10,000X (Sigma-Aldrich) was first diluted 1:500 using MilliQ water. Prior to sampling, flasks were shaken for 3 seconds to suspend B. minutum in solution. A sample of 250 μL was taken from each culture flask and diluted with 250 μL of 0.5X IMK media. Twenty-five μL of 1:500 SYBR green were applied to the samples and incubated for 15 min. An unstained sample was also processed as a control (receiving 25 μL of milliQ water). Before flow cytometry processing with a CytoFLEX LX flow cytometer (Beckman Coulter), samples were filtered through a 40 μm mesh-size strainer (pluriSelect) and vortexed to suspend cells and remove clumps. Violet SSC height was used as an acquisition trigger. Symbiodiniaceae cells were gated and counted by autofluorescence using a 405 nm violet laser (emission 763 ± 21.5 nm) (Figure S1). To differentiate SYBR green stained bacterial cells from debris and noise, 0.5X IMK media, MilliQ water and unstained samples were processed. Events measured in IMK-only samples (Figure S1A) were considered noise. Therefore, events from Symbiodiniaceae samples falling in this part of the plot were also excluded (Figure S1D). Stained bacteria were analyzed via a bivariate plot of SYBR green fluorescence (488 nm excitation, emission 525 ± 20 nm) vs 405 nm side scatter (SSC) height. Singlet cells were gated and counted using a violet SSC area vs violet SSC height bivariate plot. At least 1000 Symbiodiniaceae cells were processed per sample. Bacterial count per Symbiodiniaceae cell was calculated by dividing bacterial cell count by Symbiodiniaceae cell count. These quantities were used to determine bacterial re-inoculation densities (see Bacterial Re-inoculation below).
B. minutum cultures MMSF01 as well as MMSF01-Ax cultures treated with antibiotics were used for the heat stress experiment. These B. minutum cultures were subcultured into 20 experimental treatment culture flasks (Figure S2) at a starting density of ~5 x 105 cells mL-1. Following bacterial inoculation (see below), cultures were maintained in the ambient incubator (26°C) for 24 hours before day 0 sampling. Each culture was then separated into two flasks, for ambient and elevated temperature treatments. Ambient culture flasks were kept in the 12h light:12h dark incubator (50–60 μmol photons m−2 s−1 of photosynthetically active radiation) at 26°C for the duration of the experiment. Elevated temperature treatment culture flasks were maintained in a 12h light:12h dark incubator under the same light conditions, at 32°C for the first 8 days of the experiment, with a subsequent temperature increase to 34°C on day 9 for seven days (Figure 1).
Figure 1 Experimental Design (A) Illustrates the sequence of experiments in this study. B. minutum were cultured and treated with antibiotics and maintained for one year until inoculation with Roseovarius and subsequent subjection to a heat stress. (B) The graph represents incubator temperature during the heat stress experiment obtained from data loggers (HOBO Pendant UA-001-08) in each incubator. Elevated incubator temperature was increased from 32°C to 34°C on day 9 of the experiment. Stars denote experiment sampling days, where orange stars show additional sampling for 16S rRNA gene metabarcoding. The green arrow indicates the day that B. minutum cultures were inoculated with bacteria. The right-hand side panel defines the four bacterial treatment groups (n = 5) exposed to ambient and elevated temperatures.
For each temperature treatment, cultures were subjected to four distinct treatments. The AB+ ROS+ (antibiotic treatment, bacterial inoculation with high antioxidant abilities) group aimed to assess the contribution of Roseovarius with putative antioxidant abilities to thermal tolerance. AB+ ROS- (antibiotic treatment, bacterial inoculation with low antioxidant abilities) was introduced to understand if B. minutum were exhibiting heterotrophic feeding whereby the bacteria would have acted as a food source (Jeong et al., 2012; Dungan et al., 2021). AB+ Control (antibiotic treatment, bacterial media inoculum) offered a comparison for the effect of Roseovarius inoculation, and the AB- Control (no antibiotic treatment, bacterial media inoculum) treatment group was included to understand the effect of the antibiotic treatment.
Bacterial isolate MMSF_3448 was chosen as the ROS+ bacterial treatment as it showed positive antioxidant properties in the DPPH assay and MMSF_3432 was chosen as ROS- as it was negative in the assay. Bacteria were streaked from frozen glycerol stocks onto marine agar plates and incubated at 26°C for 4 days. Using an inoculating loop, a single colony of each culture was transferred to marine broth (Difco™ Micro Marine Broth 2216) (MB) and grown in an orbital incubator (Ratek orbital incubator) at 26°C and 300 rpm. Optical density (OD) readings were recorded at 600 nm using a plate reader (CLARIOstar Plus BGM Labtech). Escherichia coli approximations (OD600 of 0.5 is equal to 4 x 108 bacteria mL-1) were used to calculate bacterial cell densities at measured ODs (Volkmer & Heinemann, 2011). Twelve hours prior to inoculation, broth cultures were diluted with MB to reach an OD600 of 0.1 and returned to the orbital incubator. Cultures with an OD600 of ~0.5 were vortexed and used to inoculate B. minutum a day prior to the temperature stress experiment, as per the following treatment groups listed in Figure 1.
As per bacterial abundance data obtained from 16S rRNA gene metabarcoding (Table 1), it was known that Roseovarius had 0.24% relative abundance prior to antibiotic treatment and was approximated to 0.5% for calculations. This, along with the bacterial cell counts per Symbiodiniaceae cell determined using SYBR green dye, was used to calculate the volume of inoculum required to deliver enough Roseovarius cells to restore its initial relative abundance. The number of Roseovarius cells to add through inoculation was determined by multiplying the Symbiodiniaceae cell density (5 x 106 cells mL-1) by the number of bacteria present in the culture per cell (124 bacterial cells per Symbiodiniaceae cell for culture receiving ROS+ Roseovarius strain inoculum, 153 bacterial cells per Symbiodiniaceae cell for culture receiving ROS- Roseovarius strain inoculum). Of this value, 0.5% of these bacteria are Roseovarius, thus 309,584 cells mL-1 and 382,042 cells mL-1 for each treatment respectively. For 100 mL of culture, 30,958,350 and 38,204,200 Roseovarius cells were added. An inoculum with an optical density (OD600) of 0.5 contains 4 x 108 cells mL-1 (E. coli approximation). The OD600 value for the ROS+ Roseovarius strain was determined to be 0.460 and 0.463 for ROS-, thus had bacterial densities of 3.8 x 108 cells mL-1 and 3.7 x 108 cells mL-1. These densities divided by the number of Roseovarius cells determined earlier (30,958,350 and 38,204,200) calculated volumes of 84 μL for ROS+ and 103 μL ROS-. AB+ Control and AB- Control received a 90 μL (averaged value) of inoculum. These inoculum volumes were added to 100 mL Symbiodiniaceae cultures thus, the differences in inoculum volumes (80 uL – 100 uL) were deemed negligible due to the large final culture volume. To confirm inoculum viability, serial dilutions (10-1 to 10-8) of each inoculum were plated onto MA plates with three replications per dilution. MA plates were incubated at 26°C for a week before colony forming units (CFUs) were counted (Table S2).
