- 1Physical Science and Engineering Division, King Abdullah University of Science and Technology (KAUST), Thuwal, Saudi Arabia
- 2Center of Integrative Petroleum Research (CIPR), College of Petroleum Engineering and Geoscience, King Fahd University of Petroleum and Minerals, Dhahran, Saudi Arabia
- 3Faculty of Science, Mansoura University, Mansoura, Egypt
Introduction: Underground hydrogen (H2) storage is a prominent technique to enable a large-scale H2-based economy as part of the global energy mix for net-zero carbon emission. Recently, basalts have gained interest as potential caprocks for subsurface H2 storage due to their low permeability, vast extension, and potential volumetric capacity induced by structural entrapment of the buoyant H2. Wettability represents a fundamental parameter which controls the capillary-entrapment of stored gases in porous media.
Methods: The present study evaluates the wettability of basalt/H2/brine system of two basalt samples from Harrat Uwayrid, a Cenozoic volcanic field, in Saudi Arabia. The H2/basalt contact angle was measured using a relevant reservoir brine (10% NaCl) under storage conditions of 323K temperature and pressure ranges from 3 to 28 MPa using the modified sessile drop method. The surface roughness of the basaltic rocks was determined to ensure accurate results.
Results: The investigated Saudi basalt samples are water-wet, thereby they did not achieve a 100% hydrogen wetting phase even at 28 MPa pressure. The measured contact angles slightly decrease as pressure increases, thereby pressure did not significantly influences the height of the H2 column.
Discussion: We interpret this trend to the slight increase in H2 density with increasing pressure as well as to the olivine-rich mineralogical composition of the Saudi basalt. Thus, from the wettability aspects, Saudi basalt has the potential to store a large volume of H2 (>1,400 m height) and maintain its excellent storage capacity even in deep, high-pressure regimes. This study demonstrates that the basalt rock texture (pore throat radii) and mineralogy control their capacity for subsurface H2 storage.
1 Introduction
Global efforts are underway to reduce harmful anthropogenic emissions of greenhouse gases such as carbon dioxide (CO2) by adopting strict environmental regulations and transitioning toward a diversified energy mix instead of complete reliance on fossil fuels (IEA, 2018; Hashemi et al., 2021; Alanazi et al., 2023a). Hydrogen (H2) emerges as a promising clean fuel to support decarbonization by converting energy production from fossil fuels into a more efficient and environmental-friendly form, thus supporting the transition into renewable and sustainable resources (McCollom and Bach, 2009; Prinzhofer et al., 2018; Pan et al., 2021; Leila et al., 2022; Alanazi et al., 2023b). However, a wide-scale implementation of an H2-based economy requires a medium of giga-to tera-scale storage capacity, which is theoretically associated with specific geological contexts such as saline aquifers, depleted oil and gas reservoirs, and salt caverns (e.g., Tarkowski, 2019; Heinemann et al., 2021). Successful industrial-scale H2 geological storage has been implemented in salt caverns due to their impervious characteristics and perfect seal integrity (Michalski et al., 2017; Al-Mukainah et al., 2022).
Sedimentary formations with existing infrastructures such as depleted reservoirs have attracted attention for subsurface storage due to their efficient pore system, storage, and sealing capacities (Pfeiffer et al., 2017; Yekta et al., 2018; Tarkowski, 2019; Alanazi et al., 2022c). Furthermore, volcanic basaltic rocks have recently been recognized as an unconventional storage medium and a potential rival to sedimentary formations (Iglauer et al., 2020). Basaltic rocks often exist vastly on the continents in the form of extensive dykes and sills. They also cover approximately two-thirds of the oceanic crust (Gislason and Oelkers, 2014; Matter et al., 2016). Therefore, various efforts started to investigate their suitability for CO2 storage in terms of pore system, permeability, trapping mechanisms, and sealing efficiency (Gislason et al., 2010; Iglauer et al., 2020). However, the efficacy of basaltic rocks for H2 storage still need further investigation.
The primary trapping mechanisms are structural/residual trapping, dissolution, and mineralization trapping (Bui et al., 2018). The latter has shown a CO2 mineralization in basalt within time scales superior to those predicted for clastic sedimentary rocks (White et al., 2020). However, for H2 subsurface storage, the residual and structural trapping mechanisms are more pronounced. In contrast to the hydrogen storage being cyclic in nature, it might be worth emphasizing the permanent nature of CO2 storage for contrast (Ma et al., 2019; Alanazi et al., 2022a; Alanazi et al., 2022c; Ali et al., 2022b; Alanazi et al., 2023c). In addition, H2 storage requires a cushion gas (e.g., CO2, N2, and CH4) to remain in place and maintain the hydrostatic pressure of the reservoir (Kanaani et al., 2022).
The wettability of basalt/gas/brine is a crucial parameter controlling the trapping efficiency of the buoyant gas beneath a caprock (Hosseini et al., 2022c). The flow behaviour and interfacial characteristics of H2-brine in the subsurface differ from CO2 and CH4 (Chow et al., 2018). The interfacial tension between rock and gas plays a significant role in gas storage within the pore system. Therefore, the gas-rock wettability is a critical parameter in understanding the rock’s capability for entrapment. Wettability controls the gas flow and migration within a rock pore structure, affecting storage capacity and sealing efficiency (Blunt, 2016; Ali et al., 2022a). While basalt/CO2/brine wettability studies are available with limited testing conditions (Iglauer et al., 2020; Abdulelah et al., 2021; Al-Yaseri et al., 2021b), there is a noticeable lack of studies on basalt/H2 wettability limited to two studies; one theoretical and another experimental (Table 1). Al-yaseri et al. (2021a) and Al-Yaseri et al. (2021b) developed empirical correlations using contact angle measurements and densities of helium (He), carbon dioxide (CO2), nitrogen (N2), methane (CH4), and argon (Ar) to predict the three-phase contact angles of basalt/H2/brine at the same conditions (Al-Yaseri and Jha, 2021). While, Hosseini et al. (2022a) reported experimental measurements of contact angles for basalt using the tilted plate method at two temperatures (308 and 343K) and varying pressure from 5 to 20 MPa. Previous studies that tackled the basalt/H2 wettability measurements outlined a weak to intermediate water-wet behaviour for the basalt at elevated levels of pressure and temperature. This behaviour would be beneficial for storing thick hydrogen columns in the subsurface.
The present study provides an experimental investigation of the H2/brine wettability of two basalt samples collected from the Cenozoic Harrat Uwayridh volcanic field in the northwest of Saudi Arabia (Figure 1). The basalt wettability was measured at various geological storage conditions to explore their potential for subsurface H2 storage. In theory, thick basaltic rocks can provide good sealing properties for H2 storage in the underlying clastic reservoirs (Hosseini et al., 2022a). Basaltic rocks’ capillary sealing efficiency and storage capacity are significant characteristics to be tested. Accordingly, the sealing efficiency represented by capillary entry pressure and maximum static H2 column should be calculated to assess the storage feasibility using basalt rocks’ typical pore sizes (Duncan and Al-Amri, 2013; Espinoza and Santamarina, 2017; Alanazi et al., 2022a; Alanazi et al., 2022b; Ali et al., 2022b; Alanazi et al., 2022c).
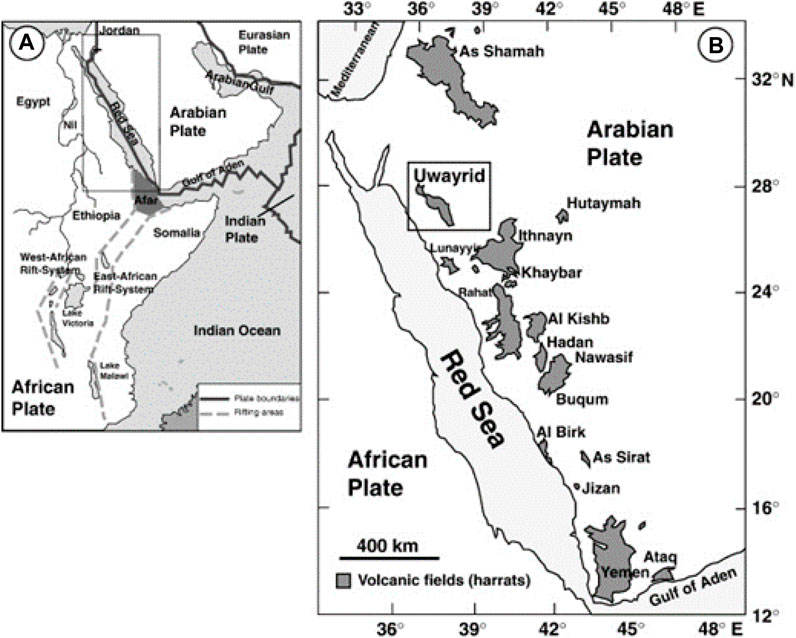
FIGURE 1. Harrat volcanic fields on the Arabian peninsula shown the tectonic map of the Arabian shield (A) and (B) a map of Harrat Uwayridh and neighbouring plates, adopted from Altherr et al. (2019).
