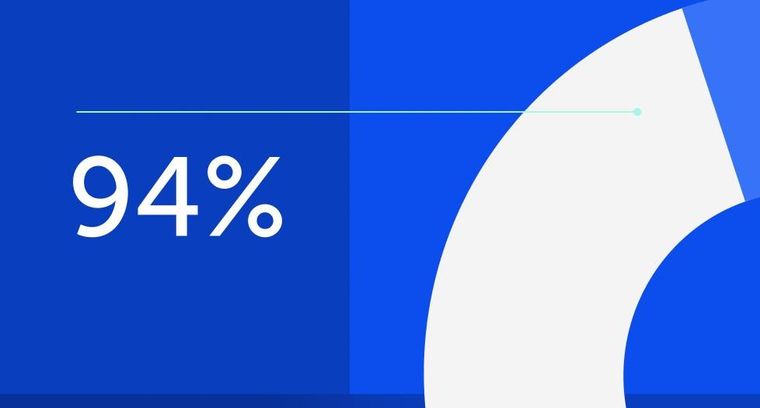
94% of researchers rate our articles as excellent or good
Learn more about the work of our research integrity team to safeguard the quality of each article we publish.
Find out more
ORIGINAL RESEARCH article
Front. Ecol. Evol., 10 July 2018
Sec. Population, Community, and Ecosystem Dynamics
Volume 6 - 2018 | https://doi.org/10.3389/fevo.2018.00096
This article is part of the Research TopicEssential Biomolecules Caught in the Food Web? View all 8 articles
PUFA (polyunsaturated fatty acids) content of food sources, in particular ω3 long-chain PUFA with three or more double bonds, are considered essential for growth, reproduction, and neural development of higher animals. Surprisingly, and in contrast to aquatic ecosystems, ω3 long-chain PUFA seem not widely available in terrestrial food webs. Far-reaching ideas indeed proclaim aquatic ecosystems as the principal source of these long-chain PUFA in the whole biosphere, including inhabitants of terrestrial ecosystems. Interestingly, de novo synthesis of ω3 long-chain PUFA, which requires the presence of Δ12 (fat-2) and ω3 (fat-1) desaturases absent in vertebrates, has been observed in nematodes, such as Caenorhabditis elegans. This raises the question if nematodes or other soil invertebrates present an important trophic link offering substantial supply in these valuable nutritional compounds in terrestrial food webs. This work followed the dietary routing of fatty acids of different C. elegans strains, including mutants defective in the PUFA biosynthesis, to two omnivorous Collembola species, Folsomia candida and Protaphorura fimata. The laboratory approach comprised microcosms offering binary links under various feeding conditions and analyzed growth, fecundity and dietary preference of consumers. Collembola did not prefer individual C. elegans strains as food source but they clearly reflected the PUFA-richness or -poorness of their nematode prey in their neutral lipid fraction. Moreover, Collembola did benefit from ω3 long-chain PUFA rich diet, as shown by significant weight gain and increased number of laid eggs. Interestingly, the comparatively high PUFA-content of Collembola's phospholipid fraction remained unchanged, even in response to almost PUFA-depleted nematode prey, suggesting that these Collembola species also possess the metabolic capability to de novo synthesize PUFA, including ω3 long-chain forms. These findings broaden the basis of long-chain PUFA sources in terrestrial food webs and question the impact of aquatic ecosystems as principal source.
The fatty acid composition of any consumer is greatly influenced by its diet because it is more energy efficient to incorporate dietary fatty acids into body tissue without modification, a process termed dietary routing (Pond, 1981; Ruess and Chamberlain, 2010). As part of lipids, fatty acids serve diverse functional roles in cells, particularly as energy storage molecules (i.e., neutral lipids) and structural components of membranes (i.e., phospholipids). For the latter, the occurrence and number of double bonds in fatty acids has profound effects on, e.g., the transition temperature, allowing for regulation of membrane fluidity and permeability (Stukey et al., 1989; Cronan, 2003; Ma et al., 2015). Monounsaturated fatty acids (MUFA) and polyunsaturated fatty acids (PUFA) are categorized according to the C-atom counting from the terminal methyl group (ω end) to the nearest double bond resulting in characteristic “ω families,” e.g., ω3 and ω6. This is biologically meaningful because these molecules (and derived metabolites) are not only metabolically distinct but can have specific physiological functions (Simopoulos, 2000). Surprisingly, metazoans, at least vertebrates, can obtain the parent C18-PUFA linoleic acid (LA; 18:2 ω6) and α-linolenic acid (ALA; 18:3 ω3) only from food. The complete de novo biosynthesis of long-chain (≥C20) PUFA including arachidonic acid (AA; 20:4 ω6), eicosapentaenoic acid (EPA; 20:5 ω3), and docosahexaenoic acid (DHA; 22:6 ω3) was considered for many years to be mainly limited to aquatic organisms, such as algae, photoautotrophic cyanobacteria, some heterotrophic protists and fungi as well as a minority of bacteria living in the deep-sea (Gladyshev et al., 2013). Two very recent analyses show that the marine oligochaete, white worm, Enchytraeus albidus (Fairchild et al., 2017) and probably multiple aquatic invertebrate species (Kabeya et al., 2018) have the ability to produce long-chain ω3 PUFA de novo. Consequently, Kabeya et al. (2018) suggests that these numerous widespread and abundant invertebrates make significant contributions to ω3 long-chain PUFA production beyond that coming from marine microbes.
In terrestrial food webs, plants are generally the major source of PUFA. ω3 PUFA dominate in leaves while ω6 PUFA dominate in seeds, however, the PUFA from plant resources are rarely longer than 18 carbons atoms. Vertebrates can further elongate and desaturate dietary ω6 LA and ω3 ALA to form various long-chain PUFA but they are unable to interconvert these two types of PUFA. Moreover, in most omnivorous vertebrates studied so far (including humans), only around 5% of ALA is converted to long-chain ω3 PUFA (Davis and Kris-Etherton, 2003; Wall et al., 2010). In this respect, the PUFA content of food sources, in particular long-chain PUFA with three or more double bonds is also considered essential for growth, reproduction, and neural development (Brett and Müller-Navarra, 1997; Müller-Navarra et al., 2000; Goedkoop et al., 2007). Of particular interest here is that PUFA abundance and pattern vary between food sources, which in turn can affect consumer physiology in vertebrates (e.g., thermoregulation, exercise performance; Hulbert and Abbott, 2012) and in invertebrates (e.g., precursor for hormones, membrane structure; Stanley-Samuelson et al., 1988).
Generally, ω3 long-chain PUFAs seem not widely available in terrestrial food webs (Crawford et al., 1999; Innis, 2011; Abedi and Sahari, 2014), whereas marine systems are rich in long-chain PUFAs, as microalgae at the food web base contain 20–50 % of their dry weight as ω3 forms (Dwyer et al., 2003). Trophic transfer of these fatty acids from phytoplankton to zooplankton and along the food chain to vertebrates was frequently reported (Müller-Navarra et al., 2000; Budge et al., 2006; Pond et al., 2006; Bell and Tocher, 2009). Far-reaching ideas proclaim aquatic ecosystems in general as the principal source of the ω3 long-chain PUFAs for many omnivorous and carnivorous animals in the biosphere, including the consumers in terrestrial ecosystems (Gladyshev et al., 2013; Colombo et al., 2016). However, little is known about the specific nutritional requirements of most species, and this is especially true for PUFAs generally essential in vertebrates (Hulbert and Abbott, 2012).