To quantify cell density over time, B. minutum cell density was obtained using a Countess II FL cell counter (Thermo Fisher scientific). Samples (100 μL) were taken from each replicate flask (five per treatment group). Of this, three 10 μL samples were processed to obtain cell density for each flask.
To assess Symbiodiniaceae photosynthetic fitness, the maximum quantum yield of PSII (Fv/Fm of PSII) was measured using Pulse Amplitude Modulation (PAM) fluorometry. From each flask, 1 mL samples were briefly centrifuged (5 sec) to concentrate cells at the bottom of the tube, dark adapted for 15 min, and pipetted directly from the pellet onto a glass slide and covered with a cover slip for Fv/Fm estimations using microscopy-PAM (IMAGING-PAM M-Series, Walz, Germany) from 1300 hr. Samples belonging to a particular treatment group were processed at the same time on each sampling day to avoid variation in Fv/Fm due to the light variation across the day. Ten areas of interest containing three or more cells were focused simultaneously in each sample using a green colored neutral density filter (only allows green light to pass through) to avoid intense light exposure to samples, and imaged using blue measuring light. To obtain Fv/Fm, dark adapted samples were exposed to a measuring light intensity of 5 µmole quanta m-2 s-1 at 4 Hz frequency to record minimal fluorescence (F0) followed by an application of saturating pulse of 309 µmole quanta m-2 s-1 for 720 ms. The gain and damping were set to 7 and 15, respectively, to minimize noise.
DNA was extracted using a salting-out method as detailed by Wilson et al. (2002) with modifications as per Hartman et al. (2020) from 200 μL samples taken from Breviolum minutum cultures which included B. minutum cells and culture medium, six months prior to the thermal stress experiment (Figure S3). Four extraction blanks were included. The same method was used during the thermal stress experiment detailed below with 200 μL samples that were taken from each culture flask on days -1, 0, 4 and 16 of the experiment. Six DNA extraction blanks were included.
To amplify hypervariable regions V5-V6 of 16S rRNA genes, the primer pair 784F (5' GTGACCTATGAACTCAGGAGTCAGGATTAGATACCCTGGTA 3') and 1061R (' CTGAGACTTGCACATCGCAGCCRRCACGAGCTGACGAC 3') were used. Underlined are the Illumina adapters attached to the primers. 16S rRNA gene PCR amplification, library preparation, and sequencing were completed as per the method detailed by Maire et al. (2021b). Briefly, bacterial 16S rRNA genes were amplified with PCR using a Thermal Cycler (Applied Biosystems, ThermoFisher Scientific) as per the following conditions: denaturation at 95°C for 3 min, 18 cycles of: denaturation at 95°C for 15 sec, annealing at 55°C for 30 sec and extension at 72°C for 30 sec, ending with extension at 72°C for 7 min. Each reaction contained 1 μL of DNA (extracted from samples mentioned earlier), 1.5 μL of 10 uM each forward and reverse primers, 7.5 μL MyTaq HSRed MasterMix (BioLine) and 3.5 μL nuclease-free water (Thermofisher). Triplicates for each sample were conducted, and pooled following PCR. Preparation for library pooling was completed as per Maire et al. (2021c). Library preparation and MiSeq Illumina sequencing was performed at the Walter and Eliza Hall Institute (WEHI) in Melbourne, Victoria.
16S rRNA gene metabarcoding data was processed using QIIME2 version 2021.8 (Bolyen et al., 2019). Metabarcoding data were obtained as paired-end, demultiplexed files with primers and adapters attached. The cutadapt plugin was used to remove primer and adapter sequences, with an error rate of 0.2. The quality of trimmed sequences was determined using the DADA2 plugin, which de-noises, filters, dereplicates, detects chimeras and merges paired-end reads (Callahan et al., 2016). Reads with low quality (Q-score < 30) were removed. To assign taxonomy, a SILVA classifier (version 138) was trained to classify bacterial 16S rRNA reads for hypervariable regions V5-V6. To construct a phylogenetic tree with mid-point rooting the alignment and phylogeny packages in QIIME2 were used (Caporaso et al., 2010). Reads that represented mitochondria and chloroplasts were filtered out. The metadata file, phylogenetic tree, and Amplicon Sequence Variant (ASV) tables were exported and analyzed in R studio.
The metadata file, phylogenetic tree, and Amplicon Sequence Variant (ASV) tables were imported into R studio for analyses, using the phyloseq package (McMurdie and Holmes, 2013). Rare ASVs (percentage abundance lower than 1.10-5) were removed from the dataset. Samples with low read numbers (less than 400) were removed: 1 x ambient AB+ ROS-, 1 x ambient AB- Control and 3 x elevated AB+ ROS+, 1 x elevated AB- Control sampled on day 4, and 1 x ambient AB+ ROS+ from day 16. PCR and DNA blanks were analyzed to reveal contaminants using the decontam package (Table S3) for heat stress experiment data, as well as samples collected six months after antibiotic treatment (Table S4) (Davis et al., 2018). A Principal Coordinates Analysis (PCoA) plot was created using the Unifrac dissimilarity index, to visualize dissimilarities between bacterial communities in B. minutum cultures over time. One outlier (Ambient AB+ROS- collected on day 0) was removed from the plot, as well as the AB- Control samples. A 3-factor nonparametric permutational multivariate analysis of variance (PERMANOVA) using UniFrac distance matrix was conducted to inform the interactions between time, temperature and bacterial treatments. Number of permutations was 999. A second PERMANOVA was used to assess the factors of temperature and bacterial treatments on day 16. The indicator value analysis (De Cáceres and Legendre, 2009) was applied on Day 16 samples, in each of the four conditions independently (AB- Control, AB+ Control, AB+ ROS-, AB+ ROS-), to detect ASVs that were significantly associated with either ambient or elevated treatment using a significance threshold α = 0.05, specificity = 0.9, and fidelity = 0.9. Four Roseovarius ASVs were detected in the metabarcoding data, however, only the major ASV present in the inocula was further analyzed, as the remaining ASVs had very low percentage abundance (i.e., 0.06%) in the inoculum samples, as well as experimental samples. Statistical test results were considered significant where α = 0.05, unless otherwise stated. Graphs were generated using GraphPad Prism version 9.2.0.
Statistical analyses were conducted using R Studio version 1.3.1073. Shapiro-Wilk tests were used to confirm normality in distributions within groups (Ghasemi & Zahediasl, 2012). For cell density data log transformations were required to normalize distributions (Keene, 1995) and fulfil the underlying assumptions underpinning analysis of variance (ANOVA) use. Levene’s tests confirmed homogeneity of variances (Gastwirth et al., 2009), thus 3 factor ANOVAs were used for statistical analyses of Symbiodiniaceae cell density and maximum quantum yield readings. Pairwise comparisons between groups were completed using Welch’s t-tests (DeCoster, 2006) for the previously mentioned datasets.