2 Materials and methods
2.1 Basaltic samples
Two Saudi basaltic samples were collected from Harrat Uwayrid, a Cenozoic volcanic field in the Medina region, NW Saudi Arabia, Figure 1 (Duncan and Al-Amri, 2013). The Harrat Uwayrid comprises an elongate NW-SE oriented volcanic field of a strongly eroded basaltic plateau extending over approximately 230 km2. The Harrat Uwayrid basalt overlies above the Cambrian continental sandstones of the Siq Formation (Coleman et al., 1983; Altherr et al., 2019). The age of Harrat Uwayrid basalts ranges from Miocene to Quaternary, contemporaneous with the several episodes of volcanic activities in Arabia (Kaliwoda et al., 2007). The Miocene basalt is strongly eroded and consists mainly of alkali olivine basalt, whereas the younger Quaternary basalt contains mantle xenoliths and megacrysts (Altherr et al., 2019). The samples were selected from two locations north and south of the elongated volcanic field. The investigation aims to study the efficiency of Harrat Uwayrid basaltic rocks as a seal and evaluate potential hydrogen storage in the underlying Cambrian sandstone formation for a practical period of time.
2.2 Sample characterization
2.2.1 X-ray diffraction and scanning electron microscopy
Whole-rock X-ray diffraction (XRD) analysis was conducted on the Saudi basalt samples. The samples were powdered and measured for XRD using a Bruker-D8 Advance diffractometer (Bruker AXS GmbH, Karlsruhe, Germany). The measurement comprised a step scan in the Bragg-Brentano geometry having a CuKα radiation of 40 kV and 40 mA to measure XRD peaks. The XRD peak interpretation was performed using Bruker-EVA software and peak comparison with the International Centre for Diffraction Data (ICCD) standard database.
The SEM analysis was performed on the two Harrat Uwayrid basalt samples using JEOL’s JSM-7001F Schottky SEM. Polished sections from the basalt samples were first mounted on aluminium stubs. The mounted sections were then coated with gold before the SEM analysis.
2.2.2 Fourier transform infrared spectroscopy (FTIR)
The spectra of Fourier Transform Infrared Spectroscopy (FTIR) are sensitive to variations in mineralogy and crystal structure, and therefore they are qualitatively utilized to identify the mineral phases in the samples based on their crystal structure. FTIR determines the absorption wavelengths related to the molecular excitation states of covalent bonds in the composition of the samples (Mbonyiryivuze et al., 2015). FTIR spectra for the Saudi basalt samples were obtained utilizing a Bruker TENSOR-27 FTIR spectrometer containing a source of infrared waves, a beam splitter, and equipped with a susceptible DigiTectTMdetector system. Approximately 0.5 mg of the powdered samples were mounted in metal disks, then dispersed in 200 mg of KBr before heating at 120 °C to release the absorbed water. The samples were scanned in the wavenumber region of 4,000–400 cm−1 to obtain the FITR spectra.
2.2.3 Total organic carbon (TOC) analysis
The total organic carbon (TOC) content in the two Saudi basalt samples was measured using Rock-Eval pyrolysis 7S (©Vinci Technologies). The apparatus is equipped with an oven allowing a high-temperature pyrolysis and air oxidation of approximately 10 mg of the powdered basalt samples. The oven temperature rates were adjusted from 0.1°C to 50°C/mn, with a 0.1 °C step.
2.2.4 Surface roughness measurement
The surface roughness of a sample can significantly affect the wettability and, in turn, the contact angles of the H2/brine/basalt system (AlRatrout et al., 2018; Mehmani et al., 2019). It has been reported that the irregularity of a solid surface or surface roughness is directly linked to the hydrophobicity status of that solid caused by fluids entrapment in the surface’s depressions (Morrow, 1975; Yen, 2015; Li et al., 2022). Thus, the surface roughness of Saudi basalt samples was measured using a surface roughness analyzer (KRÜSS GmbH, Germany). The KRÜSS GmbH uses the confocal microscopy technique to build a spatial schematic of the surface topography of high resolution using a rotating disk. The roughness of the selected vertical readings is provided based on the best root mean error of the multiple locations of the sample’s surface.
2.3 Experimental measurement of contact angle
The contact angles were measured using the modified sessile drop method utilizing a KRÜSS fluid drop analyzer (DSA100 model) and a high-pressure and high-temperature (HPHT) optical cell (Figure 2). Pure H2 gas (99.99 mol%) and a synthetic reservoir brine of 10 wt%NaCl were used for the contact angle measurements at testing conditions. First, the basalt sample was cleaned with distilled water and dichloromethane, cut into 1 cm × 1 cm × 0.5 cm dimensions. Further cleaning by purging with nitrogen was conducted prior to emplacement of the samples on a horizontal plate inside a high-temperature and pressure (HTHP) cell. Then, the H2 is injected and pressurized using an ISCO pump (500 D) of 0.001 MPa precision. The temperature is increased using an electrical heater to reach the desired temperature (323K). Afterwards, a brine droplet of 5 μL (±2 μL) is manually introduced through a needle dispenser, controlled by another ISCO pump, and released on the substrate surface. Finally, the contact angle is measured at adiabatic conditions in a continuous increment measurement where the pressure increases gradually from 3 MPa to 28 MPa, and the contact angle is measured at each pressure step. The purpose of such modified measurement from the conventional sessile drop method is to measure the hydrogen contact angle at high pressure levels which may occur in the subsurface where a continuous pressure is applied by surrounding rocks and formation fluids when H2 is injected into the geological formation for subsurface storage. Al-Mukainah et al. (2022) have recently adopted the same modified sessile drop method (applied in this work) to measure H2/shale/brine contact angles and evaluate potential H2 storage in shale formations (Al-Mukainah et al., 2022). Images were acquired using a high-resolution camera to capture the drop behaviour during the experiment. The contact angle is then analyzed using KRÜSS DSA software. The contact angle measurement was repeated three times for better accuracy. The average standard deviation for the contact angle from the two samples was approximately ±3°. Before the contact angle measurement, the surface roughness of the basalt samples was determined using the Surface Roughness Analyzer (KRÜSS GmbH) (Al-Yaseri et al., 2021c).
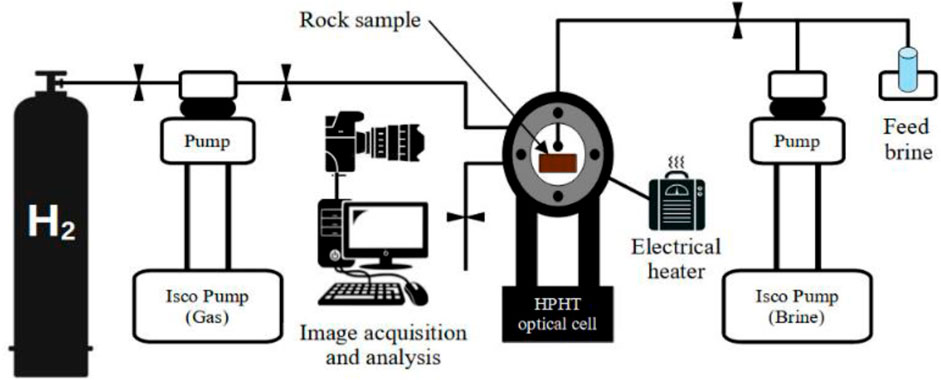
FIGURE 2. Schematic experimental design for the contact angle measurements of H2/basalt/brine systems.
3 Results and discussion
3.1 Characterization of Harrat Uwayrid basalt
The measured TOC values for the analyzed basalt samples (1 and 2) were approximately 0.05 wt% and 0.03 wt%, respectively, measured by Rock-Eval pyrolysis. The XRD results provide reveal a semi-quantitative mineralogy analysis (Figure 3). The analyzed samples were collected from two different locations (south and north) to evaluate any changes in the mineral composition that could potentially influence H2/basalt/brine wettability. The XRD analysis demonstrates a similarity in the primary minerals between the two samples, dominated by anorthite and olivine of about 44.3% and 57.1% for anorthite, 14.7%, and 24.4% for olivine in the Saudi basalt 1 and 2, respectively. Clinopyroxene (diopside) and nepheline were also found only in Saudi basalt 1 (24.8% and 16.3%, respectively), while Saudi basalt 2 contains magnesioferrite (∼17.6%) and traces of albite and vermiculite that were missing in Saudi basalt 1.