For terrestrial inhabitants, complete de novo biosynthesis of long-chain PUFAs is reported for individual soil photoautotrophs (algae, cyanobacteria) (Bigogno et al., 2002; Bhagavathy et al., 2011) and heterotrophs (protozoa, fungi) (Zelles, 1999; Hu et al., 2011). Moreover, a growing body of research on PUFA confirmed the ability to de novo synthesize the ω6 PUFA LA for a number of terrestrial invertebrate species, representing different taxonomic classes: Insecta (Borgeson et al., 1990), Gastropoda (Weinert et al., 1993), Arachnida (Aboshi et al., 2013), and Collembola (Malcicka et al., 2017). Corresponding genetic evidence succeeded so far for two sequenced Δ12 fatty acid desaturase genes of insects (Zhou et al., 2008). Clear biochemical and genetic evidence in respect to de novo synthesis of ω3 long-chain PUFA is, however, still very rare in the animal kingdom and so far limited to nematodes. Rothstein and Götz (1968) were the first in demonstrating unequivocal de novo synthesis of ω3 (and ω6) long-chain PUFA by a metazoan organism, the vinegar eelworm Turbatrix aceti. The first detailed genetic analyses on the complete biosynthetic pathway of PUFA were performed with Caenorhabditis elegans. These studies revealed an entire range of elongases and desaturases including Δ12 (FAT-2) and ω3 (FAT-1) fatty acid desaturases absent in vertebrates, allowing a complete de novo synthesis of long-chain PUFA (Watts and Browse, 2002). Later this genetic trait was also found in the C. elegans sister species (Rhabditidae). In addition, fat-1 orthologs were detected by whole genome sequencing in the free-living nematode genera Heterorhabditis (Heterorhabditidae), Rhabditophanes (Alloionematidae) and Steinernema (Steinernematidae), suggesting a ω3 FA desaturase activity in these nematodes, too.
Nematodes are well known to function as key players in terrestrial food webs (Moore and Hunt, 1988; Ferris, 2010; Yeates, 2010). They can reach densities of several million individuals per square meter soil and their communities often comprise more than 100 species (Yeates et al., 2000). Nematode structural diversity reflects the variety of ecologic niches they occupy. Many feed on bacteria and thus mobilize nutrients and minerals bound in microbial biomass, thereby co-regulating the rate of nutrient cycling. Other nematodes feed on algae, fungi, plants or live as omnivores and predators (Yeates et al., 1993). Nematodes are in turn the food of other decomposers, predominantly microarthropods, and provide an important link between microbial and faunal food web. In sum, nematodes form key-biota in the soil bacterial, fungal and root channel, the main fluxes of energy and matter in soil food webs (Moore and Hunt, 1988; Scheu et al., 2005). Recent studies using lipid pattern and stable isotope analyses suggest that a wide range of predators rely on these channels (Crotty et al., 2011; Pollierer et al., 2012) and nematodes represent presumably one of the most important connections between the microbial and faunal food web (Ferlian et al., 2012; Ferlian and Scheu, 2014; Pausch et al., 2016).
These data together with the remarkable physiological capacity of nematodes to produce ω3 (and ω6) long-chain PUFA raise the question if nematodes and/or other soil invertebrates present an important trophic link offering substantial supply in these valuable nutritional compounds. We hypothesize that nematodes enhance or even determine the availability of these important nutrients along the soil food chain. Moreover, their biochemical food quality significantly influences not only the flux of energy and matter in soil food webs but also substantially determines consumer's growth and reproduction. To test this hypothesis we designed model food chains ranging over three trophic levels, from Escherichia coli as primary decomposer at the food web base over C. elegans as bacterial feeder and primary consumer to two nematophagous Collembola species Folsomia candida and Protaphorura fimata as omnivores at the top. Using C. elegans fat-mutant strains in addition to wild type as food sources allowed to offer diets being almost free or very rich in ω3 and ω6 long-chain PUFA as well as diets containing either ω3 or ω6 long-chain PUFA. All organisms were subject of qualitative and quantitative analyses of fatty acid pattern performed by gas chromatography (GC) and mass spectrometry (MS). Moreover, the reproductive success, biomass growth and food preference of both Collembola species were determined. By doing so we demonstrate that Collembola benefit from the ω3 (and ω6) long-chain PUFA supply with their diet, although our studies also found hints that these invertebrates are able to synthesize these important nutritional compounds de novo.
The Escherichia coli Migula, 1895 Castellani and Chalmers 1919 strain OP50 served as food source for all Caenorhabditis elegans Maupas, 1896 cultures. Both organisms were maintained on nematode growth medium (NGM) agar plates according to standard procedures (Brenner, 1974; Sulston and Hodgkin, 1988). The C. elegans wild type strain used in this study was Bristol N2; the mutant strains were BX24, fat-1(wa9) which produces an inactive ω3 FA desaturase (FAT-1) and BX26, fat-2(wa17) which has an inactive Δ12 FA desaturase (FAT-2) and thereby blocked one step earlier in the PUFA biosynthesis than fat-1. All strains were kindly provided by the Caenorhabditis Genetics Center (University of Minnesota, Twin Cities, USA). In the case of fat-2, an additional supplementation with eicosapentaenoic acid (EPA) was performed to generate nematodes containing substantial amounts of EPA but being otherwise almost free of PUFA. First, NGM agar was supplemented with 80 μM EPA sodium salt (Santa Cruz Biotechnology, USA) according to Deline et al. (2013). Second, pure EPA (Cayman Chemical, USA) was mixed with OP50 bacterial suspension and seeded on the supplemented plates at final concentration of 80 μM in the bacterial lawn (Zhou et al., 2015). Preliminary tests proved that this combined supplementation resulted in the highest EPA uptake of fat-2 nematodes (data not shown). In sum, this resulted in four different nematode diets offered to Collembola: (i) C. elegans WT - wild type (control), (ii) C. elegans fat-1- no ω3 LC-PUFAs, (iii) C. elegans fat-2- no LC-PUFAs, and (iv) C. elegans fat-2 plus EPA - no LC PUFAs except EPA.
Starting with a chunk of a just starved plate, rich in L1 juveniles, cultures of all three strains as well as fat-2 plus EPA supplementation as fourth condition were maintained for four to five (in case of fat-2) days at 20°C. The harvest was achieved by rinsing the well-fed, mainly adult nematodes from NGM plates with ice-cold M9 buffer. Nematodes were allowed to settle down on ice by gravity flow, subsequently they were washed two-times with fresh M9 buffer. After that, a nematodes/M9 buffer suspension (2:1 v/v) was ready to transfer as exclusive food to the Collembola cultures. For lipid extraction of the different C. elegans strains, nematodes were separated by filtering through a 10 μm gauze membrane by using a filter unit type 16510 from Sartorius AG (Germany). The resulting filter cake with mainly adult nematodes was scraped off by using a small spatula, weighed (~50 mg aliquots, n = 6), transferred in clean, chloroform rinsed 10 mL glass tubes (equipped with a Teflon coated screw cap) and finally frozen at −20°C.
For comparison reason, also fatty acid pattern of E. coli OP50 was determined. For this, bacteria were cultivated in liquid LB media overnight, harvested by centrifugation at 3,000 × g for 15 min and washed three times with M9 buffer. About 50 mg portions (n = 6) of the final pellet were weighed, transferred to 10 mL glass tubes and frozen at −20°C until lipid extraction.
The examined Collembola were Folsomia candida Willem, 1902, a species living in the litter and top soil, and Protaphorura fimata Gisin, 1952 a species inhabiting deeper soil layers. For maintenance, mass cultures of Collembola were reared in soil mesocosoms in the laboratory, fed weekly with baker's yeast (Saccharomyces cerevisiae). For the experiments, specimens were transferred (use a clean suction tube or a brush) into plastic microcosms (height 4.5 cm, 7 cm diameter) with a 1 cm thick layer of plaster of Paris mixed with activated charcoal and deionized water in a proportion of 9:1:10 (Snider et al., 1969). For a detailed step by step instruction of Collembola cultivation and harvest see Menzel et al. (2018).
Ten freshly hatched Collembola were placed into each individual microcosms, three to six independent replicates per condition and species were applied. Collembola were fed with baker's yeast for 10 days at 20°C in the dark before switching to nematodes as diet. Every 3 days food was replaced in amounts that were not consumed by Collembola within that time. After this 10 days pre-culture, yeast were completely removed and washed nematodes were presented in several layers on a piece of fresh M9 agar (~1 cm2, 0.5 cm in high) that does not include any other nutritional source but ensures that the nematodes will not dry out. All laid eggs were counted regularly every third day. After each counting, eggs as well as exuviae and fecal pellets were removed, as Collembola feed on them thereby may alter their fatty acid pattern. After 33 and 39 days for F. candida and P. fimata, respectively, a CO2 stream was applied for 10 min to safely stun the animals and fresh weight was recorded. After that, Collembola were dried for 48 h at 60°C in a drying chamber and subsequently weighed again to obtain the dry weight.