Of the 23 bacterial isolates tested for their ROS scavenging abilities, positive, or weakly positive readings were observed for only three isolates, with 20 appearing negative in the DPPH assay (Table S5). All three bacterial strains that recorded positive results belonged to the genus Roseovarius. Bacterial isolate MMSF_3448 was chosen as the ROS+ bacterial treatment and MMSF_3432 was chosen as ROS-. Analysis of previously obtained 16S rRNA gene sequences (Maire et al., 2021c) by BLAST showed that sequences for isolates MMSF_3448 and MMSF_3432 had 99% identity based on ~800 bp long fragments. Both isolates shared >99% identity with Roseovarius confluentis, R. algicolus and R. atlanticus. Thus, the species of MMSF_3448 and MMSF_3432 could not be determined, and are henceforth referred to as Roseovarius sp.
Metabarcoding data collected six months after antibiotic treatment revealed changes in percentage abundances of bacteria belonging to the four genera of interest: Labrenzia, Marinobacter, Muricauda and Roseovarius prior to and post antibiotic treatment (Table 1). Roseovarius was entirely depleted in the antibiotic treatment cultures, with very low relative abundances of Labrenzia remaining (0.004%). Interestingly, Muricauda increased in relative abundance (0.005 to 0.15%) in the antibiotic treated B. minutum, while it was not detected in the non-treated culture, and Marinobacter showed greater relative abundance (0.5 to 65%) in antibiotic treated B. minutum. In addition to the four genera of interest, Phycisphaeraceae, Gammaproteobacteria, Pseudohongiella, and Leptospiraceae drastically diminished in relative abundance following antibiotic treatment whilst Micavibrionales and Balneola greatly increased (Figure S4). Thus, the antibiotic treatment was variably effective, but overall was successful in removing some bacterial species from B. minutum such as Roseovarius, which was then used for reinoculation.
The number of bacteria significantly increased following inoculation for all bacterial treatments with increases of greater magnitude observed for bacterial inocula compared to media alone (Figure 2; Table S6). AB+ ROS+, AB+ ROS- and AB- Control contained on average 130 bacterial cells per Symbiodiniaceae cell prior to inoculation (day -1) which increased variably depending on the treatment. Interestingly, AB+ Control had the highest number of bacterial cells on day -1 with an average of 194 bacterial cells per Symbiodiniaceae cell and AB- Control had the lowest with an average of 120. AB+ ROS- showed the highest post-inoculation count with an average of 250 cells mL-1, with AB+ Control, AB+ ROS+ and AB- Control increasing to 242, 195 and 154, respectively. The ratio of the means of bacterial cell count before and after inoculation was approximately 1.6 for AB+ ROS+ and AB+ ROS- and 1.3 for AB+ Control and AB- Control. The greater increase observed for treatments receiving Roseovarius compared to bacterial media alone confirms the success of inoculation (Table S7).
Figure 2 Bacterial cell counts increased following Roseovarius inoculation Bacterial cell count per Symbiodiniaceae cell, pre and post inoculation. Day -1 was measured prior to inoculation, and Day 0 one day after inoculation. (A) A significant increase in bacterial cell count was detected for all treatment groups by ANOVA. Bars represent the mean and error bars represent ± SD, where n = 5. Asterisk represents significant pairwise comparisons by Welch’s t-tests where p < 0.05. (B) Ratio of the mean values of bacterial cell count before and after inoculation.
Symbiodiniaceae cell density was measured throughout the heat stress experiment (Figure 3A). A three-way ANOVA informed that all three factors (time, temperature, and bacterial treatment) (Table S8), as well as the two-way and three-way interactions between these factors, had a significant effect. Overall, cell density for all elevated treatments decreased over time, but it was found that AB+ ROS+ did not decrease as much as the other treatment groups (Figure 3A). On day 16, cell density was significantly lower for cultures in elevated temperature condition compared to ambient (Figure 3C; Table S9), confirming the negative effect of elevated temperature on cell density as well as signifying that the cells were under stress. Mean cell density at ambient temperatures were on average three times higher on day 16 compared to day 0 (Table S10): AB+ ROS-, AB+ Control and AB- Control treatments observed a 51%, 54% and 64% decrease in mean cell density, respectively, between day 0 and day 16. Conversely, AB+ ROS+ exposed to elevated temperature only observed a decrease of 7% in mean cell density over time. On day 16, cell density was significantly higher in elevated AB+ ROS+ compared to AB+ ROS-, and AB+ ROS+ compared to AB+ Control (Table S10). Additionally, Symbiodiniaceae cell density was significantly higher in AB+ Control vs AB- Control on day 16 (Table S10). Together these results suggest that B. minutum experienced thermal stress at elevated temperature which lessened cell density as time progressed, however the addition of Roseovarius through inoculation minimized the negative effect of temperature on cell fitness.
Figure 3 Roseovarius may assist in the maintenance of Symbiodiniaceae cell density and maximum quantum yield at elevated temperatures (A) Cell density of Symbiodiniaceae over time at ambient (26°C) (dark colors and solid lines) and heated (34°C) temperature (faded colors and dotted lines) for AB+ ROS+ bacteria (pink), AB+ ROS- (blue), AB+ Control (green) and AB- Control (orange). Each data point represents the mean and error bars are ± SD, where n = 5. (B) Maximum quantum yield of Symbiodiniaceae PSII over time. Each data point represents the mean and error bars are ± SD, where n = 3. (C) Symbiodiniaceae cell density on day 16. Bars represent the mean and error bars are ± SD, where n = 5. Asterisk denotes significant differences by Welch’s t-tests where p < 0.05. (D) Maximum quantum yield of Symbiodiniaceae PSII on day 16. Each bar represents the mean and error bars are ± SD, where n = 3.
The maximum quantum yield (Fv/Fm) of the Symbiodiniaceae was measured as a proxy for photosynthetic fitness throughout the heat stress experiment (Figure 3B). A three-way ANOVA analysis showed that the time and temperature, as well as their interaction, had a significant effect but bacterial treatment and all other interactions did not - at least not over the course of the experiment (Table S11). For all bacterial treatments, Fv/Fm was significantly lower on day 16 compared to day 0 at elevated temperature (Table S12), indicative of heat stress in these cultures. On day 16, however, pairwise t-tests confirmed that Fv/Fm was significantly lower in elevated AB+ ROS-, AB+ Control and AB- Control when compared to their ambient counterparts (Figure 3D; Table S13), suggesting there are greater disturbances to B. minutum maximum quantum yield when Roseovarius with antioxidant abilities are absent. Conversely, no significant differences were detected in Fv/Fm for ambient compared to elevated temperature treatments for AB+ ROS+ B. minutum on day 16 (Table S13), suggesting a role for Roseovarius for photochemical performance in B. minutum at heightened temperature. It is however possible that the lack of significant difference observed between AB+ROS+ on day 16 was due to the variability of samples that limited the power of statistical analyses. The greatest difference in Fv/Fm between day 0 and day 16 was observed for elevated AB- Control (75% decrease). Overall, Fv/Fm decreased for all treatment groups at elevated temperatures, suggesting that temperature strongly impacted the maximum quantum yield of B. minutum but the addition of Roseovarius minimized these effects.