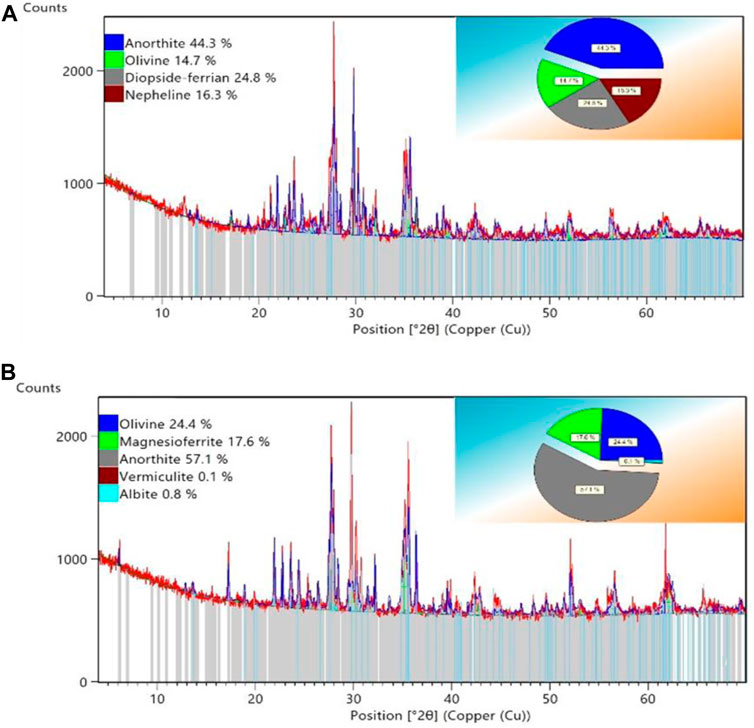
FIGURE 3. Interpreted XRD patterns illustrating the mineralogical composition of the studied Saudi basalt 1 (A) and 2 (B).
A mineralogical comparison between the investigated Saudi basalts and other basaltic rocks tested for underground gas storage (Table 2) reveals significant differences, which may influence the wettability measurement and storage efficiency of H2 beneath the basalts. Relative to other basalt samples (Iranian and Icelandic basalts), Saudi basalts are enriched in olivine relative to plagioclase and alteration products (e.g., clays). Such variation in mineralogy typifies a differential alteration (Harnois, 1988; Weltje et al., 1998; Dessert et al., 2003; Leila et al., 2018; Leila et al., 2021). For example, olivine minerals are more susceptible to chemical weathering and alteration into other phases such as serpentine; thus, enrichment of olivine phases would infer into fresh and un-altered samples. Therefore, the Saudi basalts are fresh samples, whereas other basalt samples were subjected to more severe chemical alteration. Such variation in chemical alteration may impact the wettability measurements and hence the storage potential. Notably, nano-capillary pores are more common in clays and these nanopores may become saturated with hydrogen, and hence reduce the storage capability of the basaltic rock. Additionally, the occurrence of specific clay phases that are susceptible to hydro-swelling (e.g., Montmorillonite) would decrease pore throat size and pore system connectivity (Aksu et al., 2015; Jiu et al., 2021). Thereby, variation in mineralogical composition would significantly impact the capillary behaviors and sealing capacity of the naturally-impervious rocks.
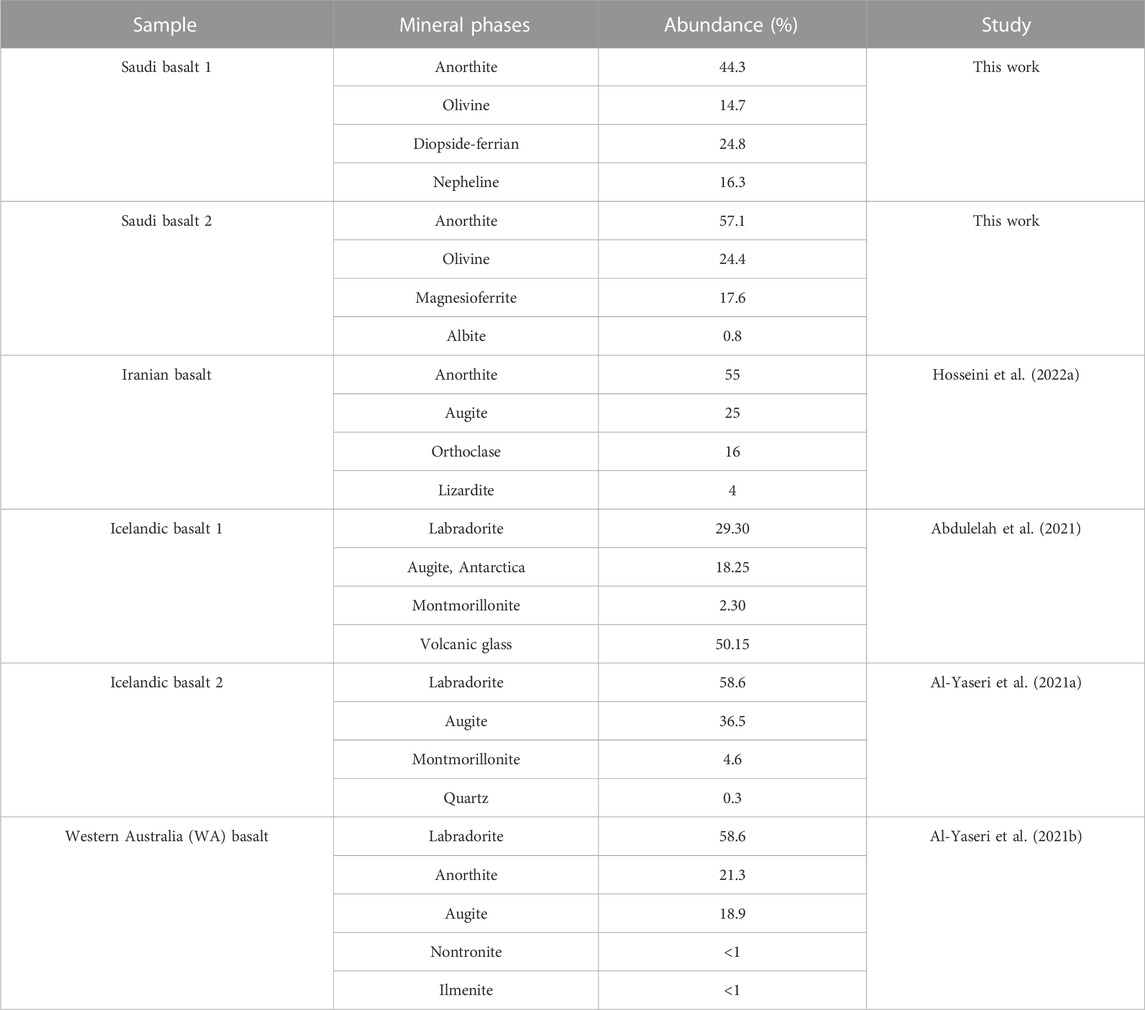
TABLE 2. A comparison of XRD quantitative analysis results of the bulk mineralogy for the Saudi basalt samples compared to the mineral compositions of the previously analyzed basalt samples for wettability and underground gas storage.
The FTIR measurement indicates the dominant chemical functional groups on a rock surface, significantly contributing to the wettability status (Madhurima et al., 2011; Cheng et al., 2014; Pillai et al., 2018). The FTIR analysis of the Saudi basalt samples demonstrated that SiO2 is the primary chemical group on the samples’ surface (Figure 4). Visible wave numbers in the range of 1,000 and 1,200 cm−1 correspond to the Si-O-Si bond (OH and Choi, 2010; Waman et al., 2011; Liu et al., 2019). The interpreted Si-O-Si bond is attributed to the presence of silica and silicate minerals in the studied basalt samples. The more pronounced Si-O-Si bond in Saudi basalt 1 is most likely related to the occurrence of nepheline which is absent in basalt 2. Weak absorption at 800 cm−1 corresponds to Si-H2/(Si-H2)n band. This band is more significant in the Saudi basalt 2 sample typifying a more hydrophilic characteristics relative to basalt 1 sample (Liu et al., 2019). FTIR is also corroborated by XRD and XRF which confirmed a silica-rich samples. According to XRF data, basalts 1 and 2 contain 36.16% and 37.50% SiO2, respectively.
The SEM analysis focuses on the basalt samples’ surface morphology and micro-scale characteristics, such as the fracture and matrix porosity, which can significantly influence the wettability measurement (Rucker et al., 2019). Micro, irregular, stylolite-like fractures were found with more intensity in Saudi basalt 1 (Figures 5A, B) compared to the Saudi basalt 2 sample (Figures 5C, D). Both samples showed low matrix porosity in the micro- and nano-scales. However, matrix porosity is relatively more significant in Saudi basalt 2 sample (Figure 5D). Notably, the fracture and matrix pores are scattered and non-connected, suggesting that the studied Saudi basalts are impervious with nano-scale pore throats. The surface roughness analyzer indicated a root-mean-square (RMS) from different vertical locations of approximately 29.74 and 25.14 µm surface roughness for Saudi basalt 1 and Saudi basalt 2, respectively (Figure 6). The RMS roughness is a measure of the standard deviation in the surface heights values, thus reflecting the extent of surface irregularities (Gadelmawla et al., 2002). The surface topography of the samples induces a paramount impact on the rock-fluid contact angle. Irregular, rough surfaces with high RMS values often enhance the wetting behavior of the sample (Johnson and Dettre, 1964). Additionally, irregular surfaces usually show water-wet behavior. Nevertheless, the influence of the surface roughness becomes insignificant below 1,000 µm (Al-Yaseri et al., 2016). Thus, it does not impact the wetting characteristics of storage formation rocks (Al-Mukainah et al., 2022).