Cultivation and harvest of Collembola intended for lipid extraction followed exactly the instruction given in the penultimate paragraph but differed with regards to the following: Instead of 10, we cultivated 25 specimen in each individual microcosm; the incubation temperature was 15°C. Moreover, after determining the fresh weight, each Collembola group (25 specimen) was transferred directly to an individual 10 mL glass tube and frozen in 1 mL methanol at −20°C.
To analyze whether or not Collembola feed preferentially on the different C. elegans strains offered, two different experimental approaches were followed. First, direct feeding of Collembola on nematodes was assessed. Once split Petri dishes were poured with M9 agar to a height that the central barrier still separates nematodes to both halves but does not hinder a Collembola (valid for both species) to pass. At the beginning, 15 well-fed adult nematodes of different strains were set without bacteria on each half. Then, one young adult Collembola was transferred on each half, having in total 30 nematodes and two Collembola specimen on each plate (n = 5). After 5, 24, and 48 h of incubation at 20°C in the dark the number of eaten versus still alive nematodes was counted. The food strain combinations always saw the C. elegans wild type on one side while all four variants, i.e. WT, fat-1, fat-2, and fat-2 plus EPA alternately occurred on the other.
Second, feeding on nematodes was indirectly assessed by counting fecal pellets deposited by Collembola around the food source (Scheu and Simmerling, 2004). This experiment was performed in the same microcosms and diet variants as described above. However, instead of one, we offered two oppositely arranged M9 agar pieces carrying the different nematode food and 10 young adult specimen of each Collembola species were placed in. Additionally, a ~5 cm2 piece of white wet filter paper was set under each agar block to allow easy pellet counting by using a dissecting microscope, carried out after 5, 24, and 48 h of incubation time. Again, five replicates were set up per Collembola species (2) and food combination (4).
For fatty acid pattern analysis the lipids from Collembola were extracted according to Ruess et al. (2004); described in great detail just recently (Menzel et al., 2018). Most important, after extraction each sample was loaded to a silica acid column (0.5 g silicic acid, mesh size 100–200 lm) to separate neutral lipid fatty acids (NLFA) from the phospholipid fatty acids (PLFA). Finally, saponification and methanolysis of lipids was performed following the procedures given for the Sherlock Microbial Identification System (MIDI Inc., Newark, Del.). Briefly, saponification of lipids was conducted in a sodium hydroxide-methanol solution, followed by acid methanolysis in HCl-methanol, extraction of fatty acid methylesters (FAMEs) into hexane/methyl tertiary butyl ether and a wash step with aqueous NaOH (for details see Ruess et al., 2004). The lipid-containing phase was transferred to GC vials and stored at −20°C until analysis. In the case of the food samples, E. coli and C. elegans, the NLFA/PLFA separation was omitted; here only FAMEs of the total lipid fatty acid (TLFA) have been extracted accordingly.
FAMEs were quantified and identified by an Agilent 7890A GC and flame ionization detector (FID), equipped with an HP Ultra 2 capillary column (25 × 0.2 mm i.d., film thickness 0.33 μm). The temperature program started with 191°C and increased by 10°C min−1 to 286°C followed by 60°C min−1 to 310°C (held for 2 min). The injection volume was 2 μL, the temperature 250°C, and the split ratio 1:30. Hydrogen was used as carrier gas. Fatty acids were identified with the Sherlock Pattern Recognition Software (MIDI Inc., USA) by comparison of their retention times to a standard mixture.
Fatty acid identity was further validated by follow-up qualitative analysis via an Agilent series 7890A gas chromatograph coupled to a mass selective detector (Agilent 7000 Triplequadrupole). The system was equipped with a polar DP-23 capillary column (60 m × 0.25 mm i.d., film thickness 0.15 μm). The injection volume was 1 μL, the split ratio 1:12.5, and the initial set point 250°C. Helium was used as carrier gas. The oven temperature program started with 130°C and increased by 9°C min−1 to 160°C (hold time 5 min), followed by 29°C min−1 to 170°C (hold time 7 min), and 11°C min−1 to 230°C (hold time 5 min). The transfer line temperature was 280°C and a mass range of 40–400 m/z was monitored in the scan mode.
Data were analyzed using one-way ANOVA. If ANOVA assigned statistical significance, a post hoc test was used and treatments were compared pairwise to each other by Bonferroni's test. All statistical analyses were performed using SigmaStat 3.5 (Systat Software Inc., USA) and are presented with corresponding F- and P-values.
The TLFAs of E. coli OP50 which served as base of the model food chain comprised of only 14 predominantly saturated fatty acid (SAFA) mainly 16:0, cyclic fatty acids 17:0 cyclo and 19:0 cyclo and fatty acids shorter than C16 (mainly 14:0, 14:0 3-OH and 12:0) (Figure 1, Supplementary Table 1). PUFA were entirely absent in E. coli OP50, whilst only C16 and C18 MUFA were present in lower concentrations (Supplementary Table 1).
Figure 1. Amount of fatty acids neutral lipid (NLFA) and phospholipid (PLFA) fatty acid fractions (in nmol fatty acid mg dry weight−1) of Collembola consumers (left - Folsomia candida, right - Protaphorura fimata) and total lipids of food sources (Escherichia coli OP50 and the different Caenorhabditis elegans strains). Short/branch/odd FA – (12:0, 13:0, 13:0 iso), 14:0, (14:0 iso, 14:1 ω7, 14:1 ω9, 15:0, 15:0 iso), 16:0 iso, 17:0, 17:0 iso and 17:1 ω8; Cyclo-FA – 17:0 cyclo, 19:0 cyclo; SAFA – 16:0, 18:0, 20:0; MUFA – (16:1 ω5), 16:1 ω7, (18:1 ω5), 18:1 ω7, 18:1 ω9, (20:1 ω6) and 20:1 ω9; ω6-PUFA – 18:2 ω6, 18:3 ω6, 20:2 ω6, 20:3 ω6 and 20:4 ω6; ω3-PUFA – 20:5 ω3. Fatty acids in parenthesis were only detected in NLFA samples and fatty acids of carbon chain-lengths < 14 were not detected in Collembola samples. One-way ANOVA followed by Bonferroni post-hoc test with * as P < 0.05 when compared to the corresponding wild type fed control. The complete data set and statistical evaluations are presented in the Supplementary Tables 1–5. WT – wild type, fat-1- no ω3 long-chain PUFAs, fat-2- no long-chain PUFAs, EPA – Eicosapentaenoic acid.
When the C. elegans wild type N2 feed on E. coli OP50, the nematode's TLFA pattern reflects the composition of the food bacteria. However, the worms de novo synthesize a large number of additional fatty acids such as branched-chain FA (15:0 iso and 17:0 iso) and particularly MUFA (18:1 ω7) and PUFA (LA, DGLA - 20:3 ω6, AA and EPA) (Figure 1, Supplementary Table 1), resulting in proportions of 28.0 ± 3.3% MUFA and 27.5 ± 3.4% PUFA of the total FA content (all data are means ± SD, n = 6). EPA corresponding to 12.6 ± 2.5% of the TLFA formed almost half of the PUFA. This picture changed in the fat-1 mutant strain. The EPA content was close to zero whilst the AA content increased almost tenfold and the DGLA content approximately doubled (Figure 1, Supplementary Table 1). The fat-2 mutant strain showed a PUFA depleted TLFA pattern with all C18 and C20 PUFA close to zero or below detection level. In contrast, the MUFA oleic acid (18:1 ω9) was greatly enriched (Figure 1, Supplementary Table 1). In sum, the different strains provided the intended PUFA source spectra to consumers with: (i) WT - both ω6 PUFA and ω3 EPA, (ii) fat-1- only ω6 PUFA, and (iii) fat-2- almost no PUFA. To complete this spectra by a fourth condition, (iv) fat-2 + EPA - only EPA as ω3 PUFA, we supplemented fat-2 nematodes for several days with EPA. The EPA content in these nematodes increased to 4.0 ± 0.5%, about a third of the portion in the wild type (Figure 1, Supplementary Table 1). Interestingly, even though the PUFA and MUFA contents in the C. elegans strains are different, the total content of fatty acids remained unchanged as assigned by the amount in nmol fatty acid per mg dry weight (Table 1).