The bacterial community composition was highly similar among samples belonging to day -1, day 0, and day 4, regardless of the temperature or bacterial treatment (Figure 4A). However, on day 16, samples obtained from elevated temperature groups clustered away from those at ambient temperatures, suggesting a combined effect of time and temperature on bacterial community structure. PERMANOVA analysis confirmed these observations, as time and temperature, as well as their interaction, were significant in explaining the dissimilarity, but bacterial treatment was not (Table S14). However, the interaction between bacterial treatment and time was also found to be significant. In light of these interactions, further PERMANOVA analysis was conducted for day 16, which showed that bacterial communities were significantly different between ambient and elevated temperatures, and between bacterial treatments (Table S15). This pattern was also seen at the family level (Figure 4B). AB- Control was most different to AB+ ROS+, AB+ ROS- and AB+ Control on day 0, with the largest percentage abundance (20%) being bacteria of the family Bacteriovoracaceae. Major community composition changes over time were similar across the three treatments AB+ ROS+, AB+ ROS- and AB+ Control. Taxa such as Algiphilaceae and Balneolaceae showed a drastic decrease in relative abundance between day 0 and day 16, where Algiphilaceae decreased from an average of 35% to <1% and Balneolaceae decreased from 10% to 3%. Rickettsiales relative abundance increased variably, regardless of temperature, showing the impact of time alone on community composition. Other taxa, such as Terasakiellaceae and Oleiphilaceae, increased in relative abundance from an average of 3% to 10% in Terasakiellaceae and <1% to 6% on average in Oleiphilaceae only at elevated temperature, highlighting the effect of temperature over time on community composition. These changes were underpinned by differences in ASV abundances at ambient and elevated temperatures for each treatment group, which were investigated with an indicator analysis (Figure S5). For elevated treatments, ASV 15 UC Terasakiellaceae and ASV 16 Oleiphilus sp. were the most abundant indicator ASVs for AB+ Control, AB+ROS-, and AB+ROS+. For elevated AB- Control ASV 40 UC Saprospiraceae and ASV 31 Pelagibius sp. appeared in higher abundances. Ambient indicator species include ASV 03 UC Paracaedibacteraceae most prevalent in AB+ROS- and AB+ Control and ASV 01 UC Rickettsiales in AB- Control and AB+ROS+. Labrenzia were more abundant at elevated temperature with ASV 28 Labrenzia sp. and ASV 32 Labrenzia marina being abundant indicator ASVs at elevated temperatures. Altogether, these results suggest that bacterial community structure changed over time as a result of temperature exposure, with variable changes observed in different bacterial taxa.
Figure 4 Bacterial communities changed throughout exposure to elevated temperature (A) PCoA plot where Axis 1 and Axis 2 are PC1 and PC2 respectively, representing the beta-diversity of bacterial communities of three of the bacterial treatments in antibiotic-treated cultures, AB+ ROS+ (Positive), AB+ ROS- (Negative) and AB+ Control (Media) at ambient and elevated temperatures. Each data point denotes a single sample, retrieved on a given sampling day: day -1, day 0, day 4 and day 16 (D-1, D0, D4 and D16, respectively) of the experiment. (B) Bar chart of percentage abundance of the top 20 bacterial families present in samples on day 0 and day 16, at ambient and elevated temperature.
To assess Roseovarius persistence following inoculation and thus the sustained success of inoculation over time, Roseovarius relative abundance was determined throughout the experiment using 16S rRNA gene metabarcoding. Following decontamination, three ASVs were found in the two inocula, all belonging to the genus Roseovarius, with one single ASV (Roseovarius sp. ASV01) making up 99% and 98% of the reads in the ROS+ and ROS- inocula, respectively which points to high inocula purity (Figure S6). The remaining 1-2% of reads were likely contaminants from library preparation, as well as the 1.9% ASV01 abundance which was observed in the control inoculum. Thus, the abundance of this major ASV that comprised the two Roseovarius inocula was further investigated. Prior to inoculation, Roseovarius sp. ASV01 relative abundances were ~4% for AB+ ROS+ and AB+ ROS-, 2% for AB+ Control and 0.6% for AB- Control, which contrasts with metabarcoding data that were obtained six months prior to the heat stress experiment where Roseovarius was not detected (Table 1). In fact, overall bacterial communities were quite different between the beginning of the heat stress experiment and the sampling conducted six months prior. Six months before the heat stress experiment, AB- cultures were dominated by Phycisphaeraceae, Stappiaceae, and Pseudohongiellaceae in comparison to AB+ cultures that mostly contained Marinobacteraceae as well as Balenoelaceae and Micavibrionales (Figure S4B). On day 0 of the heat stress experiment, Phycisphaeraceae were still highly abundant but Bacteriovoracaceae were most abundant for AB- cultures. At this time, Algiphilaceae, Balneolaceae, and Marinobacteraceae were observed in the highest abundance for AB+ cultures. Roseovarius relative abundance increased significantly over the course of the heat stress experiment (Figure 5; Table S16), particularly on day 16 for elevated treatment groups, with a 17% and 16% abundance observed for AB+ ROS+ and AB+ ROS- respectively at the conclusion of the experiment, compared to only 4% for AB+ Control and 1% for AB- Control, demonstrating that inoculated Roseovarius was able to establish itself in the B. minutum cultures. Further, on day 16, Roseovarius relative abundance was significantly higher at elevated temperature, compared to ambient, in the AB+ ROS+ and AB+ control treatments, but not for AB+ ROS- and AB- Control (Table S17). Overall, Roseovarius relative abundance showed greater increases over time after inoculation with Roseovarius, compared to negative controls confirming the successful establishment of inoculated Roseovarius into the B. minutum cultures.
Figure 5 Roseovarius persisted in B. minutum culture following inoculation Relative abundance of Roseovarius sp ASV01 in each bacterial treatment over time (day-1 to day 16). A and E denote ambient and elevated temperatures, respectively. Bars represent the mean and error bars are ± SD, where n = 5. Asterisks denote significant differences by Welch’s t-tests where p < 0.05. Significant differences were detected when comparing samples obtained prior to inoculation (day -1) and a day after inoculation (day 0), as well as comparisons between day -1 and day 16. Elevated treatment bars appear patterned on day 4 and day 16 to allow for clearer comparisons.
The relationship between Symbiodiniaceae and their bacteria is not extensively studied, and further inquiries are required to understand the functional contribution of bacteria to Symbiodiniaceae health and survival. Here, an antibiotic cocktail was administered to minimize B. minutum’s bacterial load and diversity, with subsequent inoculation with Roseovarius and exposure to elevated temperature. B. minutum inoculated with Roseovarius with high ROS-scavenging abilities showed greater cell density and smaller decreases in Fv/Fm at elevated temperatures, suggesting that Roseovarius may provide benefits to B. minutum under heat stress conditions. Analysis of Symbiodiniaceae bacterial communities through 16S rRNA gene metabarcoding revealed that Roseovarius relative abundance increased in B. minutum cultures following inoculation and increased with elevated temperature exposure, suggesting they may play a role in shielding B. minutum from the effects of thermal stress.