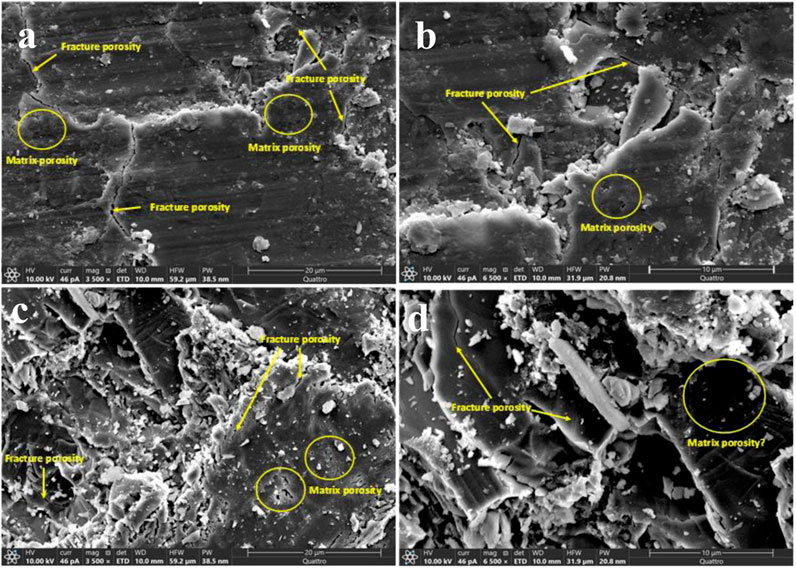
FIGURE 5. SEM microphotographs illustrating the surface morphology and textural characteristics of the studied Saudi basalt 1 (A,B) and 2 (C,D).
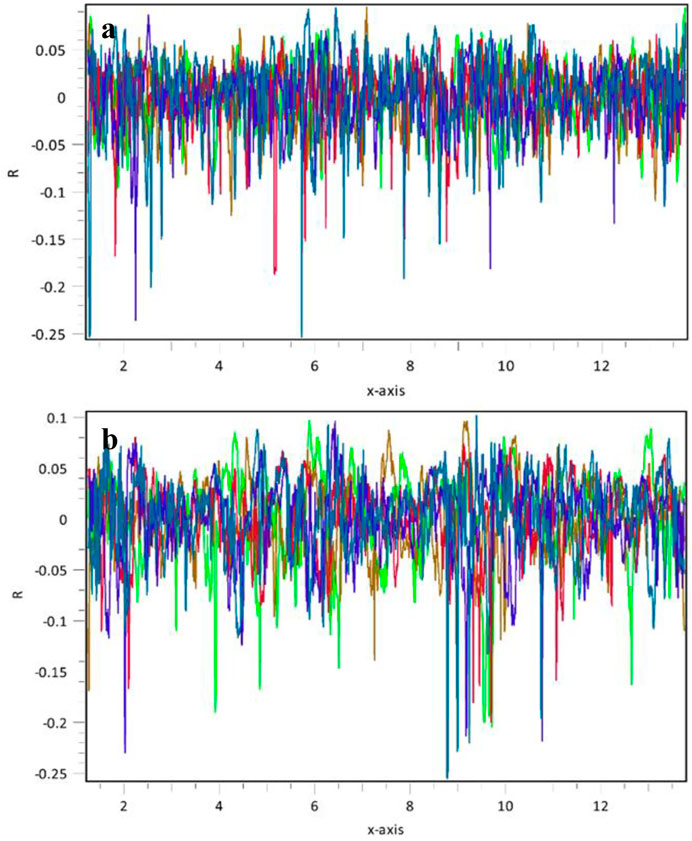
FIGURE 6. Surface roughness of Saudi basalt samples tested over 25 by 40 µm regions of each sample—(A) Saudi basalt 1 of 29.74 µm mean roughness and (B) Saudi basalt 2 of 25.14 µm mean roughness.
3.2 Effect of pressure on H2/basalt/brine wettability
The static H2/brine/basalt contact angles of the two samples were measured at different pressures up to 28 MPa and a temperature of 323K (Figure 7). The Saudi basalt 2 displayed slightly higher contact angles than Saudi basalt 1. The H2 wettability of Saudi basalt 2 demonstrated a more hydrophilic behavior than Saudi basalt 1, mainly attributed to the Si- H2 bond FTIR peak, which is higher in Saudi basalt 2 than Saudi basalt 1. However, the variation in contact angle measurements could be attributed only to surface roughness differences (AlRatrout et al., 2018; Mehmani et al., 2019). Nevertheless, both samples displayed a strong to intermediate water-wet behaviour and demonstrated a slight decrease in pressure, which is linked to the decline of interfacial between the brine and H2 gas (Figure 8). Images of drop profiles are shown in Figures 9, 10 for the different pressure steps confirming the observation of the contact angle measurements. These profiles demonstrate intermediate to strongly water-wet behaviour.
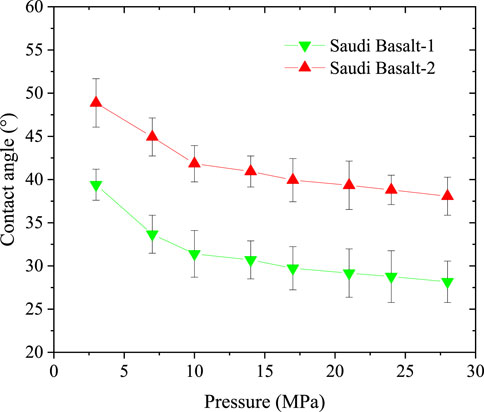
FIGURE 7. Static contact angles of H2/brine systems on Saudi basalt samples at different pressures and constant temperature of 323K. The uncertainty bars in the plots range from 2.1° to 3.3° (with the relative standard uncertainties of 1K for temperature and 0.1 psi for pressure).
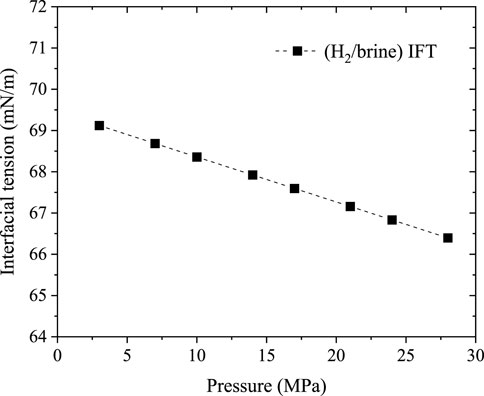
FIGURE 8. Hydrogen/brine interfacial tension at different pressures and constant temperature of 323K, extrapolated from the data in Chow et al. (2018) with ±1 mN/m uncertainty.
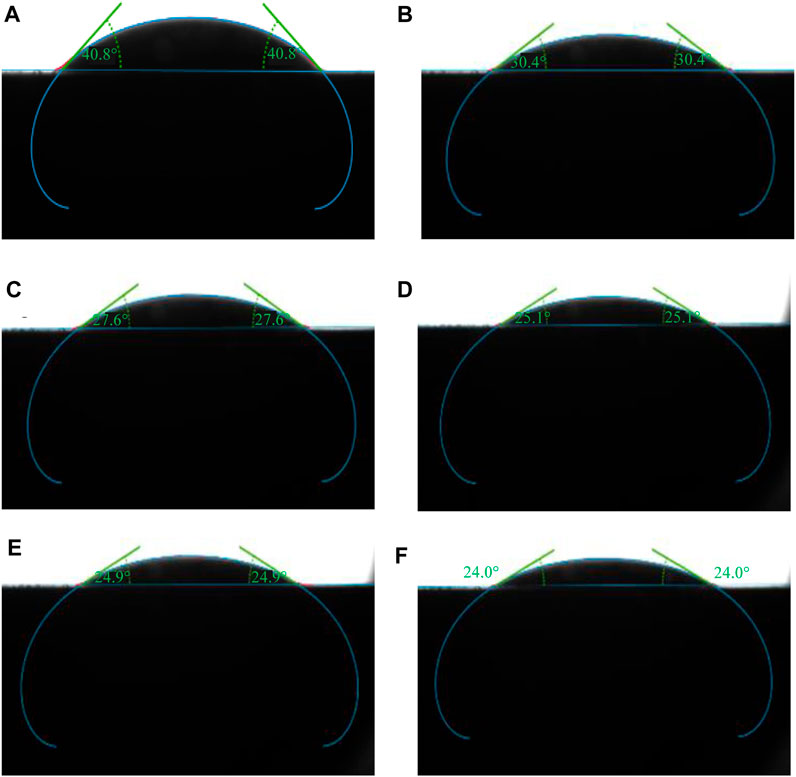
FIGURE 9. Sessile drop of brine on Saudi basalt-1 surrounded by hydrogen, measured at a constant temperature of 323K and different pressure steps (A) 3 MPa, (B) 7 MPa, (C) 14 MPa, (D) 21 MPa, (E) 24 MPa, and (F) 28 MPa.