In the next step, the fatty acid patterns of the two nematophagous Collembola, F. candida and P. fimata, were determined. Although slight differences exist, the common fatty acid content of NLFA and PLFA for both species did not significantly vary between the different food conditions (Table 1).
Figure 1 presents an overview of both NLFA and PLFA contents of F. candida (left) and P. fimata (right), respectively, and allows the comparison with the TLFA pattern of the corresponding prey nematodes shown in the center of the figure. It is apparent that NLFA patterns mainly resembled the TLFA pattern of consumed food nematodes, even if the proportion of cyclo-FA decreased whilst that of MUFA increased in the Collembola. Collembola's PUFA content of NLFA were closely related to the pattern of consumed food: evidently PUFA in case of fat-1 nematode food there was more ω6 than ω3, while in the case of fat-2 food an approximate halved PUFA content and, in comparison to the latter a compensatory effect when EPA was supplemented. It is striking, however, that the PUFA did not absolutely disappear in the absence of a dietary source, i.e., when Collembola were fed with fat-2 nematodes.
Focusing on the relative portions of all detected PUFA in the NLFA (NLPUFA; Figures 2A,B) shows that their portion in both Collembola species was much lower when they fed on fat-2 nematodes as compared to wild type or fat-1 [for F. c. F(3, 20) = 156.28, P < 0.001; for P. f. F(3, 8) = 124.03, P < 0.001]. The rescue effect when fed EPA supplemented fat-2 worms was limited to the EPA content, which was significantly higher compared to feeding on fat-2 worms in both F. candida and in P. fimata. However, these did not reach the higher values as in the wild type fed Collembola. Interestingly, F. candida consuming wild type or fat-1 worms possessed a significantly higher EPA portion in the neutral lipids than P. fimata specimen did with the same diet. Obviously, species-specific differences allow F. candida a higher enrichment of NLFA with EPA; the same applies to AA.
Figure 2. Profiles of polyunsaturated fatty acids (PUFA) in the Collembola species Folsomia candida and Protaphorura fimata, as mol % relative to the total fatty acid content of the neutral lipid (NLFA) and phospholipid (PLFA) fatty acid fractions. (A) NLFA of F. candida, (B) NLFA of P. fimata, (C) PLFA of F. candida, and (D) PLFA of P. fimata. Statistical differences were tested for each PUFA within each experimental set and Bonferroni post-hoc test results are represented by letters and full or empty symbols. Matching (or missing) letters and symbols indicate no statistical difference. Stars indicate a significantly lower proportion of total PUFA on the NLFA, when compared to the corresponding wild type sample. All corresponding F- and P-values are given in Supplementary Table 6, together with means and standard deviations.
In both species, PLFA were characterized by a higher content of SAFA and lower MUFA, when compared to their occurrence in NLFA (Figure 1). Moreover, the PLFA content in fat-2 fed Collembola was significantly reduced (Figure 1). Most strikingly was the finding that the overall composition of PLFA in both species did not vary much between the different feeding conditions. The only exception was the AA-rich/EPA-free fat-1 food, inducing a significant shift of the EPA/AA ratio toward AA in both species (Figures 2C,D). Interestingly, the fat-2 PUFA depleted food did not affect the PUFA composition, both EPA and AA remained an unabated part of the PLFA. In P. fimata the PUFA-content in the PLFA was significantly higher than in the NLFA (Figure 2D). This also applied to F. candida animals fed fat-2 (Figure 2C). The preference to serve PLFA before the NLFA with PUFA, even under the condition of PUFA-free diet (fat-2) was evident in the significant decrease of the NL-PUFA/PL-PUFA ratio, whereas the NLFA/PLFA ratio was unaltered by the different feeding conditions (Table 1). The effect even increased if EPA was supplemented, as lowest NL-PUFA/PL-PUFA ratios were reached when both species were fed on fat-2 + EPA (Table 1). Summarizing, Collembola species are obviously able to keep their PLFA composition constant, whereas NLFA pattern mainly reflected the dietary routing of consumed fatty acids.
Collembola's fitness, as assessed by reproductive output and weight gain, was affected by the different food sources. Both species laid first eggs a few days after settling in microcosms offering the different nematode strains as exclusive diet, F. candida laid ~3–4 times more eggs than P. fimata (Figures 3A,B, take into account the different Y-axis scaling). From the start of egg laying until the end of the experiment, fat-2 fed Collembola laid fewer eggs than the animals kept under different conditions (Figures 3A,B). EPA supplemented fat-2 nematodes as diet significantly compensated for this impairment allowing Collembola to achieve a reproductive output at a similar level as that of fat-1 fed animals (Figures 3A,B). This level, however, did not reach the reproduction of wild type fed Collembola for both F. candida and P. fimata (Figures 3A,B).
Figure 3. Cumulative numbers of eggs (A,B) and final dry weight per Collembola individual (C,D) of two experimental scenarios: Folsomia candida (A + C) and Protaphorura fimata (B + D), both fed on the four different Caenorhabditis elegans food sources [WT – wild type, fat-1- no ω3 LC-PUFAs, fat-2- no LC-PUFAs, fat-2 + EPA (Eicosapentaenoic acid)]. Bars (A + B) represent standard deviations, box plots (C + D) show each outlier. Statistical differences were tested within each experimental set. Significantly different Bonferroni post-hoc test results (P < 0.001) are represented by letters, the corresponding F-values are: (A) F(3, 16) = 21.15 (n = 5), (B) F(3, 16) = 28.48 (n = 5), (C) F(3, 32) = 10.06 (n = 9) (D) F(3, 32) = 16.66 (n = 9). Matching letters indicate no statistical difference.
When we determined the dry weight of Collembola after finishing the cultivation, we found that fat-2 fed animals were significantly lighter than fat-1 or wild type fed animals (Figures 3C,D). The intended rescue by EPA supplementation succeeded significantly for F. candida (Figure 3C). Also in case of P. fimata specimen showed on average a higher weight, but the values did not reach a significant difference (Figure 3D). Summarizing, Collembola benefit from especially EPA-rich food, they laid more eggs and gained more weight.
Finally, it was determined whether or not the both Collembola species prefer a PUFA-rich (wild type) nematode diet to an almost PUFA-free (fat-2) or an AA-rich/EPA-free (fat-1) nematode diet. Our first approach focused on the per capita consumption of Collembola as predators, given the choice between the wild type and another nematode strain. No significant differences were detected after 5, 24, or 48 h when almost all of the offered 2 × 15 nematodes had been consumed (Figures 4A,B). The only two exceptions from this were F. candida specimen, initially preferring fat-1 nematode to wild type once and P. fimata specimen which have preferred wild type to fat-2 nematodes at 5 h incubation (Figure 4B). In a second approach the Collembola residence time at the prey was assessed. In this assay the number of fecal pellets, deposited near the food source (offering a huge amount of nematodes) were counted. After 5, 24, or 48 h also the count of fecal pellets showed no significant differences between the different food sources (Figures 4C,D). In sum, irrespective of the fact that these C. elegans strains have a different food quality both analyzed Collembola species are unable to distinguish between C. elegans wild type and fat-1 or fat-2 mutant strains when selecting their prey.
Figure 4. Food preferences of Folsomia candida and Protaphorura fimata for different Caenorhabditis elegans strains [WT – wild type, fat-1- no ω3 LC-PUFAs, fat-2- no LC-PUFAs, fat-2 + EPA (Eicosapentaenoic acid)]. Shown are two experimental scenarios: Consumed prey animals (A,B) presented on once split Petri dishes and fecal pellets (C,D) deposited around the offered food. Box plots show each outlier (n = 5). Statistical differences were tested within each experimental set. The two significantly different Bonferroni post-hoc test results are represented by stars, the corresponding F- and P-values are: (A) F(1, 8) = 7.03, P = 0.029; (B) F(1, 8) = 19.69, P = 0.002.