The B. minutum cultures were not rendered axenic following antibiotic treatment. Of the four genera of interest (Labrenzia, Marinobacter, Muricauda, Roseovarius), Roseovarius was no longer detected in antibiotic treated B. minutum cultures, with considerable decreases seen in Labrenzia. In contrast, Muricauda and Marinobacter showed an increase in relative abundance, in antibiotic treated B. minutum. Thus, the antibiotic treatment was successful in significantly reducing the abundance of certain genera, but not all. By estimating absolute bacterial load prior to inoculation, it was found that the cultures not treated with antibiotics had fewer bacteria present compared to all antibiotic treatment cultures. This pattern is opposite to expectations and suggests that the antibiotic treatment had limited effectiveness in removing bacteria associating with Symbiodiniaceae. Alternatively, the bacteria may have been able to grow back to pre-AB treatment levels during recovery, as the cultures were maintained for one year following antibiotic treatment. Additionally, while we observed an absence of Roseovarius six months after antibiotic treatment, samples taken approximately one year after antibiotic treatment during the heat stress experiment showed a resurgence of Roseovarius, which was accompanied by other major changes in bacterial community composition. This suggests that bacteria, including Roseovarius, may have been reintroduced following antibiotic treatment, or that undetectable levels of Roseovarius were still present which later resurfaced. Similar antibiotics durably depleted Flavobacteriia from Symbiodiniaceae in an earlier study, after two months of antibiotic treatment and three months of recovery, although absolute bacterial load was not measured (Motone et al., 2020). In contrast, Xiang et al. (2013) reported the complete elimination of bacterial associates in five Symbiodiniaceae cultures.
Interestingly, cultures that did not go through antibiotic treatment showed the greatest fitness decrease over time at elevated temperature, as reflected in the cell density and photochemical efficiency data. It is possible that some bacteria that resisted the antibiotic treatment were able to thrive following the removal of antibiotic-sensitive bacteria, and have functions that assist with thermal tolerance. The antibiotic treatment restructured the microbiome and it is possible that the microbiome modifications following antibiotic treatments rendered it more beneficial during thermal stress. Further studies with the B. minutum cultures without antibiotic treatment in addition to inoculation with ROS-scavenging bacteria (ROS+) and non-ROS-scavenging bacteria (ROS-) will help to understand these observed differences. The thermally tolerant photosymbiont genus Durusdinium has previously shown the greatest microbiome stability under experimental thermal stress, suggesting that stable microbiomes assist with thermal tolerance (Camp et al., 2020). Heat-evolved Cladocopium, exposed to elevated temperature (31°C) for 6 years, housed less diverse but more stable bacterial communities than their wild type counterparts (Buerger et al., 2022), with differences in microbiome composition suspected to contribute to thermal tolerance. Thus, a heat-selected microbiome achieved through experimental evolution (Buerger et al., 2022) or inoculation with certain bacteria (Motone et al., 2020) may equip Symbiodiniaceae for elevated temperature conditions, however further functional studies are required.
Changes in bacterial community structure were observed, with temperature being a significant factor influencing these changes, although those changes did not occur immediately. This suggests that the effects of bacterial treatment were delayed and coupled with extended exposure to thermal stress conditions. Therefore, other bacteria, beyond Roseovarius, may have played a role in thermal stress tolerance. This was also reflected in studies completed by Camp et al. (2020), whereby B. minutum experienced significant bacterial community changes when exposed to elevated temperatures. B. minutum was associated with both Roseovarius and Labrenzia at similar relative abundances under ambient and elevated temperature, with little Hyphobacterium observed at elevated temperature (Camp et al., 2020). Following Symbiodiniaceae experimental evolution, the genera Labrenzia, Algiphilus, Hyphobacterium and Roseitalea were more abundant in heat-evolved Cladocopium strains compared to wild type (Buerger et al., 2022). Interestingly, we observed higher Algiphilus (family Gammaproteobacteria) relative abundance following antibiotic treatment in B. minutum, although it decreased following exposure to elevated temperature. Labrenzia are found in high relative abundance across the majority of Symbiodiniaceae strains (Lawson et al., 2018; Camp et al., 2020; Maire et al., 2021c; Díaz-Almeyda et al., 2022). Labrenzia plays a role in the production of dimethylsulfoniopropionate (DMSP) in other dinoflagellates (Sunda et al., 2002) which is a compound that scavenges ROS, thus it may function as an antioxidant and assist with heat stress tolerance. In our analysis, Labrenzia were found in higher abundance at elevated temperature on day 16. The altered community following elevated temperature exposure in our study may have influenced thermal tolerance due to the presence of Roseovarius, Labrenzia, and bacterial species that provide a functional contribution to thermal stress mitigation.
Despite the antibiotic treatment’s limited capacity to perturb the B. minutum microbiome and the resurgence of Roseovarius before the thermal stress experiment, Roseovarius established in the B. minutum cultures following inoculation. This suggests that treating Symbiodiniaceae with antibiotics may not be necessary in order to assess the contributions of inoculated bacteria. Roseovarius relative abundance had greater increases at elevated compared to ambient temperature, thus Roseovarius may be better at competing with other bacteria at elevated temperature.
Re-inoculation with ROS-scavenging Roseovarius may have contributed to greater B. minutum cell density at elevated temperatures. At the conclusion of the temperature stress experiment, cell density at elevated temperatures were significantly higher for flasks inoculated with ROS-scavenging Roseovarius, compared to other elevated temperature bacterial treatment groups, albeit lower than in the ambient treatment. In addition, smaller reductions in maximum quantum yield were detected in flasks inoculated with ROS-scavenging Roseovarius, suggesting this treatment group maintained better photosynthetic fitness compared to controls at elevated temperatures. Statistical analyses may have been affected by sample variability, in turn contributing to the non-detectable differences in maximum quantum yield observed for ROS-scavenging Roseovarius inoculated flasks. Greater sample size in future studies would disentangle whether these observations were limited by the statistical analyses. In addition, since absolute bacterial load was not measured, observed Roseovarius increases only reflect the proportional change in bacterial communities, not whether Roseovarius cell abundance increased. Thus, future studies should measure absolute abundances to conclusively determine inoculation success and subsequent influence on inoculated species. The addition of a Roseovarius strain with low ROS-scavenging abilities was a useful control group as cultured Symbiodiniaceae can feed on bacteria (Jeong et al., 2012). If heterotrophic feeding was responsible for the observed fitness increases, the same effects would have been expected for the Roseovarius inoculation with the low ROS scavenging strain. Previous cnidarian probiotic studies have failed to include such treatment groups for comparison (Rosado et al., 2019; Doering et al., 2021; Santoro et al., 2021; Zhang et al., 2021), with few including an inoculum without the beneficial function under study (Dungan et al., 2021; Dungan et al., 2022), but it should become common practice in future investigations.
In a previous study by Maire et al. (2021c), species of Roseovarius appeared both closely and loosely associated to B. minutum. Relative abundances of Roseovarius were 1% for closely associated fractions and less than 0.01% for loosely associated fractions (Maire et al., 2021c), suggesting Roseovarius preferably closely associate with Breviolum. However, we cannot confirm the particular ASV assessed here closely associates with B. minutum as samples for DNA extraction were not washed and filtered upon collection. Thus, Roseovarius in our study may simply be free-living within the culture medium. Further, Maire et al. (2021c) suggest that bacteria appear on the external surface of Symbiodiniaceae cells under culture conditions and are absent in hospite. As we cannot conclude the nature of the association of Roseovarius with B. minutum and there is a possibility that the bacteria exist within the culture medium, further research is required to determine whether Roseovarius closely associate with B. minutum and subsequently if this association confers any benefits in hospite.