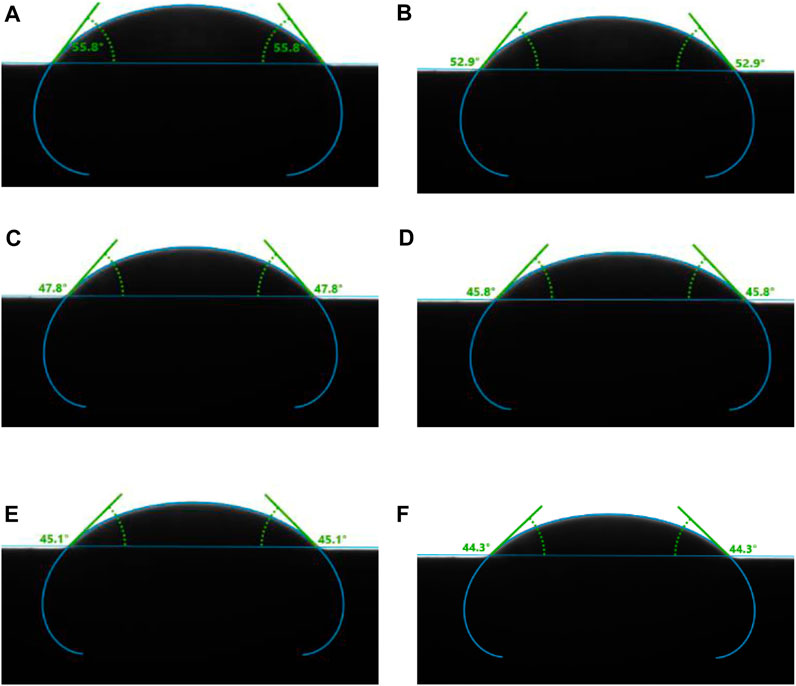
FIGURE 10. Sessile drop of brine on Saudi basalt-2 surrounded by hydrogen, measured at a constant temperature of 323K and varying pressures—(A) 3 MPa, (B) 7 MPa, (C) 14 MPa, (D) 21 MPa, (E) 24 MPa, and (F) 28 MPa.
Overall, the contact angles for both basalt samples displayed an initial steep drop as pressure increased from 2.5 MPa to 10 MPa, which started to level up at 10 MPa pressure. The observed decrease in the contact angles with pressure differs from some of the gas/basalt/brine measurements, for example, (Iglauer et al., 2020; Al-Yaseri et al., 2021a; Hosseini et al., 2022b), where the contact angle increase with pressure. In this work, the modified sessile drop is applied, where the contact angle is measured for each pressure step in a continuous pressure increment approach. Moreover, the decrease in contact angle with pressure for the H2/basalt/brine system is related to the decline of H2-brine interfacial tension (IFT) with increasing pressure (Figure 8) and the very low density of H2 compared to CO2, where the cohesive forces between gas molecules and rock matrix increase at higher pressures (Yekeen et al., 2021; Al-Mukainah et al., 2022).
3.3 H2/brine wettability of Saudi basalt compared to Iranian basalt
The present work measured the static contact angle of H2/basalt/brine. While the only available work of H2/basalt/brine wettability in the literature by Hosseini et al. (2022b) was conducted using the tilted plate method, first introduced by Lander et al. (1993), and provides dynamic contact angles. The tilted plate method theoretically attempts to represent the imbibition (wetting phase displacing non-wetting) and drainage (non-wetting phase displacing wetting) processes by correlating them to tail contact angles of a brine drop on a tilted rock surface, the receding contact angle (
In the literature, there is extensive experimental work applying the tilted plate method to investigate underground H2 storage using different rock types, where the dynamic contact angles are related to the intrinsic contact angle or the equilibrium contact angle (
Where
The above correlation was used to calculate
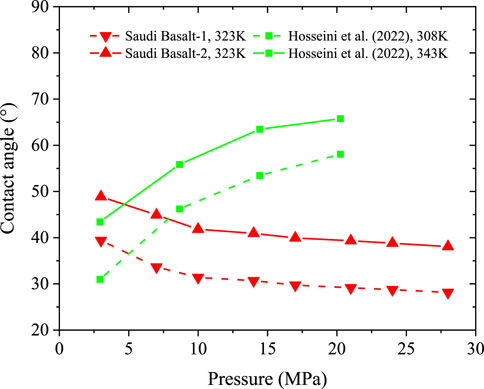
FIGURE 11. Static contact angles of H2/brine systems of Saudi basalt samples at 323K, compared to the calculated equivalent contact angles on Iranian basalts at 308K and 343K (Hosseini et al., 2022a).
The modified sessile drop used in this work depends on maintaining an isolated gas environment and measuring the change in drop contact angles at each pressure point via a subsequent increase of the pressure inside the high-pressure, high-temperature cell. In contrast, the titled-plate method measures the dynamic contact angles in a stepwise procedure involving using multiple rock samples and depressurizing the cell each step to load the new sample. Moreover, the mineralogy comparison based on XRD analysis listed in Table 2 demonstrates that the Saudi basalts have different mineral compositions than the Iranian basalt. The Iranian basalt composition contains more lizardite and plagioclase; thus, it can exhibit a wettability behaviour similar to clay-rich rocks (Abramov et al., 2019; Al-Yaseri et al., 2021c).
3.4 Capillary sealing efficiency and H2 static column height
The sealing efficiency of a caprock is evaluated by studying capillary characteristics at the interface between the formation brine in the caprock and the injected gas. A non-wetting phase (e.g., CO2 and H2) cannot invade the rock until the pressure drop exceeds a certain threshold, which is known as the capillary entry pressure
where
The sealing capacity and storage efficiency of the studied basalt samples at 323K is obtained by calculating the static height of the H2 column that can be safely trapped beneath the basaltic rocks using Eq. 5 (Dake, 1978):
where
The capillary entry pressure (
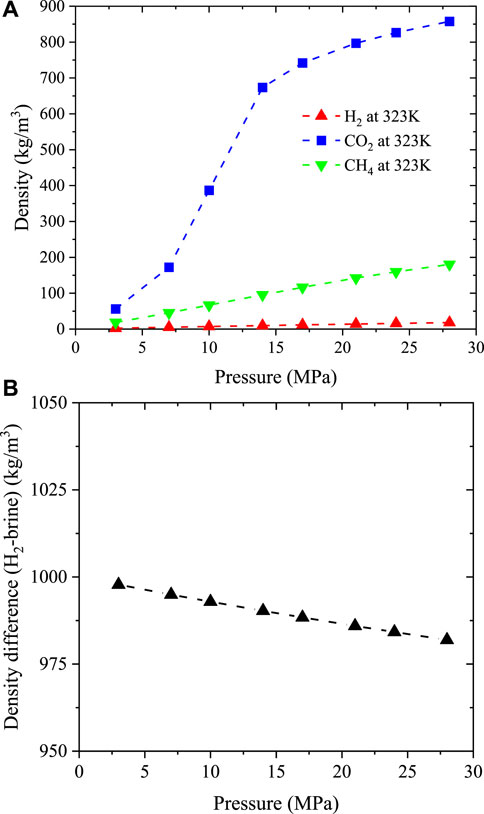
FIGURE 12. Density variation over pressure (A) and the density difference between 10%NaCl brine and hydrogen at varying pressure and constant temperature of 323K (B). Note: the data were extracted from NIST database (Lemmon et al., 2018).