Our study demonstrates that Collembola can benefit from a particularly ω3 long-chain PUFA rich diet, namely EPA, although they are additionally able to de novo synthesize this important PUFA. This key message suggests that the biosynthesis of ω3 (and also ω6) long-chain PUFA is costly and cannot be executed in unlimited extent by the observed Collembola species.
In this respect, it is of particular interest that PUFA content of free-living nematodes was suggested to be controlled by biosynthesis rather than diet and that these regularly contain ω3 long-chain PUFA (Krusberg, 1972; Hutzell and Krusberg, 1982). Collembola specimen, however, fed differing diets including a common soil fungus (Cladosporium cladosporioides), a bacterial feeding nematode (Panagrellus redivivus), mays (Zea maize), or alder (Alnus glutinosa), showed the highest content of ω3 long-chain PUFA on the nematode diet (Chamberlain et al., 2005). This last finding notably coincided with a strong increases in egg-laying rate and dry weight gain of the same Collembola species (F. candida and Proisotoma minuta) re-analyzed in a follow-up study (Chamberlain et al., 2006). Even in a freshwater systems, P. redivivus has been recommended as a suitable food source for first-feeding fish since carp larvae grown on nematodes showed a higher survival rate than the control group fed traditionally with zooplankton (Schlechtriem et al., 2004). Thus, a limited amount of ω3 long-chain PUFA is likely to be as crucial for terrestrial consumers as it is for aquatic consumers (Twining et al., 2016). Nematodes could represent an alternative to primary producer-derived ω3 long-chain PUFA that had previously received less attention but which may be essential for larger omnivores and carnivores. It is important to add that such observations (and considerations) may not exclusively restricted to ω3 long-chain PUFA. The jewel wasp Nasonia vitripennis which demonstrably possesses intrinsic Δ12 desaturase activity to de novo synthesize the ω6 PUFA LA prefers (female) LA-rich hosts for oviposition and produces (male) significantly more sex pheromone when hosts were additionally supplied with LA (Blaul and Ruther, 2011; Brandstetter and Ruther, 2016).
The relatively high amount of long-chain PUFA in free-living nematodes (and entomopathogenic as well) was suggested as an adaptation to survive periods of environmental stress (Selvan et al., 1993a,b). However, such conditions apply to many soil animals and may have resulted in comparable anabolic pathways for synthesizing long-chain ω3 (and ω6) PUFA. Indeed, biochemical analyses revealed unusually high proportions of long-chain ω3 PUFA in earthworms (Petersen and Holmstrup, 2000) and Collembola (Chamberlain and Black, 2005). For Lumbricus terrestris, Sampedro et al. (2006) proposed the existence of a microbial community in the gut that provides fatty acids to the earthworm, including high amounts of AA and EPA. A characterization of the nature of this microbiome did not occur so far. To the best of our knowledge soil bacteria can ruled out as a source of long-chain PUFA. Although Fungi's and protozoa's most abundant fatty acids tend to be of carbon chain-lengths 16 and 18 with varying degrees of unsaturation (Losel, 1988), they can possess a wide range of long-chain fatty acids (Certik and Shimizu, 1999). Most interestingly, also nematodes were detected to make a contribution to the nutrition of a tropical earthworm (Dash et al., 1980); a scenario that could be possible in the case of Lumbricus terrestris, too.
Even though Collembola's gut can also serve as vector and habitat for microorganisms, mainly bacteria but also fungi, Thimm et al. (1998) as well as Chamberlain and Black (2005) were sure that the high proportions of ω3 (and ω6) long-chain PUFA determined in various Collembola species exclusively fed on S. cerevisiae for generation in the laboratory must be present through de novo biosynthesis. Our data support this finding. S. cerevisiae does not naturally contain long-chain PUFA, C. elegans fat-2 contains only traces of EPA whereas AA was not detectable at all. Chamberlain and Black (2005) observed 24.6–48 mol % long-chain PUFA in the PLFA and 4.4–9.2 mol % in the NLFA fraction. In this work, 23.5–24.8 mol % of the PLFA and 3.4–7.2 mol % of the NLFA were determined as long-chain PUFA when the offered food was PUFA-depleted. These data strongly supports the idea that Collembola, at least the observed species, can ensure a specific minimum share of long-chain PUFA in the PLFA and NLFA, respectively. The methylene-interrupted cis-dienoic structure of PUFA seems to be important for the structural integrity and functionality of biological membranes of eukaryotes (Sargent et al., 1995) explaining the higher PUFA portion in the PLFA. The rather constant composition of PLFA is ensured by regulation at the cellular level (Stanley-Samuelson et al., 1988) but requires de novo biosynthesis and/or dietary intake of the PUFA. The NLFA, in contrast, do better reflect changes in the diet than the PLFA; our data confirm in this respect previous findings (Chen et al., 2001; Haubert et al., 2004).
In addition to individual analysis, we also analyzed the NLFA/PLFA ratio to measure the physiological status of Collembola according to Bååth (2003), with a higher ratio indicating an increase in fat storage due to an excess of energy in the resource consumed. When Collembola were fed with different bacteria, NFLA/PLFA ratios ranged from 13 to 23 for F. candida and from 5 to 33 for P. fimata (Haubert et al., 2006). The ratios dropped when Baker's yeast was used as exclusive food source: 7–10 for F. candida and about 4 for two Protaphorura sister species i.e., P. armata and P. minuta (Chamberlain and Black, 2005). In this study, ratios for both Collembola species ranged from about 16 to 19 indicating that C. elegans represents an average food quality for both F. candida and P. fimata. That these ratios did not change in response to the different C. elegans food strains reflects their over-all equivalence as food sources, varying only in the PUFA compositions but not in the total fatty acid content. However, this lack in specific PUFA in the nematode diet led to a reduction of the PLFA and NLFA content of both analyzed Collembola species, though the latter was not significant. This suggests that the biosynthesis of ω3 (and also ω6) long-chain PUFA is costly. Probably it cannot be executed in unlimited extent resulting in a supply gap when exclusively feeding on PUFA depleted C. elegans nematodes as food source. This PUFA supply gap then causes directly or more indirectly a reduction of weight gain and egg laying whereby both ω3 and ω6 long-chain PUFA seems to be of vital importance. In some way, Collembola reflected with this the phenotypic impairments of their (fat-2) food animals, such as a reduced brood size, slow growth and a small shaped body (Watts and Browse, 2002; Horikawa et al., 2008).
The attraction of Collembola to chemical compounds (of food or non-food sources) is well established (Nilsson and Bengtsson, 2004; Gutiérrez-López et al., 2011; Buse and Filser, 2014) and documented also by assays used in this work (Scheu and Simmerling, 2004). This may suggest that also a preference of PUFA-rich food by F. candida and P. fimata exist; comparable to e.g., N. vitripennis females which recognize (and prefer) LA-rich hosts for oviposition (Brandstetter and Ruther, 2016). Generally, PUFA serve also in invertebrates as important precursors for a range of signaling molecules, such as eicosanoids (Stanley, 2006; Kulas et al., 2008; Vrablik and Watts, 2013) and sex or aggregation pheromones (Tillman et al., 1999; Koutroumpa and Jacquin-Joly, 2014). In the sense of a comprehensive analysis, we have consciously chosen two tests analyzing not only choice but also consumption rates. Nevertheless, for both Collembola species no differences between PUFA-rich (like wild type) and almost PUFA-free (like fat-2) C. elegans nematodes were observed. The only two significant indication, e.g., P. fimata preferred wild type instead of fat-2 after 5 h incubation; found no further confirmation at all other time points and assays. Obviously, the specific fatty acid composition of the offered C. elegans strains and their difference in nutritional valuable ω3 long-chain PUFA was not detectable for Collembola.
In conclusion, it can be stated that the source for ω3 (and especially ω6) long-chain PUFA in the soil is formed by a significantly larger basis of biota than previously assumed. The present study showed that besides primary producers (cyanobacteria, algae) and basal decomposers (protozoa, fungi) also higher trophic levels such as nematodes and Collembola represent a considerable source for these biomolecules in belowground food chains. This raises evidence that also further invertebrate species, e.g., as representatives of Clitellata, have the ability to synthesize de novo PUFA. Thus, it seems unlikely that long-chain PUFA synthesized in aquatic food webs, although clearly important, are the essential resource for soil food webs.