Roseovarius was found to play a key role in sulfur cycling and the de-methylation of DMSP to dimethylsulfide (DMS) in the dinoflagellate Pfiesteria piscicida (Yoch, 2002; Miller and Belas, 2004). In species of Roseovarius isolated from seawater (González et al., 2003), DMS and DMSP were found to play a role in the DMSP antioxidant system and scavenge ROS (Sunda et al., 2002), which could contribute to the reduction of oxidative stress during thermal stress. Additionally, Roseovarius was detected in heat-stressed corals (Pootakham et al., 2019), and Aliiroseovarius, a closely related genus, increased in relative abundance during heat stress at high salinities in the sea anemone Exaiptasia diaphana (Randle et al., 2020), suggesting a functional contribution to thermal stress. Whole-genome sequencing of the two isolates used in our study should be undertaken to understand the mechanisms underlying the different effects they had on Symbiodiniaceae cultures, and whether it is indeed ROS-related or whether other biological processes are at play.
Microbiome manipulations such as those used in the present study are carried out to understand if Symbiodiniaceae-associated bacteria can enhance thermal tolerance. Targeted studies like our investigation as well as Motone et al. (2020) aim primarily to elucidate specific bacteria of interest that may confer beneficial functions and increase thermal tolerance of Symbiodiniaceae, and future investigations should assess the nature of the association of bacteria with Symbiodiniaceae in hospite in order to make concrete conclusions about their beneficial functions. It has been shown that bacterial communities of Symbiodiniaceae differ following experimental evolution (Buerger et al., 2022), and these changes may contribute to the increased Symbiodiniaceae heat tolerance. Further studies are required to assess if Roseovarius contribute to thermal tolerance of Symbiodiniaceae in hospite and whether there are potential implications in coral restoration. Insightful future investigations include inoculating Symbiodiniaceae, or corals experiencing thermal stress, to observe which bacterial community members are incorporated which could suggest beneficial functions.
This study begins to decipher the relationship between Symbiodiniaceae and their bacteria with a particular focus on the genus Roseovarius. The findings demonstrate the positive effect of Roseovarius inoculation on cultured Symbiodiniaceae exposed to thermal stress and suggest a potential functional role of Roseovarius in Symbiodiniaceae thermal tolerance. Further studies are required to explore whether bacterial inoculation with Roseovarius could be used to mitigate thermal stress in other Symbiodiniaceae species or in corals, and may give rise to new research avenues and coral restoration practices.
The datasets presented in this study can be found in online repositories. The names of the repository/repositories and accession number(s) can be found below: https://www.ncbi.nlm.nih.gov/, PRJNA887911 https://www.ncbi.nlm.nih.gov/, PRJNA887545.
Conceptualization: KH, JM, and MO. Formal analysis: KH and JM. Funding acquisition: MO. Investigation: KH, JM, and PD. Methodology: KH, PD, and AP-G. Supervision: MO and JM. Visualization: KH. Writing – Original Draft Preparation: KH, JM, and MO. All authors contributed to manuscript revision, read, and approved the submitted version.
This study was funded by the Australian Research Council Laureate Fellowship FL180100036 to MO. PD was supported by Gordon Betty Moore foundation (grant number – 9351).
We are grateful to: Dr. Sanjida Topa for assisting with processing metabarcoding samples and Dr. Ashley Dungan for providing assistance with metabarcoding data analysis.
The authors declare that the research was conducted in the absence of any commercial or financial relationships that could be construed as a potential conflict of interest.
All claims expressed in this article are solely those of the authors and do not necessarily represent those of their affiliated organizations, or those of the publisher, the editors and the reviewers. Any product that may be evaluated in this article, or claim that may be made by its manufacturer, is not guaranteed or endorsed by the publisher.
The Supplementary Material for this article can be found online at: https://www.frontiersin.org/articles/10.3389/fevo.2023.1079271/full#supplementary-material
Amin S. A., Green D. H., Waheeb D. A., Gardes A., Carrano C. J. (2012). Iron transport in the genus Marinobacter. Biometals 25, 135–147. doi: 10.1007/s10534-011-9491-9
Amin S. A., Hmelo L. R., van Tol H. M., Furham B. P., Carlson L. T., Heal K. R., et al. (2015). Interaction and signalling between a cosmopolitan phytoplankton and associated bacteria. Nature 522, 98–101. doi: 10.1038/nature14488
Barzilai A., Yamamoto K. (2004). DNA damage responses to oxidative stress. DNA Repair (Amst) 3(8–9):1109–1115. doi: 10.1016/j.dnarep.2004.03.002
Bolyen E., Rideout J. R., Dillon M. R., Bokulich N. A., Abnet C. C., Al-Ghalith G. A., et al. (2019). Reproducible, interactive, scalable and extensible microbiome data science using QIIME 2. Nat. Biotechnol. 37, 852–857. doi: 10.1038/s41587-019-0209-9
Buerger P., Vanstone R. T., Maire J., van Oppen M. J. H. (2022). Long-term heat selection of the coral Endosymbiont Cladocopium C1acro (Symbiodiniaceae) stabilizes associated bacterial communities. Int. J. Mol. Sci. 23 (9), 4913. doi: 10.3390/ijms23094913
Callahan B. J., Mcmurdie P. J., Rosen M. J., Han A. W., Johnson A. J. A., Holmes S. P. (2016). DADA2: high-resolution sample inference from Illumina amplicon data. Nat. Methods 13, 581. doi: 10.1038/nmeth.3869
Camp E. F., Kahlke T., Nitschke M. R., Varkey D., Fisher N. L., Fujise L., et al. (2020). Revealing changes in the microbiome of Symbiodiniaceae under thermal stress. Environ. Microbiol. 22 (4), 1294–1309. doi: 10.1111/1462-2920.14935
Caporaso J. G., Kuczynski J., Stombaugh J., Bittinger K., Bushman F. D., Costello E. K., et al. (2010). QIIME allows analysis of high-throughput community sequencing data. Nat. Methods 7, 335–336. doi: 10.1038/nmeth.f.303
Cesar H., Burke L., Pet-Soede L. (2003). The economics of worldwide coral reef degradation, Cesar Environmental Economics Consulting, Arnhem.
Costa R. M., Fidalgo C., Cardenas A., Frommlet J. C., Voolstra C. R. (2019). Protocol for the generation of axenic/bacteria-depleted Symbiodiniaceae cultures. doi: 10.17504/protocols.io.87khzkw
Davis N. M., Proctor D. M., Holmes S. P., Relman D. A., Callahan B. J. (2018). Simple statistical identification and removal of contaminant sequences in marker-gene and metagenomics data. Microbiome 6, 226. doi: 10.1186/s40168-018-0605-2
De Cáceres M., Legendre P. (2009). Associations between species and groups of sites: Indices and statistical inference. Ecology 90, 3566–3574. doi: 10.1890/08-1823.1
DeCoster J. (2006) Testing Group Differences using T-tests, ANOVA, and Nonparametric Measures. Available at: http://www.stat-help.com/notes.html (Accessed 27/09/21).