The calculations in this study using H2/basalt/brine contact angles demonstrate an insignificant influence of pressure on
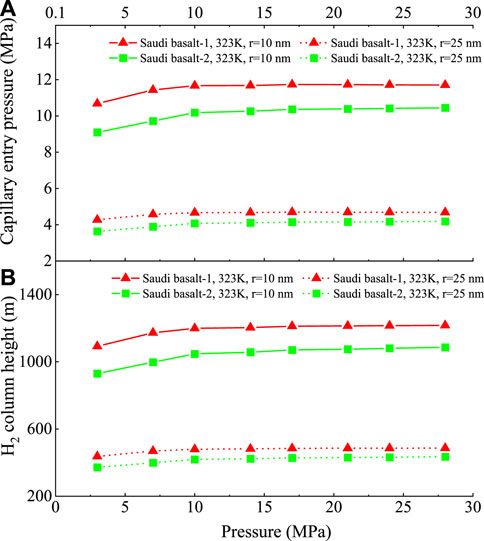
FIGURE 13. The calculated sealing efficiency of H2/basalt/brine systems versus pressure for Saudi basalts represented by—(A) The capillary entry pressure (
Notably, the
The obtained gas column height versus pressure trend of the Saudi basalts varies from that reported in the literature for the H2/Iranian basalts (Hosseini et al., 2022a) and CO2/basalts (Iglauer et al., 2020; Al-Yaseri et al., 2021b) (Figures 14A, B). The CO2/brine/basalt behaviour could be attributed to the sharp increase in CO2 density with pressure; therefore, a rapidly increasing pressure is expected. On the other hand, we hypothesize that the wide difference in H2 column height versus pressure between Saudi and Iranian basalts is most likely attributed to their different mineralogy and the presence of some silicate-rich phases (e.g., lizardite) in the Iranian basalt and, therefore, it behaves like clay-rich rocks and become fully-hydrogen wet as pressure increases.
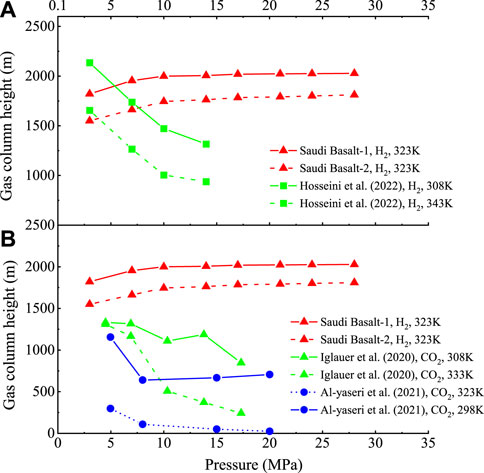
FIGURE 14. The calculated maximum H2 column heights of the Saudi basalts compared to those of—(A) H2/Iranian basalts by Hosseini et al. (2022a) and (B) CO2/basalts by Iglauer et al. (2020) and Al-Yaseri et al. (2021b).
4 Conclusion
• The present study examines the brine wettability of the Saudi basaltic rocks surrounded by H2 to explore their potential for subsurface H2 geological storage using the modified form of sessile drop contact angle measurement.
• The Saudi basalt samples are olivine and pyroxene rich with low content of alternation products (e.g., clays).
• The wettability measurements showed that the samples are water-wet. However, the impact of slight variations in mineralogy and surface roughness was paramount in the contact angle measurements.
• The H2/brine/Saudi basalt system showed trends vary significantly from those reported in the literature for CO2/basalt and H2/basalt systems which are attributed to variations in the gas phases as well as the basalt mineralogy.
• The present results demonstrate that pore throat radius has the paramount control on the H2 column height, and the Saudi basalt with average pore throat radii of 10 nm can store more than 1,200 m of H2 column. Moreover, the H2 column did not vary significantly with pressure, which is beneficial for optimal deep geological H2 storage.
Data availability statement
The original contributions presented in the study are included in the article/Supplementary Material, further inquiries can be directed to the corresponding author.
Author contributions
AA: conceptualization, methodology, investigation, data curation, software, and writing—original draft. AA-Y: conceptualization, investigation, formal analysis, writing—review and editing. MM: methodology, validation, resources, and software. ML: conceptualization, validation, formal analysis, writing—review and editing. HH: conceptualization, validation, resources, writing—review and editing, supervision. All authors contributed to the article and approved the submitted version.
Acknowledgments
The authors would like to acknowledge King Abdullah University for Science and Technology (KAUST) for supporting this work by the Research Funding Office under Award No. 4357 and King Fahd University for Petroleum and Minerals (KFUPM) for providing the required infrastructure for this work. The authors would like to thank Jafar Al-Hamad for the fruitful discussion which improved data interpretation and presentation.
Conflict of interest
The authors declare that the research was conducted in the absence of any commercial or financial relationships that could be construed as a potential conflict of interest.
Publisher’s note
All claims expressed in this article are solely those of the authors and do not necessarily represent those of their affiliated organizations, or those of the publisher, the editors and the reviewers. Any product that may be evaluated in this article, or claim that may be made by its manufacturer, is not guaranteed or endorsed by the publisher.
References
Abdulelah, H., Al-Yaseri, A., Ali, M., Giwelli, A., Negash, B. M., and Sarmadivaleh, M. (2021). CO2/Basalt’s interfacial tension and wettability directly from gas density: implications for carbon geo-sequestration. J. Pet. Sci. Eng. 204, 108683. doi:10.1016/j.petrol.2021.108683
Abramov, A., Keshavarz, A., and Iglauer, S. (2019). Wettability of fully hydroxylated and alkylated (001) alpha-quartz surface in carbon dioxide atmosphere. J. Phys. Chem. C 123, 9027–9040. doi:10.1021/acs.jpcc.9b00263
Aksu, J., Bazilevskaya, E., and Karpyn, Z. (2015). Swelling of clay minerals in unconsolidated porous media and its impact on permeability. GeoResJ 7, 1–13. doi:10.1016/j.grj.2015.02.003
Alanazi, A., Ali, M., Bawazeer, S., Yekeen, N., and Hoteit, H. (2022a). Evaluation of cubic, PC-SAFT, and GERG2008 equations of state for accurate calculations of thermophysical properties of hydrogen-blend mixtures. Energy Rep. 8, 13876–13899. doi:10.1016/J.EGYR.2022.10.257
Alanazi, A., Ali, M., Mowafi, M., and Hoteit, H. (2022b). “Effect of organics and nanofluids on capillary-sealing efficiency of caprock for hydrogen and carbon-dioxide geological storage,” in International Geomechanics Symposium. doi:10.56952/IGS-2022-009
Alanazi, A., Bawazeer, S., Ali, M., Keshavarz, A., and Hoteit, H. (2022c). Thermodynamic modeling of hydrogen-water systems with gas impurity at various conditions using cubic and PC-SAFT equations of state. Energy Convers. Manag. X 100257, 100257. doi:10.1016/J.ECMX.2022.100257
Alanazi, A., Baban, A., Ali, M., Keshavarz, A., Iglauer, S., and Hoteit, H. (2023a). Residual trapping of CO2, N2, and a CO2-N2 mixture in Indiana limestone using robust NMR coreflooding: implications for CO2 geological storage. Fuel 353, 129221. doi:10.1016/J.FUEL.2023.129221
Alanazi, A., Rasool Abid, H., Usman, M., Ali, M., Keshavarz, A., Vahrenkamp, V., et al. (2023b). Hydrogen, carbon dioxide, and methane adsorption potential on Jordanian organic-rich source rocks: implications for underground H2 storage and retrieval. Fuel 346, 128362. doi:10.1016/J.FUEL.2023.128362
Alanazi, A., Yekeen, N., Ali, M., Ali, M., Abu-Mahfouz, I. S., Keshavarz, A., et al. (2023c). Influence of organics and gas mixing on hydrogen/brine and methane/brine wettability using Jordanian oil shale rocks: implications for hydrogen geological storage. J. Energy Storage 62, 106865. doi:10.1016/J.EST.2023.106865
Ali, M., Jha, N. K., Pal, N., Keshavarz, A., Hoteit, H., and Sarmadivaleh, M. (2022a). Recent advances in carbon dioxide geological storage, experimental procedures, influencing parameters, and future outlook. Earth-Science Rev. 225, 103895. doi:10.1016/J.EARSCIREV.2021.103895
Ali, M., Pan, B., Yekeen, N., Al-Anssari, S., Al-Anazi, A., Keshavarz, A., et al. (2022b). Assessment of wettability and rock-fluid interfacial tension of caprock: implications for hydrogen and carbon dioxide geo-storage. Int. J. Hydrogen Energy. 47, 14104–14120. doi:10.1016/J.IJHYDENE.2022.02.149
Al-Mukainah, H., Al-Yaseri, A., Yekeen, N., Hamad, J. A., and Mahmoud, M. (2022). Wettability of shale–brine–H2 system and H2-brine interfacial tension for assessment of the sealing capacities of shale formations during underground hydrogen storage. Energy Rep. 8, 8830–8843. doi:10.1016/J.EGYR.2022.07.004
AlRatrout, A., Blunt, M. J., and Bijeljic, B. (2018). Wettability in complex porous materials, the mixed-wet state, and its relationship to surface roughness. Proc. Natl. Acad. Sci. U. S. A. 115, 8901–8906. doi:10.1073/pnas.1803734115