DG, AS, and DS cultivated the organisms and performed the bioassays with Collembola, AS performed exclusively the preference assays. Together with RM, both DG, AS, and DS performed the lipid extractions. Both RM and LR evaluated the GC/MS data. RM wrote the paper, LR revised it. RM and LR developed the idea and hypothesis of this work.
The authors declare that the research was conducted in the absence of any commercial or financial relationships that could be construed as a potential conflict of interest.
The financial support of RM and LR by the Deutsche Forschungsgemeinschaft (DFG) (RU780/11-1) is gratefully acknowledged. We thank Rainer Nehring, funded by the DFG (RU 780/10-1), for his excellent technical assistance in all GC/MS experiments. We are also grateful to Petra Heese for her technical assistance during lipid extraction. E. coli and C. elegans strains were provided by the CGC, which is funded by NIH Office of Research Infrastructure Programs (P40 OD010440). Finally, we are extremely thankful to Dr. Hazel Ruvimbo Maboreke for proofreading the manuscript.
The Supplementary Material for this article can be found online at: https://www.frontiersin.org/articles/10.3389/fevo.2018.00096/full#supplementary-material
Abedi, E., and Sahari, M. A. (2014). Long-chain polyunsaturated fatty acid sources and evaluation of their nutritional and functional properties. Food Sci. Nutr. 2, 443–463. doi: 10.1002/fsn3.121
Aboshi, T., Shimizu, N., Nakajima, Y., Honda, Y., Kuwahara, Y., Amano, H., et al. (2013). Biosynthesis of linoleic acid in Tyrophagus mites (Acarina: Acaridae). Insect Biochem. Mol. Biol. 43, 991–996. doi: 10.1016/j.ibmb.2013.08.002
Bååth, E. (2003). The use of neutral lipid fatty acids to indicate the physiological conditions of soil fungi. Microb. Ecol. 45, 373–383. doi: 10.1007/s00248-003-2002-y
Bell, M. V., and Tocher, D. R. (2009). “Biosynthesis of polyunsaturated fatty acids in aquatic ecosystems: general pathways and new directions,” in Lipids in Aquatic Ecosystems, eds M. Kainz, M.T. Brett, and M.T. Arts (New York, NY: Springer), 211–236.
Bhagavathy, S., Sumathi, P., and Jancy Sherene Bell, I. (2011). Green algae Chlorococcum humicola- a new source of bioactive compounds with antimicrobial activity. Asian Pac. J. Trop. Biomed. 1, S1–S7. doi: 10.1016/S2221-1691(11)60111-1
Bigogno, C., Khozin-Goldberg, I., Boussiba, S., Vonshak, A., and Cohen, Z. (2002). Lipid and fatty acid composition of the green oleaginous alga Parietochloris incisa, the richest plant source of arachidonic acid. Phytochemistry 60, 497–503. doi: 10.1016/S0031-9422(02)00100-0
Blaul, B., and Ruther, J. (2011). How parasitoid females produce sexy sons: a causal link between oviposition preference, dietary lipids and mate choice in Nasonia. Proc. R. Soc. Lond. B Biol. Sci. 278, 3286–3293. doi: 10.1098/rspb.2011.0001
Borgeson, C. E., de Renobales, M., and Blomquist, G. J. (1990). Characterization of the delta 12 desaturase in the American cockroach, Periplaneta americana: the nature of the substrate. Biochim. Biophys. Acta 1047, 135–140.
Brandstetter, B., and Ruther, J. (2016). An insect with a delta-12 desaturase, the jewel wasp Nasonia vitripennis, benefits from nutritional supply with linoleic acid. J. Sci. Nat. 103:40. doi: 10.1007/s00114-016-1365-0
Brett, M., and Müller-Navarra, D. (1997). The role of highly unsaturated fatty acids in aquatic foodweb processes. Freshw. Biol. 38, 483–499. doi: 10.1046/j.1365-2427.1997.00220.x
Budge, S. M., Iverson, S. J., and Koopman, H. N. (2006). Studying trophic ecology in marine ecosystems using fatty acids: A primer on analysis and interpretation. Mar. Mamm. Sci. 22, 759–801. doi: 10.1111/j.1748-7692.2006.00079.x
Buse, T., and Filser, J. (2014). Mucilaginous seeds and algal diets attract soil Collembola in preference tests. Eur. J. Soil Biol. 65:1–6. doi: 10.1016/j.ejsobi.2014.08.005
Certik, M., and Shimizu, S. (1999). Biosynthesis and regulation of microbial polyunsaturated fatty acid production. J. Biosci. Bioeng. 87, 1–14. doi: 10.1016/S1389-1723(99)80001-2
Chamberlain, P. M., and Black, H. I. (2005). Fatty acid compositions of Collembola: unusually high proportions of C20 polyunsaturated fatty acids in a terrestrial invertebrate. Comp. Biochem. Physiol. B Biochem. Mol. Biol. 140, 299–307. doi: 10.1016/j.cbpc.2004.10.016
Chamberlain, P. M., Bull, I. D., Black, H. I. J., Ineson, P., and Evershed, R. P. (2005). Fatty acid composition and change in Collembola fed differing diets: identification of trophic biomarkers. Soil Biol. Biochem. 37, 1608–1624. doi: 10.1016/j.soilbio.2005.01.022
Chamberlain, P. M., Bull, I. D., Black, H. I. J., Ineson, P., and Evershed, R. P. (2006). The effect of diet on isotopic turnover in Collembola examined using the stable carbon isotopic compositions of lipids. Soil Biol. Biochem. 38, 1146–1157.
Chen, J., Ferris, H., Scow, K. M., and Graham, K. J. (2001). Fatty acid composition and dynamics of selected fungal-feeding nematodes and fungi. Comp. Biochem. Physiol. Part B Biochem. Mol. Biol. 130, 135–144. doi: 10.1016/S1096-4959(01)00414-6
Colombo, S. M., Wacker, A., Parrish, C. C., Kainz, M. J., and Arts, M. T. (2016). A fundamental dichotomy in long-chain polyunsaturated fatty acid abundance between and within marine and terrestrial ecosystems. Environ. Rev. 25, 163–174. doi: 10.1139/er-2016-0062
Crawford, M. A., Bloom, M., Broadhurst, C. L., Schmidt, W. F., Cunnane, S. C., Galli, C., et al. (1999). Evidence for the unique function of docosahexaenoic acid during the evolution of the modern hominid brain. Lipids 34, S39–S47.
Cronan, J. E. (2003). Bacterial membrane lipids: where do we stand? Annu. Rev. Microbiol. 57, 203–224. doi: 10.1146/annurev.micro.57.030502.090851
Crotty, F. V., Blackshaw, R. P., and Murray, P. J. (2011). Tracking the flow of bacterially derived 13C and 15N through soil faunal feeding channels. Rapid Commun. Mass Spectrom. 25, 1503–1513. doi: 10.1002/rcm.4945
Dash, M. C., Senapati, B. K., and Mishra, C. C. (1980). Nematode feeding by tropical earthworms. Oikos 34, 322–325. doi: 10.2307/3544291
Davis, B. C., and Kris-Etherton, P. M. (2003). Achieving optimal essential fatty acid status in vegetarians: current knowledge and practical implications. Am. J. Clin. Nutr. 78, 640S-646S. doi: 10.1093/ajcn/78.3.640S
Deline, M. L., Vrablik, T. L., and Watts, J. L. (2013). Dietary supplementation of polyunsaturated fatty acids in Caenorhabditis elegans. J. Vis.Exp. 81:e50879. doi: 10.3791/50879
Dwyer, K. S., Parrish, C. C., and Brown, J. A. (2003). Lipid composition of yellowtail flounder (Limanda ferruginea) in relation to dietary lipid intake. Mar. Biol. (Berl.) 143, 659–667. doi: 10.1007/s00227-003-1101-0
Fairchild, E. A., Bergman, A. M., and Trushenski, J. T. (2017). Production and nutritional composition of white worms Enchytraeus albidus fed different low-cost feeds. Aquaculture 481, 16–24. doi: 10.1016/j.aquaculture.2017.08.019
Ferlian, O., and Scheu, S. (2014). Shifts in trophic interactions with forest type in soil generalist predators as indicated by complementary analyses of fatty acids and stable isotopes. Oikos 123, 1182–1191. doi: 10.1111/j.1600-0706.2013.00848.x
Ferlian, O., Scheu, S., and Pollierer, M. M. (2012). Trophic interactions in centipedes (Chilopoda, Myriapoda) as indicated by fatty acid patterns: Variations with life stage, forest age and season. Soil Biol. Biochem. 52, 33–42. doi: 10.1016/j.soilbio.2012.04.018
Ferris, H. (2010). Contribution of nematodes to the structure and function of the soil food web. J. Nematol. 42, 63–67.