Díaz-Almeyda E. M., Ryba T., Ohdera A. H., Collins S. M., Shafer N., Link C., et al. (2022). Thermal stress has minimal effects on bacterial communities of thermotolerant symbiodinium cultures. Front. Ecol. Evol. 10. doi: 10.3389/fevo.2022.764086
Doering T., Maire J., van Oppen M. J. H., Blackall L. L. (2023). Advancing coral mirobiome manipulation to build long-term climate resilience. Microbiol. Aust. 44, 36–40. doi: 10.1071/MA23009
Doering T., Wall M., Putchim L., Rattanawongwan T., Schroeder R., Hentschel U., et al. (2021). Towards enhancing coral heat tolerance: a “microbiome transplantation” treatment using inoculations of homogenized coral tissues. Microbiome 9, 102. doi: 10.1186/s40168-021-01053-6
Dungan A. M., Bulach D., Lin H., van Oppen M. J. H., Blackall L. L. (2021). Development of a free radical scavenging bacterial consortium to mitigate oxidative stress in cnidarians. Microbial Biotechnol. 14 (5), 2025–2040. doi: 10.1111/1751-7915.13877
Dungan A. M., Hartman L. M., Lin H., Blackall L. L., van Oppen M. J. H. (2022). Exploring microbiome engineering as a strategy for improved thermal tolerance in Exaiptasia diaphana. J. Appl. Microbiol. 132 (4), 2940–2956. doi: 10.1111/jam.15465
Floegel A., Dae-Ok K., Chung S. J., Koo S. I., Chun O. K. (2011). Comparison of ABTS/DPPH assays to measure antioxidant capacity in popular antioxidant-rich US foods. J. Food Composition Anal. 24, 2043–1048. doi: 10.1016/j.jfca.2011.01.008
Fournier A. (2013). The story of symbiosis with zooxanthellae, or how they enable their host to thrive in a nutrient poor environment. Masters Biosci. Rev., 1–8.
Gastwirth J. L., Gel Y. R., Miao W. (2009). The impact of Levene’s test of equality of variances on statistical theory and practice. Stat. Sci. 24 (3), 343–360. doi: 10.1214/09-STS301
Ghasemi A., Zahediasl S. (2012). NorMality tests for statistical analysis: A guide for non-statisticians. Int. J. Endocrinol. Metab. 10 (2), 486–489. doi: 10.5812/ijem.3505
González J. M., et al. (2003). Silicibacter pomeroyi sp. nov. and Roseovarius nubinhibens sp. nov., dimethylsulfoniopropionate-demethylating bacteria from marine environments. Int. J. Syst. Evol. Microbiol. 53 (5): 1261–1269. doi: 10.1099/ijs.0.02491-0
Hartman L. M., van Oppen M. J. H., Blackall L. L. (2020). Microbiota characterization of Exaiptasia diaphana from the Great Barrier Reef. Anim. Microbiome 2. doi: 10.1186/s42523-020-00029-5
Hughes T. P., Barnes M. L., Bellwood D. R., Cinner J. E., Cumming G. S., Jackson J. B. C., et al. (2017). Coral reefs in the anthropocene. Nature 546, 82–90. doi: 10.1038/nature22901
Jeong H. J., Yoo Y. D., Kang N. S., Lim A. S., Seong K. A., Lee S. Y., et al. (2012). Heterotrophic feeding as a newly identified survival strategy of the dinoflagellate. Symbiodinium Proc. Natl. Acad. Sci. 109 (31), 12604–12609. doi: 10.1073/pnas.1204302109
Keene O. N. (1995). The log transformation is special. Stat. Med. 14:811–819. doi: 10.1002/sim.4780140810
LaJeunesse T. C., Parkinson J. E., Gabrielson P. W., Jeong H. J., Reimer J. D., Voolstra C. R., et al. (2018). Systematic revision of symbiodiniaceae highlights the antiquity and diversity of coral endosymbionts. Curr. Biol. 28 (16), 2570–2580. doi: 10.1016/j.cub.2018.07.008
Lawson C. A., Seymour J. R., Possell M., Suggett D. J., Raina J. B. (2020). The volatilomes of symbiodiniaceae-associated bacteria are influenced by chemical derived from their algal partner. Front. Mar. Sci. 7. doi: 10.3389/fmars.2020.00106
Lesser M. P., Stochaj W. R., Tapley D. W., Shick J. M. (1990). Bleaching in coral reef anthozoans: effects of irradiance, ultraviolet radiation, and temperature on the activities of protective enzymes against active oxygen. Coral Reefs 8, 225–232. doi: 10.1007/BF00265015
Lesser M. P. (1997). Oxidative stress causes coral bleaching during exposure to elevated temperatures. Coral Reefs 16, 187–192. doi: 10.1007/s003380050073
Maire J., Blackall L. L., van Oppen M. J. H. (2021a). Intracellular bacterial symbionts in corals: challenges and future directions. Microorganisms 9 (11), 2209. doi: 10.3390/microorganisms9112209
Maire J., Blackall L. L., van Oppen M. J. H. (2021b). Microbiome characterization of defensive tissues in the model anemone Exaiptasia diaphana. BMC Microbiol. 21, 152. doi: 10.1186/s12866-021-02211-4
Maire J., Girvan S. K., Barkla S. E., Perez-Gonzalez A., Suggett D. J., Blackall L. L., et al. (2021c). Intracellular bacteria are common and taxonomically diverse in cultured and in hospite algal endosymbionts of coral reefs. ISME J. 15, 2028–2042. doi: 10.1038/s41396-021-00902-4
Maire J., van Oppen M. J. H. (2021). A role for bacterial experimental evolution in coral bleaching mitigation? Trends Microbiol. 30 (3), 217–228. doi: 10.1016/j.tim.2021.07.006
Matthews J. L., Raina J. B., Kahlke T., Seymour J. R., van Oppen M. J. H., Suggett D. J. (2020). Symbiodiniaceae-bacteria interactions: rethinking metabolite exchange in reef-building corals as multi-partner metabolic networks. Environ. Microbiol. 22, 1675. doi: 10.1111/1462-2920.14918
McMurdie P. J., Holmes S. (2013). phyloseq:An R package for reproducible interactive analysis and graphics of microbiome cencus data. PLoS One 8 (4), e61217. doi: 10.1371/journal.pone.0061217
Mehler A. H. (1951). Studies on reactions of illuminated chloroplasts. I. Mechanism of the reduction of oxygen and other hill reagents. Arch. Biochem. Biophysics 33 (1), 65–77. doi: 10.1016/0003-9861(51)90082-3
Miller T., Belas R. (2004). Dimethylsulfoniopropionate metabolism by Pfiesteria- associated Roseobacter spp. Appl. Environ. Microbiol. 70 (6), 3383–3391. doi: 10.1128/AEM.70.6.3383-3391.2004
Motone K., Takagi T., Aburaya S., Miura N., Aoki W., Ueda M. (2020). A zeaxanthin- producing bacterium isolated from the algal phycosphere protects coral endosymbionts from environmental stress. mBio 11, e01019–e01019. doi: 10.1128/mBio.01019-19
Munday P. L. (2004). Habitat loss, resource specialization, and extinction on coral reefs. Global Change Biol. 10 (10), 1642–1647. doi: 10.1111/j.1365-2486.2004.00839.x
Peixoto R. S., Sweet M., Villela H. D. M., Cardoso P., Thomas T., Voolstra C. R., et al. (2021). Coral probiotics: premise, promise, prospects. Annu. Rev. Anim. Biosci. 9, 265–288. doi: 10.1146/annurev-animal-090120-115444
Pootakham W., Mhuantong W., Yoocha T., Putchim L., Jomchai N., Sonthirod C., et al. (2019). Heat-induced shift in coral microbiome reveals several members of the Rhodobacteraceae family as indicator species for thermal stress in Porites lutea. MicrobiologyOpen 8 (12), e935. doi: 10.1002/mbo3.935
Randle J. L., Cárdenas A., Gegner H. M., Ziegler M., Voolstra C. R. (2020). Salinity-conveyed thermotolerance in the coral model Aiptasia is accompanied by distinct changes of the bacterial microbiome. Front. Mar. Sci. 7. doi: 10.3389/fmars.2020.573635
Richter M., Ruhle W., Wild A. (1990). Studies on the mechanism of photosystem II photoinhibition II. The involvement of toxic oxygen species. Photosynthesis Res. 24, 237–243. doi: 10.1007/BF00032311
Ritchie K. B. (2012). “Bacterial symbionts of corals and Symbiodinium,” in Beneficial Microorganisms in Multicellular Life Forms. Eds. Rosenberg E., Gophna U. (Berlin, Heidelberg: Springer), 136–150.