Altherr, R., Mertz-Kraus, R., Volker, F., Kreuzer, H., Henjes-Kunst, F., and Lange, U. (2019). Geodynamic setting of upper Miocene to quaternary alkaline basalts from Harrat al ‘Uwayrid (NW Saudi Arabia): constraints from K–Ar dating, chemical and Sr-Nd-Pb isotope compositions, and petrological modeling. Lithos 330–331, 120–138. doi:10.1016/J.LITHOS.2019.02.007
Al-Yaseri, A., and Jha, N. K. (2021). On hydrogen wettability of basaltic rock. J. Pet. Sci. Eng. 200, 108387. doi:10.1016/j.petrol.2021.108387
Al-Yaseri, A. Z., Lebedev, M., Barifcani, A., and Iglauer, S. (2016). Receding and advancing (CO2 + brine + quartz) contact angles as a function of pressure, temperature, surface roughness, salt type and salinity. J. Chem. Thermodyn. 93, 416–423. doi:10.1016/J.JCT.2015.07.031
Al-Yaseri, A., Ali, M., Abbasi, G. R., Abid, H. R., and Jha, N. K. (2021a). Enhancing CO2 storage capacity and containment security of basaltic formation using silica nanofluids. Int. J. Greenh. Gas. Control 112, 103516. doi:10.1016/J.IJGGC.2021.103516
Al-Yaseri, A., Ali, M., Ali, M., Taheri, R., and Wolff-Boenisch, D. (2021b). Western Australia basalt-CO2-brine wettability at geo-storage conditions. J. Colloid Interface Sci. 603, 165–171. doi:10.1016/J.JCIS.2021.06.078
Al-Yaseri, A., Wolff-Boenisch, D., Fauziah, C. A., and Iglauer, S. (2021c). Hydrogen wettability of clays: implications for underground hydrogen storage. Int. J. Hydrogen Energy 46, 34356–34361. doi:10.1016/j.ijhydene.2021.07.226
Blunt, M. J. (2016). Multiphase flow in permeable media, multiphase flow in permeable media. doi:10.1017/9781316145098
Bui, M., Adjiman, C. S., Bardow, A., Anthony, E. J., Boston, A., Brown, S., et al. (2018). Carbon capture and storage (CCS): the way forward. Energy Environ. Sci. 11, 1062–1176. doi:10.1039/C7EE02342A
Cheng, W. M., Xue, J., Zhou, G., Nie, W., and Liu, L. S. (2014). Study of coal dust wettability based on FTIR. Meitan Xuebao/Journal China Coal Soc. 39. doi:10.13225/j.cnki.jccs.2013.1715
Chow, Y. T. F., Maitland, G. C., and Trusler, J. P. M. (2018). Interfacial tensions of (H2O + H2) and (H2O + CO2 + H2) systems at temperatures of (298–448) K and pressures up to 45 MPa. Fluid Phase Equilib. 475, 37–44. doi:10.1016/J.FLUID.2018.07.022
Coleman, R. G., Gregory, R. T., and Brown, G. F. (1983). Cenozoic volcanic rocks of Saudi Arabia. U.S. Geological Survey. United States Department of the Interior, Geological Survey (Open-File Report 83-788). doi:10.3133/ofr83788
Dessert, C., Dupré, B., Gaillardet, J., François, L. M., and Allègre, C. J. (2003). Basalt weathering laws and the impact of basalt weathering on the global carbon cycle. Chem. Geol. 202, 257–273. doi:10.1016/j.chemgeo.2002.10.001
Duncan, R. A., and Al-Amri, A. M. (2013). Timing and composition of volcanic activity at Harrat Lunayyir, western Saudi Arabia. J. Volcanol. Geotherm. Res. 260, 103–116. doi:10.1016/J.JVOLGEORES.2013.05.006
Espinoza, N., and Santamarina, J. C. (2017). CO2 breakthrough—caprock sealing efficiency and integrity for carbon geological storage. Int. J. Greenh. Gas. Control 66, 218–229. doi:10.1016/J.IJGGC.2017.09.019
Gadelmawla, E. S., Koura, M. M., Maksoud, T. M. A., Elewa, I. M., and Soliman, H. H. (2002). Roughness parameters. J. Mater. Process. Technol. 123, 133–145. doi:10.1016/S0924-0136(02)00060-2
Gislason, S. R., and Oelkers, E. H. (2014). Carbon storage in basalt. Science (80-. ) 344, 373–374. doi:10.1126/science.1250828
Gislason, S. R., Wolff-Boenisch, D., Stefansson, A., Oelkers, E. H., Gunnlaugsson, E., Sigurdardottir, H., et al. (2010). Mineral sequestration of carbon dioxide in basalt: A pre-injection overview of the CarbFix project. Int. J. Greenh. Gas. Control 4, 537–545. doi:10.1016/j.ijggc.2009.11.013
Harnois, L. (1988). The CIW index: A new chemical index of weathering. Sediment. Geol. 55, 319–322. doi:10.1016/0037-0738(88)90137-6
Hashemi, L., Glerum, W., Farajzadeh, R., and Hajibeygi, H. (2021). Contact angle measurement for hydrogen/brine/sandstone system using captive-bubble method relevant for underground hydrogen storage. Adv. Water Resour. 154, 103964. doi:10.1016/J.ADVWATRES.2021.103964
Heinemann, N., Alcalde, J., Miocic, J. M., Hangx, S. J. T., Kallmeyer, J., Ostertag-Henning, C., et al. (2021). Enabling large-scale hydrogen storage in porous media – The scientific challenges. Energy Environ. Sci. 14, 853–864. doi:10.1039/D0EE03536J
Hosseini, M., Ali, M., Fahimpour, J., Keshavarz, A., and Iglauer, S. (2022a). Basalt-H2-brine wettability at geo-storage conditions: implication for hydrogen storage in basaltic formations. J. Energy Storage 52, 104745. doi:10.1016/J.EST.2022.104745
Hosseini, M., Ali, M., Fahimpour, J., Keshavarz, A., and Iglauer, S. (2022b). Assessment of rock-hydrogen and rock-water interfacial tension in shale, evaporite and basaltic rocks. J. Nat. Gas. Sci. Eng. 106, 104743. doi:10.1016/J.JNGSE.2022.104743
Hosseini, M., Fahimpour, J., Ali, M., Keshavarz, A., and Iglauer, S. (2022c). Capillary sealing efficiency analysis of caprocks: implication for hydrogen geological storage. Energy and Fuels acs.energyfuels.2c00281 36, 4065–4075. doi:10.1021/ACS.ENERGYFUELS.2C00281
Iglauer, S., Al-Yaseri, A. Z., and Wolff-Boenisch, D. (2020). Basalt-CO2-brine wettability at storage conditions in basaltic formations. Int. J. Greenh. Gas. Control 102, 103148. doi:10.1016/J.IJGGC.2020.103148
Iglauer, S. (2022). Optimum geological storage depths for structural H2 geo-storage. J. Pet. Sci. Eng. 212, 109498. doi:10.1016/j.petrol.2021.109498
Jiu, B., Huang, W., Li, Y., and He, M. (2021). Influence of clay minerals and cementation on pore throat of tight sandstone gas reservoir in the eastern Ordos Basin, China. J. Nat. Gas Sci. Eng. 87, 103762.
Kaliwoda, M., Altherr, R., and Meyer, H. P. (2007). Composition and thermal evolution of the lithospheric mantle beneath the Harrat Uwayrid, eastern flank of the Red Sea rift (Saudi Arabia). Lithos 99, 105–120. doi:10.1016/j.lithos.2007.06.013
Kanaani, M., Sedaee, B., and Asadian-Pakfar, M. (2022). Role of cushion gas on underground hydrogen storage in depleted oil reservoirs. J. Energy Storage 45, 103783. doi:10.1016/j.est.2021.103783
Kwok, D. Y., and Neumann, A. W. (1999). Contact angle measurement and contact angle interpretation. Adv. Colloid Interface Sci. 81, 167–249. doi:10.1016/S0001-8686(98)00087-6
Lander, L. M., Siewierski, L. M., Britain, W. J., and Vogler, E. A. (1993). A systematic comparison of contact angle methods. Langmuir 9, 2237–2239. doi:10.1021/la00032a055
Leila, M., Moscariello, A., and Šegvić, B. (2018). Geochemical constraints on the provenance and depositional environment of the messinian sediments, onshore nile delta, Egypt: implications for the late Miocene paleogeography of the mediterranean. J. Afr. Earth Sci. 143, 215–241. doi:10.1016/j.jafrearsci.2018.03.024
Leila, M., Levy, D., Battani, A., Piccardi, L., Segvic, B., Badurina, L., et al. (2021). Origin of continuous hydrogen flux in gas manifestations at the Larderello geothermal field, Central Italy. Chem. Geol. 585, 120564. doi:10.1016/j.chemgeo.2021.120564
Leila, M., Loisseau, K., and Moretti, I. (2022). Controls on generation and accumulation of blended gases (CH4/H2/He) in the Neoproterozoic Amadeus Basin, Australia. Mar. Pet. Geol. 140, 105643. doi:10.1016/j.marpetgeo.2022.105643
Lemmon, E. W., Bell, I. H., Huber, M. L., and McLinden, M. O. (2018). NIST Standard Reference Database 23: Reference Fluid Thermodynamic and Transport Properties—REFPROP, Version 10.0. Gaithersburg, MD: National Institute of Standards and Technology, Standard Reference Data Program.