Gladyshev, M. I., Sushchik, N. N., and Makhutova, O. N. (2013). Production of EPA and DHA in aquatic ecosystems and their transfer to the land. Prostag. Other Lipid Mediat. 107, 117–126. doi: 10.1016/j.prostaglandins.2013.03.002
Goedkoop, W., Demandt, M., and Ahlgren, G. (2007). Interactions between food quantity and quality (long-chain polyunsaturated fatty acid concentrations) effects on growth and development of Chironomus riparius. Can. J. Fish. Aquat. Sci. 64, 425–436. doi: 10.1139/f07-016
Gutiérrez-López, M., Salmon, S., and Trigo, D. (2011). Movement response of Collembola to the excreta of two earthworm species: Importance of ammonium content and nitrogen forms. Soil Biol. Biochem. 43, 55–62. doi: 10.1016/j.soilbio.2010.09.010
Haubert, D., Haggblom, M. M., Langel, R., Scheu, S., and Ruess, L. (2006). Trophic shift of stable isotopes and fatty acids in Collembola on bacterial diets. Soil Biol. Biochem. 38, 2004–2007. doi: 10.1016/j.soilbio.2005.11.031
Haubert, D., Haggblom, M. M., Scheu, S., and Ruess, L. (2004). Effects of fungal food quality and starvation on the fatty acid composition of Protaphorura fimata (Collembola). Comp. Biochem. Physiol. B Biochem. Mol. Biol. 138, 41–52. doi: 10.1016/j.cbpc.2004.02.009
Horikawa, M., Nomura, T., Hashimoto, T., and Sakamoto, K. (2008). Elongation and desaturation of fatty acids are critical in growth, lipid metabolism and ontogeny of Caenorhabditis elegans. J. Biochem. 144, 149–158. doi: 10.1093/jb/mvn055
Hu, J. R., Zhou, P. P., Zhu, Y. M., Ren, L., and Yu, L. J. (2011). Isolation of eicosapentaenoic acid-producing fungi from soil based on polymerase chain reaction amplification. Z. Naturforsch. Sect. C J. Biosci. 66, 429–433. doi: 10.1515/znc-2011-7-816
Hulbert, A. J., and Abbott, S. K. (2012). Nutritional ecology of essential fatty acids: an evolutionary perspective. Aust. J. Zool. 59, 369–379. doi: 10.1071/ZO11064
Hutzell, P. A., and Krusberg, L. R. (1982). Fatty acid compositions of Caenorhabditis elegans and C. briggsae. Comp. Biochem. Physiol. B Comp. Biochem. 73, 517–520. doi: 10.1016/0305-0491(82)90068-2
Innis, S. M. (2011). Metabolic programming of long-term outcomes due to fatty acid nutrition in early life. Matern. Child Nutr. 7, 112–123. doi: 10.1111/j.1740-8709.2011.00318.x
Kabeya, N., Fonseca, M. M., Ferrier, D. E. K., Navarro, J. C., Bay, L. K., Francis, D. S., et al. (2018). Genes for de novo biosynthesis of omega-3 polyunsaturated fatty acids are widespread in animals. Sci. Adv. 4:eaar6849. doi: 10.1126/sciadv.aar6849
Koutroumpa, F. A., and Jacquin-Joly, E. (2014). Sex in the night: fatty acid-derived sex pheromones and corresponding membrane pheromone receptors in insects. Biochimie 107, 15–21. doi: 10.1016/j.biochi.2014.07.018
Krusberg, L. R. (1972). Fatty acid composition of Turbatrix aceti and its culture medium. Comp. Biochem. Physiol. 41, 89–98.
Kulas, J., Schmidt, C., Rothe, M., Schunck, W. H., and Menzel, R. (2008). Cytochrome P450-dependent metabolism of eicosapentaenoic acid in the nematode Caenorhabditis elegans. Arch. Biochem. Biophys. 472, 65–75. doi: 10.1016/j.abb.2008.02.002
Losel, D. M. (1988). “Fungal lipids,” in Microbial Lipids, eds C. Ratledge and S. G. Wilkinson (London: Academic Press), 699–806.
Ma, D. K., Li, Z., Lu, A. Y., Sun, F., Chen, S., Rothe, M., et al. (2015). Acyl-CoA dehydrogenase drives heat adaptation by sequestering fatty acids. Cell 161, 1152–1163. doi: 10.1016/j.cell.2015.04.026
Malcicka, M., Ruther, J., and Ellers, J. (2017). De novo synthesis of linoleic acid in multiple Collembola species. J. Chem. Ecol. 43, 911–919. doi: 10.1007/s10886-017-0878-0
Menzel, R., Nehring, R., Simsek, D., and Ruess, L. (2018). Fatty acid 13C isotopologue profiling provides insight into trophic carbon transfer and lipid metabolism of invertebrate consumers. J. Vis.Exp. 134:e57110. doi: 10.3791/57110
Moore, J. C., and Hunt, H. W. (1988). Resource compartmentation and the stability of real ecosystems. Nature 333:261–263.
Müller-Navarra, D. C., Brett, M. T., Liston, A. M., and Goldman, C. R. (2000). A highly unsaturated fatty acid predicts carbon transfer between primary producers and consumers. Nature 403, 74–77. doi: 10.1038/47469
Nilsson, E., and Bengtsson, G. (2004). Death odour changes movement pattern of a Collembola. Oikos 104, 509–517. doi: 10.1111/j.0030-1299.2004.12921.x
Pausch, J., Kramer, S., Scharroba, A., Scheunemann, N., Butenschoen, O., Kandeler, E., et al. (2016). Small but active - pool size does not matter for carbon incorporation in below-ground food webs. Funct. Ecol. 30, 479–489. doi: 10.1111/1365-2435.12512
Petersen, S. O., and Holmstrup, M. (2000). Temperature effects on lipid composition of the earthworms Lumbricus rubellus and Eisenia nordenskioeldi. Soil Biol. Biochem. 32, 1787–1791. doi: 10.1016/S0038-0717(00)00059-6
Pollierer, M. M., Dyckmans, J., Scheu, S., and Haubert, D. (2012). Carbon flux through fungi and bacteria into the forest soil animal food web as indicated by compound-specific 13C fatty acid analysis. Funct. Ecol. 26, 978–990. doi: 10.1111/j.1365-2435.2012.02005.x
Pond, C. M. (1981). “Storage,” in Physiological Ecology: An Evolutionary Approach to Resource use, eds. C.R. Townsend & P. Calow. (London: Blackwell), 190–219.
Pond, D. W., Leakey, R. J. G., and Fallick, A. E. (2006). Monitoring microbial predator-prey interactions: an experimental study using fatty acid biomarker and compound-specific stable isotope techniques. J. Plankton Res. 28, 419–427. doi: 10.1093/plankt/fbi130
Rothstein, M., and Götz, P. (1968). Biosynthesis of fatty acids in the free-living nematode, Turbatrix aceti. Arch. Biochem. Biophys. 126, 131–140.