Rosado P. M., Leite D. C. A., Duarte G. A. S., Chaloub R. M., Jospin G., Nunes da Rocha U., et al. (2019). Marine probiotics: increasing coral resistance to bleaching through microbiome manipulation. ISME J. 13 (4), 921–936. doi: 10.1038/s41396-018-0323-6
Santoro E. P., Borges R. M., Espinoza J. L., Freire M., Messias C. S. M. A., Villela H. D. M., et al. (2021). Coral microbiome manipulation elicits metabolic and genetic restructuring to mitigate heat stress and evade mortality. Sci. Adv. 7 (33), eabg3088. doi: 10.1126/sciadv.abg308
Suggett D. J., Smith D. J. (2019). Coral bleaching patterns are the outcome of complex biological and environmental networking. Global Change Biol. 26 (1), 68–79. doi: 10.1111/gcb.14871
Sunda W., Kieber D. J., Kiene R. P., Huntsman S. (2002). An antioxidant function for DMSP and DMS in marine algae. Nature 418, 317–320. doi: 10.1038/nature00851
Szabó M., Larkum A. W. D., Vass I. (2020). “A review: the role of reactive oxygen species in mass coral bleaching,” in Photosynthesis in Algae: Biochemical and Physiological Mechanisms, vol. 45 . Eds. Larkum A., Grossman A., Raven J. (Cham: Springer).
Tortorelli G., Belderok R., Davy S. K., McFadden G. I., van Oppen M. J. H. (2020). Host genotypic effect on algal symbiosis establishment in the coral model, the anemone Exaiptasia diaphana, from the great barrier reef. Front. Mar. Sci. 6. doi: 10.3389/fmars.2019.00833
van Oppen M. J. H., Blackall L. L. (2019). Coral microbiome dynamics, functions and design in a changing world. Nat. Rev. Microbiol. 17, 557–567. doi: 10.1038/s41579-019-0223-4
van Oppen M. J. H., Oliver J. K., Putnam H. M., Gates R. D. (2015). Building coral reef resilience through assisted evolution. Proc. Natl. Acad. Sci. 112 (8):2307–2313. doi: 10.1073/pnas.1422301112
Volkmer B., Heinemann M. (2011). Condition-dependent cell volume and concentration of Escherichia coli to facilitate data conversion for systems biology modeling. PLoS One 6 (7):e23126. doi: 10.1371/journal.pone.0023126
Weis V. M. (2008). Cellular mechanisms of Cnidarian bleaching: stress causes the collapse of symbiosis. J. Exp. Biol. 211, 3059–3066. doi: 10.1242/jeb.009597
Wilson K., Li Y., Whan V., Lehnert S., Byrne K., Moore S., et al. (2002). Genetic mapping of the black tiger shrimp Penaeus monodon with amplified fragment length polymorphism. Aquaculture 204, 297–309. doi: 10.1016/S0044-8486(01)00842-0
Xiang T., Hambleton E. A., DeNofrio J. C., Pringle J. R., Grossman A. R. (2013). Isolation of clonal axenic strains of the symbiotic dinoflagellate Symbiodinium and their growth and host specificity. J. Phycol 49 (3), 447–458. doi: 10.1111/jpy.12055
Yellowlees D., Rees T. A. V., Leggat W. (2008). Metabolic interactions between algal symbionts and invertebrate hosts. Plant Cell Environ. 31, 679–694. doi: 10.1111/j.1365-3040.2008.01802.x
Yoch D. C. (2002). Dimethylsulfoniopropionate: its sources, role in the marine food web, and biological degradation to dimethylsulfide. Appl. Environ. Microbiol. 68, 5804–5815. doi: 10.1128/AEM.68.12.5804-5815.2002
Keywords: bacteria, probiotics, reactive oxygen species, Symbiodiniaceae, coral
Citation: Heric K, Maire J, Deore P, Perez-Gonzalez A and van Oppen MJH (2023) Inoculation with Roseovarius increases thermal tolerance of the coral photosymbiont, Breviolum minutum. Front. Ecol. Evol. 11:1079271. doi: 10.3389/fevo.2023.1079271
Received: 25 October 2022; Accepted: 21 July 2023;
Published: 10 August 2023.
Edited by:
Kevin R. Theis, Wayne State University, United StatesReviewed by:
Lauren Frances Messer, University of Stirling, United KingdomCopyright © 2023 Heric, Maire, Deore, Perez-Gonzalez and van Oppen. This is an open-access article distributed under the terms of the Creative Commons Attribution License (CC BY). The use, distribution or reproduction in other forums is permitted, provided the original author(s) and the copyright owner(s) are credited and that the original publication in this journal is cited, in accordance with accepted academic practice. No use, distribution or reproduction is permitted which does not comply with these terms.
*Correspondence: Karla Heric, a2FybGEuaGVyaWNAdW5pbWVsYi5lZHUuYXU=
Disclaimer: All claims expressed in this article are solely those of the authors and do not necessarily represent those of their affiliated organizations, or those of the publisher, the editors and the reviewers. Any product that may be evaluated in this article or claim that may be made by its manufacturer is not guaranteed or endorsed by the publisher.
Research integrity at Frontiers
Learn more about the work of our research integrity team to safeguard the quality of each article we publish.