Li, D., and Neumann, A. W. (1992). Equation of state for interfacial tensions of solid-liquid systems. Adv. Colloid Interface Sci. 39, 299–345. doi:10.1016/0001-8686(92)80064-5
Li, M., Bussonnière, A., Xiang, B., Manica, R., and Liu, Q. (2022). Effect of solid wettability on three-phase hydrodynamic cavitation. Min. Eng. 180, 107455. doi:10.1016/j.mineng.2022.107455
Liang, X., Zhou, F., Liang, T., Su, H., Yuan, S., and Li, Y. (2020). Impacts of pore structure and wettability on distribution of residual fossil hydrogen energy after imbibition. Int. J. Hydrogen Energy 45, 14779–14789. doi:10.1016/J.IJHYDENE.2020.03.208
Liu, H., Luo, B., Shen, S., and Li, L. (2019). Design and mechanical tests of basalt fiber cloth with MAH grafted reinforced bamboo and poplar veneer composite. Eur. J. Wood Wood Prod. 77, 271–278. doi:10.1007/s00107-018-1378-9
Ma, J., Li, Q., Kempka, T., and Kühn, M. (2019). Hydromechanical response and impact of gas mixing behavior in subsurface CH4 Storage with CO2-based cushion gas. Energy Fuels 33, 6527–6541. doi:10.1021/acs.energyfuels.9b00518
Madhurima, V., Purkayastha, D. D., and Rao, N. V. S. (2011). Wettability, FTIR and dielectric studies of 1,4-dioxane and water system. J. Colloid Interface Sci. 357, 229–233. doi:10.1016/j.jcis.2011.01.090
Matter, J. M., Stute, M., Snæbjörnsdottir, S., Oelkers, E. H., Gislason, S. R., Aradottir, E. S., et al. (2016). Rapid carbon mineralization for permanent disposal of anthropogenic carbon dioxide emissions. Sci. (80-. ) 352, 1312–1314. doi:10.1126/science.aad8132
Mbonyiryivuze, A., Mwakikunga, B., Dhlamini, S. M., Maaza, M., Mokhotjwa Dhlamini, S., Park, E., et al. (2015). Morphological and chemical composition characterization of commercial sepia melanin. Phys. Mater. Chem. 3, 22–27. doi:10.12691/ajn-3-1-3
McCollom, T., and Bach, W. (2009). Thermodynamic constraints on hydrogen generation during serpentinization of ultramafic rocks. Geochim. Cosmochim. Acta 73, 856–875. doi:10.1016/j.gca.2008.10.032
Mehmani, A., Kelly, S., Torres-Verdín, C., and Balhoff, M. (2019). Capillary trapping following imbibition in porous media: microfluidic quantification of the impact of pore-scale surface roughness. Water Resour. Res. 55, 9905–9925. doi:10.1029/2019WR025170
Michalski, J., Bünger, U., Crotogino, F., Donadei, S., Schneider, G., Pregger, T., et al. (2017). Hydrogen generation by electrolysis and storage in salt caverns: potentials, economics and systems aspects with regard to the German energy transition.
Morrow, N. R. (1975). Effects of surface roughness on contact angle with special reference to petroleum recovery. J. Can. Pet. Technol. 14, 42–53. doi:10.2118/75-04-04/2166536/PETSOC-75-04-04.PDF/1
Oh, T., and Choi, C. K. (2010). Comparison between SiOC thin film by plasma enhance chemical vapor deposition and SiO2 thin film by fourier Transform infrared spectroscopy. J. Korean Phys. Soc. 56, 1150–1155. doi:10.3938/jkps.56.1150
Pan, B., Yin, X., Ju, Y., and Iglauer, S. (2021). Underground hydrogen storage: influencing parameters and future outlook. Adv. Colloid Interface Sci. 294, 102473. doi:10.1016/j.cis.2021.102473
Pfeiffer, W. T., Beyer, C., and Bauer, S. (2017). Hydrogen storage in a heterogeneous sandstone formation: dimensioning and induced hydraulic effects. Pet. Geosci. 23, 315–326. doi:10.1144/petgeo2016-050
Pillai, P., Kumar, A., and Mandal, A. (2018). Mechanistic studies of enhanced oil recovery by imidazolium-based ionic liquids as novel surfactants. J. Ind. Eng. Chem. 63, 262–274. doi:10.1016/j.jiec.2018.02.024
Prinzhofer, A., Cissé, C., and Diallo, A. (2018). Discovery of a large accumulation of natural hydrogen in Bourakebougou (Mali). Int. J. Hydrog. Energy 43, 19315–19326. doi:10.1016/j.ijhydene.2018.08.193
Rucker, M., Naderi, M., Yesufu-Rufai, S., Lowe, S., Marcelis, F., Rayan, M., et al. (2019). Impact of texture and mineralogy on the wettability of rock surfaces. AGU fall meetings. Abstracts.
Shah, V., Broseta, D., Mouronval, G., and Montel, F. (2008). Water/acid gas interfacial tensions and their impact on acid gas geological storage. Int. J. Greenh. Gas. Control 2, 594–604. doi:10.1016/j.ijggc.2008.02.002
Tadmor, R. (2004). Line energy and the relation between advancing, receding, and Young contact angles. Langmuir 20, 7659–7664. doi:10.1021/la049410h
Tarkowski, R. (2019). Underground hydrogen storage: characteristics and prospects. Renew. Sustain. Energy Rev. 105, 86–94. doi:10.1016/j.rser.2019.01.051
Waman, V. S., Funde, A. M., Kamble, M. M., Pramod, M. R., Hawaldar, R. R., Amalnerkar, D. P., et al. (2011). Hydrogenated nanocrystalline silicon thin films prepared by hot-wire method with varied process pressure. J. Nanotechnol. 2011, 1–10. doi:10.1155/2011/242398
Weltje, G. J., Meijer, X. D., and De Boer, P. L. (1998). Stratigraphic inversion of siliciclastic basin fills: A note on the distinction between supply signals resulting from tectonic and climatic forcing. Basin Res. 10, 129–153. doi:10.1046/j.1365-2117.1998.00057.x
White, S. K., Spane, F. A., Schaef, H. T., Miller, Q. R. S., White, M. D., Horner, J. A., et al. (2020). Quantification of CO2Mineralization at the wallula basalt pilot project. Environ. Sci. Technol. 54, 14609–14616. doi:10.1021/ACS.EST.0C05142
Yekeen, N., Padmanabhan, E., Abdulelah, H., Irfan, S. A., Okunade, O. A., Khan, J. A., et al. (2021). CO2/brine interfacial tension and rock wettability at reservoir conditions: A critical review of previous studies and case study of black shale from Malaysian formation. J. Pet. Sci. Eng. 196, 107673. doi:10.1016/j.petrol.2020.107673
Yekta, A. E., Manceau, J.-C. C., Gaboreau, S., Pichavant, M., and Audigane, P. (2018). Determination of hydrogen–water relative permeability and capillary pressure in sandstone: application to underground hydrogen injection in sedimentary formations. Transp. Porous Media 122, 333–356. doi:10.1007/S11242-018-1004-7
Keywords: hydrogen, Saudi basalt, Harrat Uwayrid, wettability, seal integrity, subsurface storage
Citation: Alanazi A, Al-Yaseri A, Mowafi M, Leila M and Hoteit H (2023) First assessment of hydrogen/brine/Saudi basalt wettability: implications for hydrogen geological storage. Front. Earth Sci. 11:1225131. doi: 10.3389/feart.2023.1225131
Received: 18 May 2023; Accepted: 04 September 2023;
Published: 14 September 2023.
Edited by:
Ali Abedini, Urmia University, IranReviewed by:
Linda Stalker, Commonwealth Scientific and Industrial Research Organisation (CSIRO), AustraliaAngela Goodman, National Energy Technology Laboratory (DOE), United States
Copyright © 2023 Alanazi, Al-Yaseri, Mowafi, Leila and Hoteit. This is an open-access article distributed under the terms of the Creative Commons Attribution License (CC BY). The use, distribution or reproduction in other forums is permitted, provided the original author(s) and the copyright owner(s) are credited and that the original publication in this journal is cited, in accordance with accepted academic practice. No use, distribution or reproduction is permitted which does not comply with these terms.
*Correspondence: Mahmoud Leila, bWFobW91ZF9sb3RmeUBtYW5zLmVkdS5lZw==