Ruess, L., and Chamberlain, P. M. (2010). The fat that matters: Soil food web analysis using fatty acids and their carbon stable isotope signature. Soil Biol. Biochem. 42, 1898–1910. doi: 10.1016/j.soilbio.2010.07.020
Ruess, L., Häggblom, M. M., Langel, R., and Scheu, S. (2004). Nitrogen isotope ratios and fatty acid composition as indicators of animal diets in belowground systems. Oecologia 139, 336–346. doi: 10.1007/s00442-004-1514-6
Sampedro, L., Jeannotte, R., and Whalen, J. K. (2006). Trophic transfer of fatty acids from gut microbiota to the earthworm Lumbricus terrestris L. Soil Biol. Biochem. 38, 2188–2198. doi: 10.1016/j.soilbio.2006.02.001
Sargent, J. R., Bell, M. V., Bell, J. G., Henderson, R. J., and Tocher, D. R. (1995). “Origins and functions of n-3 polyunsaturated fatty acids in marine organisms,” in Phospholipids: Characterization, metabolism and novel biological applications, eds. G. Cevc & F. Paltauf. (Urbana, IL: American Oil Chemists Society), 248–259.
Scheu, S., Ruess, L., and Bonkowski, M. (2005). “Interactions between microorganisms and soil micro-and mesofauna,” in Microorganisms in soils: roles in genesis and functions. Soil Biology Series Vol. 3, eds. F. Buscot & A. Varma. (Berlin, Heidelberg: Springer), 253–275.
Scheu, S., and Simmerling, F. (2004). Growth and reproduction of fungal feeding Collembola as affected by fungal species, melanin and mixed diets. Oecologia 139, 347–353. doi: 10.1007/s00442-004-1513-7
Schlechtriem, C., Ricci, M., Focken, U., and Becker, K. (2004). The suitability of the free-living nematode Panagrellus redivivus as live food for first-feeding fish larvae. J. Appl. Ichthyol. 20, 161–168. doi: 10.1111/j.1439-0426.2004.00542.x
Selvan, S., Gaugler, R., and Grewal, P. S. (1993a). Water content and fatty acid composition of infective juvenile entomopathogenic nematodes during storage. J. Parasitol. 79, 510–516.
Selvan, S., Gaugler, R., and Lewis, E. E. (1993b). Biochemical energy reserves of entomopathogenic nematodes. J. Parasitol. 79, 167–172. doi: 10.2307/3283503
Simopoulos, A. P. (2000). Human requirement for n-3 polyunsaturated fatty acids. Poult. Sci. 79, 961–970. doi: 10.1093/ps/79.7.961
Snider, R. J., Shaddy, J. H., and Butcher, J. W. (1969). Culture techniques for rearing soil arthropods. Mich. Entomol. 1, 357–362.
Stanley, D. (2006). Prostaglandins and other eicosanoids in insects: biological significance. Annu. Rev. Entomol. 51, 25–44. doi: 10.1146/annurev.ento.51.110104.151021
Stanley-Samuelson, D. W., Jurenka, R. A., Cripps, C., Blomquist, G. J., and de Renobales, M. (1988). Fatty acids in insects: composition, metabolism, and biological significance. Arch. Insect Biochem. Physiol. 9, 1–33.
Stukey, J. E., Mcdonough, V. M., and Martin, C. E. (1989). Isolation and characterization of OLE1, a gene affecting fatty acid desaturation from Saccharomyces cerevisiae. J. Biol. Chem. 264, 16537–16544.
Sulston, J. E., and Hodgkin, J. (1988). “Methods,” in The Nematode Caenorhabditis elegans, ed. W.B. Wood (Cold Spring Harbor, NY: CSHL Press), 587–606.
Thimm, T., Hoffmann, A., Borkott, H., Charles Munch, J., and Tebbe, C. C. (1998). The gut of the soil microarthropod Folsomia candida (Collembola) is a frequently changeable but selective habitat and a vector for microorganisms. Appl. Environ. Microbiol. 64, 2660–2669.
Tillman, J. A., Seybold, S. J., Jurenka, R. A., and Blomquist, G. J. (1999). Insect pheromones - An overview of biosynthesis and endocrine regulation. Insect Biochem. Mol. Biol. 29, 481–514.
Twining, C. W., Brenna, J. T., Hairston, N. G., and Flecker, A. S. (2016). Highly unsaturated fatty acids in nature: what we know and what we need to learn. Oikos 125, 749–760. doi: 10.1111/oik.02910
Vrablik, T. L., and Watts, J. L. (2013). Polyunsaturated fatty acid derived signaling in reproduction and development: insights from Caenorhabditis elegans and Drosophila melanogaster. Mol. Reprod. Dev. 80, 244–259. doi: 10.1002/mrd.22167
Wall, R., Ross, R. P., Fitzgerald, G. F., and Stanton, C. (2010). Fatty acids from fish: the anti-inflammatory potential of long-chain omega-3 fatty acids. Nutr. Rev. 68, 280–289. doi: 10.1111/j.1753-4887.2010.00287.x
Watts, J. L., and Browse, J. (2002). Genetic dissection of polyunsaturated fatty acid synthesis in Caenorhabditis elegans. Proc. Natl. Acad. Sci. U.S.A. 99, 5854–5859. doi: 10.1073/pnas.092064799
Weinert, J., Blomquist, G. J., and Borgeson, C. E. (1993). De novo biosynthesis of linoleic acid in two non-insect invertebrates: the land slug and the garden snail. Experientia 49, 919–921.
Yeates, G. W. (2010). “Nematodes in ecological webs,” in Encyclopedia of Life Sciences (New York, NY: John Wiley & Sons, Ltd), 1–11. doi: 10.1002/9780470015902.a0021913
Yeates, G. W., Bongers, T., Degoede, R. G. M., Freckman, D. W., and Georgieva, S. S. (1993). Feeding-habits in soil nematode families and genera - an outline for soil ecologists. J. Nematol. 25, 315–331.
Yeates, G. W., Hawke, M. F., and Rijkse, W. C. (2000). Changes in soil fauna and soil conditions under Pinus radiata agroforestry regimes during a 25-year tree rotation. Biol. Fertil. Soils 31, 391–406. doi: 10.1007/s003749900186
Zelles, L. (1999). Fatty acid patterns of phospholipids and lipopolysaccharides in the characterisation of microbial communities in soil: a review. Biol. Fertil. Soils 29, 111–129. doi: 10.1007/s003740050533
Zhou, X. R., Horne, I., Damcevski, K., Haritos, V., Green, A., and Singh, S. (2008). Isolation and functional characterization of two independently-evolved fatty acid delta 12-desaturase genes from insects. Insect Mol. Biol. 17, 667–676. doi: 10.1111/j.1365-2583.2008.00841.x
Keywords: omega-3 PUFA, dietary routing, soil food web, nematodes, Collembola, Caenorhabditis elegans
Citation: Menzel R, Geweiler D, Sass A, Simsek D and Ruess L (2018) Nematodes as Important Source for Omega-3 Long-Chain Fatty Acids in the Soil Food Web and the Impact in Nutrition for Higher Trophic Levels. Front. Ecol. Evol. 6:96. doi: 10.3389/fevo.2018.00096
Received: 27 February 2018; Accepted: 20 June 2018;
Published: 10 July 2018.
Edited by:
Jennifer Watts, Washington State University, United StatesReviewed by:
Martin Holmstrup, Aarhus University, DenmarkCopyright © 2018 Menzel, Geweiler, Sass, Simsek and Ruess. This is an open-access article distributed under the terms of the Creative Commons Attribution License (CC BY). The use, distribution or reproduction in other forums is permitted, provided the original author(s) and the copyright owner(s) are credited and that the original publication in this journal is cited, in accordance with accepted academic practice. No use, distribution or reproduction is permitted which does not comply with these terms.
*Correspondence: Ralph Menzel, cmFscGgubWVuemVsQGJpb2xvZ2llLmh1LWJlcmxpbi5kZQ==
Disclaimer: All claims expressed in this article are solely those of the authors and do not necessarily represent those of their affiliated organizations, or those of the publisher, the editors and the reviewers. Any product that may be evaluated in this article or claim that may be made by its manufacturer is not guaranteed or endorsed by the publisher.
Research integrity at Frontiers
Learn more about the work of our research integrity team to safeguard the quality of each article we publish.